3D bioartificial stretchable scaffolds mimicking the mechanical hallmarks of human cardiac fibrotic tissue
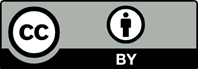
Human cardiac fibrotic tissues are characterized by a higher stiffness relative to healthy cardiac tissues. These tissues are unable to spontaneously contract and are subjected to passive mechanical stimulation during heart functionality. Moreover, scaffolds that can recapitulate the in vivo mechanical properties of the cardiac fibrotic tissues are lacking. Herein, this study aimed to design and fabricate mechanically stretchable bioartificial scaffolds with biomimetic composition and stiffness comparable to human cardiac fibrotic tissues. Poly(ε-caprolactone) (PCL) scaffolds with a stretchable mesh architecture were initially designed through structural and finite element method (FEM) analyses and subsequently fabricated by melt extrusion additive manufacturing (MEX). Scaffolds were surface functionalized by 3,4-dihydroxy-DL-phenylalanine (DOPA) polymerization (polyDOPA) to improve their interaction with natural polymers. Scaffold pores were then filled with different concentrations (5%, 7%, and 10% w/v) of gelatin methacryloyl (GelMA) hydrogels to support in vitro human cardiac fibroblasts (HCFs) 3D culture, thereby producing bioartificial PCL/GelMA scaffolds. Uniaxial tensile mechanical tests in static and dynamic conditions (1 Hz; 22% maximum strain) demonstrated that the bioartificial scaffolds had in vivo-like stretchability and similar stiffness to that of pathological cardiac tissue (tailored as a function of the number of PCL scaffold layers and GelMA hydrogel concentration). In vitro cell tests on bioartificial scaffolds using HCF-embedded GelMA hydrogels under static conditions displayed increasing cell viability, spreading, and cytoskeleton organization with decreasing GelMA hydrogel concentration. Moreover, α-smooth muscle actin (α-SMA)-positive cells were detected after 7 days of culture in static conditions followed by another 7 days of culture in dynamic conditions and not in HCF-loaded scaffolds cultured in static conditions for 14 days. These results suggested that in vitro culture under cyclic mechanical stimulations could induce an HCF phenotypic switch into myofibroblasts.
- World Health Organization (WHO). Cardiovascular Diseases (CVDs). https://www.Who.Int/En/News-Room/Fact-Sheets/Detail/ Cardiovascular-Diseases-(Cvds); 2019.
- Talman V, Ruskoaho H. Cardiac fibrosis in myocardial infarction—from repair and remodeling to regeneration. Cell Tissue Res. 2016;365(3):563-581. doi: 10.1007/s00441-016-2431-9
- Woodruff MA, Hutmacher DW. The return of a forgotten polymer – polycaprolactone in the 21st century. Prog Polym Sci. 2010;35(10):1217-1256. doi: 10.1021/acsami.3c04362
- Nichol JW, Koshy ST, Bae H, Hwang CM, Yamanlar S, Khademhosseini A. Cell-laden microengineered gelatin methacrylate hydrogels. Biomaterials. 2010;31(21):5536-5544. doi: 10.1016/j.biomaterials.2010.03.064
- Deddens JC, Sadeghi AH, Hjortnaes J, et al. Modeling the human scarred heart in vitro: toward new tissue engineered models. Adv Healthc Mater. 2017;6(3):1-21. doi: 10.1002/adhm.201600571
- Olvera D, Sohrabi Molina M, Hendy G, Monaghan MG. Electroconductive melt electrowritten patches matching the mechanical anisotropy of human myocardium. Adv Funct Mater. 2020;30(44):1-10. doi: 10.1002/adfm.201909880
- Castilho M, van Mil A, Maher M, et al. Melt electrowriting allows tailored microstructural and mechanical design of scaffolds to advance functional human myocardial tissue formation. Adv Funct Mater. 2018;28(40):1-10. doi: 10.1002/adfm.201803151.
- Castilho M, Feyen D, Flandes-Iparraguirre M, et al. Melt electrospinning writing of poly-hydroxymethylglycolide-co-ε-Caprolactone-based scaffolds for cardiac tissue engineering. Adv Healthc Mater. 2017;6(18):1-9. doi: 10.1002/adhm.201700311
- Paxton NC, Daley R, Forrestal DP, Allenby MC, Woodruff MA. Auxetic tubular scaffolds via melt electrowriting. Mater Des. 2020;193:108787. doi: 10.1016/j.matdes.2020.108787.
- Bas O, D’Angella D, Baldwin JG, et al. An integrated design, material, and fabrication platform for engineering biomechanically and biologically functional soft tissues. ACS Appl Mater Interfaces. 2017;9(35):29430-29437. doi: 10.1021/acsami.7b08617
- Saidy NT, Wolf F, Bas O, et al. Biologically inspired scaffolds for heart valve tissue engineering via melt electrowriting. Small. 2019;15(24):e1900873. doi: 10.1002/smll.201900873
- Robinson TM, Hutmacher DW, Dalton PD. The next frontier in melt electrospinning: taming the jet. Adv Funct Mater. 2019;29(44):1904664. doi: 10.1002/adfm.201904664
- Hochleitner G, Jüngst T, Brown TD, et al. Additive manufacturing of scaffolds with sub-micron filaments via melt electrospinning writing. Biofabrication. 2015;7(3):035002. doi: 10.1088/1758-5090/7/3/035002
- Hutmacher DW, Schantz T, Zein I, Ng KW, Teoh SH, Tan KC. Mechanical properties and cell cultural response of polycaprolactone scaffolds designed and fabricated via fused deposition modeling. J Biomed Mater Res. 2001;55(2):203-216. doi : 10.1002/1097-4636(200105)55:2<203::aid-jbm1007>3.0.CO;2-7
- Agarwala MK, Jamalabad VR, Langrana NA, Safari A, Whalen PJ, Danforth SC. Structural quality of parts processed by fused deposition. Rapid Prototyp J. 1996:2(4):4-19. doi: 10.1108/13552549610732034
- Meng Z, He J, Li J, Su Y, Li D. Melt-based, solvent-free additive manufacturing of biodegradable polymeric scaffolds with designer microstructures for tailored mechanical/biological properties and clinical applications. Virtual Phys Prototyp. 2020;15(4):417-444. doi: 10.1080/17452759.2020.1808937
- Emig R, Zgierski-Johnston CM, Timmermann V, et al. Passive myocardial mechanical properties: meaning, measurement, models. Biophys Rev. 2021;13(5):587-610. doi: 10.1007/s12551-021-00838-1
- Zhang W, Huang G, Xu F. Engineering biomaterials and approaches for mechanical stretching of cells in three dimensions. Front Bioeng Biotechnol. 2020;8:589590. doi: 10.3389/fbioe.2020.589590
- Huang G, Wang L, Wang S, et al. Engineering three-dimensional cell mechanical microenvironment with hydrogels. Biofabrication. 2012;4(4):042001. doi: 10.1088/1758-5082/4/4/042001
- Park N, Kim J. Hydrogel‐based artificial muscles: overview and recent progress. Adv Intell Syst. 2020;2(4):1900135. doi: 10.1002/aisy.201900135
- Boffito M, Di Meglio F, Mozetic P, et al. Surface functionalization of polyurethane scaffolds mimicking the myocardial microenvironment to support cardiac primitive cells. PLoS One. 2018;13(7):e0199896. doi: 10.1371/journal.pone.0199896
- Chiono V, Ciardelli G, Vozzi G, et al. Enzymatically-modified melt-extruded guides for peripheral nerve repair. Eng Life Sci. 2008; 8(3):226-237. doi: 10.1002/elsc.200700069
- Chiono V, Vozzi G, Acunto MD, et al. Characterisation of blends between poly (ε-caprolactone) and polysaccharides for tissue engineering applications. Mater Sci Eng C. 2009;29(7):2174-2187. doi: 10.1016/j.msec.2009.04.020
- Spedicati M, Ruocco G, Zoso A, et al. Biomimetic design of bioartificial scaffolds for the in vitro modelling of human cardiac fibrosis. Front Bioeng Biotechnol. 2022;10:983872. doi: 10.3389/fbioe.2022.983872.
- Bari E, Scocozza F, Perteghella S, et al. 3D bioprinted scaffolds containing mesenchymal stem/stromal lyosecretome: next generation controlled release device for bone regenerative medicine. Pharmaceutics. 2021;13(4):515. doi: 10.3390/pharmaceutics13040515
- Chen QZ, Harding SE, Ali NN, Lyon AR, Boccaccini AR. Biomaterials in cardiac tissue engineering: ten years of research survey. Mater Sci Eng R Rep. 2008;59(1–6): 1-37. doi: 10.1016/j.mser.2007.08.001
- Guimarães CF, Gasperini L, Marques AP, Reis RL. The stiffness of living tissues and its implications for tissue engineering. Nat Rev Mater. 2020;5:351-370. doi: 10.1038/s41578-019-0169-1
- Chelnokova NO, Golyadkina AA, Kirillova IV, Polienko AV, Ivanov DV. Morphology and biomechanics of human heart. Proc SPIE. 2016;9710:971013. doi: 10.1117/12.2208423
- Visone R, Paoletti A, Cordiale L, et al. In vitro mechanical stimulation to reproduce the pathological Hallmarks of human cardiac fibrosis on a beating chip and predict the efficacy of drugs and advanced therapies. Adv Healthc Mater. 2024;13(4):e2301481. doi: 10.1002/adhm.202301481
- Paoletti C, Divieto C, Tarricone G, Di Meglio F, Nurzynska D, Chiono V. MicroRNA-mediated direct reprogramming of human adult fibroblasts toward cardiac Phenotype. Front Bioeng Biotechnol. 2020;8:529. doi: 10.3389/fbioe.2020.00529
- Nicoletti L, Paoletti C, Tarricone G, et al. Lipoplexes for effective in vitro delivery of microRNAs to adult human cardiac fibroblasts for perspective direct cardiac cell reprogramming. Nanomedicine. 2022;45:102589. doi: 10.1016/j.nano.2022.102589
- Schipani R, Nolan DR, Lally C, Kelly DJ. Integrating finite element modelling and 3D printing to engineer biomimetic polymeric scaffolds for tissue engineering. Connect Tissue Res. 2020;61(2):174-189. doi: 10.1080/03008207.2019.1656720
- Carmagnola I, Chiono V, Ruocco G, et al. Plga membranes functionalized with gelatin through biomimetic mussel-inspired strategy. Nanomaterials. 2020;10(11):1-17. doi: 10.3390/nano10112184
- Melilli G, Carmagnola I, Tonda-Turo C, et al. DLP 3D printing meets lignocellulosic biopolymers: carboxymethyl cellulose inks for 3D biocompatible hydrogels. Polymers (Basel). 2020;12(8):1-11. doi: 10.3390/POLYM12081655
- Ding H, Illsley NP, Chang RC. 3D Bioprinted GelMA based models for the study of Trophoblast cell invasion. Sci Rep. 2019;9(1):18854. doi: 10.1038/s41598-019-55052-7
- Fedorovich NE, Oudshoorn MH, van Geemen D, Hennink WE, Alblas J, Dhert WJ. The effect of photopolymerization on stem cells embedded in hydrogels. Biomaterials. 2009;30(3):344-353. doi: 10.1016/j.biomaterials.2008.09.037
- Sadeghi AH, Shin SR, Deddens JC, et al. Engineered 3D cardiac fibrotic tissue to study fibrotic remodeling. Adv Healthc Mater. 2017;6(11):1-14. doi: 10.1002/adhm.201601434
- McCain ML, Lee H, Aratyn-Schaus Y, Kléber AG, Parker KK. Cooperative coupling of cell-matrix and cell-cell adhesions in cardiac muscle. Proc Natl Acad Sci U S A. 2012;109(25):9881-9886. doi: 10.1073/pnas.1203007109
- Rajabi N, Kharaziha M, Emadi R, Zarrabi A, Mokhtari H, Salehi S. An adhesive and injectable nanocomposite hydrogel of thiolated gelatin/gelatin methacrylate/Laponite® as a potential surgical sealant. J Colloid Interface Sci. 2020;564:155-169. doi: 10.1016/j.jcis.2019.12.048
- Chen YW, Wang K, Ho CC, Kao CT, Ng HY, Shie MY. Cyclic tensile stimulation enrichment of Schwann cell-laden auxetic hydrogel scaffolds towards peripheral nerve tissue engineering. Mater Des. 2020;195:108982. doi: 10.1016/j.matdes.2020.108982
- Donderwinkel I, Van Hest JCM, Cameron NR. Bio-inks for 3D bioprinting: recent advances and future prospects. Polym Chem. 2017;8:4451-71. doi: 10.1039/c7py00826k
- Ward M, Iskratsch T. Mix and (mis-)match – the mechanosensing machinery in the changing environment of the developing, healthy adult and diseased heart. Biochim Biophys Acta Mol Cell Res. 2020;1867(3):118436. doi: 10.1016/j.bbamcr.2019.01.017
- Sirry MS, Butler JR, Patnaik SS, et al. Characterisation of the mechanical properties of infarcted myocardium in the rat under biaxial tension and uniaxial compression. J Mech Behav Biomed Mater. 2016;63:252-264. doi: 10.1016/j.jmbbm.2016.06.029
- Arani A, Arunachalam SP, Chang ICY, et al. Cardiac MR elastography for quantitative assessment of elevated myocardial stiffness in cardiac amyloidosis. J Magn Reson Imaging. 2017;46(5):1361-1367. doi: 10.1002/JMRI.25678
- Vedadghavami A, Minooei F, Mohammadi MH, et al. Manufacturing of hydrogel biomaterials with controlled mechanical properties for tissue engineering applications. Acta Biomater. 2017;62:42-63. doi: 10.1016/j.actbio.2017.07.028
- Chaudhuri O, Cooper-White J, Janmey PA, Mooney DJ, Shenoy VB. Effects of extracellular matrix viscoelasticity on cellular behaviour. Nature. 2020;584(7822): 535-546. doi: 10.1038/s41586-020-2612-2O
- Choi JR, Yong KW, Choi JY, Cowie AC. Recent advances in photo-crosslinkable hydrogels for biomedical applications. Biotechniques. 2019;66(1):40-53. doi: 10.2144/btn-2018-0083
- Ruocco G, Zoso A, Mortati L, Carmagnola I, Chiono V. Biomimetic electrospun scaffold-based in vitro model resembling the Hallmarks of human myocardial fibrotic tissue. ACS Biomater Sci Eng. 2023;9(7):4368-4380. doi: 10.1021/acsbiomaterials.3c00483