- 1School of Life and Environmental Sciences, Plant Breeding Institute, University of Sydney, Sydney, NSW, Australia
- 2Department of Animal, Plant and Soil Sciences, La Trobe University, AgriBio, Bundoora, VIC, Australia
- 3Agriculture Victoria, AgriBio, Centre for AgriBioscience, Bundoora, VIC, Australia
- 4USDA-ARS Cereal Disease Laboratory, Department of Plant Pathology, University of Minnesota, St. Paul, MN, United States
Barley leaf rust (BLR), caused by Puccinia hordei, is best controlled through genetic resistance. An efficient resistance breeding program prioritizes the need to identify, characterize, and map new sources of resistance as well as understanding the effectiveness, structure, and function of resistance genes. In this study, three mapping populations were developed by crossing Israelian barley lines “AGG-396,” “AGG-397,” and “AGG-403” (carrying unknown leaf rust resistance) with a susceptible variety “Gus” to characterize and map resistance. Genetic analysis of phenotypic data from rust testing F3s with a P. hordei pathotype 5457 P+ revealed monogenic inheritance in all three populations. Targeted genotyping-by-sequencing of the three populations detected marker trait associations in the same genomic region on the short arm of chromosome 2H between 39 and 57 Mb (AGG-396/Gus), 44 and 64 Mb (AGG-397/Gus), and 31 and 58 Mb (AGG-403/Gus), suggesting that the resistance in all three lines is likely conferred by the same locus (tentatively designated RphAGG396). Two Kompetitive allele-specific PCR (KASP) markers, HvGBSv2-902 and HvGBSv2-932, defined a genetic distance of 3.8 cM proximal and 7.1 cM distal to RphAGG396, respectively. To increase the marker density at the RphAGG396 locus, 75 CAPS markers were designed between two flanking markers. Integration of marker data resulted in the identification of two critical recombinants and mapping RphAGG396 between markers- Mloc-28 (40.75 Mb) and Mloc-41 (41.92 Mb) narrowing the physical window to 1.17 Mb based on the Morex v2.0 reference genome assembly. To enhance map resolution, 600 F2s were genotyped with markers- Mloc-28 and Mloc-41 and nine recombinants were identified, placing the gene at a genetic distance of 0.5 and 0.2 cM between the two markers, respectively. Two annotated NLR (nucleotide-binding domain leucine-rich repeat) genes (r2.2HG0093020 and r2.2HG0093030) were identified as the best candidates for RphAGG396. A closely linked marker was developed for RphAGG396 that can be used for marker-assisted selection.
Introduction
Cultivated barley, Hordeum vulgare, is considered as a founder crop of modern agriculture (Park et al., 2015) ranking fourth in terms of global production after maize, rice, and wheat. Barley can be affected by four rust diseases: leaf rust, crown rust, stripe rust, and stem rust. Among these, barley leaf rust (BLR; also known as brown rust), caused by the fungus Puccinia hordei Otth, is one of the most destructive and widely distributed worldwide. The disease has caused yield losses documented at up to 32% in Australia and North America, with losses in very susceptible varieties even reported as being as high as 60% (Cotterill et al., 1992; Castro et al., 2012; Park et al., 2015). BLR is best controlled through deployment of resistant cultivars (Singh et al., 2015; Rehman et al., 2017; Sardar et al., 2022) and therefore breeding for leaf rust resistance is one of the prime objectives of many barley breeding programs worldwide.
To date, 28 Rph (resistance to Puccinia hordei) genes have been cataloged and mapped in barley (Mehnaz et al., 2021a), comprising 25 seedling or all stage resistance (ASR) genes (Rph1-Rph19, Rph21-Rph22, and Rph25-Rph28) and three adult plant resistance (APR) genes-Rph20, Rph23, and Rph24 (Hickey et al., 2011; Singh et al., 2015; Ziems et al., 2017). Most of the cataloged ASR genes have limited value in breeding because they have been rendered ineffective by pathotypes of P. hordei with matching virulence (Park et al., 2015) or due to possible associated linkage drag (Bishnoi et al., 2022) in the case of those derived from wild species (H. spontaneum or H. bulbosum). To enhance the genetic base and durability of effective resistance in combating epidemics caused by new leaf rust pathotypes, there is a need to identify, characterize and map new resistance genes as well as understand their effectiveness, structure, and function (Park et al., 2015; Li et al., 2016; Wani et al., 2022).
Mapping the genetic basis of disease resistance requires the development of populations segregating for disease resistance response, phenotypic accuracy, marker genotyping, and subsequent trait association analysis to locate the target locus (Hamwieh et al., 2018). Population size, structure, and the type and density of molecular markers employed for mapping play vital roles in determining the resolution of the maps generated (Richards et al., 2017; Tomar et al., 2022). A variety of molecular markers have been developed over the last 30 years and used in mapping traits of interest in crops. For example, in barley, Rph13 (Jost et al., 2020), Rph26 (Yu et al., 2018), and Rph27 (Rothwell et al., 2020) have been mapped/fine-mapped in bi-parental mapping populations using SNPs (single nucleotide polymorphisms), CAPS (cleaved amplified polymorphic sequences) and HRM (High resolution melting), and indels (insertion–deletion events), respectively. Gene mapping provides the foundation for map-based cloning, which ultimately helps in understanding the structure and function of genes (Wani et al., 2022). Markers identified through gene mapping can be used in marker-assisted selection (MAS) to increase plant breeding efficiency as well in gene pyramiding to attain durable resistance.
In the current study, three bi-parental mapping populations were developed to undertake inheritance studies and the identification of genomic regions conferring resistance to BLR. Three barley genotypes (AGG-396, AGG-397, and AGG-403) were selected from a Middle Eastern and Central Asian barley germplasm collection based on their resistance to all predominant Australian pathotypes of P. hordei (Mehnaz et al., 2021b). We conducted the present studies with three objectives: (i) to identify the genomic regions associated with resistance to P. hordei in genotypes AGG-396, AGG-397 and AGG-403; (ii) to fine map the resistance (tentatively designated as RphAGG396); and (iii) to develop closely linked markers for MAS of RphAGG396.
Materials and methods
Plant and pathogen material
Three barley lines [AGG-396 (AUS#403467), AGG-397 (AUS#403468) and AGG-403 (AUS#403774)] from Israel were investigated in this study. The lines were obtained from Australian Grains Genebank (AGG) Horsham, Victoria. Mapping populations were developed by crossing each of the three lines with a leaf rust susceptible (S) genotype “Gus.” F1s (first filial generation) derived from the three crosses were grown, selfed, and advanced to the F2 generation. The F2s were sown as space planted long rows in the field and single plants were harvested as F3 families (AGG-396/Gus = 105; AGG-397/Gus = 120; AGG-403/Gus = 126 families). A single seed from each F3 family was then planted in a 20 cm pot @ three lines per pot to advance to the F4 generation. Sixteen pathotypes of P. hordei (14 of Australian origin and two of North American origin; Table 1) were used according to the objectives of the corresponding experiments.
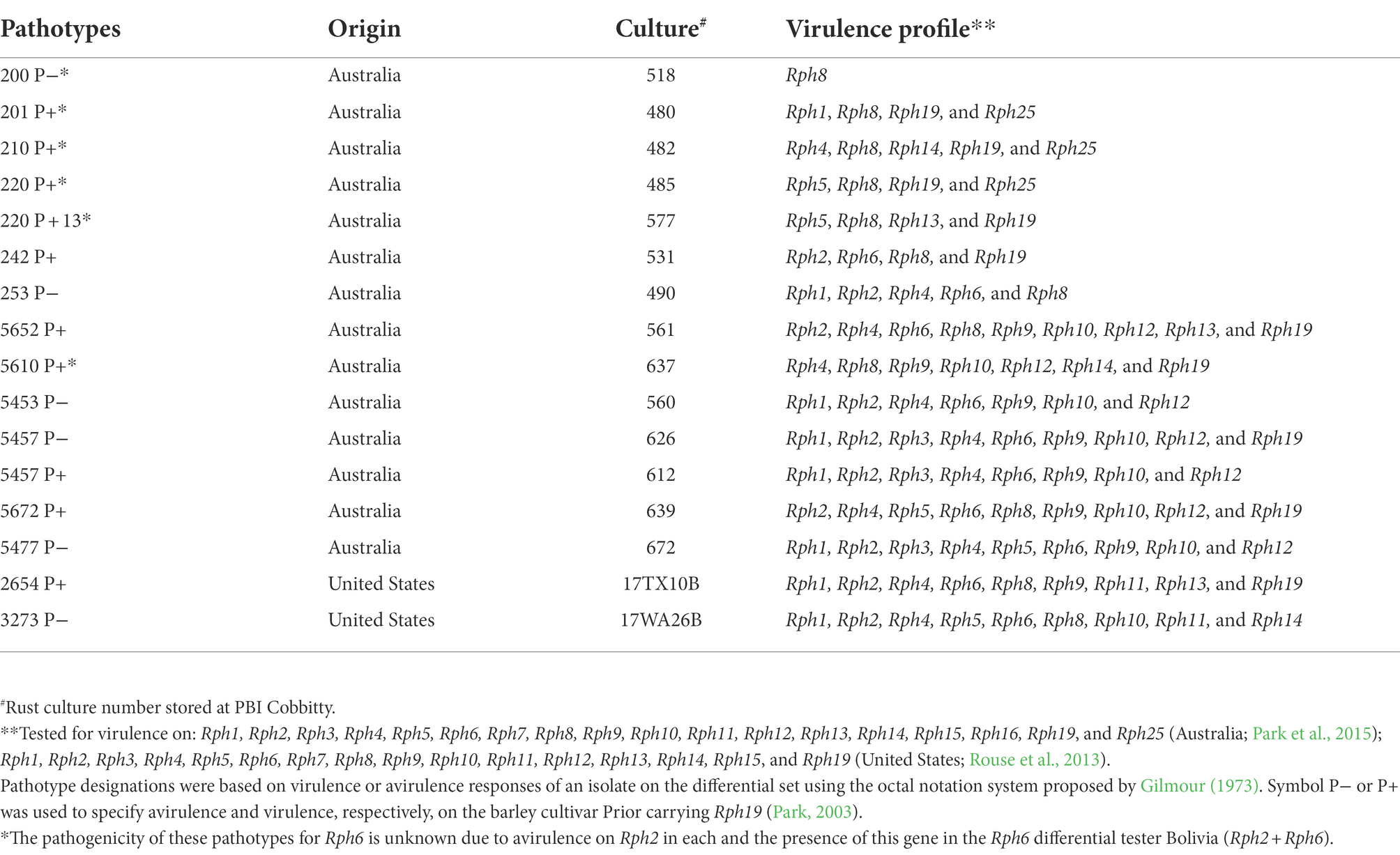
Table 1. Puccinia hordei pathotypes used in this study, along with origin and their virulence profile.
Sowing and inoculations
Australia
The test lines and control genotypes (three lines/pot in clumps @ 8–10 seedlings/pot) and F3s (1 line/pot dispersed @ 20 seedlings/pot) were sown in 90 mm diameter pots containing Grange Horticultural® soil premix comprised of 10% composted pine bark, 80% pine bark, 10% propagating sand, 1 kg/m3 gypsum, 1 kg/m3 superphosphate, 0.25 kg/m3 potassium nitrate, 0.25 kg/m3 nitroform, and 1.5 kg/m3 magrilime. Prior to sowing, all pots were fertilized with Aquasol @ 25 g/10 l of water. After sowing, pots were kept at 24°C in a temperature-controlled growth room. Ten-days old seedlings were inoculated with a P. hordei pathotype 5457 P+. A suspension mixture was prepared by adding 10 mg urediniospores /10 ml of isopar, a light mineral oil (Univar., Ingleburn, NSW, Australia), for 200 pots and the mixture was then atomized over the seedlings with a mist atomizer. Following inoculation, seedlings were incubated at 25°C in a dark chamber for 24 h. An ultrasonic humidifier was used to create mist in the incubation chamber. After 24 h of incubation, seedlings were shifted to microclimate rooms maintained at 24°C with natural light and an automated irrigation system.
United States
The three test lines were also rust tested in Minnesota, United States with two North American P. hordei pathotypes (17TX10B and 17WA26B). Test lines and control genotypes (four lines/pot in clumps @ 20 seedlings/pot) were sown in plastic pots (6.7-cm width × 6.7-cm length × 5.7-cm height) filled with vermiculite (Sun Gro Horticulture). Approximately 4 days after sowing and 1 week after inoculation, the pots were fertilized with a 20:20:20 NPK fertilizer @ 5 g/L of water. After sowing, pots were kept in a greenhouse maintained at 19°C–22°C with a photoperiod of 16 h facilitated by supplemental lighting. Approximately nine-days old seedlings were inoculated with a 15 mg urediniospores/0.75 ml Soltrol 70 lightweight mineral oil (ConocoPhillips Inc.) suspension inside a gelatin capsule from selected P. hordei isolates. Inoculations were facilitated by a custom rust inoculator (St. Paul machine shop, University of Minnesota) pressurized by an air pump (30 kPa). After inoculation, plants were placed under a fume hood to allow the oil to evaporate for 20 min. Plants were then placed in a dew chamber where humidity was maintained by ultrasonic humidifiers (V5100NS; Vicks) turned on for 2 min every 15 min for 16 h without light. Then, 400 W high-pressure sodium vapor lamps (LR217718; Kavita Canada Inc.) were turned on above the dew chambers that possessed a transparent plastic roof, allowing light penetration to the plants. After 2 h, the doors of the dew chambers were opened, and the plants were moved to a greenhouse maintained at 19°C–22°C with a photoperiod of 16 h facilitated by supplemental lighting.
Disease scoring
Rust responses in Australia and United States were recorded using a “0”–“4” infection type scale (“0”-“hypersensitive flecks”; no sporulation and “1”–“4” increasing sporulation in the pustules) proposed by Park and Karakousis (2002). Plants were scored when the susceptible control Gus reached infection type (IT) of “3+” (typically 8–10 days after inoculation).
DNA extraction, genotyping and targeted genotyping by sequencing
Genomic DNA was extracted from leaf tissues of single plants of F3 families for all three populations using a CTAB (Cetyl Trimethyl Ammonium Bromide) protocol (Fulton et al., 1995). Concentration of DNA was determined by using spectrophotometer (NanoDropTM, Biolab, Melbourne, VIC, Australia) and quality was determined by running all the samples on 0.8% agarose gel. To rule out the possible presence and involvement of Rph7 and Rph15, all three AGG lines used as resistant parents in this study were genotyped with Indel markers closely linked to Rph7 (Dracatos et al., unpublished) or a highly diagnostic KASP marker for Rph15 (Chen et al., 2021). DNA from individual plants of F3 families from each of the three populations (AGG-396/Gus, AGG 397/Gus and AGG-403/Gus) showing monogenic inheritance with pathotype 5457 P+ was diluted to 100 ng/μl and sent to Agriculture Victoria, AgriBio Bundoora, Australia, for targeted genotyping by sequencing (tGBS) analysis on a fee-for service basis.
Development of molecular markers (KASP and CAPS) for AGG-396/Gus
The target region identified through tGBS for the AGG-396/Gus F3 mapping population was enriched with both KASP and CAPS (Cleaved Amplified Polymorphic Sequences) markers. For KASP markers, SNPs identified in the target region were used directly to develop KASP assays by designing two allele-specific forward primers and one common reverse primer or vice versa using batch primer 3.1
KASP assays were performed in 96 well plates with an 8 μl reaction volume containing 4 μl of genomic DNA (10 ng/ul), 3.89 μl KASP mix (LGC Biosearch Technologies) and 0.11 μl of primer mix. All KASP reactions were conducted using a real time PCR machine-CFX96 (Biorad, United States) with 94°C for 15 min, 10 touchdown cycles at 94°C for 20 s, 65 to 58°C (reducing 0.6°C /cycle) and 35 cycles at 94°C for 20 s, 1 min at 55°C. Plates were read at 40°C and data were analyzed using allelic discrimination function.
For developing CAPS markers, sequence information for chromosome 2H based on Morex v2.0 (Mascher, 2019) was downloaded from IPK barley blast server and primers were designed to the pseudomolecules of chromosome 2H using primer 3 plus.2 PCR conditions for these markers were optimized and all markers were subsequently screened for polymorphism on both parental genotypes (AGG-396 and Gus). A 50 μl reaction was set comprising 15 μl of genomic DNA (10 ng/ μl), 10 μl Mi-Fi buffer, 0.5 μl of taq DNA polymerase (Bioline), 5 μl of each of forward and reverse primers (1.5 μM), and 14.5 μl of double distilled water. PCR conditions comprised an initial denaturation step at 95°C for 10 min, followed by 30 cycles with denaturation at 94°C for 30 s, annealing at 60°C for 30 s and extension at 72°C for 30 s. A final extension step of 10 min at 72°C was used.
The amplicons from parents (AGG-396 and Gus) were purified using Agencourt AMPure protocol- “000601v024” (Agencourt Bioscience Corporation). Forty microliters of AMPure XP was added to 40 μl of PCR product in a 96 well plate and mixed by pipetting. The samples were kept on a magnetic SPRI (solid-phase reversible immobilization) plate for 1 min. Liquid was eluted and DNA samples were washed twice with 120 μl of 70% ethanol to remove any contamination. Ethanol was discarded and samples were kept at room temperature for 5 min to evaporate any remaining liquid in the plate. One hundred microliters of 10 mM Tris (pH = 8) was added and mixed by pipetting. Samples were again kept on the SPRI magnetic plate for 1 min. Purified DNA (30 μl) was eluted and transferred to the new plate. In order to sequence, 8 μl purified PCR product was mixed with 4 μl of primer and sent to AGRF (Australian Genome Research Facility) for Sanger sequencing. For CAPS assays, PCR products were restricted using specific endonucleases as per the manufacturers protocol (New Biolab England, Australia).
All CAPS primers were first used to amplify the parental genotypes (susceptible vs. resistant parent) and CAPS primers that were successfully amplified on the parents were converted to CAPS. Out of the 75 CAPS and 15 KASP markers designed (Supplementary File Tables S1, S2), 18 (3 KASP and 15 CAPS) were polymorphic between the parents and were used to genotype all F3 progeny (n = 105) for mapping of the RphAGG396 resistance. Once flanking markers were identified, a further 600 F2 plants were genotyped with flanking markers for high resolution mapping of RphAGG396. Of the four markers that co-segregated with RphAGG396 in the high-resolution mapping population, MLoc-70 was validated on 70 Australian barley cultivars (Figure 1; Supplementary File Table S3) that are known to lack Rph14 (Singh et al., 2020).
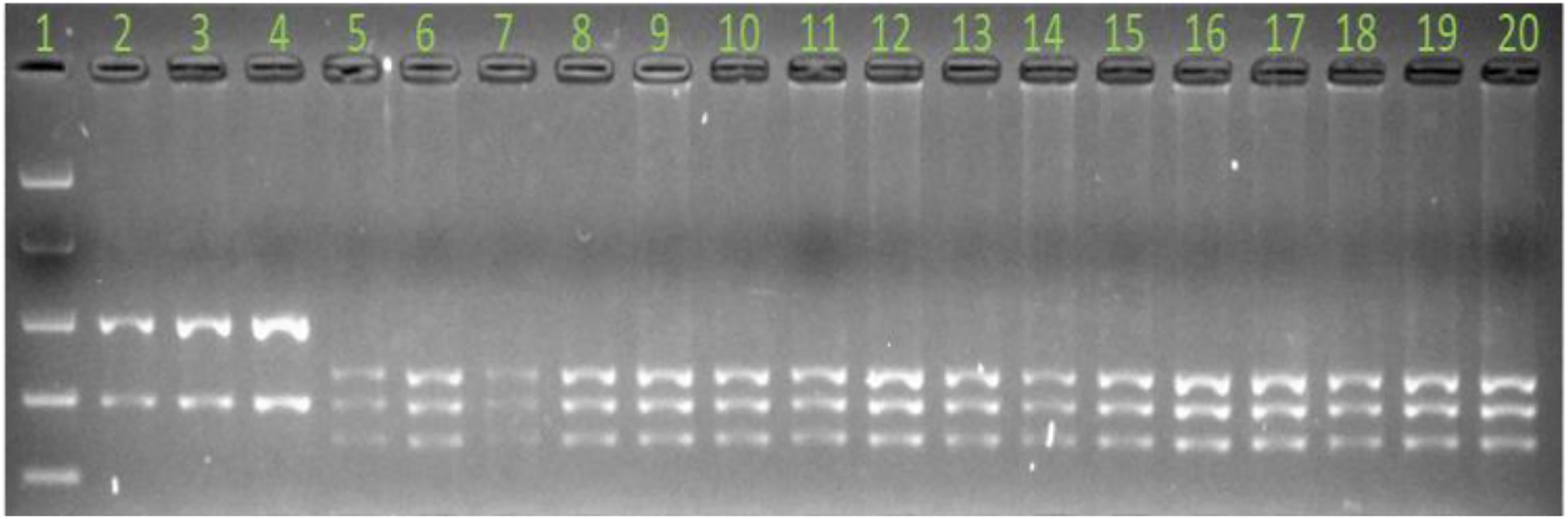
Figure 1. Marker validation on Australian barley cultivars with marker MLoc-70. Products were digested with restriction enzyme BSrI. Lane number 1 = easy ladder (Bioline). Lane numbers 2, 3, and 4 = PI 584760, Bowman + Rph14 and resistant parent AGG-396, respectively. Lane numbers 5 to 7 = Gus, Bowman and Morex, respectively, and lane numbers 8 to 20 Australian Barley cultivars. Note the presence of susceptible allele for all cultivars shown in this image relative to lanes 2, 3, and 4, which are leaf rust resistant controls.
Gel electrophoresis
Digested PCR products were loaded onto 2% agarose gels. Each gel was prepared by mixing 5 g agarose in 250 ml of TAE buffer and boiling to dissolve the mixture. The gel was cooled under running tap water and then gel red was added (1 μl per 100 ml of solution). Five microliter loading buffer [98% formamide, 10 mM EDTA (pH 8.0), 0.05% Bromophenol blue and 0.05% xylene cyanol] was mixed to 15 μl digested PCR product and was spun down. 5 μl product was then loaded to each well using 2 kb Easy Ladder (Bioline) as a size reference. Electrophoresis was carried out at 110 volts for 60 min or more depending upon the size of digested products. Separated products were visualized using Gel-Doc IT imaging system (Model-M-26, Bioimaging Systems, CA, United States). The gel was scored as A = resistant, B = susceptible and H = heterozygous. Marker genotyping data was compared with the F4 phenotype.
Sample size for initial mapping and high-resolution mapping
For initial mapping studies, 10 to 12 seeds of each F3 family were phenotyped. DNA was extracted from single leaf of each F3 family, and that DNA was used for marker genotyping. A single seed from each family was then advanced to the F4 generation and marker genotyping data was plotted against F4 phenotype. In case of RphAGG396 mapping, all F3 families (n = 105) were used for phenotyping and genotyping. For fine mapping studies, 600 F2 plants were phenotyped and genotyped.
Statistical analysis
F3 families were scored as non-segregating resistant (NSR—when all plants of individual family were resistant), non-segregating susceptible (NSS—when all plants of individual family were susceptible), or segregating (Seg—when both resistant and susceptible plants were found within a family). The data obtained were subjected to Pearson’s Chi-squared analysis (χ2) at significance level α = 0.05 to determine the goodness-of-fit of the observed ratios with expected genetic ratios. Online calculator Quickcalcs (GraphPad Software Inc., United States) was used to determine the p-values from χ2 values.
For tGBS mapping, the genotype calling trimmed sequence data was aligned against the IBSC genome assembly of Morex v1.0 using Nuclear software (GYDLE Inc.) to map each read to the best possible chromosome location. Associations were reported when at least 80% of the read length aligned, where three mismatches were tolerated in a window of 70 bases (i.e., ~96% identity). Genotypes were called when a SNP (Single Nucleotide Polymorphism) had a minimum read depth of 4, for SNPs that occurred in at least 4 samples, using custom perl scripts (a version 4.2 vcf file was generated). A marker was considered to be putatively linked with the target trait when one of the following criteria was met: minimum 50% call rate and minimum 70% allele fixation in the resistant progeny lines (i.e., fixed in resistant only); the susceptible progeny lines (i.e., fixed in susceptible only); minimum 50% call rate and minimum 70% allele fixation across each of the resistant and susceptible progeny lines (i.e., fixed in resistant and susceptible).
CAPS and KASP markers were designed within interval of 39 to 57 Mb (Mega bases) using Morex reference genome assembly v2.0. Sequence information of polymorphic KASP and CAPS primers is given in Tables 2, 3, respectively. The nucleotide sequences for both parents were analyzed for SNPs using Sequencher 5.1 software (Gene Codes, Ann Arbor, MI, United States). The identified SNPs were further subjected to dCAPS (Derived Cleaved Amplified Polymorphic Sequences) Finder 2.0 to identify restriction endonucleases.
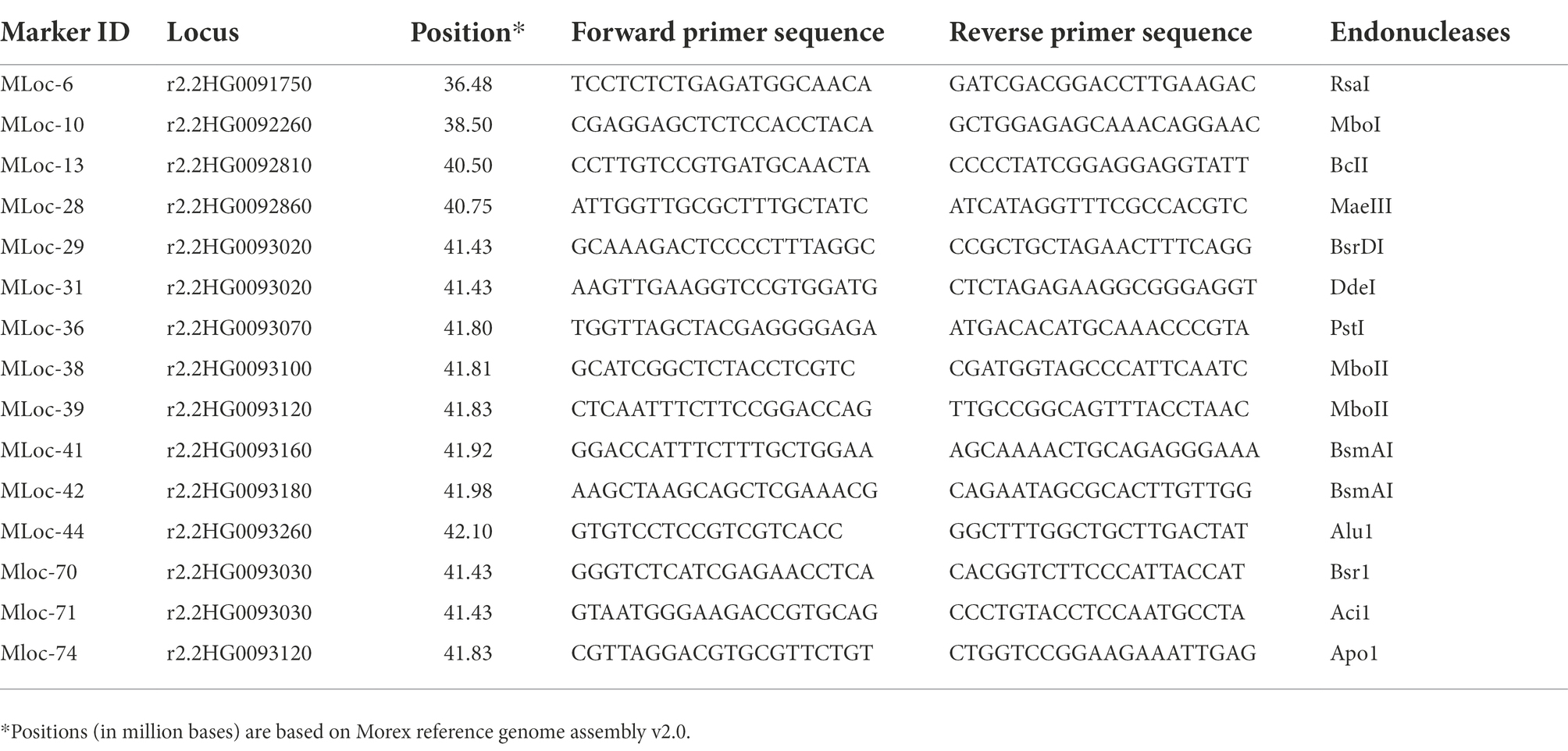
Table 3. Sequence information for polymorphic CAPS primers and respective restriction enzymes used to map RphAGG396.
Results
Infection type response and genotyping of the parents
The three resistant parents investigated in this study produced very low to intermediate ITs, while the susceptible parent Gus produced a high IT with eight Australian pathotypes (Table 4). The parental line AGG-397 showed relatively lower infection type compared to AGG-396 and AGG-403 with pathotypes 200 P− and 220 P+ suggesting the presence of an additional gene in AGG-397. All three resistant parents carried susceptibility alleles when genotyped with markers linked to Rph7 (Dracatos et al., unpublished) and Rph15 (Chen et al., 2021), supporting the likely absence of Rph7 and Rph15 (effective against all known Australian pathotypes) in the resistant parents (Supplementary File Table S4).
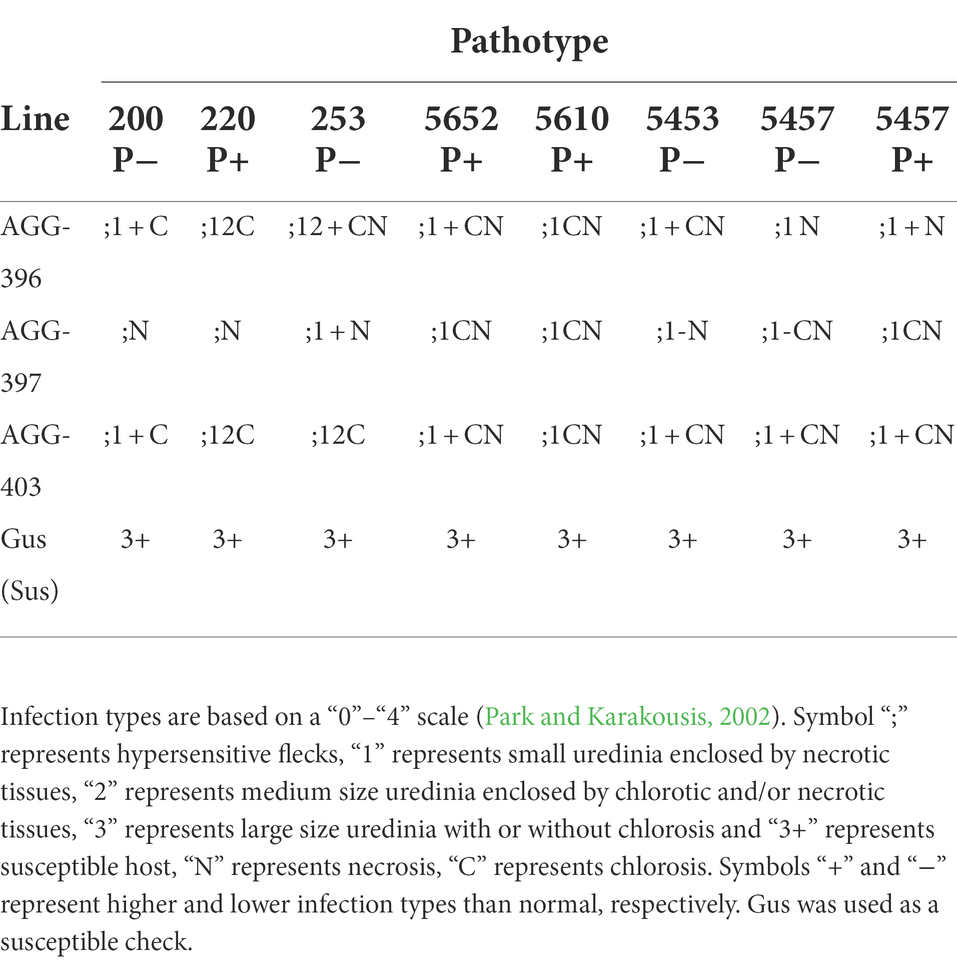
Table 4. Infection types produced by AGG-396, AGG-397, and AGG-403 when tested against eight Puccinia hordei pathotypes in the greenhouse.
Phenotyping and genetic analysis of mapping populations
The F1s derived from three crosses showed infection type similar to that of the respective resistant parents involved indicating that the resistance in all three lines is dominant. The F3 families derived from all three populations segregated for resistant (;CN to;12CN) and susceptible (3+) IT responses when tested with pathotype 5457 P+. Chi squared analysis of phenotypic data of F3 families revealed a goodness of fit of the data for a single gene segregation conforming to a 1:2:1 (NSR:Seg:NSS) genetic ratio (p > 0.6 to 0.8) in all three populations (Table 5). The pooled analysis based on resistant (R) and susceptible (S) individuals within segregating lines showed goodness of fit for a 3R:1S ratio in all three populations (AGG-396/Gus = 603R:227S, p > 0.11; AGG-397/Gus = 578R: 211S, p > 0.25; AGG403/Gus = 680R:233S, p > 0.71) confirming that resistance in all three lines is dominant. To determine if the difference in IT response of AGG-397 relative to AGG-396 and AGG-403 is due to presence of an additional gene, the F3s of AGG-397/Gus were also tested with pathotype 200 P−. The population segregated (27NSR:52Seg:23NSS) in a similar fashion with this pathotype as that with pathotype 5457 P+ and conformed to a genetic ratio (1:2:1, p > 0.8) expected for a single dominant gene.
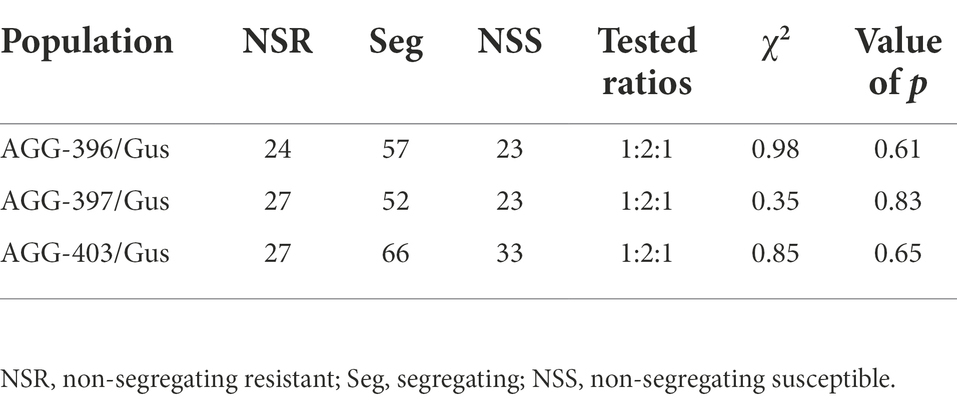
Table 5. Distribution and Chi-squared analysis of F3 families derived from crosses between AGG-396/Gus, AGG-397/Gus, and AGG-403/Gus when tested against Puccinia hordei pathotype 5457 P+ in greenhouse.
Locating genomic regions associated with resistance to Puccinia hordei
To determine chromosomal regions associated with the observed leaf rust resistance, all three populations (AGG-396/Gus, AGG-397/Gus, and AGG-403/Gus) were genotyped using tGBS. In all three populations, markers linked to resistance were detected on the short arm of chromosome 2H between 39 and 57 Mb, 44 and 64 Mb, and 31 and 58 Mb, respectively (Figure 2) based on the Morex reference assembly v.1 (Mascher et al., 2017).
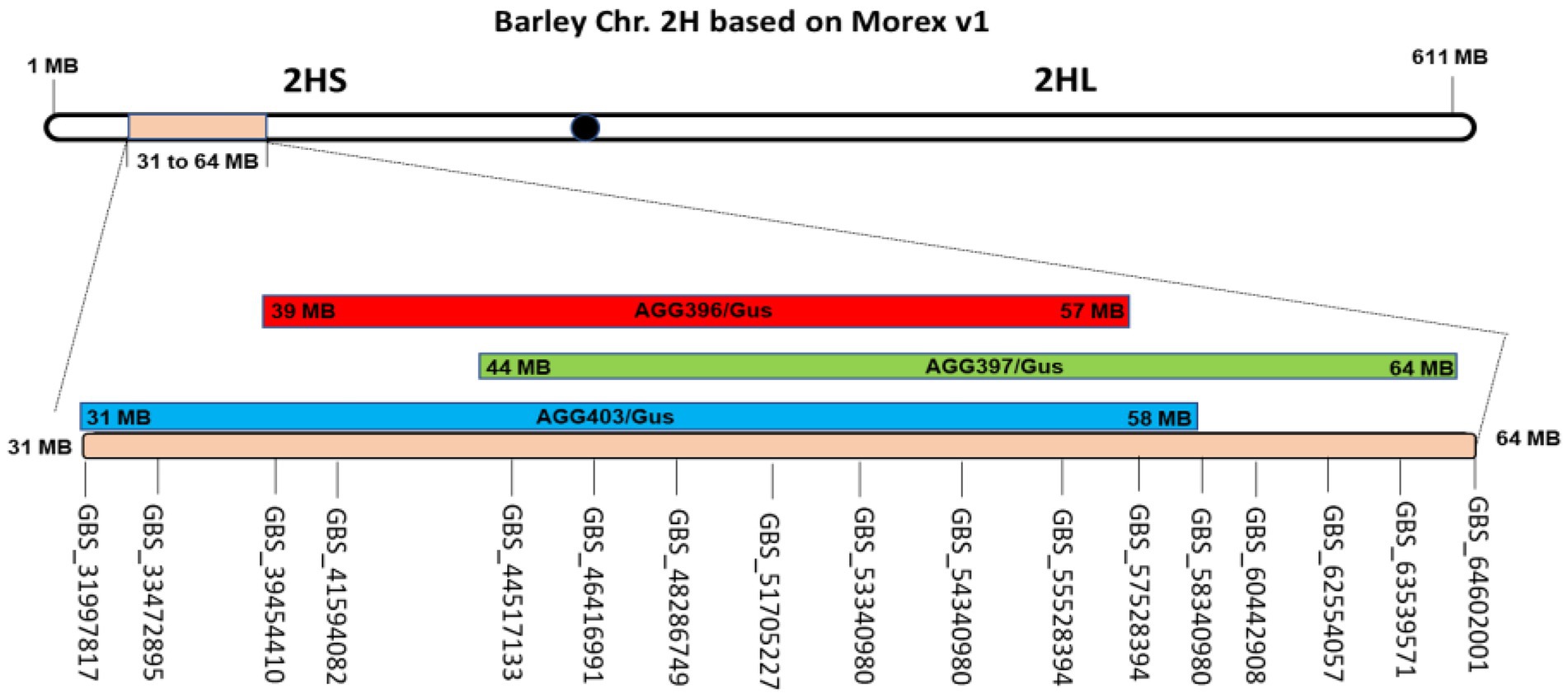
Figure 2. Genomic regions for AGG-396 (red), 397 (green), and 403/Gus (blue) F3 populations identified on the short arm of chromosome 2H through tGBS. For AGG-396/Gus, AGG-397/Gus and AGG-403/Gus, the intervals linked to resistance were found from 39 to 57, 44 to 64, and 31 to 58 Mb on 2HS, respectively.
Relationship of RphAGG396, RphAGG397, and RphAGG403 with previously reported genes on 2HS
The leaf rust resistance carried by three lines (tentatively designated RphAGG396, RphAGG397, and RphAGG403) was detected in the same region as that reported previously for genes Rph14, Rph15, and Rph16 on chromosome 2HS (Martin et al., 2020; Chen et al., 2021). The possibility of the resistance in these lines being Rph15/Rph16 (demonstrated as the same genes, Chen et al., 2021) was rejected based on all three lines being negative for a diagnostic marker for Rph15. The recently reported physical location of Rph14 (45.7–57.3 Mb) reported by Martin et al. (2020) using GBS SNPs suggested the possible presence of Rph14 in these lines.
To assess this possibility more critically, the parents AGG-396, 397, and 403 along with the Rph14 donor line-PI 584760, a line near isogenic to Bowman carrying Rph14, Bowman and Gus were phenotyped with additional P. hordei pathotypes 5610 P+ (Figure 3A) and 210 P+ (Figure 3B), both considered to carry virulence for Rph14 (Park RF, unpublished). AGG-396, 397 and 403 were all resistant (IT ranging from “;+N” to “;1+CN”) to pathotypes 5610 P+ and 210 P+, while Bowman, Bowman + Rph14 and PI 584760 were susceptible (IT “3” to “3+”) to both pathotypes indicating that the locus involved in three test lines is distinct from Rph14. To further validate these results, the three lines were sent to Minnesota, United States and tested with two additional isolates 17TX10B (avirulent on Rph14) and 17WA26B (virulent on Rph14). The tests however showed specificity allied to Rph14; all three lines being susceptible with isolate 17WA26B and resistant with isolate 17TX10B. The incompatible IT response of AGG-396, AGG-397 and AGG-403 to Australian Rph14-virulent pathotypes 5610 P+ and 210 P+ in contrast to Rph14-specific response with the North American pathotypes implied that resistance in these lines is likely conditioned either by a distinct allele of Rph14 or a gene very closely linked to Rph14 which is not effective to North American isolate 17WA26B.
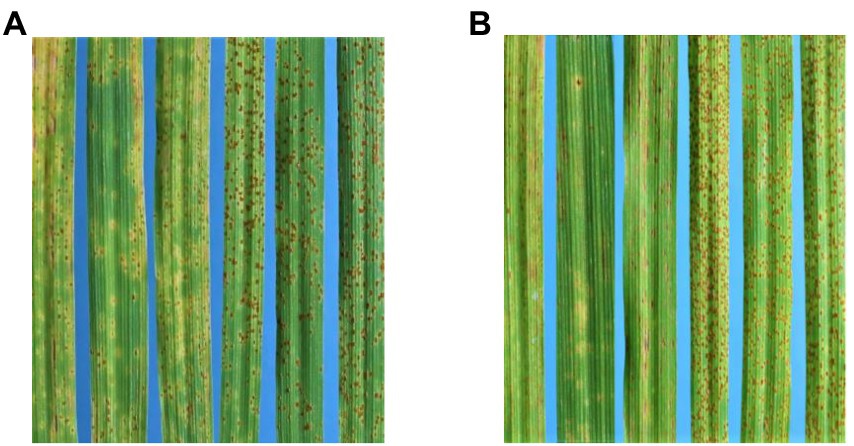
Figure 3. (A) From left to right, infection types of AGG-396, 397, 403, PI584760, Bowman + Rph14, and Bowman, respectively, with 5610 P+. (B) Infection types of AGG-396, 397, 403, PI584760, Bowman + Rph14, and Bowman, respectively, with 210 P+.
Mapping RphAGG396
Detection of the same physical region linked to resistance in AGG-396, AGG-397, and AGG-403 (based on the Morex reference genome assembly) suggested the possible involvement of the same locus in the three lines. Based on this assumption, further experiments were conducted to fine map only the resistance locus RphAGG396. This locus was preferred because it was the smallest physical region (18 Mb) compared to RphAGG397 (20 Mb) and RphAGG403 (22 Mb).
Two KASP markers, HvGBSv2-902 and HvGBSv2-932, defined a genetic distance of 3.8 cM (with 8 recombinants) proximal, and 7.1 cM (with 15 recombinants) distal to RphAGG396, respectively. The RphAGG396 locus was further saturated with 15 CAPS markers developed between the two flanking markers (Figure 4). Integration of marker data to F4 phenotypes resulted in the identification of two critical recombinants, placing RphAGG396 between two closely linked markers Mloc-28 (40.75 Mb) and Mloc-41 (41.92 Mb) at a genetic distance of 0.47 cM at proximal and distal ends (Figure 5). The physical window between the newly determined flanking markers was narrowed to a 1.17 Mb region based on the Morex v2.0 reference genome assembly (Mascher, 2019).
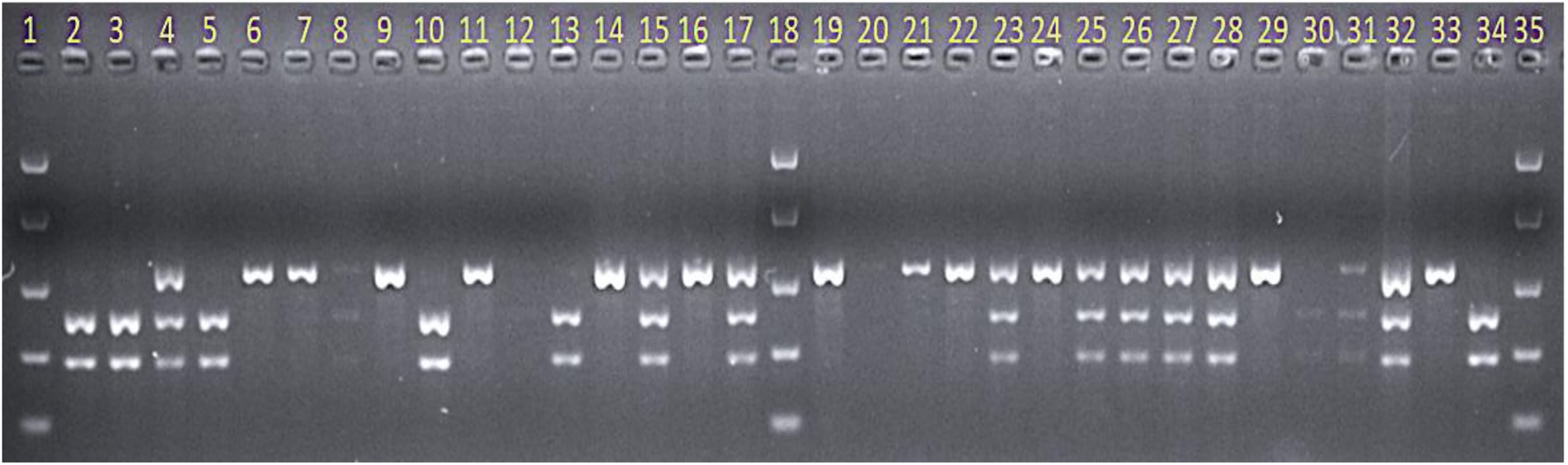
Figure 4. Gel image showing screening of the AGG-396/Gus derived F3 population with CAPS marker Mloc-39 when digested with restriction enzyme MboII. Lane numbers 1, 18, and 35 = Easy Ladder (Bioline). Lane numbers 33 and 34 = resistant parent AGG-396 and susceptible parent Gus, respectively. All other lanes show F3 progeny from the AGG-396/Gus population.
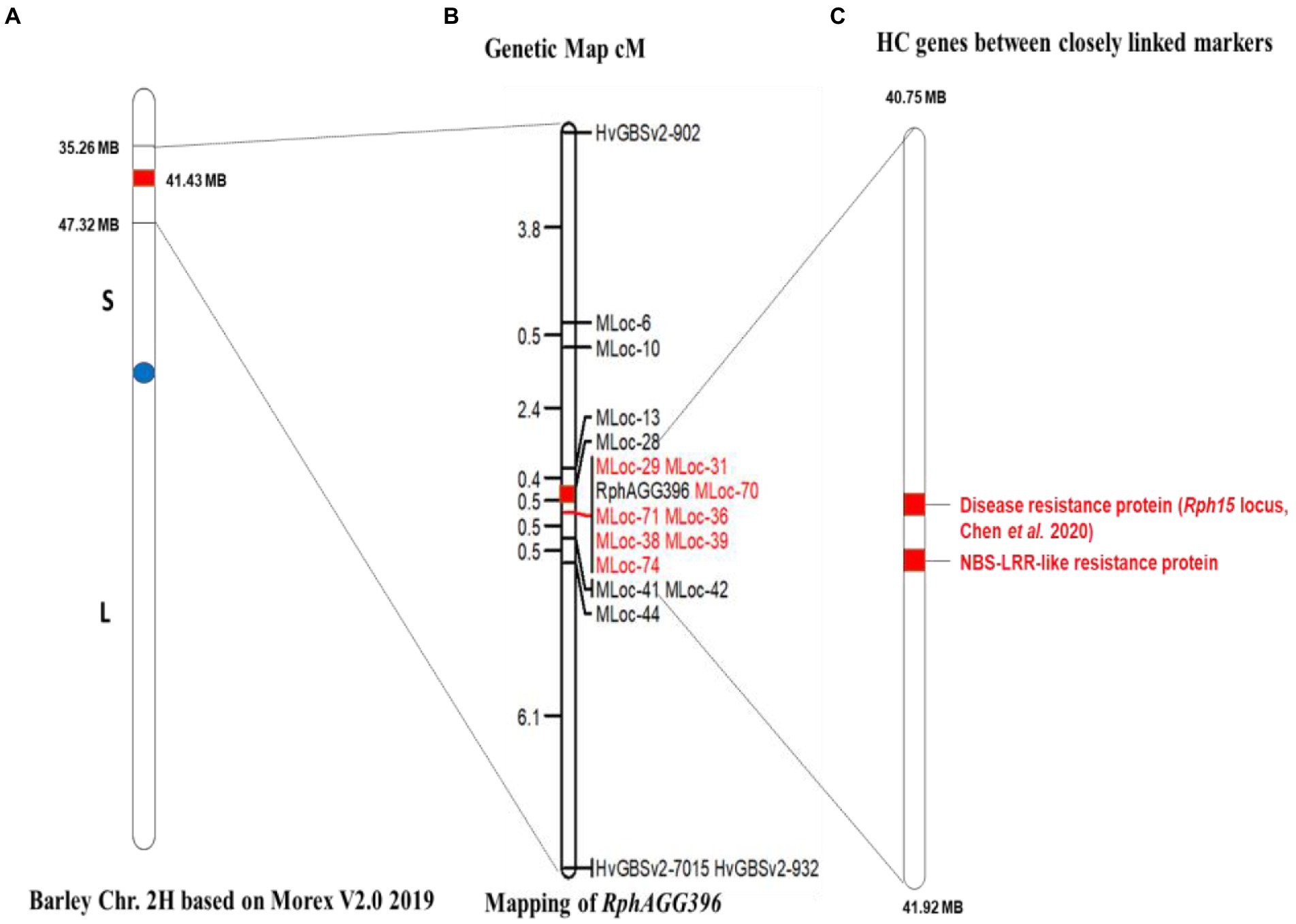
Figure 5. Genetic and physical map for RphAGG396 based on the Morex genome assembly 2019 (v2). (A) Barley chromosome 2H showing the physical window between 35 and 47 Mb for RphAGG396 based on polymorphic KASP and CAPS markers, (B) fine map of RphAGG396 placing the locus between MLoc-28 and MLoc-41 within a physical interval of 1.17 Mb. Markers co-segregating with RphAGG396 are highlighted red (C) predicted NLRs genes (red highlighted) between MLoc-28 and MLoc-41.
High resolution mapping of RphAGG396 and marker validation
In order to enhance map resolution, 600 F2 plants were genotyped with the markers Mloc-28 and Mloc-41, and nine recombinants were identified. Phenotyping and genotyping of these recombinants placed the gene at genetic distances of 0.5 and 0.2 cM between Mloc-28 and Mloc-41, respectively. Eight markers (MLoc-29, MLoc-31, MLoc-36, MLoc-38, MLoc-39, MLoc-70, MLoc-71, and MLoc-74) were found to co-segregate with RphAGG396 in a low resolution F3 mapping population (Figure 1), among which four (MLoc-29, MLoc-31, MLoc-70, and MLoc-71) co-segregated with the resistance phenotype in a high resolution F2 population (AGG396/Gus).
Marker MLoc-70, derived directly from the sequence of putative candidate gene-r2.2HG0093030 in the target interval was validated on 70 Australian barley cultivars (Supplementary File Table S3) that are considered to lack Rph14 (Singh et al., 2020). MLoc-70 amplified susceptible alleles in all 70 Australian barley cultivars and two leaf rust susceptible controls (Gus and Bowman) and the resistant allele in PI 584760, Bowman + Rph14 and AGG-396. Furthermore, the susceptible allele was amplified from Morex DNA suggesting that the RphAGG396 candidate gene may not be present in the reference genome (Figure 1).
Gene annotation
The genomic region between Mloc-28 and Mloc-41 was searched for high confidence (HC) genes using the Morex reference genome assembly v.2. The region between these markers carries 17 HC genes (Table 6). The functional annotation of these HC genes was retrieved using IPK Gatersleben.3 The annotated genes in the target interval include two NLR genes, r2.2HG0093020, which is a locus for Rph15 as reported by Chen et al. (2021), and r2.2HG0093030, which encodes an NBS-LRR-like resistance protein.
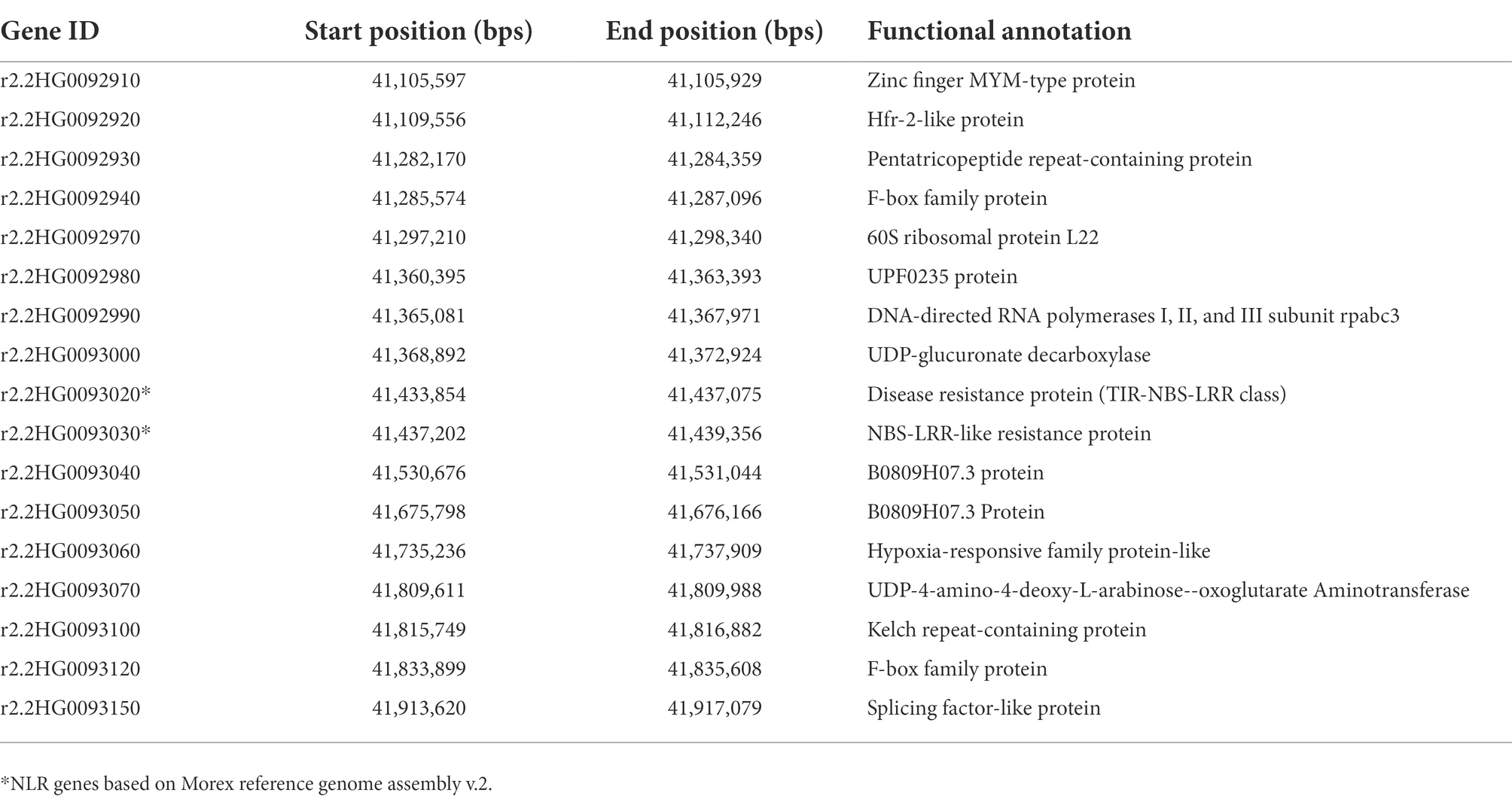
Table 6. High confidence genes annotated in the target interval between the flanking markers Mloc-28 and Mloc-41.
Discussion
This study was conducted to characterize and map leaf rust resistance in three Israeli barley lines (AGG-396, AGG-397, and AGG-403) based on previous results that suggested the lines carried uncharacterised all stage resistance (Mehnaz et al., 2021b). All three lines originated from the same geographical area and produced very similar infection types with all test pathotypes, and it was therefore hypothesized that the resistance in all three lines is likely conferred by the same resistance locus. Targeted genotype by sequencing (tGBS) conducted in this study supported our hypothesis as resistance was mapped to the same overlapping genomic region on the short arm of chromosome 2H in three mapping populations (AGG-396/Gus: 39–57 Mb; AGG-397/Gus: 44–64 Mb; AGG-403/Gus: 31–58 Mb).
Previously reported Rph genes on the short arm of chromosome 2H include Rph14 (Golegaonkar et al., 2009), Rph15 (Weerasena et al., 2004; Chen et al., 2021), Rph16 (Ivandic et al., 1998), and Rph17 (Pickering et al., 1998). Several previous studies conducted to determine the genetic relationship between the Rph genes on chromosome 2HS established that Rph14 and Rph16 are either allelic or closely linked to Rph15 (Ivandic et al., 1998; Weerasena et al., 2004) and Rph14 is independent of Rph15 (Chicaiza, 1996) or closely linked (Derevnina et al., 2015). All these studies were conducted prior to the release of the Morex reference genome assembly of barley and therefore the exact physical location of the genes was not known. However, a recent study by Martin et al. (2020) placed Rph14 and Rph15 on 2HS from 45.7 to 57.3 Mb and 44.8 to 57.3 Mb in the Morex reference genome v.1 based on GBS-based SNP markers. Their findings also established that Rph14 and Rph15 are closely linked, independent genes. The latest findings in this context using Illumina whole genome sequencing in donor lines for Rph14, Rph15, and Rph16 concluded that Rph14 is independent from Rph15 and that Rph15 and Rph16 are the same gene (Chen et al., 2021).
The three resistant lines investigated in this study tested negative for markers linked to Rph15 (Chen et al., 2021), therefore Rph15/16 is most probably not present in these lines. Based on different pathotypic specificity (observed with Australian pathotypes 5610 P+ and 210 P+) compared to the Rph14 donor accession (PI 584760), and on other hand Rph14-specificity with North American pathotypes, it can be concluded that the resistance locus in AGG-396, 397, and 403 could be an allele of Rph14 or an independent locus which is not effective against the Rph14-virulent North American pathotype used. However, further confirmation is recommended through a test of allelism between the three AGG lines and Rph14 donor stock. Sequencing of AGG lines and PI 584760 (Rph14) may also help in revealing any differences at nucleotide level and understanding the nature of the underlying resistance.
In this study, we successfully fine mapped RphAGG396 and narrowed down the physical region to 1.17 Mb. Functional annotation of the genes in the identified target region detected 17 high confidence genes including two NLR genes (r2.2HG0093020 and r2.2HG0093030). Among various known classes for resistance genes in plants, the most prevalent and commonly identified class of genes providing resistance to several pathogens encode immune receptor proteins that contain a nucleotide-binding site (NBS) and a leucine-reach repeat domain (referred to as NLRs; Dangl and Jones, 2001; Tan and Wu, 2012). Most disease resistance genes in cereals isolated so far belong to this class of gene, for example, Rph1 (Dracatos et al., 2019) and Rph15 (Chen et al., 2021) in barley and leaf rust resistance genes Lr1, Lr10, and Lr21 (Feuillet et al., 2003; Huang et al., 2003; Cloutier et al., 2007), stem rust resistance genes Sr33, Sr35, and Sr50 (Periyannan et al., 2013; Saintenac et al., 2013; Mago et al., 2015) and yellow rust resistance genes Yr5, Yr10, and Yr27 (Liu et al., 2014; Marchal et al., 2018; Athiyannan et al., 2022) in wheat.
The two annotated NLR genes (r2.2HG0093020 and r2.2HG0093030) were identified as the best candidates for RphAGG396, the former was however reported as Rph15 (Chen et al., 2021). Martin et al. (2020) reported that Rph14 and Rph15 are closely linked genes between 45 and 57 Mb in reference genome assembly v.1. The two markers (MLoc-29 and MLoc-31) designed to the CDS (coding sequences) of NLR gene-r2.2HG0093020 and further two (MLoc-70 and MLoc-71) designed to the CDS of second NLR gene-r2.2HG0093030 co-segregated with RphAGG396 in a high-resolution mapping population. Therefore, these two NLRs could be candidate genes for RphAGG396. As previous studies also reported that both Rph14 and Rph15 are tightly linked genes (Derevnina et al., 2015; Martin et al., 2020; Chen et al., 2021) and the CDS of two identified NLR genes in this study are at 5 kb distance from one another in Morex reference genome v.2, it was not surprising that all four markers co-segregated with both of these NLR genes. It is probable that there are cultivar specific genes possibly not present in Morex that may represent both Rph14 and RphAGG396. NLRs clusters in genomes evolve mainly through divergent evolution and lineage specific duplication events (Michelmore and Meyers, 1998; Zhong et al., 2018).
Of four co-segregating markers, MLoc-70 was validated on 70 Australian barley cultivars (Supplementary File Table S3) that are believed to lack Rph14 (Singh et al., 2020). The susceptible allele was amplified in all 70 barley cultivars and the leaf rust susceptible controls Gus and Bowman, while the resistance allele was amplified in PI 584760, Bowman + Rph14 and the resistant parent AGG-396, suggesting that this marker is highly predictive for RphAGG396 and can be used for marker-assisted selection.
Data availability statement
Existing datasets are available in a NCBI GenBank publicly accessible repository (https://www.ncbi.nlm.nih.gov). Publicly available datasets were analyzed in this study. This data can be found at: https://www.ncbi.nlm.nih.gov - accession numbers OP584888, OP584889, OP584890, OP584891, OP584892, OP584893, OP584894, OP584895, OP584897, OP584898, OP584899, OP584900, OP584901, and OP584902.
Author contributions
MM lead the studies. DS designed the studies. DS, PMD, and RP supervised the studies. RP provided all Australian pathotypes. MM and DS performed phenotyping and data analysis and wrote the manuscript. MR conducted phenotyping in United States. MM, PMD, KF, and HXD performed recombination and/or tGBS analysis. All authors contributed to the article and approved the submitted version.
Acknowledgments
Authors are thankful to Matthew Williams, Gary Standen, and Bethany Clark for their technical supports. Naeela Qureshi provided tGBS selective genotyping services. The facilities provided by the Plant Breeding Institute Cobbitty are gratefully acknowledged.
Conflict of interest
The authors declare that the research was conducted in the absence of any commercial or financial relationships that could be construed as a potential conflict of interest.
Publisher’s note
All claims expressed in this article are solely those of the authors and do not necessarily represent those of their affiliated organizations, or those of the publisher, the editors and the reviewers. Any product that may be evaluated in this article, or claim that may be made by its manufacturer, is not guaranteed or endorsed by the publisher.
Supplementary material
The Supplementary material for this article can be found online at: https://www.frontiersin.org/articles/10.3389/fpls.2022.980870/full#supplementary-material
Footnotes
1. ^https://probes.pw.usda.gov/cgi-bin/batchprimer3/batchprimer3.cgi
2. ^http://www.bioinformatics.nl/cgi-bin/primer3plus/primer3plus.cgi
References
Athiyannan, N., Abrouk, M., Boshoff, W. H., Cauet, S., Rodde, N., Kudrna, D., et al. (2022). Long-read genome sequencing of bread wheat facilitates disease resistance gene cloning. Nat. Genet. 54, 227–231. doi: 10.1038/s41588-022-01022-1
Bishnoi, S. K., Patial, M., Lal, C., and Verma, R. P. S. (2022). “Barley breeding,” in Fundamentals of Field Crop Breeding. eds. D. K. Yadava, H. K. Dikshit, G. P., Mishra, S., Tripathi. Singapore: Springer. 259–308.
Castro, A. J., Gamba, F., German, S., Gonzalez, S., Hayes, P. M., Pereyra, S., et al. (2012). Quantitative trait locus analysis of spot blotch and leaf rust resistance in the BCD47× Baronesse barley mapping population. Plant Breed. 131, 258–266. doi: 10.1111/j.1439-0523.2011.01930.x
Chen, C., Jost, M., Clark, B., Martin, M., Matny, O., Steffenson, B. J., et al. (2021). BED domain-containing NLR from wild barley confers resistance to leaf rust. Plant Biotechnol. J. 19, 1206–1215. doi: 10.1111/pbi.13542
Chicaiza, O. (1996). Genetic control of leaf rust in barley. PhD dissertation. Fargo: North Dakota State University, United States.
Cloutier, S., McCallum, B. D., Loutre, C., Banks, T. W., Wicker, T., Feuillet, C., et al. (2007). Leaf rust resistance gene Lr1, isolated from bread wheat (Triticum aestivum L.) is a member of the large psr567 gene family. Plant Mol. Biol. 65, 93–106. doi: 10.1007/s11103-007-9201-8
Cotterill, P. J., Rees, R. G., Platz, G. J., and Dillmacky, R. (1992). Effects of leaf rust on selected Australian barleys. Austral. J. Agric. Res. 32, 747–751. doi: 10.1071/EA9920747
Dangl, J. L., and Jones, J. D. (2001). Plant pathogens and integrated defence responses to infection. Nature 411, 826–833. doi: 10.1038/35081161
Derevnina, L., Singh, D., and Park, R. F. (2015). The genetic relationship between barley leaf rust resistance genes located on chromosome 2HS. Euphytica 203, 211–220. doi: 10.1007/s10681-014-1315-x
Dracatos, P. M., Bartos, J., Elmansour, H., Singh, D., Karafiatova, M., Zhang, P., et al. (2019). The coiled-coil NLR Rph1, confers leaf rust resistance in barley cultivar Sudan. Plant Physiol. 179, 1362–1372. doi: 10.1104/pp.18.01052
Feuillet, C., Travella, S., Stein, N., Albar, L., Nublat, A., and Keller, B. (2003). Map-based isolation of the leaf rust disease resistance gene Lr10 from the hexaploid wheat (Triticum aestivum L.) genome. Proc. Natl. Acad. Sci. 100, 15253–15258. doi: 10.1073/pnas.2435133100
Fulton, T. M., Chunwongse, J., and Tanksley, S. D. (1995). Microprep protocol for extraction of DNA from tomato and other herbaceous plants. Plant Mol. Biol. Report. 13, 207–209. doi: 10.1007/BF02670897
Gilmour, J. (1973). Octal notation for designating physiologic races of plant pathogens. Nature 242:620. doi: 10.1038/242620a0
Golegaonkar, P. G., Karaoglu, H., and Park, R. F. (2009). Molecular mapping of leaf rust resistance gene Rph14 in Hordeum vulgare. Theor. Appl. Genet. 119, 1281–1288. doi: 10.1007/s00122-009-1132-0
Hamwieh, A., Alo, F., and Ahmed, S. (2018). Molecular tools developed for disease resistant genes in wheat, barley, lentil and chickpea: a review. Arab J. Plant Protect. 36, 50–56. doi: 10.22268/AJPP-036.1.050056
Hickey, L. T., Lawson, W., Platz, G. J., Dieters, M., Arief, V. N., German, S., et al. (2011). Mapping Rph20: a gene conferring adult plant resistance to Puccinia hordei in barley. Theor. Appl. Genet. 123, 55–68. doi: 10.1007/s00122-011-1566-z
Huang, L., Brooks, S. A., Li, W., Fellers, J. P., Trick, H. N., and Gill, B. S. (2003). Map-based cloning of leaf rust resistance gene Lr21 from the large and polyploid genome of bread wheat. Genetics 164, 655–664. doi: 10.1093/genetics/164.2.655
Ivandic, V., Walther, U., and Graner, A. (1998). Molecular mapping of a new gene in wild barley conferring complete resistance to leaf rust (Puccinia hordei Otth). Theor. Appl. Genet. 97, 1235–1239. doi: 10.1007/s001220051015
Jost, M., Singh, D., Lagudah, E., Park, R. F., and Dracatos, P. M. (2020). Fine mapping of leaf rust resistance gene Rph13 from wild barley. Theor. Appl. Genet. 133, 1887–1895. doi: 10.1007/s00122-020-03564-6
Li, Y. I., Van, D. G., Raj, A., Knowles, D. A., Petti, A. A., Golan, D., et al. (2016). RNA splicing is a primary link between genetic variation and disease. Science 352, 600–604. doi: 10.1126/science.aad9417
Liu, W., Frick, M., Huel, R., Nykiforuk, C. L., Wang, X., Gaudet, D. A., et al. (2014). The stripe rust resistance gene Yr10 encodes an evolutionary-conserved and unique CC–NBS–LRR sequence in wheat. Mol. Plant 7, 1740–1755. doi: 10.1093/mp/ssu112
Mago, R., Zhang, P., Vautrin, S., Simkova, H., Bansal, U., Luo, M. C., et al. (2015). The wheat Sr50 gene reveals rich diversity at a cereal disease resistance locus. Nat. Plants 1, 1–3. doi: 10.1038/nplants.2015.186
Marchal, C., Zhang, J., Zhang, P., Fenwick, P., Steuernagel, B., Adamski, N. M., et al. (2018). BED-domain-containing immune receptors confer diverse resistance spectra to yellow rust. Nat. Plants 4, 662–668. doi: 10.1038/s41477-018-0236-4
Martin, M. J., Chicaiza, O., Caffarel, J. C., Sallam, A. H., Druka, A., Waugh, R., et al. (2020). Development of barley introgression lines carrying the leaf rust resistance genes Rph1 to Rph15. Crop. Sci. 60, 282–302. doi: 10.1002/csc2.20057
Mascher, M. (2019). Pseudomolecules and annotation of the second version of the reference genome sequence assembly of barley cv. Morex [Morex V.2]. Plant Genomics and Phenomics Research Data Repository (PGP), IPK Gatersleben, Seeland OT Gatersleben, Corrensstraße 3, 06466, Germany.
Mascher, M., Gundlach, H., Himmelbach, A., Beier, S., Twardziok, S. O., Wicker, T., et al. (2017). A chromosome conformation capture ordered sequence of the barley genome. Nature 544, 427–433. doi: 10.1038/nature22043
Mehnaz, M., Dracatos, P., Park, R. F., and Singh, D. (2021b). Mining middle eastern and central Asian barley germplasm to understand diversity of resistance to Puccinia hordei, causal agent of leaf rust. Agronomy 11:2146. doi: 10.3390/agronomy11112146
Mehnaz, M., Dracatos, P., Pham, A., March, T., Maurer, A., Pillen, K., et al. (2021a). Discovery and fine mapping of Rph28: a new gene conferring resistance to Puccinia hordei from wild barley. Theor. Appl. Genet. 134, 2167–2179. doi: 10.1007/s00122-021-03814-1
Michelmore, R. W., and Meyers, B. C. (1998). Clusters of resistance genes in plants evolve by divergent selection and a birth-and-death process. Genome Res. 8, 1113–1130. doi: 10.1101/gr.8.11.1113
Park, R. F. (2003). Pathogenic specialization and pathotype distribution of Puccinia hordei in Australia, 1992 to 2001. Plant Dis. 87, 1311–1316. doi: 10.1094/PDIS.2003.87.11.1311
Park, R. F., Golegaonkar, P. G., Derevnina, L., Sandhu, K. S., Karaoglu, H., Elmansour, H. M., et al. (2015). Leaf rust of cultivated barley: pathology and control. Annu. Rev. Phytopathol. 53, 565–589. doi: 10.1146/annurev-phyto-080614-120324
Park, R. F., and Karakousis, A. (2002). Characterization and mapping of gene Rph19 conferring resistance to Puccinia hordei in the cultivar “Reka 1”and several Australian barleys. Plant Breed. 121, 232–236. doi: 10.1046/j.1439-0523.2002.00717.x
Periyannan, S., Moore, J., Ayliffe, M., Bansal, U., Wang, X., Huang, L., et al. (2013). The gene Sr33, an ortholog of barley Mla genes, encodes resistance to wheat stem rust race Ug99. Science 341, 786–788. doi: 10.1126/science.1239028
Pickering, R. A., Steffenson, B. J., Hill, A. M., and Borovkova, I. (1998). Association of leaf rust and powdery mildew resistance in a recombinant derived from a Hordeum vulgare× Hordeum bulbosum hybrid. Plant Breed. 117, 83–84. doi: 10.1111/j.1439-0523.1998.tb01453.x
Rehman, S., Pal, R., and Verma, S. (2017). Proceedings of the 2nd International Workshop on Barley Leaf Diseases. Rabat, Morocco.
Richards, J. K., Friesen, T. L., and Brueggeman, R. S. (2017). Association mapping utilizing diverse barley lines reveals net form net blotch seedling resistance/susceptibility loci. Theor. Appl. Genet. 130, 915–927. doi: 10.1007/s00122-017-2860-1
Rothwell, C. T., Singh, D., Dracatos, P. M., and Park, R. F. (2020). Inheritance and characterization of Rph27: a third race-specific resistance gene in the barley cultivar Quinn. Phytopathology 110, 1067–1073. doi: 10.1094/PHYTO-12-19-0470-R
Rouse, M. N., Griffey, C. A., and Brooks, W. S. (2013). First detection of Puccinia hordei virulence to barley leaf rust resistance gene Rph3 and combination with virulence to Rph7 in North America. Plant. Dis. 97:838
Saintenac, C., Zhang, W., Salcedo, A., Rouse, M. N., Trick, H. N., Akhunov, E., et al. (2013). Identification of wheat gene Sr35 that confers resistance to Ug99 stem rust race group. Science 341, 783–786. doi: 10.1126/science.1239022
Sardar, A., Sarbaev, A., Tileubayeva, Z., Aytzhanova, M., and Galymbek, K. (2022). Assessment of barley crop infestation by leaf and stem phytopathogenic fungi. Arch. Phytopathol. Plant Protect. 55, 1102–1116. doi: 10.1080/03235408.2022.2081761
Singh, D., Dracatos, P., Derevnina, L., Zhou, M., and Park, R. F. (2015). Rph23: a new designated additive adult plant resistance gene to leaf rust in barley on chromosome 7H. Plant Breed. 134, 62–69. doi: 10.1111/pbr.12229
Singh, D., Dracatos, P., Mehnaz, M., and Park, R. F. (2020). Australian barley cultivar pedigree and leaf rust seedling and adult plant resistance genotype information. Cereal Rust Rep. Plant Breed. Instit. 17, 1–9.
Tan, S., and Wu, S. (2012). Genome wide analysis of nucleotide-binding site disease resistance genes in Brachypodium distachyon. Compar. Funct. Genomics 2012, 1–12. doi: 10.1155/2012/418208
Tomar, R. S., Singh, P., Tiwari, S., Tripathi, M. K., Singh, S., Naik, K. B., et al. (2022). Genomics approaches for restoration and conservation of agro-biodiversity. Agro Biodiv. Agri Ecosyst. Manage. 40, 273–283. doi: 10.1007/978-981-19-0928-3_14
Wani, S. H., Choudhary, M., Barmukh, R., Bagaria, P. K., Samantara, K., Razzaq, A., et al. (2022). Molecular mechanisms, genetic mapping, and genome editing for insect pest resistance in field crops. Theor. Appl. Genet. 12, 1–21. doi: 10.1007/s00122-022-04060-9
Weerasena, J. S., Steffenson, B. J., and Falk, A. B. (2004). Conversion of an amplified fragment length polymorphism marker into a co-dominant marker in the mapping of the Rph15 gene conferring resistance to barley leaf rust, Puccinia hordei Otth. Theor. Appl. Genet. 108, 712–719. doi: 10.1007/s00122-003-1470-2
Yu, X., Kong, H. Y., Meiyalaghan, V., Casonato, S., Chng, S., Jones, E. E., et al. (2018). Genetic mapping of a barley leaf rust resistance gene Rph26 introgressed from Hordeum bulbosum. Theor. Appl. Genet. 131, 2567–2580. doi: 10.1007/s00122-018-3173-8
Zhong, C., Zheng, M., and Zhu, J. (2018). Lymphoid tissue inducer - a divergent member of the ILC family. Cytokine Growth Factor Rev. 42, 5–12. doi: 10.1016/j.cytogfr.2018.02.004
Keywords: characterization, genetic resistance, KASP, NLR, RphAGG396
Citation: Mehnaz M, Dracatos PM, Dinh HX, Forrest K, Rouse MN, Park RF and Singh D (2022) A novel locus conferring resistance to Puccinia hordei maps to the genomic region corresponding to Rph14 on barley chromosome 2HS. Front. Plant Sci. 13:980870. doi: 10.3389/fpls.2022.980870
Edited by:
Sujan Mamidi, HudsonAlpha Institute for Biotechnology, United StatesReviewed by:
Prem Lal Kashyap, Indian Institute of Wheat and Barley Research (ICAR), IndiaShailendra Kumar Jha, Indian Agricultural Research Institute (ICAR), India
Copyright © 2022 Mehnaz, Dracatos, Dinh, Forrest, Rouse, Park and Singh. This is an open-access article distributed under the terms of the Creative Commons Attribution License (CC BY). The use, distribution or reproduction in other forums is permitted, provided the original author(s) and the copyright owner(s) are credited and that the original publication in this journal is cited, in accordance with accepted academic practice. No use, distribution or reproduction is permitted which does not comply with these terms.
*Correspondence: Davinder Singh, davinder.singh@sydney.edu.au
†ORCID: Davinder Singh https://orcid.org/0000-0003-1411-9291