Applications and Prospects of Microfluidic Chips in Orthopaedic Diseases
- 1Peking University Shenzhen Institute, Peking University, Shenzhen, China
- 2School of Materials Science and Engineering, South China University of Technology, Guangzhou, China
- 3National Engineering Research Center for Tissue Restoration and Reconstruction, South China University of Technology, Guangzhou, China
- 4Guangzhou Regenerative Medicine and Health Guangdong Laboratory, Guangzhou, China
With the advent of an ageing society, bone-related diseases such as osteoporosis have become a major human health issue, and osteolytic lesions caused by cancer bone metastasis have always had a poor prognosis. Researchers have studied the pathogenesis of these orthopaedic diseases to obtain efficacious treatments. Microfluidic chip technology is a popular technology developed in recent years. This technology can build an experimental platform for a bionic in vivo microenvironment in vitro, which has been favored by an increasing number of researchers. In this review, we will take osteoporosis and cancer bone metastasis as examples to illustrate the pathogenesis, introduce the latest progress in the research of orthopaedics-related diseases of the microfluidic model, and describe the current approaches and their limitations.
Introduction
Orthopaedics, as an important branch of modern clinical medicine, mainly studies the physiology and pathology of the skeletal-muscle system and maintains the normal function and morphology of this system with the help of drugs, surgery, or other physical means. With the progress of society and changes over time, the injury spectrum of orthopaedics has also changed significantly. For example, diseases such as polio, osteomyelitis, and bone and tuberculosis, which were common in the past, have been significantly reduced, and trauma caused by traffic accidents has increased significantly. The ageing of the population has also led to a corresponding increase in senile osteoporosis, rheumatoid arthritis, and bone tumours (Li and Zhang, 2018). This change indicates that the focus of orthopaedic research must also adapt and suggests the future development of orthopaedics. The future development of orthopaedics should not only strengthen the integration with basic medicine but also transfer the research results of other disciplines into their available technologies. For instance, the further improvement of artificial joints cannot be separated from the development of materials science and technology. The timely and effective application of new technological achievements in other disciplines to clinical orthopaedics can substantially improve the diagnosis and treatment of orthopaedic conditions.
In recent years, microfluidic chip technology has gradually gained popularity and has shown broad application prospects in biomedical research and other fields. Cells are the basic unit of life activity. In recent years, research on cells has evolved from the cellular level to the molecular level, and research on morphology has determined sub-microstructures and molecular structures to further understand the laws of life activity and development. The emergence of microfluidic chip technology has been regarded as a new important technology platform for cell research. A microfluidic chip is also called lab-on-a-chip, which refers to a new type of science and technology that integrates biological, chemical and other experiments on a chip of a few square centimetres, which can integrate sample preparation, reaction, separation, and detection into one. In cell culture, sorting, and lysing experiments, various basic operating units are integrated on a small chip to form a microchannel network, which is perfused with a controlled fluid, to achieve the various requirements of conventional chemical or biological laboratories (Lee et al., 2019).
Microfluidic chip technology used in cell research has the following advantages: 1) the chip size is small, which can usually be controlled within the range of 10–100 μm, while the mammalian cell diameter is approximately 10–20 μm, which is suitable for its growth; 2) the channel environment of the chip is relatively closed, and a multidimensional network structure can be constructed, which can form cell space characteristics close to the physiological state; 3) the microscale channel environment increases the rate of heat transfer, mass transfer, and gas exchange; 4) it can be used for high-throughput cell analysis and can acquire many biological signals at the same time; 5) multiple units on the chip can be flexibly combined, and integrated cell research is possible. Therefore, the process of cell injection, culture, screening, lysis, separation and detection can be integrated on the same chip. In addition to the controllable chip structure, there are many options for chip materials. Polydimethylsiloxane (PDMS), a high molecular weight organic silicon compound, is one of the most widely used materials in the preparation of microfluidic chips. Table 1 lists its advantages and limitations in microfluidic applications.
Combining biomedical clinical research with microfluidic chip technology can bring this technology to a new field and can also result in some breakthroughs in biomedical research. This article uses the most common condition, osteoporosis, and cancer cell metastasis in orthopaedics as examples and lists the important role played by microfluidic chip technology.
Establishment and Application of a Microfluidic Model of Osteoporosis
Osteoporosis is currently one of the most common orthopaedic metabolic diseases in the clinic. It is caused by a variety of causes of the decrease in bone mass per unit volume and the destruction of bone microstructure. The clinical features are mainly bone pain, increased bone fragility and easy fracture. Osteoporosis can be divided into three categories: primary, secondary and idiopathic. Primary osteoporosis accounts for approximately 90% and is divided into type I (postmenopausal osteoporosis, PMOP) and type II (senile osteoporosis, SOP). Secondary osteoporosis is induced by diseases such as diabetes, thyroid dysfunction, leukaemia, and malignant tumours or the application of drugs such as glucocorticoids, heparin, and immunosuppressants. Idiopathic osteoporosis is more common in adolescents aged 8–14, with a higher proportion of women than men, and usually involves a genetic medical history.
In the past 2 decades, people have also studied the pathological process of osteoporosis by various means. They showed that defects in bone formation during bone reconstruction are the main pathological mechanism of osteoporosis. Under the stress principle, bones are constantly renewed, osteoclasts remove old bone (bone resorption), and osteoblasts then add new bone (bone formation). When the osteoblast-mediated bone formation is not as effective as bone resorption by osteoclasts, bone mass loss occurs and bone mineral density (BMD) decreases, and osteoporosis occurs in severe cases (Bartelt et al., 2018). At the cellular level, the interaction and coupling of osteoblasts and osteoclasts constitute the smallest functional unit of bone, and some important molecules coordinate the activities between these two cells during bone reconstruction (Kim and Koh, 2019). Osteoclasts are monocyte-macrophage precursor branches derived from haematopoietic stem cells. Fully activated multinucleated osteoclasts are differentiated from osteoclast precursor cells. The differentiation process is regulated by macrophage colony-stimulating factor and receptor activator of nuclear factor-κB ligand (RANKL). Many different hormones and inflammatory factors regulate the physiology of osteoclasts through the RANKL pathway. Osteoblasts are differentiated from bone marrow mesenchymal stem cells. The rate and efficiency of precursor cell differentiation into mature osteoblasts and the lifetime of osteoblasts determine the rate of bone formation (Chen et al., 2018). At the molecular level, the activation of the classical Wnt/β catenin pathway is an important switch for osteoblast differentiation. Bone sclerostin and Dickkopf1-related protein 1 (Dkk1) inhibit this anabolic pathway by binding to and disrupting the Wnt receptor LRP-5. Osteoblasts release signal molecules such as sclerostin and Dkk1 to keep osteoblasts at rest. The periodic mechanical load can downregulate the expression levels of these inhibitory factors by bone cells, thereby activating the development of osteoblasts to form new bone. A mouse ulnar load study showed that in the higher strain regions of the ulna, Osteosclerosis protein production was significantly reduced, and local osteogenesis at these sites was also enhanced after 7 days (Robling et al., 2008). These regulatory mechanisms are very important and are the main goals of new drug development. For example, antibodies to sclerostin and Dkk1 can be used clinically to treat osteolytic lesions and osteoporosis.
Therefore, osteogenic differentiation and bone remodeling are the keys to the treatment of osteoporosis, but for various reasons, the in vivo research of osteoblasts still faces major challenges. It is difficult to maintain the original physiological functions of primary bone cells. Even in the case of 3D culture with the help of biological scaffolds, dynamic perfusion in the bioreactor and the application of mechanical loads, it is difficult for osteoblasts to form stable 3D network structures during in vitro culture. For example, Boukhechba et al. (2009) used biphasic calcium phosphate (BCP) particles to culture primary human osteoblasts. Compared with that in two-dimensional culture, the differentiation to an osteoblast-like phenotype was significant, osteoblast-specific markers were downregulated, and osteocytic markers were upregulated. However, random cell aggregates were generated in the BCP particle gap of 40–80 μm, and no ordered 3D cell network was generated. To simulate the bone remodeling environment in vitro, Mulcahy et al. (2011) used the biomimetic self-buried process of cells in Matrigel to successfully culture an osteocyte-like mouse cell line (MLO-Y4) into a 3D grid structure, but the cell spacing in this structure was approximately 100 μm, which cannot be controlled to a size of 20–30 μm in simulated mouse bone. Cell size is important for signal transmission during bone cell growth and mechanical conduction. Similarly, as the gel culture time increased, bone cells proliferated freely and eventually destroyed the original ordered cell network structure after 28 days.
The microfluidic 3D tissue model can better simulate the physiological and clinically relevant microenvironment. Lee et al. (2012) integrated a glass slide printed with a micro-pattern and a microfluidic device with eight culture chambers made of polydimethylsiloxane, and successfully cultivated 3D "primary bone tissue" in the microfluidic culture chamber. Further research is needed to determine whether osteoblasts may have differentiated into bone cells, and the strength of the tissue structure is not sufficient to cope with mechanical loads. As shown in Figure 1A, Gu et al. (2015) explored a new biomimetic method to replicate the 3D bone network structure. They assembled biphasic calcium phosphate microbeads with a size of 20–25 µm and murine early bone cells (MLO-A5) in a PDMS microfluidic chamber to simulate the physiological microenvironment of the 3D lacrimal duct structure. Bone cells could be cultured by long-term microfluidic perfusion, and finally, a dense and mechanically stable 3D network tissue structure was obtained. This method is useful for observing the mineral deposits of osteoblasts and has been widely used for the study of in vitro mechanical transduction of osteocytes. George et al. (2018) established a physiological lab-on-a-chip (LOC) to integrate the three main types of bone cells (osteocytes, osteoblasts, and osteoclasts) and eliminate space-time constraints. The platform facilitated mechanical loading and bone remodeling. To better observe the cells, Sheyn et al. (2019) prepared a PDMS microfluidic bone chip, which combined with optical imaging methods to monitor cell survival, proliferation and osteogenic differentiation in real-time. It proved that the osteogenic differentiation of cells is higher under constant and continuous medium flow conditions. This chip design can provide a platform for in vitro testing of cell responses to the environment and optical imaging of cells.
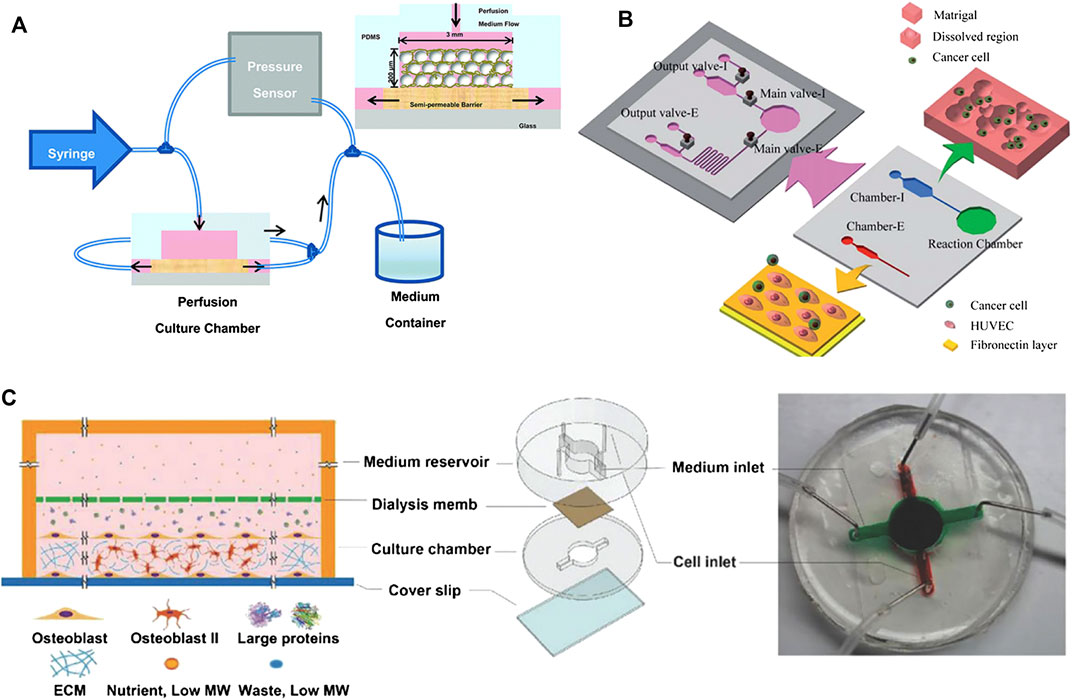
FIGURE 1. Examples of microfluidic bone tissue model. (A) A 3D bone network structure was cultivated in a microfluidic chamber through microbead-guided reconstruction. Reproduced with permission from Gu et al. (2015). Copyright 2015, Royal Society of Chemistry. (B) A microfluidic chip that measures cell invasion and extravasation simultaneously. Reproduced with permission from Shin et al. (2011). Copyright 2011, Royal Society of Chemistry. (C) A 3D mineralized collagen bone tissue chip for coculture of breast cancer cells and osteoblasts. Reproduced with permission from Hao et al. (2018). Copyright 2018, Wiley-VCH.
Furthermore, many 3D microsystems based on osteoblasts for drug screening have been developed. As one example, Nason et al. (2011) designed a microfluidic device for in vitro testing of cellular pharmacology as a tool to distinguish the concentration range that guarantees the effectiveness of the drug for antiosteoporosis therapy with strontium ranelate.
Exploration of the Mechanism of Bone Metastasis Through Microfluidics
Many cancers, including prostate cancer, breast cancer, multiple myeloma, and lung cancer, have a high probability of bone metastases in advanced stages, leading to a significant decrease of survival rate (Hao et al., 2018). These metastases can cause a range of bone-related diseases, such as spinal cord compression, pathological fractures, hypercalcemia and bone pain. Despite multiple treatment strategies, the metastatic bone disease has a poor prognosis (Wu et al., 2017). Therefore, it is necessary to construct a bone metastasis model of the bionic bone microenvironment to better understand malignant bone metastases and develop new effective bone metastasis treatment methods. In the past few decades, research involving three-dimensional cell culture has substantially reduced the gap between in vitro cell culture models and in vivo animal models (Chaicharoenaudomrung et al., 2019). In 1989, Stephen Paget elaborated the "seed and soil" hypothesis, comparing the metastasis of cancer cells to the spread of plant seeds. To facilitate the formation of metastatic foci, the "soil" (i.e., the meta-environment) must be able to nourish the "seed" (i.e., the spreading cancer cells) (Paget, 1989). When bone metastasis occurs, cancer cells extravasate and experience a continuous fluid microenvironment. Therefore, the fluid pressure experienced by cancer cells needs to be considered when modelling the metastatic microenvironment. Therefore, microfluidic systems with high throughput and lightweight characteristics have attracted the attention of many researchers. This system can achieve high analytical accuracy and sensitivity with very few reagents and can construct a 3D flow environment similar to the body in vitro.
Low-fluid conditions have balanced fluid pressure, which helps the fusion of the endothelial and functional microchannel lumen and will provide nutritional support for malignant tumours. Compared with those cultured on a 2D biological platform, cancer cells cultured in a microfluidic biological system formed spherical cell aggregates, the expression levels of epithelial markers (e.g., CD326) of these aggregates were downregulated, and the expression levels of interstitial markers (e.g., vimentin, N-cadherin, and fibronectin) were increased (Kuo et al., 2012). During cancer bone metastasis, osteoporotic cancer cells undergo transendothelial movement (infiltration and exudation) and directional migration. The speed of penetration across the endothelium is related to the active interaction between stromal cells and cancer cells, and the targeted migration of cancer cells depends on the chemical attraction of the homing site. To date, many researchers have used microfluidic systems to establish concentration gradients of inducing factors, simulate the shear stress stimulus applied to cancer cells by the endothelial network during transendothelial migration, and more realistically simulate the state of cancer cells in the circulatory system. Hydrodynamic models made with the help of microfluidic chips can assess changes in morphology, genetic material, and protein levels associated with tumour cell migration. To simulate the microenvironment closer to in vivo conditions, Huang et al. (2009) fabricated a parallel microfluidic channel separated by linear arrays of regularly spaced posts that can image real-time interactions between cells based on autocrine or paracrine signal molecules in a 3D ECM environment. Vickerman et al. (2008) developed a microfluidic platform for three-dimensional cell culture in bioderived or synthetic hydrogels, which can monitor cell dynamics in real-time. By regulating stimulating factors such as epidermal growth factor (EGF), CXCL12, and interstitial flow, Polacheck et al. (2011) successfully migrated cancer cells across a single layer of endothelial cells into a collagen gel in a microfluidic device, indicating that the cancer cell exudation process was well simulated. Besides, as shown in Figure 1B, Shin et al. (2011) designed a microfluidic chip with two main culture chambers. Chamber-I is an intravasation chamber for cell migration and the invasion assay, and chamber-E is an extravasation chamber for quantitative detection. The platform can simultaneously simulate the process of invasion and extravasation in cancer metastasis. This result shows that in the lumen of blood vessels, cancer cells can be detached from the matrix gel under strong stress and then adhere to the adhesion molecules in the extravascular cavity.
Microfluidic devices can mimic the skeletal environment and contribute to the dynamic bone metastasis process of cancer. Hsiao et al. (2009) seeded a three-culture cell sphere containing endothelial cells, osteoblasts and prostate cancer cells into a microfluidic biochip. The microfluidic chip can monitor the growth of cells in the sphere in real-time and can inhibit the proliferation of prostate cancer cells without compromising the viability of other cells. Jeon et al. (2015) developed a PDMS microfluidic 3D in vitro model through soft lithography technology, cocultured endothelial cells and bone mesenchymal stem cells (BMSCs) to establish a vascularized bone-like microenvironment that can be used to study the extravasation of breast cancer cells. Coincidentally, Bersini et al. (2014) designed a vascularized microfluidic device for endothelial cells and BMSCs, including three medium channels and four independent gel channels, and introduced breast cancer MDA-MB-231 cells to form a micrometastasis model of extravasated cancer cells. As shown in Figure 1C, Hao et al. (2018) designed a microfluidic bone chip, which consists of a top medium reservoir and a bottom cell growth chamber separated by a dialysis membrane. The bone chip can spontaneously grow into mature 3D mineralized collagen bone tissue with a thickness of 85 μm, which contains a large number of mineralized collagen fibers. The collagen fibers formed naturally within 720 h without the need for differentiation agents. They also maximized the chance of cancer cells interacting with the bone matrix and could be frequently observed. Because of miniaturization, simple manipulation and ultralow unit cost, this spontaneous 3D model will guide the study of cancer bone metastasis in vitro.
Conclusion and Future Perspectives
Microfluidic technology can mimic the dynamic microenvironment of bone cells in vivo by combining functionalized cells, extracellular matrix, cell growth factors, and other biochemical stimuli. This technology helps to assess cell-to-cell interactions, thereby elucidating the pathogenesis of bone-related diseases, such as osteoporosis and bone metastatic cancer, and can lead to the development of new treatment strategies.
However, this technology still has a long way to go before it can be widely used in the field of orthopaedics. On the one hand, the internal environment of the human body is complex and delicate, and current models cannot simulate the entire system. In particular, during bone metastasis, cancer cells undergo a continuous and closely related multistep process, including many inseparable cellular events (Qiao and Tang, 2018). On the other hand, many microfluidic disease models now use immortalized cell lines instead of patient-derived live cells. Using personalized cells instead of cell lines can help develop customized therapies that can guide patient treatment more effectively. In the past decade, induced pluripotent stem cells (iPSCs) have been extensively studied in regenerative medicine. They are considered as effective cell sources of engineered bone tissue and have strong bone regeneration potential in vitro studies (Rana et al., 2019). Observation and evaluation of iPSCs interaction with microfluidic technology is a new idea for future research, which has great potential in the study of the pathogenesis of bone-related diseases (such as osteoporosis and bone metastasis) and contributes to the development of new treatment strategies. In general, the use of microfluidic chip technology to generate bionic bone microenvironments has made encouraging progress, and researchers need to continue their efforts to better understand the mechanism of bone diseases, cure bone-related diseases, and improve the quality of life for humans.
Author Contributions
XS and QT conceived the idea. XY and QT wrote the draft. All authors contributed to the editing, discussion, and revision of the final version of the manuscript.
Funding
We would like to thank the Shenzhen Science and Technology Program (JCYJ20170815153105076, GJHZ20180411143347603), National Key Research and Development Program of China (2018YFA0703000), the Science and Technology Program of Guangdong Province (2019B010941002, 2017B090911008), the National Natural Science Foundation of China (32022041), Youth science and technology innovation talent of Guangdong TeZhi plan (2017TQ04R046), the National Nature Science Foundation of China (Grants U1801252), the Science and Technology Program of Guangzhou (201704020168, 201903010032, 201804020060, 202007020002).
Conflict of Interest
The authors declare that the research was conducted in the absence of any commercial or financial relationships that could be construed as a potential conflict of interest.
References
Bartelt, A., Behler-Janbeck, F., Beil, F. T., Koehne, T., Mueller, B., Schmidt, T., et al. (2018). Lrp1 in osteoblasts controls osteoclast activity and protects against osteoporosis by limiting PDGF-RANKL signaling. Bone Res 6, 1–10. doi:10.1038/s41413-017-0006-3
Bersini, S., Jeon, J. S., Dubini, G., Arrigoni, C., Chung, S., Charest, J. L., et al. (2014). A microfluidic 3D in vitro model for specificity of breast cancer metastasis to bone. Biomaterials 35, 2454–2461. doi:10.1016/j.biomaterials.2013.11.050
Boukhechba, F., Balaguer, T., Michiels, J. F., Ackermann, K., Quincey, D., Bouler, J. M., et al. (2009). Human primary osteocyte differentiation in a 3D culture system. J. Bone Miner. Res 24, 1927–1935. doi:10.1359/JBMR.090517
Chaicharoenaudomrung, N., Kunhorm, P., and Noisa, P. (2019). Three-dimensional cell culture systems as an in vitro platform for cancer and stem cell modeling. World J. Stem Cell 11, 1065. doi:10.4252/wjsc.v11.i12.1065
Chen, X., Zhi, X., Wang, J., and Su, J. (2018). RANKL signaling in bone marrow mesenchymal stem cells negatively regulates osteoblastic bone formation. Bone Res 6, 1–8. doi:10.1038/s41413-018-0035-6
George, E. L., Truesdell, S. L., York, S. L., and Saunders, M. M. (2018). Lab-on-a-chip platforms for quantification of multicellular interactions in bone remodeling. Exp. Cell Res 365, 106–118. doi:10.1016/j.yexcr.2018.02.027
Gu, Y., Zhang, W., Sun, Q., Hao, Y., Zilberberg, J., and Lee, W. Y. (2015). Microbead-guided reconstruction of the 3D osteocyte network during microfluidic perfusion culture. J. Mater. Chem. B 3, 3625–3633. doi:10.1039/c5tb00421g
Hao, S., Ha, L., Cheng, G., Wan, Y., Xia, Y., Sosnoski, D. M., et al. (2018). A Spontaneous 3D bone-on-a-chip for bone metastasis study of breast cancer cells. Small 14, 1702787. doi:10.1002/smll.201702787
Hsiao, A. Y., Torisawa, Y. S., Tung, Y. C., Sud, S., Taichman, R. S., Pienta, K. J., et al. (2009). Microfluidic system for formation of PC-3 prostate cancer co-culture spheroids. Biomaterials 30, 3020–3027. doi:10.1016/j.biomaterials.2009.02.047
Huang, C. P., Lu, J., Seon, H., Lee, A. P., Flanagan, L. A., Kim, H. Y., et al. (2009). Engineering microscale cellular niches for three-dimensional multicellular co-cultures. Lab Chip 9, 1740–1748. doi:10.1039/b818401a
Jeon, J. S., Bersini, S., Gilardi, M., Dubini, G., Charest, J. L., Moretti, M., et al. (2015). Human 3D vascularized organotypic microfluidic assays to study breast cancer cell extravasation. Proc. Natl. Acad. Sci. USA 112, 214–219. doi:10.1073/pnas.1417115112
Kim, B. J., and Koh, J. M. (2019). Coupling factors involved in preserving bone balance. Cell. Mol. Life Sci 76, 1243–1253. doi:10.1007/s00018-018-2981-y
Kuo, C. T., Chiang, C. L., Huang, R. Y. J., Lee, H., and Wo, A. M. (2012). Configurable 2D and 3D spheroid tissue cultures on bioengineered surfaces with acquisition of epithelial-mesenchymal transition characteristics. NPG Asia Mater 4, e27. doi:10.1038/am.2012.50
Lee, D. W., Choi, N., and Sung, J. H. (2019). A microfluidic chip with gravity-induced unidirectional flow for perfusion cell culture. Biotechnol. Prog 35, e2701. doi:10.1002/btpr.2701
Lee, J. H., Gu, Y., Wang, H., and Lee, W. Y. (2012). Microfluidic 3D bone tissue model for high-throughput evaluation of wound-healing and infection-preventing biomaterials. Biomaterials 33, 999–1006. doi:10.1016/j.biomaterials.2011.10.036
Li, J., and Zhang, Y. (2018). History of orthopaedics in China: a brief review. Int. Orthop 42, 713–717. doi:10.1007/s00264-018-3829-7
Mulcahy, L. E., Taylor, D., Lee, T. C., and Duffy, G. P. (2011). RANKL and OPG activity is regulated by injury size in networks of osteocyte-like cells. Bone 48, 182–188. doi:10.1016/j.bone.2010.09.014
Nason, F., Morganti, E., Collini, C., Ress, C., Bersini, S., Pennati, G., et al. (2011). Design of microfluidic devices for drug screening on in-vitro cells for osteoporosis therapies. Microelectron. Eng 88, 1801–1806. doi:10.1016/j.mee.2011.02.115
Paget, S. (1989). The distribution of secondary growths in cancer of the breast. Cancer Metast. Rev 8, 98–101.
Polacheck, W. J., Charest, J. L., and Kamm, R. D. (2011). Interstitial flow influences direction of tumor cell migration through competing mechanisms. Proc. Natl. Acad. Sci. U.S.A 108, 11115–11120. doi:10.1073/pnas.1103581108
Qiao, H., and Tang, T. (2018). Engineering 3D approaches to model the dynamic microenvironments of cancer bone metastasis. Bone Res 6, 1–12. doi:10.1038/s41413-018-0008-9
Rana, D., Kumar, S., Webster, T. J., and Ramalingam, M. (2019). Impact of induced pluripotent stem cells in bone repair and regeneration. Curr. Osteoporos. Rep 17, 226–234. doi:10.1007/s11914-019-00519-9
Robling, A. G., Niziolek, P. J., Baldridge, L. A., Condon, K. W., Allen, M. R., Alam, I., et al. (2008). Mechanical stimulation of bone in vivo reduces osteocyte expression of Sost/sclerostin. J. Biol. Chem 283, 5866–5875. doi:10.1074/jbc.M705092200
Sheyn, D., Cohn-Yakubovich, D., Ben-David, S., De Mel, S., Chan, V., Hinojosa, C., et al. (2019). Bone-chip system to monitor osteogenic differentiation using optical imaging. Microfluid. Nanofluid 23, 99. doi:10.1007/s10404-019-2261-7
Shin, M. K., Kim, S. K., and Jung, H. (2011). Integration of intra- and extravasation in one cell-based microfluidic chip for the study of cancer metastasis. Lab Chip 11, 3880–3887. doi:10.1039/c1lc20671k
Vickerman, V., Blundo, J., Chung, S., and Kamm, R. (2008). Design, fabrication and implementation of a novel multi-parameter control microfluidic platform for three-dimensional cell culture and real-time imaging. Lab Chip 8, 1468–1477. doi:10.1039/b802395f
Keywords: microfluidics, chip, bone microenvironment, osteoporosis, bone metastasis
Citation: Yang X, Tang Q, Lai C, Wu K and Shi X (2021) Applications and Prospects of Microfluidic Chips in Orthopaedic Diseases. Front. Mater. 7:610558. doi: 10.3389/fmats.2020.610558
Received: 26 September 2020; Accepted: 23 November 2020;
Published: 08 January 2021.
Edited by:
Milana C. Vasudev, University of Massachusetts Dartmouth, United StatesReviewed by:
Xin Zhao, Hong Kong Polytechnic University, Hong KongSasan Jalili-Firoozinezhad, Koch Institute for Integrative Cancer Research at MIT, United States
Copyright © 2021 Shi, Yang, Tang, Lai and Wu. This is an open-access article distributed under the terms of the Creative Commons Attribution License (CC BY). The use, distribution or reproduction in other forums is permitted, provided the original author(s) and the copyright owner(s) are credited and that the original publication in this journal is cited, in accordance with accepted academic practice. No use, distribution or reproduction is permitted which does not comply with these terms.
*Correspondence: Xuetao Shi, shxt@scut.edu.cn
†These authors have contributed equally to this work