Current insights into transcriptional role(s) for the nutraceutical Withania somnifera in inflammation and aging
- 1PhytoVeda Pvt. Ltd., Mumbai, India
- 2Viridis Biopharma Pvt. Ltd., Mumbai, India
The health-beneficial effects of nutraceuticals in various diseases have received enhanced attention in recent years. Aging is a continuous process wherein physiological activity of an individual declines over time and is characterized by various indefinite hallmarks which contribute toward aging-related comorbidities in an individual which include many neurodegenerative diseases, cardiac problems, diabetes, bone-degeneration, and cancer. Cellular senescence is a homeostatic biological process that has an important function in driving aging. Currently, a growing body of evidence substantiates the connection between epigenetic modifications and the aging process, along with aging-related diseases. These modifications are now being recognized as promising targets for emerging therapeutic interventions. Considering that almost all the biological processes are modulated by RNAs, numerous RNA-binding proteins have been found to be linked to aging and age-related complexities. Currently, studies have shed light on the ability of the nutraceutical Withania somnifera (Ashwagandha) to influence RNA expression, stability, and processing, offering insights into its mechanisms of action. By targeting RNA-related pathways, Withania somnifera may exhibit promising effects in ameliorating age-associated molecular changes, which include modifications in gene expression and signaling networks. This review summarizes the potential role of Withania somnifera as a nutraceutical in modulating RNA-level changes associated with aging, encompassing both in vitro and in vivo studies. Taken together, the putative role(s) of Withania in modulation of key RNAs will provide insights into understanding the aging process and facilitate the development of various preventive and therapeutic strategies employing nutraceuticals for healthy aging.
1 Introduction
1.1 Aging
Aging is considered as a progressive deterioration of physiological activity and is characterized by various indefinite hallmarks at both cellular and molecular levels (1). Apart from genomic instability and telomere attrition, ongoing research in this arena reveals various other contributing factors making this process multifaceted and complicated. Senescence occurring at both cellular and organ level leads to age-related diseases like cardiovascular conditions, Alzheimer’s disease, cancer, and sarcopenia, often presenting as comorbidities (2). With the world’s population aged 60 and above projected to double and reach 22% by the year 2050, there is a noticeable increase in both illness and mortality rates among the population (3). Escalating global aging poses a healthcare challenge due to the significant health risks accompanying aging. Therefore, it is necessary to understand the specific mechanisms which drive aging as well as aging-related complications (4, 5). Aging and aging-related complexities are consequences of external factors (environmental) and internal factors (modulation of gene expression) (6). Genetic alteration is considered as crucial in all organic processes starting with maturation of embryo to biological aging (7). Transcriptomic studies including alterations in all RNA species (including mRNA, rRNA, tRNA and non-coding RNAs) inferred that genetic alterations may often lead to aging-related comorbidities namely diabetes, neurological disorders, obesity, cancer and viral infections (6). Although certain illnesses or ailments might exhibit recognizable genetic or molecular markers such as transcriptomic modifications contributing to their simultaneous occurrence, numerous comorbidities stem from complex interplays among genetic, environmental, and lifestyle elements, sometimes without apparent transcriptomic changes (8).
1.2 Withania somnifera
Withania somnifera (WS) (Figure 1) is a perennial, woody shrub that typically reaches a height ranging from 0.5 to 2.0 meters, belonging to family Solanaceae and is recognized by names like “Winter cherry” or “Indian Ginseng” (in English); “Ashwagandha” (in Sanskrit) and “Asgandh” (in Hindi) (10). WS is established in the regions of Middle East, Africa, Sri Lanka, China, India, Canary Islands and in the warm areas of Europe and Australia where the climate is hot and dry (11).
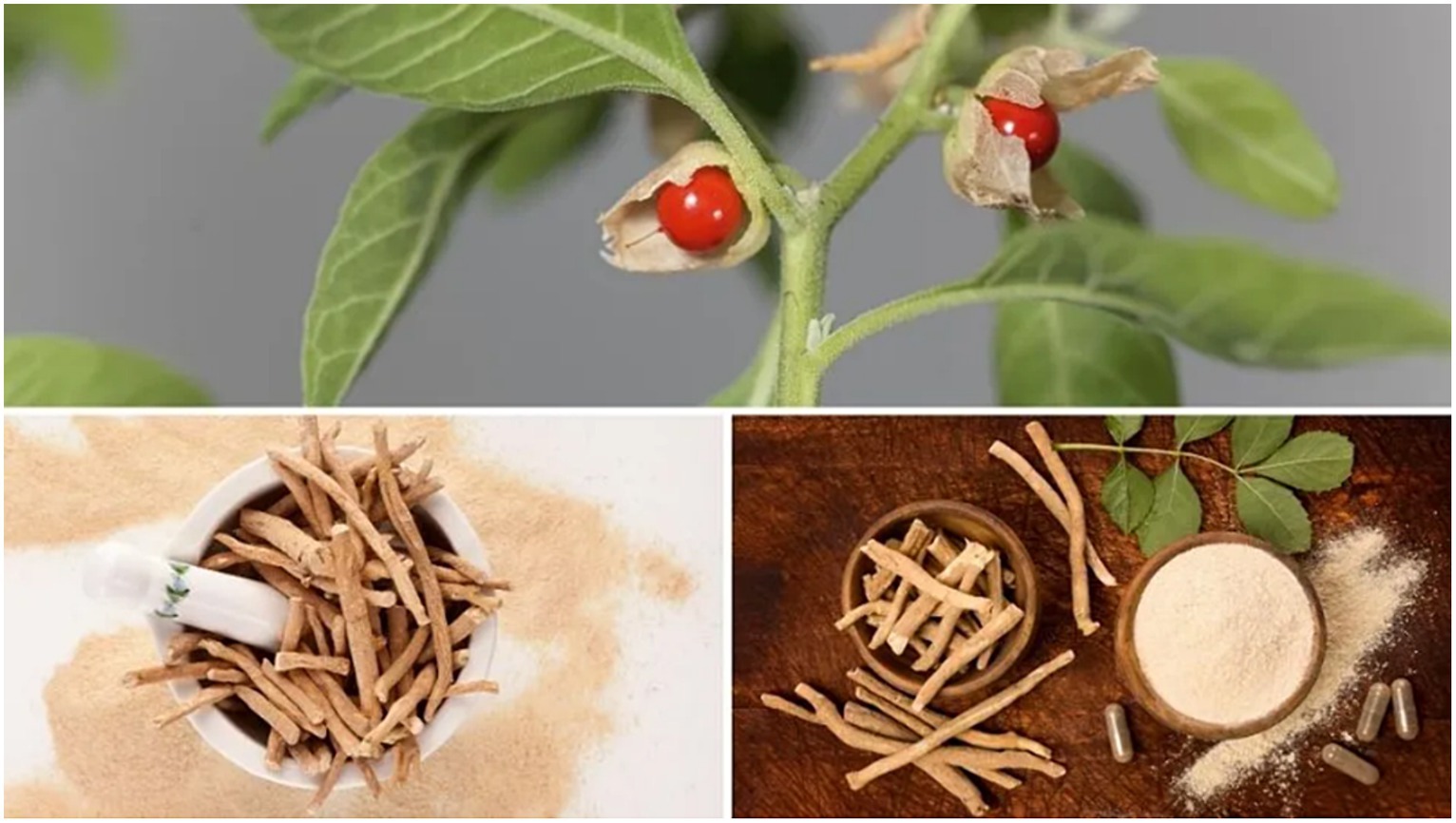
Figure 1. Withania somnifera (9).
The active biochemical components found in WS comprise of alkaloids such as isopelletierine, anaferine, cuseohygrine, anahygrine, etc., and steroidal lactones which include withanolides, withanosides (glucose conjugated), withaferin and saponins (12). The chemical structures of major withanolides which include Withaferin A, Withanolide and Withanone are illustrated in Figures 2A–C respectively. Structures of the remaining constituents has been published elsewhere (11). The anti-stress agents in WS comprise sitoindosides and acylsterylglucosides and withaferin A (WA). It was reported that using WA and sitoindosides can alleviate stress in experimental models (13).
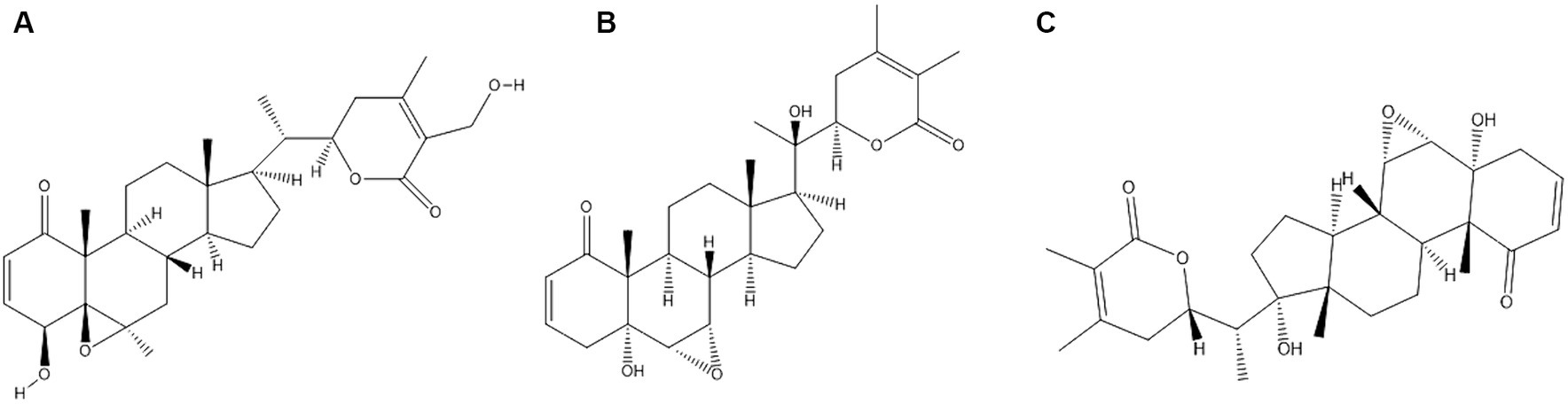
Figure 2. Chemical structures of major constituents of Withania somnifera (A) Withaferin A; (B) Withanlide; (C) Withanone. Structures of the constituents were drawn using Chemdraw Version 20.1.1.125.
1.3 Mechanism for pharmacologic activity of Withania
1.3.1 Immune regulation by Withania
The immune system is an important factor in the origin and mechanisms of different diseases, contributing significantly to biomedical research advancement (14). Conditions affecting the skin, gastrointestinal tract, respiratory system, joints, vital organs, and infectious illnesses are increasingly recognized as primarily influenced by immunological factors (15). A wide range of studies have shown that most of the major components of WS have immunomodulatory effects in mice (16) and humans (17). The immunomodulatory role of WS in various inflammatory complications such as inflammation (reducing type 2 cytokines, and inflammatory markers such as TNF-α and IgE) has been reported (18). The extract derived from WS root showcases robust hemopoietic, antioxidant, adaptogenic, and immune-stimulating properties (19). WS plays a role in immunomodulation by significantly improving the immune profile by altering innate and adaptive immune systems (20). In a randomized and controlled clinical trial, the immune-stimulatory effect of WS was evaluated and it was reported that WS enhanced CD4+/CD8+ and expression of CD3+/CD19+/CD45+, thus, increasing the antibody and antibody-forming cells (20). The extract from WS has demonstrated a notable ability to boost cell-mediated immunity in mice (21). Levels of interferon gamma (IFN-y), interleukin-2 (IL-2), and granulocyte macrophage colony-stimulating factor (GM-CSF) have been elevated in experimental mouse models when treated with WS; this indicates potential immune-enhancing and myelo-protective effects of root extracts of WS (22). Furthermore, it enhances the body’s immune system, specifically enhancing cell-mediated immunity, which is vital in defending against diseases (23).
1.3.2 Modulation of nitric oxide (NO) signaling by Withania
WS amplifies the nitric oxide synthetase (NOS) activity in macrophages, consequently augmenting the microbial eradication capability of these immune cells. It has been elucidated that WS enhances the activity of nitric oxide via NOS induction (22). Indeed, WS boasts a wide array of beneficial effects on the body. Additionally, it promotes a balance in reproductive and sexual function contributing to an improved reproductive health (23). WS’s adaptogenic properties make the body more resilient to the harmful effects of stress (24). Furthermore, viscosalactone B and 27-O-glucoside (a derivative of WA) extracted from alcoholic leaf extract have demonstrated significant antiproliferative activity when treated with different cell lines viz. CNS cancer SF-268, breast cancer MCF-7, colon cancer HCT-116 and lung cancer NCI-H460 cell line (11).
1.3.3 Antioxidant activities of Withania
Studies have shown antioxidant activities of WS concluding that it helps in prevention of glycation-induced pathogenesis as well as decreasing the levels of many oxidative stress markers indicating its potential therapeutic effects in managing healthy aging (25–27). WS’s potent antioxidant properties have an important part in protecting cells against free radicals (23). Hydroalcoholic root extracts of WS contains a diverse range of molecules namely saponins, particularly sitoinsides 7 and 8, withanolides and alkaloids which have anti-stress, anti-inflammatory and anti-oxidant properties, respectively, (28). Anti-inflammatory potential of withanolides and alkaloids is reported in in vitro study using RAW 264.7 cell line. Anti-stress properties of sitoindosides 7 and 8 were demonstrated using Wistar albino rats and albino mice (28). Also, studies conducted to investigate the effect of alkaloids in RAW 264.7 cells showed that the expression of iNOS and COX-2 proteins were upregulated (29). In addition, alkaloids inhibited LPS-induced inflammation, indicating a putative anti-inflammatory potential of alkaloids (29). Alkaloids from WS (withanamides A-I) are also reported to lower the rate of lipid peroxidation along with possessing anti-oxidant properties (30).
WS is known for its versatility in treating a range of conditions, such as immunomodulation, rejuvenation, enhancement of cognitive function, inflammation, enhancing concentration, etc. However, a synthetic review exploring its potential role in ameliorating aging and aging-related disorders is currently lacking. In this review, we summarize mechanistic pathways modulated by WS via coding and non-coding RNAs in aging relying on information gleaned from both in vitro and in vivo studies. The RNA biomarkers regulated by WS can potentially serve as promising targets to mitigate aging-related degenerative changes and, perhaps, to some extent, reverse biological aging. This may facilitate the development of various preventive and therapeutic strategies employing WS as a nutraceutical for healthy aging.
2 Aging
Aging can be described as a gradual decline over time in physiological functions essential for survival and reproductive capabilities (31). The attributes associated with aging, distinct from age-related illness like cancer and heart diseases, impact every species (31). In mammals, the occurrence of aging is heterogenous wherein it is accompanied by organs and tissues deterioration and their functional capabilities gradually decrease over time but not necessarily degrading into pathophysiology in healthy aging (32). Consequently, aging is considered as an important factor in pathogenesis of many diseases such as dementia, osteoarthritis, cardiovascular disease, cancer, type 2 diabetes, idiopathic pulmonary fibrosis and glaucoma (33). To classify aging through a biological measurement, a biomarker needs to possess specificity, systemic relevance, and practical utility (34). Biomarkers of cellular aging have been categorized into ten different aspects viz. telomere shortening, genetic instability, nuclear body disorders, cell cycle arrest, epigenetic changes, mitochondrial impairment, signaling pathway rerouting, epigenetic changes, loss of proteostasis, metabolic alterations and senescence-associated secondary phenotype (35, 36).
2.1 Hallmarks of aging
Exploring biological aging and understanding the factors related to decelerating organism function and the development of diseases is essential (37). Scientists have made significant progress in unravelling the solutions through elucidation of nine different processes that contribute to the gradual decline and loss of bodily functions. These functions are also known as hallmarks of aging (38). The hallmarks are categorized into 3 groups (38). The first category includes primary hallmarks (causes of age-associated damage); second, antagonistic hallmarks (response to damage) and third, integrative hallmarks (response consequences and culprits of aging). Primary hallmarks include genomic uncertainty, attenuation in telomere strength, epigenetic alterations and proteostasis deprivation. Antagonistic hallmarks include deregulated nutrient sensing, affliction in mitochondrial function and senescence. Integrative hallmarks include stem cell exhaustion and alter intercellular communication (33). All the above-mentioned hallmarks of aging are illustrated in Figure 3.
2.1.1 Primary hallmarks
The primary causes of aging are related to various degradations, alterations and instabilities to our genomic framework and further cascading processes (39). Genomic instability and telomere shortening are major causes of DNA damage, while epigenetic alterations cause post-translational modification of histones and chromatin remodeling (40). Proteostasis irregulation can also have major impacts on aging due to refolding of proteins (41). Telomeres, protein structures which protect the terminal regions of the DNA, go through an attrition process as a part of cell cycle, while oxidative stress, inflammation and chronic stress are also known to increase the rate of attrition leading to degradation in the protective regions making DNA more susceptible to damage (42, 43). To add to it, genomic instability caused by both internal and external factors can alter the genetic code; this kind of DNA damage hinders the process of protein production used to damage repair and accelerates the process of aging (40, 44). Structural changes due to epigenetic alternations degrade the cellular function and are associated with a range of age-related diseases such as cancer, diabetes, osteoporosis, neurological diseases, and increased inflammation (45). Proteostasis, also known as protein homeostasis, helps maintain protein integrity to ensure proper functioning (46). Dysregulation due to various internal and external factors leads to refolding which can have serious health implications such as neurodegenerative disorders, impaired muscle function and low cardiac health (41).
2.1.2 Antagonistic hallmarks
Response of the cells to nutrients is considered as nutrient sensing (47). Mammalian target of Rapamycin (mTOR) is a crucial unit which incorporates nutrient sensing with different cellular processes that can lead to growth and proliferation of cells (48). In growing organisms, cell proliferation and cell growth are very crucial and, therefore mTOR is activated (49). mTOR is naturally decreased in aging (50). Over activating this pathway can cause increased aging and increase in cancer rate, heart conditions, metabolic disorders, bone loss, neurodegenerative conditions and sarcopenia (50). The mitochondrion serves as the cell’s energy hub, where most of the body’s energy is generated (51). Aging tends to decrease the efficiency of the mitochondria due to reduced biogenesis (51). Accumulation of reactive oxygen species and deficiencies (DNA polymerase γ) are the major causes of mitochondrial dysfunctions (52). Cellular senescence, or cell aging, is a process wherein the cell cycle is arrested and accompanied by characteristic phenotypic changes (53). The number of senescent cells tends to rise with age, leading to an increase in inflammatory markers that contribute to the aging process (1).
2.1.3 Integrative hallmarks
Adult stem cells are necessary for continuity of tissue homeostasis and regeneration (54). Consequently, the qualitative and quantitative decrease in functions of stem cells during the entirety of life are referred to as its exhaustion (1). Decline in stem cell regeneration is considered as a marker of aging since the proportion of stem cells and their pace of division gradually decreases over time (55). Stem cell exhaustion in adults can lead to cognitive decline, neurological degenerative diseases, delayed healing, lowered immune functions, and unfavorable heart conditions (55). Cells communicate with each other based on different chemical and electrical means such as endocrine, neuroendocrine and neuronal level. Thus, neurohormonal level is deregulated in aging as inflammatory reactions increase (56). One of the most prominent changes in cell signaling biomarkers is “inflammaging” indicating chronic inflammation development in elderly population (57).
2.2 Cellular senescence
Of the nine hallmarks of aging, cellular senescence has been implicated as a major cause of aging and age-related diseases (33). The phenomenon of senescence was initially elucidated in 1961 by Hayflick and Moorhead using human diploid fibroblasts cells who reported that the cells undergo at least 40–60 divisions before arresting their cell cycle (58). The process of senescence is characterized by cell-cycle arrest in the G1 or probably G2 phase, which prevents the proliferation of damaged cells (59). Cellular senescence can also occur at embryonic development and is activated by cellular impairment including the processes of DNA damage response (DDR), telomere attrition or dysfunction, activation of various oncogenes, or loss of ability of tumor suppressor genes, epigenetic changes and organelle damage (60). The major risk for cellular senescence is DNA damage which initiates the canonical p53-p21 pathway and DNA damage response (61). p21 downregulates CDK complex inhibiting the formation of DREAM complex suppressing genes involved in cell cycle through binding with their homology regions (62).
A permanent shunt of cell cycle confirms that cells altered due to genomic changes do not pass on their genomes (63). The process is initiated by the activation of p16/Rb and/or p53/p21 pathways (64). An analysis of several genome wide association studies inferred that INK4a/ARF locus, also known as genomic locus, contributes to a plethora of aging-related pathologies (65). p15INK4b and p16INK4a inhibit CDK4/6 affecting the functionality of cell cycle. In contrast, activation of ARF leads to inhibition of murine double minute 2 (MDM2) which leads to crosstalk between p53/p21CIPI pathways (66). p16INK4a is accumulated during aging and is recognized as a biomarker in aging and aging-related diseases (33).
2.3 Oxidative stress
Oxidative stress occurs when the ROS production and cellular defense mechanisms are imbalanced (67). ROS are essential for maintenance and growth of cells as they play important roles in cellular defense mechanisms (68). Free radical formation is a crucial step for the cell protection and cell survival within its physiological limits (69). This process escalates with age and affects the normal functioning of various tissues in the body (70). Furthermore, various prolonged diseases related to aging such as cardiovascular disease, diabetes, pulmonary, bones and muscle related disorders are linked to oxidative stress (71). Oxidative stress is induced when there is an elevation in ROS production and reduction in ROS neutralization (67). Since the ROS are produced rapidly, their toxic levels are attained and lead to higher Ca2+ stimulation in TCA cycle, this leads to the increased activity of ETC and NADPH production (72). Autooxidation with high production of glucose guides to the alteration of metabolic activity that leads to inhibition of enzymes glutathione peroxidase (GPx) and catalase (CAT) while ROS causes DNA damage (73). In aging, there is deposition of ROS-induced damage which leads to age-associated functional losses (74). Aging and associated disorders which are induced by oxidative stress cause degradation in soft tissues and disruption of homeostasis (75). Moreover, oxidative stress activates irregular mitochondrial signaling leading to alteration of homeostasis of mitochondria and causes age-dependent cellular damage (75).
2.4 Metabolic stress as a marker in aging
Diseases related to abnormal functions in metabolism have been on the rise because of various lifestyle changes that include caloric surplus diet, smoking, etc. (76). This leads to metabolic symptoms such as obesity, diabetes, insulin resistance and hypertension. These diseases are also known as metabolic syndrome (77). Even after many clinical advancements to improve the conditions for cardiovascular disease, patients who are suffering from metabolic syndrome have low life expectancy as well as suffer from premature aging (78). Metabolic disorders are linked to an early onset of cardiovascular aging risk, which encompasses cardiac remodeling, improper functioning of cardiac pump, endothelial damage, and calcification, consequently elevating the susceptibility to heart failure (79). Alterations in metabolic homeostasis is one of the major cause of premature aging (80). Autophagy is referred to as a “process of degradation of injured cell organelles and proteins” and it lowers with aging. Inhibition of this process leads to shortening of life-expectancy rate and resulting in premature aging (81). The process of autophagy tends to be actively involved in pathogenesis of disease such as cardiac arrest, CHD, atherosclerosis (82). Metabolic stress-induced impairment in the autophagic clearance mechanism has a role in shifting the cellular, tissue, or organ health from a normal state to a pre-senescent condition marked by visible pathology (80). Multiple advantages have been postulated in extending lifespan and mitigating age-related issues, both in clinical practice and experimental research (78). Dysregulation of metabolic and physiological stress have shown to affect telomere length and maintenance which leads to aging (83). Additionally, mTOR signaling is a major regulator of cell metabolism and autophagy by consistently inducing autophagy in cells (84). mTOR signaling is prone to alterations made in nutritive environments such as IGF-1, fatty and amino acids (49). mTOR regulation and aging are very closely related as the level of mTOR elevated in hematopoietic stem cells in elderly population suggesting that modulation in mTOR pathway can serve as a potential therapeutic marker in aging (85).
3 Biosynthesis and metabolism of Withania compounds
There has been extensive use of WS for its several medicinal properties which are responsible for many beneficial pharmacological activities (86). Withanolides are crucial secondary metabolites demonstrating pharmacological activity (87). To date, multiple withanolides, including prominent ones like withanolides, withaferin A (WA) and withanone are extracted from WS (88). Therefore, due to the growing demand of WS in the medicinal industry, it led to the search of a wide range of approaches for the mass production of roots of WS (89). Activation of metabolic pathway leads to the WS constituents in various parts. The major withanolides are produced via mevalonate (MVA) and methylerythritol 4-PO4 (MEP) pathways occurring in plastids and cytosol, respectively, (90). Chemically, withanolides are also known as triterpenoids which are 30-carbon compounds (91). Triterpenoids generally are biosynthesized through a metabolic pathway. This require isoprene units such as isopentenyl pyrophosphate (IPP) and dimethyl allyl pyrophosphate (DMAPP) as precursors (91). The MVA pathway engages seven enzymes to create precursor molecules IPP and DMAPP for terpenoid biosynthesis (92). Initially, it starts by condensing two acetyl-CoA molecules into acetoacetyl (AcAc)-CoA, facilitated by the enzyme AcAc-CoA thiolase to form 3-hydroxy-3-methylglytaryl-coenzyme A (HMG-CoA). Further, HMG-CoA reductase catalyzes a double reduction reaction which results in mevalonate biosynthesis from HMG-CoA that converts into IPP. IPP further converts to DMAPP. IPP from MEP pathway is converted to farnesyl pyrophosphate (FPP) (90). Squalene biosynthesis occurs by the FPP condensation in an NADPH-dependent manner. The delta-lactonization of 24-methylenecholesterol synthesis is an important step leading to withanolide biosynthesis (90). The biosynthetic pathway of withanolides including MVA and MEP pathway is illustrated in Figure 4.
Senthil et al. (93) carried out transcriptomic analysis of WS leaf and root tissues cultured in vitro. This provided insights on gene expression involved in biosynthesis and accumulation of essential withanolides (93). Various critical genes, such as hydroxymethylglutaryl reductase (HMGR), farnesyl pyrophosphate synthase (FPPS), glucosyltransferases (GT), cycloartenol synthase (CAS), squalene (SE) have specific roles in the process (94). For instance, HMGR facilitates HMG-CoA to mevalonate conversion, while FPPS led to the production of FPP, a fundamental element in numerous pathways (95). Phytosterols and triterpenoids are produced with the help of SE and CAS genes. Furthermore, GT facilitates the glycosylation of various natural compounds, aiding in the detoxification process (96). FPPS, another crucial enzyme, starts the biosynthesis of triterpenoid precursors to support withanolide production within the isoprenoid pathway (97). Expression of these genes involved in withanolide production displayed varying patterns along with essential withanolides like withanolide A and withaferin A. CAS, following the production of 2,3-epoxy squalene, induces the following step which leads to 24-methylene cholesterol synthesis, a precursor vital for withanolide synthesis (93). Also, a study (98) was conducted where virus induced gene silencing technique was used to assess the genes involved in withanolides’ biosynthesis. Through quantitative RT-PCR studies, it was revealed that biosynthesis of terpenoids is modulated by complex coordination of various enzymes and flux distribution of several metabolic channels (98).
Soni et al. (99) evaluated the function of endophytic Bacopa monnieri after isolation and identification of the fungal endophytes with plant growth promoting potential. By isolating endophytes under in-vitro conditions, secondary metabolites were produced. The study inferred that B. monnieri helps in withanolide production and biosynthesis in a cost-effective manner (99).
Gupta et al. (100) performed transcriptomic analyses for WS root and leaf to synthesize Withanolide A and Withaferin A, respectively, to further facilitate the understanding of Withanolide synthesis pathway. Analyses of the data shed light on the genes participating in synthesis of withanolide (100). Also, the investigation pinpointed genes such as CYP450, glycosyltransferase (GT), and methyltransferase (MT) that showed distinctive occurrence or expression in roots and leaves, potentially contributing to tissue-specific withanolide synthesis. The resulting sequencing for WS highlighted insights into the biosynthetic pathways of secondary plant products that are specific to tissues. Furthermore, it presents opportunities for devising strategies to enhance withanolide biosynthesis through biotechnological interventions (100).
4 Role of Withania somnifera in modulating RNAs in aging and inflammation
4.1 Non-coding RNAs in aging and inflammation
There is a scarcity of literature on non-coding RNA in aging and inflammation. MicroRNAs (miRNAs) play major role(s) in progression of many medical complications, including osteoarthritis (OA) (101). OA is traditional age-related disorder as the chances of OA increases with age due to sarcopenia and structural loss of bones in increasing age (102). Destruction of articular cartilage, limitation of movement, bone inflammation and synovitis are characteristics of OA (103). miR-25 modulates the expression of inflammatory markers and is downregulated in OA conditions (104). To check the role of Withaferin A in modulation of miR-25, a study was conducted (105) to investigate the role of Withaferin A in osteoarthritis using rabbit articular chondrocytes. It was reported that treatment of Withaferin A increased miR-25 expression by inducing the expression of cyclooxygenase 2 (COX2) providing a therapeutic approach in the treatment of OA (105). Further, miR-181c-5p is associated with the promotion of NF-κB mediated inflammation by downregulating protein tyrosine phosphatase nonreceptor type 4 (PTPN4) and therefore miR-181c-5p level may serve as a potential therapeutic factor in inflammation (106). However, Shuaib et al. elucidated the impact of WS on miR-181c-5p, and it was inferred that Withaferin A potentially upregulated the expression of miR-181c-5p (107).
4.2 Messenger RNAs involved in inflammation and aging-associated diseases
4.2.1 mRNAs involved in inflammation
One of the prevalent features related to aging is inflammation and, in the absence of any kind of open infection, this chronic inflammation is referred to as “inflammaging” (108). It poses a major risk factor in major health degradation and mortality in elderly populations (109). Human keratinocytes have a crucial role in inflammation by mediating tumor necrosis factor (TNF)-α and interleukins (IL) (110). Many skin diseases that are related to inflammation such as psoriasis, atopic dermatitis and allergic contact dermatitis are highly associated with keratinocytes and cytokines (111). Therefore, to study the anti-inflammatory effects of WS in modulation of these cytokines, Sikandan et al. (112) demonstrated the effect of Ashwagandha water extract (ASH-WEX) in human keratinocyte (HaCaT) cell line and 6-week-old male C57BL/6 J mice. RNA was isolated after the fifth day of treatment from mice skin and RT-qPCR was performed to check the transcriptional regulation of inflammatory cytokines in both HaCaT cells and C57BL/6 J mice. It was inferred that ASH-WEX inhibited TNF-α, and interleukins (IL-6, -8, -1β, and -12) while the expression of TGF-β1 was reduced in a dose-proportional manner (112). Also, mRNA expression studies performed in mice showed that ASH-WEX treatment inhibited TNF- α expression while it overexpressed TGF-β at mRNA level (112). Furthermore, a study was conducted (113) to demonstrate the beneficial effects of dry leaf extract of WS on anxiety and neuroinflammation which results from obesity. Using mRNA expression analysis by qRT-PCR, it was observed that WS led to inhibition of IKKα/β which further suppressed NF-κB signaling, and reduced the expression of iNOS, GFAP, IL-1β, TNF-α, PPARγ and MCP-1. Also, WS treatment led to upregulation of Bcl-xL and downregulation of Bad mRNA which further inhibited high fat diet-induced apoptosis in rats (113).
Signaling pathways including modulation of RNA by Withania somnifera in inflammation are summarized in Figure 5.
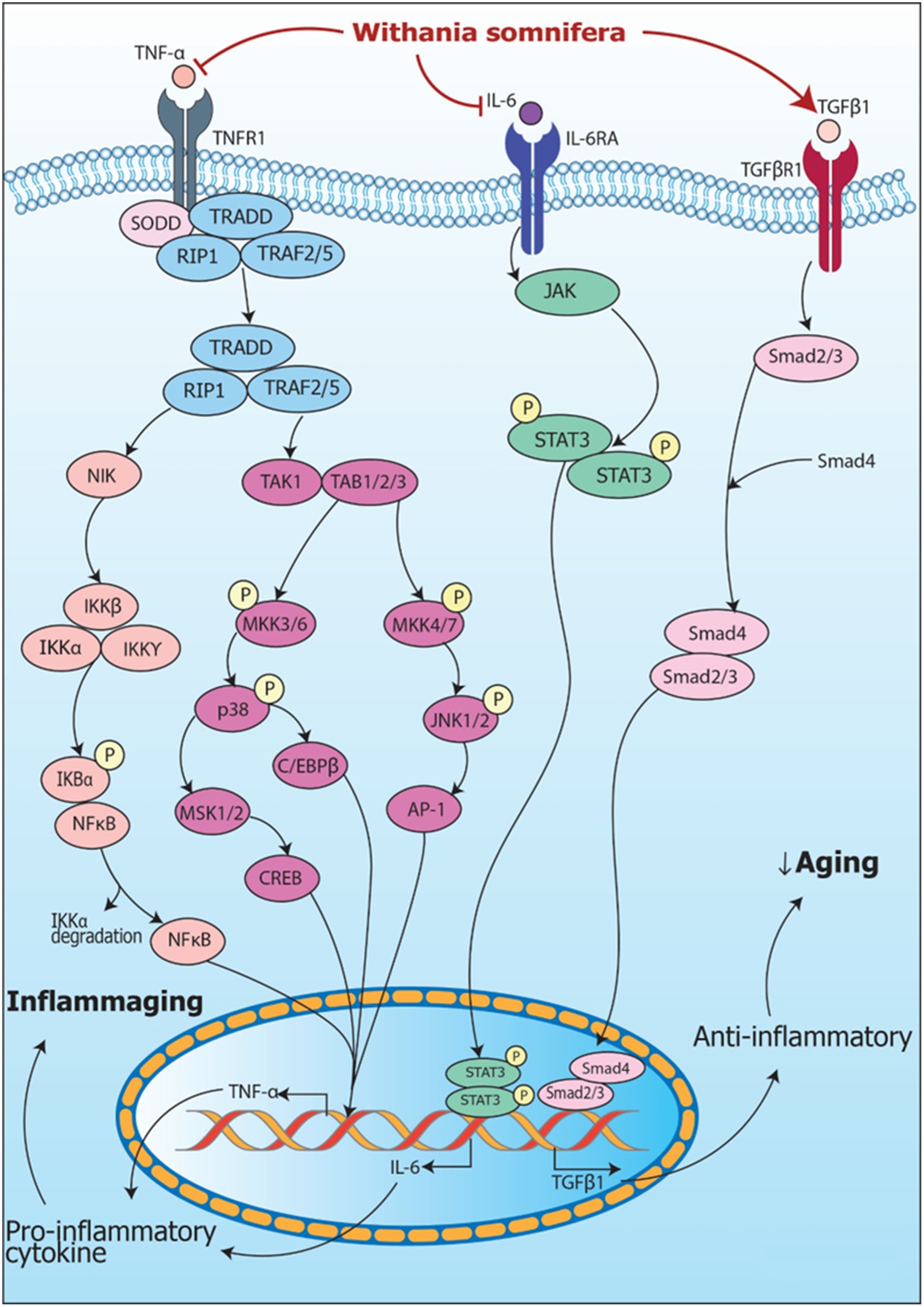
Figure 5. Signaling pathways implicating Withania somnifera in modulation of RNAs in inflammation. (1) TNFα, a tumor suppressive cytokine, binds to TNFR1 releasing SODD and activating TRADD complex which further activates NIK and TAK/TAB1/2/3 complex resulting in NF-κB and MAPK signaling pathway activation that transcribes TNFα mRNA via NF-κB, CREB, C/EBPβ and AP-1 signaling. (2) TNFα is inhibited by Withania somnifera at transcriptional level which further inhibits inflammation. In addition, IL-6 binds to IL-6R activating JAK/STAT signaling pathway which transcribes IL-6 mRNA. Withania somnifera inhibits IL-6 at transcriptional level which, in turn, inhibits JAK/STAT signaling. Moreover, TGFβ1, anti-inflammatory cytokine binds to TGFβR1 initiating SMAD signaling leading to transcription of TGFβ1 mRNA. Withania somnifera induces the expression of TGFβ1 resulting in attenuation of inflammation.
4.2.2 mRNAs involved in neurodegeneration
A key neurodegeneration hallmark includes accumulating advanced glycation endproducts (AGE) in the brain (114). Similarly, receptors for advanced glycation endproducts (RAGE) are overexpressed in brain (115). Gathering of amyloid-beta (Aβ) is considered as a major indicator of neurogenerative disease (116). Two crucial proteins, nuclear factor-κB (NF-κB) and (NLRP3), play significant roles in the neurodegenerative disorders development which include Alzheimer’s disease (AD) (117). Additionally, epigenetic modulation which includes histone deacetylase 2 (HDAC2) alteration is considered as a pivotal factor in Alzheimer’s disease (AD) pathogenesis (118). Hence, to investigate the therapeutic role of WS in modulation of these genes in AD, Venkata et al. (119) performed gene expression analysis using SH-SY5Y cells overexpressing amyloid precursor protein (SH-APP) cells. The results revealed that treatment with WA decreased NF-κB and IL-1β expression significantly, concluding that both of these genes have crucial roles in NF-κB-mediated neuroinflammation. Additionally, Withaferin A administration in SH-APP cells led to a downregulation of Aβ, an important mediator in the neuroinflammatory processes implicated in the pathogenesis of AD (119).
The memory process can be affected by a range of pathological factors, such as neurodegenerative disorders, stroke, tumors, head injuries, oxygen deficiency, cardiac surgery, nutritional deficiencies, neurological complexities such as depression and anxiety as well as side-effects of different medications and the natural process of aging (120). Scopolamine, a cholinergic antagonist, induces amnesia in humans as well as in rat models (121). These models have found extensive application in comprehending the molecular, biochemical, and behavioral alterations, serving as valuable tools for identifying potential therapeutic targets in the context of memory impairment (121). To evaluate the role of Withania somnifera in memory impairment, Konar et al. (122) assessed the therapeutic targets using alcoholic extracts of Withania leaves (i-Extract) in scopolamine-induced male Swiss albino mice of 12 weeks. From RT-PCR analysis, it was reported that scopolamine downregulated the mRNA expression of brain derived neurotropic factor (BDNF) and glial fibrillary acidic protein (GFAP) in mouse cerebrum in proportion to time and dose. Furthermore, it was also observed that i-Extract treatment attenuated the downregulation of scopolamine treatment in proportion to dose suggesting that WS serve as putative therapeutic agents to minimize the progression of neurodegenerative disorders (122).
Signaling pathways involving RNA in neurodegeneration and their modulation by W. somnifera are summarized in Figure 6.
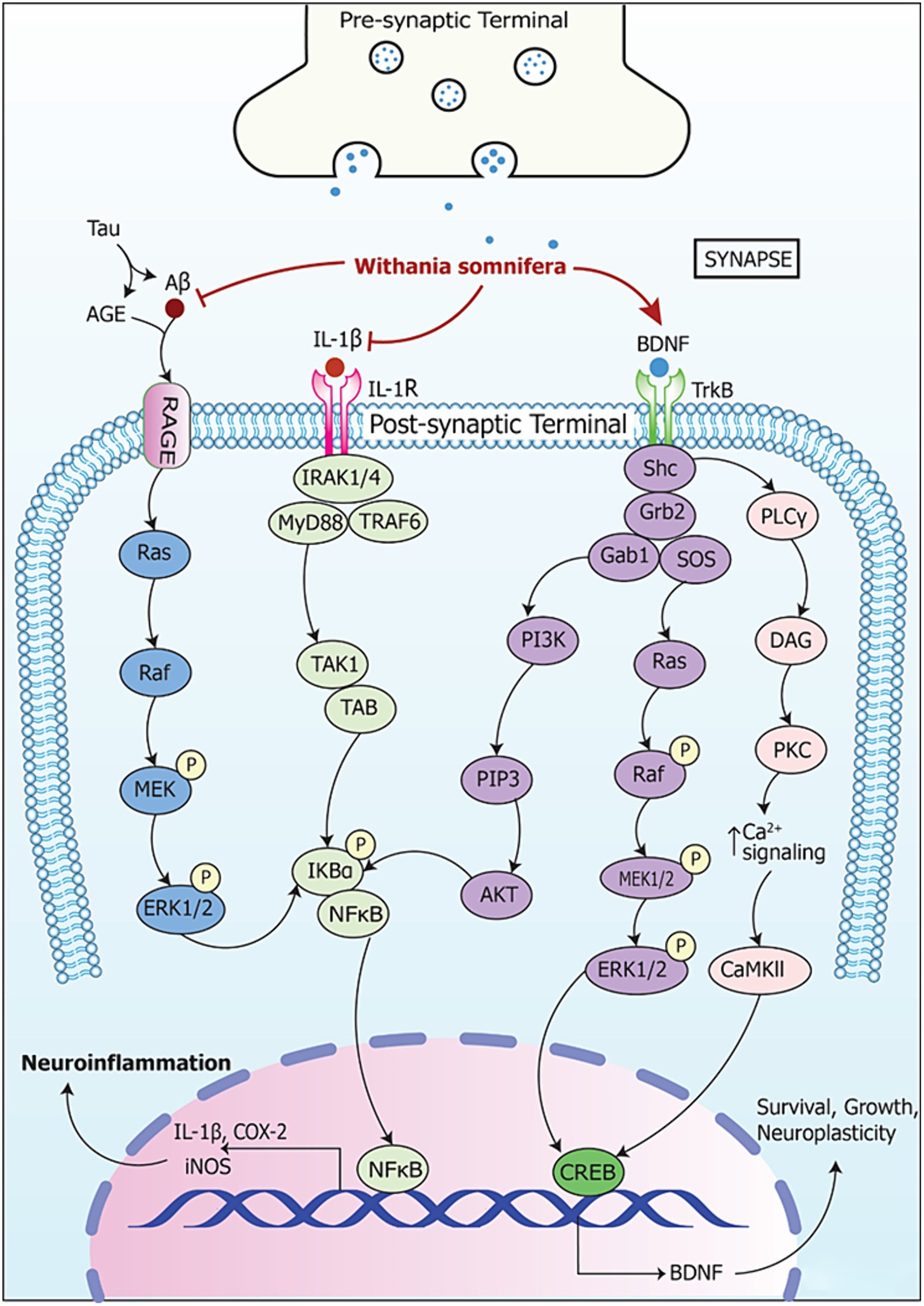
Figure 6. Signaling pathways implicating Withania somnifera in modulation of RNAs in neurodegeneration. AGE (advanced glycation endproduct) is made and secreted by microgial cells when activated. This induces RAGE expression in neurons leading to cell death causing neurodegenerative diseases. Also, iNOS is activated by AGE which further enhances apoptosis and degeneration of neuronal cells. AGE, when increased in quantity, augments formation of amyloid-beta (Aβ), tau protein and amyloid precursor protein (APP). This, in turn, induces tau hyperphosphorylation as well as AGE-Aβ cross-linking. Further, Aβ binds to RAGEs to activate ERK1/2 pathway. This leads to phosphorylation of NF-κB and proteasomal degradation of IKBα. This, in turn, enables NF-κB translocation toward nucleus and their gene target is expressed simultaneously. Moreover, IL-1β binds to IL-1R and activates IRAK1/4/MyD88/TRAF6 complex leading to NF-κB signaling via TAK1/TAB. This results in expression of their target genes which contribute to neurodegeneration. Withania somnifera inhibits the expression of Aβ and IL-1β at transcriptional level which leads to further inhibition of the downstream pathways. Thus, it reduces the expression of pro-inflammatory genes. Furthermore, Withania somnifera induces the activity of brain-derived neurotropic factor (BDNF) which is inhibited in neurodegeneration. The binding of BDNF to tropomyosin-related kinase receptor type B (TrkB) leads to homodimerization and activation of adaptor protein such as Src homology domain 2 (SH2). Thereafter, SH2 activation leads to the activation of phosphoinositide 3-kinases (PI3K)-AKT, Ras-mitogen-activated kinase (Ras-MAPK) and phospholipase Cγ1-protein kinase C (PKC) signaling pathway.
4.2.3 mRNAs involved in stress
The aging process is linked to alterations in endoplasmic reticulum (ER) chaperones and expression of folding enzymes (123). This leads to proteostasis disruption and elevation of misfolded proteins (123). Lowering the level of regulatory unfolded proteins results in ER stress which is linked to the unfolded protein response (124). Prolonged endoplasmic reticulum (ER) stress stimulates protein kinase-like ER-resident kinase (PERK), activates transcription factor-6 (ATF-6) and inositol requiring 1 (IRE1) apoptotic signaling (125). This leads to overexpression of C/EBP homologous protein (CHOP) expression, a proapoptotic-transcription factor (induced in ER stress), and is activated by p38 MAPK (126, 127). CHOP expression is usually regulated at mRNA level via PERK/eIF-2α/ATF6 pathway, ATF4 and IRE1/XBP1 pathway (128). Thus, to investigate the effect of Withaferin A in inducing apoptosis through ER stress, Choi et al. (129) conducted mRNA expression study of ER-stress specific X-box binding protein (XBP1) using human renal carcinoma cell line (Caki). Real-time PCR analysis showed that splicing of XBP1 mRNA was initiated following treatment with WA which in turn inactivates CHOP. Eukaryotic initiation factor-2α (EIF-2α) phosphorylation is activated by PERK which takes place during ER stress that leads to translational initiation and protein synthesis (130). These results suggest key signaling events implicated in ER-stress mediated apoptosis using WA which may facilitate chemopreventive and chemotherapeutic strategies based on Withaferin A (129).
“Adaptogens” are considered as plant-derived extracts or compounds which help organisms to adapt and survive during stress response (131). Activation of the gene that regulates stress response, which in turn postpones aging or ameliorates aging, is an effective way of tackling aging-associated diseases (132). Panossian et al. (133) studied adaptogens effect in stress and age-related diseases by using dunal root extract of WS in human T98G neuroglia cell line using mRNA expression profiling. It was reported that WS along with other adaptogens plays a major role in altering homeostasis. Also, it was observed that WS extracts downregulated PDE9A gene at transcriptional level. This indicated their potential to modulate transcriptional regulation in preventing aging-related and stress-induced disorders (133).
As people get older, the consistency or stability of circadian clock gene expression patterns is understood to potentially change or become less resilient (134). The suprachiasmatic nucleus (SCN) serves as the central master pacemaker, orchestrating the regulation of peripheral clocks found in all body tissues (135). In aging or in age-related complexities circadian timekeeping systems decrease (136). Circadian clocks operate through intricate transcriptional translational feedback loops (137). Two supplementary loops which include RORs, NFIL3, DBP and REV-ERBs, collaborate to produce discernible rhythms within a period of 24 h (138). Kukkemane et al. (139) evaluated WS influence on rhythmic alteration and levels on a daily basis with regard to age. They examined the expression of a hydroalcoholic leaf extract of WS using male Wistar rats. Through qRT-PCR analysis, they observed a significant chrono modulatory effect of WS. This effect was evident in the restoration of rhythms and pulses of clock genes namely Sirtuin1 and NRF2 daily. It was concluded that WS may offer therapeutic benefits in mitigating age-related disruptions in the circadian clock. This potential benefit is proposed to operate through two key pathways: SIRT1 modulation and antioxidant effects (139).
The regulation of signaling pathways in stress by Withania somnifera at RNA level are summarized in Figure 7.
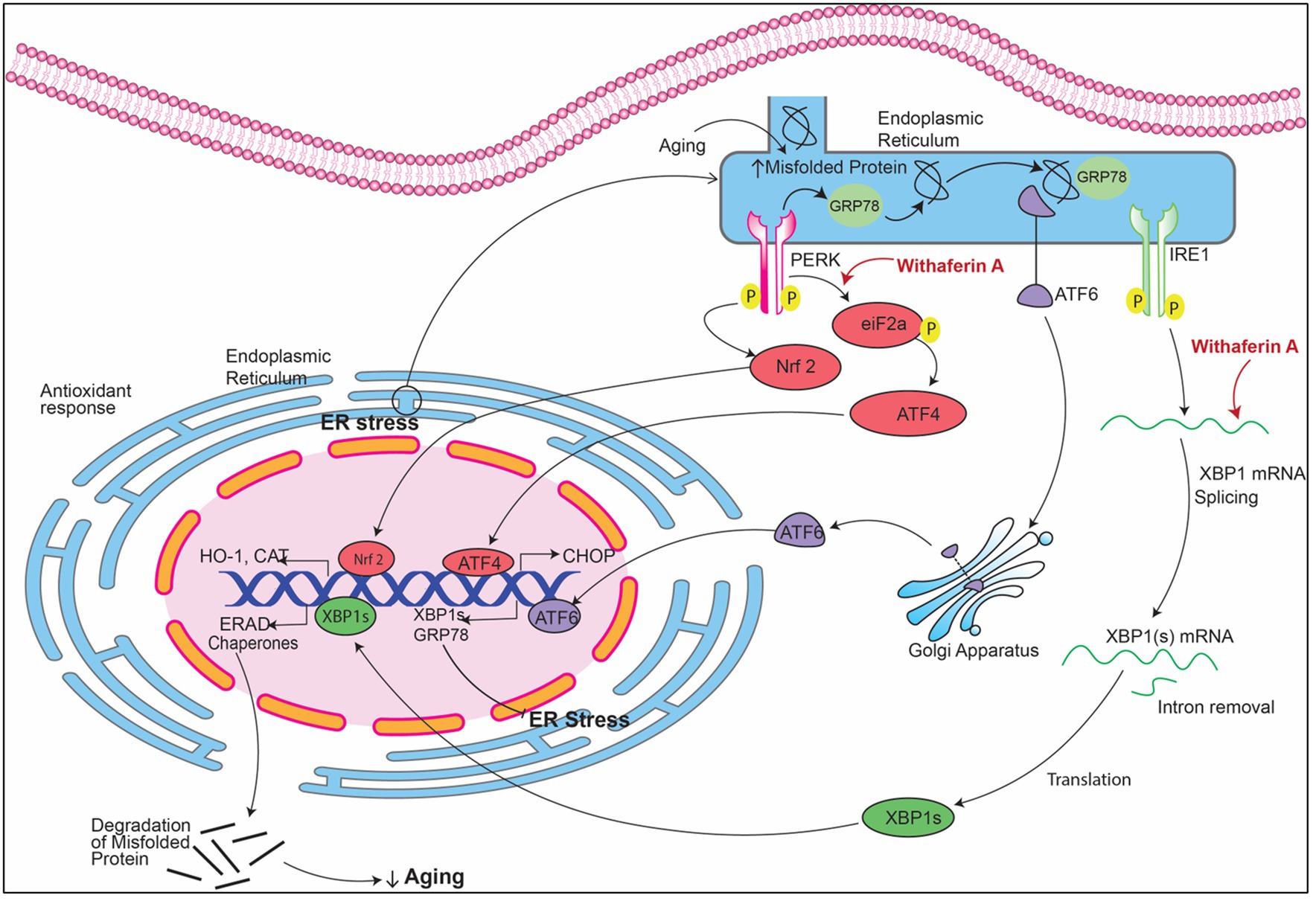
Figure 7. Signaling pathways implicating Withania somnifera in modulation of RNAs in ER stress. In ER stress, GRP78 (glucose-regulated protein) attaches to the misfolded proteins present in the endoplasmic reticulum (ER) which releases transmembrane proteins from endoplasmic reticulum (ER) namely inositol-requiring enzyme (IRE1), PRKR-like ER kinase (PERK) and activating transcription factor 6 (ATF6). These three proteins, when activated, initiate UPR signaling cascades. XBP1 mRNA is transcribed into XBP1s after cleaving by IRE1. PERK dimerization leads to phosphorylation of eIF2a that causes inhibition of protein translation. Withaferin A induces the splicing of XBP1 which in turn activates CHOP expression which resulting in inhibition of mitochondrial mediated apoptosis.
4.2.4 mRNAs involved in organ fibrosis
Fibrosis is characterized by endpoint of pathological remodeling which triggers the progression of various chronic disorders and aging-associated organ damage (140). Hence, it is important to alleviate the symptoms or inhibit the downstream pathways to prevent fibrosis. To investigate the potential role of Withaferin A as a preventive constituent in fibrosis, a study was conducted by Gu et al. (141) using male 8-week-old C57/BL6 mice. Silent information regulator (SIR) protein family exhibits an important role in cell cycle regulation, mitochondrial homeostasis maintenance, caloric restriction and lipid metabolism to regulate glucose levels. Studies have elucidated that SIRT3 deficiency can aggravate liver injury while SIRT3 activation led to alleviate liver fibrosis (142). In this study, mRNA expression level of SIRT3 was assessed after treatment of WA in C57/BL6 mice. It was reported that WA led to decrease of liver fibrosis in a SIRT3-dependent manner (141).
Aged kidney is susceptible to kidney injury which is indicated by extended inflammation that can increase dysfunction of renal tissue (143). NF-κB signaling pathway is a mediator between inflammation and fibrosis in kidney (144). Several stimuli can trigger NF-κB activity in the kidney such as TNF (145) and angiotensin II (146), and both of these are linked to chronic kidney disease. The target genes of NF-κB are long but include CCL2 and CCL5 which are linked to renal fibrosis (147). Therefore, to assess the role of WS in regulating these inflammatory genes, Grunz-Borgmann et al. (148) studied the expression using hot water soluble extracts of WS in rat kidney NRK-52E cell line. Transcriptomic analysis was performed using real-time PCR by using different doses of WS. It was reported that WS pre-treatment gradually inhibited CCL2 and CCL5 expression NRK-52E cells. Also, pre-treatment with WS reduced the effect of LPS-induced NF-κB activity indicating anti-inflammatory effect of WS and alleviating renal fibrosis (148).
Hepatic toxicity has been proved to be aggravated during aging especially by the consumption of acetaminophen (APAP) (149). APAP is generally used as an analgesic and antipyretic drug (150). However, when used in large concentrations, it causes hepatotoxicity and necrosis in humans (151). Devkar et al. (152) studied the outcome of withanolide-rich fraction (WRF) in liver protection using male Swiss albino mice. Expression of TNF-α, IL-1β, iNOS and COX-II was assessed at the transcriptional level in APAP-treated mice. It was reported that WRF significantly downregulated TNF-α and IL-1β transcriptionally corelating with the dose level. However, mRNA expression of iNOS and COX-II was reduced at higher dose (200 mg/kg) of WRF. Reduced expression of COX-2, iNOS, IL-1β, TNF-α achieved by WRF intervention showed anti-inflammatory and antioxidant properties of WS leading to enhanced liver protection (152).
The modulation of RNA in signaling involved in organ fibrosis by Withania somnifera is summarized in Figure 8.
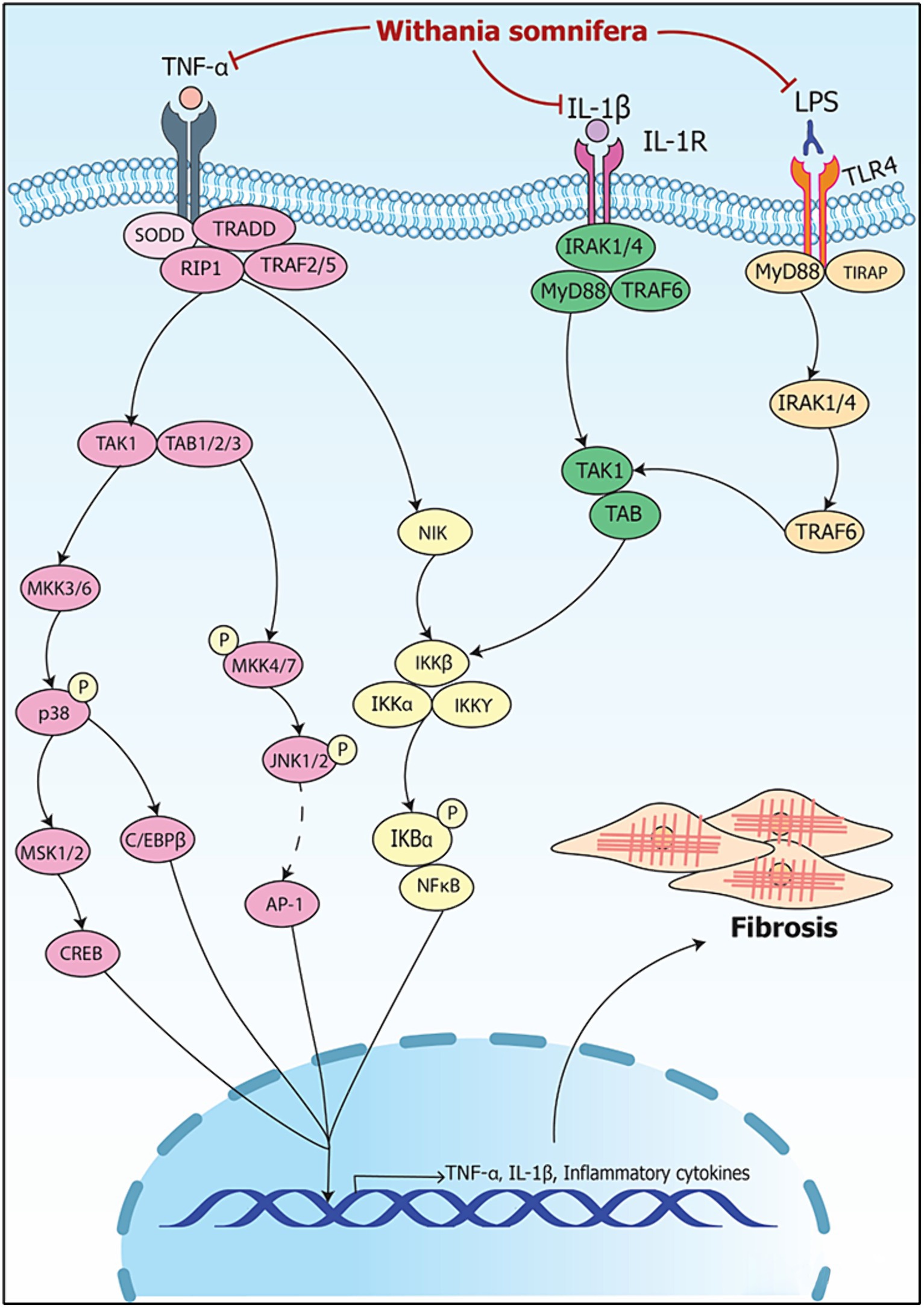
Figure 8. Signaling pathways implicating Withania somnifera in modulation of RNAs in organ fibrosis. TNF-α binds to TNFR and releases SODD leading to activation of TRADD/RIP1/TRAF2/5 complex; the complex activates TAK1/TAB1/2/3 and NIK; TAK1/TAB1/2/3 activates CREB and C/EBPβ via p38 and MKK4/7-JNK signaling. NIK activates IKK/NFκB signaling; IL-1 ligand receptor complex activates IRAK1/4/MyD88/TRAF6 complex further activating IKK/NFκB signaling. Moreover, LPS binds to TLR4 activating NFκB via TRAF6 signaling. CREB, C/EBPβ and NFκB results in the transcription of IL-1β and TNF-α. WS inhibits the binding of TNF-α, IL-1β and LPS to TNFR, IL-1R and TLR4, respectively, and suppresses inflammation resulting in attenuation of fibrosis.
4.2.5 mRNAs involved in muscle impairment
The decline in mass and function of skeletal muscle typically commences around the age of 40, with muscle mass potentially diminishing at a rate of 1–2% annually after reaching 50 years of age (153). This can lead to escalating complications in health-related outcomes which lead to functional restriction of the body (154). During aging, reduced muscle building capacity leads to loss of muscle mass (154). It is believed that the loss of muscle due to aging is influenced by apoptosis, however the mechanisms behind it remain unclear (153). The Bcl-2 protein family is implicated in apoptotic signaling pathway, consisting of Bax and Bcl-2 proteins, which regulate the process of mitochondria-mediated cell death (155). While Bcl-2 helps prevent cell apoptosis, Bax, a protein like Bcl-2, promotes cell death (156). To understand the effect of WS on the regulation of apoptotic proteins, Panda et al. (153) conducted transcriptomic analysis using hydroalcoholic extracts of WS in male Sprague Dawley rats. Their study revealed that expression of Bax was reduced after the treatment with WS, along with an increase in Bcl-2 expression. It was therefore inferred that WS may offer a therapeutic approach to address muscle weakness and deterioration (153).
Signaling pathway modulated by Withania somnifera in muscle impairment is depicted in Figure 9.
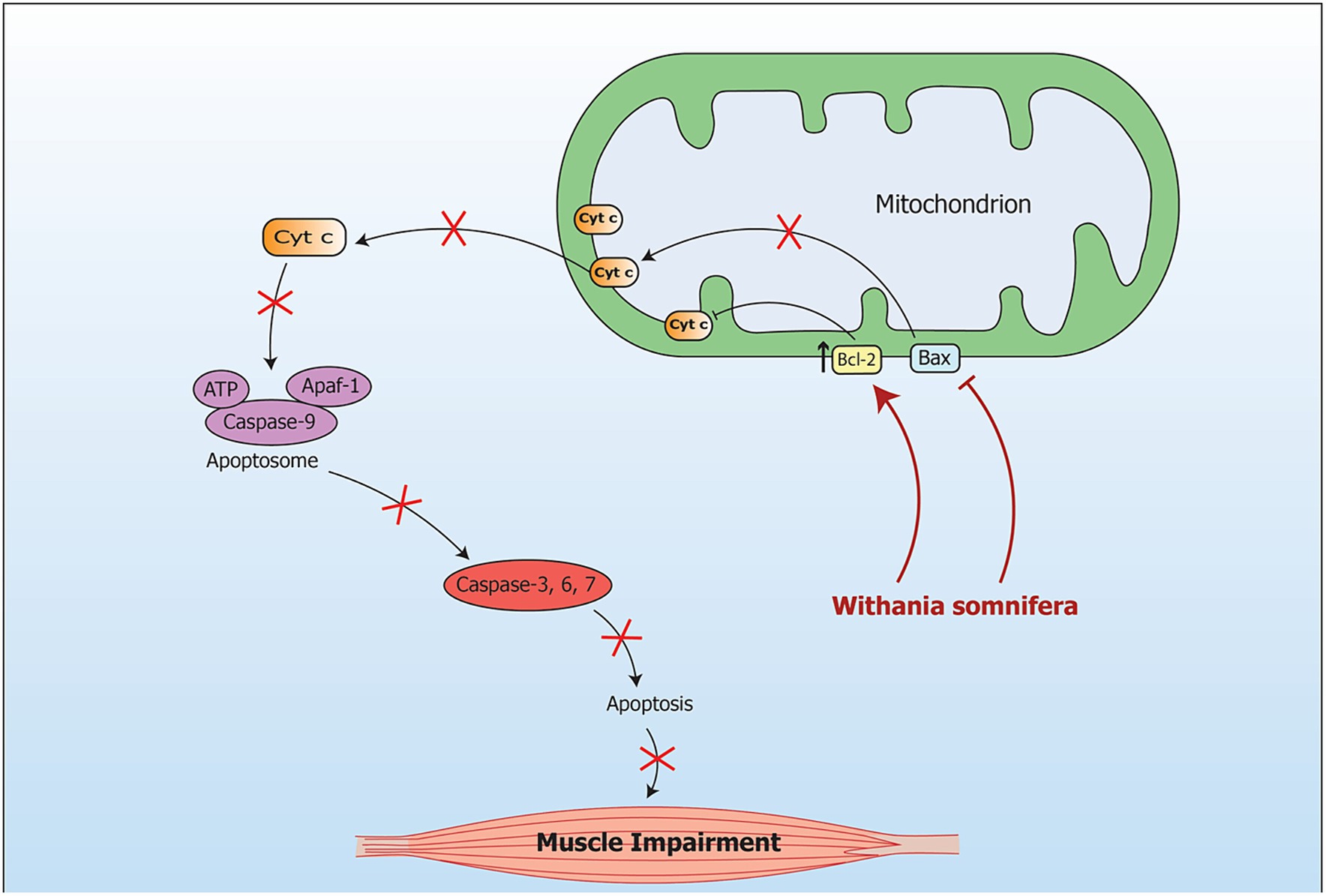
Figure 9. Signaling pathway implicating Withania somnifera in modulation of RNAs in muscle impairment. During oxidative stress, the mRNA level of Bax is increased while the mRNA level of Bcl is decreased in mitochondria. This leads to muscular apoptosis. Another pathway which is caspase dependent includes apoptosis due to stimulation of Caspase-3. Further, cytochrome c oozes out of the mitochondria in response to changes in Bax/Bcl-2 ratio. Introduction of WS inhibits Bax expression at transcriptional level which further inhibits apoptosis in muscle.
4.2.6 Miscellaneous mRNAs in aging
Telomerase shortening is considered as the main factor which escalates the rate of aging and promotes degeneration processes (157). Telomere is progressively shortened with each DNA replication, which leads to appearance of critically short telomeres (158). Low telomerase activity is targeted by both exogenous and endogenous factors which lead to DNA damage and low maintenance of cell function (159). Withanolide, an active constituent of WS indicated 29.7% extension in the lifespan of C.elegans via regulation of insulin/IGF-1 signaling pathway (160, 161).
Cellular senescence refers to cell-cycle suspension and secretion of inflammatory mediators which leads to aging and complications associated with aging (162). Obesity is an important comorbidity with increase in burden of senescent cell as well as neuropsychiatric disorders such as anxiety and depression (163). Withaferin A aids in alleviating several metabolic disorders. Therefore, to evaluate the protective effect of Withaferin A in conditions such as obesity, Abu Bakar et al. (164) performed a study using HFD-induced obese mice. They treated the mice with Withaferin A for 12 weeks. Pro-inflammatory cytokines were reduced following WA treatment. Additionally, using transcriptional analysis by real-time PCR, expression of all genes involved in inflammation such as TLR4, CCR2, NF-κB, COX2, TNF-α, IL-1β and CCL2/3 were downregulated following WA treatment. Also, WA downregulated the mRNA expression of main regulators of lipid metabolism (PPARα and γ, CD36, FAS and CPT1). These results suggested that WA treatment can help in regulating lipid and glucose metabolism in liver at the transcriptional level and provide a therapeutic response for WA in obesity (164).
Changes in vascular extracellular matrix in aging appear as one the major facets of dysregulated angiogenesis (165). In the process of angiogenesis, endothelial cells lead to the formation of new vasculature (166). Additionally, it is implicated in the progression of tumor cells and metastasis (167). The uniform release of VEGF, bFGF, PDGF and TGF lead to the activation of angiogenesis (168). Transcriptional as well as post-transcriptional mechanisms tightly regulate the expression level of VEGF mRNA (169). Recent research has unveiled the role of signaling pathways and genetic components governing this expression. The activation of the transcription factor Sp1 precedes VEGF promoter activity, showing that VEGF overexpression is regulated by Sp1 activation (170). This overexpression leads to angiogenesis and cancer progression (171). Sp1 has been evaluated as a crucial factor in tumor angiogenesis and aids in the pathogenesis of pancreatic adenocarcinoma (172). Santhekadur et al. (170) performed an experiment to explore the antiangiogenic effects elicited by WS, which resulted in a reduction in ascites fluid and VEGF expression, regulated by the Sp1 transcription factor, using Ehrlich ascites tumor (EAT) cells-bearing mice. Nuclear extracts were obtained from both untreated and withaferin A-treated EAT cells, and the binding activity of Sp1-DNA was studied using Electrophoretic Mobility Shift Assay (EMSA) with Sp1 oligonucleotides. The findings showed that Sp1 binding to VEGF gene promoter region was inhibited by withaferin A (170). Further, a study was carried out by Sajida and Prabhu (173) to analyze the activity of root extracts of WS using A549 human lung carcinoma cell line via inhibition mechanisms including antioxidant, autophagy and angiogenic inhibition. The expressions of VEGF, angiogenin and MMP-2 were assessed at the transcriptional level using qRT-PCR. It was reported that angiotensin and VEGF gene expression was significantly downregulated in WS extract-treated cells but there was a lower effect on MMP-2 expression (173).
The signaling pathways involving Withania somnifera in modulating RNAs in above-mentioned cellular events are illustrated in Figure 10. In addition, RNAs modulated by Withania somnifera are represented in Table 1 and summarized in Figure 11.
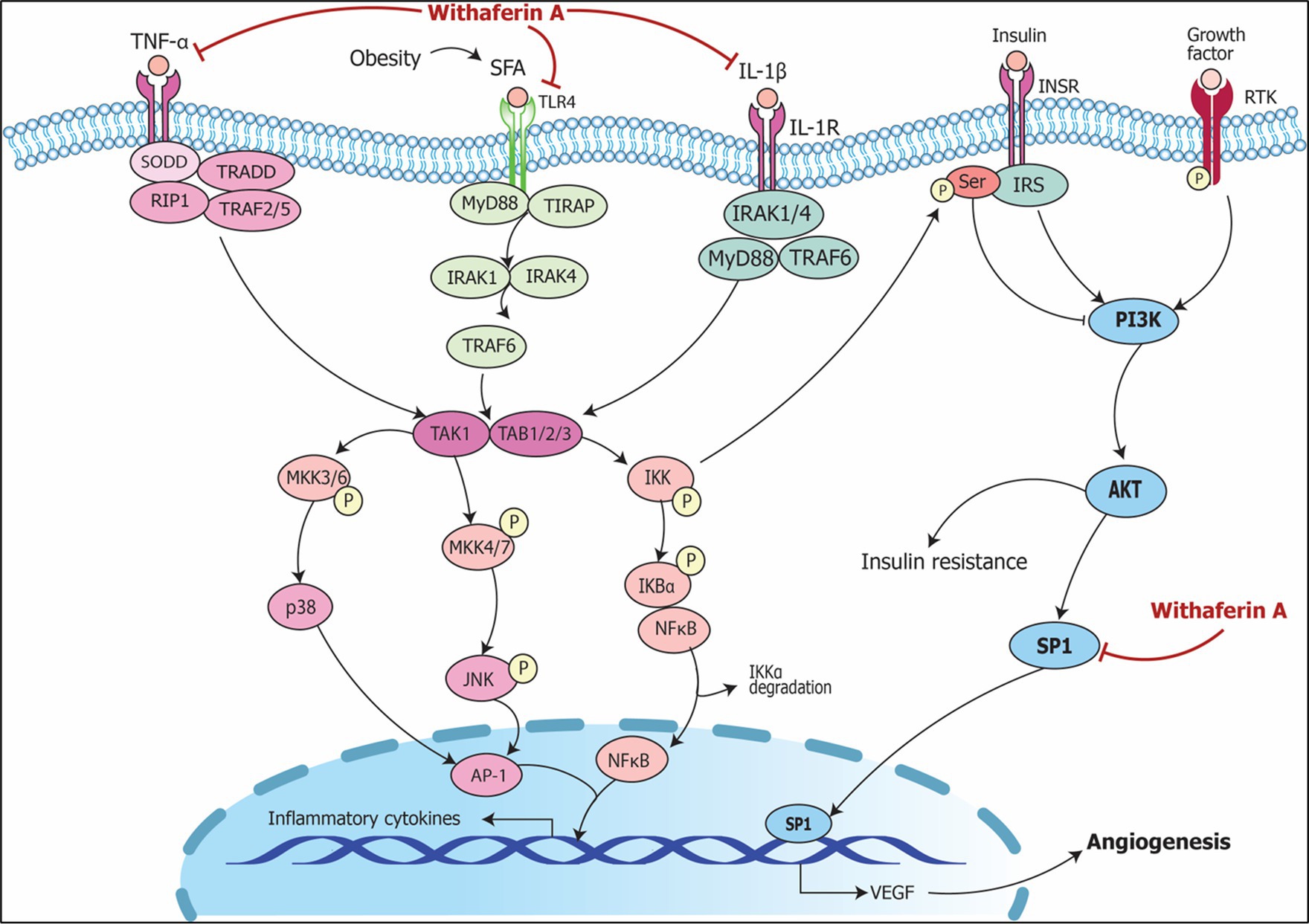
Figure 10. Signaling pathways implicating Withania somnifera in modulation of RNAs in other complications. Growth factor (GF) and receptor tyrosine kinase (RTK) binding leads to its phosphorylation which further initiates PI3K/AKT/SP1 signaling; RAS/RAF activates SP1 via MAP3K; both the events lead to transcription of VEGF resulting in angiogenesis. Withaferin A inhibits SP1 suppressing VEGF expression which results in the prevention of angiogenesis and oncogenic potential.
5 Sequencing studies with Withania somnifera in aging/inflammation
Panossian et al. (133) studied the expression of various plant-based adaptogens using mRNA sequencing to infer the role of plant extracts in alleviating complications that are concomitant with aging and stress. They performed RNA-seq to assess changes in gene expressions in adaptogen-treated T98G human neuroglia cells. The functional relevance of dysregulated genes to adaptive stress signaling was analyzed. Seventy-five genes were found to encode various biological components, which includes enzymes used in metabolism; neurohormones such as CRH, UCN, GNRH1; GPCR and transmembrane receptors like PLXNA4, GPR19, TLR9, PRLR, GPR158, GP1BA, VIPR2, CHRNE; transmembrane channels; protein kinases including FLT1, ROS1, MAPK10, MERTK, TTN, MAPK13, PRKCH; ligand-dependent nuclear receptor RORA; phosphatases namely PTPRD, PTPRR; transcription regulators such as ZNF467, FOS, SCX, ZFPM2, ZNF396, STAT5A, FOXO6; peptidases such as TLL1, PAPPA2; and various other proteins (133). These findings indicated that adaptogens might impact multiple adaptive stress-response signals, including those related to cyclic AMP, MAPK, NRF2 oxidative stress, glucocorticoid receptor (GR or NR3C1), maturation of neurons as well as CREB pathway which occurs in neurons and synapses, corticotropin releasing hormone, AMPK, melatonin, endothelial nitric oxide synthase, nutrient sensing in enteroendocrine cells mediated by GPCR, Gαs, GP6, opioid, renin-angiotensin and conditions such as neuropathic pain, and neuroinflammation. These results suggested that adaptogens provide a diverse biological function, eliciting numerous effects in regulating cellular metabolism and maintaining homeostasis at the transcriptional level (133).
Moreover, another study by Panossian et al. (174) elucidated anti-inflammatory properties of WS using T98G human neuroglia cell line using mRNA sequencing. A total of 14 genes which were included in eicosanoids pathway were altered by WS extracts. This includes arachidonate 5-lipoxygenase activating protein (ALOX5AP), GPCR such as PTGFR, TBXA2R, PTGER2, PTGER3, and several enzymes. It was inferred that downregulation of ALOX12 expression plays a key role in neurotoxicity. In addition, WS downregulated ALOX5AP, leukotriene C4 synthase (LTC4S) and DPEP2 genes involved in biosynthesis of leukotrienes pathway resulting in its inhibition. This, in turn, inhibited neuroinflammation and neurodegeneration leading to the suppression of Alzheimer’s disease complications. Also, PTGER3 expression was increased by WS which aids in ulcer prevention in the duodenum and small intestine. Moreover, EP3 receptor genes are upregulated in breast cancer patients. Therefore, suppression of PTGR3 can be used in treating breast cancer. Combination of Rhodiola and Withania inhibited LTC4 signaling by downregulation of LTC4S and upregulation of PTGER3 at genetic level which may serve as a promising approach in treating allergic asthma (174).
6 Clinical trials including RNA markers in aging and inflammation
Several trials have been performed in various parts of the world to study the effects of RNA markers (which include mRNAs and non-coding RNAs) on various aging and inflammation-related conditions such as CVD, neuroinflammatory and neurodegenerative complications, as well as complications related to metabolism in humans. Clinical trials data have been extracted from ClinicalTrials.gov (176), EU Clinical Trials Register (177) and Brazilian Registry of Clinical Trials (ReBEC) (178). These trials aim to evaluate the RNA-based interventions, as well as to identify biomarkers of response and resistance. These results may provide new understanding about aging and inflammation and insights into their mechanisms at the molecular level. This will pave the way for the advancement of novel therapeutic and preventive strategies in aging-related diseases. Clinical trials including RNA markers in aging and inflammation are summarized in Tables 2, 3.
7 Conclusion and future perspectives
Management of aging is difficult due to its progressive and irreversible nature, as well as the comorbidities associated with aging. However, the quality of biological aging can be improvised by recent advancements including intervention with nutraceuticals that can modulate the transcriptional activity of different genes implicated in aging and age-related complications. We have discussed the role(s) of Withania somnifera in modulating RNAs and alleviating the aging-associated comorbidities. Interestingly, Martínez-Mármol et al. (208) revealed that in brain organoids, long COVID prompted fusion among neurons and between neurons and glial cells. This fusion was attributed to a viral fusogen induced by the infection, driven by S-protein expression of SARS-CoV-2. These findings reveal how the nervous system is impacted by COVID-19 virus, potentially causing various neuropathologies. This indicates a potentially beneficial role for WS in long COVID management. However, the specific involvement of WS in the development of neuropathologies resulting from SARS-CoV-2 infection remains to be fully understood.
With the advent of new high-throughput ‘omics’ technologies and updation of the reference human genome, we are now better placed to investigate aging at the transcriptional level. Indeed, the field of RNA biology has grown tremendously with the development of antisense oligonucleotides (209, 210), aptamers (211), RNA vaccines (212), siRNAs (213, 214) and CRISPR/Cas9 gene editing (215). Further, the platforms used for RNA delivery are also expanding with nanocarriers (216), lipid nanoparticles (217) and exosomes (218) being evaluated as novel drug delivery systems. Future avenues of research will likely include a deeper understanding of Withania effects on the transcriptome by the use of next-generation sequencing followed by formulation of Withania in a suitable dosage form to modulate the genome. In this review, we summarize the promising effects of Withania in ameliorating age-related molecular changes by encompassing both in vitro and in vivo studies. We have also summarized clinical trials studying modulation of RNAs in aging to provide current insights into possible interventions for aging-related complications. Moreover, we delineate key signaling pathways regulated by Withania compounds and key aging biomarkers in the aging/inflammation process. Taken together, given the modulation of key RNA markers in aging and inflammation pathways, there is tremendous potential for harnessing the beneficial effects of Withania for achieving healthy aging.
Author contributions
PS: Writing – original draft. SA: Writing – original draft. DMa: Writing – original draft. SS: Writing – original draft. DMe: Writing – review & editing. SN: Supervision, Conceptualization, Project administration, Writing – review & editing.
Funding
The author(s) declare that no financial support was received for the research, authorship, and/or publication of this article.
Acknowledgments
The authors thank PhytoVeda Pvt. Ltd. for the support and Viridis Biopharma Pvt. Ltd. for electronic access to scientific databases.
Conflict of interest
PS, SA, DMa, SS, DMe, and SN were employed by PhytoVeda Pvt. Ltd. and Viridis Biopharma Pvt. Ltd., Mumbai, India.
The author(s) declared that they were an editorial board member of Frontiers, at the time of submission. This had no impact on the peer review process and the final decision.
Publisher’s note
All claims expressed in this article are solely those of the authors and do not necessarily represent those of their affiliated organizations, or those of the publisher, the editors and the reviewers. Any product that may be evaluated in this article, or claim that may be made by its manufacturer, is not guaranteed or endorsed by the publisher.
References
1. López-Otín, C, Blasco, MA, Partridge, L, Serrano, M, and Kroemer, G. The hallmarks of aging. Cell. (2013) 153:1194–217. doi: 10.1016/j.cell.2013.05.039
2. He, J, Tu, C, and Liu, Y. Role of lncRNAs in aging and age-related diseases. Aging Med Milton NSW. (2018) 1:158–75. doi: 10.1002/agm2.12030
3. Ageing and Health [Internet]. Available at: https://www.who.int/news-room/fact-sheets/detail/ageing-and-health (Accessed April 9, 2024)
4. GBD 2016 Disease and Injury Incidence and Prevalence Collaborators. Global, regional, and national incidence, prevalence, and years lived with disability for 328 diseases and injuries for 195 countries, 1990-2016: a systematic analysis for the global burden of disease study 2016. Lancet Lond Engl. (2017) 390:1211–59. doi: 10.1016/S0140-6736(17)32154-2
5. Dwolatzky, T, Brodsky, J, Azaiza, F, Clarfield, AM, Jacobs, JM, and Litwin, H. Coming of age: health-care challenges of an ageing population in Israel. Lancet Lond Engl. (2017) 389:2542–50. doi: 10.1016/S0140-6736(17)30789-4
6. Jiapaer, Z, Su, D, Hua, L, Lehmann, HI, Gokulnath, P, Vulugundam, G, et al. Regulation and roles of RNA modifications in aging-related diseases. Aging Cell. (2022) 21:e13657. doi: 10.1111/acel.13657
7. Rodríguez-Rodero, S, Fernández-Morera, JL, Menéndez-Torre, E, Calvanese, V, Fernández, AF, and Fraga, MF. Aging genetics and aging. Aging Dis. (2011) 2:186–95.
8. Sánchez-Valle, J, and Valencia, A. Molecular bases of comorbidities: present and future perspectives. Trends Genet. (2023) 39:773–86. doi: 10.1016/j.tig.2023.06.003
9. How to use Ashwagandha for dementia. Ayur Times [Internet]. (2020). Available at: https://www.ayurtimes.com/ashwagandha-for-dementia/ (Accessed March 31, 2024)
10. (PDF) Morphology of Withania somnifera (Distribution, Morphology, Phytosociology of Withania somnifera L. Dunal) [Internet]. Available at: https://www.researchgate.net/publication/328699590_MORPHOLOGY_OF_WITHANIA_SOMNIFERA_Distribution_Morphology_Phytosociology_of_Withania_somnifera_L_Dunal (Accessed April 9, 2024)
11. Saleem, S, Muhammad, G, Hussain, MA, Altaf, M, and Bukhari, SNA. Withania somnifera L.: insights into the phytochemical profile, therapeutic potential, clinical trials, and future prospective. Iran J Basic Med Sci. (2020) 23:1501–26.
12. Mishra, LC, Singh, BB, and Dagenais, S. Scientific basis for the therapeutic use of Withania somnifera (Ashwagandha): a review. Altern Med Rev J Clin Ther. (2000) 5:334–46.
13. Speers, AB, Cabey, KA, Soumyanath, A, and Wright, KM. Effects of Withania somnifera (Ashwagandha) on stress and the stress-related neuropsychiatric disorders anxiety, depression, and insomnia. Curr Neuropharmacol. (2021) 19:1468–95. doi: 10.2174/1570159X19666210712151556
14. Donlon, WC. Immunology in dentistry. J Am Dent Assoc. (1980) 100:220–31. doi: 10.14219/jada.archive.1980.0052
15. Ziauddin, M, Phansalkar, N, Patki, P, Diwanay, S, and Patwardhan, B. Studies on the immunomodulatory effects of Ashwagandha. J Ethnopharmacol. (1996) 50:69–76. doi: 10.1016/0378-8741(95)01318-0
16. Mandlik Ingawale, DS, and Namdeo, AG. Pharmacological evaluation of Ashwagandha highlighting its healthcare claims, safety, and toxicity aspects. J Diet Suppl. (2021) 18:183–226. doi: 10.1080/19390211.2020.1741484
17. Mikolai, J, Erlandsen, A, Murison, A, Brown, KA, Gregory, WL, Raman-Caplan, P, et al. In Vivo effects of Ashwagandha (Withania somnifera) extract on the activation of lymphocytes. J Altern Complement Med. (2009) 15:423–30. doi: 10.1089/acm.2008.0215
18. Alanazi, HH, and Elfaki, E. The immunomodulatory role of withania somnifera (L.) dunal in inflammatory diseases. Front Pharmacol [Internet]. (2023) 14:1084757. doi: 10.3389/fphar.2023.1084757
19. Priyanka, G, Anil Kumar, B, Lakshman, M, Manvitha, V, and Kala, KB. Adaptogenic and immunomodulatory activity of Ashwagandha root extract: An experimental study in an equine model. Front Vet Sci. (2020) 7:541112. doi: 10.3389/fvets.2020.541112
20. Tharakan, A, Shukla, H, Benny, IR, Tharakan, M, George, L, and Koshy, S. Immunomodulatory effect of Withania somnifera (Ashwagandha) extract—A randomized, double-blind, placebo controlled trial with an open label extension on healthy participants. J Clin Med. (2021) 10:3644. doi: 10.3390/jcm10163644
21. Iuvone, T, Esposito, G, Capasso, F, and Izzo, AA. Induction of nitric oxide synthase expression by Withania somnifera in macrophages. Life Sci. (2003) 72:1617–25. doi: 10.1016/S0024-3205(02)02472-4
22. Tiwari, R, Chakraborty, S, Saminathan, M, Dhama, K, and Singh, SV. Ashwagandha (Withania somnifera): role in safeguarding health, immunomodulatory effects, combating infections and therapeutic applications: A review. J Biol Sci. (2014) 14:77–94. doi: 10.3923/jbs.2014.77.94
23. Singh, N, Bhalla, M, de Jager, P, and Gilca, M. An overview on Ashwagandha: A Rasayana (rejuvenator) of ayurveda. Afr J Tradit Complement Altern Med. (2011) 8:208–13. doi: 10.4314/ajtcam.v8i5S.9
24. Salve, J, Pate, S, Debnath, K, and Langade, D. Adaptogenic and anxiolytic effects of Ashwagandha root extract in healthy adults: a double-blind, randomized, placebo-controlled clinical study. Cureus. (2019) 11:e6466. doi: 10.7759/cureus.6466
25. Babu, PVA, Gokulakrishnan, A, Dhandayuthabani, R, Ameethkhan, D, Kumar, CVP, and Ahamed, MIN. Protective effect of Withania somnifera (Solanaceae) on collagen glycation and cross-linking. Comp Biochem Physiol B Biochem Mol Biol. (2007) 147:308–13. doi: 10.1016/j.cbpb.2007.01.011
26. Pradhan, R, Kumar, R, Shekhar, S, Rai, N, Ambashtha, A, Banerjee, J, et al. Longevity and healthy ageing genes FOXO3A and SIRT3: serum protein marker and new road map to burst oxidative stress by Withania somnifera. Exp Gerontol. (2017) 95:9–15. doi: 10.1016/j.exger.2017.05.013
27. Gómez Afonso, A, Fernandez-Lazaro, D, Adams, DP, Monserdà-Vilaró, A, and Fernandez-Lazaro, CI. Effects of Withania somnifera (Ashwagandha) on hematological and biochemical markers, hormonal behavior, and oxidant response in healthy adults: A systematic review. Curr Nutr Rep. (2023) 12:465–77. doi: 10.1007/s13668-023-00481-0
28. Bhattacharya, SK, Goel, RK, Kaur, R, and Ghosal, S. Anti-stress activity of sitoindosides VII and VIII, new acylsterylglucosides from Withania somnifera. Phytother Res. (1987) 1:32–7. doi: 10.1002/ptr.2650010108
29. Orrù, A, Marchese, G, and Ruiu, S. Alkaloids in Withania somnifera (L.) Dunal root extract contribute to its anti-inflammatory activity. Pharmacology. (2023) 108:301–7. doi: 10.1159/000527656
30. Jayaprakasam, B, Strasburg, GA, and Nair, MG. Potent lipid peroxidation inhibitors from Withania somnifera fruits. Tetrahedron. (2004) 60:3109–21. doi: 10.1016/j.tet.2004.01.016
31. Gilbert, SF. Aging: the biology of senescence. In: Developmental biology, 6th edition. Sinauer Associates; (2000). Available at: https://www.ncbi.nlm.nih.gov/books/NBK10041/ (Accessed September 16, 2023)
32. Niccoli, T, and Partridge, L. Ageing as a risk factor for disease. Curr Biol. (2012) 22:R741–52. doi: 10.1016/j.cub.2012.07.024
33. McHugh, D, and Gil, J. Senescence and aging: causes, consequences, and therapeutic avenues. J Cell Biol. (2018) 217:65–77. doi: 10.1083/jcb.201708092
34. Colloca, G, Di Capua, B, Bellieni, A, Fusco, D, Ciciarello, F, Tagliaferri, L, et al. Biological and functional biomarkers of aging: definition, characteristics, and how they can impact everyday cancer treatment. Curr Oncol Rep. (2020) 22:115. doi: 10.1007/s11912-020-00977-w
35. Aging Biomarker ConsortiumBao, H, Cao, J, Chen, M, Chen, M, Chen, W, et al. Biomarkers of aging. Sci China Life Sci. (2023) 66:893–1066. doi: 10.1007/s11427-023-2305-0
36. Cai, Y, Song, W, Li, J, Jing, Y, Liang, C, Zhang, L, et al. The landscape of aging. Sci China Life Sci. (2022) 65:2354–454. doi: 10.1007/s11427-022-2161-3
37. Guo, J, Huang, X, Dou, L, Yan, M, Shen, T, Tang, W, et al. Aging and aging-related diseases: from molecular mechanisms to interventions and treatments. Signal Transduct Target Ther. (2022) 7:1–40. doi: 10.1038/s41392-022-01251-0
38. The nine hallmarks of aging: how do they affect your longevity and how can you reverse their effects? [Internet]. Available at: https://www.healthylongevity.clinic/blog/nine-hallmarks-of-aging (Accessed September 16, 2023)
39. Langie, SAS, Koppen, G, Desaulniers, D, Al-Mulla, F, Al-Temaimi, R, Amedei, A, et al. Causes of genome instability: the effect of low dose chemical exposures in modern society. Carcinogenesis. (2015) 36:S61–88. doi: 10.1093/carcin/bgv031
40. De Bont, R, and van Larebeke, N. Endogenous DNA damage in humans: a review of quantitative data. Mutagenesis. (2004) 19:169–85. doi: 10.1093/mutage/geh025
41. Ottens, F, Franz, A, and Hoppe, T. Build-UPS and break-downs: metabolism impacts on proteostasis and aging. Cell Death Differ. (2021) 28:505–21. doi: 10.1038/s41418-020-00682-y
42. Gobbini, E, Trovesi, C, Cassani, C, and Longhese, MP. Telomere uncapping at the crossroad between cell cycle arrest and carcinogenesis. Mol Cell Oncol. (2014) 1:e29901. doi: 10.4161/mco.29901
43. Armanios, M, Alder, JK, Parry, EM, Karim, B, Strong, MA, and Greider, CW. Short telomeres are sufficient to cause the degenerative defects associated with aging. Am J Hum Genet. (2009) 85:823–32. doi: 10.1016/j.ajhg.2009.10.028
44. Yao, Y, and Dai, W. Genomic instability and cancer. J Carcinog Mutagen. (2014) 5:1000165. doi: 10.1161/CIRCULATIONAHA.110.956839
45. Wang, K, Liu, H, Hu, Q, Wang, L, Liu, J, Zheng, Z, et al. Epigenetic regulation of aging: implications for interventions of aging and diseases. Signal Transduct Target Ther. (2022) 7:374. doi: 10.1038/s41392-022-01211-8
46. Díaz-Villanueva, JF, Díaz-Molina, R, and García-González, V. Protein folding and mechanisms of Proteostasis. Int J Mol Sci. (2015) 16:17193–230. doi: 10.3390/ijms160817193
47. Efeyan, A, Comb, WC, and Sabatini, DM. Nutrient sensing mechanisms and pathways. Nature. (2015) 517:302–10. doi: 10.1038/nature14190
48. Panwar, V, Singh, A, Bhatt, M, Tonk, RK, Azizov, S, Raza, AS, et al. Multifaceted role of mTOR (mammalian target of rapamycin) signaling pathway in human health and disease. Signal Transduct Target Ther. (2023) 8:1–25. doi: 10.1038/s41392-023-01608-z
49. Saxton, RA, and Sabatini, DM. mTOR signaling in growth, metabolism, and disease. Cell. (2017) 168:960–76. doi: 10.1016/j.cell.2017.02.004
50. Papadopoli, D, Boulay, K, Kazak, L, Pollak, M, Mallette, F, Topisirovic, I, et al. mTOR as a central regulator of lifespan and aging. F1000Res. (2019) 8:F1000 Faculty Rev-998. doi: 10.12688/f1000research.17196.1
51. Casanova, A, Wevers, A, Navarro-Ledesma, S, and Pruimboom, L. Mitochondria: it is all about energy. Front Physiol. (2023) 14:1114231. doi: 10.3389/fphys.2023.1114231
52. Sahin, E, and DePinho, RA. Axis of ageing: telomeres, p53 and mitochondria. Nat Rev Mol Cell Biol. (2012) 13:397–404. doi: 10.1038/nrm3352
53. Kumari, R, and Jat, P. Mechanisms of cellular senescence: cell cycle arrest and senescence associated secretory phenotype. Front Cell Dev Biol [Internet]. (2021) 9:645593. doi: 10.3389/fcell.2021.645593
54. Luo, X, Liu, Z, and Xu, R. Adult tissue-specific stem cell interaction: novel technologies and research advances. Front Cell Dev Biol [Internet]. (2023) 11:1220694. doi: 10.3389/fcell.2023.1220694
55. Behrens, A, van Deursen, JM, Rudolph, KL, and Schumacher, B. Impact of genomic damage and ageing on stem cell function. Nat Cell Biol. (2014) 16:201–7. doi: 10.1038/ncb2928
56. Zhang, G, Li, J, Purkayastha, S, Tang, Y, Zhang, H, Yin, Y, et al. Hypothalamic programming of systemic ageing involving IKK-β, NF-κB and GnRH. Nature. (2013) 497:211–6. doi: 10.1038/nature12143
57. Panda, A, Arjona, A, Sapey, E, Bai, F, Fikrig, E, Montgomery, RR, et al. Human innate immunosenescence: causes and consequences for immunity in old age. Trends Immunol. (2009) 30:325–33. doi: 10.1016/j.it.2009.05.004
58. Naylor, R, Baker, D, and van Deursen, J. Senescent cells: A novel therapeutic target for aging and age-related diseases. Clin Pharmacol Ther. (2013) 93:105–16. doi: 10.1038/clpt.2012.193
59. Di Leonardo, A, Linke, SP, Clarkin, K, and Wahl, GM. DNA damage triggers a prolonged p53-dependent G1 arrest and long-term induction of Cip1 in normal human fibroblasts. Genes Dev. (1994) 8:2540–51. doi: 10.1101/gad.8.21.2540
60. Kandhaya-Pillai, R, Miro-Mur, F, Alijotas-Reig, J, Tchkonia, T, Schwartz, S, Kirkland, JL, et al. Key elements of cellular senescence involve transcriptional repression of mitotic and DNA repair genes through the p53-p16/RB-E2F-DREAM complex. Aging. (2023) 15:4012–34. doi: 10.18632/aging.204743
61. Rossiello, F, Herbig, U, Longhese, MP, Fumagalli, M, and d’Adda di Fagagna, F. Irreparable telomeric DNA damage and persistent DDR signalling as a shared causative mechanism of cellular senescence and ageing. Curr Opin Genet Dev. (2014) 26:89–95. doi: 10.1016/j.gde.2014.06.009
62. Uxa, S, Bernhart, SH, Mages, CFS, Fischer, M, Kohler, R, Hoffmann, S, et al. DREAM and RB cooperate to induce gene repression and cell-cycle arrest in response to p53 activation. Nucleic Acids Res. (2019) 47:9087–103. doi: 10.1093/nar/gkz635
63. Crane, MM, Russell, AE, Schafer, BJ, Blue, BW, Whalen, R, Almazan, J, et al. DNA damage checkpoint activation impairs chromatin homeostasis and promotes mitotic catastrophe during aging. eLife. 8:e50778. doi: 10.7554/eLife.50778
64. Zhang, L, Pitcher, LE, Yousefzadeh, MJ, Niedernhofer, LJ, Robbins, PD, and Zhu, Y. Cellular senescence: a key therapeutic target in aging and diseases. J Clin Invest. 132:e158450. doi: 10.1172/JCI158450
65. Jeck, WR, Siebold, AP, and Sharpless, NE. Review: a meta-analysis of GWAS and age-associated diseases. Aging Cell. (2012) 11:727–31. doi: 10.1111/j.1474-9726.2012.00871.x
66. Fontana, R, Ranieri, M, La Mantia, G, and Vivo, M. Dual role of the alternative Reading frame ARF protein in Cancer. Biomol Ther. (2019) 9:87. doi: 10.3390/biom9030087
67. Pizzino, G, Irrera, N, Cucinotta, M, Pallio, G, Mannino, F, Arcoraci, V, et al. Oxidative stress: harms and benefits for human health. Oxidative Med Cell Longev. (2017) 2017:1–13. doi: 10.1155/2017/8416763
68. Cutler, RG. Oxidative stress and aging: catalase is a longevity determinant enzyme. Rejuvenation Res. (2005) 8:138–40. doi: 10.1089/rej.2005.8.138
69. Lobo, V, Patil, A, Phatak, A, and Chandra, N. Free radicals, antioxidants and functional foods: impact on human health. Pharmacogn Rev. (2010) 4:118–26. doi: 10.4103/0973-7847.70902
70. Pham-Huy, LA, He, H, and Pham-Huy, C. Free radicals, antioxidants in disease and health. Int J Biomed Sci. (2008) 4:89–96. doi: 10.59566/IJBS.2008.4089
71. Cabello-Verrugio, C, Simon, F, Trollet, C, and Santibañez, JF. Oxidative stress in disease and aging: mechanisms and therapies 2016. Oxidative Med Cell Longev. (2017) 2017:1–2. doi: 10.1155/2017/4310469
72. Newsholme, P, Gaudel, C, and Krause, M. Mitochondria and diabetes. An intriguing pathogenetic role. Adv Exp Med Biol [Internet]. (2012) 942:235–47. doi: 10.1007/978-94-007-2869-1_10
73. Hajam, YA, Rani, R, Ganie, SY, Sheikh, TA, Javaid, D, Qadri, SS, et al. Oxidative stress in human pathology and aging: molecular mechanisms and perspectives. Cells. (2022) 11:552. doi: 10.3390/cells11030552
74. Kirshenbaum, LA, and Singal, PK. Antioxidant changes in heart hypertrophy: significance during hypoxia-reoxygenation injury. Can J Physiol Pharmacol. (1992) 70:1330–5. doi: 10.1139/y92-186
75. Biala, AK, Dhingra, R, and Kirshenbaum, LA. Mitochondrial dynamics: orchestrating the journey to advanced age. J Mol Cell Cardiol. (2015) 83:37–43. doi: 10.1016/j.yjmcc.2015.04.015
76. Dobrowolski, P, Prejbisz, A, Kuryłowicz, A, Baska, A, Burchardt, P, Chlebus, K, et al. Metabolic syndrome – a new definition and management guidelines. Arch Med Sci AMS. (2022) 18:1133–56. doi: 10.5114/aoms/152921
77. Rask Larsen, J, Dima, L, Correll, CU, and Manu, P. The pharmacological management of metabolic syndrome. Expert Rev Clin Pharmacol. (2018) 11:397–410. doi: 10.1080/17512433.2018.1429910
78. Ren, J, Sowers, JR, and Zhang, Y. Metabolic stress, autophagy, and cardiovascular aging: from pathophysiology to therapeutics. Trends Endocrinol Metab. (2018) 29:699–711. doi: 10.1016/j.tem.2018.08.001
79. Wang, Z, Chen, J, Zhu, L, Jiao, S, Chen, Y, and Sun, Y. Metabolic disorders and risk of cardiovascular diseases: a two-sample mendelian randomization study. BMC Cardiovasc Disord. (2023) 23:529. doi: 10.1186/s12872-023-03567-3
80. Schafer, MJ, Miller, JD, and LeBrasseur, NK. Cellular senescence: implications for metabolic disease. Mol Cell Endocrinol. (2017) 455:93–102. doi: 10.1016/j.mce.2016.08.047
81. Kroemer, G. Autophagy: a druggable process that is deregulated in aging and human disease. J Clin Invest. (2015) 125:1–4. doi: 10.1172/JCI78652
82. Jiang, B, Zhou, X, Yang, T, Wang, L, Feng, L, Wang, Z, et al. The role of autophagy in cardiovascular disease: cross-interference of signaling pathways and underlying therapeutic targets. Front Cardiovasc Med [Internet]. (2023) 10:1088575. doi: 10.3389/fcvm.2023.1088575
83. Lever-van Milligen, BA, Verhoeven, JE, Schmaal, L, van Velzen, LS, Révész, D, Black, CN, et al. The impact of depression and anxiety treatment on biological aging and metabolic stress: study protocol of the MOod treatment with antidepressants or running (MOTAR) study. BMC Psychiatry. (2019) 19:425. doi: 10.1186/s12888-019-2404-0
84. Kim, YC, and Guan, KL. mTOR: a pharmacologic target for autophagy regulation. J Clin Invest. (2015) 125:25–32. doi: 10.1172/JCI73939
85. Chen, C, Liu, Y, Liu, Y, and Zheng, P. mTOR regulation and therapeutic rejuvenation of aging hematopoietic stem cells. Sci Signal. (2009) 2:ra75. doi: 10.1126/scisignal.2000559
86. Ahmad, M, and Dar, NJ. 8 – Withania somnifera: ethnobotany, pharmacology, and therapeutic functions In: D Bagchi, editor. Sustained energy for enhanced human functions and activity [Internet]. Cambridge, MA: Academic Press (2017). 137–54.
87. Deepesh, A, Meitei, KV, Raksha, M, and Ali, SA. Validation of harvesting period for obtaining optimum concentrations of withanolides from Withania somnifera at different phenological stages of plant. Indian J Trop Biodivers. (2009, 2009) 17:257–60. doi: 10.1016/B978-0-12-805413-0.00008-9
88. Mirjalili, MH, Moyano, E, Bonfill, M, Cusido, RM, and Palazón, J. Steroidal lactones from Withania somnifera, an ancient plant for novel medicine. Mol Basel Switz. (2009) 14:2373–93. doi: 10.3390/molecules14072373
89. Sharma, V, Sharma, S, Pracheta, P, and Paliwal, R. Withania somnifera: A rejuvenating ayurvedic medicinal herb for the treatment of various human ailments. Int J PharmTech Res. (2011) 3:974–4304.
90. Thirugnanasambantham, P, Roy, I, Charles, S, and Senthil, K. Ontogenetic assessment of withanolide biogenesis and expression of selected pathway genes in Withania somnifera, a traditional medicinal herb. J Pharm Res. (2014) 8:1344–51.
91. Bhat, WW, Lattoo, SK, Razdan, S, Dhar, N, Rana, S, Dhar, RS, et al. Molecular cloning, bacterial expression and promoter analysis of squalene synthase from Withania somnifera (L.). Dunal Gene. (2012) 499:25–36. doi: 10.1016/j.gene.2012.03.004
92. Kuzuyama, T, and Seto, H. Two distinct pathways for essential metabolic precursors for isoprenoid biosynthesis. Proc Jpn Acad Ser B Phys Biol Sci. (2012) 88:41–52. doi: 10.2183/pjab.88.41
93. Senthil, K, Jayakodi, M, Thirugnanasambantham, P, Lee, SC, Duraisamy, P, Purushotham, PM, et al. Transcriptome analysis reveals in vitro cultured Withania somnifera leaf and root tissues as a promising source for targeted withanolide biosynthesis. BMC Genomics. (2015) 16:14. doi: 10.1186/s12864-015-1214-0
94. Singh, P, Guleri, R, Singh, V, Kaur, G, Kataria, H, Singh, B, et al. Biotechnological interventions in Withania somnifera (L.) Dunal. Biotechnol Genet Eng Rev. (2015) 31:1–20. doi: 10.1080/02648725.2015.1020467
95. Friesen, JA, and Rodwell, VW. The 3-hydroxy-3-methylglutaryl coenzyme-A (HMG-CoA) reductases. Genome Biol. (2004) 5:248. doi: 10.1186/gb-2004-5-11-248
96. Dhar, N, Rana, S, Bhat, WW, Razdan, S, Pandith, SA, Khan, S, et al. Dynamics of withanolide biosynthesis in relation to temporal expression pattern of metabolic genes in Withania somnifera (L.) Dunal: a comparative study in two morpho-chemovariants. Mol Biol Rep. (2013) 40:7007–16. doi: 10.1007/s11033-013-2820-z
97. Dhar, MK, Koul, A, and Kaul, S. Farnesyl pyrophosphate synthase: a key enzyme in isoprenoid biosynthetic pathway and potential molecular target for drug development. New Biotechnol. (2013) 30:114–23. doi: 10.1016/j.nbt.2012.07.001
98. Agarwal, AV, Singh, D, Dhar, YV, Michael, R, Gupta, P, Chandra, D, et al. Virus-induced silencing of key genes leads to differential impact on withanolide biosynthesis in the medicinal plant, Withania somnifera. Plant Cell Physiol. (2018) 59:262–74. doi: 10.1093/pcp/pcx179
99. Soni, SK, Singh, R, Ngpoore, NK, Niranjan, A, Singh, P, Mishra, A, et al. Isolation and characterization of endophytic fungi having plant growth promotion traits that biosynthesizes bacosides and withanolides under in vitro conditions. Braz J Microbiol. (2021) 52:1791–805. doi: 10.1007/s42770-021-00586-0
100. Gupta, P, Goel, R, Pathak, S, Srivastava, A, Singh, SP, Sangwan, RS, et al. De novo assembly, functional annotation and comparative analysis of Withania somnifera leaf and root transcriptomes to identify putative genes involved in the withanolides biosynthesis. PLoS One. (2013) 8:e62714. doi: 10.1371/journal.pone.0062714
101. Sondag, GR, and Haqqi, TM. The role of microRNAs and their targets in osteoarthritis. Curr Rheumatol Rep. (2016) 18:56. doi: 10.1007/s11926-016-0604-x
102. Anderson, AS, and Loeser, RF. Why is osteoarthritis an age-related disease? Best Pract Res Clin Rheumatol. (2010) 24:15–26. doi: 10.1016/j.berh.2009.08.006
103. Chen, D, Shen, J, Zhao, W, Wang, T, Han, L, Hamilton, JL, et al. Osteoarthritis: toward a comprehensive understanding of pathological mechanism. Bone Res. (2017) 5:1–13. doi: 10.1038/boneres.2016.44
104. Fioravanti, A, Tinti, L, Pascarelli, NA, Di Capua, A, Lamboglia, A, Cappelli, A, et al. In vitro effects of VA441, a new selective cyclooxygenase-2 inhibitor, on human osteoarthritic chondrocytes exposed to IL-1β. J Pharmacol Sci. (2012) 120:6–14. doi: 10.1254/jphs.12016FP
105. Kim, JH, and Kim, SJ. Overexpression of MicroRNA-25 by withaferin A induces cyclooxygenase-2 expression in rabbit articular chondrocytes. J Pharmacol Sci. (2014) 125:83–90. doi: 10.1254/jphs.13232FP
106. Wang, S, Ge, L, Zhang, D, Wang, L, Liu, H, Ye, X, et al. MiR-181c-5p promotes inflammatory response during hypoxia/Reoxygenation injury by downregulating protein tyrosine phosphatase nonreceptor type 4 in H9C2 cardiomyocytes. Oxidative Med Cell Longev. (2020) 2020:1–13. doi: 10.1155/2020/7913418
107. Shuaib, M, Prajapati, KS, Gupta, S, and Kumar, S. Natural steroidal lactone induces G1/S phase cell cycle arrest and intrinsic apoptotic pathway by up-regulating tumor suppressive miRNA in triple-negative breast Cancer cells. Metabolites. (2022) 13:29. doi: 10.3390/metabo13010029
108. Sanada, F, Taniyama, Y, Muratsu, J, Otsu, R, Shimizu, H, Rakugi, H, et al. Source of chronic inflammation in aging. Front Cardiovasc Med. (2018) 5:12. doi: 10.3389/fcvm.2018.00012
109. Ferrucci, L, and Fabbri, E. Inflammageing: chronic inflammation in ageing, cardiovascular disease, and frailty. Nat Rev Cardiol. (2018) 15:505–22. doi: 10.1038/s41569-018-0064-2
110. Juráňová, J, Franková, J, and Ulrichová, J. The role of keratinocytes in inflammation. J Appl Biomed. (2017) 15:169–79. doi: 10.1016/j.jab.2017.05.003
111. Albanesi, C, Scarponi, C, Giustizieri, ML, and Girolomoni, G. Keratinocytes in inflammatory skin diseases. Curr Drug Targets Inflamm Allergy. (2005) 4:329–34. doi: 10.2174/1568010054022033
112. Sikandan, A, Shinomiya, T, and Nagahara, Y. Ashwagandha root extract exerts anti-inflammatory effects in HaCaT cells by inhibiting the MAPK/NF-κB pathways and by regulating cytokines. Int J Mol Med. (2018) 42:425–34. doi: 10.3892/ijmm.2018.3608
113. Kaur, T, and Kaur, G. Withania somnifera as a potential candidate to ameliorate high fat diet-induced anxiety and neuroinflammation. J Neuroinflammation. (2017) 14:201. doi: 10.1186/s12974-017-0975-6
114. Reddy, U, Jakhotia, S, Reddy, SS, and Reddy, G. Advanced glycation end products in brain during aging. Chem Biol Interact. (2022) 355:109840.
115. Rungratanawanich, W, Qu, Y, Wang, X, Essa, MM, and Song, BJ. Advanced glycation end products (AGEs) and other adducts in aging-related diseases and alcohol-mediated tissue injury. Exp Mol Med. (2021) 53:168–88. doi: 10.1038/s12276-021-00561-7
116. Sadigh-Eteghad, S, Sabermarouf, B, Majdi, A, Talebi, M, Farhoudi, M, and Mahmoudi, J. Amyloid-Beta: A crucial factor in Alzheimer’s disease. Med Princ Pract. (2015) 24:1–10. doi: 10.1159/000369101
117. Liang, R, Qi, X, Cai, Q, Niu, L, Huang, X, Zhang, D, et al. The role of NLRP3 inflammasome in aging and age-related diseases. Immun Ageing. (2024) 21:14. doi: 10.1186/s12979-023-00395-z
118. Santana, DA, De AC, SM, and Chen, ES. Histone modifications in Alzheimer’s disease. Genes. (2023) 14:347. doi: 10.3390/genes14020347
119. Atluri, VSR, Tiwari, S, Rodriguez, M, Kaushik, A, Yndart, A, Kolishetti, N, et al. Inhibition of amyloid-Beta production, associated Neuroinflammation, and histone deacetylase 2-mediated epigenetic modifications prevent neuropathology in Alzheimer’s disease in vitro model. Front Aging Neurosci [Internet]. (2020) 11:342. doi: 10.3389/fnagi.2019.00342
120. Budson, AE, and Price, BH. Memory dysfunction. N Engl J Med. (2005) 352:692–9. doi: 10.1056/NEJMra041071
121. Klinkenberg, I, and Blokland, A. The validity of scopolamine as a pharmacological model for cognitive impairment: a review of animal behavioral studies. Neurosci Biobehav Rev. (2010) 34:1307–50. doi: 10.1016/j.neubiorev.2010.04.001
122. Konar, A, Shah, N, Singh, R, Saxena, N, Kaul, SC, Wadhwa, R, et al. Protective role of Ashwagandha leaf extract and its component withanone on scopolamine-induced changes in the brain and brain-derived cells. PLoS One. (2011) 6:e27265. doi: 10.1371/journal.pone.0027265
123. Brown, MK, and Naidoo, N. The endoplasmic reticulum stress response in aging and age-related diseases. Front Physiol. (2012) 3:263.
124. Uddin, MS, Yu, WS, and Lim, LW. Exploring ER stress response in cellular aging and neuroinflammation in Alzheimer’s disease. Ageing Res Rev. (2021) 70:101417. doi: 10.1016/j.arr.2021.101417
125. Siwecka, N, Rozpędek-Kamińska, W, Wawrzynkiewicz, A, Pytel, D, Diehl, JA, and Majsterek, I. The structure, activation and signaling of IRE1 and its role in determining cell fate. Biomedicines. (2021) 9:156. doi: 10.3390/biomedicines9020156
126. Oyadomari, S, and Mori, M. Roles of CHOP/GADD153 in endoplasmic reticulum stress. Cell Death Differ. (2004) 11:381–9. doi: 10.1038/sj.cdd.4401373
127. Oyadomari, S, Araki, E, and Mori, M. Endoplasmic reticulum stress-mediated apoptosis in pancreatic β-cells. Apoptosis. (2002) 7:335–45. doi: 10.1023/A:1016175429877
128. Yamamoto, K, Yoshida, H, Kokame, K, Kaufman, RJ, and Mori, K. Differential contributions of ATF6 and XBP1 to the activation of endoplasmic reticulum stress-responsive cis-acting elements ERSE, UPRE and ERSE-II. J Biochem (Tokyo). (2004) 136:343–50. doi: 10.1093/jb/mvh122
129. Choi, MJ, Park, EJ, Min, KJ, Park, JW, and Kwon, TK. Endoplasmic reticulum stress mediates withaferin A-induced apoptosis in human renal carcinoma cells. Toxicol In Vitro. (2011) 25:692–8. doi: 10.1016/j.tiv.2011.01.010
130. Harding, HP, Calfon, M, Urano, F, Novoa, I, and Ron, D. Transcriptional and translational control in the mammalian unfolded protein response. Annu Rev Cell Dev Biol. (2002) 18:575–99. doi: 10.1146/annurev.cellbio.18.011402.160624
131. Panossian, A. Understanding adaptogenic activity: specificity of the pharmacological action of adaptogens and other phytochemicals. Ann N Y Acad Sci. (2017) 1401:49–64. doi: 10.1111/nyas.13399
132. Haigis, MC, and Yankner, BA. The aging stress response. Mol Cell. (2010) 40:333–44. doi: 10.1016/j.molcel.2010.10.002
133. Panossian, A, Seo, EJ, and Efferth, T. Novel molecular mechanisms for the adaptogenic effects of herbal extracts on isolated brain cells using systems biology. Phytomedicine. (2018) 50:257–84. doi: 10.1016/j.phymed.2018.09.204
134. Barth, E, Srivastava, A, Wengerodt, D, Stojiljkovic, M, Axer, H, Witte, OW, et al. Age-dependent expression changes of circadian system-related genes reveal a potentially conserved link to aging. Aging. (2021) 13:25694–716. doi: 10.18632/aging.203788
135. Mohawk, JA, Green, CB, and Takahashi, JS. Central and peripheral circadian clocks in mammals. Annu Rev Neurosci. (2012) 35:445–62. doi: 10.1146/annurev-neuro-060909-153128
136. Jagota, A. Age-induced alterations in biological clock: therapeutic effects of melatonin In: MK Thakur and R SIS, editors. Brain aging and therapeutic interventions [internet]. Dordrecht: Springer Netherlands (2012). 111–29.
137. Benito, J, Zheng, H, Ng, FS, and Hardin, PE. Transcriptional feedback loop regulation, function and ontogeny in Drosophila. Cold Spring Harb Symp Quant Biol. (2007) 72:437–44. doi: 10.1101/sqb.2007.72.009
138. Takahashi, JS. Transcriptional architecture of the mammalian circadian clock. Nat Rev Genet. (2017) 18:164–79. doi: 10.1038/nrg.2016.150
139. Kukkemane, K, and Jagota, A. Therapeutic effects of hydro-alcoholic leaf extract of Withania somnifera on age-induced changes in daily rhythms of Sirt1, Nrf2 and rev-erbα in the SCN of male Wistar rats. Biogerontology. (2020) 21:593–607. doi: 10.1007/s10522-020-09875-x
140. Ghafouri-Fard, S, Abak, A, Talebi, SF, Shoorei, H, Branicki, W, Taheri, M, et al. Role of miRNA and lncRNAs in organ fibrosis and aging. Biomed Pharmacother Biomedecine Pharmacother. (2021) 143:112132. doi: 10.1016/j.biopha.2021.112132
141. Gu, J, Chen, C, Wang, J, Chen, T, Yao, W, Yan, T, et al. Withaferin A exerts preventive effect on liver fibrosis through oxidative stress inhibition in a Sirtuin 3-dependent manner. Oxidative Med Cell Longev. (2020) 2020:1–17. doi: 10.1155/2020/2452848
142. Wang, Y, Li, C, Gu, J, Chen, C, Duanmu, J, Miao, J, et al. Celastrol exerts anti-inflammatory effect in liver fibrosis via activation of AMPK-SIRT3 signalling. J Cell Mol Med. (2020) 24:941–53. doi: 10.1111/jcmm.14805
143. Wang, X, Bonventre, JV, and Parrish, AR. The aging kidney: increased susceptibility to nephrotoxicity. Int J Mol Sci. (2014) 15:15358–76. doi: 10.3390/ijms150915358
144. White, S, Lin, L, and Hu, K. NF-κB and tPA signaling in kidney and other diseases. Cells. (2020) 9:1348. doi: 10.3390/cells9061348
145. Lin, H, Hou, CC, Cheng, CF, Chiu, TH, Hsu, YH, Sue, YM, et al. Peroxisomal proliferator-activated receptor-alpha protects renal tubular cells from doxorubicin-induced apoptosis. Mol Pharmacol. (2007) 72:1238–45. doi: 10.1124/mol.107.037523
146. Esteban, V, Lorenzo, O, Rupérez, M, Suzuki, Y, Mezzano, S, Blanco, J, et al. Angiotensin II, via AT1 and AT2 receptors and NF-kappaB pathway, regulates the inflammatory response in unilateral ureteral obstruction. J Am Soc Nephrol JASN. (2004) 15:1514–29. doi: 10.1097/01.ASN.0000130564.75008.F5
147. Lv, W, Booz, GW, Wang, Y, Fan, F, and Roman, RJ. Inflammation and renal fibrosis: recent developments on key signaling molecules as potential therapeutic targets. Eur J Pharmacol. (2018) 820:65–76. doi: 10.1016/j.ejphar.2017.12.016
148. Grunz-Borgmann, E, Mossine, V, Fritsche, K, and Parrish, AR. Ashwagandha attenuates TNF-α- and LPS-induced NF-κB activation and CCL2 and CCL5 gene expression in NRK-52E cells. BMC Complement Altern Med. (2015) 15:434. doi: 10.1186/s12906-015-0958-z
149. Yoon, E, Babar, A, Choudhary, M, Kutner, M, and Pyrsopoulos, N. Acetaminophen-induced hepatotoxicity: a comprehensive update. J Clin Transl Hepatol. (2016) 4:131–42. doi: 10.14218/JCTH.2015.00052
150. Gerriets, V, Anderson, J, Patel, P, and Nappe, TM. Acetaminophen. In: StatPearls [internet]. Treasure Island (FL): StatPearls Publishing; (2024). Available at: http://www.ncbi.nlm.nih.gov/books/NBK482369/ (Accessed April 9, 2024)
151. Larson, AM, Polson, J, Fontana, RJ, Davern, TJ, Lalani, E, Hynan, LS, et al. Acetaminophen-induced acute liver failure: results of a United States multicenter, prospective study. Hepatol Baltim Md. (2005) 42:1364–72. doi: 10.1002/hep.20948
152. Devkar, ST, Kandhare, AD, Zanwar, AA, Jagtap, SD, Katyare, SS, Bodhankar, SL, et al. Hepatoprotective effect of withanolide-rich fraction in acetaminophen-intoxicated rat: decisive role of TNF-α, IL-1β, COX-II and iNOS. Pharm Biol. (2016) 54:2394–403. doi: 10.3109/13880209.2016.1157193
153. Panda, V, Deshmukh, A, Hare, A, Singh, S, Hingorani, L, and Sudhamani, S. Effect of Withania somnifera hydroalcoholic extract and other dietary interventions in improving muscle strength in aging rats. J Ayurveda Integr Med. (2021) 12:623–32. doi: 10.1016/j.jaim.2021.06.001
154. Volpi, E, Nazemi, R, and Fujita, S. Muscle tissue changes with aging. Curr Opin Clin Nutr Metab Care. (2004) 7:405–10. doi: 10.1097/01.mco.0000134362.76653.b2
155. Hardwick, JM, and Soane, L. Multiple functions of BCL-2 family proteins. Cold Spring Harb Perspect Biol. (2013) 5:a008722. doi: 10.1101/cshperspect.a008722
156. Qian, S, Wei, Z, Yang, W, Huang, J, Yang, Y, and Wang, J. The role of BCL-2 family proteins in regulating apoptosis and cancer therapy. Front Oncol. (2022) 12:985363. doi: 10.3389/fonc.2022.985363
157. Blackburn, EH. Switching and signaling at the telomere. Cell. (2001) 106:661–73. doi: 10.1016/S0092-8674(01)00492-5
158. Flores, I, Benetti, R, and Blasco, MA. Telomerase regulation and stem cell behaviour. Curr Opin Cell Biol. (2006) 18:254–60. doi: 10.1016/j.ceb.2006.03.003
159. Shammas, MA. Telomeres, lifestyle, cancer, and aging. Curr Opin Clin Nutr Metab Care. (2011) 14:28–34. doi: 10.1097/MCO.0b013e32834121b1
160. Sharma, R, and Martins, N. Telomeres, DNA damage and ageing: potential leads from Ayurvedic Rasayana (anti-ageing) drugs. J Clin Med. (2020) 9:2544. doi: 10.3390/jcm9082544
161. Akhoon, BA, Pandey, S, Tiwari, S, and Pandey, R. Withanolide A offers neuroprotection, ameliorates stress resistance and prolongs the life expectancy of Caenorhabditis elegans. Exp Gerontol. (2016) 78:47–56. doi: 10.1016/j.exger.2016.03.004
162. Chin, T, Lee, XE, Ng, PY, Lee, Y, and Dreesen, O. The role of cellular senescence in skin aging and age-related skin pathologies. Front Physiol. (2023) 14:1297637. doi: 10.3389/fphys.2023.1297637
163. Ogrodnik, M, Zhu, Y, Langhi, LGP, Tchkonia, T, Krüger, P, Fielder, E, et al. Obesity-induced cellular senescence drives anxiety and impairs neurogenesis. Cell Metab. (2019) 29:1061–1077.e8. doi: 10.1016/j.cmet.2018.12.008
164. Abu Bakar, MH, Azmi, MN, Shariff, KA, and Tan, JS. Withaferin A protects against high-fat diet-induced obesity via attenuation of oxidative stress, inflammation, and insulin resistance. Appl Biochem Biotechnol. (2019) 188:241–59. doi: 10.1007/s12010-018-2920-2
165. Xiao, P, Zhang, Y, Zeng, Y, Yang, D, Mo, J, Zheng, Z, et al. Impaired angiogenesis in ageing: the central role of the extracellular matrix. J Transl Med. (2023) 21:457. doi: 10.1186/s12967-023-04315-z
166. Adair, TH, and Montani, JP. Overview of angiogenesis. In: Angiogenesis [Internet]. San Rafael, CA: Morgan & Claypool Life Sciences; (2010). Available at: https://www.ncbi.nlm.nih.gov/books/NBK53238/ (Accessed April 9, 2024)
167. Zetter, BR. Angiogenesis and tumor metastasis. Annu Rev Med. (1998) 49:407–24. doi: 10.1146/annurev.med.49.1.407
168. Lugano, R, Ramachandran, M, and Dimberg, A. Tumor angiogenesis: causes, consequences, challenges and opportunities. Cell Mol Life Sci. (2020) 77:1745–70. doi: 10.1007/s00018-019-03351-7
169. Milanini, J, Viñals, F, Pouysségur, J, and Pagès, G. p42/p44 MAP kinase module plays a key role in the transcriptional regulation of the vascular endothelial growth factor gene in fibroblasts*. J Biol Chem. (1998) 273:18165–72. doi: 10.1074/jbc.273.29.18165
170. Santhekadur, P, and Puttananjaiah, S. Withaferin A suppresses the expression of vascular endothelial growth factor in Ehrlich ascites tumor cells via Sp1 transcription factor. Curr Trends Biotechnol Pharm. (2009) 3:138–48.
171. Duffy, AM, Bouchier-Hayes, DJ, and Harmey, JH. Vascular endothelial growth factor (VEGF) and its role in non-endothelial cells: autocrine signalling by VEGF. In: Madame Curie bioscience database [Internet]. Austin, TX: Landes Bioscience; (2013). Available at: https://www.ncbi.nlm.nih.gov/books/NBK6482/ (Accessed April 9, 2024)
172. Yao, JC, Wang, L, Wei, D, Gong, W, Hassan, M, Wu, TT, et al. Association between expression of transcription factor Sp1 and increased vascular endothelial growth factor expression, advanced stage, and poor survival in patients with resected gastric cancer. Clin Cancer Res. (2004) 10:4109–17. doi: 10.1158/1078-0432.CCR-03-0628
173. Sajida,, and Prabhu, A. Anti-angiogenic, apoptotic and matrix metalloproteinase inhibitory activity of Withania somnifera (Ashwagandha) on lung adenocarcinoma cells. Phytomedicine Int J Phytother Phytopharm. (2021) 90:153639. doi: 10.1016/j.phymed.2021.153639
174. Panossian, A, Seo, EJ, and Efferth, T. Effects of anti-inflammatory and adaptogenic herbal extracts on gene expression of eicosanoids signaling pathways in isolated brain cells. Phytomedicine. (2019) 60:152881. doi: 10.1016/j.phymed.2019.152881
175. Hofmann, P, Sommer, J, Theodorou, K, Kirchhof, L, Fischer, A, Li, Y, et al. Long non-coding RNA H19 regulates endothelial cell aging via inhibition of STAT3 signalling. Cardiovasc Res. (2019) 115:230–42. doi: 10.1093/cvr/cvy206
176. Home | ClinicalTrials.gov [Internet]. Available at: https://www.clinicaltrials.gov/ (Accessed December 22, 2023)
177. Clinical Trials Register [Internet]. Available at: https://www.clinicaltrialsregister.eu/ctr-search/trial/2021-001654-65/DK (Accessed November 16, 2023)
178. 1 nova mensagem [Internet]. Available at: https://ensaiosclinicos.gov.br/#menu (Accessed December 23, 2023)
179. Albert Einstein College of Medicine. Metformin in Longevity Study (MILES) [Internet]. clinicaltrials.gov; (2021). Report No.: NCT02432287. Available at: https://clinicaltrials.gov/study/NCT02432287 (Accessed January 1, 2023)
180. Mazzotti, DR. Genetic and physiological aspects of oxidative profile in sleep and well-succeed aging [Internet]. clinicaltrials.gov; (2015). Report No.: NCT01480037. Available at: https://clinicaltrials.gov/study/NCT01480037 (Accessed January 1, 2023)
181. Clinica Universidad de Navarra, Universidad de Navarra. Dysfunction of adipose tissue in obesity, inflammation and aging: mechanisms and effects of physical exercise and omega-3 fatty acids [Internet]. clinicaltrials.gov; (2020). Report No.: NCT03300388. Available at: https://clinicaltrials.gov/study/NCT03300388 (Accessed January 1, 2023)
182. Königstein, K. Vascular effects of non-linear periodized exercise training in sedentary adults with elevated cardiovascular risk – The VascuFit Project [Internet]. clinicaltrials.gov; (2022). Report No.: NCT05235958. Available at: https://clinicaltrials.gov/study/NCT05235958 (Accessed January 1, 2023)
183. Banchereau, J. Genomics and epigenomics of the elderly response to pneumococcal vaccines [Internet]. clinicaltrials.gov; (2021). Report No.: NCT03104075. Available at: https://clinicaltrials.gov/study/NCT03104075 (Accessed January 1, 2023)
184. University of California, Davis. The psychobiological effects of a one-month insight meditation retreat, a sub-study of The Shamatha Project: a longitudinal, randomized waitlist control study of cognitive, emotional, and neural effects of intensive meditation training [Internet]. clinicaltrials.gov; (2017). Report No.: NCT03056105. Available at: https://clinicaltrials.gov/study/NCT03056105 (Accessed January 1, 2023)
185. Johns Hopkins University. Effect of orally delivered phytochemicals, alone, and in combination, on aging and inflammation-related effects in the skin [Internet]. clinicaltrials.gov; (2020). Report No.: NCT03289832. Available at: https://clinicaltrials.gov/study/NCT03289832 (Accessed January 1, 2023)
186. MAZA MPDL. Calorie restriction retards the aging process by inducing sirtuins and uncoupling protein 3, thereby reducing the production of reactive oxygen species [Internet]. clinicaltrials.gov; (2012). Report No.: NCT01508091. Available at: https://clinicaltrials.gov/study/NCT01508091 (Accessed January 1, 2023)
187. Nuver, J. Longitudinal assessment of therapy-related early ageing in adolescent and young adult (AYA) cancer patients [Internet]. clinicaltrials.gov; (2023). Report No.: NCT05062707. Available at: https://clinicaltrials.gov/study/NCT05062707 (Accessed January 1, 2023)
188. Amazentis, SA. A randomized, double-blind, placebo-controlled study to investigate the efficacy of AMAZ-02 on muscle function in otherwise healthy middle-aged, overweight and inactive individuals [Internet]. clinicaltrials.gov; (2022). Report No.: NCT03464500. Available at: https://clinicaltrials.gov/study/NCT03464500 (Accessed January 1, 2023)
189. University of Florida. The effect of intermittent fasting on adaptive oxidative stress response and mitochondrial biogenesis [Internet]. clinicaltrials.gov; (2015). Report No.: NCT02132091. Available at: https://clinicaltrials.gov/study/NCT02132091 (Accessed January 1, 2023)
190. Murphy, K. The impact of HIV on accelerated aging in the female genital tract: a pilot trial of topical estradiol to improve the vaginal microbiome and symptoms of vaginal atrophy in menopausal women with HIV [Internet]. clinicaltrials.gov; (2023). Report No.: NCT04079218. Available at: https://clinicaltrials.gov/study/NCT04079218 (Accessed January 1, 2023)
191. Pierre Fabre Dermo Cosmetique. Exploratory study to assess clinical and biological anti-aging efficacy of a cosmetic cream in women after 2 months of daily use, under dermatological control [Internet]. clinicaltrials.gov; (2023). Report No.: NCT05895591. Available at: https://clinicaltrials.gov/study/NCT05895591 (Accessed January 1, 2023)
192. Quadram Institute Bioscience. A longitudinal study in a cohort aged 60 years and older to obtain mechanistic knowledge of the role of the gut microbiome during Normal healthy ageing in order to develop strategies that will improve lifelong health and wellbeing [Internet]. clinicaltrialsgov; (2022). Report No.: NCT04199195. Available at: https://clinicaltrials.gov/study/NCT04199195 (Accessed January 1, 2023)
193. University of Copenhagen. Slow age: a randomized, controlled clinical trial of interventions to slow aging in humans [Internet]. clinicaltrials.gov; (2023). Report No.: NCT05593939. Available at: https://clinicaltrials.gov/study/NCT05593939 (Accessed January 1, 2023)
194. King’s College London. Mechanistic insight into ovarian granulosa cell function based on age and cause of infertility [internet]. clinicaltrials.gov; (2021). Report No.: NCT04945265. Available at: https://clinicaltrialsgov/study/NCT04945265 (Accessed January 1, 2023)
195. Lytle, KA. Adipose tissue blood flow in aging humans [Internet]. clinicaltrials.gov; (2023). Report No.: NCT06096532. Available at: https://clinicaltrials.gov/study/NCT06096532 (Accessed January 1, 2023)
196. Barzilai, N. Study of changes in muscle and fat gene transcription with acarbose treatment: a crossover study [Internet]. clinicaltrials.gov; (2022). Report No.: NCT02953093. Available at: https://clinicaltrials.gov/study/NCT02953093 (Accessed January 1, 2023)
197. Chang, A. Discovery of effects of retinol on human skin aging in individuals of east asian descent [Internet]. clinicaltrials.gov; (2019). Report No.: NCT02906566. Available at: https://clinicaltrials.gov/study/NCT02906566 (Accessed January 1, 2023)
198. University of California, San Francisco. The role of inflammation and aging in HIV-associated cardiovascular risk [Internet]. clinicaltrials.gov; (2015). Report No.: NCT01333644. Available at: https://clinicaltrials.gov/study/NCT01333644 (Accessed January 1, 2023)
199. University of Southampton. A pilot study of dietary taxifolin/dihydroquercetin and ergothioneine and immune biomarkers in healthy volunteers [Internet]. clinicaltrials.gov; (2023). Report No.: NCT05190432. Available at: https://clinicaltrials.gov/study/NCT05190432 (Accessed January 1, 2023)
200. Comenius University. Active aging: effect of life-long endurance exercises on the circadian regulation of physiological processes and their maintenance in aging [Internet]. clinicaltrials.gov; (2021). Report No.: NCT05053282. Available at: https://clinicaltrials.gov/study/NCT05053282 (Accessed January 1, 2023)
201. Tarnopolsky, M. Nutraceutical supplementation for male subfertility [Internet]. clinicaltrials.gov; (2023). Report No.: NCT06091969. Available at: https://clinicaltrials.gov/study/NCT06091969 (Accessed January 1, 2023)
202. Universitaire Ziekenhuizen KU Leuven. Trial in elderly with musculoskeletal problems due to underlying sarcopenia – faeces to unravel gut and inflammation translationally [Internet]. clinicaltrials.gov; (2023). Report No.: NCT05008770. Available at: https://clinicaltrials.gov/study/NCT05008770 (Accessed January 1, 2023)
203. Gresele, P. Role of inflammation in the pathogenesis of frailty in the elderly: studies on the contribution of blood platelets – PIPAF platelets in the pathogenesis of ageing associated frailty [Internet]. clinicaltrials.gov; (2023). Report No.: NCT05798637. Available at: https://clinicaltrials.gov/study/NCT05798637 (Accessed January 1, 2023)
204. Salonia, A. Definition of a personalized signature of chronic inflammation and early aging predictive of the development of comorbidities in infertile men [Internet]. clinicaltrials.gov; (2023). Report No.: NCT06082362. Available at: https://clinicaltrials.gov/study/NCT06082362 (Accessed January 1, 2023)
205. VA Office of Research and Development. Impacts of nicotinamide riboside on functional capacity and muscle physiology in older veterans [Internet]. clinicaltrials.gov; (2023). Report No.: NCT04691986. Available at: https://clinicaltrials.gov/study/NCT04691986 (Accessed January 1, 2023)
206. EudraCT Number 2015-002682-30 – Clinical trial results – EU Clinical Trials Register [Internet]. Available at: https://www.clinicaltrialsregister.eu/ctr-search/trial/2015-002682-30/results (Accessed November 16, 2023)
207. REBEC [Internet]. Available at: https://ensaiosclinicos.gov.br/rg/RBR-85kywk3 (Accessed November 16, 2023)
208. Martínez-Mármol, R, Giordano-Santini, R, Kaulich, E, Cho, AN, Przybyla, M, Riyadh, MA, et al. SARS-CoV-2 infection and viral fusogens cause neuronal and glial fusion that compromises neuronal activity. Sci Adv. (2023) 9:eadg2248. doi: 10.1126/sciadv.adg2248
209. Mortberg, MA, Gentile, JE, Nadaf, NM, Vanderburg, C, Simmons, S, Dubinsky, D, et al. A single-cell map of antisense oligonucleotide activity in the brain. Nucleic Acids Res. (2023) 51:7109–24. doi: 10.1093/nar/gkad371
210. Yu, J, Pandey, SK, Khatri, H, Prakash, TP, Swayze, EE, and Seth, PP. Synthesis and antisense properties of 2’-O-(2S-methoxypropyl)-RNA-modified gapmer antisense oligonucleotides. ChemMedChem. (2014) 9:2040–4. doi: 10.1002/cmdc.201402099
211. Litke, JL, and Jaffrey, SR. Highly efficient expression of circular RNA aptamers in cells using autocatalytic transcripts. Nat Biotechnol. (2019) 37:667–75. doi: 10.1038/s41587-019-0090-6
212. Li, H, Peng, K, Yang, K, Ma, W, Qi, S, Yu, X, et al. Circular RNA cancer vaccines drive immunity in hard-to-treat malignancies. Theranostics. (2022) 12:6422–36. doi: 10.7150/thno.77350
213. Janas, MM, Schlegel, MK, Harbison, CE, Yilmaz, VO, Jiang, Y, Parmar, R, et al. Selection of GalNAc-conjugated siRNAs with limited off-target-driven rat hepatotoxicity. Nat Commun. (2018) 9:723. doi: 10.1038/s41467-018-02989-4
214. Humphreys, SC, Davis, JA, Iqbal, S, Kamel, A, Kulmatycki, K, Lao, Y, et al. Considerations and recommendations for assessment of plasma protein binding and drug-drug interactions for siRNA therapeutics. Nucleic Acids Res. (2022) 50:6020–37. doi: 10.1093/nar/gkac456
215. Zou, Y, Sun, X, Yang, Q, Zheng, M, Shimoni, O, Ruan, W, et al. Blood-brain barrier-penetrating single CRISPR-Cas9 nanocapsules for effective and safe glioblastoma gene therapy. Sci Adv. (2022) 8:eabm8011. doi: 10.1126/sciadv.abm8011
216. Parayath, NN, Stephan, SB, Koehne, AL, Nelson, PS, and Stephan, MT. In vitro-transcribed antigen receptor mRNA nanocarriers for transient expression in circulating T cells in vivo. Nat Commun. (2020) 11:6080. doi: 10.1038/s41467-020-19486-2
217. Liu, JQ, Zhang, C, Zhang, X, Yan, J, Zeng, C, Talebian, F, et al. Intratumoral delivery of IL-12 and IL-27 mRNA using lipid nanoparticles for cancer immunotherapy. J Control Release. (2022) 345:306–13. doi: 10.1016/j.jconrel.2022.03.021
Keywords: nutraceuticals, aging, inflammation, Withania somnifera, Ashwagandha, RNA, noncoding RNA, transcription
Citation: Saha P, Ajgaonkar S, Maniar D, Sahare S, Mehta D and Nair S (2024) Current insights into transcriptional role(s) for the nutraceutical Withania somnifera in inflammation and aging. Front. Nutr. 11:1370951. doi: 10.3389/fnut.2024.1370951
Edited by:
Mahendra P. Singh, Deen Dayal Upadhyay Gorakhpur University, IndiaReviewed by:
Laxmi Rathor, University of Florida, United StatesSimran Kauts, Lovely Professional University, India
Copyright © 2024 Saha, Ajgaonkar, Maniar, Sahare, Mehta and Nair. This is an open-access article distributed under the terms of the Creative Commons Attribution License (CC BY). The use, distribution or reproduction in other forums is permitted, provided the original author(s) and the copyright owner(s) are credited and that the original publication in this journal is cited, in accordance with accepted academic practice. No use, distribution or reproduction is permitted which does not comply with these terms.
*Correspondence: Sujit Nair, sujit108@gmail.com