- 1Plastic Surgery Department, Fujian Children’s Hospital, Fuzhou, China
- 2Fujian Maternity and Child Health Hospital, Affiliated Hospital of Fujian Medical University, Fuzhou, China
- 3Developmental and Behavior Pediatrics Department, Fujian Children’s Hospital, Fuzhou, China
- 4Child Healthcare Department, Fuzhou Maternal and Child Health Hospital, Fuzhou, China
Background: Tourette syndrome (TS) is associated with immunological dysfunction. The DA system is closely related to TS development, or behavioral stereotypes. Previous evidence suggested that hyper-M1-polarized microglia may exist in the brains of TS individuals. However, the role of microglia in TS and their interaction with dopaminergic neurons is unclear. In this study, we applied iminodipropionitrile (IDPN) to establish a TS model and focused on the inflammatory injury in the striatal microglia-dopaminergic-neuron crosstalk.
Methods: Male Sprague–Dawley rats were intraperitoneally injected with IDPN for seven consecutive days. Stereotypic behavior was observed to verify the TS model. Striatal microglia activation was evaluated based on different markers and expressions of inflammatory factors. The striatal dopaminergic neurons were purified and co-cultured with different microglia groups, and dopamine-associated markers were assessed.
Results: First, there was pathological damage to striatal dopaminergic neurons in TS rats, as indicated by decreased expression of TH, DAT, and PITX3. Next, the TS group showed a trend of increased Iba-1 positive cells and elevated levels of inflammatory factors TNF-α and IL-6, as well as an enhanced M1-polarization marker (iNOS) and an attenuated M2-polarization marker (Arg-1). Finally, in the co-culture experiment, IL-4-treated microglia could upregulate the expression of TH, DAT, and PITX3 in striatal dopaminergic neurons vs LPS-treated microglia. Similarly, the TS group (microglia from TS rats) caused a decreased expression of TH, DAT, and PITX3 compared with the Sham group (microglia from control rats) in the dopaminergic neurons.
Conclusion: In the striatum of TS rats, microglia activation is M1 hyperpolarized, which transmits inflammatory injury to striatal dopaminergic neurons and disrupts normal dopamine signaling.
1 Introduction
Tourette syndrome (TS) is a childhood-onset developmental neurological disease characterized by motor and vocal behavioral stereotypes (1). The estimated pooled prevalence rate of TS is 0.53%, with a male predominance (2–4). About 1% of school-age children are affected by TS, and boys are approximately four times more likely to develop TS than girls (5, 6). The main affected brain regions include the basal ganglia and the related corticostriatal-thalamocortical (CSTC) circuit (7) or the substantia nigra-striatum network. In this circuit, it is widely accepted that dopamine (DA) is the main excitatory neurotransmitter, and the DA system, including the important factor dopamine transporter (DAT), can affect locomotion behavior, and theoretically, it plays an important role in the pathophysiology of TS (8). Besides DA, TS associated abnormalities in neurotransmission include glutamate (Glu) and gamma-aminobutyric acid (GABA) (9, 10).
Microglia-mediated immune overactivation is an important cause of central nervous system injury. Normally, under the condition of microglia, they can polarize into either the M1 (pro-inflammatory type) or M2 (anti-inflammatory type) phenotype in response to different micro-environmental disturbances (11). M1 polarization has a variety of biological functions but often damages adjacent neurons through inflammatory cytokines and the corresponding neurotoxicity (12–15). Previous evidence has suggested that hyper-M1-polarized microglia may exist in the brains of TS individuals (16–19). However, there is a lack of direct evidence to reveal the role of microglia in TS, as well as its downstream interaction with dopaminergic neurons (considering the DA system is closely related to TS development or behavioral stereotypes) (20–25).
Iminodipropionitrile (IDPN) is a synthetic organic nitrile, and it is the most commonly used inducer of TS, which has a long-term effect (19). We have used this model to observe changes in different systems and tissues (26). Peripherally, IDPN triggers immune dysfunction through impairment of mature Th cells, especially the Treg subset. In this study, we probed the role of striatal microglia-dopaminergic-neuron crosstalk in a rat model of TS induced by IDPN. For the first time, we confirmed that neuroinflammation triggered by M1 polarization of microglia can cause significant injuries to dopaminergic neurons in the striatum of TS rats.
2 Materials and methods
2.1 TS model
Male Sprague–Dawley rats (6–8 weeks, weight 200 g) were used for TS model establishment. The TS group was intraperitoneally injected with iminodipropionitrile (IDPN, 300 mg/kg/day, Sigma-Aldrich, USA) for seven consecutive days, and the control group was intraperitoneally injected with saline (5 ml/kg/day) for 7 days. The TS model was verified by stereotypical behaviors. If the rats had a stereotypic score lower than 1, the sample was removed.
After behavioral observation, each rat was sacrificed, and the striatal tissue was isolated. A part of the striatal tissues was fixed in 4% paraformaldehyde, the remaining tissues were homogenized, and protein was extracted for ELISA and Western blot assays. Another batch of animals was used for the purification of microglia, and these microglia were named the Sham group or TS group in the cell experiment.
The above animal experiments were approved by the ethics committee of Fujian Maternity and Child Health Hospital.
2.2 Co-culture of striatal dopaminergic neurons and microglia
First, a primary culture of dopaminergic neurons was performed. The ventral midbrain region containing the striatum was dissected and washed three times with Hank’s balanced salt solution containing 10 mM HEPES and 20 mM glucose. The tissue was mechanically dissociated at room temperature and suspended in Neurobasal medium (Gibco, Invitrogen, Carlsbad, CA, USA) containing 2 mM glutamine and B27 supplement (Gibco, Invitrogen). Cells were seeded on poly-D-lysine/laminin-coated plates (105 cells/well) and cultured in Neurobasal medium (Gibco, Invitrogen, Carlsbad, CA, USA) containing 2 mM glutamine and 2% B27 supplement (Gibco, Invitrogen) at 37°C in a humidified 5% CO2 atmosphere. Half of the medium was changed every 2 days until treatment. Co-culture was performed after 7 days of seeding. For each well, an equal amount of microglia (105 cells) from different groups was added; after 48 h, the microglia were washed out slightly, and the adherent dopaminergic neurons were collected for the assay. The added microglia were divided into the following groups: For different types of stimulation, the LPS group (of microglia) was added LPS (1 μg/ml), and the IL-4 group was added IL-4 (20 μg/ml), and a 24-hour treatment was allowed before microglia collection. Next, two groups of microglia were added to the pool of dopaminergic neurons. In the other batch, an equal amount of microglia from the TS or control rats (namely, the TS group or Sham group) was added.
2.3 Immunohistochemical and immunofluorescence staining
For IHC staining, the tissues were embedded in paraffin and cut into 5 mm sections. Sections were mounted on slides. The sections of striatum were subjected to antigen, followed by antibody hybridization (the primary antibody was the rabbit anti-Iba-1 antibody, and the secondary antibody was the mouse anti-rabbit antibody). Targets were visualized by 3,3-diaminobenzidine. For immunofluorescence staining, tissues were fixed in 4% paraformaldehyde overnight, dehydrated in 20% sucrose (0.1 M PBS) for 24 h at 4°C, and further dehydrated in 30% sucrose (0.1 M PBS) for 24 h at 4°C. The sections were cut into 15-μm sections on a cryostat. The sections were rinsed in 0.01 M PBS and blocked for 2 h with donkey serum (in 0.3% Tween-20 and 0.01 M PBS) and then incubated with the primary antibodies at 4°C overnight (1:500). Subsequently, sections were washed three times in 0.01 M PBS for 5 min and incubated with several conjugates with FITC (1:200) or CY3 (1:200). Next, sections were incubated with DAPI for nucleus staining for 15 min and washed three times for 5 min each. Finally, sections were cover-slipped, and images were captured under a fluorescence microscope. For cellular immunofluorescence, cells were fixed with 4% PFA for 30 min, washed with 0.01 M PBS, permeabilized with 0.1% Triton X-100 for 5 min, and blocked with goat serum for 1 h. Labeling was performed by incubating cells for 1 h with specific rabbit antibodies (including CD86, Arg-1, TH, DAT, and PITX3). After three washes (using PBS), cells were incubated with the specific secondary antibody (Alexa594-conjugated goat anti-rabbit antibody, Life Technologies). Cells were washed three times with PBS and incubated in DAPI for 5 min. Samples were observed under a fluorescence microscope.
2.4 ELISA assay
Rat striatal tissues were homogenized in a lysis buffer with protein inhibitors and PMSF (1 mM). The lysates were centrifuged at 1,000 rpm for 5 min, and the supernatant was stored at 2 to 8°C. The above samples were used to detect the concentration of inflammatory factors (TNF-α, IL-6, and IL-10), and microglia polarization markers (iNOS or Arg-1), by ELISA. All ELISA operations followed the official instructions. Briefly, 50 μl of the sample was added to each well, and the standard curve was plotted based on the corresponding OD values. The concentration of each inflammatory factor was calculated according to the curve equation.
2.5 Western blotting
The tissues or cells were lysed using an ice-cold lysis buffer (50 mM Tris, pH 7.4, 150 mM NaCl, 1% SDS, 1 mM EDTA, 1% NP-40) containing 1 mM protein inhibitor and 1 mM PMSF. The lysates were centrifuged at 10,000×g at 4°C for 10 min, and the supernatants were collected. Protein concentration was measured using the BCA protein assay. Equal amounts of protein were separated using 10% SDS-PAGE before being transferred to PVDF membranes. Membranes were incubated with primary mouse antibodies or anti-GAPDH antibodies (Santa Cruz Biotechnology, Santa Cruz, CA, USA). The blotting was developed using HRP-conjugated goat anti-mouse IgG (Santa Cruz Biotechnology) and detected by an ECL kit (Amersham, Piscataway, NJ, USA). The applied primary antibodies were as follows: rabbit anti-TH (1:500), rabbit anti-DAT (1:500), rabbit anti-PITX3 (1:250), rabbit anti-iNOS (1:500), and rabbit anti β-actin (1:10,000).
2.6 Statistical analysis
Results were expressed as means ± standard error. For comparison between two groups, Student’s t-test was used after the normal distribution test; for groups with unequal variance, Welch’s t-test was used for comparison. For more than two groups, one-way ANOVA was used; nonparametric tests were used when data were not normally distributed. Additionally, Bonferroni correction was used to control family-wise error rates. A P value <0.05 was considered statistically significant.
3 Results
3.1 Pathological damage to striatal dopaminergic neurons in TS rats
First, the TS model was validated with respect to stereotypical behavior induced by IDPN. The IDPN-treated rats had significantly more counts of biting, head twitching, shaking claws, and continuous rotation, and the stereotypic behavior score was significantly increased. The detailed behavioral changes have been reported in our previous work (27). To assess the damage to striatal dopaminergic neurons, three associated markers were used: tyrosine hydroxylase (TH, the classic marker of striatal dopaminergic neurons), dopamine transporter (DAT, an important marker in the presynaptic membrane in dopaminergic neurons), and PITX3 [involved in the production, maintenance, and survival of dopaminergic neurons, as well as an important and specific transcription factor in the development of midbrain dopaminergic neurons (28)]. We found that the expression of TH, DAT, and PITX3 were significantly decreased in the striatum of TS rats (assessed by Western blotting, Figures 1A, B), which suggests that IDPN injection can induce pathological damage to striatal dopaminergic neurons.
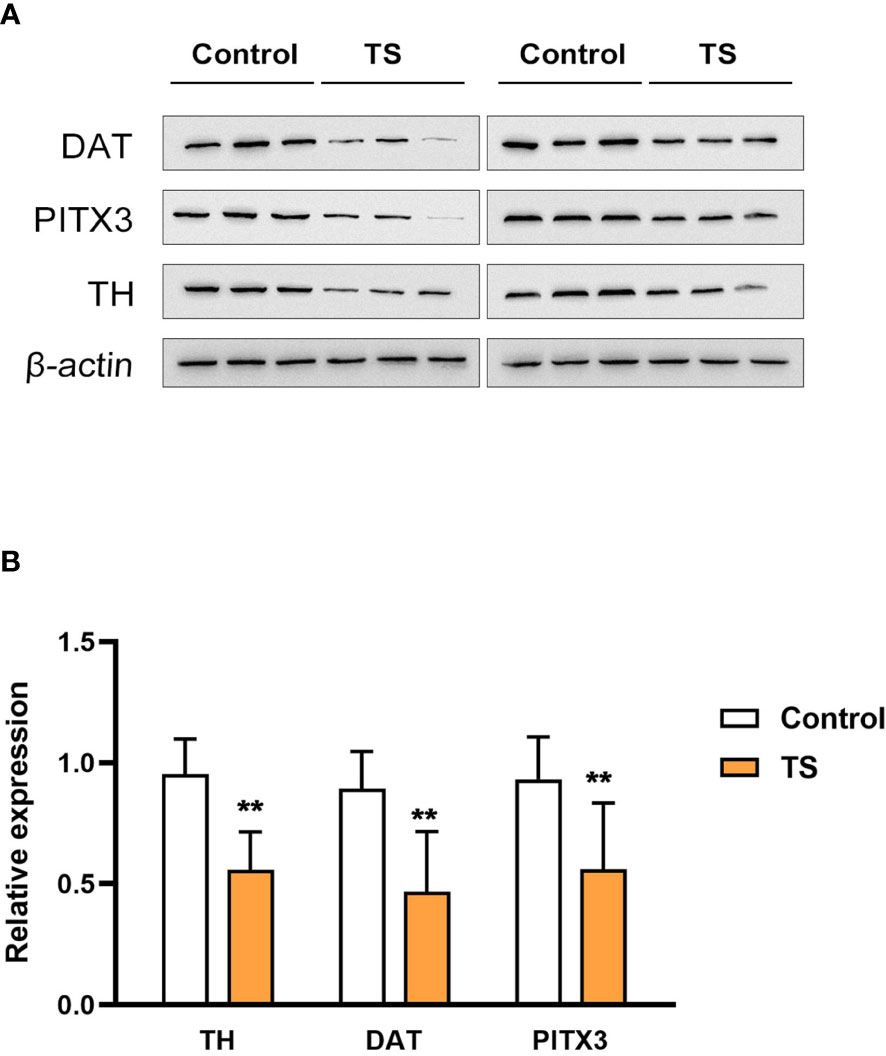
Figure 1 The expression of tyrosine hydroxylase (TH), dopamine transporter (DAT), and PITX3 is significantly decreased in the striatum of the Tourette syndrome (TS) rats. (A) The blots of TH, DAT, and PITX3. (B) The statistical analysis of the protein expression. **P <0.01.
3.2 M1 over-polarization of striatal microglia is involved in TS
A possible mechanism of TS-related injuries in striatal dopaminergic neurons may be the activation (especially polarization) of striatal microglia. First, the TS group showed a trend of increased Iba-1 positive cells with asymmetrical branches and an amoeba-like appearance (Figure 2A). Meanwhile, ELISA assays showed that the levels of inflammatory factors TNF-α and IL-6 were significantly increased (P <0.01, Figure 2B), and intriguingly, the anti-inflammatory factor IL-10 level was also increased (P <0.01, Figure 2B) in the striatum of TS individuals. In addition, iNOS (the M1-polarization marker) expression was elevated (P <0.01; Figure 2B) and Arg-1 (the M2-polarization marker) expression was decreased (P <0.01; Figure 2B) in the striatum. Taken together, there is a M1 over-polarization of microglia in the striatum of TS individuals, and the associated inflammatory signaling may be involved in the central mechanism of TS development.
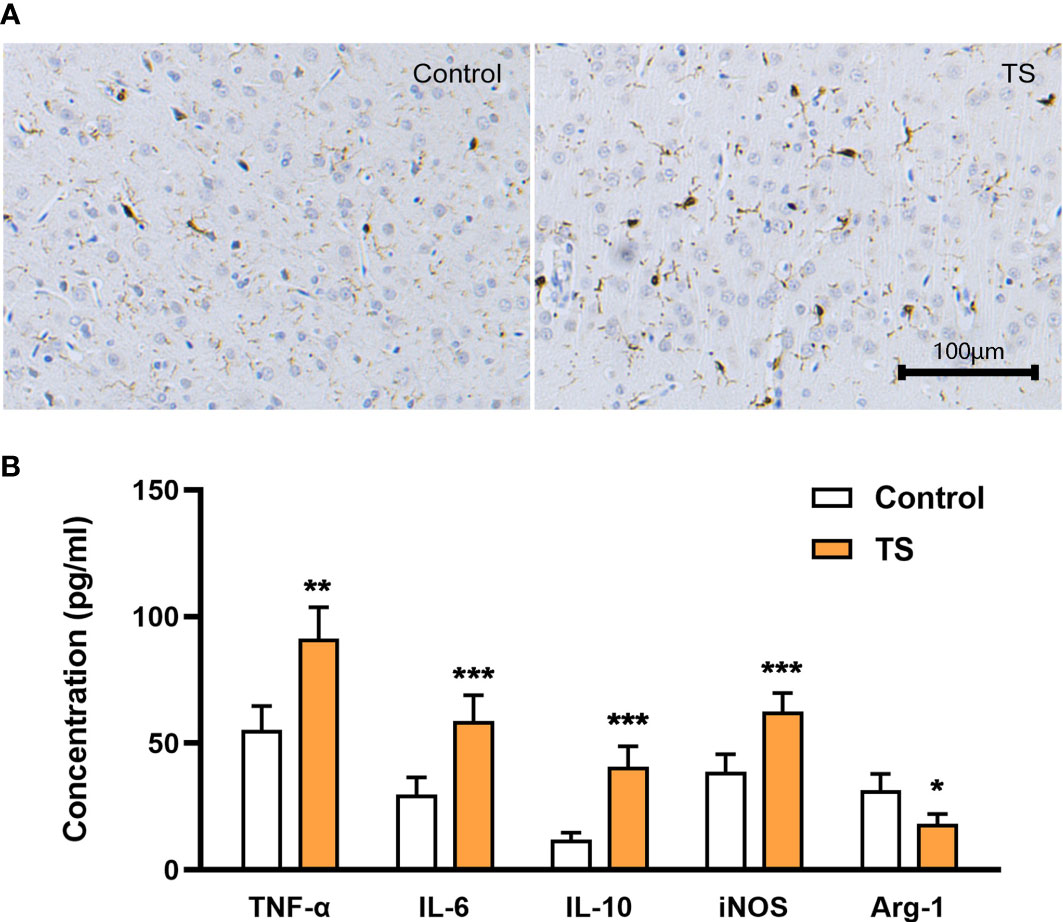
Figure 2 Activation of striatal microglia is involved in TS. (A) The TS group has a trend toward increased Iba-1 positive cells, with asymmetrical branches and an amoeba-like appearance. (B) The levels of inflammatory factors TNF-α, IL-6, IL-10, and iNOS are significantly increased in the striatum of TS individuals, while the M2 marker Arg-1 is decreased. *P <0.05, **P <0.01, ***P <0.001.
3.3 The possible microglia-neuron crosstalk in TS
Based on the M1 over-polarization of microglia, it is reasonable to assume that the inflammatory crosstalk between microglia and neurons may be an important pathogenic mechanism for TS-related injuries. Therefore, we isolated microglia from two groups of animals. After 4 days of purification culture, the morphology of microglia (Figure 3A) in two groups was as follows: most of the cells in Sham group had small, narrow, and long cytosol, few amoeboid like cells; while the morphology of microglia in TS group was diverse, many cells had asymmetric branches, and most of them were amoeboid like cells. Next, we stimulated normal microglia using LPS (towards M1 phenotype) and IL-4 (towards M2 phenotype), and the striatal dopaminergic neurons were co-cultured with different groups of microglia. As expected, LPS treatment effectively induced a M1 polarization of microglia (verified by the marker CD86 and increased iNOS expression, Figures 3B, D), and IL-4 triggered a M2 polarization (verified by the marker Arg-1 and increased CD206 expression, Figures 3C, D). Subsequently, striatal dopaminergic neurons were cultured in vitro (Figure 4A), and they were co-cultured with microglia of different polarization status for 48 h. The expression of TH, DAT, and PITX3 was determined after co-culture. The IL-4 treated microglia induced higher levels of TH, DAT, and PITX3 in neurons, as observed by immunofluorescence staining (Figures 4B-D) and Western blotting (Figure 4E). Similarly, striatal dopaminergic neurons were co-cultured with microglia from different rats (the Sham group or TS group), and the TS-group microglia (vs the Sham-group) induced a decreased expression of TH, DAT, and PITX3 in the dopaminergic neurons (Figures 4B-E). These findings, at least partially, indicate that the decreased TH, DAT, and PITX3 may be caused by the crosstalk with the M1-type microglia in the TS individual.
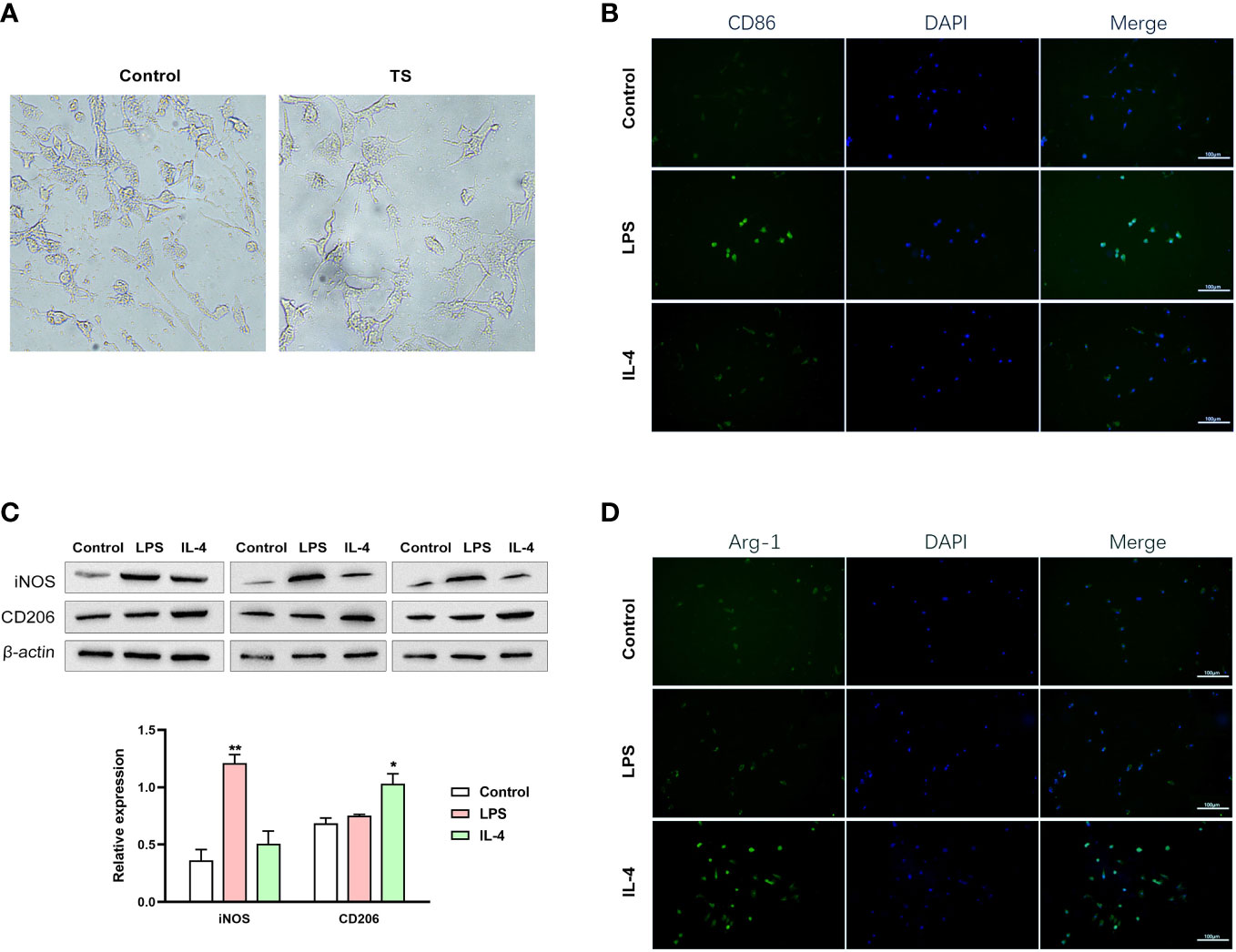
Figure 3 Different groups of microglia. (A) The morphology of microglia in two groups. (B–D) Normal microglia are treated using lipopolysaccharide (LPS) (towards the M1 phenotype) or IL-4 (towards the M2 phenotype). (B) LPS treatment effectively induced a M1 polarization of microglia (verified by the IF of the marker CD86). (C) IL-4 triggered a M2 polarization (verified by the IF of the marker Arg-1). (D) Western blot analysis of iNOS and CD206 expression in two treatment groups. *P <0.05, **P <0.01.
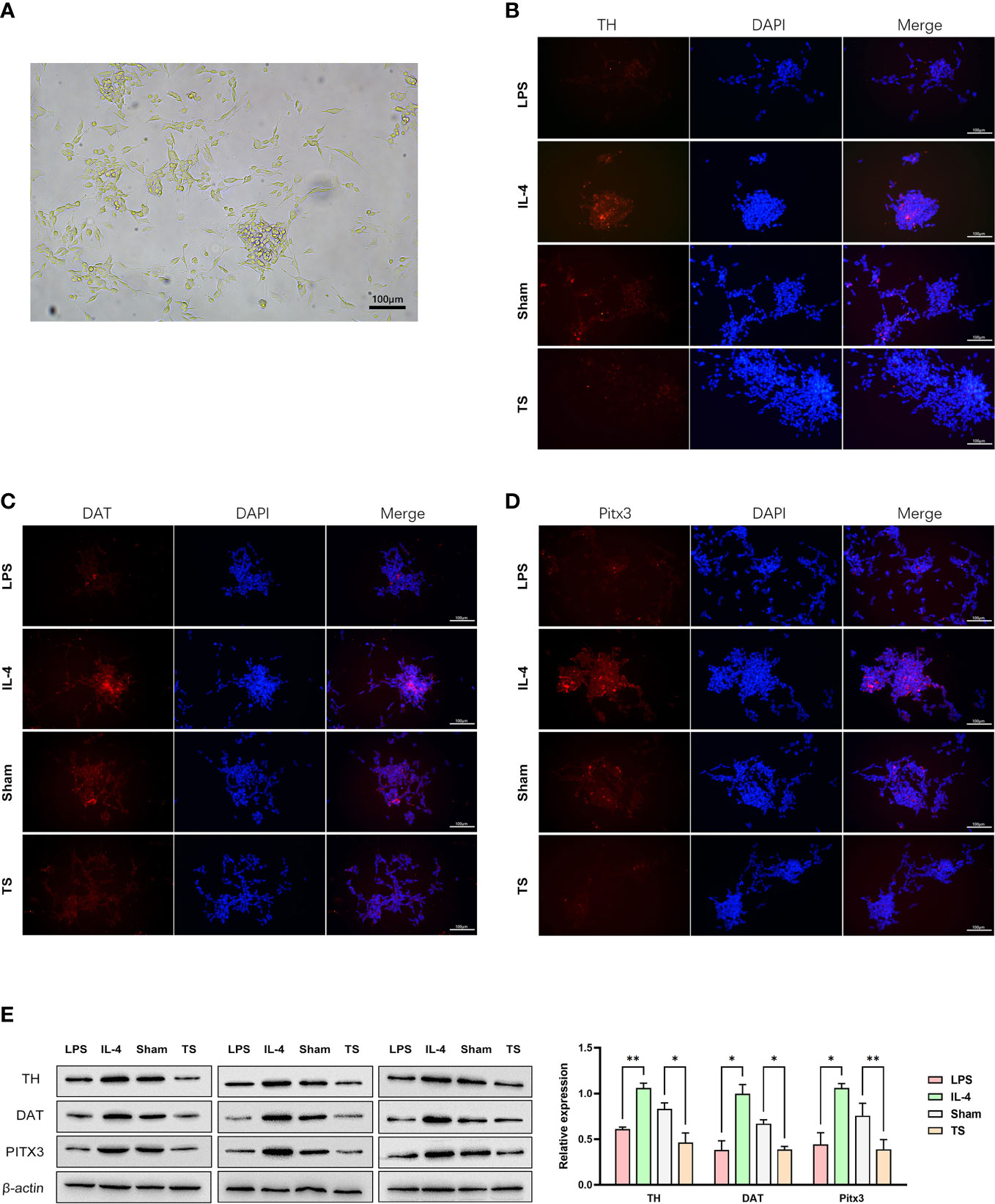
Figure 4 Striatal dopaminergic neurons were co-cultured with microglia of different polarization statuses. (A) Striatal dopaminergic neurons are cultured. (B) Immunofluorescence staining of TH expression in different groups: co-cultured with LPS-treated microglia, IL-4 treated microglia, control-animal-derived striatal microglia, and TS-rat-derived microglia. (C) Immunofluorescence staining of DAT expression in different groups. (D) Immunofluorescence staining of PITX3 expression in different groups. (E) Western blot analysis of the expression of TH, DAT, and PITX3 in different co-culture groups. *P <0.05, **P <0.01.
4 Discussion
In this study, we observed inflammatory injury to striatal dopaminergic neurons in TS rats. We also observed an increased M1 polarization and decreased M2 polarization of the striatal microglia and the interaction between microglia and dopaminergic neurons, which may transmit the inflammatory injury. Based on these findings, we tentatively propose a hypothesis for the pathogenesis of TS (as shown in Figure 5): in the striatum of TS rats, microglia are hyperactivated, with excessive M1 polarization and overexpression of inflammatory factors that cause sustained neurotoxicity to striatal dopaminergic neurons, which drive the development of stereotypical behaviors.
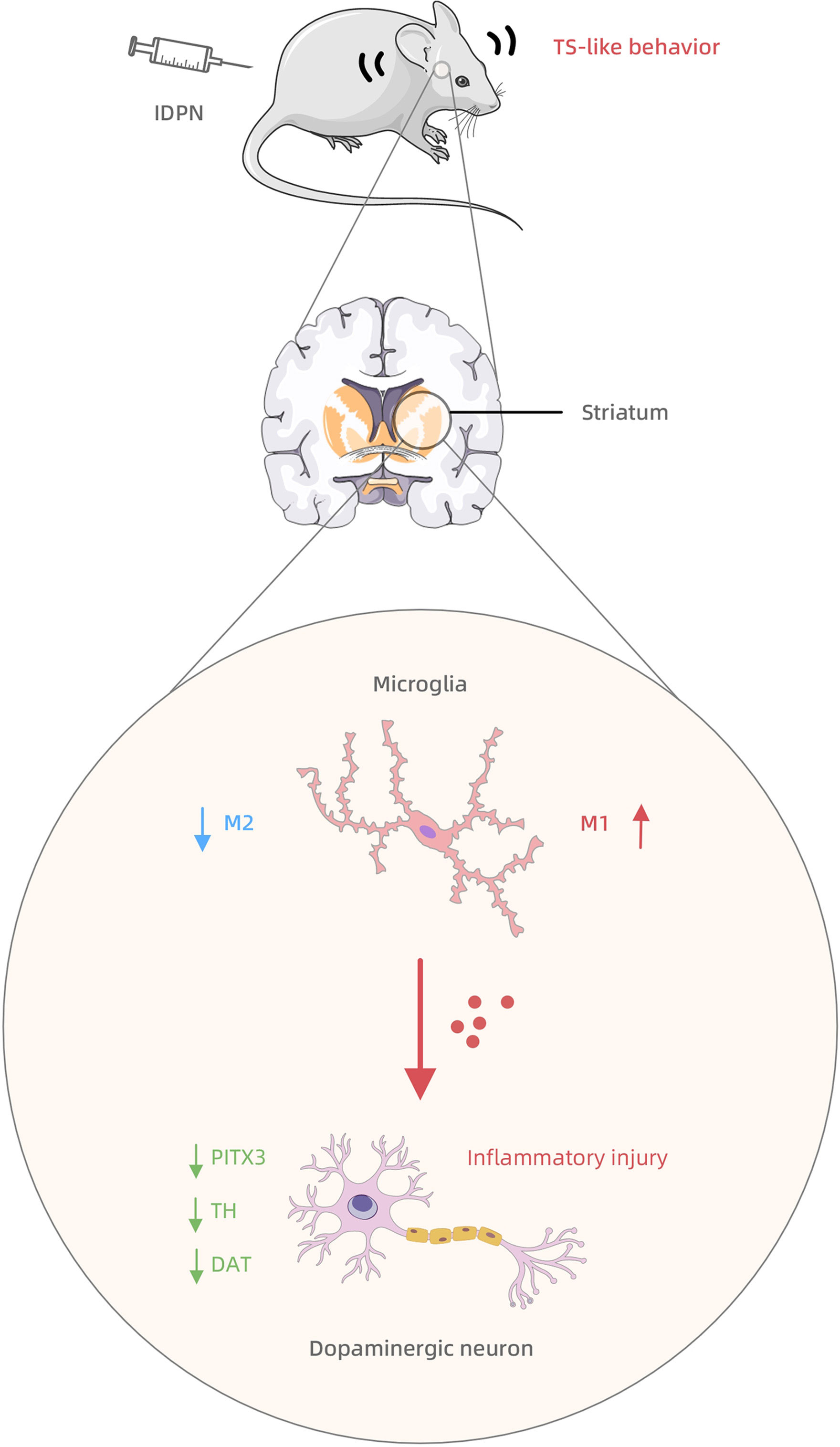
Figure 5 The mechanism summary diagram of IDPN-induced TS development involving the striatal microglia-dopaminergic-neuron crosstalk.
Neuroinflammation plays a crucial role in the pathophysiology of neural disorders. Microglia activation is a major event following central nervous system inflammation. In particular, M1 polarization has been regarded as the biological basis for many abnormal behaviors and neuropsychiatric disorders, especially those associated with neuroinflammation (29–33). The imbalanced M1/M2 polarization can cause neurological disorders in all possibilities (34, 35), and many scholars were attempting to answer why microglia kill neurons after neural disorders (36). Increasing studies have reported that the neuroinflammation caused by microglia in the striatum mediates various central diseases, such as Parkinson’s disease (37), schizophrenia (38), Alzheimer’s disease (39, 40), and other negative affective conditions (41); and the potential molecular mechanisms include IL-13 (42), IL-6 (41), TREM2 (39), BIN1 (39), and toll-like receptors (36). As far as we know, only a few studies have shown that the neuroimmune response mediated by microglia may be involved in TS (16–19) and the associated molecular mechanism include L-6, TNF-α, CD45, IFN-γ, histamine, etc. (43–49). We here highlight the role of microglia polarization in TS development and confirm the increased M1 type and decreased M2 type, which have not been fully reported previously [instead, known studies have mainly focused on T cells (50)].
Further, we here innovatively discover that striatal dopaminergic neurons are the key cell targets of microglia in TS. This is in line with previous conclusions. The striatal dopaminergic neurons are vulnerable to neurotoxicity (including different products of microglia) (51, 52), and their functions are involved in Parkinson’s disease and Huntington’s chorea (53, 54). Robust dopaminergic neuron function helps maintain a healthy microenvironment in the brain. For example, the cerebral dopamine neurotrophic factor possesses immune-modulatory properties that benefit brain diseases (37). Furthermore, many studies have indicated that stereotypic behavior is associated with the dopaminergic system (55–58). Moreover, the striatum is indeed one of the most important brain regions associated with the pathogenesis of TS (59). DAT plays a role in many neurodevelopmental diseases, e.g., altered DA availability mediated through DAT may affect autistic traits in autism spectrum disorders (60–62). The loss of DAT affects the reuptake of DA and causes an accumulation of DA in the synaptic cleft, which triggers an exorbitant DA signal and drives pathological stereotypic behavior. The current study further discovers that decreased DAT, as well as TH and PITX3, may be the result of inflammatory injuries delivered by microglia. The deficiency in tyrosine hydroxylase (TH) function is closely linked to neurodevelopmental behavioral disorders (63). The decreased TH expression (STX1A+/TH+ cells) in the striatum of TS individuals has also been observed in our previous article (27), which is a clear indicator of dopaminergic neuron injury. PITX3 is a homeodomain-containing transcription factor belonging to the pituitary homeodomain family. It is involved in the production, maintenance, and survival of dopaminergic neurons. The PITX3 deficiency can lead to the loss of the substantia nigra striatum path, the deprivation of dopaminergic neurons in the substantia nigra, and the impairment of dopaminergic development, which may drive the development of Parkinson’s disease (64). In a TH-Cre/Pitx3-fl/fl (Pitx3cKO) mouse model, it was noticed that Pitx3 deficiency promotes age-dependent alterations in striatal medium spiny neurons (65). Although many studies have shown the link between PITX3 and Parkinson’s disease, the clear association with TS is largely unknown. This work is the first to show a decreased striatal PITX3 expression in TS rats, which suggests a potential role for PITX3 in the pathogenesis of TS.
Still, the present study has some limitations. First, some of the findings of this study are inconsistent with previous studies, and the exact reasons for these inconsistencies are unclear. For example, we noticed that the IL-4 treatment is beneficial for dopaminergic neurons in comparison with LPS. IL-4, a well-known anti-inflammatory cytokine, is expressed in microglia in the brain. It can regulate the polarization of the peripheral macrophage phenotype and inhibit the production of inflammatory mediators, such as interleukin-1β and TNF-a (66–69). However, it has been reported that IL-4 expressed in LPS-activated microglia contributes to striatal neurodegeneration, in which M1/M2 polarization is implicated, and the neutralizing antibody for IL-4 can protect striatal neurons against LPS-induced neurotoxicity in vivo (70). Also, we noticed increased IL-10 expression in the striatum of individual TS, despite its well-known anti-inflammatory factor. A possible reason is that IL-10 can be produced by other neural cells (e.g., Treg cells) to modulate microglia to the M2 phenotype (71, 72). However, the above speculations lack direct evidence.
In conclusion, microglia activation is M1 hyperpolarized, which transmits inflammatory injury to striatal dopaminergic neurons and disrupts normal dopamine signaling in the striatum of TS rats.
Data availability statement
The raw data supporting the conclusions of this article will be made available by the authors, without undue reservation.
Ethics statement
The animal study was reviewed and approved by The ethics committee of Fujian Maternity and Child Health Hospital, Affiliated Hospital of Fujian Medical University.
Author contributions
XW and XL made substantial contributions to the design of the present study. XW, LC, and XZ collected and investigated the data. The data analysis was performed by XW and XL. XW, LC, and XZ drafted the work. XL critically revised the manuscript for important intellectual content. All authors contributed to the article and approved the submitted version.
Funding
This study was funded by the National Natural Science Foundation of China (no. 81871076), the Natural Science Foundation of Fujian Province (no. 2021J01424), and the Fujian Children’s Hospital Excellent Talent Training Plan (YCXY202103 and YCXZ202203).
Conflict of interest
The authors declare that the research was conducted in the absence of any commercial or financial relationships that could be construed as a potential conflict of interest.
Publisher’s note
All claims expressed in this article are solely those of the authors and do not necessarily represent those of their affiliated organizations, or those of the publisher, the editors and the reviewers. Any product that may be evaluated in this article, or claim that may be made by its manufacturer, is not guaranteed or endorsed by the publisher.
References
1. Hsu CJ, Wong LC, Lee WT. Immunological dysfunction in tourette syndrome and related disorders. Int J Mol Sci (2021), 22. doi: 10.3390/ijms22020853
2. Scharf JM, Miller LL, Gauvin CA, Alabiso J, Mathews CA, Ben-Shlomo Y. Population prevalence of tourette syndrome: a systematic review and meta-analysis. Mov Disord (2015) 30:221–8. doi: 10.1002/mds.26089
3. Greene DJ, Schlaggar BL, Black KJ. Neuroimaging in tourette syndrome: research highlights from 2014-2015. Curr Dev Disord Rep (2015) 2:300–08. doi: 10.1007/s40474-015-0062-6
4. Ganos C, Martino D. Tics and tourette syndrome. Neurol Clin (2015) 33:115–36. doi: 10.1016/j.ncl.2014.09.008
5. Robertson MM. The prevalence and epidemiology of gilles de la tourette syndrome. part 1: the epidemiological and prevalence studies. J Psychosom Res (2008) 65:461–72. doi: 10.1016/j.jpsychores.2008.03.006
6. Conte G, Valente F, Fioriello F, Cardona F. Rage attacks in tourette syndrome and chronic tic disorder: a systematic review. Neurosci Biobehav Rev (2020) 119:21–36. doi: 10.1016/j.neubiorev.2020.09.019
7. Müller-Vahl KR, Grosskreutz J, Prell T, Kaufmann J, Bodammer N, Peschel T. Tics are caused by alterations in prefrontal areas, thalamus and putamen, while changes in the cingulate gyrus reflect secondary compensatory mechanisms. BMC Neurosci (2014) 15:6. doi: 10.1186/1471-2202-15-6
8. Buse J, Schoenefeld K, Münchau A, Roessner V. Neuromodulation in tourette syndrome: dopamine and beyond. Neurosci Biobehav Rev (2013) 37:1069–84. doi: 10.1016/j.neubiorev.2012.10.004
9. Ramamoorthi K, Lin Y. The contribution of GABAergic dysfunction to neurodevelopmental disorders. Trends Mol Med (2011) 17:452–62. doi: 10.1016/j.molmed.2011.03.003
10. Singer HS, Morris C, Grados M. Glutamatergic modulatory therapy for tourette syndrome. Med Hypotheses (2010) 74:862–7. doi: 10.1016/j.mehy.2009.11.028
11. Guo S, Wang H, Yin Y. Microglia polarization from M1 to M2 in neurodegenerative diseases. Front Aging Neurosci (2022) 14:815347. doi: 10.3389/fnagi.2022.815347
12. Colonna M, Butovsky O. Microglia function in the central nervous system during health and neurodegeneration. Annu Rev Immunol (2017) 35:441–68. doi: 10.1146/annurev-immunol-051116-052358
13. Jha MK, Lee WH, Suk K. Functional polarization of neuroglia: implications in neuroinflammation and neurological disorders. Biochem Pharmacol (2016) 103:1–16. doi: 10.1016/j.bcp.2015.11.003
14. Wang Q, Yao H, Liu W, Ya B, Cheng H. Xing z et al. microglia polarization in alzheimer's disease: mechanisms and a potential therapeutic target. Front Aging Neurosci (2021) 13:772717. doi: 10.3389/fnagi.2021.772717
15. Jiang CT, Wu WF, Deng YH, Ge JW. Modulators of microglia activation and polarization in ischemic stroke (Review). Mol Med Rep (2020) 21:2006–18. doi: 10.3892/mmr.2020.11003
16. Martino D, Zis P, Buttiglione M. The role of immune mechanisms in tourette syndrome. Brain Res (2015) 1617:126–43. doi: 10.1016/j.brainres.2014.04.027
17. Frick L, Pittenger C. Microglial dysregulation in OCD, tourette syndrome, and PANDAS. J Immunol Res (2016) 2016:8606057. doi: 10.1155/2016/8606057
18. Lennington JB, Coppola G, Kataoka-Sasaki Y, Fernandez TV, Palejev D, Li Y, et al. Transcriptome analysis of the human striatum in tourette syndrome. Biol Psychiatry (2016) 79:372–82. doi: 10.1016/j.biopsych.2014.07.018
19. Zhao L, Cheng N, Sun B, Wang S, Li A, Wang Z, et al. Regulatory effects of ningdong granule on microglia-mediated neuroinflammation in a rat model of tourette's syndrome. Biosci Trends (2020) 14:271–78. doi: 10.5582/bst.2020.03262
20. Lin L, Lan Y, Zhu H, Yu L, Wu S, Wan W, et al. Effects of chemogenetic inhibition of D1 or D2 receptor-containing neurons of the substantia nigra and striatum in mice with tourette syndrome. Front Mol Neurosci (2021) 14:779436. doi: 10.3389/fnmol.2021.779436
21. Salvador A, Worbe Y, Delorme C, Coricelli G, Gaillard R, Robbins TW, et al. Specific effect of a dopamine partial agonist on counterfactual learning: evidence from gilles de la tourette syndrome. Sci Rep (2017) 7:6292. doi: 10.1038/s41598-017-06547-8
22. Lin L, Yu L, Xiang H, Hu X, Yuan X, Zhu H, et al. Effects of acupuncture on behavioral stereotypies and brain dopamine system in mice as a model of tourette syndrome. Front Behav Neurosci (2019) 13:239. doi: 10.3389/fnbeh.2019.00239
23. Blagotinšek Cokan K, Mavri M, Rutland CS, Glišić S, Senćanski M, Vrecl M, et al. Critical impact of different conserved endoplasmic retention motifs and dopamine receptor interacting proteins (DRIPs) on intracellular localization and trafficking of the D(2) dopamine receptor (D(2)-r) isoforms. Biomolecules (2020) 10. doi: 10.3390/biom10101355
24. Lai M, Li Y, Luo D, Xu J, Li J. Dopamine-2 receptor antibody encephalitis presenting as pure tongue-biting in a tourette syndrome patient: a case report. BMC Psychiatry (2022) 22:47. doi: 10.1186/s12888-021-03683-4
25. Nikolaus S, Mamlins E, Antke C, Dabir M, Müller HW, Giesel FL. Boosted dopamine and blunted serotonin in tourette syndrome - evidence from in vivo imaging studies. Rev Neurosci (2022). doi: 10.1515/revneuro-2022-0035
26. Liu X, Wang X, Cao A, Zhang X. Immune function changes of the IDPN-induced tourette syndrome rat model. Int J Dev Neurosci (2021) 81:159–66. doi: 10.1002/jdn.10085
27. Yang L, Wang X, Liu X, Chen X. Striatal syntaxin 1A is associated with development of tourette syndrome in an iminodipropionitrile-induced animal model. Dis Markers (2022) 2022:1148191. doi: 10.1155/2022/1148191
28. Cisbani G, Drouin-Ouellet J, Gibrat C, Saint-Pierre M, Lagacé M, Badrinarayanan S, et al. Cystamine/cysteamine rescues the dopaminergic system and shows neurorestorative properties in an animal model of parkinson's disease. Neurobiol Dis (2015) 82:430–44. doi: 10.1016/j.nbd.2015.07.012
29. Ajami B, Bennett JL, Krieger C, Tetzlaff W, Rossi FM. Local self-renewal can sustain CNS microglia maintenance and function throughout adult life. Nat Neurosci (2007) 10:1538–43. doi: 10.1038/nn2014
30. Colton C, Wilcock DM. Assessing activation states in microglia. CNS Neurol Disord Drug Targets (2010) 9:174–91. doi: 10.2174/187152710791012053
31. Wohleb ES, Delpech JC. Dynamic cross-talk between microglia and peripheral monocytes underlies stress-induced neuroinflammation and behavioral consequences. Prog Neuropsychopharmacol Biol Psychiatry (2017) 79:40–8. doi: 10.1016/j.pnpbp.2016.04.013
32. Umpierre AD, Wu LJ. How microglia sense and regulate neuronal activity. Glia (2021) 69:1637–53. doi: 10.1002/glia.23961
33. Town T, Nikolic V, Tan J. The microglial "activation" continuum: from innate to adaptive responses. J Neuroinflamm (2005) 2:24. doi: 10.1186/1742-2094-2-24
34. Arcuri C, Mecca C, Bianchi R, Giambanco I, Donato R. The pathophysiological role of microglia in dynamic surveillance, phagocytosis and structural remodeling of the developing CNS. Front Mol Neurosci (2017) 10:191. doi: 10.3389/fnmol.2017.00191
35. Hu X, Leak RK, Shi Y, Suenaga J, Gao Y, Zheng P, et al. Microglial and macrophage polarization–new prospects for brain repair. Nat Rev Neurol (2015) 11:56–64. doi: 10.1038/nrneurol.2014.207
36. Gomes-Leal W. Why microglia kill neurons after neural disorders? the friendly fire hypothesis. Neural Regener Res (2019) 14:1499–502. doi: 10.4103/1673-5374.255359
37. Tseng KY, Wu JS, Chen YH, Airavaara M, Cheng CY, Ma KH. Modulating Microglia/Macrophage activation by CDNF promotes transplantation of fetal ventral mesencephalic graft survival and function in a hemiparkinsonian rat model. Biomedicines (2022) 10. doi: 10.3390/biomedicines10061446
38. Rim C, Park HS, You MJ, Yang B, Kim HJ, Sung S, et al. Microglia involvement in sex-dependent behaviors and schizophrenia occurrence in offspring with maternal dexamethasone exposure. Schizophr (Heidelb) (2022) 8:71. doi: 10.1038/s41537-022-00280-6
39. Li H, Knight WC, Yang P, Guo Y, Perlmutter JS, Morris JC, et al. Microglia implicated in tauopathy in the striatum of neurodegenerative disease patients from genotype to phenotype. Int J Mol Sci (2020) 21. doi: 10.3390/ijms21176047
40. Radenovic L, Nenadic M, Ułamek-Kozioł M, Januszewski S, Czuczwar SJ, Andjus PR, et al. Heterogeneity in brain distribution of activated microglia and astrocytes in a rat ischemic model of alzheimer's disease after 2 years of survival. Aging (Albany NY) (2020) 12:12251–67. doi: 10.18632/aging.103411
41. Klawonn AM, Fritz M, Castany S, Pignatelli M, Canal C, Similä F, et al. Microglial activation elicits a negative affective state through prostaglandin-mediated modulation of striatal neurons. Immunity (2021) 54:225–34.e6. doi: 10.1016/j.immuni.2020.12.016
42. Hong AR, Jang JG, Chung YC, Won SY, Jin BK. Interleukin 13 on microglia is neurotoxic in lipopolysaccharide-injected striatum in vivo. Exp Neurobiol (2022) 31:42–53. doi: 10.5607/en21032
43. Leckman JF, Katsovich L, Kawikova I, Lin H, Zhang H, Krönig H, et al. Increased serum levels of interleukin-12 and tumor necrosis factor-alpha in tourette's syndrome. Biol Psychiatry (2005) 57:667–73. doi: 10.1016/j.biopsych.2004.12.004
44. Yeon SM, Lee JH, Kang D, Bae H, Lee KY, Jin S, et al. A cytokine study of pediatric tourette's disorder without obsessive compulsive disorder. Psychiatry Res (2017) 247:90–6. doi: 10.1016/j.psychres.2016.11.005
45. Gabbay V, Coffey BJ, Guttman LE, Gottlieb L, Katz Y, Babb JS, et al. A cytokine study in children and adolescents with tourette's disorder. Prog Neuropsychopharmacol Biol Psychiatry (2009) 33:967–71. doi: 10.1016/j.pnpbp.2009.05.001
46. Tao Y, Xu P, Zhu W, Chen Z, Tao X, Liu J, et al. Changes of cytokines in children with tic disorder. Front Neurol (2021) 12:800189. doi: 10.3389/fneur.2021.800189
47. Long H, Ruan J, Zhang M, Wang C, Huang Y. Rhynchophylline attenuates tourette syndrome via BDNF/NF-κB pathway in vivo and In Vitro. Neurotox Res (2019) 36:756–63. doi: 10.1007/s12640-019-00079-x
48. Cheng YH, Zheng Y, He F, Yang JH, Li WB, Wang ML, et al. Detection of autoantibodies and increased concentrations of interleukins in plasma from patients with tourette's syndrome. J Mol Neurosci (2012) 48:219–24. doi: 10.1007/s12031-012-9811-8
49. Frick L, Rapanelli M, Abbasi E, Ohtsu H, Pittenger C. Histamine regulation of microglia: gene-environment interaction in the regulation of central nervous system inflammation. Brain Behav Immun (2016) 57:326–37. doi: 10.1016/j.bbi.2016.07.002
50. Li Y, Wang X, Yang H, Li Y, Gui J, Cui Y. Profiles of proinflammatory cytokines and T cells in patients with tourette syndrome: a meta-analysis. Front Immunol (2022) 13:843247. doi: 10.3389/fimmu.2022.843247
51. Mitsumoto Y, Nagai Y, Takata R, Mori A. Rapid eye movement sleep deprivation enhances vulnerability of striatal dopaminergic neurons to 1-methyl-4-phenyl-1,2,3,6-tetrahydropyridine neurotoxicity in mice. Psychogeriatrics (2020) 20:129–30. doi: 10.1111/psyg.12456
52. Márquez-Valadez B, Aquino-Miranda G, Quintero-Romero MO, Papacostas-Quintanilla H, Bueno-Nava A, López-Rubalcava C, et al. The systemic administration of the histamine H(1) receptor Antagonist/Inverse agonist chlorpheniramine to pregnant rats impairs the development of nigro-striatal dopaminergic neurons. Front Neurosci (2019) 13:360. doi: 10.3389/fnins.2019.00360
53. Huot P, Lévesque M, Parent A. The fate of striatal dopaminergic neurons in parkinson's disease and huntington's chorea. Brain (2007) 130:222–32. doi: 10.1093/brain/awl332
54. Huot P, Lévesque M, Morissette M, Calon F, Dridi M, Di Paolo T, et al. L-dopa treatment abolishes the numerical increase in striatal dopaminergic neurons in parkinsonian monkeys. J Chem Neuroanat (2008) 35:77–84. doi: 10.1016/j.jchemneu.2007.06.004
55. Chohan MO, Kopelman JM, Yueh H, Fazlali Z, Greene N, Harris AZ, et al. Developmental impact of glutamate transporter overexpression on dopaminergic neuron activity and stereotypic behavior. Mol Psychiatry (2022) 27:1515–26. doi: 10.1038/s41380-021-01424-3
56. Bellini S, Fleming KE, De M, McCauley JP, Petroccione MA, D'Brant LY, et al. Neuronal glutamate transporters control dopaminergic signaling and compulsive behaviors. J Neurosci (2018) 38:937–61. doi: 10.1523/JNEUROSCI.1906-17.2017
57. Berridge KC, Aldridge JW, Houchard KR, Zhuang X. Sequential super-stereotypy of an instinctive fixed action pattern in hyper-dopaminergic mutant mice: a model of obsessive compulsive disorder and tourette's. BMC Biol (2005) 3:4. doi: 10.1186/1741-7007-3-4
58. Capetian P, Roessner V, Korte C, Walitza S, Riederer F, Taurines R, et al. Altered urinary tetrahydroisoquinoline derivatives in patients with tourette syndrome: reflection of dopaminergic hyperactivity? J Neural Transm (Vienna) (2021) 128:115–20. doi: 10.1007/s00702-020-02289-6
59. Hienert M, Gryglewski G, Stamenkovic M, Kasper S, Lanzenberger R. Striatal dopaminergic alterations in tourette's syndrome: a meta-analysis based on 16 PET and SPECT neuroimaging studies. Transl Psychiatry (2018) 8:143. doi: 10.1038/s41398-018-0202-y
60. Saha S, Chatterjee M, Shom S, Sinha S, Mukhopadhyay K. Functional SLC6A3 polymorphisms differentially affect autism spectrum disorder severity: a study on Indian subjects. Metab Brain Dis (2022) 37:397–410. doi: 10.1007/s11011-021-00876-4
61. DiCarlo GE, Aguilar JI, Matthies HJ, Harrison FE, Bundschuh KE, West A, et al. Autism-linked dopamine transporter mutation alters striatal dopamine neurotransmission and dopamine-dependent behaviors. J Clin Invest (2019) 129:3407–19. doi: 10.1172/JCI127411
62. Stewart A, Mayer FP, Gowrishankar R, Davis GL, Areal LB, Gresch PJ, et al. Behaviorally penetrant, anomalous dopamine efflux exposes sex and circuit dependent regulation of dopamine transporters. Mol Psychiatry (2022) 27:4869–80. doi: 10.1038/s41380-022-01773-7
63. Viaggi C, Gerace C, Pardini C, Corsini GU, Vaglini F. Serotonin abnormalities in engrailed-2 knockout mice: new insight relevant for a model of autism spectrum disorder. Neurochem Int (2015) 87:34–42. doi: 10.1016/j.neuint.2015.05.004
64. Ding S, Li L, Zhou FM. Nigral dopamine loss induces a global upregulation of presynaptic dopamine D1 receptor facilitation of the striatonigral GABAergic output. J Neurophysiol (2015) 113:1697–711. doi: 10.1152/jn.00752.2014
65. Chen X, Yang Z, Shao Y, Kim K, Wang Y, Wang Y, et al. Pitx3 deficiency promotes age-dependent alterations in striatal medium spiny neurons. Front Aging Neurosci (2022) 14:960479. doi: 10.3389/fnagi.2022.960479
66. Shapouri-Moghaddam A, Mohammadian S, Vazini H, Taghadosi M, Esmaeili SA, Mardani F, et al. Macrophage plasticity, polarization, and function in health and disease. J Cell Physiol (2018) 233:6425–40. doi: 10.1002/jcp.26429
67. Gadani SP, Cronk JC, Norris GT, Kipnis J. IL-4 in the brain: a cytokine to remember. J Immunol (2012) 189:4213–9. doi: 10.4049/jimmunol.1202246
68. Nam JH, Park KW, Park ES, Lee YB, Lee HG, Baik HH, et al. Interleukin-13/-4-induced oxidative stress contributes to death of hippocampal neurons in aβ1-42-treated hippocampus in vivo. Antioxid Redox Signal (2012) 16:1369–83. doi: 10.1089/ars.2011.4175
69. Bok E, Cho EJ, Chung ES, Shin WH, Jin BK. Interleukin-4 contributes to degeneration of dopamine neurons in the lipopolysaccharide-treated substantia nigra in vivo. Exp Neurobiol (2018) 27:309–19. doi: 10.5607/en.2018.27.4.309
70. Jang J, Hong A, Chung Y, Jin B. Interleukin-4 aggravates LPS-induced striatal neurodegeneration in vivo via oxidative stress and polarization of Microglia/Macrophages. Int J Mol Sci (2022) 23. doi: 10.3390/ijms23010571
71. Tan Y, Wang Z, Liu T, Gao P, Xu S, Tan L. RNA Interference-mediated silencing of DNA methyltransferase 1 attenuates neuropathic pain by accelerating microglia M2 polarization. BMC Neurol (2022) 22:376. doi: 10.1186/s12883-022-02860-6
Keywords: Tourette syndrome, microglia, M1 polarization, striatum, dopaminergic neuron
Citation: Wang X, Liu X, Chen L and Zhang X (2023) The inflammatory injury in the striatal microglia-dopaminergic-neuron crosstalk involved in Tourette syndrome development. Front. Immunol. 14:1178113. doi: 10.3389/fimmu.2023.1178113
Received: 02 March 2023; Accepted: 06 April 2023;
Published: 28 April 2023.
Edited by:
Kun Xiong, Independent Researcher, Changsha, ChinaCopyright © 2023 Wang, Liu, Chen and Zhang. This is an open-access article distributed under the terms of the Creative Commons Attribution License (CC BY). The use, distribution or reproduction in other forums is permitted, provided the original author(s) and the copyright owner(s) are credited and that the original publication in this journal is cited, in accordance with accepted academic practice. No use, distribution or reproduction is permitted which does not comply with these terms.
*Correspondence: Xiumei Liu, xmliu0615@fjmu.edu.cn
†These authors have contributed equally to this work