Weissella cibaria riboflavin-overproducing and dextran-producing strains useful for the development of functional bread
- 1Departamento de Biotecnología Microbiana y de Plantas, Centro de Investigaciones Biológicas Margarita Salas, CSIC, Madrid, Spain
- 2Instituto de Biología Funcional y Genómica, CSIC-Universidad de Salamanca, Salamanca, Spain
- 3Department of Agriculture Food Natural Science Engineering, University of Foggia, Foggia, Italy
This work describes a method for deriving riboflavin overproducing strains of Weissella cibaria by exposing three strains (BAL3C-5, BAL3C-7, and BAL3C-22) isolated from dough to increasing concentrations of roseoflavin. By this procedure, we selected one mutant overproducing strain from each parental strain (BAL3C-5 B2, BAL3C-7 B2, and BAL3C-22 B2, respectively). Quantification of dextran and riboflavin produced by the parental and mutant strains in a defined medium lacking riboflavin and polysaccharides confirmed that riboflavin was only overproduced by the mutant strains, whereas dextran production was similar in both mutant and parental strains. The molecular basis of the riboflavin overproduction by the mutants was determined by nucleotide sequencing of their rib operons, which encode the enzymes of the riboflavin biosynthetic pathway. We detected a unique mutation in each of the overproducing strains. These mutations, which map in the sensor domain (aptamer) of a regulatory element (the so-called FMN riboswitch) present in the 5’ untranslated region of the rib operon mRNA, appear to be responsible for the riboflavin-overproducing phenotype of the BAL3C-5 B2, BAL3C-7 B2, and BAL3C-22 B2 mutant strains. Furthermore, the molecular basis of dextran production by the six W. cibaria strains has been characterized by (i) the sequencing of their dsr genes encoding dextransucrases, which synthesize dextran using sucrose as substrate, and (ii) the detection of active Dsr proteins by zymograms. Finally, the parental and mutant strains were analyzed for in situ production of riboflavin and dextran during experimental bread making. The results indicate that the mutant strains were able to produce experimental wheat breads biofortified with both riboflavin and dextran and, therefore, may be useful for the manufacture of functional commercial breads.
Introduction
Riboflavin (vitamin B2) is a water-soluble vitamin produced by plants and many microorganisms. Riboflavin is the precursor of flavin mononucleotide (FMN) and flavin adenine dinucleotide (FAD), both of which act as electron carriers in oxidation–reduction reactions, functioning as coenzymes for hundreds of FMN- or FAD-dependent enzymes called flavoproteins (1). Humans do not synthesize riboflavin, which, consequently, has to be obtained from the gut microbiota and the diet. Any excess of riboflavin is eliminated via the urinary tract (2). Deficiency of this vitamin (ariboflavinosis) can provoke damage to the liver or skin, as well as cerebral changes, and alteration of glucose metabolism. Riboflavin is also involved in the prevention of migraine, anemia, cancer, hyperglycemia, hypertension, diabetes mellitus, and directly or indirectly in oxidative stress (2, 3).
The recommended daily dose of riboflavin for a healthy adult is in the range 0.9–1.6 mg (4, 5). In developed countries these dosage levels can be achieved via a balanced diet, as vitamin B2 is present in green vegetables, cereals and dried fruits, and also from eggs, meat and dairy products (6). Nevertheless, ariboflavinosis is a problem in underdeveloped countries, and riboflavin supplements are required by population groups at high risk of deficiency due to specific diets, like vegans/vegetarians (low intake or complete exclusion of dairy products and meat) or pregnant women (especially those with lactose intolerance and/or little meat intake) (7). Furthermore, processing and cooking of vegetable products causes a loss of B-group vitamins, including riboflavin (8).
Some food grade lactic acid bacteria (LAB) synthesize vitamin B2. Thus, fermentation with these LAB offers opportunities to improve the nutritional value of food products and the development of novel foods with an enhanced vitamin content. In addition, the adaptability of LAB to fermentation processes, their biosynthetic capacity and metabolic versatility are features that advocate their industrial application for producing and/or increasing riboflavin concentration in foods.
In order to isolate riboflavin-overproducing strains, the toxic compound roseoflavin (a structural analog of riboflavin) has been widely used as a selection agent for isolating spontaneous riboflavin-overproducing mutants, mainly belonging to Lactococcus lactis, Lactiplantibacillus plantarum (previously Lactobacillus plantarum), Limosilactobacillus fermentum (previously Lactobacillus fermentum), and Leuconostoc mesenteroides species (9–12). Fermented products made with the riboflavin-overproducing strains have been reported as a convenient and efficient food-grade biotechnological procedure (11, 13–18).
Some LAB are also able to produce exopolysaccharides (EPS), including dextrans, which have many industrial applications (19–25). The high molecular weight dextran produced by LAB (e.g., L. mesenteroides and lactobacilli), as well as by Saccharomyces cerevisiae, were labeled as “food grade” by EFSA in the year 2001 (21). These biopolymers possess a linear backbone composed of glucopyranosides with α-(1→6) linkages in the principal chain and with variable percentages of α-(1→4), α-(1→3), or α-(1→2) branches (20). Dextrans are hydrocolloid-like compounds that retain water and increase the viscosity of a food matrix without affecting taste (22). Therefore, dextrans are widely used as food additives (23). For example, they are used to increase palatability: (i) by the bakery industry and (ii) in the production of ice-creams, milk shakes, etc., (24). Various authors have demonstrated the improvement of bread quality by using dough enriched with dextrans (25, 26). LAB dextrans can affect the technological properties of doughs and breads by improving moisture retention and rheology, and increasing the final volume, the softness of the crumb and the shelf life of the final product (22). Furthermore, the addition of enzymatically produced dextran improved the volume and the texture of white bread, and also of bread containing 20% of rye flour (27). Due to their ability to bind water and to retain the CO2 produced during dough fermentation, dextrans can mimic the viscoelastic properties of gluten, making these bacterial EPS highly suitable for the manufacture of gluten-free or low-gluten bakery products (22). A dextran-rich sourdough obtained using a specific L. mesenteroides strain gave rise to several kinds of baked goods (from wheat rich dough products to rye sourdough bread), which had improved freshness, crumb structure, mouthfeel, and softness (28). Dextrans produced in situ by three LAB strains (belonging to W. cibaria, W. confusa, and L. fermentum species) isolated from sorghum significantly improved the rheological properties of a dough made of sorghum and wheat flour (29). Also, the addition of 20% sourdough fermented with a dextran-producing W. cibaria strain reduced significantly crumb hardness in breads based on buckwheat, teff, quinoa and wheat flours, as well as the staling rate in buckwheat, teff and wheat sourdough breads (30). High molecular weight dextrans synthesized by LAB are immuno-stimulants in vitro and appear to have anti-inflammatory properties, supporting their ability to improve the functionality of various products, including the preparation of fermented functional foods (31).
For the above reasons, the food industry is increasingly interested in obtaining LAB strains for the in situ production of dextran during food processing. If these strains were also able to produce riboflavin, then the industrial interest would be even higher. With this aim, we have previously isolated from mother doughs made from rye, three strains of W. cibaria (BAL3C-5, BAL3C-7 y BAL3C-22), which produce riboflavin and high levels of dextran (32). In this work, these three strains were used for selection of three riboflavin-overproducing W. cibaria strains (named BAL3C-5 B2, BAL3C-7 B2, and BAL3C-22 B2). The ability of these B2 strains to synthesize high levels of riboflavin and dextran has been tested and validated both under laboratory growth conditions and with the manufacture of experimental breads.
Materials and methods
Bacteria and growth conditions
The W. cibaria strains used in this work, and their characteristics, are detailed in Table 1. The bacteria were grown at 30°C without shaking in MRS medium (Man, Rogosa, and Sharpe medium, Condalab, Spain), MRS supplemented with 5% sucrose (MRSS), BD Difco™ Riboflavin assay medium (RAM, Thermo Fisher Scientific, USA) containing 2% glucose or RAM supplemented with 2% sucrose (RAMS). The LAB strains were grown in test tubes in a water bath or in microtiter plates (Sterile 96-Well Optical White w/Lid Cell Culture, Thermo Fisher Scientific, Rochester, NY, United States) in a Varioskan Flask System (Thermo Fisher Scientific, Waltham, MA, United States). The bacterial growth was determined by measurement of the optical density at 600 nm (OD600 nm). The growth rate (μ) of the LAB in liquid media was determined as previously described (32). The LAB CFU/mL of the liquid cultures was determined by plating 100 μL aliquots of the appropriate dilutions in MRS-agar medium and further incubation at 30°C for 48 h.
Selection of riboflavin-overproducing strains
The W. cibaria BAL3C-5, BAL3C-7, and BAL3C-22 strains were individually grown in MRS medium to an OD600 nm of 1.5. Then, the bacterial cultures were diluted 1:100 in RAM medium supplemented with roseoflavin (10 μg/mL) and grown until the end of the exponential phase. Afterward, the LAB were exposed to increasing concentrations of roseoflavin (50, 75, 100, 150, and 200 μg/mL) by sequential dilution and further growth in RAM supplemented with the riboflavin homolog. The BAL3C-7 and BAL3C-22 cultures grew even in the presence of roseoflavin at 200 μg/mL, whereas W. cibaria BAL3C-5 showed tolerance up to 150 μg/mL of the toxic compound. The roseoflavin resistant cultures were plated on MRS-agar and incubated for 48 h. Subsequently, three colonies from each roseoflavin-treated parental strain were randomly chosen to evaluate their riboflavin production, and the highest producer of each group was selected and designated as BAL3C-5 B2, BAL3C-7 B2, and BAL3C-22 B2, respectively. Finally, the bacteria present in the selected colonies were recovered by growth in liquid MRS and stored at −80°C in MRS supplemented with glycerol at 20%.
Determination and analysis of the deoxyribonucleic acid sequence of the rib operons and of the dsr genes
The determination of the DNA sequences from the LAB genomes was performed by the “chain termination method” (Sanger sequencing), with a strategy of “primer walking” and utilizing PCR fragments containing either the rib operons or the dsr genes as DNA template. The oligonucleotides used are reported in Supplementary Table 1. The assembled and annotated DNA sequence reads have been deposited in the NCBI-GenBank. The GenBank accession numbers of the dsr genes are: ON677429 (BAL3C-5), ON677430 (BAL3C-7), ON677431 (BAL3C-22), ON677432 (BAL3C-5 B2), ON677433 (BAL3C-7 B2), and ON677434 (BAL3C-22 B2). The GenBank accession numbers of the rib operons are: ON420949 (BAL3C-5), ON420950 (BAL3C-7), ON420951 (BAL3C-22), ON420953 (BAL3C-5 B2), ON420954 (BAL3C-7 B2), and ON420952 (BAL3C-22 B2). The obtained DNA sequences of the dsr genes and the rib operons were compared with those of the selected W. cibaria and W. confusa strains held in GenBank using the BLASTN software (33). Sequences were aligned using the ClustalW software (34). Phylogenetic trees were made/inferred using the neighbour joining analysis (35). Evolutionary analysis was conducted using MEGA11 software (36).
Gene analysis was performed with the EditSeq ® and SeqBuilder Pro ® software (Version 15.3, DNASTAR, Madison, WI, USA) to infer the amino acid sequences of their products. The sequence reads were assembled and compared by using the SeqMan Pro ® and MegAlign ® software (Version 15.3, DNASTAR, Madison, WI, USA), respectively.
Secondary structure predictions of the sensor domain of the FMN riboswitch were obtained by using the RNAfold web server (The ViennaRNA Web Services, version 2.4.18). RNA secondary structure drawings were performed with VARNA 3.9 software (37).
The Signal P 6.0 server was used to predict the leader peptide of the Dsr and the location of the processing site in the W. cibaria strains (38).
In situ detection of dextransucrases (Dsr) produced by the BAL3C-5 B2, BAL3C-7 B2, and BAL3C-22 B2 strains by zymogram
The riboflavin-overproducing LAB were grown overnight in MRS. Then, after sedimentation by centrifugation at 9,000 × g for 10 min, the bacteria were used to inoculate either RAMS or RAM to give an initial OD600 nm of 0.1. The cultures were further incubated at 30°C for 24 h. Afterward, the bacteria were sedimented by centrifugation and the supernatants were subjected to PAGE in an 8% SDS-polyacrylamide gel at constant voltage (100 V). Afterward, Dsr activity was assayed in situ following the method of Miller and Robyt (39) with the modifications previously described (40). Briefly, the method used included a washing step for SDS removal, synthesis of the dextran by Dsr in sodium acetate buffer supplemented with 10% sucrose during 17 h and detection of the Dsr activity by staining with periodic acid-Schiff staining. To estimate the molecular weight of Dsr, the pre-stained Precision Plus Protein Dual Color Standards (Bio-Rad, CA, USA) including 15 polypeptides in the range of 10–250 kDa was used.
Analysis of riboflavin and dextran produced by bacterial cultures grown in RAMS and RAM medium
The cells from overnight cultures of W. cibaria strains grown in MRS were sedimented by centrifugation at 9,000 × g for 10 min and used to inoculate either RAMS or RAM to give an initial OD600 nm of 0.1 and the bacteria were further grown at 30°C as indicated in the Results section.
The fluorescence of the riboflavin present in the bacterial cultures or supernatants was measured upon excitation at a wavelength of 440 nm and detection of emission at a wavelength of 520 nm by using a Varioskan Flask System (Thermo Fisher Scientific, USA). Finally the concentration of the riboflavin was determined using a calibration curve as previously described (12).
The dextran present in the culture supernatants was precipitated with three volumes of absolute ethanol and washed twice with 80% (v/v) ethanol and its concentration estimated as neutral carbohydrate content determined by the phenol–sulphuric acid method using a glucose calibration curve (41).
Laboratory production of experimental breads
The LAB were grown in MRSS at 30°C for 2–3 h with low and constant aeration. After sedimentation of the bacterial cells by centrifugation and two washings with 0.9% saline solution, the cultures were diluted prior to inoculation of the dough. To generate the doughs, refined organic wheat flour with strength W200, from Molinos del Duero y Compañía General de Harinas, S.L. (Carr. de Villalpando, 13, 49029 Zamora) was used. The doughs contained 400 g of flour, 300 mL of water, 5% sucrose, and 0.64% NaCl, and were prepared in a dough mixer. Subsequently, the doughs were divided into portions of 50 g and inoculated with the corresponding LAB [1 × 109 colony forming units (CFU)/mL]. After kneading, each inoculated dough portion was subdivided in another three portions of 15 g each, then fermentation was carried out at 30°C for 16 h and the experimental breads were generated in triplicate by baking at 210°C for 15 min. Prior to baking, the final concentration of LAB (CFU/g) in the fermented dough was determined by plating (Supplementary Table 2).
Analysis of riboflavin, flavins, and dextran concentrations in the laboratory breads
The laboratory prepared breads would contain free riboflavin and other flavins synthesized by the LAB as well as flavins naturally present in the flours. Therefore, soluble riboflavin was extracted and quantified, as well as any other flavins converted into riboflavin prior to quantification. Also, dextran produced by the LAB could be in a soluble or insoluble form. Therefore, soluble and total dextrans were independently extracted and quantified.
To extract free riboflavin and soluble dextran from the breads, samples (1.5 g) were placed in 15 mL Falcon™ (Corning Science, México) tubes and distilled water (3 mL) was added. After vigorous vortexing, samples were incubated at 20°C for 24 h. Then, to convert dextran into isomaltose 150 μL of a solution containing 0.18 g of Chaetomium erraticum dextranase (Sigma-Aldrich, Darmstadt, Germany) was added and samples were incubated at 30°C for 18 h, centrifuged at 8,000 × g for 10 min, and supernatants filtered by using a 0.22 μm filter. Afterward, aliquots were stored at −20°C until further analysis.
To extract flavins from the breads and to convert them into riboflavin, samples (1 g) were placed in 25 mL flasks, and 0.1 M HCl (10 mL) was added. Then, the samples were autoclaved at 121°C for 30 min. Subsequently, the pH of the suspensions was neutralized to a pH 6.5 by addition of 1.4 mL of 4 M sodium acetate pH 9.5. Furthermore, to convert dextran into isomaltose, 0.5 mL of a solution containing 0.6 g of C. erraticum dextranase was added and the samples were incubated at 30°C for 18 h. To remove solid residues, the samples were centrifuged at 8,000 × g for 10 min, and the supernatants filtered through a cheesecloth and subsequently through a 0.22 μm filter. Afterward, the filtrate was aliquoted and kept frozen at −20°C until further analysis.
The concentration of riboflavin in the processed samples was determined by measuring its fluorescence as described in section “Analysis of riboflavin and dextran produced by bacterial cultures grown in RAMS and RAM medium”, as well as by chromatographic analyses. These latter analyses were performed with a HPLC equipment composed of a degasser system, a quaternary pump, an automated injector, a column oven, an ultraviolet–visible diode array detector (UV–vis-DAD) and a fluorescence detector (FLD) (Agilent-1200/1260 Infinity II Series, Palo Alto, CA, USA). A Kinetex EVO C 18 100 Å 4.6 × 150 mm, 5 μm internal diameter analytical column with a SecurityGuard ULTRA Cartridges UHPLC C18 (Phenomenex, Torrance, CA, USA) thermostated at 40°C, was used for the analytical determination of riboflavin. A ChemStation computer software (Agilent, Palo Alto, CA, USA) recorded signals. HPLC analyses were achieved by an isocratic elution at 0.6 mL/min using the conditions described by Jakobsen et al. (42), with a mobile phase constituted by a methanol/water (40:60 v/v) mixture, freshly prepared every day. A fluorescence detector set at an excitation wavelength of 449 nm and an emission wavelength of 516 nm monitored the eluate. Spectral analyses of the riboflavin standard and samples were performed to verify the method’s selectivity.
The total dextran concentration was determined by quantification of the isomaltose generated by the polymer hydrolysis by gas chromatography-mass spectrometry (GC-MS) using myo-inositol as internal standard, after derivatization with hydroxylamine chloride in pyridine to form the oxime of the isomaltose and generation of the trimethylsilylated derivative by treatment with bis-trimethylsilyl trifluoroacetamide as described (40). Quantification of isomaltose concentration was performed according to peak area, corrected with the response factors calculated for each compound using the internal standard and the software GC-ChemStation Rev. E.02.00 (2008) from Agilent (Palo Alto, CA, USA).
Statistical analysis
Exopolysaccharides (EPS) produced by the strains in growth media was quantified using the phenol-sulphuric acid method. T-tests were performed to determine if the values of the parental and the mutant strains were significantly different, and p-values were adjusted for multiple testing by the Benjamini and Hochberg method (43). In addition, differences between groups for EPS production by all strains (mutant and parental), as well as for total and soluble dextran fractions in breads, were performed with a one-way analysis of variance, and mean pairwise comparisons were computed with a Tukey’s test. Results are marked with letters and means with the same letter are not significantly different (α = 0.05). All analyses were performed with the R software version 4.1.3 (44).
In the case of evaluation of riboflavin production in growth medium, for every parental-mutant pair, the effects of strains, culture media, and their interaction were analyzed with a two-way analysis of variance. A p value ≤ 0.05 was considered significant. When interactions were significant, independent t-tests were performed for each medium, and p-values were adjusted for multiple testing by the Benjamini and Hochberg method (43). Again, to establish differences between groups for the riboflavin produced by all strains (mutant and parental) as well as for flavins and free riboflavin in breads, a one-way analysis of variance was performed and mean pairwise comparisons were computed with a Tukey’s test. Results are shown with letters and means with the same letter are not significantly different (α = 0.05). All analyses were performed with the R software version 4.1.3 (44).
Results and discussion
Selection and analysis of W. cibaria riboflavin-overproducing strains
Three W. cibaria strains (BAL3C-5, BAL3C-7, and BAL3C-22) previously isolated from fermented rye dough and characterized for their ability to produce both dextran and riboflavin (32) were selected in this study and treated with roseoflavin, with the aim to identify riboflavin-overproducing strains, potentially useful for the production of functional bread enriched in riboflavin and dextran.
The three parental strains were independently subjected to cycles of treatment with increasing concentrations of roseoflavin and three strains resistant to the riboflavin homologue (one from each wild-type treated strain) were obtained. These spontaneous mutants were designated as BAL3C-5 B2, BAL3C-7 B2, and BAL3C-22 B2 and, using their parental strains as control, were analyzed to determine their capability to produce riboflavin and dextran in liquid medium. The RAMS medium (containing 2% sucrose and lacking riboflavin and polysaccharides), which we have shown to be suitable for the analysis of the riboflavin and dextran produced by BAL3C-5, BAL3C-7, and BAL3C-22 strains (33), was used.
The Dsr from LAB produce extracellularly dextran by hydrolysis of sucrose molecules coupled to the transfer of glucose to the nascent α-glucan polymer (16). Moreover, we have shown that riboflavin produced by W. cibaria BAL3C-5, BAL3C-7, and BAL3C-22, as is the case in other LAB (12, 45), can be detected and quantified in culture supernatants (32). Therefore, after growing the six LAB for 23 h in RAMS, the content of dextran and riboflavin present in the culture supernatants was determined, and the final biomass estimated by measuring the OD600 nm and by plating (Table 2).
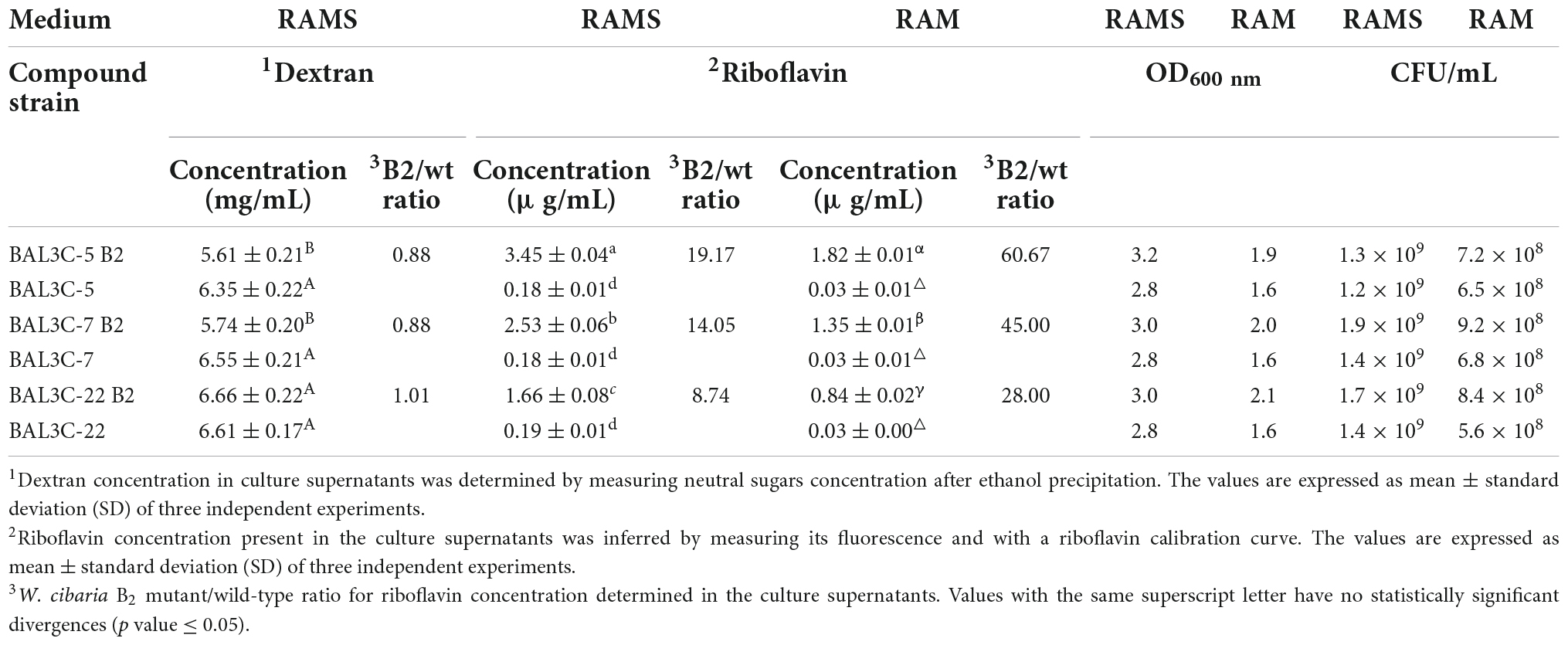
Table 2. Comparative analysis of riboflavin and dextran production by the wild-type and mutant W. cibaria strains in RAMS and RAM media.
The six strains produced similar levels of dextran, ranging from 5.61 to 6.66 mg/mL with BAL3C-22 B2 being the highest producer. Moreover, statistical analysis revealed that only BAL3C-5 B2 and BAL3C-7 B2 produced slightly lower levels of dextran compared to the parental BAL3C-5 (p = 0.0323) and BAL3C-7 (p = 0.0209) (Table 2 and Supplementary Figure 1).
With regards to the riboflavin levels, bacterial cultures of the six strains grown in RAMS or RAM were tested. The B2 strains produced statistically significant higher levels of riboflavin than their corresponding or the other two parental strains (p = 7.33 × 10–20 or p = 3.45 × 10–21 in RAMS or RAM) (Table 2 and Supplementary Figures 2, 3). After 23 h of growth in RAMS (containing glucose plus sucrose) versus in RAM (containing only glucose), all the strains reached a higher OD600 nm (3.2–2.8 in RAMS and 2.1–1.6 in RAM) correlating with higher levels of CFU/mL (1.9 × 109–1.2 × 109 in RAMS and 9.2 × 108–5.6 × 108 in RAM) and consequently produced higher levels of riboflavin. Apart from that, the behavior of the strains was the same in both media, with the highest levels of riboflavin being synthesized and released to the media by BAL3C-5 B2 (3.45 and 1.82 μg/mL in RAMS and RAM, respectively). In addition, the increase of the riboflavin production by the B2 mutants compared with the production of the corresponding parental strains was more pronounced in RAM (ranging from 28- to 61-fold) than in RAMS (ranging from 8- to 19-fold), with the lowest being for the pair BAL3C-22 B2 and BAL3C-22.
Furthermore, a simultaneous analysis of the bacterial growth in RAM or in RAMS by measuring their OD600 nm and production of riboflavin by fluorescence detection in real time was performed and the growth rates during the exponential phase of growth inferred (Figure 1). The highest μ was observed for the BAL3C-7 B2 strain when grown in either RAM (μ = 0.99 h−1) or RAMS (μ = 0.93 h−1) medium and the lowest for BAL3C-5 in both RAM (μ = 0.78 h−1) and RAMS (μ = 0.79 h−1). Moreover, correlating with the data presented in Table 2, the six strains reached a higher final biomass in RAMS than in RAM. In addition, as expected, the BAL3C-5 B2, BAL3C-7 B2, and BAL3C-22 B2 strains produced high levels of riboflavin in either RAMS or RAM media and during both the exponential and stationary phases of growth.
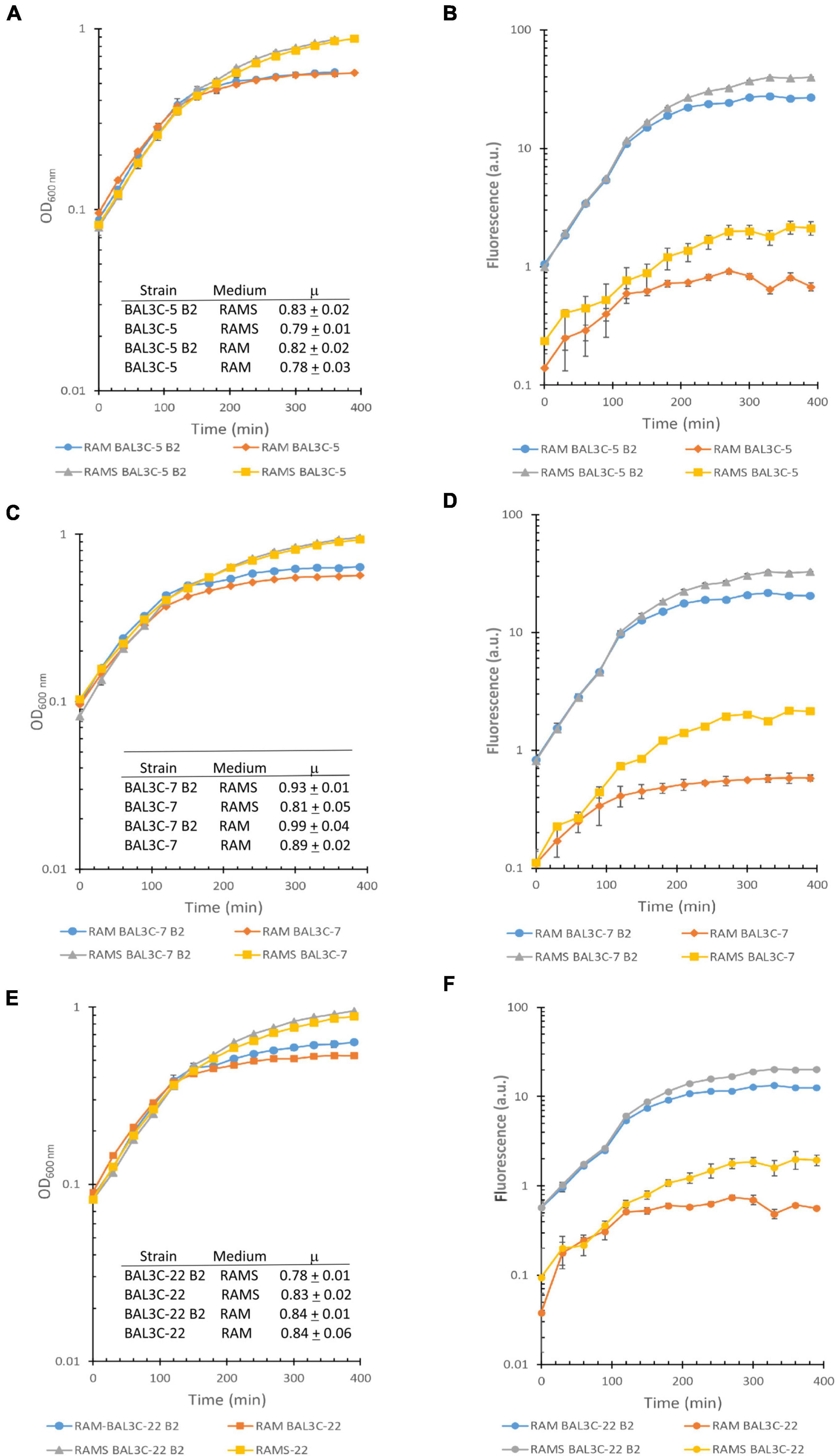
Figure 1. Detection of riboflavin production by the Weissella cibaria strains. Real time monitoring of growth (A,C,E) and riboflavin (B,D,F) production of the different W. cibaria strains analyzed in this study [BAL3C-5 and BAL3C-5 B2 (A,B); BAL3C-7 and BAL3C-7 B2 (C,D); BAL3C-22 and BAL3C-22 B2 (E,F) in RAM or in RAMS]. In the left panels, the plots display the growth curves of each strain, with the symbols and error bars representing, respectively, the average and standard deviation of three independent experiments. Growth rate constants (μ) were calculated during the exponential phase for each of the strains in the two-culture medium. The μ values are expressed as mean ± standard deviation of three independent experiments. In the right panels, the plots display the riboflavin production curves of each strain. Symbols and error bars represent, respectively, the average and standard deviation of three independent riboflavin fluorescence measurements.
Taken together our results reveal that the W. cibaria BAL3C-5 B2, BAL3C-7 B2, and BAL3C-22 B2 strains overproduce riboflavin and maintain the capability to produce dextran.
Characterization of the mutations of the riboflavin-overproducing strains
The enzymes involved in the riboflavin biosynthetic pathway are encoded by the rib operon composed of the ribG, ribB, ribA, and ribH genes, whose expression is regulated by a transcriptional FMN riboswitch located in the 5’ untranslated region of the messenger RNA. This regulatory element consists of a sensor domain (the aptamer) that contains five hairpins (P2/L2 to P6/L6) and is closed by the P1 basal helix (Figure 2A), whose 3’-end is connected to the regulatory domain (or expression platform). The regulatory domain is predicted to adopt two alternative configurations that correspond to the ON state (characterized by an anti-terminator secondary structure) and to the OFF state, which includes an intrinsic transcriptional terminator preventing expression of the rib operon [Figure 2B; (45)]. Binding of the effector (FMN) to the aptamer induces a conformational change in the expression platform from the ON to the OFF state, thus avoiding the metabolic burden of expressing the genes involved in riboflavin biosynthesis when not required.
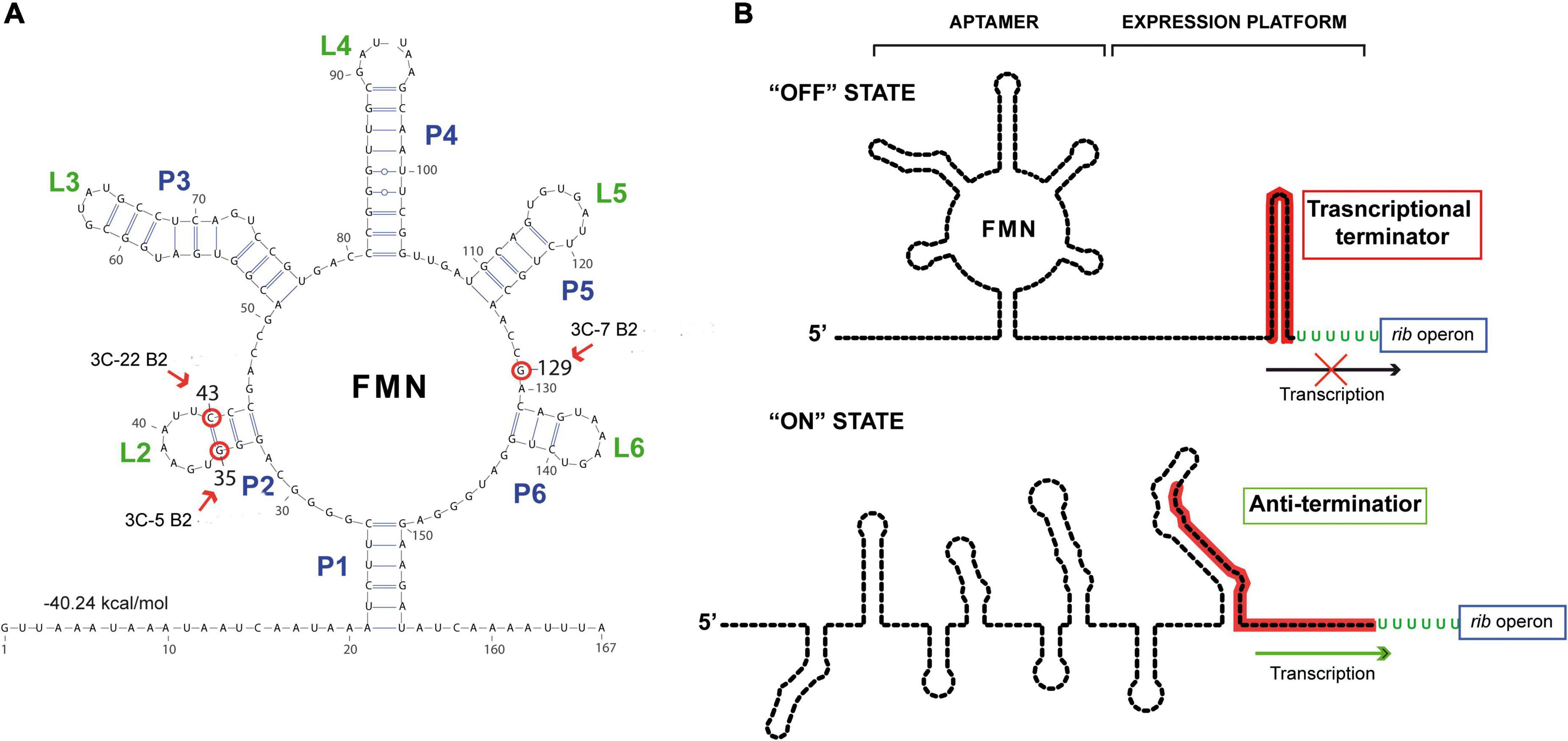
Figure 2. Secondary structure predictions of the riboswitch domains. (A) Secondary structure prediction obtained by using RNAfold web server with the sequence of the nascent rib operon mRNA of Weissella cibaria BAL3C-5. The free energy associated with this aptameric conformation is shown. Red arrows and red circles indicate altered ribonucleotides in the aptamer in BAL3C-22 B2 (U at position 43), BAL3C-5 B2 (U at position 35) and BAL3C-7 B2 (A at position 129) due to the mutations present in the DNA of these strains. The strain containing the base change is indicated in each case. (B) Schematic representation of the possible “ON” and “OFF” conformations of the FMN riboswitch. The FMN riboswitch mechanism of gene expression is based on ligand binding and signal transduction through conformational changes from the “ON” state (anti-terminator structure) to the “OFF” state (transcriptional terminator). Aptamer and expression platform domains are indicated over the “OFF” state structure. The sequence involved in the formation of the transcriptional terminator is red colored. Arrows indicate transcription of the rib operon.
Roseoflavin-resistant mutants of LAB usually harbor mutations that impair the regulatory activity of the rib operon riboswitch, enabling the bacterial cells to synthesize riboflavin in the presence of either FMN or roseoflavin. Therefore, to localize and characterize the mutations of the riboflavin-overproducing W. cibaria strains, the DNA sequence of the entire rib operon, including the FMN riboswitch, was determined for each of them as well as for the respective parental strains. No differences were detected between the DNA sequences of the rib operons of the three parental strains. The operons of BAL3C-5 B2, BAL3C-7 B2, and BAL3C-22 B2 each showed only one mutation located in the untranslated region of the rib mRNA.
Analysis of the folding of this region with the RNAfold software predicted the existence of an FMN riboswitch aptamer (matching the consensus sequence and structure) where the three mutations were located (Figure 2A). In this context, strain BAL3C-5 B2, which showed the highest production of riboflavin in liquid growth media, carries in its DNA the mutation G35T, and as a consequence in its FMN riboswitch there is a U instead of a G at the position 35 (position 1 corresponding to the putative start site of the rib mRNA), a change that would destabilize the P2 stem of the aptamer. The BAL3C-22 B2 strain has the lower riboflavin-overproducing phenotype and contains the mutation C43T, that results in an aptamer with a U43 instead of the C43 (which is predicted to pair with the G35 in the wild-type riboswitch). This mutation should also affect the stability of the P2 helix, although to a lesser extent, because a base pair GU could still be formed. Finally, BAL3C-7 B2 carries the mutation G129A, that results in the change of one ribonucleotide in the aptamer at position 129. Previous 3D crystallographic studies performed with the FMN riboswitch of Fusobacterium nucleatum have demonstrated that the same relative position in the aptamer is involved in the direct interaction with the FMN effector (46).
The location of the mutations in BAL3C-5 B2, BAL3C-7 B2, and BAL3C-22 B2 indicates that these changes could impair or prevent the binding of FMN to the aptamer, thereby avoiding its inhibitory effect and being responsible for the riboflavin-overproducing phenotype of these strains.
To support this hypothesis, growth, and production of riboflavin by the mutant and parental strains in RAMS supplemented with FMN was analyzed in real time (Supplementary Figure 4). All the strains showed the same growth pattern (Supplementary Figure 4A). The addition of FMN altered the pattern of fluorescence of the cultures of the three parental strains ascribed to flavins production during growth. By contrast, with the results obtained in absence of FMN (Supplementary Figure 4B), with detection of riboflavin production from the beginning of the growth (Figures 1B,D,F), the fluorescence decreased during the early stage of growth, as we have previously detected for L. plantarum wild–type strains (12), and did not start to increase until the middle of the exponential phase (Supplementary Figure 4), as we have previously observed for these W. cibaria strains in the presence of riboflavin (32). These results were expected due to an inhibitory effect of the FMN, upon internalization, in the riboflavin biosynthesis, proceeding to a latter expression of the rib operon when levels of the effector are exhausted in the cells. However, as expected the presence of the FMN had no influence on riboflavin production by the three mutant strains, since increase of fluorescence due to the presence of flavins was observed from the beginning of the exponential growth phase, as we have previously observed for riboflavin-overproducing L. plantarum mutants also carrying point mutations located in its corresponding aptamer of the FMN riboswitch (45). Consequently, these results and the fact that no mutations were found in the rib operon encoding the enzymes involved in the riboflavin biosynthetic pathway, strongly suggest that the mutations in the FMN-riboswitch are responsible for the riboflavin-overproducing phenotype of the W. cibaria B2 strains. However, to prove this hypothesis a deep transcriptional analysis has to be performed in a future work, as has been already done for a L. plantarum riboflavin-overproducing mutant (45).
In addition, we performed a comparative analysis of the rib operon sequences of some W. cibaria and W. confusa strains whose genome sequences are publicly available. In this analysis, the rib operons of BAL3C-5, BAL3C-7, and BAL3C-22 were included. The DNA sequence of the rib operon from the three W. cibaria parental strains showed 100% identity with that of W. cibaria CH2, a strain isolated from cheese of the occidental Himalayas. Usually, phylogenetic investigations of the genus Weissella are based on rrs (encoding the 16S rRNA) and/or pheS genes sequences (32, 47). Recently, a comparative genomic analysis of Weissella species allowed the construction of a whole genome phylogenetic tree based on single-copy core orthologs (48). Either based on single gene sequences or whole genome comparisons, W. cibaria and W. confusa appear to be phylogenetically closely related, grouping together in the same cluster.
The phylogenetic relationship of BAL3C-5, BAL3C-7, and BAL3C-22 with other W. cibaria and W. confusa strains based on rib operon sequences is depicted in Figure 3A. In spite of the high degree of rib operon sequence similarity shared by all the strains, BAL3C-5, BAL3C-7, and BAL3C-22 grouped together with CH2 in a separate branch, as expected by its 100% sequence identity. It is worth noting that all W. cibaria and W. confusa strains included in the analysis were placed in divergent branches although, as stated before, the two species belong to the same phylogenetic cluster. This fact may support the use of the rib operon as marker for the discrimination between Weissella species in future phylogenetic studies.
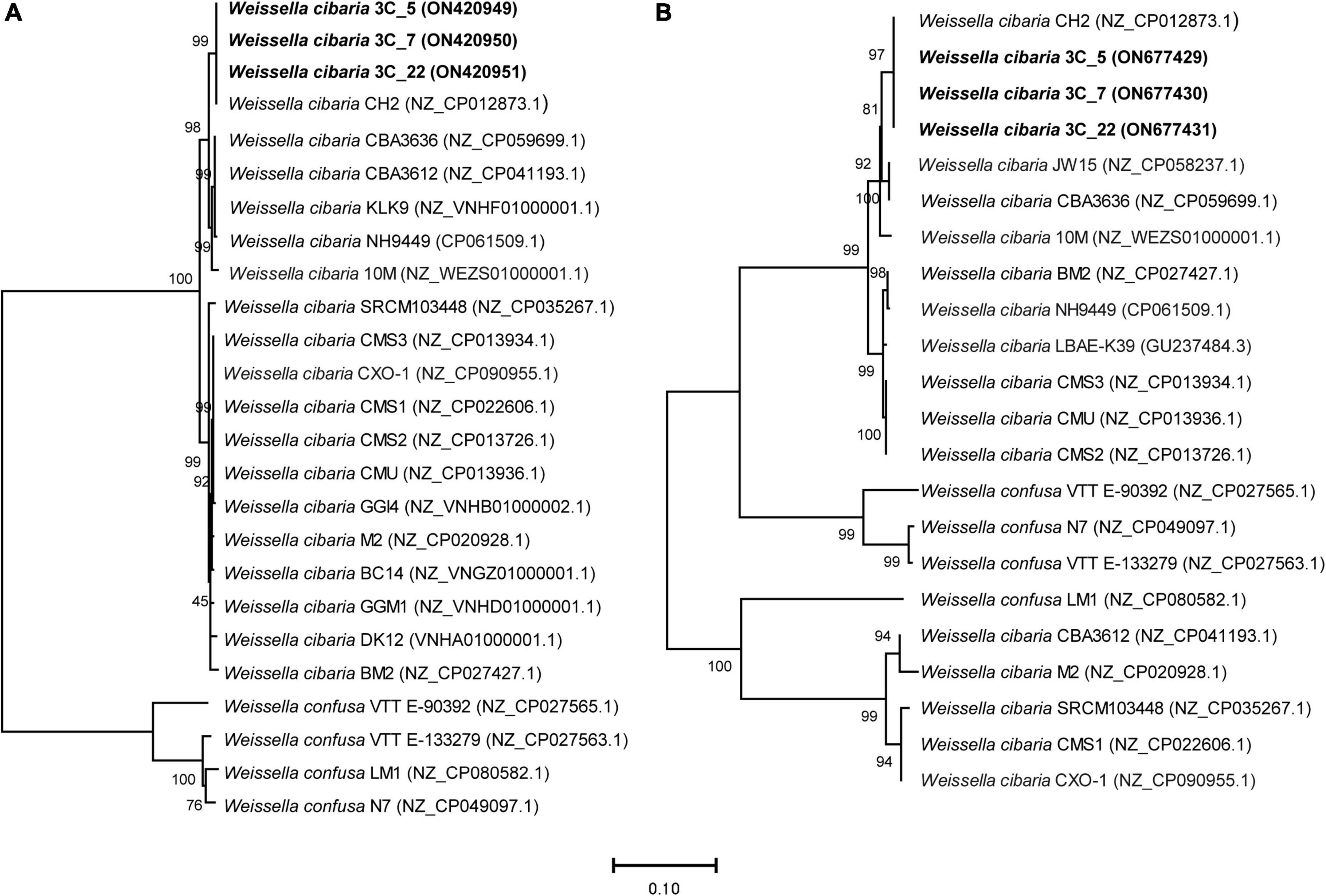
Figure 3. Neighbor-joining phylogenetic rooted trees based on the sequences of the rib operon genes [3,290 nt; panel (A)] and of the dsr genes [4,546 nt; panel (B)], showing the taxonomic location of the analyzed strains. The percentage of replicate trees in which the associated taxa clustered together in the bootstrap test (1,000 replicates) are shown above the branches (64). The tree is drawn to scale, with branch lengths in the same units as those of the evolutionary distances used to infer the phylogenetic tree. The evolutionary distances were computed using the Maximum Composite Likelihood method (65) and are in the units of the number of base substitutions per site. This analysis involved 25 nucleotide sequences in the case of the rib operon sequences and 22 for the dsr gene sequences. All ambiguous positions were removed for each sequence pair (pairwise deletion option). The total number of positions in the final dataset was 3,650 and 4,584 position for the rib operon and dsr analysis, respectively. Accession numbers from GenBank are given in brackets.
Identification of the dsr genes and detection of active Dsr enzymes synthesized by the mutant strains
Only one Dsr enzyme encoded by a dsr gene is required for the synthesis of the dextran. Therefore, the DNA sequences of the dsr genes from the three parental and the three mutant strains were determined. The genes of the six strains had a length of 4,342 bp and were identical, and also 99% identical to the corresponding gene of W. cibaria CH2. We also analyzed the phylogenetic relationship of these strains with other W. cibaria and W. confusa strains, based on the dsr gene sequences (Figure 3B). As observed in the tree based on the rib operon sequences, W. cibaria BAL3C-5, BAL3C-7, and BAL3C-22 dsr genes grouped together with that of the CH2 strain in the same branch, confirming the close relationship between the four strains. However, this group is the exception when comparing the trees based on the dsr gene and on the rib operon, since different evolutionary relationships were detected. The most important observation was that all the W. cibaria strains analyzed were not placed together in the same divergent branch, indicating that the use of this marker will not discriminate between W. cibaria and W. confusa within the same phylogenetic cluster.
The inferred sequence of amino acids of Dsr showed that the dsr genes encode a protein of 159.099 kDa, which has an amino-terminal signal peptide involved in protein processing and secretion in Gram-positive bacteria. The analysis of the amino acid sequence of Dsr with the program Signal P 6.0 allowed us to predict that the processed extracellular Dsr has a molecular mass of 156.411 kDa.
Furthermore, since Dsr is extracellular, and with the aim of detecting the active form of the enzyme, supernatants of cultures of BAL3C-5 B2, BAL3C-7 B2, and BAL3C-22 B2 grown in RAMS or in RAM for 24 h were used to perform zymograms by in situ synthesis of dextrans after fractionation in SDS-polyacrylamide gel (Figure 4). The development of the gel revealed only one intense band at the expected position (156 kDa) in the culture supernatants of the three strains grown in RAMS and a very faint band in the samples grown in RAM. As a consequence, the results revealed that the production of active Dsr in this W. cibaria strains is induced when sucrose is present in the growth medium.
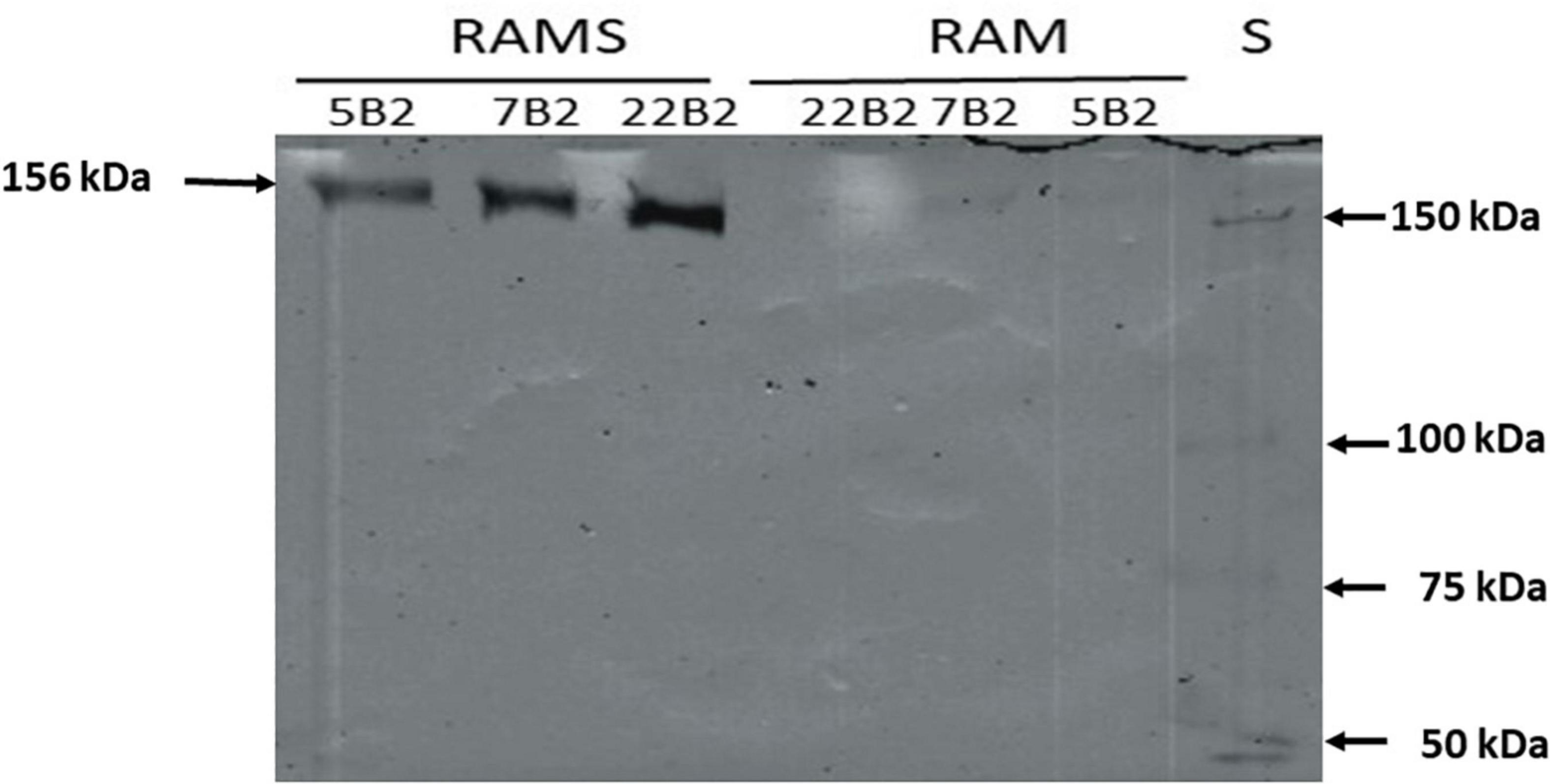
Figure 4. In situ detection of dextransucrase activity in cell free supernatants of LAB cultures. The indicated Weissella cibaria riboflavin-overproducing strains were grown in the presence of glucose [Riboflavin assay medium (RAM)] or glucose plus sucrose [Riboflavin assay medium with sucrose (RAMS)], the culture supernatants were subjected to SDS-PAGE and after protein renaturation, were analyzed in situ for Dsr activity. S, protein Mw standard.
We have previously detected, with the same in situ methodology, this behavior for W. cibaria Av2ou, W. confusa FS54 and Leuconostoc lactis AV1n strains isolated from various Tunisian habitats (40). Moreover, in the case of L. lactis AV1n isolated from avocado, like for L. mesenteroides NRRL B-512F (49), it has been demonstrated that in the presence of sucrose induction of the dsr genes expression takes place at the transcriptional level (50). However, this is not a general feature of LAB, since zymogram analysis of soluble Dsr from five W. cibaria strains and one W. confusa isolated from sourdoughs revealed higher enzyme activity when the bacteria were previously grown in the presence of glucose instead of sucrose (51). This behavior was also observed for the W. confusa V30 strain isolated from an olive tree leaf (40) and for Lactobacillus sakei MN1 isolated from meat (52). Consequently, two different patterns of response to the presence of sucrose have been detected for the expression of the dsr of LAB independently of the isolation habitat including strains belonging to the Weissella genus and W. cibaria species.
Evaluation of BAL3C-5 B2, BAL3C-7 B2, and BAL3C-22 B2 for experimental bread making
Preparation of experimental breads and extraction of dextran and flavins
The riboflavin-overproducing and the parental W. cibaria strains were independently tested for their capability to produce riboflavin and dextran in wheat doughs supplemented with sucrose and fermented for 16 h. To induce the synthesis of the Dsr, the W. cibaria were grown in MRSS medium containing sucrose, prior to inoculation of the doughs. As a control, a dough without W. cibaria strains was used. After the fermentation period, LAB viability was evaluated by plating (Supplementary Table 2). In the control dough, a bacterial concentration of 4.37 × 106 CFU/g was detected, whereas in all the other doughs inoculated with W. cibaria strains, the LAB concentration ranged from 9.1 × 108–2.8 × 109 CFU/g. Since the LAB were inoculated prior to fermentation, at a concentration of 1 × 108 CFU/g, the results suggested a good survival and further growth of the inoculated W. cibaria strains during dough fermentation. Furthermore, after the baking process, breads were analyzed for their content in flavins and dextran (Figures 5, 6).
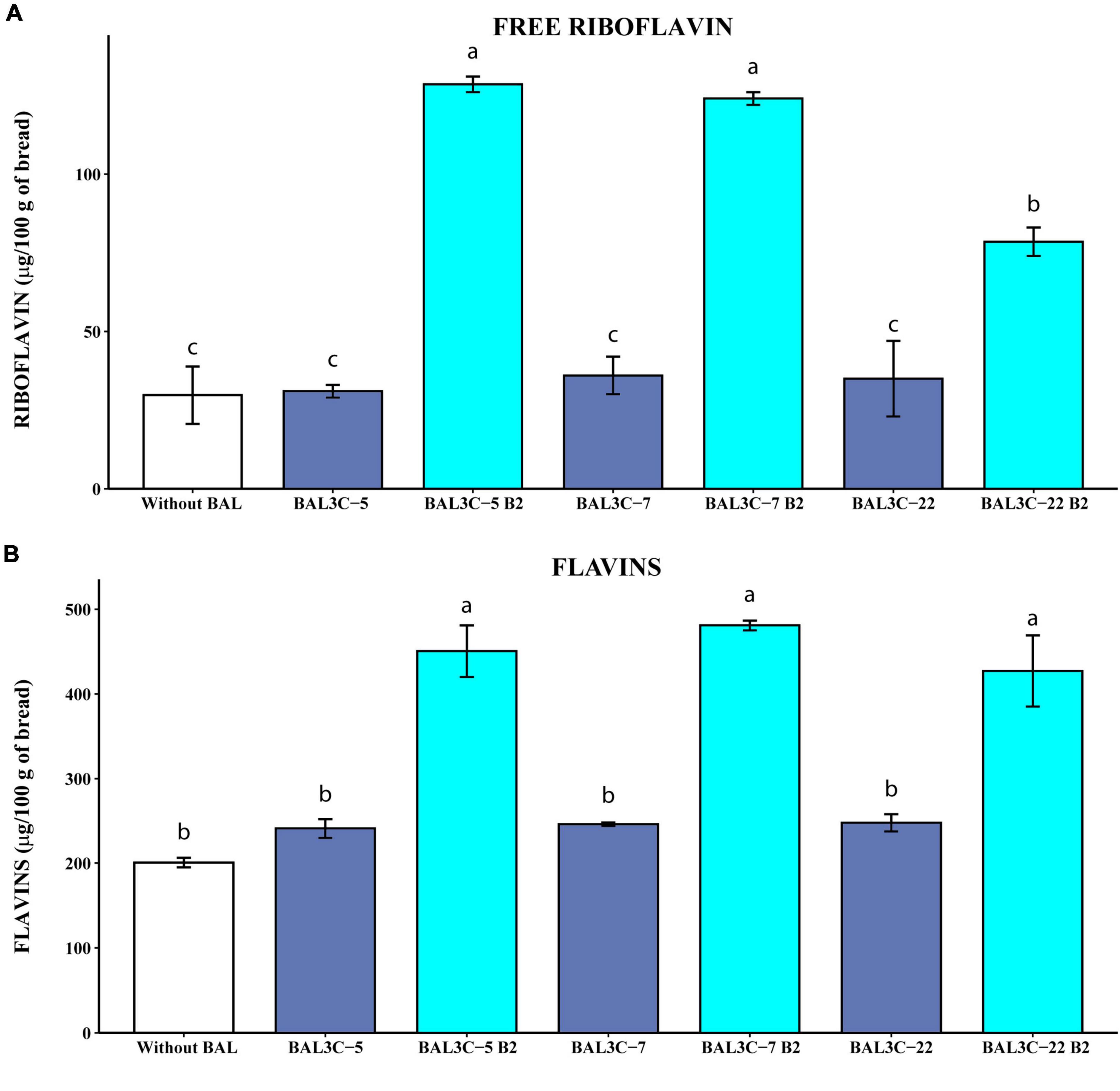
Figure 5. Determination of the free riboflavin and the flavin levels in breads produced with Weissella cibaria strains. Levels of water-soluble riboflavin (free riboflavin) (A) or riboflavin generated by acidic hydrolysis at high temperature of flavins (flavins) (B) present in the breads are depicted. The means of two determinations and the standard deviations are indicated. Values with different superscript letters indicate that the levels differed significantly (p ≤ 0.05, see details of statistical analysis in Supplementary Figure 6).
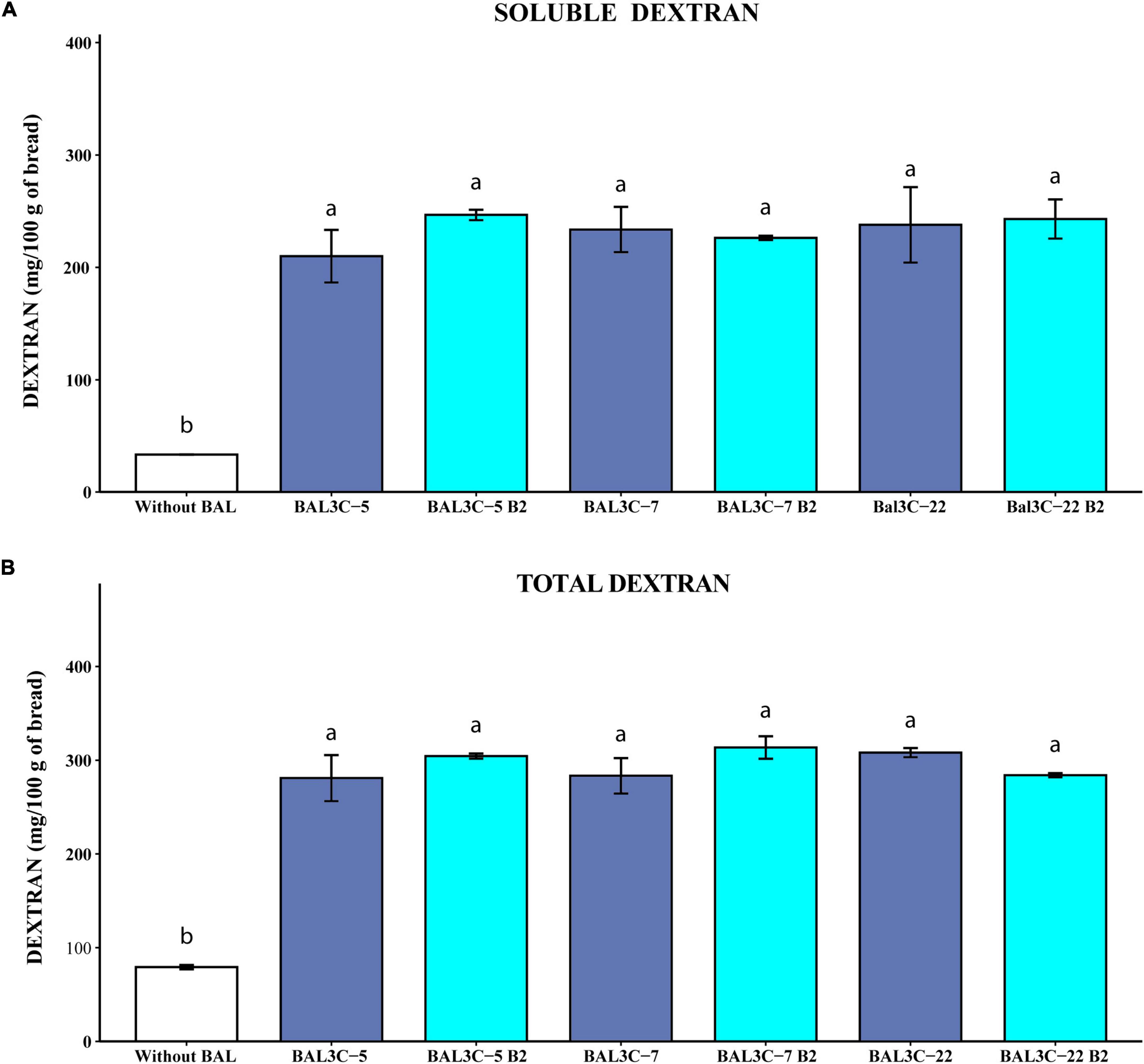
Figure 6. Determination of soluble and total dextran levels in breads produced with Weissella cibaria strains. Levels of water soluble dextran (soluble) (A) or dextran extracted after acidic and thermal treatments and hydrolyzed with dextranase (total) (B) present in the breads are indicated. The means of two determinations and the standard deviations are indicated. Different superscript letters indicate that the levels differed significantly (p ≤ 0.05, see details of statistical analysis in Supplementary Figure 7).
To determine specifically the concentration of the dextran without interference of other potential polyglucans present in bread, the C. erraticum dextranase was used to hydrolyze the polymer, and the isomaltose generated was detected and quantitated by GC-MS analysis (Figure 5 and Supplementary Figure 5). The dextranase of C. erraticum specifically hydrolyses dextran to isomaltose (53), and it has been previously used in a specific manner to convert dextran synthesized in situ by W. confusa in wheat sourdough into the disaccharide (54). However, in that particular case, the dextranase was used in conjunction with an α-glucosidase from Aspergillus niger, to convert the isomaltose in glucose, that was subsequently quantified (54).
In addition, two procedures were applied for the extraction of the compounds of interest. On one hand, free riboflavin and soluble dextran were extracted from the breads by incubation at 20°C for 24 h, upon water addition. Then, the riboflavin fluorescence (Figure 5A) and the levels of isomaltose generated by dextran hydrolysis (Figure 6A) were quantified. On the other hand, to determine levels of flavins and total dextran present in the breads, the extraction and conversion was performed at high temperature under acidic conditions and further treatment with the dextranase of C. erraticum. This procedure resulted in conversion of the flavins present in the sample into riboflavin (Figure 5B) and dextran to isomaltose (Figure 6B). Next, riboflavin was quantified by fluorescence spectrometry, directly (Figure 6) or after HPLC fractionation (Supplementary Table 3).
Free riboflavin and flavins content in experimental breads
The results obtained when the levels of riboflavin were measured without HPLC fractionation, revealed that the parental strains did not increase significantly the concentration of either free riboflavin or flavins (Figure 5) compared with those obtained by fermentation with only the dough microbiota. However, the riboflavin-overproducing strains increased with statistical significance the concentrations of free riboflavin (6.1- to 10-fold; p = 2.76 × 10–5) (Supplementary Figure 6A) and flavins (2.1- to 2.4-fold; p = 8.32 × 10–5) (Supplementary Figure 6B) over the control sample levels. Likewise, the three mutant strains enriched the breads in riboflavin and flavins more than their corresponding parental strains (Supplementary Figure 6). In addition, both BAL3C-5 B2 and BAL3C-7 B2 provided similarly high levels of free riboflavin (around 125 μg/100 g of bread). Also, in the breads obtained here by fermentation only with one of these two mutant LAB, in the absence of yeast, we detected around 465 μg of flavins converted into riboflavin/100 g, this value being similar to the 681 μg/100 g obtained with the riboflavin-overproducing mutant L. plantarum B2 of industrial interest during bread production in co-fermentation with yeast, taking into account that products obtained with yeast only contained up to 241 μg/100 g (55). Thus, a synergetic effect of L. plantarum B2 and yeast was observed, also detected for L. fermentum mutants (11) and for BAL3C-5 B2 and BAL3C-7 B2 strains (unpublished results). Furthermore, the levels of riboflavin in the breads produced with each of the 3 W. cibaria mutant strains (428–480 μg/100 g) were higher than that reported for Czech white bread (208 μg/100 g) (56) or for the top five white bread products high in riboflavin (337–383 μg/100 g).1 Thus, these results show a good ability of the riboflavin-overproducing W. cibaria to generate bread biofortified with vitamin B2.
We have previously shown that riboflavin produced by riboflavin-overproducing L. plantarum mutants in growth medium could be reliably quantified by direct measurement of its fluorescence without HPLC fractionation, and that the low levels produced by the parental strains could not be detected after the chromatographic fractionation (12). Therefore, to validate that the method used to convert flavins in riboflavin and the direct fluorescent detection of this compound were reliable, samples of the treated bread were first fractionated by HPLC and then the riboflavin was detected by fluorescence and quantified. Supplementary Table 3 depicts the levels of riboflavin detected by direct measurement of the fluorescence or after HPLC fractionation. After the chromatographic step, a fluorescent peak corresponding to riboflavin was observed in the samples of the breads fermented with the parental and mutant strains (result not shown), that was quantified. However, when the results obtained by the two methods were compared, we detected only similar levels for the breads fermented with BAL3C-5 B2, BAL3C-7 B2, or BAL3C-22 B2, the ratio of direct/HPLC levels being 0.81, 0.78, or 0.93, respectively (Supplementary Table 3). In the case of the breads produced with the parental strains BAL3C-5, BAL3C-7, and BAL3C-22, or spontaneously fermented without LAB inoculation, the ratios of the quantified riboflavin levels were 2.90, 1.5, 1.9, or 2.2, respectively. Therefore, these results validate the direct quantification method to determine concentration of flavins extracted from bread, and suggest that when the riboflavin production is low, it is more reliable than the HPLC method.
In recent years, riboflavin biofortification of fermented foods rather than vitamin supplementation has attracted great interest in the food industry, although as far as we know there are not yet any commercialized cereal products biofortified with vitamin B2 by LAB. In particular, L. plantarum and L. fermentum strains were successfully employed to obtain vitamin B2-enriched experimental bread (11, 14). Although in a previous study we reported on the selection of riboflavin-producing W. cibaria strains (32), this is the first work where robust vitamin-B2 overproducing derivatives belonging to this genera have been obtained. Indeed, apart from the widely reported applications of L. plantarum strains (57, 58), only few species of food-grade bacteria including L. lactis (9), L. mesenteroides, P. freudenreichii (10), L. fermentum (11) and Limosilactobacillus reuteri (59) have been reported for the vitamin B2 bio-enrichment of fermented foods endorsing the biotechnological importance of exploring the microbial biodiversity of LAB from different species and ecological niches.
Soluble and total dextran content in experimental breads
Supplementary Figure 5 depicts examples of representative chromatograms obtained from the GC-MS analysis of soluble dextran present in the wheat dough, and in breads obtained by fermentation with only the dough microbiota, or in presence of either BAL3C-22 B2 or BAL3C-22 (Supplementary Figures 5A,B). The peak corresponding to the isomaltose was detected in all breads analyzed (Supplementary Figures 5A–C), but not in the wheat dough (Supplementary Figure 5D). In addition, the dough contained maltose that was still present in all the breads. Moreover, production of lactic acid as well as high concentration of fructose (presumably generated by the catalysis performed by the Dsr) was only observed in breads fermented with the LAB strains (Supplementary Figures 5A,B).
Taking into account the quantification of the isomaltose detected by the GC-MS analysis, the results reported in Figure 6 revealed that the six strains tested produced similarly high levels of soluble (210–247 mg/100 g of bread) and total (280–310 mg/100 g of bread) dextran significantly higher than those present in the control sample (34 and 80 mg/100 g of bread, respectively, with p = 7.58 × 10–5 and p = 3.80 × 10–5) (Figures 6A,B, respectively), and with no differences between each couple of parental and mutant strains (Supplementary Figures 7A,B). Therefore, the overall results obtained here support the potential usage of the W. cibaria riboflavin-overproducing strains to generate bread enriched in riboflavin and dextran.
In this context, the use of W. confusa has been reported as a promising strategy for efficient in situ production of dextrans in sourdoughs without strong acidification resulting in bread with improved volume and crumb softness (25). Similarly, dextran synthesized in situ by W. confusa influenced the rheological, technological and nutritional properties of whole grain pearl millet bread, leading to increased free phenolic content and antioxidant activity, as well as lowered glycemic index and improved in vitro protein digestibility (60). In a recent study, a mixed fermentation with W. confusa and Propionibacterium freudenreichii has been proposed for in situ fortification of soya flour and rice bran in order to improve texture and vitamin B12 content of bread (61).
The use of dextran is not widely spread in the bakery field even though its impact on bread volume and texture was shown (62). Strains of Weissella cibaria used as starter cultures for wheat and sorghum sourdoughs synthesized EPS (0.08 to 0.8%) and enhanced the texture, nutritional value, shelf life, and machinability of wheat, rye, and gluten-free breads (28). The addition to the dough of 10% dextran-enriched sourdough as a starter, was sufficient to achieve significant increase in wheat bread volume (63). Also, the crumb hardness of sorghum, buckwheat, teff, and quinoa breads is reduced by dextrans produced in situ by strains of W. cibaria (29, 30). Dextran content in the experimental bread prepared using parental (BAL3C-5, BAL3C-7, and BAL3C-22) or riboflavin overproducing (BAL3C-5 B2, BAL3C-7 B2, and BAL3C-22 B2) strains was about 0.30%, which is within the concentration range of hydrocolloids commercially applied in bread making (22).
Conclusion and future perspectives
As far as we know this is the first report related to the isolation of W. cibaria riboflavin-overproducing mutants that are able to produce high levels of dextran. Moreover, the mutants were able to produce both riboflavin and dextran, in situ, during dough fermentation. Therefore, the W. cibaria BAL3C-5 B2, BAL3C-7 B2, and BAL3C-22 B2 strains seems to have the potential to be used for the production of functional food. As a further prospect, we will investigate whether sourdoughs fermented with the LAB mutants are able to enhance the texture, nutritional value and shelf life of sourdough-breads made at a pilot plant scale.
Data availability statement
The datasets presented in this study can be found in online repositories. The names of the repository/repositories and accession number(s) can be found below: https://www.ncbi.nlm.nih.gov/genbank/, ON677429; https://www.ncbi.nlm.nih.gov/genbank/, ON677430; https://www.ncbi.nlm.nih.gov/genbank/, ON677431; https://www.ncbi.nlm.nih.gov/genbank/, ON677432; https://www.ncbi.nlm.nih.gov/genbank/, ON677433; https://www.ncbi.nlm.nih.gov/genbank/, ON677434; https://www.ncbi.nlm.nih.gov/genbank/, ON420949; https://www.ncbi.nlm.nih.gov/genbank/, ON420950; https://www.ncbi.nlm.nih.gov/genbank/, ON420951; https://www.ncbi.nlm.nih.gov/genbank/, ON420952; https://www.ncbi.nlm.nih.gov/genbank/, ON420953; and https://www.ncbi.nlm.nih.gov/genbank/, ON420954.
Author contributions
MT and PL: conceptualization. AH-A, MM, PR, and RC: methodology. AH-A and JR-M: software. AH-A, JR-M, MM, PR, and RC: investigation. GSp and MM: data curation. AH-A, JR-M, MM, and PR: writing—original draft preparation. GSo, GSp, and PL: writing—review and editing. GSo, GSp, MT, and PL: supervision. GSo, PL, and MT: funding acquisition. All authors read and agreed to the published version of the manuscript.
Funding
This research was funded by the Spanish Ministry of Science, Innovation and Universities, (grant RTI2018-097114-B-I00) and the Regional Government of Junta de Castilla y León and the University of Salamanca (TCUE Plan 2021–2023, Proof of Concept grant PC_TCUE18-20P_029). Programa “Escalera de Excelencia” de la Junta de Castilla y León Ref.: CLU-2017-03 cofinanciación por el P.O. FEDER de Castilla y León 14–20. PR was the beneficiary of a grant by MIUR in the framework of “AIM: Attraction and International Mobility” (PON R&I2014-2020) (practice code D74I18000190001).
Acknowledgments
We thank Guillermo Padilla Alonso for his valuable assistance in the biostatistical analysis, to Leonor Rodríguez for her assistance in the performance and the interpretation of the GC-MS analysis and to Stephen Elson for the critical reading of the manuscript. We acknowledge support of the publication fee by the CSIC Open Access Publication Support Initiative through its Unit of Information Resources for Research (URICI). In addition, this work is also based upon the work from European COST Action 18101, SOURDOMICS—Sourdough biotechnology network toward novel, healthier, and sustainable food and bioprocesses, supported by COST, where PL, GSo, MM, PR, JR-M, and MT were members. COST is a funding agency for research and innovation networks.
Conflict of interest
The authors declare that the research was conducted in the absence of any commercial or financial relationships that could be construed as a potential conflict of interest.
Publisher’s note
All claims expressed in this article are solely those of the authors and do not necessarily represent those of their affiliated organizations, or those of the publisher, the editors and the reviewers. Any product that may be evaluated in this article, or claim that may be made by its manufacturer, is not guaranteed or endorsed by the publisher.
Supplementary material
The Supplementary Material for this article can be found online at: https://www.frontiersin.org/articles/10.3389/fnut.2022.978831/full#supplementary-material
Footnotes
References
1. Gu Q, Li P. Biosynthesis of vitamins by probiotic bacteria. In: Rao L, Rao LG editors. Probiotics and prebiotics in human nutrition and health. London: InTech (2016). p. 135–48. doi: 10.5772/63117
2. LeBlanc, JG, Laiño, JE, del Valle, MJ, de Giori, GS, Sesma, F, and Taranto, MP. “B-Group vitamins production by probiotic lactic acid bacteria”, in Biotechnology of lactic acid bacteria novel applications, eds F. Mozzi, R.R. Raya, and G.M. Vignolo (Oxford: John Wiley & Sons) (2015) 279–296. doi: 10.1002/9781118868386.ch17
3. Thakur K, Tomar SK, Singh AK, Mandal S, Arora S. Riboflavin and health: A review of recent human research. Crit Rev Food Sci Nutr. (2017) 57:3650–60. doi: 10.1080/10408398.2016.1145104
4. Turck D, Bresson JL, Burlingame B, Dean T, Fairweather-Tait S, Heinonen M, et al. Dietary reference values for riboflavin. EFSA J. (2017) 15:4919. doi: 10.2903/j.efsa.2017.4919
5. Sherwood RA. Methods for assessment of vitamin B2. In: Harrington D editor. Laboratory assessment of vitamin status. (Cambridge, MA: Academic Press) (2019). p. 165–72. doi: 10.1016/B978-0-12-813050-6.00007-3
6. Mazur-Bialy AI, Pochec E, Plytycz B. Immunomodulatory effect of riboflavin deficiency and enrichment – Reversible pathological response versus silencing of inflammatory activation. J Physiol Pharmacol. (2015) 66: 793–802.
7. Harvard TH. Chan school of public health’s department of nutrition. The nutrition source. Riboflavin – vitamin B2. (2022). Available online at: https://www.hsph.harvard.edu/nutritionsource/riboflavin-vitamin-b2/ (accessed June 10, 2022).
8. Titcomb TJ, Tanumihardjo SA. Global concerns with B vitamin statuses: Biofortification, fortification, hidden hunger, interactions, and toxicity. Compr Rev Food Sci Food Saf. (2019) 18:1968–84. doi: 10.1111/1541-4337.12491
9. Burgess C, O’Connell-Motherway M, Sybesma W, Hugenholtz J, Van Sinderen D. Riboflavin production in Lactococcus lactis: Potential for in situ production of vitamin-enriched foods. Appl Environ Microbiol. (2004) 70:5769–77. doi: 10.1128/AEM.70.10.5769-5777.2004
10. Burgess CM, Smid EJ, Rutten G, van Sinderen D. A general method for selection of riboflavin-overproducing food grade micro-organisms. Microb Cell Fact. (2006) 5:24. doi: 10.1186/1475-2859-5-24
11. Russo P, Capozzi V, Arena MP, Spadaccino G, Dueñas MT, López P, et al. Riboflavin-overproducing strains of Lactobacillus fermentum for riboflavin-enriched bread. Appl Microbiol Biotechnol. (2014) 98:3691–700. doi: 10.1007/s00253-013-5484-7
12. Mohedano ML, Hernández-Recio S, Yépez A, Requena T, Martínez-Cuesta MC, Peláez C, et al. Real-time detection of riboflavin production by Lactobacillus plantarum strains and tracking of their gastrointestinal survival and functionality in vitro and in vivo using mCherry labeling. Front Microbiol. (2019) 10:1748. doi: 10.3389/fmicb.2019.01748
13. Ewe JA, Wan-Abdullah WN, Liong MT. Viability and growth characteristics of Lactobacillus in soymilk supplemented with B-vitamins. Int J Food Sci Nutr. (2010) 61:87–107. doi: 10.3109/09637480903334163
14. Capozzi V, Russo P, Dueñas MT, López P, Spano G. Lactic acid bacteria producing B-group vitamins: A great potential for functional cereals products. Appl Microbiol Biotechnol. (2012) 96:1383–94. doi: 10.1007/s00253-012-4440-2
15. Thakur K, Tomar SK, De S. Lactic acid bacteria as a cell factory for riboflavin production. Microb Biotechnol. (2016) 9:441–51. doi: 10.1111/1751-7915.12335
16. Llamas-Arriba MG, Hernández-Alcántara AM, Yépez A, Aznar R, Dueñas MT, López P. Functional and nutritious beverages produced by lactic acid bacteria. Nutr Beverag. (2019) 12:419–65. doi: 10.1016/B978-0-12-816842-4.00012-5
17. Rollán GC, Gerez CL, Leblanc JG. Lactic fermentation as a strategy to improve the nutritional and functional values of pseudocereals. Front Nutr. (2019) 6:98. doi: 10.3389/fnut.2019.00098
18. Montemurro M, Coda R, Rizzello CG. Recent advances in the use of sourdough biotechnology in pasta making. Foods. (2019) 8:1–21. doi: 10.3390/foods8040129
19. Naessens M, Cerdobbel A, Soetaert W, Vandamme EJ. Leuconostoc dextransucrase and dextran: Production, properties and applications. J Chem Technol Biotechnol. (2005) 80:845–60. doi: 10.1002/jctb.1322
20. Xu Y, Cui Y, Yue F, Liu L, Shan Y, Liu B, et al. Exopolysaccharides produced by lactic acid bacteria and Bifidobacteria: Structures, physiochemical functions and applications in the food industry. Food Hydrocoll. (2019) 94:475–99. doi: 10.1016/j.foodhyd.2019.03.032
21. European Commission. Commission Decision of 30 January 2001 on authorising the placing on the market of a dextran preparation produced by Leuconostoc mesenterorides as a novel food ingredient in bakery products under Regulation (EC) No 258/97 of the European Parliament and of the Council. Decision 2001/122/EC. Off J Eur Communities. (2001) L 044:0046–0047.
22. Ramos L, Alonso-Hernando A, Martínez-Castro M, Morán-Pérez JA, Cabrero-Lobato P, Pascual-Maté A, et al. Sourdough biotechnology applied to gluten-free baked goods: Rescuing the tradition. Foods. (2021) 10:1498. doi: 10.3390/foods10071498
23. Goff D, Guo Q. “The role of hydrocolloids in the development of food structure,” in Handbook of food structure development, eds F. Spryropoulos, A. Lazidis, and I. Norton (London, UK: Royal Society of Chemistry) (2019) 1–28. doi: 10.1039/9781788016155
24. Damini K, Deeplina D, Seema P, Arun G. Dextran and food application. In: Ramawat K, Merillon J editors. Polysaccharides boactivity and botechnology. New York, NY: Springer International Publishing AG (2015). p. 735–52.
25. Wang Y, Sorvali P, Laitila A, Maina NH, Coda R, Katina K. Dextran produced in situ as a tool to improve the quality of wheat-faba bean composite bread. Food Hydrocoll. (2018) 84:396–405. doi: 10.1016/j.foodhyd.2018.05.042
26. Zhang Y, Guo L, Xu D, Li D, Yang N, Chen F, et al. Effects of dextran with different molecular weights on the quality of wheat sourdough breads. Food Chem. (2018) 256:373–9. doi: 10.1016/j.foodchem.2018.02.146
27. Chen XY, Levy C, Gänzle MG. Structure-function relationships of bacterial and enzymatically produced reuterans and dextran in sourdough bread baking application. Int J Food Microbiol. (2016) 239:95–102. doi: 10.1016/j.ijfoodmicro.2016.06.010
28. Lacaze G, Wick M, Cappelle S. Emerging fermentation technologies: Development of novel sourdoughs. Food Microbiol. (2007) 24:155–60. doi: 10.1016/j.fm.2006.07.015
29. Falasconi I, Fontana A, Patrone V, Rebecchi A, Garrido GD, Principato L, et al. Genome-assisted characterization of Lactobacillus fermentum, Weissella cibaria, and Weissella confusa strains isolated from sorghum as starters for sourdough fermentation. Microorganisms (2020) 8:1–16. doi: 10.3390/microorganisms8091388
30. Wolter A, Hager AS, Zannini E, Czerny M, Arendt EK. Influence of dextran-producing Weissella cibaria on baking properties and sensory profile of gluten-free and wheat breads. Int J Food Microbiol. (2014) 172:83–91. doi: 10.1016/j.ijfoodmicro.2013.11.015
31. Zarour K, Llamas MG, Prieto A, Rúas-Madiedo P, Dueñas MT, de Palencia PF, et al. Rheology and bioactivity of high molecular weight dextrans synthesised by lactic acid bacteria. Carbohydr Polym. (2017) 174:646–57. doi: 10.1016/j.carbpol.2017.06.113
32. Llamas-Arriba MG, Hernández-Alcántara AM, Mohedano ML, Chiva R, Celador-Lera L, Velázquez E, et al. Lactic acid bacteria isolated from fermented doughs in spain produce dextrans and riboflavin. Foods. (2021) 10:2004. doi: 10.3390/FOODS10092004
33. Altschul SF, Gish W, Miller W, Myers EW, Lipman DJ. Basic local alignment search tool. J Mol Biol. (1990) 215:403–10. doi: 10.1016/S0022-2836(05)80360-2
34. Sievers F, Wilm A, Dineen D, Gibson TJ, Karplus K, Li W, et al. Fast, scalable generation of high-quality protein multiple sequence alignments using Clustal Omega. Mol Syst Biol. (2011) 7:539. doi: 10.1038/MSB.2011.75
35. Saitou N, Nei M. The neighbor-joining method: A new method for reconstructing phylogenetic trees. Mol Biol Evol. (1987) 4:406–25. doi: 10.1093/OXFORDJOURNALS.MOLBEV.A040454
36. Tamura K, Stecher G, Kumar S. MEGA11: Molecular evolutionary genetics analysis version 11. Mol Biol Evol. (2021) 38:3022–7. doi: 10.1093/MOLBEV/MSAB120
37. Darty K, Denise A, Ponty YVARNA. Interactive drawing and editing of the RNA secondary structure. Bioinformatics. (2009) 25:1974–5. doi: 10.1093/bioinformatics/btp250
38. Teufel F, Almagro Armenteros JJ, Johansen AR, Gíslason MH, Pihl SI, Tsirigos KD, et al. SignalP 6.0 predicts all five types of signal peptides using protein language models. Nat Biotechnol. (2022) 40:1023–5. doi: 10.1038/s41587-021-01156-3
39. Miller AW, Robyt JF. Detection of dextransucrase and levansucrase on polyacrylamide gels by the periodic acid-Schiff stain: Staining artifacts and their prevention. Anal Biochem. (1986) 156:357–63. doi: 10.1016/0003-2697(86)90266-6
40. Besrour-Aouam N, Fhoula I, Hernández-Alcántara AM, Mohedano ML, Najjari A, Prieto A, et al. The role of dextran production in the metabolic context of Leuconostoc and Weissella Tunisian strains. Carbohydr Polym. (2021) 253:117254. doi: 10.1016/j.carbpol.2020.117254
41. Dubois M, Gilles KA, Hamilton JK, Rebers PA, Smith F. Colorimetric method for the determination of sugars and related substances. Anal Chem. (1956) 28:350–6. doi: 10.1021/ac60111a017
42. Jakobsen J. Optimisation of the determination of thiamin, 2-(1-hydroxyethyl)thiamin, and riboflavin in food samples by use of HPLC. Food Chem. (2008) 106:1209–17. doi: 10.1016/J.FOODCHEM.2007.06.008
43. Benjamini Y, Hochberg Y. Controlling the false discovery rate: A practical and powerful approach to multiple testing. J R Stat Soc Ser B. (1995) 57:289–300. doi: 10.1111/j.2517-6161.1995.tb02031.x
44. R Core Team. R: A language and environment for statistical computing. (2022). Available online at: https://www.r-project.org/
45. Ripa I, Ruiz-Masó JÁ, De Simone N, Russo P, Spano G, del Solar G. A single change in the aptamer of the Lactiplantibacillus plantarum rib operon riboswitch severely impairs its regulatory activity and leads to a vitamin B2- overproducing phenotype. Microb Biotechnol. (2022) 15:1253–69. doi: 10.1111/1751-7915.13919
46. Serganov A, Huang L, Patel DJ. Coenzyme recognition and gene regulation by a flavin mononucleotide riboswitch. Nature. (2009) 458:233–7. doi: 10.1038/nature07642
47. Fusco V, Quero GM, Cho GS, Kabisch J, Meske D, Neve H, et al. The genus Weissella: Taxonomy, ecology and biotechnological potential. Front Microbiol. (2015) 6:155. doi: 10.3389/FMICB.2015.00155/BIBTEX
48. Panthee S, Paudel A, Blom J, Hamamoto H, Sekimizu K. Complete genome sequence of Weissella hellenica 0916-4-2 and its comparative genomic analysis. Front Microbiol. (2019) 0:1619. doi: 10.3389/FMICB.2019.01619
49. Quirasco M, López-Munguía A, Remaud-Simeon M, Monsan P, Farrés A. Induction and transcription studies of the dextransucrase gene in Leuconostoc mesenteroides NRRL B-512F. Appl Environ Microbiol. (1999) 65:5504–9. doi: 10.1128/aem.65.12.5504-5509.1999
50. Besrour-Aouam N, Mohedano ML, Fhoula I, Zarour K, Najjari A, Aznar R, et al. Different modes of regulation of the expression of dextransucrase in Leuconostoc lactis AV1n and Lactobacillus sakei MN1. Front Microbiol. (2019) 10:959. doi: 10.3389/fmicb.2019.00959
51. Bounaix MS, Robert H, Gabriel V, Morel S, Remaud-Siméon M, Gabriel B, et al. Characterization of dextran-producing Weissella strains isolated from sourdoughs and evidence of constitutive dextransucrase expression. FEMS Microbiol Lett. (2010) 311:18–26. doi: 10.1111/j.1574-6968.2010.02067.x
52. Nácher-Vázquez M, Iturria I, Zarour K, Mohedano ML, Aznar R, Pardo MÁ, et al. Dextran production by Lactobacillus sakei MN1 coincides with reduced autoagglutination, biofilm formation and epithelial cell adhesion. Carbohydr Polym. (2017) 168:22–31. doi: 10.1016/j.carbpol.2017.03.024
53. Bailey RW, Clarke RTJ. A bacterial dextranase. Biochem J. (1959) 72:49–54. doi: 10.1042/bj0720049
54. Katina K, Maina NH, Juvonen R, Flander L, Johansson L, Virkki L, et al. In situ production and analysis of Weissella confusa dextran in wheat sourdough. Food Microbiol. (2009) 26:734–43. doi: 10.1016/j.fm.2009.07.008
55. Capozzi V, Menga V, Digesù AM, De Vita P, Van Sinderen D, Cattivelli L, et al. Biotechnological production of vitamin B2-enriched bread and pasta. J Agric Food Chem. (2011) 59:8013–20. doi: 10.1021/jf201519h
56. Škrovánková S, Sikorová P. Vitamin B2 (Riboflavin) content in cereal products. Acta Univ Agric Silvic Mendelianae Brun. (2010) 58:377–82. doi: 10.11118/actaun201058050377
57. Kim JY, Choi EJ, Lee JH, Yoo MS, Heo K, Shim JJ, et al. Probiotic potential of a novel vitamin B2-overproducing Lactobacillus plantarum strain, HY7715, isolated from Kimchi. Appl Sci. (2021) 11:5765. doi: 10.3390/app11135765
58. Ge Y-YY, Zhang J-RR, Corke H, Gan R-YY. Screening and spontaneous mutation of pickle-derived Lactobacillus plantarum with overproduction of eiboflavin, related Mechanism, and food application. Foods. (2020) 9:88. doi: 10.3390/foods9010088
59. Spacova I, Ahannach S, Breynaert A, Erreygers I, Wittouck S, Bron PA, et al. Spontaneous riboflavin-overproducing Limosilactobacillus reuteri for biofortification of fermented foods. Front Nutr. (2022) 9:916607. doi: 10.3389/fnut.2022.916607
60. Wang Y, Compaoré-Sérémé D, Sawadogo-Lingani H, Coda R, Katina K, Maina NH. Influence of dextran synthesized in situ on the rheological, technological and nutritional properties of whole grain pearl millet bread. Food Chem. (2019) 285:221–30. doi: 10.1016/j.foodchem.2019.01.126
61. Wang Y, Xie C, Pulkkinen M, Edelmann M, Chamlagain B, Coda R, et al. In situ production of vitamin B12 and dextran in soya flour and rice bran: A tool to improve flavour and texture of B12-fortified bread. LWT. (2022) 161:113407. doi: 10.1016/j.lwt.2022.113407
62. Galle S, Schwab C, Arendt E, Gänzle M. Exopolysaccharide-forming Weissella strains as starter cultures for sorghum and wheat sourdoughs. J Agric Food Chem. (2010) 58:5834–41. doi: 10.1021/jf1002683
63. Galle S, Schwab C, Dal Bello F, Coffey A, Gänzle MG, Arendt E. Comparison of the impact of dextran and reuteran on the quality of wheat sourdough bread. J Cereal Sci. (2012) 56:531–7. doi: 10.1016/j.jcs.2012.07.001
64. Felsenstein J. Confidence limits on phylogenies: An approach using the bootstrap. Evolution. (1985) 39:783–91.
Keywords: lactic acid bacteria, Weissella cibaria, dextran, exopolysaccharide, riboflavin, vitamin B2, functional food, functional bread
Citation: Hernández-Alcántara AM, Chiva R, Mohedano ML, Russo P, Ruiz-Masó JÁ, del Solar G, Spano G, Tamame M and López P (2022) Weissella cibaria riboflavin-overproducing and dextran-producing strains useful for the development of functional bread. Front. Nutr. 9:978831. doi: 10.3389/fnut.2022.978831
Received: 26 June 2022; Accepted: 05 September 2022;
Published: 04 October 2022.
Edited by:
Bhawani Chamlagain, University of Helsinki, FinlandReviewed by:
Ying Hu, Hubei University of Technology, ChinaKostyantyn Dmytruk, Institute for Cell Biology, Ukraine
Copyright © 2022 Hernández-Alcántara, Chiva, Mohedano, Russo, Ruiz-Masó, del Solar, Spano, Tamame and López. This is an open-access article distributed under the terms of the Creative Commons Attribution License (CC BY). The use, distribution or reproduction in other forums is permitted, provided the original author(s) and the copyright owner(s) are credited and that the original publication in this journal is cited, in accordance with accepted academic practice. No use, distribution or reproduction is permitted which does not comply with these terms.
*Correspondence: Paloma López, plg@cib.csic.es