- 1Department of Integrative Biotechnology, Sungkyunkwan University, Suwon, South Korea
- 2Department of Vaccine and Drugs, The National Research and Innovation Agency, Jakarta, Indonesia
- 3Cardiovascular Research Center, Massachusetts General Hospital, Harvard Medical School, Boston, MA, United States
- 4Division of Cardiology and Corrigan Minehan Heart Center, Massachusetts General Hospital, Harvard Medical School, Boston, MA, United States
- 5Yeom Chang-Hwan Hospital, Seoul, South Korea
- 6Humanitas College, Kyung Hee University, Yongin, South Korea
Lymphedema is a debilitating chronic disease that mostly develops as an adverse reaction to cancer treatment modalities such as chemotherapy, surgery, and radiotherapy. Lymphedema also appears to be a deteriorating consequence of roundworm infections, as best represented by filariasis. According to its origin, lymphedema is classified as primary lymphedema and acquired lymphedema. The latter is an acquired condition that, hitherto, received a considerably low attention owing to the less number of fatal cases been reported. Notably, despite the low mortality rate in lymphedema, it has been widely reported to reduce the disease-free survival and thus the quality of life of affected patients. Hence, in this review, we focused on acquired lymphedema and orchestration of molecular interplays associated with either stimulation or inhibition of lymphedema development that were, in vast majority, clearly depicted in animal models with their specific and distinct technical approaches. We also discussed some recent progress made in phytochemical-based anti-lymphedema intervention strategies and the specific mechanisms underlying their anti-lymphedema properties. This review is crucial to understand not only the comprehensive aspects of the disease but also the future directions of the intervention strategies that can address the quality of life of affected patients rather than alleviating apparent symptoms only.
1 Introduction
Lymphedema has been characterized as a morbid chronic disease, that is, typically developed following cancer treatment, mainly chemotherapy (Kim et al., 2015), radiotherapy (Daley et al., 2010; Kunkler et al., 2015), and surgery (Garza et al., 2017), and has affected 130–250 million people worldwide (Park et al., 2013). Lymphedema represents an adverse yet inevitable pathophysiological response to treatment against specific cancers, particularly breast cancer (Fu, 2014; McDuff et al., 2019), and gynecologic cancer (Lim et al., 2014; Rowlands et al., 2014; Biglia et al., 2017; Saner et al., 2019). It is a clinical manifestation of impaired lymphatic drainage (Biglia et al., 2017), which leads to the accumulation of interstitial fluid with high protein content in the affected subcutaneous tissues (Bok et al., 2018) and further induces stiffness and thickness of the tissue microstructures (Lee et al., 2020). Despite having infrequent mortality, it lowers quality of life because of the co-occurring disabilities of the affected or adjacent parts of the body, particularly the limbs. Although surgical and post-surgical therapies have enormously advanced worldwide, lymphedema is poorly overcome and limitedly managed (Saito et al., 2013). To date, no drug specifically targeting lymphedema has been licensed (Bruns et al., 2003; Gardenier et al., 2017), suggesting that the development of an efficacious anti-lymphedema is has a long way. However, according to the findings of a few in vivo and in vitro studies, altering the status of lymphedema from incurable to curable chronic disease is not impossible. Therefore, in the present review, we highlight primary molecular events or characteristics of acquired lymphedema, particularly in its association with anticancer treatments, and update to the most recent progress regarding some phytochemical-based substances that exhibit anti-lymphedema properties. Overall, the present review depicts acquired lymphedema as a long-due challenge and shows some promising opportunities for less-explored substances to contribute profoundly to the global search for specific and efficacious drugs to reverse or regulate the contra-productive effects of the disease.
2 Secondary (acquired) lymphedema
According to its origin or initial cause, lymphedema is classified as 1) primary lymphedema and 2) secondary (acquired) lymphedema, the latter of which is the focus of this article. However, we have introduced primary lymphedema also.
Primary lymphedema is a rare condition that affects approximately 1.2 patients in 100,000 patients aged below 20 years (Maclellan and Greene, 2014). This is associated with several gene mutations such as VEGFR-3 (Milroy disease), SOX18 (hypotrichosis-telangiectasia-lymphedema), CCBE (Hennekam syndrome), and FOXC2 (lymphedema distichiasis) (Maclellan and Greene, 2014; Kambhampati et al., 2016). Primary lymphedema may occur in different age groups. Congenital lymphedema occurs at an age of up to 2 years, whereas lymphedema precox and lymphedema tarda occur at an age below and above 35 years, respectively (Kambhampati et al., 2016). Although lymphedema has been initially recognized prior to more than a century, understanding of its causal aspects is considerably less progressive (Karkkainen et al., 2000).
Unlike primary lymphedema, which is a clinical manifestation of insufficient lymphatic transport, acquired lymphedema is a consequence of traumatic perturbation of the lymphatic system, mainly due to malignancy, inflammation, and infections (Rutkowski et al., 2006; Padera et al., 2016; Borman, 2018; Hong et al., 2019; Bertelli et al., 2020). Acquired lymphedema constitutes more than 90% cases of lymphedema worldwide (Greene and Goss, 2018/04).
In order to provide adequate consideration for intervention strategies, the International Society of Lymphology (ISL) has classified lymphedema based on superficial physical appearances into three stages (International Society of Lymphology, 2020) as described in Table 1. Each stage carries a particular level of severity according to the volume differences measured in the affected limb. It is considered minimal when the increase is less than 20%, moderate when the volume increases by 20%–40%, and severe when the limb volume rises by more than 40% (Tretbar et al., 2008). Lymphedema diagnosed at an early stage is conventionally treated with combined physical therapy to alleviate edema. However, this treatment is less longer effective for an advanced stage due to the presence of adipose tissue deposition (Hoffner et al., 2018) and accumulation of collagen and fibrotic tissue (Cho et al., 2017) which is irreversible and can only be removed by surgery (Hoffner et al., 2018). Anticancer treatment-induced lymphedema is barely reversible (Paskett et al., 2012), whereas obesity-induced counterpart lymphedema might have less detrimental effects when the body weight is maintained under the right proportion (Nitti et al., 2016). This leaves us with a major query on what builds up the gap between these differences with respect to the emergence of pathological features and the recovery between the two most common inducers of acquired lymphedema. Hence, we put our best efforts to tailor several prior-reported pathways associated with the development of acquired lymphedema. We also discuss the progress made so far with regard to animal models of lymphedema, one of which was developed in our laboratory. This is crucial to support the future studies focused on formulating the best and practical intervention strategies to alleviate the impacts of lymphedema in the clinical realm.
3 Clinical hallmarks of lymphedema
3.1 Perturbed lymphatic vasculature
The human lymphatic system is made up of lymphatic vessels, lymphatic organs, interstitial fluid, and migrating cells (Rovenská and Rovenský, 2011; Gavins et al., 2020). However, although the building blocks are diverse, the system works as a whole, and is an important part of the immune system (Rovenská and Rovenský, 2011).
The lymphatic vasculature is found in most organs and comprises lymphatic capillaries, collecting vessels with valves, and lymph nodes. The lymphatic capillaries are blind-ended and highly permeable, lined by a single layer of loosely connected endothelial cells; they merge into the collecting vessels and then into the thoracic duct and right lymphatic duct, and eventually empty lymph into the venous system (Oliver et al., 2020). Lymphatic development involves modification of a subpopulation of embryonic venous endothelial cells in the cardinal veins expressing prospero-related homeobox 1 (PROX1), a master transcription factor, into lymphatic endothelial cell (LEC) progenitors, which subsequently bud off from cardinal veins to form lymph sacs (Oliver et al., 2020). The activation of PROX1 induces chicken ovalbumin upstream promoter-transcription factor 2 (COUP-TF II) (Lee et al., 2008/09) and SRY box transcription factor 18 (SOX18) to initiate differentiation of LECs (Srinivasan et al., 2010). After forming the sacs, LECs sprout to produce peripheral lymphatic network, which is involved in regulation of the entire growth and remodeling of the lymphatic system (Escobedo and Oliver, 2017; Kim and Song, 2017; Oliver et al., 2020).
The lymphatic system is responsible for fluid transportation and maintenance of the overall fluid balance in the body. In the interstitial space, the fluid is at sub-atmospheric pressure. However, to reach the venous system it requires a pressure of approximately 20 cm H2O. This pressure difference is overcome by active pumping of collecting lymphatic vessels. In addition, passive vessels lead to further pumping (Moore and Bertram, 2018). However, a few causes or risk factors may impair this flow system, leading to the increased volume of interstitial fluid due to either increased inflow or decreased outflow or both; thus, the fluid balance is compromised (Ridner, 2013). This emerges as swelling (edema) and is the most common clinical symptom of lymphedema (Maclellan and Greene, 2014; Azhar et al., 2020) against which potential drugs/medicines are formulated.
3.2 Impaired lymphangiogenesis mediated by the VEGF-C/VEGFR-3 signaling pathway
Lymphangiogenesis is a process in which new lymphatic vessels are generated either from pre-existing lymphatic vessels or initial formation during embryogenesis (Varner and Schwab, 2011). This is a dynamic process during embryogenesis, but is almost absent postnatal under normal physiological conditions and only occurs in pathological conditions such as tissue remodeling, inflammation, and tumor growth (Christiansen and Detmar, 2011). It was highly associated with unfavorable clinical outcomes in tumors, particularly with respect to the recurrence-free survival rate and tumor size (Sha et al., 2019).
Under physiological conditions in adults, the lymphatic network system is remodeled into mature collecting vessels owing to the strong expression of VEGF-receptor-3 (VEGFR-3) in luminal valves and less strong in lymphangion. VEGFR-3 is a cognate ligand of vascular endothelial growth factor-C (VEGF-C) (Norrmén et al., 2011). Hence, the VEGF-C)/VEGFR-3 signaling pathway is apical to lymphangiogenesis (Veikkola et al., 2001). VEGF-C is a VEGF/platelet-derived growth factor (PDGF) family homolog with cysteine-rich C-terminal. Once it is proteolytically processed, it binds to VEGFR-3, previously termed as Fms-like tyrosine kinase-4 (FLT4) and induces autophosphorylation (Joukov et al., 1996). VEGFR-3 expression is detected in LECs, whereas VEGF-C is chemostatic and expressed by immune cell infiltrate, which is primarily a macrophage subset (Gousopoulos et al., 2017). It was reported that in mouse tail lymphedema model, VEGF-C was overexpressed by CD68+ cells, a macrophage marker, leading to the worsening of lymphedema’s most distinctive symptom, edema, and this was positively correlated with vascular leakage that initiated the fluid influx into the tissue. Blocking VEGF-C abolished edema development and vascular leakage (Gousopoulos et al., 2017).
VEGFR-3 alone was shown to be sufficient to induce lymphangiogenesis, as suggested by Veikkola et al., who developed transgenic mice overexpressing a VEGFR-3-specific mutant of VEGF-C (VEGF-C156S) or VEGF-D in epidermal keratinocytes using the keratin 14 promoter. In this study, lymphatic vessels in the skin were observed to be induced by both transgenes without affecting the architecture of the adjacent blood vessels (Veikkola et al., 2001).
During the state of lymphedema, lymphangiogenesis is perturbed, which results in the accumulation of protein-rich interstitial fluid, and thereby swelling, in certain sites of the body. Subsequently, the fluid induces inflammatory responses, which further initiate fibrosis, adipocyte deposition, and impaired immune response and wound healing (Norrmén et al., 2011). Therefore, in the treatment of cancer, where lymphangiogenesis facilitates the spread of cancerous cells into lymph nodes, should be abrogated; therefore, anti-inflammatory drugs such as docetaxel are generally used as a therapeutic strategy. However, the use of docetaxel, being toxic, as an adjuvant chemotherapy has been reported to be associated with a high risk of lymphedema in some breast cancer cohorts (Harris et al., 2018). This is intriguing yet conflicting because lymphangiogenesis is unfavorable in cancers but favorable in lymphedema; moreover, we agreed that lymphedema itself is an adverse consequence of anticancer treatment as described earlier in this review. This strongly indicates a potential molecular crosstalk between lymphangiogenesis and tumorigenesis (Gomes et al., 2013; Lee et al., 2015), which is of major interest, particularly with regard to developing drugs against cancer without triggering lymphedema or drugs that negate the emerging clinical hallmarks of lymphedema (Anton and Rockson, 2019). As described earlier that lymphangiogenesis is initiated through the VEGF-C/VEGFR-3 signaling pathway, VEGF-C and VEGFR-3 are two main targets proposed in both synthetic-based and phytochemical-based drug platforms, which are discussed in this article (6. Phytochemical-based substances with anti-lymphedema properties).
A selective VEGFR-3 tyrosine kinase inhibitor, SAR131675, was shown to inhibit lymphangiogenesis in both in vitro and in vivo studies through regulation of the pro-inflammatory chemokine-expressing M1 macrophages, which further leads to decreased fibrosis (Hwang et al., 2019). Although the VEGF-C/VEGFR-3 axis is crucial for lymphangiogenesis to enable the trapped lymphatic fluid to re-flow properly, this might trigger a contra-productive clinical manifestation since lymphangiogenesis is supportive for cancer metastasis. This is certainly of concern for researchers and clinicians worldwide because the vast majority of patients with acquired lymphedema are receiving treatment for various stages of cancer. This urges further studies focusing not only on the crosstalk of lymphangiogenesis in simultaneous cancer and lymphedema, but also on drugs targeting other than lymphatic-related development. Anti-fibrosis and anti-adipogenesis are the two most proposed strategies, which are elucidated in the following sections.
3.3 Excessive fibrosis
In addition to lymphangiogenesis, fibrosis is a major hallmark of acquired lymphedema. Fibrosis and lymphangiogenesis are in opposite direction. While lymphangiogenesis is favorable for alleviating lymphedema, fibrosis is one of critical players in lymphedema. Both differ in terms of dependency on the VEGF-C/VEGFR-3 gradient. Fibrosis is a manifestation of excessive accumulation of extracellular matrix, mainly collagen, which subsequently harden the sites of wounds or injuries, inducing chronic inflammatory responses (Wynn, 2008). In contrast to lymphangiogenesis, that is, greatly suppressed in lymphedema and occurs due to the involvement of both VEGF-C and VEGFR-3, fibrosis was modulated in a VEGF-C-independent manner as demonstrated by Avraham et al. (2009) and earlier by Rutkowski et al. (2006), where only weak expression of VEGF-C was detected in the swollen tail of murine models (Avraham et al., 2009). Fibrosis is widely known as a key inhibitor of the tissue fluid accumulation which causes tissue expansion. This is a barrier to lymphangiogenesis (Avraham et al., 2009). It causes accumulation of collagen (Ghanta et al., 2015) and α-smooth muscle actin (α-SMA) around the podoplanin-expressing collecting lymphatic vessels (García Nores et al., 2018) takes a longer time than that of the interstitial fluid which results in a delayed lymphedema (Ghanta et al., 2015).
Fibrosis development is strongly associated with the M2-phenotype of macrophages (Ghanta et al., 2015) and Th2-skewed inflammatory response of CD4+ T cells (García Nores et al., 2018). M2-macrophages promote fibrosis by releasing transforming growth factor (TGF)-β1 as a profibrotic cytokine (Ghanta et al., 2015). In addition, they produce cytokines such as IL-13, IL-10, IL-4, tumor necrosis factor-α (TNF-α), and IL-1 (Van Linthout et al., 2014) which synergistically activate fibroblasts, recruit myofibroblasts, and exacerbate inflammatory cell infiltration to the injured site (Van Linthout et al., 2014; Ghanta et al., 2015). TNF-α, in particular, is shown to positively loop feedback the production of TGF-β1 via a kinase-specific pathway. However, the nuclear factor-κB (NF-κB) pathway is profoundly involved in the production of pro-inflammatory cytokines (Van Linthout et al., 2014). The significance of M2-macrophages in fibrosis is evident as their depletion ameliorates fibrosis. Notably, the depletion is selective and not general; during progressive injury, macrophage depletion leads to reduced fibrosis (Duffield et al., 2005), which is a favorable clinical feature in any of the proposed anti-lymphedema strategies. However, in the recovery state, this depletion is unable to overcome the recruitment of cellular and matrix components of the fibrotic response (Duffield et al., 2005), thus increasing fibrosis and accumulation of CD4+ T cells. In contrast, VEGF-C expression is weakened, suggesting macrophages as a source of VEGF-C along with LECs (Ghanta et al., 2015).
The CD4+ T cell subset plays a crucial role in fibrosis similar to that in M2-macrophages. This perspective was triggered following a critical finding by Nierdermeier et al. (2009) that fibrocyte differentiation was determined by the presence of CD4+ T cells. Fibrocytes are collagen-type I producing hematopoietic cells. Activation of the CD4+ T cell subset initiates the release of some soluble cytokines such as TNF, IL-4, IFN-γ, and IL-2, which prevent massive growth of fibrocytes. Interestingly, in the presence of calcineurin inhibitors and absence of mTOR inhibitors, when CD4+ T cells were in their activation state, marked collagen I deposition was detected, indicating the steady growth of fibrocytes. These results suggest that the activation of CD4+ T cells determines the fate of fibrocytes. This finding was supported by Tapmier et al. (2010), who demonstrated that depletion of CD4+ T cells using monoclonal antibodies was observed to significantly decrease the interstitial expansion and collagen deposition in a ureteric-obstructed RAG knock-out murine model setting. As such, CD4+ T cell blocking is considered a potential strategy for anti-fibrosis intervention. More recently, collagen III was also indicated to play a role in fibrotic skin of grade 3 lymphedema with the gene transcription level was detected much higher up to 39 fold than that seen for collagen I (Karayi et al., 2020). This bulks up potential targets of anti-lymphedema intervention although much more in-depth studies are necessitated to discern underlying mechanisms of both activation and regulation of collagen III from gene to protein levels and potential functional exchange between collagen I and collagen III.
In the global efforts to seek a cure for acquired lymphedema, the overall orchestration of fibrosis has recently received a lot of attention. Unlike targeting lymphangiogenesis, which not only allows re-building of the previously impaired lymphatic system but also re-promote cancer cell metastasis (Schlereth et al., 2014), targeting fibrosis is considered more appealing because it is an unfavorable prognostic factor for both cancer and lymphedema; hence, fibrosis suppression is expected to improve the outcomes for patients with cancer (Chandler et al., 2019) as well as for those suffering from lymphedema post cancer treatment (Gardenier et al., 2017; Mehrara et al., 2021).
3.4 Adipogenesis
One aspect that causes acquired lymphedema to be less likely, if not impossible, to cure is its progression cycle. The regions initially affected by lymphedema expand outwards to the skin through neighboring fatty tissues, resulting in concomitant changes in the lymphatic system and adipose tissue that must be focused to address lymphedema (Tashiro et al., 2017).
Adipose tissue is a lobulated septal network niched to diverse cell types, including adipocytes, fibroblasts, vascular endothelial cells (VECs), vascular smooth muscle cells, and immune cells mainly macrophages and lymphocytes (Tashiro et al., 2017). As represented by specialized organelles inside adipocytes, adipose tissue is responsible for the storage of triglycerides and cholesterol esters in the form of lipid droplets. Therefore, changes in the amount of lipids stored in adipocytes affect the fat cell size (DM et al., 2007). Adipocytes, the predominant cells residing in adipose tissue, are generated through a process termed adipogenesis, in which differentiation and maturation of adipocytes from mesenchymal stem cells (MSCs) are facilitated (Lane and Lennarz, 2013). This is a biphasic process and necessitates cell-cell communication via surface molecules, extracellular matrix, and direct contact. When preadipocytes completely develop into adipocytes, the cells use extracellular matrix to make envelops; thus, direct cell-cell contact is no longer possible (Schling and Löffler, 2002).
Transcriptional regulators modulate Adipogenesis. Zfp423 is a multi-zinc finger transcription factor that accounts for cell commitment to the adipogenic lineage (Gupta et al., 2012) and has exceptionally dense CpG sites in its promoter (Yang et al., 2013). This factor regulates peroxisome proliferator-activated receptor-γ (PPARγ), which is required for the formation of white and brown adipose tissue in the body, and CCAAT-enhancer-binding protein (C/EBPs) to facilitate differentiation of preadipocytes into adipocytes (Gupta et al., 2012). Zfp423 activation is facilitated via the bone morphogenetic protein (BMP)/Caenorhabditis elegans SMA and mothers against decapentaplegic (SMAD) signaling pathway (Bond et al., 2018) and the overall functions are mainly controlled by Wnt1-inducible signaling pathway protein 2 (WISP2), which forms a complex with the Zfp423 molecules in the cytoplasm. BMP/SMAD signaling enables dissociation of the WISP2-Zfp423 complex and Zfp423 enters the nucleus. Subsequently, transcriptional activation of some downstream genes is triggered (Yang et al., 2013; Bond et al., 2018; de sá et al., 2017).
In acquired lymphedema, adipogenesis is dramatically upregulated, causing the affected tissue to be suffused with adipocytes, further upregulating fat differentiation markers (Azhar et al., 2020) such as PPARγ, C/EBP-α/β, and FABP4 (Lowe et al., 2011; Ji et al., 2014; Roh et al., 2017a; Roh et al., 2020; Koc et al., 2021). Therefore, adipogenesis, along with other superficial clinical hallmarks, is a promising target for the treatment of lymphedema. These ideas have attracted increased attention after the achievement of successful treatment along with prolonged survival rate and improved prognosis by blocking or modifying adipogenesis activation-associated markers in some cases of cancer and obesity, particularly in women (Yang et al., 2013; Wang et al., 2017; Wu et al., 2019; Oshi et al., 2021).
4 Risk factors of lymphedema
4.1 Anticancer treatments
4.1.1 Chemotherapy
Chemotherapy is, thus far, the mainstay of cancer treatment; however, in some cases, it fails to elevate the quality of life of the patient receiving chemotherapy. Chemotherapy is associated with the fluid retention in extremities. Although direct mechanisms linking chemotherapy and emergence of lymphedema is yet to be delineated but the association of both is evident, particularly that of taxane-based (Qin et al., 2011; Swaroop et al., 2015) which was reported to increase 2-years accumulative incidence of lymphedema to 22.76% which was much higher than the incidence found in non taxane based treatment (Swaroop et al., 2015). Hidding et al. (2018) demonstrated that after completion of adjuvant treatment with docetaxel, doxorubicin, and cyclophosphamide, breast cancer patients developed lymphedema and experienced significant decline in physical function because of the increased swelling, heaviness, and volume changes in the upper arm. Similar concerns were also reported for patients receiving paclitaxel (Swaroop et al., 2015). Later, Kim et al. reported an increased propensity of node-positive breast cancer patients treated with adjuvant chemotherapy across types (Kim et al., 2015).
Despite these widely reported long-term adverse effects, chemotherapy is still regarded as the most common regimen for both solid and blood cancers because it is undeniably capable of inducing cell death via apoptosis, programmed cell death, or necrosis characterized by accidental cell death. While apoptotic cancer cells are specifically and markedly detected by tagging small portions of the cells with a few sets of molecular markers, the necrotic counterpart cells lack these markers (Ricci and Zong, 2006). Nonetheless, necrotic cell death activates tissue inflammation in the adjacent tissue in response to the release of intracellular materials termed damage-associated molecular patterns (DAMPs). Subsequently, pro-inflammatory cytokines, chemokines, and adhesion molecules are produced (Yang et al., 2015); hence, the balance of Th1/Th2 immune response is perturbed and skewed into Th2 that triggers a phenotypic switch of macrophages to M2 macrophages (Dijkgraaf et al., 2013). Following this shift, either VEGF-C or VEGFR-3 is downregulated and lymphatic function is impaired (Yeh et al., 2017; Tacconi et al., 2019).
Macrophages are immune cell subsets that are markedly upregulated at the site of lymphedema (Duan et al., 2016). Macrophages polarize into either pro-inflammatory M1 macrophages or anti-inflammatory or reparative M2 macrophages, based on the circulating signals they receive from their microenvironment (Mishima et al., 2017). Among these two phenotypes, M2 macrophages are responsible for lymphangiogenesis (Yang et al., 2015); thus, it is a major focus in the search of anti-lymphedema drugs.
It was observed that tumors co-implanted with macrophages derived from paclitaxel-treated tumor-bearing mice regressed the phenotype of lymphatic vessels. This regression was detected to a lesser extent in paclitaxel + VEGFR-3 specific antibody (31C1)-treated counterpart mice. Although the difference was not statistically significant, this indicated that the phenotypic changes of lymphatic vessels observed in tumors after co-implantation were mediated by VEGFR-3-expressing macrophages (Alishekevitz et al., 2016).
4.1.2 Radiotherapy
Radiotherapy produces synergetic effect with chemotherapy, and it is also a predictor of lymphedema emergence (Warren et al., 2015). The therapy is designed to destroy targeted cancer cells via direct or indirect pathways, as illustrated in Figure 1.
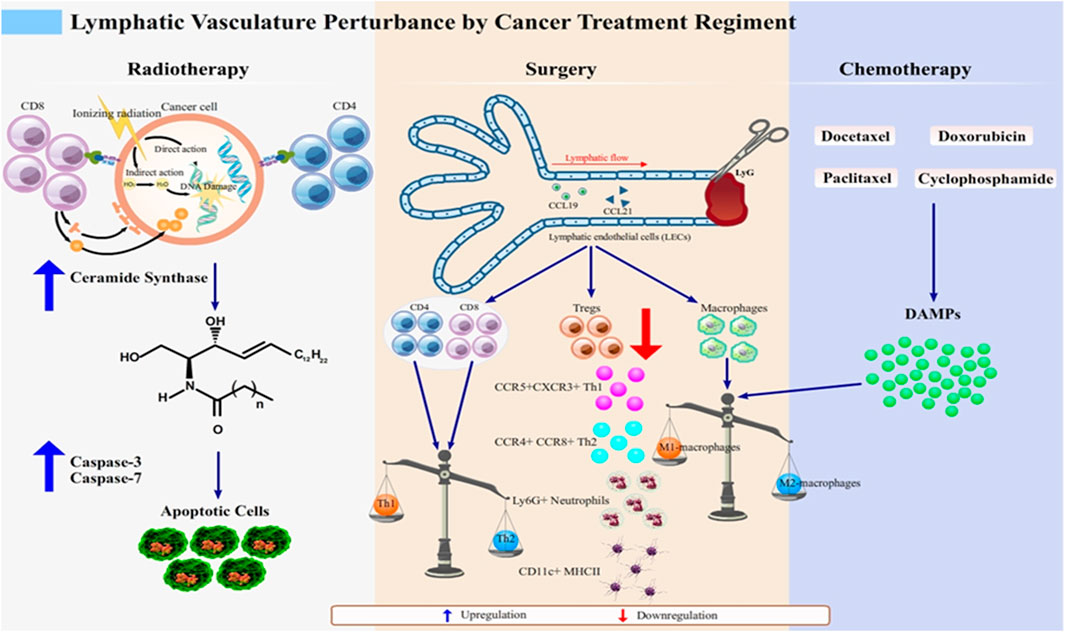
FIGURE 1. Lymphatic vasculature perturbation by anticancer regimens. Lymphedema develops after cancer treatment through various molecular events, which together highlight the disturbance of normal lymphatic physiology. Radiotherapy as an anticancer regimen enhances the expression of MHC class I molecules to improve the ability of recognition of cancerous cells by CD8+ T cell subset (Choi et al., 2007). However, as the proximal lymphatic vasculature, which is lined up by LECs, is unavoidably injured, the breaks in double-stranded DNA in irradiated LECs causes further damage to the mitochondria. This damage subsequently signals the de novo synthesis of ceramide that interacts with caspase-3 and caspase-7 for apoptosis of LECs. This initiates lymphatic dysfunction, which manifests as lymphedema (Overgaard, 2007; Warren et al., 2015; Mortezaee and Najafi, 2020).Surgery and chemotherapy share their mechanisms to induce lymphedema as the imbalanced state of pro- and anti-inflammatory macrophages. Chemotherapeutic agents such as docetaxel, doxorubicin, cyclophosphamide, and paclitaxel (Wang et al., 2017; Roh et al., 2020) induce the release of DAMPs in response to the inflammatory state of the affected tissues. DAMPs skew the Th1/Th2 balanced immune response into the Th2 response, which causes phenotypic switch of macrophages to M2 macrophages (Swaroop et al., 2015). In surgery-induced lymphedema, phenotype shifting of not only macrophage subset but also of T cells (CD4+, CD8+, and Tregs), occurs, thus mediating inflammation. T cells respond to this by secreting anti-inflammatory cytokines, IL-4 and IL-13 (Hayes et al., 2008; Degnim et al., 2012). Tregs, which phenotypically are FOXP-3 expressing CD4+ T cells, are downregulated, causing recruitment of various subsets such as CCR5+CXCR3+ Th1 and CCR4+CCR8+ Th2, Ly-6G + neutrophils, and CD11c+ MHCII + dendritic cells (DCs) to the inflamed tissue (Swaroop et al., 2015). Altogether, these mechanisms are attributable to lymphedema development and are promising targets for the treatment of lymphedema.
Classic dogma in radiobiology suggests that cancer cells are destroyed by ionizing radiation that can either directly damage the DNA or indirectly generate reactive oxygen species (ROS) after water radiolysis (Overgaard, 2007; Mortezaee and Najafi, 2020). Radiation breaks double-stranded DNA into two-ended DNA breaks, rendering them prone to oxidation-induced damage (Toulany, 2019; Huang and Zhou, 2020). The damage effect of radiation is time-dependent. This was modelled more than two decades ago by Mortimer et al. (1991) who irradiated Large White pigs’ skins with a single dose of 18Gy for several weekly time settings. Ischaemic and oedema massively surface in week 6–12. This corresponds to vasodilation of the dermal blood vessels. The longer the waiting, the worse impacts were seen in the skins. In week 52, the dermal thinning and subcutaneous atrophy started clinically evident (Mortimer et al., 1991).
In the molecular level, radiotherapy-associated damage induces mitochondrial ceramide synthase to mediate the de novo synthesis of ceramide that acts as a second messenger in apoptosis. The ceramide pathway then interact with caspase-3 and caspase-7 in intrinsic and extrinsic pathways in commencing programmed cell death (Sia et al., 2020). Caspase-3 and caspase-7 belong to the subgroup of executioner caspases that cleave the cellular target (Ponder and Boise, 2019). It was shown to be upregulated in irradiated LECs, suggesting that irradiation-induced tissue injury may sensitize LECs to apoptosis in a dose-dependent manner, leading to lymphatic dysfunction, which further surface as lymphedema (Avraham et al., 2010). Therefore, it is crucial to find approaches to formulate a low-risk lymphedema regimen for cancer patients receiving radiotherapy. However, it is obvious for majority of clinicians to primarily think about cancer treatment and any rooting potential they may bring; but these efforts are less meaningful as they create a disabling health condition.
A study by Daley et al. (2010) has paved the way for the above query. The findings of their proof-of-principle study showed beneficial effects of amifostine, a thiol derivative, in improving tissue lymphostasis and ameliorating limb lymphedema, along with no activity that favors tumorigenesis in a rodent lymphedema model. Unfortunately, reports on the use of amifostine and other substances that share similar activities in lymphedema are limited. Most reports presented amifostine as a scavenger of free radicals produced during radiotherapy in some cancer cases in humans (Rosenzweig et al., 2010; Belderbos et al., 2018), without any direct relation to the emergence of lymphedema after radiotherapy. Daley et al. successfully demonstrated the anti-lymphedema properties of amifostine, and its translation into clinical trials was expedited. Moreover, the study findings trigger redefinition and reinvention of many substances as anti-lymphedema drugs that were applied initially as a protective agent to radiotherapy.
4.1.3 Surgery
Surgery is one of the well-identified inducers of lymphedema. It has been associated the most with breast cancer. There have reports exhibiting a strong association between breast cancer-associated surgery and incidence of lymphedema. As many as 33% of Australian women suffered from lymphedema in 6–18 months after their surgeries, of those 40% had long-term lymphedema (Hayes et al., 2008). Similarly, but seen in Brazilian women with breast cancers, cumulative incidence of lymphedema was observed at 13.5% within 2 years of follow up time and this increased up to 41.1% after a decade (Mortimer et al., 1991).
Several studies have demonstrated that patients who underwent surgery with axillary node removal showed a significantly higher probability of developing breast lymphedema than those who underwent only breast surgery or sentinel lymph node biopsy (Degnim et al., 2012; Boughey et al., 2014). Breast lymphedema occurs in approximately one-half of women who undergo breast or axillary surgery, that is, initially intended to remove neighboring lymph nodes and vessels (Ridner, 2013) to prevent cancerous cells from metastasizing to distant sites (Nagata et al., 2004; Zheng et al., 2018). This is expected to reduce the carrying capacity of the lymphatic system. Surgical procedures unavoidably impair the normal anatomy, which obstruct the physiological function ofthe lymphatic vessels. Moreover, surgery-induced injuries negatively impact the neighboring muscles, reducing their potential and driving force to distribute the lymphatic fluid throughout the body. In contrast, surgery may increase the blood flow rate. Stenosis in the arterial and venous axillary and brachial vessels on the limb ipsilateral to the surgery has been suggested to be responsible for the increase in flow. This change affects the contralateral limb prior to forming conditions that favor lymphedema generation (Ridner, 2013). To date, axillary lymph node dissection (ALND) and sentinel lymph node biopsy (SLNB) are standard surgical procedures for breast cancer (Gherghe et al., 2015).
Lymphatic injury following ALND might mediate local inflammation causing T cells, mainly CD4+ cells, to infiltrate (Nores et al., 2018). These CD4+ T cells produced either IL-4 or IL-13, the two most common anti-inflammatory cytokines, and were key to the severity of lymphedema (Avraham et al., 2013; Shimizu et al., 2013). In addition to CD4+ T cell infiltration, increase in regulatory T cells (Tregs), phenotypically characterized as FOXP3-expressing CD4+ T cells, was observed in lymphedema (Nores et al., 2018). Elimination of this particular subset exacerbates edema and recruits diverse subsets of CCR5+CXCR3+ Th1, CCR4+CCR8+ Th2, Ly-6G + neutrophils, and CD11c+ MHCII + dendritic cells (DCs), suggesting that Tregs also play a pivotal role in the regulation of the overall leukocyte infiltration, which marks the improvement of lymphedema (Swaroop et al., 2015).
To put the risk of surgery leading to lymphedema as low as possible, SLNB is a better alternative. Compared to breast cancer patients who underwent SLNB, those treated with ALND showed a significantly high probability of experiencing lymphedema symptoms (Miller et al., 2012). SLNB is better suited to patients with locally contralateral advanced breast cancer but not to those undergoing prophylactic mastectomy (Zhou et al., 2011).
Considering all these published reports, we concluded that the existing surgery platform, either ALND or SLNB, could not be avoided without thorough consideration. Although these reports reveal post-surgery risk of lymphedema, it is required to find a substitute therapy with similar efficiency. Previous explanation of the recruitment of Tregs post ALND to the site of injury suggest to consider Tregs as a novel curative approach for lymphedema (Gousopoulos et al., 2016). However, additional studies involving different cohorts of different primary diseases that require surgery at any stage are required. Until then, surgery remains one of the mainstay regimens for cancer, particularly breast cancer. Hence, novel strategy development against surgery-mediated lymphedema by the researchers across the globe is widely focused on providing prior protection against injury during surgery to the compartments of the lymphatic system.
4.2 Infections
Lymphatic filariasis is the most common pathophysiological condition caused by bacterial infection and characterized by impaired lymphatic transport affecting local immune response. Globally, more than 140 million people have been reported to suffer from lymphatic filariasis, mainly caused by Wuchereria bancrofti and less frequently by Brugia malayi (Kambhampati et al., 2016).
Interestingly, a recent case report by Hong et al. (2019) established a causative association between bacterial infection and lymphedema in the lower extremities of a patient with intestinal tuberculosis in Korea. Although an extremely rare case, it can broaden our horizon that lymphedema is not solely attributed to the adverse effects of anticancer regimens. Anti-tuberculosis drugs impaired lymphatic drainage, which resulted in ineffectiveness of the pertinent drug after the lymphatic system was damaged. These results were in line with those from previous studies by Hoda and Rab. (1974) in Korea. Both observed that although anti-tuberculosis drugs initially regressed lymph nodes and pulmonary lesions, lymphedema recurred after 7–9 months and persisted.
4.3 Obesity
Obesity has been considered a strong risk factor for lymphedema (Savetsky et al., 2014; Nitti et al., 2016), causing a serious clinical issue (Shimizu et al., 2013). Obesity and lymphedema have been suggested to have a reciprocal interplay (Mehrara and Greene, 2014). This was clearly observed in adipose tissues isolated from lymphedema, which were identical to fat depots in obese patients; both results from the proliferation and hypertrophy of adipocytes, which subsequently leads to the infiltration of macrophages and lymphocytes, characteristic of chronic inflammation (Zampell et al., 2012; Mehrara and Greene, 2014). At the molecular level, C/EPB-α is an essential transcription factor for determining the fate of adipocyte lineage, and adiponectin, a peptide hormone that promotes differentiation of preadipocytes into adipocytes, is upregulated in the area proximal to the region of lymphatic obstruction. The upregulation of these two proteins decreases in the distal area, according to the gradient of lymphatic stasis (Aschen et al., 2012).
In the obese state, the plasma concentration of adiponectin is decreased. The functional role of adiponectin in the lymphatic system was well described in a mouse model with ablation of tail surface lymphatic network. In this model, adiponectin-KO mice showed a greater diameter of wounded tails than that of wild-type mice. Adiponectin deficiency was associated with a low number of LECs. This phenotype can be reversed by adiponectin administration, which restores the lymphatic vasculature. In vitro, adiponectin promotes the differentiation and viability of LECs through the AMP-activated protein kinase (AMPK)-Akt-endothelial nitric oxide synthase pathways (Shimizu et al., 2013).
In contrast to adiponectin, leptin has been shown to be upregulated in the obese state (Korda et al., 2008; Grossmann et al., 2010). It acts through the leptin receptor in the hypothalamus to suppress food intake, but obese patients are leptin-resistant (Grossmann et al., 2010). In addition to the brain, leptin exerts its biological actions on multiple peripheral tissues, including vascular and lymphatic endothelial cells (Grossmann et al., 2010).
In the context of angiogenesis, leptin mediates neovascularization by inducing the proliferation of vascular endothelial cells and remodeling the extracellular matrix through regulation of metalloproteinases (MMPs) and tissue inhibitors of metalloproteinases (Park et al., 2001).
A high concentration of leptin, as observed in obese patients, compromises lymphatic endothelial cell homeostasis, whereas a physiological concentration of leptin maintains cellular homeostasis. A high concentration of leptin induces the expression of the proinflammatory cytokine IL-6 in human dermal lymphatic endothelial cells. Furthermore, IL-6 rescues the compromised cell proliferation and tube formation caused by high-dose leptin treatment either in an autocrine or paracrine manner. This finding suggests that leptin and IL-6 are promising intervening agents to reduce the incidence of postoperative lymphedema (Sato et al., 2016).
4.4 Lipedema
Lipedema is linked to lymphedema, and both are often misdiagnosed (Peled and Kappos, 2016; Buso et al., 2019). This is a clinical manifestation of bilaterally symmetrical perturbed fat deposition in the lower or upper extremities with frequent ecchymosis, and can be triggered by a minor injury (Shavit et al., 2018); lipedema is a specific disease associated to fat distribution, a distinctive feature from lymphedema.
Lipedema affects approximately 18.8% of patients with enlargement of the lower limbs and is exclusively seen in women (Forner-Cordero et al., 2012). It has been reported that 6.5% of children diagnosed with lymphedema were actually suffered from lipedema (Szél et al., 2014). Moreover, approximately 60% of lipedema cases have been reported to be genetically acquired. Although lipedema-inducing genes are yet to be inherently studied (Grossmann et al., 2010), but studies by Makinen et al. and Harvey et al. have suggested the probable genes involved in it. Mäkinen et al. (2001) showed that VEGFR-3 missense mutation played a key role in the process of subcutaneous adipose tissue thickening, whereas. Harvey et al. (2005) demonstrated that the gene PROX1 was inactivated in an adult-onset obesity and lymphatic vascular disease mouse model. Notably, both genes have been studied for their relevance in lymphedema development, strongly suggesting molecular crosstalk between lymphedema and lipedema.
Lipedema has recently received increased attention, and efforts have been made to gain a comprehensive understanding of the pathological mechanism of the disease for the development of specific intervention strategies for lipedema distinguishable from those of lymphedema. We, hitherto, are left with a major query that whether lymphedema leads to lipedema or vice versa. Future studies should be directed to unveiling the molecular crosstalk between the two.
5 Animal models developed to discern the acquired lymphedema
Despite the agreement that the eventual translational field of all the knowledge and concepts regarding acquired lymphedema is in the human body system, it is undeniably impossible to intentionally induce lymphedema in the human body with an expectation to derive genuine decipherment with regard to the molecular and cellular interplay that builds it. Investigators can only analyze and evaluate the pre-established lymphedema in the human body. Thus, induction and development of lymphedema is a poorly studied attribute. To comprehensively understand the development and suppression of lymphedema in the human body, primarily by the immune system, lymphedema animal models, mainly rodent models, have been proposed and used since the past three decades.
Rodents-based lymphedema model was first established by Lee-Donaldson et al. (1999) who successfully developed hind limb lymphedema in Wistar-Fuzzy rats by either microsurgical ablation (S) of the groin nodes or 4500-rad groin irradiation (R) alone as well as the combinations of the two with S followed by R and vice versa.
Tail-and hindlimb-based models are the two most common rodent models (Park et al., 2013). The tail-based model is favorable as its offers simplicity (Park et al., 2013), however the nonanatomic location and considerably small size of lymphatic vessels, to some extent, complicate surgical interventions. The hindlimb-based model, on the other hand, requires a morbid state, but the swelling resulting from the procedure is reliable, although it will not be sustained for long duration (Ghanta et al., 2016).
Our laboratory has developed a hindlimb -based murine lymphedema model by removing superficial, popliteal, and deep inguinal lymph nodes through femoral lymphatic blockade; we termed this model as SPDF-derived lymphedema. Unlike other published lymphedema models that were generated using relatively simpler methods, we focused on a sustained acquired lymphedema model; however, with regard to procedural interventions, our study required more rigorous skills to ascertain that the lymph nodes of interest were thoroughly removed and the right lymphatic vessel was blocked. We have published the details of the SPDF procedures elsewhere (Roh et al., 2017a; Roh et al., 2017b; Cho et al., 2017).
In the same year, Tran et al. (2018) established a porcine model for surgical prevention of lymphedema by inserting a central venous catheter into the jugular veins of female pigs. It was the first successful model generated through the lymphatic microsurgical preventive healing approach (LYMPHA) for lymphadenectomy. Unlike our murine model, where the mice were kept alive, this was a non-survival porcine model with some complications, including when a correlation of real-time lymphatic clearance rate with clinical lymphedema was urged to be addressed. However, Tran’s team overcame this problem by developing optically enriched fluorophores that allowed observation of lymphadenectomy using small volume injections and spectrophotometry (Tran et al., 2018). We summarize the establishment of rodent model within the last 5 years to allow an integrative understanding of purposive intricacies in model development (Table 2).
As listed in Table 2 and many other published reports that have not been included in the table, it is clear that rats and mice are the two most exploited rodents for lymphedema models. Despite the technical drawback of small-sized lymphatic compartments, the reproducibility of lymphedema in rats and mice that mimic the actual clinical symptoms observed in patients might have attracted the researchers to use these models. The models were established to justify specific interests of the researchers; hence, the interventions were quite different. However, we can highlight that, despite the differences in surgical intervention strategy, lymphatic drainage should be firmly perturbed to allow manifestation of clinical outcomes of interest. These differently built models also provide diverse directions for future studies, which are expected to intersect at a point where an alleviative strategy needs to be successfully formulated to suppress the emergence or relapse of lymphedema as either chronic individual disease or comorbidity and to improve the quality of life of the affected patients.
6 Phytochemicals-based substances against lymphedema
Despite the prevailing information about lymphedema induction and development, its intervention strategy is yet to be formulated. Currently, there is no licensed medicine for lymphedema. This is so unfortunate considering that the number of patients suffering from lymphedema, particularly the patients with breast cancer, is growing by multiple times (Pappalardo et al., 2021). Anti-inflammatory capacity, thus far, is seen as an underlying mechanism rendering some prior chemical-based drugs are recommended to treat lymphedema (Forte et al., 2019).
In 2004, benzopyrone, which is generally prescribed to reduce vascular permeability, was observed to be promising as an anti-lymphedema drug because of its ability to enhance macrophage activity and accelerate the lysis of proteins to block fibrosis in the lymphodematous limbs. Unfortunately, this claim was not strongly supported by the results of a meta-analysis; hence, its effectiveness remains questionable (Badger et al., 2004). A decade later, ubenimex, a synthetic organic compound, which has the ability to promote lymphatic flow similar to that observed with benzopyrone, entered a phase 2 clinical trial. However, ubenimex is only available in Japan and the United States (Chiu et al., 2020). Deupirfenidone (LYT-100), a synthetic chemical compound, is the most recently developed anti-lymphedema drug in phase 2a clinical trial, and studies on its safety, pharmacokinetics, and tolerability are being conducted. Deupirfenidone is principally a deuterated form of pirfenidone, which is known to possess inhibitory activity on the release of collagen and cytokines (https://adisinsight.springer.com/drugs/800038358). Pirfenidone alone has been used to treat idiopathic pulmonary fibrosis (Sgalla et al., 2018; Cottin et al., 2019), which led to a more recent hypothesis that pirfenidone might also work to alleviate pulmonary-related deterioration in COVID-19 patients (George et al., 2020; Seifirad, 2020).
Moreover, Chen et al. (2015) demonstrated that calycosin and gallic acid, chemical derivatives from Radix astragal and Radix Paeoniae, respectively, synergistically induced the expression of leukotriene B4 dehydrogenase (LTB4DH), which attenuated leukotriene B4 (LTB4) (Cheng et al., 2015). LTB4 belongs to a family of eicosanoid inflammatory mediators involved in the innate immune response and is highly associated with the pathophysiology of lymphedema (Jiang et al., 2018). In a surgery model of lymphedema in mouse, LTB4 was observed to be elevated, which was counterproductive towards the repair of the lymphatic tissues affected by surgery; hence, blocking of LTB4 has been observed to negate this effect (Tian et al., 2017). In addition to calycosin and gallic acid, Goreisan has been implicated in alleviation of lower-limb lymphedema (LLL) in patients with gynecologic cancers who underwent pelvic lymphadenectomy. Goreisan is a traditional Japanese herb comprising “bukuryo” (hoelen), “takusha” (Alismatis rhizoma), “sojutsu” (Atractylodes lanceae rhizoma), “chorei” (polyporus), and “keihi” (cinnamon bark). This herb is available in China and Korea. LLL patients who received Goreisan showed a remarkable reduction in extracellular water (ECW)/total body water (TBW) ratio, suggesting that the herb ameliorated the excessive accumulation of extracellular fluid that restricted free physical movement of extremities proximal to the lymphadenectomy-affected sites (Yoshikawa et al., 2020).
Our laboratory contributed to this global effort by demonstrating that sulfuretin extracted from Rhus verniciflua is a promising anti-lymphedema agent (Roh et al., 2017a). Rhus verniciflua belongs to the family Anacardiaceae and is commonly known as the lacquer tree, which is indigenous to Korea, Japan, and China. This has been used as an alternative herbal medicine to cure patients with diabetes and stomach-related diseases (Choi et al., 2007). Oral administration of the extract has shown to reduce adipocyte deposition in lymphedematous tissue in SPDF-induced lymphedema-bearing murine model. In contrast, the expression of VEGFR-3, a marker of lymphangiogenesis, was induced after sulfuretin treatment (Roh et al., 2017a). Similarly, saponins extracted from Panax notoginseng (PNS), which are commonly used in traditional Chinese medicine for cardiovascular and cerebrovascular diseases, promote lymphangiogenesis in a VEGF-C/VEGFR-3 fashion in zebrafish. Furthermore, ERK1/2, PI3K, and P38 MAPK signaling pathways were deemed to be pivotally involved. This widened the horizon on suitable therapeutic agents for acquired lymphedema (Sgalla et al., 2018) which previously and even to date is thought to be a frustrating dead-end. Moreover, all these studies have stimulated additional studies on plant-derived chemicals exerting anti-lymphedema activity. The above descriptions are listed in Table 3.
7 Preventive management of acquired lymphedema
Despite the well-identified of clinical risk factors for lymphedema, firm scientific evidence for its preventive methodology is lacking, which lead anxiety for those affected patients. Regardless, a common sense approach is deemed to be adoptable while optimal prevention platform are yet to find its best structure (Cemal et al., 2011). The prevention strategy covers all steps from measuring the risk factors to preventing the accumulation of fibro-adipose tissue produced by chronic lymph stasis (Prevention of Lymphedema, 2022) which is best exemplified in breast cancer therapy-related lymphedema. So far, among other cancer types, breast cancer is regarded as the highest contributor to lymphedema (American Cancer Society, 2022). As the first step of prevention, BMI, lymph nodes status and radiotherapy effects for those who have undergone radical mastectomy are needed to be carefully evaluated by clinicians to avoid complication (Hua-Ping et al., 2012). As reported by Jammallo et al. (2013), pre- and post-operative BMI were significantly associated with risk of lymphedema. Either a pre-operative BMI ≥30 (Jammallo et al., 2013; Can et al., 2016) or a huge fluctuation, regardless a 10 pounds-gain or loss increases risk of lymphedema markedly (Jammallo et al., 2013). Like BMI, the number of metastatic lymph node is also regarded as one of determinant lymphedema inducers. It was evident as the number of patients developing lymphedema after receiving breast conserving surgery (BCS) in combination with ALND was not statistically significant compared to that of patients with no lymphedema (Can et al., 2016). Moreover, other study demonstrated that prophylactic lymphovenous anastomosis (LVA) conducted after lymphadenectomy was potentially lower the risk of cancer-related lymphedema without negating the cancer treatment (Ciudad et al., 2022). In gynaecological cancer cases, early prevention of complex decongestive therapy (CDT) combined with physical exercise prevents lower extremity lymphedema post operation (Wu et al., 2021). CDT is designed to alleviate the swelling, enhance the condition of skin, improve mobility, reduce the risk of infection and optimize the quality of health (OncoLink Team, 2022). CDT encompasses two-phase approach:1) phase I (intensive) is aimed at mobilizing, reducing the congested protein-enriched fluid softening the connective tissue and 2) phase II (maintenance) is aimed at preservering results derived from phase I (Michopoulos et al., 2020). Up to date, CDT is referred as the gold standard of lymphedema treatment (LymphCare, 2021). Although specific mechanisms are yet to be clear and so far the study of CDT are likely to be in randomized trials, this has been widely shown to alleviate lymphedema in different patients’ cohorts (Yesil et al., 2017; Omidi et al., 2020; Wu et al., 2021). This suggests that, although finding an effective preventive startegy is yet to see its ends, CDT is feasible to implement.
8 Discussion
Acquired lymphedema, despite its well-characterized clinical symptoms, remains a major problem to solve. This emergence reduces the quality of life of affected patients and worsens when a relapse recurs. Several studies have shown that relapsed lymphedema is almost impossible to reverse. As an adverse effect to cancer treatment, lymphedema exhibits multi-complex molecular and cellular orchestration, which could either favor or unfavor tumorigenesis and lymphangiogenesis. This is a favorable mechanism to alleviate the excess lymphatic fluid accumulation preceded by lymphatic injury. However, lymphangiogenesis is unfavorable for tumorigenesis suppression. This is of conflicting profiles, which demands further in-depth investigation of curative agents against cancers that concomitantly act as preventive agents against potentially developed lymphedema. To discern intricacies in lymphedema, diverse types of animal models have been developed, with murine-based model being the most commonly exploited. Although models do not precisely and frequently address all our queries, they resemble the best to human molecular pathways; hence, they are able to provide evidences related to the anticancer mechanism. This is also the most feasible living tool to evaluate loss-gain functions, as consequences of up/down regulations of well-known lymphedema-associated genes, mainly VEGF-C/VEGFR-3, TFG-β1, and C/EBP-α/β, through which clear functions of the pertinent genes can be mapped firmly. In addition, the probability of negation functions of the associated genes as well as crosstalk predisposition can be evaluated. When this is contraindicated to the overall health status and quality of life post treatment, a strategy to prevent further detrimental body reactions can be specifically formulated. Phytochemical-based substances generally emerge as a suitable approach to this debilitating comorbid disease. Their anti-lymphedema properties, which are mediated via different pathways, are attractive for further exploration.
Author contributions
Article conception, writing and drafting of the manuscript were prepared by IN and pre-evaluated by KR. SL, HK, and CY performed manuscript restructuring and overall supervision. All authors have reviewed and approved the final version of the manuscript.
Funding
This research was supported by Yeom Chang-Hwan Hospital, Seoul, South Korea and Rappeler Co.
Acknowledgments
We thank Sohee Song for designing the scientific illustration.
Conflict of interest
The authors declare that the research was conducted in the absence of any commercial or financial relationships that could be construed as a potential conflict of interest.
Publisher’s note
All claims expressed in this article are solely those of the authors and do not necessarily represent those of their affiliated organizations, or those of the publisher, the editors and the reviewers. Any product that may be evaluated in this article, or claim that may be made by its manufacturer, is not guaranteed or endorsed by the publisher.
References
Alishekevitz, D., Gingis-Velitski, S., Kaidar-Person, O., Gutter-Kapon, L., Scherer, S. D., Raviv, Z., et al. (2016). Macrophage-induced lymphangiogenesis and metastasis following paclitaxel chemotherapy is regulated by VEGFR3. Cell. Rep. 17 (5), 1344–1356. doi:10.1016/j.celrep.2016.09.083
American Cancer Society (2022). For people at risk of lymphedema. [Internet]. [cited 2022 Sep 16]. Available from: https://www.cancer.org/treatment/treatments-and-side-effects/physical-side-effects/lymphedema/for-people-at-risk-of-lymphedema.html.
Anton, N. (2019). “Sidawy. Lymphedema : Evaluation and decision making,” in Rutherford’s vascular surgery and endovascular therapy [internet]. Editor Stanley G. Rockson. 9th ed. (Washington (DC): Elsevier), 2193–2205. e2. Available from: https://www.clinicalkey.com/#!/content/book/3-s2.0-B9780323427913001687.
Aschen, S., Zampell, J. C., Elhadad, S., Weitman, E., Andrade, M. de B., and Mehrara, B. J. (2012). Regulation of adipogenesis by lymphatic fluid stasis: Part II. Expression of adipose differentiation genes. Plast. Reconstr. Surg. 129 (4), 838–847. doi:10.1097/PRS.0b013e3182450b47
Avraham, T., Clavin, N. W., Daluvoy, S. V., Fernandez, J., Soares, M. A., Cordeiro, A. P., et al. (2009). Fibrosis is a key inhibitor of lymphatic regeneration. Plast. Reconstr. Surg. 124 (2), 438–450. doi:10.1097/PRS.0b013e3181adcf4b
Avraham, T., Yan, A., Zampell, J. C., Daluvoy, S. V., Haimovitz-Friedman, A., Cordeiro, A. P., et al. (2010). Radiation therapy causes loss of dermal lymphatic vessels and interferes with lymphatic function by TGF-beta1-mediated tissue fibrosis. Am. J. Physiol. Cell. Physiol. 299 (3), C589–C605. doi:10.1152/ajpcell.00535.2009
Avraham, T., Zampell, J. C., Yan, A., Elhadad, S., Weitman, E. S., Rockson, S. G., et al. (2013). Th2 differentiation is necessary for soft tissue fibrosis and lymphatic dysfunction resulting from lymphedema. FASEB J. [Internet 27 (3), 1114–1126. doi:10.1096/fj.12-222695
Azhar, S. H., Lim, H. Y., Tan, B. K., and Angeli, V. (2020). The unresolved pathophysiology of lymphedema. Front. Physiol. 1–11, 137. doi:10.3389/fphys.2020.00137
Badger, C., Preston, N., Seers, K., and Mortimer, P. (2004). Benzo-pyrones for reducing and controlling lymphoedema of the limbs. Cochrane Database Syst. Rev. 2004 (2), CD003140. doi:10.1002/14651858.CD003140.pub2
Belderbos, J., Gaspar, L., Demiral, A. N., and Marks, L. B. (2018). “42 - acute and late toxicities of thoracic radiotherapy: Pulmonary, esophagus, and heart,” in IASLC thoracic oncology. Editors H. I. Pass, D. Ball, and G. V. Scagliotti. Second EditionSecond Edi (Philadelphia: Elsevier), 393–408. [Internet]e4. Available from: https://www.sciencedirect.com/science/article/pii/B9780323523578000421.
Bertelli, M., Kiani, A. K., Paolacci, S., Manara, E., Dautaj, A., Beccari, T., et al. (2020). Molecular pathways involved in lymphedema: Hydroxytyrosol as a candidate natural compound for treating the effects of lymph accumulation. J. Biotechnol. 308, 82–86. doi:10.1016/j.jbiotec.2019.11.017
Biglia, N., Zanfagnin, V., Daniele, A., Robba, E., and Bounous, V. E. (2017). Lower body lymphedema in patients with gynecologic cancer. Anticancer Res. 37 (8), 4005–4015. doi:10.21873/anticanres.11785
Bok, S. K., Jeon, Y., Lee, J. A., and Ahn, S. Y. (2018). Evaluation of stiffness in postmastectomy lymphedema using acoustic radiation force impulse imaging: A prospective randomized controlled study for identifying the optimal pneumatic compression pressure to reduce stiffness. Lymphat. Res. Biol. 16 (1), 36–42. doi:10.1089/lrb.2016.0048
Bond, H. M., Scicchitano, S., Chiarella, E., Amodio, N., Lucchino, V., Aloisio, A., et al. (2018). ZNF423: A new player in estrogen receptor-positive breast cancer. Front. Endocrinol. 9, 255. doi:10.3389/fendo.2018.00255
Borman, P. (2018). Lymphedema diagnosis, treatment, and follow-up from the view point of physical medicine and rehabilitation specialists. Turk. J. Phys. Med. Rehabil. 64 (3), 179–197. doi:10.5606/tftrd.2018.3539
Boughey, J. C., Hoskin, T. L., Cheville, A. L., Miller, J., Loprinzi, M. D., Thomsen, K. M., et al. (2014). Risk factors associated with breast lymphedema. Ann. Surg. Oncol. 21 (4), 1202–1208. doi:10.1245/s10434-013-3408-5
Bruns, F., Micke, O., and Bremer, M. (2003). Current status of selenium and other treatments for secondary lymphedema. J. Support. Oncol. 1 (2), 121–130.
Buso, G., Depairon, M., Tomson, D., Raffoul, W., Vettor, R., and Mazzolai, L. (2019). Lipedema: A call to action. Obesity 27 (10), 1567–1576. doi:10.1002/oby.22597
Can, A. G., Ekşioğlu, E., Bahtiyarca, Z. T., and Çakcı, F. A. (2016). Assessment of risk factors in patients who presented to the outpatient clinic for breast cancer-related lymphedema. J. Breast Health 12 (1), 31–36. doi:10.5152/tjbh.2015.2801
Cemal, Y., Pusic, A., and Mehrara, B. J. (2011). Preventative measures for lymphedema: Separating fact from fiction. J. Am. Coll. Surg. 213 (4), 543–551. doi:10.1016/j.jamcollsurg.2011.07.001
Chandler, C., Liu, T., Buckanovich, R., and Coffman, L. G. (2019). The double edge sword of fibrosis in cancer. Transl. Res. 209, 55–67. doi:10.1016/j.trsl.2019.02.006
Cheng, Y., Zhao, J., Tse, H. F., Le, X. C., and Rong, J. (2015). Plant natural products calycosin and gallic acid synergistically attenuate neutrophil infiltration and subsequent injury in isoproterenol-induced myocardial infarction: A possible role for leukotriene B4 12-hydroxydehydrogenase? Oxid. Med. Cell. Longev. 2015, 434052. doi:10.1155/2015/434052
Chiu, T-W., Kong, S-L., Cheng, K-F., and Leung, P-C. (2020). Treatment of post-mastectomy lymphedema with herbal medicine: An innovative pilot study. Plast. Reconstr. Surg. Glob. Open 8 (6), e2915. doi:10.1097/GOX.0000000000002915
Cho, S., Roh, K., Park, J., Park, Y. S., Lee, M., Cho, S., et al. (2017). Hydrolysis of hyaluronic acid in lymphedematous tissue alleviates fibrogenesis via TH1 cell-mediated cytokine expression. Sci. Rep. 7 (1), 35–11. doi:10.1038/s41598-017-00085-z
Choi, H. S., Kim, M. K., Park, H. S., Yun, S. E., Mun, S. P., Kim, J. S., et al. (2007). Biological detoxification of lacquer tree (Rhus verniciflua Stokes) stem bark by mushroom species. Food Sci. Biotechnol. 16 (6), 935–942.
Christiansen, A., and Detmar, M. (2011). Lymphangiogenesis and cancer. Genes. Cancer 2 (12), 1146–1158. doi:10.1177/1947601911423028
Ciudad, P., Escandón, J. M., Bustos, V. P., Manrique, O. J., and Kaciulyte, J. (2022). Primary prevention of cancer-related lymphedema using preventive lymphatic surgery: Systematic review and meta-analysis. Indian J. Plast. Surg. 55 (1), 18–25. doi:10.1055/s-0041-1740085
Cottin, V., Wollin, L., Fischer, A., Quaresma, M., Stowasser, S., and Harari, S. (2019). Fibrosing interstitial lung diseases: Knowns and unknowns. Eur. Respir. Rev. 28 (151), 180100. doi:10.1183/16000617.0100-2018
Daley, S. K., Bernas, M. J., Stea, B. D., Bracamonte, F., McKenna, M., Stejskal, A., et al. (2010). Radioprotection from radiation-induced lymphedema without tumor protection. Lymphology 43 (2), 48–58.
Daneshgaran, G., Lo, A. Y., Paik, C. B., Cooper, M. N., Sung, C., Jiao, W., et al. (2019). A pre-clinical animal model of secondary head and neck lymphedema. Sci. Rep. 9 (1), 18264–18312. doi:10.1038/s41598-019-54201-2
de sá, P. M., Richard, A. J., Hang, H., and Stephens, J. M. (2017). Transcriptional regulation of adipogenesis. Compr. Physiol. 7 (2), 635–674. doi:10.1002/cphy.c160022
Degnim, A. C., Miller, J., Hoskin, T. L., Boughey, J. C., Loprinzi, M., Thomsen, K., et al. (2012). A prospective study of breast lymphedema: Frequency, symptoms, and quality of life. Breast Cancer Res. Treat. 134 (3), 915–922. doi:10.1007/s10549-012-2004-x
Dijkgraaf, E. M., Heusinkveld, M., Tummers, B., Vogelpoel, L. T. C., Goedemans, R., Jha, V., et al. (2013). Chemotherapy alters monocyte differentiation to favor generation of cancer-supporting M2 macrophages in the tumor microenvironment. Cancer Res. 73 (8), 2480–2492. doi:10.1158/0008-5472.CAN-12-3542
Dm, S., null N, E. T. M., Adipocyte, E. S. P., and Scherer, P. E. (2007). Adipocyte, adipose tissue, and infectious disease. Infect. Immun. 75 (3), 1066–1078. doi:10.1128/IAI.01455-06
Duan, M., Steinfort, D. P., Smallwood, D., Hew, M., Chen, W., Ernst, M., et al. (2016). CD11b immunophenotyping identifies inflammatory profiles in the mouse and human lungs. Mucosal Immunol. 9 (2), 550–563. doi:10.1038/mi.2015.84
Duffield, J. S., Forbes, S. J., Constandinou, C. M., Clay, S., Partolina, M., Vuthoori, S., et al. (2005). Selective depletion of macrophages reveals distinct, opposing roles during liver injury and repair. J. Clin. Investig. 115 (1), 56–65. doi:10.1172/JCI22675https://pubmed.ncbi.nlm.nih.gov/15630444
Escobedo, N., and Oliver, G. (2017). The lymphatic vasculature: Its role in adipose metabolism and obesity. Cell. Metab. 26 (4), 598–609. doi:10.1016/j.cmet.2017.07.020
Forner-Cordero, I., Szolnoky, G., Forner-Cordero, A., and Lipedema, Kemény L. (2012). Lipedema: An overview of its clinical manifestations, diagnosis and treatment of the disproportional fatty deposition syndrome - systematic review. Clin. Obes. 2 (3–4), 86–95. doi:10.1111/j.1758-8111.2012.00045.x
Forte, A. J., Boczar, D., Huayllani, M. T., Lu, X., and McLaughlin, S. A. (2019). Pharmacotherapy agents in lymphedema treatment: A systematic review. Cureus 11 (12), e6300. doi:10.7759/cureus.6300
Fu, M. R. (2014). Breast cancer-related lymphedema: Symptoms, diagnosis, risk reduction, and management. World J. Clin. Oncol. 5 (3), 241–247. doi:10.5306/wjco.v5.i3.241
García Nores, G. D., Ly, C. L., Cuzzone, D. A., Kataru, R. P., Hespe, G. E., Torrisi, J. S., et al. (2018). CD4+ T cells are activated in regional lymph nodes and migrate to skin to initiate lymphedema. Nat. Commun. 9 (1), 1970. doi:10.1038/s41467-018-04418-y
Gardenier, J. C., Kataru, R. P., Hespe, G. E., Savetsky, I. L., Torrisi, J. S., Nores, G. D. G., et al. (2017). Topical tacrolimus for the treatment of secondary lymphedema. Nat. Commun. 8, 14345. doi:10.1038/ncomms14345
Garza, R., Skoracki, R., Hock, K., and Povoski, S. P. (2017). A comprehensive overview on the surgical management of secondary lymphedema of the upper and lower extremities related to prior oncologic therapies. BMC Cancer 17 (1), 468–518. doi:10.1186/s12885-017-3444-9
Gavins, F. N. E., and Alexander, J. S. (2020). “Lymphatic structure and function in health and disease,” in Gavins FNE, alexander JSBT-LS and. Editor D. F in H and (Academic Press), 1–4. Available from: https://www.sciencedirect.com/science/article/pii/B9780128156452000010.
George, P. M., Wells, A. U., and Jenkins, R. G. (2020). Pulmonary fibrosis and COVID-19: The potential role for antifibrotic therapy. Lancet. Respir. Med. 8 (8), 807–815. doi:10.1016/S2213-2600(20)30225-3
Ghanta, S., Cuzzone, D. A., and Mehrara, B. J. (2016). “Animal models of lymphedema,” in Lymphedema: Complete medical & surgical management. Editors P. C. Neligen, J. Masia, and N. B. Piller (New York: Thieme), 583–595.
Ghanta, S., Cuzzone, D. A., Torrisi, J. S., Albano, N. J., Joseph, W. J., Savetsky, I. L., et al. (2015). Regulation of inflammation and fibrosis by macrophages in lymphedema. Am. J. Physiol. Heart Circ. Physiol. 308 (9), H1065–H1077. doi:10.1152/ajpheart.00598.2014
Gherghe, M., Bordea, C., and Blidaru, A. (2015). Sentinel lymph node biopsy (SLNB) vs. axillary lymph node dissection (ALND) in the current surgical treatment of early stage breast cancer. J. Med. Life 8 (2), 176–180.
Gomes, F. G., Nedel, F., Alves, A. M., Nör, J. E., and Tarquinio, S. B. C. (2013). Tumor angiogenesis and lymphangiogenesis: Tumor/endothelial crosstalk and cellular/microenvironmental signaling mechanisms. Life Sci. 92 (2), 101–107. doi:10.1016/j.lfs.2012.10.008
Gousopoulos, E., Proulx, S. T., Bachmann, S. B., Dieterich, L. C., Scholl, J., Karaman, S., et al. (2017). An important role of VEGF-C in promoting lymphedema development. J. Investig. Dermatol. 137 (9), 1995–2004. doi:10.1016/j.jid.2017.04.033
Gousopoulos, E., Proulx, S. T., Bachmann, S. B., Scholl, J., Dionyssiou, D., Demiri, E., et al. (2016). Regulatory T cell transfer ameliorates lymphedema and promotes lymphatic vessel function. JCI Insight 1 (16), e89081. doi:10.1172/jci.insight.89081
Greene, A. K., and Goss, J. A. (2018/04/09201). Diagnosis and staging of lymphedema. Semin. Plast. Surg. 32 (1), 12–16. doi:10.1055/s-0038-1635117
Grossmann, M. E., Ray, A., Nkhata, K. J., Malakhov, D. A., Rogozina, O. P., Dogan, S., et al. (2010). Obesity and breast cancer: Status of leptin and adiponectin in pathological processes. Cancer Metastasis Rev. 29 (4), 641–653. doi:10.1007/s10555-010-9252-1
Gupta, R. K., Mepani, R. J., Kleiner, S., Lo, J. C., Khandekar, M. J., Frontini, A., et al. (2012). Zfp423 expression identifies committed preadipocytes and localizes to adipose endothelial and perivascular cells. Cell Metab. 15 (2), 230–239. doi:10.1016/j.cmet.2012.01.010
Harris, A. R., Perez, M. J., and Munson, J. M. (2018). Docetaxel facilitates lymphatic-tumor crosstalk to promote lymphangiogenesis and cancer progression. BMC Cancer 18 (1), 718–816. doi:10.1186/s12885-018-4619-8
Harvey, N. L., Srinivasan, R. S., Dillard, M. E., Johnson, N. C., Witte, M. H., Boyd, K., et al. (2005). Lymphatic vascular defects promoted by Prox1 haploinsufficiency cause adult-onset obesity. Nat. Genet. 37 (10), 1072–1081. doi:10.1038/ng1642
Hayashida, K., Yoshida, S., Yoshimoto, H., Fujioka, M., Saijo, H., Migita, K., et al. (2017). Adipose-derived stem cells and vascularized lymph node transfers successfully treat mouse hindlimb secondary lymphedema by early reconnection of the lymphatic system and lymphangiogenesis. Plast. Reconstr. Surg. 139 (3), 639–651. doi:10.1097/PRS.0000000000003110
Hayes, S. C., Janda, M., Cornish, B., Battistutta, D., and Newman, B. (2008). Lymphedema after breast cancer: Incidence, risk factors, and effect on upper body function. J. Clin. Oncol. 26 (21), 3536–3542. doi:10.1200/JCO.2007.14.4899
Hidding, J. T., Beurskens, C. H. G., van der Wees, P. J., Bos, W. C. A. M., Nijhuis-van der Sanden, M. W. G., and van Laarhoven, H. W. M. (2018). Changes in volume and incidence of lymphedema during and after treatment with docetaxel, doxorubicin, and cyclophosphamide (TAC) in patients with breast cancer. Support. Care Cancer 26 (5), 1383–1392. doi:10.1007/s00520-017-3907-1
Hoda, S., and Rab, S. M. (1974). Two cases of tuberculous lymphoedema. Br. Med. J. 3 (5934), 786. doi:10.1136/bmj.3.5934.786
Hoffner, M., Peterson, P., Månsson, S., and Brorson, H. (2018). Lymphedema leads to fat deposition in muscle and decreased muscle/water volume after liposuction: A magnetic resonance imaging study. Lymphat. Res. Biol. 16 (2), 174–181. doi:10.1089/lrb.2017.0042
Hong, J. W., Park, H. E., Shin, M. J., Shin, Y. B., and Yoon, J. A. (2019). Secondary lymphedema after intestinal tuberculosis: A case report. Ann. Rehabil. Med. 43 (6), 725–729. doi:10.5535/arm.2019.43.6.725
Hua-Ping, H., Jian-Rong, Z., and Zeng, Q. (2012). Risk factors associated with lymphedema among postmenopausal breast cancer survivors after radical mastectomy and axillary dissection in China. Breast Care (Basel) 7 (6), 461–464. doi:10.1159/000345459
Huang, R. X., and Zhou, P. K. (2020). DNA damage response signaling pathways and targets for radiotherapy sensitization in cancer. Signal Transduct. Target. Ther. 5 (1), 60. doi:10.1038/s41392-020-0150-x
Hwang, S. D., Song, J. H., Kim, Y., Lim, J. H., Kim, M. Y., Kim, E. N., et al. (2019). Inhibition of lymphatic proliferation by the selective VEGFR-3 inhibitor SAR131675 ameliorates diabetic nephropathy in db/db mice. Cell. Death Dis. 10 (3), 219–316. doi:10.1038/s41419-019-1436-1
International Society of Lymphology (2020). The diagnosis and treatment of peripheral lymphedema: 2020 consensus document of the international society of Lymphology. Lymphology 53 (1), 3–19. doi:10.2458/lymph.4649
Jammallo, L. S., Miller, C. L., Singer, M., Horick, N. K., Skolny, M. N., Specht, M. C., et al. (2013). Impact of body mass index and weight fluctuation on lymphedema risk in patients treated for breast cancer. Breast Cancer Res. Treat. 142 (1), 59–67. doi:10.1007/s10549-013-2715-7
Ji, E., Jung, M. Y., Park, J. H., Kim, S., Seo, C. R., Park, K. W., et al. (2014). Inhibition of adipogenesis in 3T3-L1 cells and suppression of abdominal fat accumulation in high-fat diet-feeding C57BL/6J mice after downregulation of hyaluronic acid. Int. J. Obes. 38 (8), 1035–1043. doi:10.1038/ijo.2013.202
Jiang, X., Nicolls, M. R., Tian, W., and Rockson, S. G. (2018). Lymphatic dysfunction, leukotrienes, and lymphedema. Annu. Rev. Physiol. 80 (1), 49–70. doi:10.1146/annurev-physiol-022516-034008
Joukov, V., Pajusola, K., Kaipainen, A., Chilov, D., Lahtinen, I., Kukk, E., et al. (1996). A novel vascular endothelial growth factor, VEGF-C, is a ligand for the Flt4 (VEGFR-3) and KDR (VEGFR-2) receptor tyrosine kinases. EMBO J. 15 (2), 1751–1758. doi:10.1002/j.1460-2075.1996.tb00521.x
Kambhampati, S., and Rockson, S. (2016). “Causes and classification of lymphatic disorders,” in Lymphedema: Complete medical & surgical management. Editors P. C. Neligan, J. Masia, and N. B. Piller (New York: Thieme), 277–290.
Karayi, A. K., Basavaraj, V., Narahari, S. R., Aggithaya, M. G., Ryan, T. J., and Pilankatta, R. (2020). Human skin fibrosis: Up-regulation of collagen type III gene transcription in the fibrotic skin nodules of lower limb lymphoedema. Trop. Med. Int. Health 25 (3), 319–327. doi:10.1111/tmi.13359
Karkkainen, M. J., Ferrell, R. E., Lawrence, E. C., Kimak, M. A., Levinson, K. L., McTigue, M. A., et al. (2000). Missense mutations interfere with VEGFR-3 signalling in primary lymphoedema. Nat. Genet. 25 (2), 153–159. doi:10.1038/75997
Kim, K. W., and Song, J. H. (2017). Emerging roles of lymphatic vasculature in immunity. Immune Netw. 17 (1), 68–76. doi:10.4110/in.2017.17.1.68
Kim, M., Park, I. H., Lee, K. S., Ro, J., Jung, S. Y., Lee, S., et al. (2015). Breast cancer-related lymphedema after neoadjuvant chemotherapy. Cancer Res. Treat. 47 (3), 416–423. doi:10.4143/crt.2014.079
Koc, M., Wald, M., Varaliová, Z., Ondrůjová, B., Čížková, T., Brychta, M., et al. (2021). Lymphedema alters lipolytic, lipogenic, immune and angiogenic properties of adipose tissue: A hypothesis-generating study in breast cancer survivors. Sci. Rep. 11 (1), 8171–8217. doi:10.1038/s41598-021-87494-3
Korda, M., Kubant, R., Patton, S., and Malinski, T. (2008). Leptin-induced endothelial dysfunction in obesity. Am. J. Physiol. Heart Circ. Physiol. 295 (4), H1514–H1521. doi:10.1152/ajpheart.00479.2008
Kunkler, I. H., Williams, L. J., Jack, W. J. L., Cameron, D. A., and Dixon, J. M. (2015). Breast-conserving surgery with or without irradiation in women aged 65 years or older with early breast cancer (prime II): A randomised controlled trial. Lancet. Oncol. 16 (3), 266–273. doi:10.1016/S1470-2045(14)71221-5
Lane, M. D. (2013). “Adipogenesis,” in Lane MDBT-E of BC. Editor W. J. Lennarz. Second E (Waltham: Academic Press), 52–56. Available from: http://www.sciencedirect.com/science/article/pii/B978012378630200089X.
Lee, E., Pandey, N. B., and Popel, A. S. (2015). Crosstalk between cancer cells and blood endothelial and lymphatic endothelial cells in tumour and organ microenvironment. Expert Rev. Mol. Med. 17, e3. doi:10.1017/erm.2015.2
Lee, S., Kang, J., Yoo, J., Ganesan, S. K., Cook, S. C., Aguilar, B., et al. (2008/09/24200). Prox1 physically and functionally interacts with COUP-TFII to specify lymphatic endothelial cell fate. Blood 113 (8), 1856–1859. doi:10.1182/blood-2008-03-145789
Lee, Y. L., Huang, Y. L., Chu, S. Y., Chan, W. H., Cheng, M. H., Lin, Y. H., et al. (2020). Characterization of limb lymphedema using the statistical analysis of ultrasound backscattering. Quant. Imaging Med. Surg. 10 (1), 48–56. doi:10.21037/qims.2019.10.12
Lee-Donaldson, L., Witte, M. H., Bernas, M., Witte, C. L., Way, D., and Stea, B. (1999). Refinement of a rodent model of peripheral lymphedema. Lymphology 32 (3), 111–117.
Li, J., Chen, Y., Zhang, L., Xing, L., Xu, H., Wang, Y., et al. (2016). Total saponins of panaxnotoginseng promotes lymphangiogenesis by activation VEGF-C expression of lymphatic endothelial cells. J. Ethnopharmacol. 193, 293–302. doi:10.1016/j.jep.2016.08.032
Lim, M. C., Lee, J. S., Nam, B. H., Seo, S. S., Kang, S., and Park, S. Y. (2014). Lower extremity edema in patients with early ovarian cancer. J. Ovarian Res.Journal Ovarian Res. 7 (1), 28–8. doi:10.1186/1757-2215-7-28
Lowe, C. E., O’Rahilly, S., and Rochford, J. J. (2011). Adipogenesis at a glance. J. Cell. Sci. 124 (16), 2681–2686. doi:10.1242/jcs.079699
LymphCare (2021). Complex decongestive therapy (CDT). [cited 2022 Sep 17]. Available from: https://www.lymphcare.com/na-en/treating-your-condition/treating-lymphedema/.
Maclellan, R. A., and Greene, A. K. (2014). Lymphedema. Semin. Pediatr. Surg. 23 (4), 191–197. doi:10.1053/j.sempedsurg.2014.07.004
Mäkinen, T., Jussila, L., Veikkola, T., Karpanen, T., Kettunen, M. I., Pulkkanen, K. J., et al. (2001). Inhibition of lymphangiogenesis with resulting lymphedema in transgenic mice expressing soluble VEGF receptor-3. Nat. Med. 7 (2), 199–205. doi:10.1038/84651
McDuff, S. G. R., Mina, A. I., Brunelle, C. L., Salama, L., Warren, L. E. G., Abouegylah, M., et al. (2019). Timing of lymphedema following treatment for breast cancer: When are patients most at-risk? Int. J. Radiat. Oncol. Biol. Phys. 103 (1), 62–70. doi:10.1016/j.ijrobp.2018.08.036
Mehrara, B. J., and Greene, A. K. (2014). Lymphedema and obesity: Is there a link? Plast. Reconstr. Surg. 134 (1), 154e–160e. doi:10.1097/PRS.0000000000000268
Mehrara, B. J., Park, H. J., Kataru, R. P., Bromberg, J., Coriddi, M., Baik, J. E., et al. (2021). Pilot Study of Anti-Th2 Immunotherapy for the Treatment of Breast Cancer-Related Upper Extremity Lymphedema, 10.Biology
Michopoulos, E., Papathanasiou, G., Vasilopoulos, G., Polikandrioti, M., and Dimakakos, E. (2020). Effectiveness and safety of complete decongestive therapy of phase I: A lymphedema treatment study in the Greek population. Cureus 12 (7), e9264. doi:10.7759/cureus.9264
Miller, C. L., Specht, M. C., Skolny, M. N., Jammallo, L. S., Horick, N., O’Toole, J., et al. (2012). Sentinel lymph node biopsy at the time of mastectomy does not increase the risk of lymphedema: Implications for prophylactic surgery. Breast Cancer Res. Treat. 135 (3), 781–789. doi:10.1007/s10549-012-2231-1
Mishima, T., Ito, Y., Nishizawa, N., Amano, H., Tsujikawa, K., Miyaji, K., et al. (2017). RAMP1 signaling improves lymphedema and promotes lymphangiogenesis in mice. J. Surg. Res. 219, 50–60. doi:10.1016/j.jss.2017.05.124
Moore, J. E., and Bertram, C. D. (2018). Lymphatic system flows. Annu. Rev. Fluid Mech. 50, 459–482. doi:10.1146/annurev-fluid-122316-045259
Mortezaee, K., and Najafi, M. (2020). Immune system in cancer radiotherapy: Resistance mechanisms and therapy perspectives. Crit. Rev. Oncol. Hematol. 157, 103180. doi:10.1016/j.critrevonc.2020.103180
Mortimer, F. S., Simmonds, R. H., Rezvani, M., Robbins, M. E. C., Ryan, T. J., and Hopewell, J. W. (1991). Time-related changes in lymphatic clearance in pig skin after a single dose of 18 Gy of X rays. Br. J. Radiol. 64 (768), 1140–1146. doi:10.1259/0007-1285-64-768-1140
Nagata, H., Arai, T., Soejima, Y., Suzuki, H., Ishii, H., and Hibi, T. (2004). Limited capability of regional lymph nodes to eradicate metastatic cancer cells. Cancer Res. 64 (22), 8239–8248. doi:10.1158/0008-5472.CAN-04-1182
Niedermeier, M., Reich, B., Gomez, M. R., Denzel, A., Schmidbauer, K., Göbel, N., et al. (2009). CD4+ T cells control the differentiation of Gr1+ monocytes into fibrocytes. Proc. Natl. Acad. Sci. U. S. A. 106 (42), 17892–17897. doi:10.1073/pnas.0906070106
Nitti, M. D., Hespe, G. E., Kataru, R. P., García Nores, G. D., Savetsky, I. L., Torrisi, J. S., et al. (2016). Obesity-induced lymphatic dysfunction is reversible with weight loss. J. Physiol. 594 (23), 7073–7087. doi:10.1113/JP273061
Nores, G. D. G., Ly, C. L., Savetsky, I. L., Kataru, R. P., Ghanta, S., Hespe, G. E., et al. (2018). Regulatory T cells mediate local immunosuppression in lymphedema. J. Investig. Dermatol. 138 (2), 325–335. doi:10.1016/j.jid.2017.09.011
Norrmén, C., Tammela, T., Petrova, T. V., and Alitalo, K. (2011). Biological basis of therapeutic lymphangiogenesis. Circulation 123 (12), 1335–1351. doi:10.1161/CIRCULATIONAHA.107.704098
Ogino, R., Hayashida, K., Yamakawa, S., and Morita, E. (2020). Adipose-derived stem cells promote intussusceptive lymphangiogenesis by restricting dermal fibrosis in irradiated tissue of mice. Int. J. Mol. Sci. 21 (11), 38855–E3918. doi:10.3390/ijms21113885
Oliver, G., Kipnis, J., Randolph, G. J., and Harvey, N. L. (2020). The lymphatic vasculature in the 21st century: Novel functional roles in homeostasis and disease. Cell. 182 (2), 270–296. doi:10.1016/j.cell.2020.06.039
Omidi, Z., Kheirkhah, M., Abolghasemi, J., and Haghighat, S. (2020). Effect of lymphedema self-management group-based education compared with social network-based education on quality of life and fear of cancer recurrence in women with breast cancer: A randomized controlled clinical trial. Qual. Life Res. 29 (7), 1789–1800. doi:10.1007/s11136-020-02455-z
OncoLink Team (2022). Treatment for lymphedema: Complete decongestive therapy. [Internet]. [cited 2022 Sep 17]. Available from: https://www.oncolink.org/support/side-effects/lymphedema/lymphedema-what-you-need-to-know/treatment-for-lymphedema-complete-decongestive-therapy.
Oshi, M., Tokumaru, Y., Angarita, F. A., Lee, L., Yan, L., Matsuyama, R., et al. (2021). Adipogenesis in triple-negative breast cancer is associated with unfavorable tumor immune microenvironment and with worse survival. Sci. Rep. 11 (1), 12541. doi:10.1038/s41598-021-91897-7
Overgaard, J. (2007). Hypoxic radiosensitization: Adored and ignored. J. Clin. Oncol. 25 (26), 4066–4074. doi:10.1200/JCO.2007.12.7878
Padera, T. P., Meijer, E. F. J., and Munn, L. L. (2016). The lymphatic system in disease processes and cancer progression. Annu. Rev. Biomed. Eng. 11 (18), 125–158. doi:10.1146/annurev-bioeng-112315-031200
Pappalardo, M., Starnoni, M., Franceschini, G., Baccarani, A., and De Santis, G. (2021). Breast cancer-related lymphedema: Recent updates on diagnosis, severity and available treatments. J. Pers. Med. 11 (5), 402. doi:10.3390/jpm11050402
Park, H. S., Jung, I. M., Choi, G. H., Hahn, S., Yoo, Y. S., and Lee, T. (2013). Modification of a rodent hindlimb model of secondary lymphedema: Surgical radicality versus radiotherapeutic ablation. Biomed. Res. Int. 2013, 208912. doi:10.1155/2013/208912
Park, H. Y., Kwon, H. M., Lim, H. J., Hong, B. K., Lee, J. Y., Park, B. E., et al. (2001). Potential role of leptin in angiogenesis: Leptin induces endothelial cell proliferation and expression of matrix metalloproteinases in vivo and in vitro. Exp. Mol. Med. 33 (2), 95–102. doi:10.1038/emm.2001.17
Paskett, E. D., Dean, J. A., Oliveri, J. M., and Harrop, J. P. (2012). Cancer-related lymphedema risk factors, diagnosis, treatment, and impact: A review. J. Clin. Oncol. 30 (30), 3726–3733. doi:10.1200/JCO.2012.41.8574
Peled, A. W., and Kappos, E. A. (2016). Lipedema: Diagnostic and management challenges. Int. J. Womens Health 8, 389–395. doi:10.2147/IJWH.S106227
Ponder, K. G., and Boise, L. H. (2019). The prodomain of caspase-3 regulates its own removal and caspase activation. Cell. Death Discov. 5 (1), 56–10. doi:10.1038/s41420-019-0142-1
Prevention of Lymphedema (2022). lymphaticsurgery. [Internet]. [cited 2022 Sep 16]. Available from: https://lymphaticsurgery.it/lymphedema-prevention/.
Qin, Y-Y., Li, H., Guo, X-J., Ye, X-F., Wei, X., Zhou, Y-H., et al. (2011). Adjuvant chemotherapy, with or without taxanes, in early or operable breast cancer: A meta-analysis of 19 randomized trials with 30698 patients. PLoS One 6 (11), e26946. doi:10.1371/journal.pone.0026946
Ricci, M. S., and Zong, W. (2006). Chemotherapeutic approaches for targeting cell death pathways. Oncologist 11 (4), 342–357. doi:10.1634/theoncologist.11-4-342
Ridner, S. H. (2013). Pathophysiology of lymphedema. Semin. Oncol. Nurs. 29 (1), 4–11. doi:10.1016/j.soncn.2012.11.002
Roh, K., Cho, S., Park, J. H., Yoo, B. C., Kim, W. K., Kim, S. K., et al. (2017). Therapeutic effects of hyaluronidase on acquired lymphedema using a newly developed mouse limb model. Exp. Biol. Med. 242 (6), 584–592. doi:10.1177/1535370216688570
Roh, K., Kim, S., Kang, H., Ku, J. M., Park, K. W., and Lee, S. (2017). Sulfuretin has therapeutic activity against acquired lymphedema by reducing adipogenesis. Pharmacol. Res. 121, 230–239. doi:10.1016/j.phrs.2017.05.003
Roh, K., Lee, J. hun, Kang, H., Park, K. W., Song, Y., Lee, S., et al. (2020). Synthesis and evaluation of butein derivatives for in vitro and in vivo inflammatory response suppression in lymphedema. Eur. J. Med. Chem. 197, 112280. doi:10.1016/j.ejmech.2020.112280
Rosenzweig, K. E., Chen, C. P., Yom, S. S., and Krug, L. M. (2010). “35 - tumors of the lung, pleura, and mediastinum,” in TL, roach MBT-L and PT of RO. Editors R. T. Hoppe, and Phillips. Third E (Philadelphia: W.B. Saunders), 737–771. Available from: https://www.sciencedirect.com/science/article/pii/B9781416058977000366.
Rovenská, E., and Rovenský, J. (2011). Lymphatic vessels: Structure and function. Isr. Med. Assoc. J. 13 (12), 762–768.
Rowlands, I. J., Beesley, V. L., Janda, M., Hayes, S. C., Obermair, A., Quinn, M. A., et al. (2014). Quality of life of women with lower limb swelling or lymphedema 3-5 years following endometrial cancer. Gynecol. Oncol. 133 (2), 314–318. doi:10.1016/j.ygyno.2014.03.003
Rutkowski, J. M., Moya, M., Johannes, J., Goldman, J., and Swartz, M. A. (2006). Secondary lymphedema in the mouse tail: Lymphatic hyperplasia, VEGF-C upregulation, and the protective role of MMP-9. Microvasc. Res. 72 (3), 161–171. doi:10.1016/j.mvr.2006.05.009
Saito, Y., Nakagami, H., Kaneda, Y., and Morishita, R. (2013). Lymphedema and therapeutic lymphangiogenesis. Biomed. Res. Int. 2013, 804675. doi:10.1155/2013/804675
Saner, F. A. M., Schötzau, A., Mackay, G., Heinzelmann-Schwarz, V., and Sartorius, C. M. (2019). Fibrin-thrombin sealant does not reduce lymphocele formation in patients with inguinofemoral lymphadenectomy for vulvar cancer. Cancer Manag. Res. 11, 3575–3582. doi:10.2147/CMAR.S197143
Sato, A., Kamekura, R., Kawata, K., Kawada, M., Jitsukawa, S., Yamashita, K., et al. (2016). Novel mechanisms of compromised lymphatic endothelial cell homeostasis in obesity: The role of leptin in lymphatic endothelial cell tube formation and proliferation. PLoS One 11 (7), e0158408–e0158417. doi:10.1371/journal.pone.0158408
Savetsky, I. L., Torrisi, J. S., Cuzzone, D. A., Ghanta, S., Albano, N. J., Gardenier, J. C., et al. (2014). Obesity increases inflammation and impairs lymphatic function in a mouse model of lymphedema. Am. J. Physiol. Heart Circ. Physiol. 307 (2), 165–172. doi:10.1152/ajpheart.00244.2014
Schlereth, S. L., Refaian, N., Iden, S., Cursiefen, C., and Heindl, L. M. (2014). Impact of the prolymphangiogenic crosstalk in the tumor microenvironment on lymphatic cancer metastasis. Biomed. Res. Int. 2014, 639058. doi:10.1155/2014/639058
Schling, P., and Löffler, G. (2002). Cross talk between adipose tissue cells: Impact on pathophysiology. News Physiol. Sci. 17 (3), 99–104. doi:10.1152/nips.01349.2001
Seifirad, S. (2020). Pirfenidone: A novel hypothetical treatment for COVID-19. Med. Hypotheses 144, 110005. doi:10.1016/j.mehy.2020.110005
Sgalla, G., Iovene, B., Calvello, M., Ori, M., Varone, F., and Richeldi, L. (2018). Idiopathic pulmonary fibrosis: Pathogenesis and management. Respir. Res. 19 (1), 32. doi:10.1186/s12931-018-0730-2
Sha, M., Jeong, S., Wang, X., Tong, Y., Cao, J., Sun, H-Y., et al. (2019). Tumor-associated lymphangiogenesis predicts unfavorable prognosis of intrahepatic cholangiocarcinoma. BMC Cancer 19 (1), 208. doi:10.1186/s12885-019-5420-z
Shavit, E., Wollina, U., and Alavi, A. (2018). Lipoedema is not lymphoedema: A review of current literature. Int. Wound J. 15 (6), 921–928. doi:10.1111/iwj.12949
Shimizu, Y., Shibata, R., Ishii, M., Ohashi, K., Kambara, T., Uemura, Y., et al. (2013). Adiponectin-mediated modulation of lymphatic vessel formation and lymphedema. J. Am. Heart Assoc. 2 (5), 0004388–e511. doi:10.1161/JAHA.113.000438
Sia, J., Szmyd, R., Hau, E., and Gee, H. E. (2020). Molecular mechanisms of radiation-induced cancer cell death: A primer. Front. Cell. Dev. Biol. 1–8, 41. doi:10.3389/fcell.2020.00041
Srinivasan, R. S., Geng, X., Yang, Y., Wang, Y., Mukatira, S., Studer, M., et al. (2010). The nuclear hormone receptor Coup-TFII is required for the initiation and early maintenance of Prox1 expression in lymphatic endothelial cells. Genes. Dev. 24 (7), 696–707. doi:10.1101/gad.1859310
Swaroop, M. N., Ferguson, C. M., Horick, N. K., Skolny, M. N., Miller, C. L., Jammallo, L. S., et al. (2015). Impact of adjuvant taxane-based chemotherapy on development of breast cancer-related lymphedema: Results from a large prospective cohort. Breast Cancer Res. Treat. 151 (2), 393–403. doi:10.1007/s10549-015-3408-1
Szél, E., Kemény, L., Groma, G., and Szolnoky, G. (2014). Pathophysiological dilemmas of lipedema. Med. Hypotheses 83 (5), 599–606. doi:10.1016/j.mehy.2014.08.011
Tacconi, C., Ungaro, F., Correale, C., Arena, V., Massimino, L., Detmar, M., et al. (2019). Activation of the VEGFC/VEGFR3 pathway induces tumor immune escape in colorectal cancer. Cancer Res. 79 (16), 4196–4210. doi:10.1158/0008-5472.CAN-18-3657
Tapmeier, T. T., Fearn, A., Brown, K., Chowdhury, P., Sacks, S. H., Sheerin, N. S., et al. (2010). Pivotal role of CD4+ T cells in renal fibrosis following ureteric obstruction. Kidney Int. 78 (4), 351–362. doi:10.1038/ki.2010.177
Tashiro, K., Feng, J., Wu, S. H., Mashiko, T., Kanayama, K., Narushima, M., et al. (2017). Pathological changes of adipose tissue in secondary lymphoedema. Br. J. Dermatol. 177 (1), 158–167. doi:10.1111/bjd.15238
Tian, W., Rockson, S. G., Jiang, X., Kim, J., Begaye, A., Shuffle, E. M., et al. (2017). Leukotriene B4 antagonism ameliorates experimental lymphedema. Sci. Transl. Med. 9 (389), eaal3920. doi:10.1126/scitranslmed.aal3920
Toulany, M. (2019). Targeting DNA double-strand break repair pathways to improve radiotherapy response. Genes. 10 (1), E25–E20. doi:10.3390/genes10010025
Tran, B. N. N., Angelo, J. P., Lee, J. H., Ruan, Q. Z., Laurence, R. G., Choi, H. S., et al. (2018). A novel pilot animal model for the surgical prevention of lymphedema: The power of optical imaging. J. Surg. Res. 221, 285–292. doi:10.1016/j.jss.2017.08.029
Tretbar, L. L., Morgan, C. L., Lee, B. B., Simonian, S. J., and Blondeau, B. (2008). Lymphedema: Diagnosis and treatment [internet]. London: Springer-Verlag.
Van Linthout, S., Miteva, K., and Tschöpe, C. (2014). Crosstalk between fibroblasts and inflammatory cells. Cardiovasc. Res. 102 (2), 258–269. doi:10.1093/cvr/cvu062
Varner, J. A. (2011). “Lymphangiogenesis,” in Encyclopedia of cancer [internet]. Editor M. Schwab (Berlin, Heidelberg: Springer Berlin Heidelberg), 2117–2119. doi:10.1007/978-3-642-16483-5_3446
Veikkola, T., Jussila, L., Makinen, T., Karpanen, T., Jeltsch, M., Petrova, T. V., et al. (2001). Signalling via vascular endothelial growth factor receptor-3 is sufficient for lymphangiogenesis in transgenic mice. EMBO J. 20 (6), 1223–1231. doi:10.1093/emboj/20.6.1223
Wang, B., Fu, X., Zhu, M-J., and Du, M. (2017). Retinoic acid inhibits white adipogenesis by disrupting GADD45A-mediated Zfp423 DNA demethylation. J. Mol. Cell. Biol. 9 (4), 338–349. doi:10.1093/jmcb/mjx026
Warren, L. E. G., Miller, C. L., Horick, N., Skolny, M. N., Jammallo, L. S., Sadek, B. T., et al. (2015). The impact of radiation therapy on the risk of lymphedema after treatment for breast cancer: A prospective cohort study. Int. J. Radiat. Oncol. Biol. Phys. 88 (3), 565–571. doi:10.1016/j.ijrobp.2013.11.232
Weiler, M. J., Cribb, M. T., Nepiyushchikh, Z., Nelson, T. S., and Dixon, J. B. (2019). A novel mouse tail lymphedema model for observing lymphatic pump failure during lymphedema development. Sci. Rep. 9 (1), 10405–10415. doi:10.1038/s41598-019-46797-2
Will, P. A., Rafiei, A., Pretze, M., Gazyakan, E., Ziegler, B., Kneser, U., et al. (2020). Evidence of stage progression in a novel, validated fluorescence-navigated and microsurgical-assisted secondary lymphedema rodent model. PLoS One 15 (7), 02359655–e236022. doi:10.1371/journal.pone.0235965
Wu, Q., Li, B., Li, Z., Li, J., Sun, S., and Sun, S. (2019). Cancer-associated adipocytes: Key players in breast cancer progression. J. Hematol. Oncol. 12 (1), 95. doi:10.1186/s13045-019-0778-6
Wu, X., Liu, Y., Zhu, D., Wang, F., Ji, J., and Yan, H. (2021). Early prevention of complex decongestive therapy and rehabilitation exercise for prevention of lower extremity lymphedema after operation of gynecologic cancer. Asian J. Surg. 44 (1), 111–115. doi:10.1016/j.asjsur.2020.03.022
Wynn, T. A. (2008). Cellular and molecular mechanisms of fibrosis. J. Pathol. 214 (2), 199–210. doi:10.1002/path.2277
Yang, C. Y., Nguyen, D. H., Wu, C. W., Fang, Y. H. D., Chao, K. T., Patel, K. M., et al. (2014). Developing a lower limb lymphedema animal model with combined lymphadenectomy and low-dose radiation. Plast. Reconstr. Surg. Glob. Open 2 (3), e121–e126. doi:10.1097/GOX.0000000000000064
Yang, Q. Y., Liang, J. F., Rogers, C. J., Zhao, J. X., Zhu, M. J., and Du, M. (2013). Maternal obesity induces epigenetic modifications to facilitate Zfp423 expression and enhance adipogenic differentiation in fetal mice. Diabetes 62 (11), 3727–3735. doi:10.2337/db13-0433
Yang, Y., Jiang, G., Zhang, P., and Fan, J. (2015). Programmed cell death and its role in inflammation. Mil. Med. Res. 2 (1), 12. doi:10.1186/s40779-015-0039-0
Yeh, Y-W., Cheng, C-C., Yang, S-T., Tseng, C-F., Chang, T-Y., Tsai, S-Y., et al. (2017). Targeting the VEGF-C/VEGFR3 axis suppresses Slug-mediated cancer metastasis and stemness via inhibition of KRAS/YAP1 signaling. Oncotarget 8 (3), 5603–5618. doi:10.18632/oncotarget.13629
Yesil, H., Eyigör, S., Caramat, İ., and Işık, R. (2017). Effects of complex decongestive therapy on quality of life, depression, neuropathic pain, and fatigue in women with breast cancer-related lymphedema. Turk. J. Phys. Med. Rehabil. 63 (4), 329–334. doi:10.5606/tftrd.2017.779
Yoshikawa, N., Kajiyama, H., Otsuka, N., Tamauchi, S., Ikeda, Y., Nishino, K., et al. (2020). The therapeutic effects of goreisan, a traditional Japanese herbal medicine, on lower-limb lymphedema after lymphadenectomy in gynecologic malignancies: A case series study. Evid. Based. Complement. Altern. Med. 2020, 6298293. doi:10.1155/2020/6298293
Zampell, J. C., Aschen, S., Weitman, E. S., Yan, A., Elhadad, S., De Brot, M., et al. (2012). Regulation of adipogenesis by lymphatic fluid stasis: Part I. Adipogenesis, fibrosis, and inflammation. Plast. Reconstr. Surg. 129 (4), 825–834. doi:10.1097/PRS.0b013e3182450b2d
Zeng, H., Zhang, W., Gong, Y., and Xie, C. (2019). Radiotherapy activates autophagy to increase CD8+ T cell infiltration by modulating major histocompatibility complex class-I expression in non-small cell lung cancer. J. Int. Med. Res. 47 (8), 3818–3830. doi:10.1177/0300060519855595
Zheng, J., Jia, L., Mori, S., and Kodama, T. (2018). Evaluation of metastatic niches in distant organs after surgical removal of tumor-bearing lymph nodes. BMC Cancer 18 (1), 608. doi:10.1186/s12885-018-4538-8
Keywords: lymphedema, therapy, phytochemicals, adipogenesis, fibrosis, animal models
Citation: Nurlaila I, Roh K, Yeom C-H, Kang H and Lee S (2022) Acquired lymphedema: Molecular contributors and future directions for developing intervention strategies. Front. Pharmacol. 13:873650. doi: 10.3389/fphar.2022.873650
Received: 17 February 2022; Accepted: 13 October 2022;
Published: 25 October 2022.
Edited by:
Runqiu Jiang, Nanjing University, ChinaReviewed by:
S. R. Narahari, Institute of Applied Dermatology, IndiaXinguo Jiang, Stanford University, United States
Ramalingam Bethunaickan, National Institute of Research in Tuberculosis (ICMR), India
Alexander Kwarteng, Kwame Nkrumah University of Science and Technology, Ghana
Copyright © 2022 Nurlaila, Roh, Yeom, Kang and Lee. This is an open-access article distributed under the terms of the Creative Commons Attribution License (CC BY). The use, distribution or reproduction in other forums is permitted, provided the original author(s) and the copyright owner(s) are credited and that the original publication in this journal is cited, in accordance with accepted academic practice. No use, distribution or reproduction is permitted which does not comply with these terms.
*Correspondence: Sukchan Lee, cell4u@skku.edu; Hee Kang, shehee@khu.ac.kr