In situ bioprinting for cartilage repair using a parallel manipulator
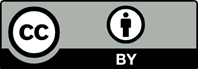
Regeneration of large-sized cartilage injury is a challenging endeavor. In vitro bioprinting for cartilage repair has several drawbacks, such as the tedious process of material preparation, potential contamination, and the mismatch between implant and defect. This study aimed to investigate the application of in situ bioprinting in cartilage repair using a parallel manipulator. In particular, the material extrusion rate and printing speed were adjusted to obtain the suitable forming parameters in a custom-made parallel manipulator. Cell experiments were conducted to determine the biocompatibility. Finally, a rabbit cartilage defect model was used to evaluate the feasibility of in situ bioprinting combined with machine vision. The results showed that to achieve optimum printing using the custom-made three-dimensional printer, 400–560 mm/min should be set as the standard printing speed, with an extrusion multiplier of 0.09–0.10. Cartilage defects can be precisely and easily segmented using a bimodal method with a 2% deviation error. In vitro experiments revealed that the utilized materials are highly biocompatible. Furthermore, according to the results from in vivo experiments, in situ bioprinting lends itself useful in the repair of cartilage defects. The overall results confirmed the feasibility of applying a parallel manipulator in in situ bioprinting for cartilage repair. Additional optimizations of the proposed approach are warranted prior to translation into clinical applications in the future.
- Li M, Yin H, Yan Z, et al. The immune microenvironment in cartilage injury and repair. Acta Biomater. 2022;140:23-42. doi: 10.1016/j.actbio.2021.12.006
- Guo X, Ma Y, Min Y, et al. Progress and prospect of technical and regulatory challenges on tissue-engineered cartilage as therapeutic combination product. Bioact Mater. 2023;20:501-518. doi: 10.1016/j.bioactmat.2022.06.015
- Wei W, Dai H. Articular cartilage and osteochondral tissue engineering techniques: Recent advances and challenges. Bioact Mater. 2021;6(12):4830-4855. doi: 10.1016/j.bioactmat.2021.05.011
- Chen YR, Yan X, Yuan FZ, et al. Kartogenin-conjugated double-network hydrogel combined with stem cell transplantation and tracing for cartilage repair. Adv Sci (Weinh). 2022;9(35):e2105571. doi: 10.1002/advs.202105571
- Zhang Y, Liu X, Zeng L, et al. Polymer fiber scaffolds for bone and cartilage tissue engineering. Adv Funct Mater. 2019;29(36):1903279. doi: 10.1002/adfm.201903279
- Browe D, Burdis R, Diaz-Payno PJ, et al. Promoting endogenous articular cartilage regeneration using extracellular matrix scaffolds. Mater Today Bio. 2022;16:100343. doi: 10.1016/j.mtbio.2022.100343
- Li X, Zheng F, Wang X, et al. Biomaterial inks for extrusion-based 3D bioprinting: Property, classification, modification, and selection. Int J Bioprint. 2022;9(2). doi: 10.18063/ijb.v9i2.649
- Sadeghianmaryan A, Naghieh S, Yazdanpanah Z, et al. Fabrication of chitosan/alginate/hydroxyapatite hybrid scaffolds using 3D printing and impregnating techniques for potential cartilage regeneration. Int J Biol Macromol. 2022;204:62-75. doi: 10.1016/j.ijbiomac.2022.01.201
- Sang S, Mao X, Cao Y, et al. 3D Bioprinting using synovium-derived MSC-laden photo-cross-linked ECM bioink for cartilage regeneration. ACS Appl Mater Interfaces. 2023;15(7):8895-8913. doi: 10.1021/acsami.2c19058
- Costa J, Silva-Correia J, Pina S, et al. Indirect printing of hierarchical patient-specific scaffolds for meniscus tissue engineering. Bio-Des Manuf. 2019;2(4):225-241. doi: 10.1007/s42242-019-00050-x
- Zou Q, Grottkau BE, He Z, et al. Biofabrication of valentine-shaped heart with a composite hydrogel and sacrificial material. Mater Sci Eng C Mater Biol Appl. 2020;108:110205. doi: 10.1016/j.msec.2019.110205
- MacAdam A, Chaudry E, McTiernan CD, Cortes D, Suuronen EJ, Alarcon EI. Development of in situ bioprinting: A mini review. Front Bioeng Biotechnol. 2022;10:940896. doi: 10.3389/fbioe.2022.940896
- Mahmoudi Z, Sedighi M, Jafari A, et al. In situ 3D bioprinting: A promising technique in advanced biofabrication strategies. Bioprinting. 2023;e00260. doi: 10.1016/j.bprint.2023.e00260
- Zhao W, Hu C, Xu T. In vivo bioprinting: Broadening the therapeutic horizon for tissue injuries. Bioact Mater. 2023;25:201-222. doi: 10.1016/j.bioactmat.2023.01.018
- Li L, Yu F, Shi J, et al. In situ repair of bone and cartilage defects using 3D scanning and 3D printing. Sci Rep. 2017;7(1):9416. doi: 10.1038/s41598-017-10060-3
- O’Connell CD, Di Bella C, Thompson F, et al. Development of the Biopen: A handheld device for surgical printing of adipose stem cells at a chondral wound site. Biofabrication. 2016;8(1):015019. doi: 10.1088/1758-5090/8/1/015019
- Hakimi N, Cheng R, Leng L, et al. Handheld skin printer: In situ formation of planar biomaterials and tissues. Lab Chip. 2018;18(10):1440-1451. doi: 10.1039/c7lc01236e
- Chen H, Ma X, Gao T, Zhao W, Xu T, Liu Z. Robot-assisted in situ bioprinting of gelatin methacrylate hydrogels with stem cells induces hair follicle-inclusive skin regeneration. Biomed Pharmacother. 2023;158:114140. doi: 10.1016/j.biopha.2022.114140
- Moncal K, Gudapati H, Godzik P, et al. Intra-operative bioprinting of hard, soft, and hard/soft composite tissues for craniomaxillofacial reconstruction. Adv Funct. Mater. 2021;31(29). doi: 10.1002/adfm.202010858
- Ozbolat IT, Chen H, Yu Y. Development of ‘multi-arm bioprinter’ for hybrid biofabrication of tissue engineering constructs. Rob Comput Integr Manuf. 2014;30(3):295-304. doi: 10.1016/j.rcim.2013.10.005
- Moncal KK, Yeo M, Celik N, et al. Comparison of in-situ versus ex-situ delivery of polyethylenimine-BMP-2 polyplexes for rat calvarial defect repair via intraoperative bioprinting. Biofabrication. 2022;15(1). doi: 10.1088/1758-5090/ac9f70
- Di Bella C, Duchi S, O’Connell CD, et al. In situ handheld three-dimensional bioprinting for cartilage regeneration. J Tissue Eng Regen Med. 2018;12(3):611-621. doi: 10.1002/term.2476
- Ma K, Zhao T, Yang L, et al. Application of robotic-assisted in situ 3D printing in cartilage regeneration with HAMA hydrogel: An in vivo study. J Adv Res. 2020;23: 123-132. doi: 10.1016/j.jare.2020.01.010
- Wang Y, Pereira RF, Peach C, Huang B, Vyas C, Bartolo P. Robotic in situ bioprinting for cartilage tissue engineering. Int J Extreme Manuf. 2023;5(3). doi: 10.1016/j.jare.2020.01.010
- Li L, Shi J, Ma K, et al. Robotic in situ 3D bio-printing technology for repairing large segmental bone defects. J Adv Res. 2021;30:75-84. doi: 10.1016/j.jare.2020.11.011
- Gholami P, Ahmadi-Pajouh MA, Abolftahi N, et al. Segmentation and measurement of chronic wounds for bioprinting. IEEE J Biomed Health Inform. 2018;22(4): 1269-1277. doi: 10.1109/jbhi.2017.2743526
- Lankton S, Tannenbaum A. Localizing region-based active contours. IEEE Trans Image Process. 2008;17(11):2029-2039. doi: 10.1109/TIP.2008.2004611
- Yu Y, Wang C, Fu Q, et al. Techniques and challenges of image segmentation: A review. Electronics. 2023;12(5). doi: 10.3390/electronics12051199
- Dexter A, Race AM, Steven RT, et al. Two-phase and graph-based clustering methods for accurate and efficient segmentation of large mass spectrometry images. Anal Chem. 2017;89(21):11293-11300. doi: 10.1021/acs.analchem.7b01758
- Shi H, Lee W. Image segmentation using K-means clustering, Gabor filter and moving mesh method. Imaging Sci J. 2023; 69(5-8):407-416. doi: 10.1080/13682199.2022.2161159
- Zhang F, Sun Z, Song M, Lang X. Progressive 3D shape segmentation using online learning. Comput Aided Design. 2015;58:2-12. doi: 10.1016/j.cad.2014.08.008
- Niri R, Gutierrez E, Douzi H, et al. Multi-view data augmentation to improve wound segmentation on 3D surface model by deep learning. IEEE Access. 2021;9: 157628-157638. doi: 10.1109/ACCESS.2021.3130784
- Lei H, Song C, Liu Z, et al. Rational design and additive manufacturing of alumina-based lattice structures for bone implant. Mater Design. 2022;221. doi: 10.1016/j.matdes.2022.111003
- Mathworks, Computer Vision Toolbox, https://ww2. mathworks.cn/help/vision/index
- Murdock M, Badylak S. Biomaterials-based in situ tissue engineering. Curr Opin Biomed Eng. 2017;1:4-7. doi: 10.1016%2Fj.cobme.2017.01.001
- Singh S, Choudhury D, Yu F, Mironov V, Naing MW. In situ bioprinting - Bioprinting from benchside to bedside? Acta Biomater. 2020;101:14-25. doi: 10.1016/j.actbio.2019.08.045
- Richter F, Lu J, Orosco RK, Yip MC. Robotic tool tracking under partially visible kinematic chain: A unified approach. IEEE Trans Rob. 2022;38(3):1653-1670. doi: 10.48550/arXiv.2102.06235
- Feng L, Zhang W, Gong Z, Lin G, Liang D. Developments of delta-like parallel manipulators - A review. Robot (China). 2014; 36(3):375-384. doi: 10.5772/61744
- Dong H, Hu B, Zhang W, et al. Robotic-assisted automated in situ bioprinting. Int J Bioprint. 2023;9(1):629. doi: 10.18063%2Fijb.v9i1.629
- Gao Q, Niu X, Shao L, et al. 3D printing of complex GelMA-based scaffolds with nanoclay. Biofabrication. 2019;11(3):035006. doi: 10.1088/1758-5090/ab0cf6
- Fritz R, Chaudhari A, Boutin R. Preoperative MRI of articular cartilage in the knee: A practical approach. J Knee Surg. 2020;33(11):1088-1099. doi: 10.1055/s-0040-1716719
- Potter H, Black B, Chong le R. New techniques in articular cartilage imaging. Clin Sports Med. 2009;28(1):77-94. doi: 10.4103%2F0971-3026.137028
- Chen X, Jiang C, Wang T, Zhu T, Li X, Huang J. Hyaluronic acid-based biphasic scaffold with layer-specific induction capacity for osteochondral defect regeneration. Mater Des. 2022;216. doi: 10.1016/j.matdes.2022.110550
- Schuurmans C, Mihajlovic M, Hiemstra C, Ito K, Hennink WE, Vermonden T. Hyaluronic acid and chondroitin sulfate (meth)acrylate-based hydrogels for tissue engineering: Synthesis, characteristics and pre-clinical evaluation. Biomaterials. 2021;268:120602. doi: 10.1016/j.biomaterials.2020.120602
- Agarwal G, Agiwa S, Srivastava A. Hyaluronic acid containing scaffolds ameliorate stem cell function for tissue repair and regeneration. Int J Biol Macromol. 2020; 165(Pt A):388-401. doi: 10.1016/j.ijbiomac.2020.09.107
- Amann E, Wolff P, Breel E, van Griensven M, Balmayor ER. Hyaluronic acid facilitates chondrogenesis and matrix deposition of human adipose derived mesenchymal stem cells and human chondrocytes co-cultures. Acta Biomater. 2017;52:130-144. doi: 10.1016/j.ijbiomac.2020.09.107
- da Silva LP, Santos T, Rodrigues D, et al. Stem cell-containing hyaluronic acid-based spongy hydrogels for integrated diabetic wound healing. J Invest Dermatol. 2017;137(7):1541-1551. doi: 10.1016/j.jid.2017.02.976
- Agrawal P, Pramanik K, Vishwanath V, et al. Enhanced chondrogenesis of mesenchymal stem cells over silk fibroin/ chitosan-chondroitin sulfate three dimensional scaffold in dynamic culture condition. J Biomed Mater Res B Appl Biomater. 2018;106(7):2576-2587. doi: 10.1002/jbm.b.34074
- Lafuente-Merchan M, Ruiz-Alonso S, Zabala A, et al. Chondroitin and dermatan sulfate bioinks for 3D bioprinting and cartilage regeneration. Macromol Biosci. 2022;22(3):e2100435. doi: 10.1002/mabi.202100435
- Tan G, Tabata Y. Chondroitin-6-sulfate attenuates inflammatory responses in murine macrophages via suppression of NF-kappaB nuclear translocation. Acta Biomater. 2014;10(6):2684-2692. doi: 10.1016/j.actbio.2014.02.025
- Wang D, Varghese S, Sharma B, et al. Multifunctional chondroitin sulphate for cartilage tissue-biomaterial integration. Nat Mater. 2007;6(5): 385-392. doi: 10.1038/nmat1890
- Kwon H, Brown W, Lee C, et al. Surgical and tissue engineering strategies for articular cartilage and meniscus repair. Nat Rev Rheumatol. 2019;15(9):550-570. doi: 10.1038%2Fs41584-019-0255-1
- 53. Trengove A, Di Bella C, O’Connor A. The challenge of cartilage integration: Understanding a major barrier to chondral repair. Tissue Eng Part B Rev. 2022;28(1): 114-128. doi: 10.1089/ten.teb.2020.0244