Identifying fit-for purpose methods for monitoring fish communities
- 1Thünen Institute of Baltic Sea Fisheries, Rostock, Germany
- 2Institute of Marine Ecosystem and Fisheries Science (IFM), Center for Earth System Research and Sustainability (CEN), University of Hamburg, Hamburg, Germany
Scientific monitoring is a fundamental basis of scientific advice. Among others, monitoring aims at contributing towards understanding the influence of anthropogenic use (e.g. fisheries), the health of a stock and individuum and effectiveness of management and conservation measures (e.g. MPAs). Monitoring of demersal and benthic fish communities is often based on invasive methods like bottom trawling, however in some cases less invasive methods might be available. The need for developing alternative and less invasive monitoring methods is supported by an increasing number of Marine Protected Areas and Windfarms where traditional methods such as trawls cannot be deployed due to conservational or technical and safety reasons. To support the development of new monitoring concepts, we conducted a literature review to identify limits and opportunities of methods that are already available. Furthermore, we present a fit-for purpose guide that can help identifying the appropriate method for individual purposes. We defined eight different methods which were analyzed using four different criteria and listed their advantages and disadvantages. We further apply this guide to monitoring in Marine Protected Areas in the Baltic Sea as a case study, indicating that besides traditional bottom trawling, alternative and less invasive methods could be sufficient for specific research purposes. We therefore, encourage scientists and managers to consider alternative data collection methods to minimize environmental impact of scientific sampling. However, our results also indicate that most of the methods still need further refinement especially regarding sampling design, standardization of methods and comparability with established survey methods.
1 Introduction
Monitoring is often understood as a part or form of resource assessment, research or baseline surveys (Day, 2008). It can provide a context for marine science and has enabled the development of a science based understanding of marine ecosystems and human impacts affecting it (Bean et al., 2017). Monitoring hence serves a strategic purpose in the decision-making process and supports to establish and compare the baseline status of an ecosystem component in relation to a management target as well as to improve the relevance, efficiency and effectiveness of policy decisions (Bean et al., 2017). Further, it is an integral tool of marine environmental management and e.g. plays a fundamental part in sustainable fisheries and ecosystem based management (Trenkel et al., 2019) as well as impact assessment. Therefore, monitoring is not only question or target driven by the interests of scientists, it furthermore becomes mandatory by the legislation to review environmental directives. Beside the provision of information about ‘target species’ (dedicated monitoring) it can provide additional information about non-target species (non-dedicated monitoring), although the design for the latter is often not optimal. However, an example for a legislate monitoring is the monitoring within the framework of the Marine Strategy Framework Directive (MSFD), which was introduced to protect the marine ecosystem and biodiversity and to achieve good environmental status (MSFD, 2008/56/EC).
However, current monitoring approaches are strongly impacted by an increased interest in protecting and reserving nature, biodiversity and ecosystems, which will result in the establishment of more protected areas (EU, 2021), and by the increasing anthropogenic use of our seas. Besides recreational use, the seas e.g. become more important for the production of emission-free energy, which results in the expansion of offshore wind farms (OWFs) (Stelzenmüller et al., 2022). On the one side, monitoring becomes necessary in this context to i) review the achievements towards the goal of a good environmental status and the effectiveness of marine protected areas and ii) to understand the impacts of OWFs on the ecosystem. On the other side, especially in the context of monitoring in fisheries research the establishment of OWFs and MPAs can become challenging, since monitoring targeting demersal and benthic species is often conducted by scientific bottom trawling. While, scientific bottom trawl surveys are primary conducted to provide fishery-independent data to support fish stock assessment, scientific bottom trawling is more recently also used for multidisciplinary monitoring of ecosystems (Maureaud et al., 2021).
However, in the above-mentioned areas it is i) questionable whether the use of invasive monitoring methods such as bottom trawling are still in line with the conservation objectives (e.g. in MPAs with a benthic conservation target), and ii) will be prohibited in some parts (e.g. MPAs) or will be impossible due to technical and/or safety circumstances (e.g. in OWF). These potential `no fishing areas’ may become a blind spot for fisheries research if no other alternative monitoring method is available to collect necessary information (Haase et al., 2023). On the other hand, MPAs are conservation measures and may help in sustaining fisheries (Kriegl et al., 2021) and hence their efficacy needs to be monitored and understood, as well as the impacts of OWFs on the marine ecosystem and associated fish communities that are not yet fully understood (Lindeboom et al., 2011; Abramic et al., 2022). In consequence, there is a need for reconsidering fisheries monitoring and the consideration of alternative and less invasive monitoring approaches. Towards this goal, we here present a literature review that allowed us to design a fit-for-purpose guide to support the decision-making process in identifying ways for a less invasive monitoring in fisheries research and impact assessment. The developed guide can serve as a starting point for further investigations and analysis of additional methods not covered in this review. We further, demonstrate the application of the guide to the case of Baltic Sea MPAs because solutions are currently needed for MPAs where bottom-towed fishing will most likely be excluded in the near future.
2 Literature search
We conducted a literature review evaluating both traditional and modern methods used for monitoring fish stocks and communities. We distinguished traditional methods such as active and passive fishing gears from “modern” approaches such as hydroacoustics, underwater video footage and molecular methods. We conducted the literature search using the database Google Scholar (https://scholar.google.de/) applying terms related to monitoring and sampling of fish communities/assemblages in the marine environment (see Supplementary Table 1). Based on an initial selection of publications, further publications were added to the analysis applying a snowball system. We incorporated eight distinct methods in the analysis: trawling, passive fishing gear, stationary video, towed video, split-beam echosounder, acoustic camera, acoustic tagging and eDNA. All methods were analyzed according to four criteria: the sampling strategy used, the type of information provided, the target species and the target habitat along with an evaluation of their respective advantages and disadvantages (see Tables 1, 2). In total we initially skimmed 179 articles for information about different monitoring methods of which 86 were considered relevant for this review. An article was considered relevant if it provided a review of different methods or one method, mainly focused on the marine environment and/or contained comparisons of different methods or addressed the context of Marine protected areas and fisheries closures.
3 Traditional methods
3.1 Active gears
Active fishing gear includes among others pelagic- or bottom trawls, seine nets and dredges. We focus here on bottom towed gear, especially bottom trawling since we mainly target monitoring of benthic and demersal fish communities. Bottom towed gears include beam trawls, otter trawls and dredges but also other more specific gears. The tradition of scientific bottom trawling dates back to the 1900s and is still probably the most commonly used method for monitoring demersal fish species on continental shelfs and slopes (Garces et al., 2006; Trenkel et al., 2019; Maureaud et al., 2021). Considering only standardized surveys with otter trawls, there are about 95 surveys investigating the demersal marine fauna of continental shelves and slopes worldwide (Maureaud et al., 2021). In Europe, multiple surveys are conducted with bottom trawls, for example the ICES (International Council for the Exploration of the Sea) coordinated Beam Trawl Survey (BTS), the International Bottom Trawl Survey (IBTS) or the Baltic International Trawl Survey (BITS) (for more details regarding the surveys see ICES.dk). Many of these surveys have been performed continuously over years and produce long time series and therefore provide a unique opportunity to track species range shifts and improve the assessment of biodiversity under global change (Maureaud et al., 2021). Standard large-scale scientific bottom trawl surveys support both fish stock assessments and the identification of spatio-temporal changes of fish species and communities in the shelf areas worldwide (Trenkel et al., 2019). Regular bottom trawl surveys mainly vary with respect to the width, height and mesh sizes of the gears used depending on the target species and driven by the scientific objective.
The main advantages of scientific bottom trawling are that due to their extractive nature they are more precise and simpler in identifying species compared to some non-extractive methods. Furthermore, the extrapolation to abundance per area is easier because the area sampled is approximately known by towing time and speed as well as the dimension of the fishing gear. Another advantage is the wide variety of important and necessary information that is provided by individual fish like weight, length, sex, maturity, age and other condition parameters that are essential for fisheries management. The major disadvantage of bottom trawling are potential unwanted effects on the ecosystem especially on habitats and organisms associated with the seafloor (Johnson et al., 2015; Oberle et al., 2016). Even though the impact of scientific bottom trawling is negligible compared to commercial fishing with bottom-towed fishing gears, it might still be desirable to minimize these potential impacts (Trenkel et al., 2019), especially in ecosystems with very sensitive benthic components and a risk of bycatch of e.g. threatened species. Moreover, large-scale fisheries independent monitoring programmes are time-consuming and involve expensive surveys on research vessels (Biber, 2011; Maureaud et al., 2021). Another drawback is that trawls also have a certain selectivity (e.g. mesh size, towing speed, ground gear) and can be more sensitive towards smaller and slow-moving fish (Côté and Perrow, 2006; Murphy and Jenkins, 2010). Furthermore, bottom trawling is not possible everywhere. Many habitats, especially highly structured habitats, pose too high risk for trawling, as nets could be damaged or even lost. A relatively recent problem arises from the exclusion of bottom trawling in additional areas, such as wind parks and MPAs. This can lead to a non-representative survey of the respective target species and impact assessments are not possible with this traditional fishing methods due to conservational (in MPAs) or technical and safety (bottom constructions) reasons.
3.2 Passive gears
Passive gears for monitoring fish stocks such as traps, gillnets as well as hook and line rely on an active encounter by the fish (Côté and Perrow, 2006). As a consequence, passive gears can only provide relative abundance data and surveys require high level of standardization. Similar to bottom trawling, passive gears are extractive methods, which allows for more precise identification of fish species compared to other, non-extractive methods. Fish traps are commonly applied to determine the abundance of a number of fish and invertebrate species (Recksiek et al., 1991; Jones et al., 2003; Wells et al., 2008; Rudershausen et al., 2010). In the south-eastern United States, for example, the assessment and management of black sea bass (Centropristis striata) relies partly on fishery-independent trap data (SEFIS-Survey) (Southeast Data Assessment and Review, 2011; Bacheler et al., 2013b). Trapping is considered to catch most species largely independent of environmental conditions. Traps can be further distinguished into pot gears, fyke nets and trapping barriers (Côté and Perrow, 2006). Traps can also be used for estimating fish densities and movement patterns (Murphy and Jenkins, 2010) and usually provide reliable CPUE data if design and size are standardized (Côté and Perrow, 2006). They are applicable in various habitats and depths (Thrush et al., 2002; Harvey et al., 2012; Bacheler et al., 2013b; Wakefield et al., 2013; Beentjes, 2019) and are therefore, useful for the detection of fish inhabiting structural complex habitats (Wells et al., 2008). Traps can also be placed along transects at a set distance (McClanahan and Mangi, 2000; Kaunda-Arara and Rose, 2004) and can fish unattended (Bacheler et al., 2013b). A great advantage of trap fishing is that fish remain in their natural habitat and can often be released alive (Miller, 1990), if information on age, maturity and sex is not required. However, a determination of the sampled area is often impossible and the influence of environmental parameters (e.g. currents) and bait plume on gear performance are hardly quantifiable (Stoner, 2004).
Hook- and line fishing is a kind of remote surface sampling and used to offset the behavior of fish to divers (Willis et al., 2000) and to catch large predatory fish that occur in low densities (Côté and Perrow, 2006). Hooks are generally baited appropriately for the target species (Murphy and Jenkins, 2010), making hook- and line fishing highly selective and therefore unsuitable for monitoring entire fish communities (Côté and Perrow, 2006). However, the method can generate CPUE statistics and has been used e.g. to produce area estimates of relative densities of snapper and blue cod (Willis et al., 2000; Côté and Perrow, 2006). Hook- and line fishing is furthermore relatively cheap but may present a significant risk to marine birds or other groups (Côté and Perrow, 2006). Additionally, a major drawback of hook-and line fishing is the significant damage and stress to which the fish are exposed which often results in the mortality of the individuals (Côté and Perrow, 2006; Murphy and Jenkins, 2010).
Gillnets are mostly used to catch mobile fish species and are considered to be the most selective nets (Côté and Perrow, 2006). Mesh size can be adjusted to the target species, so that non-target species bycatch can be reduced effectively. In the case of multi-species surveys, different mesh sizes can be deployed across the net or less selective nets like trammel nets can be used. Gillnets are relatively cheap and long lasting and catches can be reliably expressed as CPUE (Côté and Perrow, 2006). However, estimating the sampled area is not possible and removing caught fish and other organic or inorganic material out of nets can be time consuming. Furthermore, gillnets pose a risk of catching marine mammals and birds in some areas (Côté and Perrow, 2006). Additionally, gillnets can cause high mortality of the fish caught, especially if nets are set over a long period of time (Côté and Perrow, 2006).
4 Modern methods
4.1 Hydroacoustics
Hydroacoustic methods are based on the sound transmission properties in water (Tessier et al., 2016). Thereby, sound is generated by the conversion of a pulse of electricity produced by a transmitter. The sound then travels through the water column until it encounters an object and the echo is returned to the boat, where it is picked up by a transducer (Côté and Perrow, 2006). By that, echoes can provide information on the size and number of fishes and their distribution (Côté and Perrow, 2006; Johnston et al., 2006; Boswell et al., 2007). The areas of application are diverse and range from characterizing habitats to surveying the spatial and temporal movements of large mobile fish species (Murphy and Jenkins, 2010). Depending on the objective target different methodologies can be applied. In terms of detection of fish the common methodology is a split-beam echosounder, but also acoustic cameras, acoustic tagging and passive acoustics can be used to monitor fish assemblages (Murphy and Jenkins, 2010). Passive acoustics differs from other methods since the sound is not actively generated by a transmitter. Instead, underwater sounds are recorded using underwater microphones. In this way, ambient sounds, like sound coming from animals can be recorded. In the following, we focus on split-beam echosounder, acoustic cameras and acoustic tagging and will not discuss passive acoustics.
4.1.1 Split-beam echosounder
Split-beam echosounders are probably the most common method of hydroacoustic monitoring of fisheries resources and are used in a variety of traditional surveys. They are commonly used in monitoring pelagic fish stocks, like the Baltic International Acoustic Survey (BIAS) or the Baltic Acoustic Spring Survey (BASS). Other application areas include deep waters off the continental shelf (Johnston et al., 2006), shallow estuaries (Boswell et al., 2007) and mangroves (Krumme and Saint-Paul, 2003; Murphy and Jenkins, 2010). They commonly use horizontal and vertical beams to record the movement of fish and post-processing echo integration software to measure recorded biomasses. Advantages of this method are the ability of estimating absolute population estimates, the ability to map large areas quickly in a non-destructive way (Johnston et al., 2006) and immediately retrieve results. However, the exact taxonomic identification and individual fish length has to be proved by ground-truthing, which requires additional fishing hauls. Also split-beam echosounders are unable to detect species closer than 2m off the seafloor (Johnston et al., 2006), and therefore, are not appropriate for most demersal fish species.
4.1.2 Acoustic camera
The dual frequency identification sonar (DIDSON) produces near video-quality images of fishes by using sound (Holmes et al., 2006; Murphy and Jenkins, 2010). DIDSON can detect fish at ranges up to 15m from the camera at high frequency setting and 40 m distance at low frequency settings (Holmes et al., 2006; Murphy and Jenkins, 2010). Types of data include abundance estimates, length data and fish behavior (Moursund et al., 2003; Rose et al., 2005). The acoustic camera can be used in turbid and low light waters, and thereby, provide a great alternative to underwater visual census (UVC) or underwater video. It has already been proven to be an effective tool for fisheries stock assessments (Moursund et al., 2003). However, the application of DIDSON is limited to small-scale studies, and habitat structure can block the beams (Holmes et al., 2006; Murphy and Jenkins, 2010). Further, it only provides low taxonomic resolution, is expensive, and image analysis is relatively time-consuming (Holmes et al., 2006; Murphy and Jenkins, 2010).
4.1.3 Acoustic tagging
Acoustic tagging, besides other form of tagging such as radio frequency (RFID) tagging or satellite tagging is mostly used for studies on larger, mobile species and in association with MPA monitoring. Areas of research include homing behavior, movement and activity patterns, migration and use of space (Lowe et al., 2003; Egli and Babcock, 2004; Meyer et al., 2007). The type of acoustic tag used depends on the exact research objective. For short-term but fine scale movement data, active tracking of the acoustically marked animal is carried out with the help of directional hydrophones and acoustic receivers (Zeller, 1998; Afonso et al., 2008; Murphy and Jenkins, 2010). Residency and movement pattern data can be provided by a passive monitoring system using radio acoustic positioning telemetry (RAPT) buoy systems. Acoustically tagged fish within the RAPT system range are detected by acoustic receivers on buoys which communicate with a base station by radio signals or cable (Jorgensen et al., 2006). Long-term and large-scale studies use independent acoustic receivers to record movement patterns of acoustically tagged fish (Starr et al., 2005; Murphy and Jenkins, 2010). Thereby, acoustic receivers record time, date and identity of the tagged fish. Acoustic tagging techniques provide a great method to investigate spatial and temporal movement patterns of various species without recapture, which is required for standard tagging. However, due to high costs associated with tags, sample sizes are usually small, reducing statistical power (Zeller, 1999; Jorgensen et al., 2006). Additionally, the lifespan of tags is limited. Furthermore, fish need to have reached a certain size in order to be tagged. Further, fish must be extracted prior to tagging using conventional methods and therefore cannot be considered isolated from other methods. Tagging, consequently, always involves the use of any of the passive or active fishing methods, which is the focus of our manuscript, which needs to be considered when selecting a tagging method.
4.2 Underwater video
The development of underwater video technology can be traced back to the middle of the 20th century (Barnes, 1952). Technological developments since then have led to an enormous improvement in these methods and thus also to an increasing use of camera techniques in marine research (Mallet and Pelletier, 2014). The fields of application, as well as the technical details of the different methods vary greatly and offer a large pool of possibilities for non-destructive sampling methods. Video methods in general offer several advantages but also disadvantages compared to conventional methods like trawling. Video methods are by their design non-invasive and non-extractive and therefore have very little impact on the marine environment and can be applied in almost every habitat. They generate large datasets with extensive spatial coverage over a wide depth range (Letessier et al., 2015). In addition, video techniques can be applied in habitats where the use of other methods is not possible or prohibited. Thus, video techniques are more suitable for highly structured habitats (Starr et al., 2016) and possibly provide an option for use in offshore wind farms (OWFs) or wave farms (Sheehan et al., 2010). Video techniques require less scientific staff and can also be used from smaller vessels (Sheehan et al., 2010). Further, species selectivity is considered lower for video techniques (Harvey et al., 2012; Bacheler et al., 2013a; Christiansen et al., 2020), and the probability of detecting rare species is considered to be higher (Goetze et al., 2019; Langlois et al., 2020). However, systematic selectivity studies for video-techniques are still seldom as well as studies focusing on effective sampling design. Furthermore, analysis of video-material can be rather time-consuming, which might be the biggest challenge for future research. Another major limitation is the dependency of video-recording on relatively good visibility in the water.
4.2.1 Stationary video
A stationary unit can be anything, and hardware configuration can range from small tripods to large and heavy metal slides. They are categorized as remote underwater video (RUV) and baited remote underwater video (BRUV). Within these categories, there are wide variations in hardware and technical details, most of which are applicable to both categories (Table 3). First of all, a distinction can be made between the camera orientation, which can be either horizontally (H-RUV, H-BRUV) or vertically (V-RUV, V-BRUV) aligned (Fedra and Machan, 1979; Ellis and DeMartini, 1995; Priede and Merrett, 1996; Willis and Babcock, 2000; Willis et al., 2000; Jan et al., 2007). A horizontal orientation of the cameras is the most common, providing a greater field of view and reducing the risk of the system affecting behavior. Vertical alignment, on the other hand, offers the advantage of a simple calculation of the field of view as well as the possibility to easily perform length measurements. However, to our knowledge no studies focusing on fish using V-RUV have been conducted. Some studies also focus on a 360° view, which can be achieved either by using multiple cameras in an array, 360° cameras or rotating systems (Wells et al., 2008; Aguzzi et al., 2011; Pelletier et al., 2012; Schobernd et al., 2014; Starr et al., 2016; Mallet et al., 2021; Pelletier et al., 2021a; Pelletier et al., 2021b). Many of these techniques can be used not only with a single camera but also with a stereo camera, allowing more accurate length and field of view calculations (Harvey et al., 2002). This variety of technical setups can be applied to both RUVS and BRUVS. The precision of the monitoring differs from individual setups and purposes of the programme. Generally, studies using stationary systems assess species richness, relative abundance and length. Most studies use the relative abundance measure of MaxN (Priede and Merrett, 1996), which describes the maximum number of individuals of one species in a single frame. Video based methods, especially BRUV-systems have become very popular (De Vos et al., 2014; Whitmarsh et al., 2017). Besides the general advantages video-based methods offer, stationary systems have the advantage over towed video systems that fish are not scared by noise or movement of the system. They also have the potential to be used by non-scientific staff (Mallet et al., 2014) offering the integration into citizen science programs. Additionally, they can be deployed over long time periods offering the possibility to observe different activity patterns influenced by diurnal variation. Since stationary systems similar to passive gears are dependent on the activity of the fish, calculation of total abundances is not possible, observation duration is rather long and zero counts are common. Though the use of bait can attract a larger number of animals, it can introduce bias towards predatory and scavenger fish whose presence may deter other species. Further, the effect of bait and bait plume remains rather unknown (Mallet and Pelletier 2014). In addition to the general disadvantages of video-based systems, the above-mentioned limitations and the typically poor visibility in temperate waters result in a seldom use of stationary systems in temperate regions.
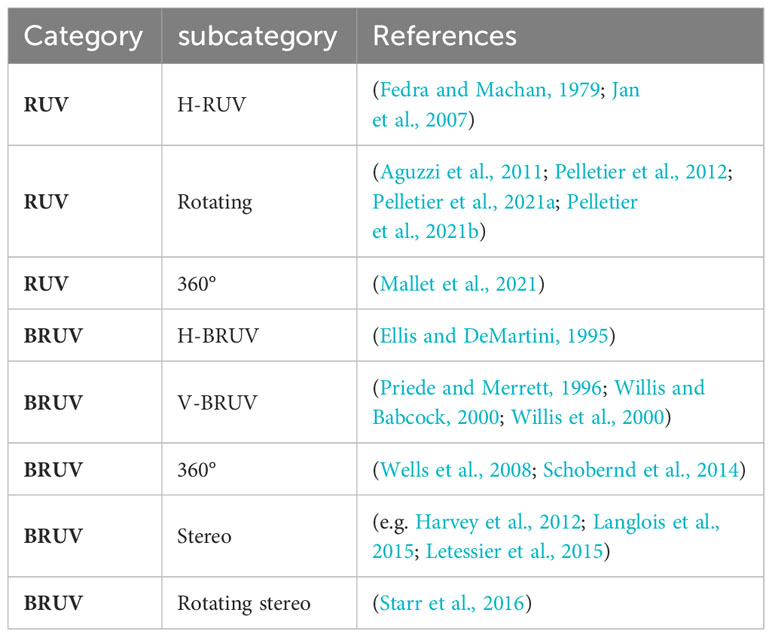
Table 3 Overview of different stationary video observation categories, subcategories and references.
4.2.2 Towed video
Towed camera systems can be either sledges that lightly touch the bottom or towed bodies that hover just above the seabed. Most of the above-named technical details for stationary systems (section 4.2.1) are simultaneously applicable to towed systems. They can be either oriented vertically or horizontally, or use a combination of both (Trobbiani et al., 2018). Towed systems can also be equipped with either a single or a stereo camera, but to our best knowledge there has not been a device using 360° cameras. Towed systems are used for the detection of benthic invertebrates, habitat classification and/or detection of fish and are e.g. used in standardized surveys for anglerfish (Lophius spp.) (McIntyre et al., 2013). They can be used in various depths and are often not limited by habitat structure. Comparisons of beam trawls, diver transects and towed video for estimating the abundance of juvenile flatfishes have shown equal performance of the different methods (Spencer et al., 2005), making towed video an adequate non-destructive sampling alternative, which is inexpensive and simple to operate (Spencer et al., 2005; McIntyre et al., 2013; McIntyre et al., 2015). These systems are unrestricted to tow duration and efficient to record large areas of the seafloor quickly (Sheehan et al., 2010; McIntyre et al., 2015). However, a main disadvantage of towed systems may be due to the stability of the device, as towed camera systems usually have difficulties maintaining a constant height above the bottom (McIntyre et al., 2015). Consequently, difficulties arise in determining the exact transect size and complex evaluations of inclination and distance from the device are required. Other disadvantages and limitations might be the influence of environmental conditions on towing speed or visibility (McIntyre et al., 2013) or bias caused by fish behavioral responses to the towed video system (Stoner et al., 2008).
4.3 Molecular methods
Environmental DNA (eDNA) is a relatively new and promising non-invasive tool of molecular methods for marine environmental monitoring (Hansen et al., 2018; Garlapati et al., 2019; Salter et al., 2019; Maureaud et al., 2021). It is based on the principle that DNA is continuously released from organisms in the environment (Hansen et al., 2018). It describes the process of collecting DNA from different environmental samples (such as water, sediment, etc.) with the objective of obtaining information about biodiversity (Garlapati et al., 2019). Commonly water samples are first filtrated, followed by the extraction and preservation of DNA. eDNA is then extracted from the filter, sequenced, detected and then analyzed (see Garlapati et al., 2019). eDNA analysis can be based on two different approaches, depending on the aim of the study. The species-specific approach is based on eDNA barcoding, while the multispecies approach is based on eDNA metabarcoding (Hansen et al., 2018; Garlapati et al., 2019). eDNA offers a cost-efficient, quick and sensitive method (Hansen et al., 2018; Garlapati et al., 2019) to detect e.g. invasive species (Gold et al., 2021). Thereby, it can potentially detect the presence of fish in bottom waters and is regarded to be especially promising for studying rare marine species, which are hard to detect by traditional methods (Garlapati et al., 2019; Salter et al., 2019; Russo et al., 2020; Afzali et al., 2021; Maureaud et al., 2021). For instance, studies in the West Antarctic Peninsula revealed signatures of benthic invertebrates, endemic fishes and king crabs (Cowart et al., 2018). Also studies in the Baltic Sea proved eDNA to be a successful tool in detecting fish communities (Thomsen et al., 2012; Sigsgaard et al., 2017). And species-specific analysis indicate that eDNA concentrations correlate with biomass and abundance (Takahara et al., 2012; Maruyama et al., 2014; Doi et al., 2015; Hansen et al., 2018).
However, abundance estimation is usually problematic, as concentrations of eDNA are influenced by many factors. First, organismal production rates, release of DNA, can differ between species, size and metabolic rates. Therefore, studies need to investigate the influence of environmental factors on metabolic rate and release of particles for different species and sizes. Second, degradation rates of eDNA particles can vary between ecosystems and areas most likely related to other environmental processes. Studies on the influence of abiotic and biotic factors on degradation have shown that temperature, solar radiation and pH-concentration cause large variations in the persistence of eDNA particles (Strickler et al., 2015; Hansen et al., 2018). Third, the physical transport in order to determine the exact origin of the eDNA is largely unknown, which is another challenging issue in terms of eDNA analysis (Hansen et al., 2018). Currently fish is the most studied group with the eDNA approach and there is already good concordance between eDNA surveys and traditional survey methods in terms of species detection (Maruyama et al., 2014; O’Donnell et al., 2017; Hansen et al., 2018; Salter et al., 2019). However, eDNA results are still seldomly used in ecosystem management, as the effective use of eDNA tools would require further addressing of the above mentioned uncertainties that exist in data elucidation (Garlapati et al., 2019). A major problem arises with the false positives and false negatives and the reasons for those have to be investigated further (Garlapati et al., 2019).
5 Fit-for purpose monitoring – how to choose
Monitoring is a complex issue and several factors need to be considered when deciding on the appropriate monitoring concept. A monitoring concept should be designed based on the questions to be answered and data requirements, on habitat characteristics, target species and resource availability (Henseler and Oesterwind, 2023) as well as conservation management decisions and technical restrictions. This also means that in cases where extractive methods are not absolutely necessary to answer the specific question (e.g. documentation of abundance & biodiversity), the use of alternative less invasive methods can be considered. Based on this review we identified criteria, which we suggest are fundamental in order to choose the appropriate method for monitoring (Figure 1). It should be noted here, that the respective classification of methods is referring to various criteria and is not always appropriate for all subcategories of the individual methods. In this context, it is important to emphasize that none of the described methods can provide true species richness or abundance/biomass information (Hansen et al., 2018). This is due to the specific selectivity and catchability of the individual method, which is why it is crucial to estimate selectivity patterns for each type of method within the development of monitoring programs (Christiansen et al., 2020). Additionally, combining different methods can be effective in reducing method specific bias and can increase the range and detail of data from spatial surveys (Willis et al., 2000; Watson et al., 2005; Murphy and Jenkins, 2010). Another issue that should be considered is the required workload. Despite certain methods might be less expensive and are less labor intensive in the field, post-fieldwork workload can increase compared to traditional methods. This applies in particular to hydroacoustic and video-based methods, as post-processing of data can be very time-consuming. However, with the rapid development of artificial intelligence-based methods, the workload for the processing of these data might soon be reduced.
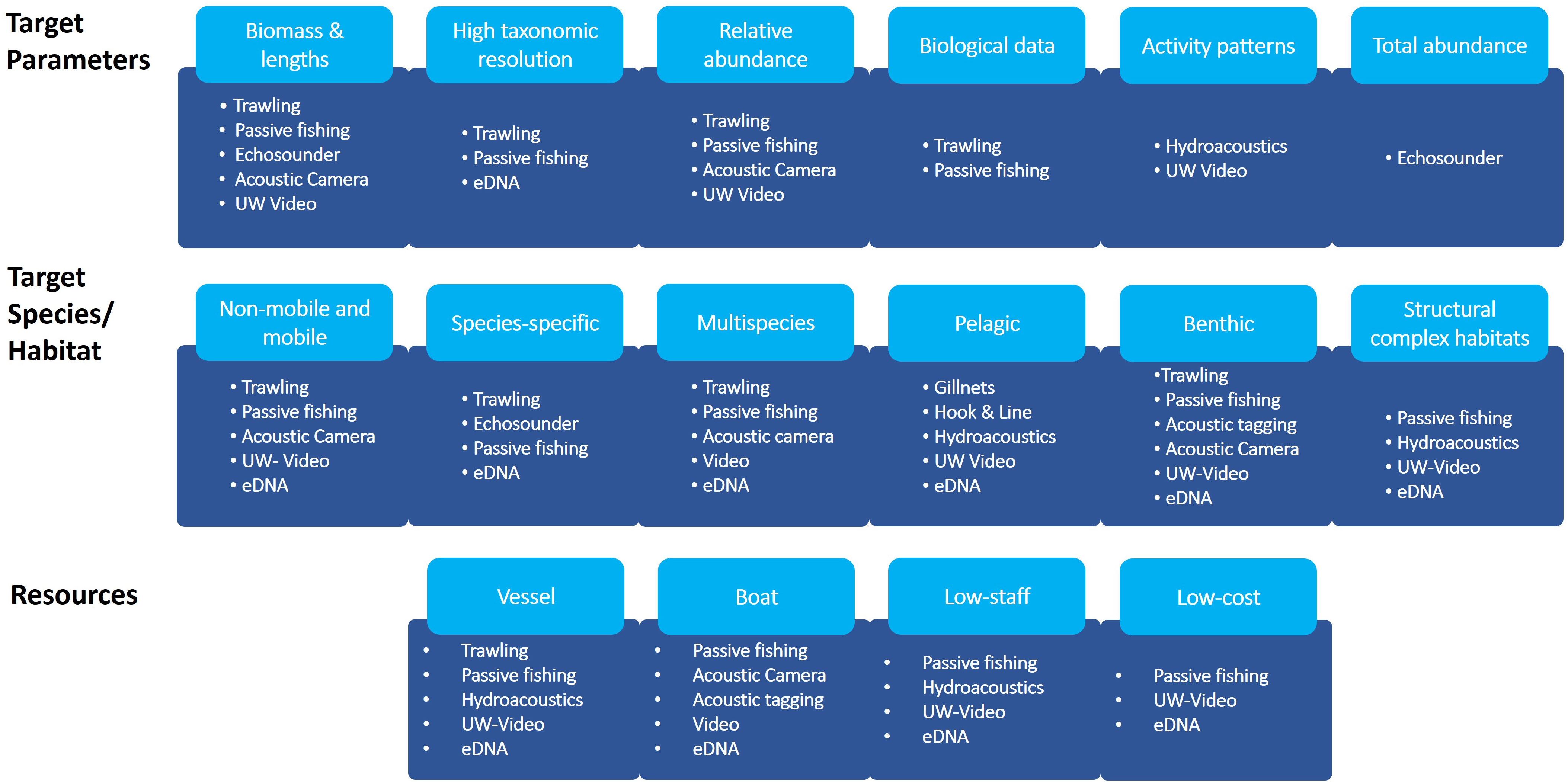
Figure 1 Different target criteria and attribution of methods. The first row refers to criteria related to the target parameters, second row refers to criteria related to target species/groups and habitats, third row refers to criteria related to resources needed. In case not all methods belonging to one observational category are appropriate for the respective target, individual methods are listed.
6 Baltic case study
The Baltic sea is a semi-enclosed, low-diversity brackish sea with a long history of anthropogenic use and currently suffers from multiple stressors like eutrophication, deoxygenation, acidification, warming and overfishing (Reusch et al., 2018). There is an increasing demand of Offshore Windfarms with a planned increase in the long term from 31 OWFs with a spatial expansion of around 500 km² to 149 OWFs with an approximate 5-fold expansion (Haase et al., 2023). Currently fishing for commercial use and fisheries research is prohibited (Haase et al., 2023) within OWFs. Besides the drastic expansion of OWFs in the Baltic Sea, marine protected areas (MPAs) will likely be expanded so that 30% of the EEZ are under protection and 10% of those under special protection by 2030 (EU, 2021). A special case of MPAs are the ‘sites of community interest’ (SCI) and ‘special protection areas’ (SPA) which form the Natura2000 network. In the German EEZ of the Baltic Sea six of those MPAs were implemented in 2017 with different management options. At least in parts of the MPAs commercial fishing with mobile bottom contacting gears will be excluded most likely in 2024, to protect reef and sandbank structures. Since it is not finally clarified whether traditional monitoring methods like bottom trawling are still viable in MPAs and are no longer an option in OWFs, the areas where traditional mobile bottom contacting methods have been used decrease while the area of “black boxes” in fisheries science increase. For example, some sampling stations of the ICES coordinated Baltic International Trawl Survey (BITS), which aims to provide data on demersal commercial species, in fact collide with OWFs in the Baltic Sea (Haase et al., 2023) (Figure 2) and become “black boxes” for fisheries management. Therefore, adaptive methods to collect data are required to be able to i) detect changes in fish communities occurring in these areas and ii) provide information for fisheries management (ICES, 2023) if necessary.
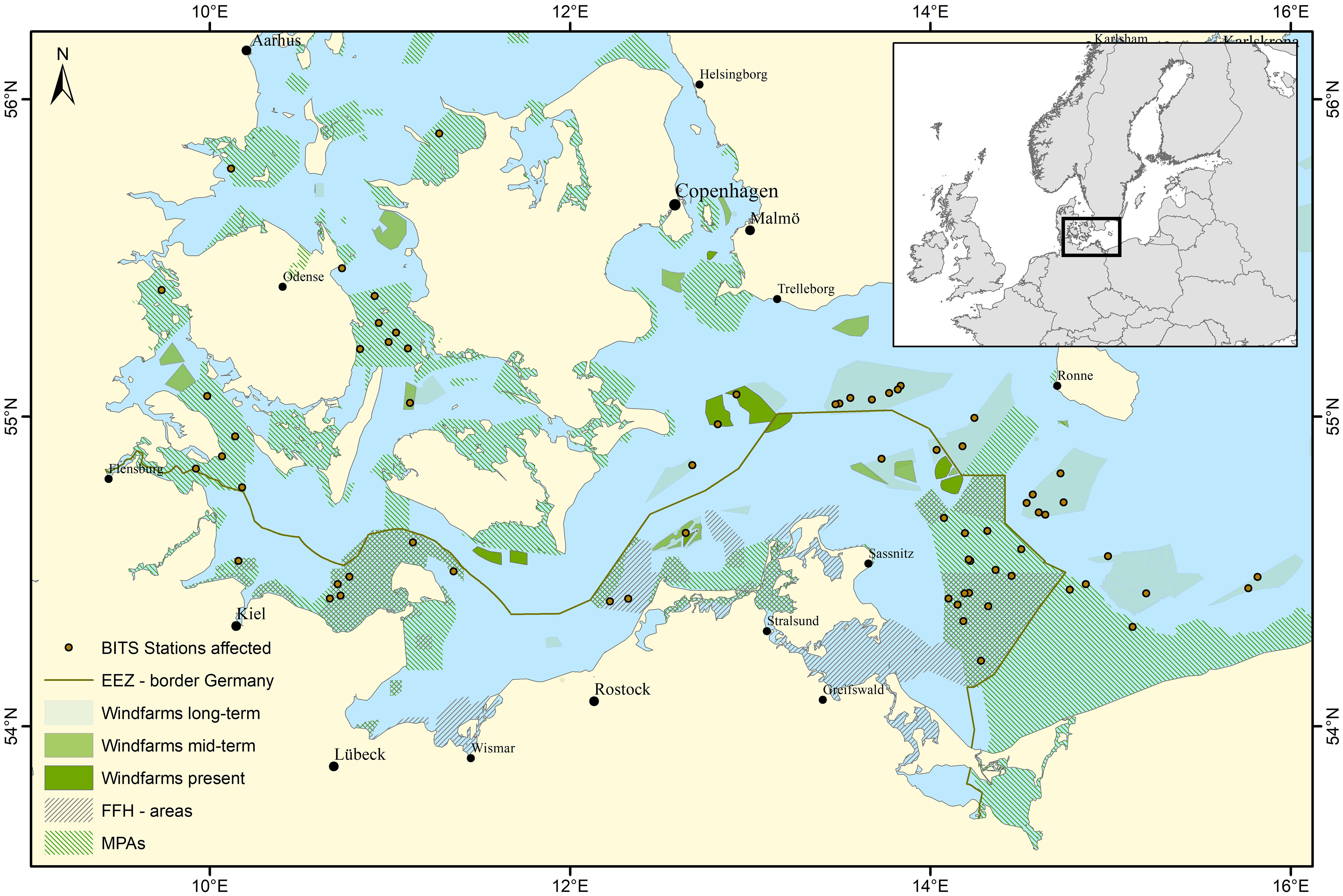
Figure 2 Western Baltic Sea including FFH–areas in- and outside of the German EEZ and coastal waters, Offshore Windfarms (OWFs) of different scenarios (defined according to Stelzenmüller et al., 2022) and affected stations of the Baltic International Trawl Survey (BITS).
To do so, data is required to evaluate relative changes in fish species diversity, abundance and biomass, as well as in food web, when assuming that the benthic fauna is changing after the exclusion of mobile bottom contacting gears or due to reef effects due to OWPs, in order to address the condition of fishes and fish communities (see Figure 1). However, in order to assess changes in species diversity a multi-species approach might be required. Furthermore, the respective method should be applicable in multiple and structural complex habitats since Baltic Sea MPAs are characterized by gravel and coarse, stony reefs or sandy bottoms. Therefore, a combination of different methods might be the best solution for a future monitoring strategy in MPAs and OWFs.
Common methods for monitoring MPAs include underwater video census (UVC), video-based observations, passive fishing gear or hydroacoustic methods. However, as mentioned above, most of them are often used in tropical reef areas, where conditions differ from those in the Baltic Sea. UVC methods for example require the engagement of divers, which in return results in depth and time limitations. Further, visibility and light conditions are often better in tropical and reef areas compared to temperate deeper areas, which results in problems using underwater video. In the Baltic Sea, for example, increased primary production results in a reduction of light penetration during blooming periods (Hopkins, 2000). However, since video-based methods have successfully been used for studies in Baltic Sea cobble and artificial reef areas and sand habitat (Rhodes et al., 2020; Wilms et al., 2021), they can be considered as a promising alternative. Since MPAs cannot be isolated from many activities and impacts outside their boundaries (Halpern et al., 2010), changes in MPAs are more likely expected to occur in less-mobile or less-migratory species (Pilyugin et al., 2016), especially in the Baltic MPAs which are relatively small. Less-mobile species are often associated with a benthic lifestyle, which further minimizes the pool of appropriate methods. Therefore, most hydroacoustic methods are not applicable here (see 4.1). However, acoustic cameras still provide a good opportunity for collecting abundance data in a relatively large spatial frame. Monitoring MPAs often aims to provide data on species diversity and follows a multi-species approach. Therefore, high taxonomic-resolution is needed, which might require the use of complementary methods like eDNA or extractive methods like potting or gill-netting. Methods used in studies evaluating the effect of OWFs on fish communities include trawling, seine and gill netting, angling, hydroacoustics, underwater video, dredging and UVC (Andersson et al., 2007). Since, the use of mobile bottom contacting gears is prohibited in OWFs in the German EEZ dredging, trawling and seine netting will most likely not be possible. Further, the above-mentioned data requirements and constraints for MPAs will most likely also apply to the case of OWFs. Consequently, methods that might be fit for purpose for monitoring fish communities in MPAs or OWFs include passive fishing (e.g. gill netting, angling), underwater video (towed or stationary), eDNA or hydroacoustic methods (acoustic tagging, acoustic camera). However, none of the above-mentioned methods will be able to show the complete picture. Due to gear specific catchability and selectivity, biases of each method have to be assessed beforehand in order to develop an appropriate monitoring strategy. In a MPA case study in the Baltic, pre-investigation on the use of alternative methods, especially video stations and eDNA, have so far only been able to detect a few species. However, eDNA in particular was able to record species that were not found in the catches of the 2m or 3m beam trawl (Hammerl, unpublished). Overall, the sampling effort for the alternative methods was low and a direct comparison with the beam trawl catches was not always possible. The number of species observed will most likely increase with increased effort. Beside the testing of alternative monitoring methods, monitoring design and optimal effort will be developed.
7 Conclusion
Our study revealed that while new sampling methods are already accessible in certain cases, they often require further refinement and development and provide less information compared to traditional bottom trawling. However, in some cases the less available data can be sufficient to address specific research questions. Since all methods have diverse and perspective-dependent advantages and disadvantages, identification of a suitable method can be challenging. The use of non-or less invasive monitoring methods in our case study is still very limited. Designing such a monitoring can be complex, especially in terms of standardization, sampling design and effort. Information about gear performance, effort quantification, catchability and selectivity of different gears have to be collected to design a cost-efficient and statistically robust monitoring program for marine protected areas and OWFs where mobile bottom trawling will likely be or is excluded. Additionally, integrating the here discussed methods into existing long-time series requires calibration experiments to ensure the quality and the right interpretation of the data. Moreover, the results show that bottom trawling is still needed, because there is currently no alternative sampling method for the data requirements of EU fisheries management for example. Data on age structure and reproductive capacity cannot be generated without extracting the fish and often requires the dissection of the fish. However, it becomes clear, that due to the current and future restrictions in fisheries data collection methods, alternative data collection methods become increasingly important.
Author contributions
CH: Conceptualization, Investigation, Writing – original draft, Methodology, Visualization. CM: Supervision, Writing – review & editing. DO: Funding acquisition, Supervision, Writing – review & editing.
Funding
The author(s) declare financial support was received for the research, authorship, and/or publication of this article. This work was carried out as part of the DAM pilot missions “MGF-Ostsee” and “MGF-Ostsee II” funded by the German Federal Ministry of Education and Research (funding codes 03F0848E/03F0937E).
Acknowledgments
We thank everyone involved in ICYMARE for the organization of the special issue and funding of the publishing costs. We also thank Nakula Plantener for providing help with figures and designing Figure 2, as well Christopher Zimmermann for his valuable comments during the writing process.
Conflict of interest
The authors declare that the research was conducted in the absence of any commercial or financial relationships that could be construed as a potential conflict of interest.
Publisher’s note
All claims expressed in this article are solely those of the authors and do not necessarily represent those of their affiliated organizations, or those of the publisher, the editors and the reviewers. Any product that may be evaluated in this article, or claim that may be made by its manufacturer, is not guaranteed or endorsed by the publisher.
Supplementary material
The Supplementary Material for this article can be found online at: https://www.frontiersin.org/articles/10.3389/fmars.2023.1322367/full#supplementary-material
References
Abramic A., Cordero-Penin V., Haroun R. (2022). Environmental impact assessment framework for offshore wind energy developments based on the marine Good Environmental Status. Environ. Impact Assess. Rev. 97, 106862. doi: 10.1016/j.eiar.2022.106862
Afonso P., Fontes J., Holland K., Santos R. (2008). Social status determines behaviour and habitat usage in a temperate parrotfish: implications for marine reserve design. Mar. Ecol. Prog. Ser. 359, 215–227. doi: 10.3354/meps07272
Afzali S. F., Bourdages H., Laporte M., Mérot C., Normandeau E., Audet C., et al. (2021). Comparing environmental metabarcoding and trawling survey of demersal fish communities in the Gulf of St. Lawrence, Canada. Environ. DNA 3, 22–42. doi: 10.1002/edn3.111
Aguzzi J., Mànuel A., Condal F., Guillén J., Nogueras M., Del Rio J., et al. (2011). The new seafloor observatory (OBSEA) for remote and long-term coastal ecosystem monitoring. Sensors 11, 5850–5872. doi: 10.3390/s110605850
Andersson M. H., Gullström M., Asplund M. E., Öhman M. C. (2007). Importance of using multiple sampling methodologies for estimating of fish community composition in offshore wind power construction areas of the Baltic Sea. Ambio 36(8), 634. doi: 10.1579/0044-7447(2007)36[634:IOUMSM]2.0.CO;2
Bacheler N. M., Schobernd C. M., Schobernd Z. H., Mitchell W. A., Berrane D. J., Kellison G. T., et al. (2013a). Comparison of trap and underwater video gears for indexing reef fish presence and abundance in the southeast United States. Fish. Res. 143, 81–88. doi: 10.1016/j.fishres.2013.01.013
Bacheler N. M., Schobernd Z. H., Berrane D. J., Schobernd C. M., Mitchell W. A., Geraldi N. R. (2013b). When a trap is not a trap: converging entry and exit rates and their effect on trap saturation of black sea bass (Centropristis striata). ICES J. Mar. Sci. 70, 873–882. doi: 10.1093/icesjms/fst062
Barnes H. (1952). Under-water television and marine biology. Nature 169, 477–479. doi: 10.1038/169477a0
Bean T. P., Greenwood N., Beckett R., Biermann L., Bignell J. P., Brant J. L., et al. (2017). A review of the tools used for marine monitoring in the UK: combining historic and contemporary methods with modeling and socioeconomics to fulfill legislative needs and scientific ambitions. Front. Mar. Sci. 4. doi: 10.3389/fmars.2017.00263
Beentjes M. P. (2019). Blue cod potting surveys: standards and specifications: Version 2. N. Z. Fish. Assess. Rep. 21,62. doi: 10.13140/RG.2.2.21226.13766
Boswell K. M., Wilson M. P., Wilson C. A. (2007). Hydroacoustics as a tool for assessing fish biomass and size distribution associated with discrete shallow water estuarine habitats in Louisiana. Estuaries Coasts 30, 607–617. doi: 10.1007/BF02841958
Christiansen H. M., Switzer T. S., Keenan S. F., Tyler-Jedlund A. J., Winner B. L. (2020). Assessing the relative selectivity of multiple sampling gears for managed reef fishes in the Eastern Gulf of Mexico. Mar. Coast. Fish. 12, 322–338. doi: 10.1002/mcf2.10129
Côté I. M., Perrow M. R. (2006). “Fish,” in Ecological Census Techniques: A Handbook. Ed. Sutherland W. J. (Cambridge University Press), 250–275.
Cowart D. A., Murphy K. R., Cheng C. H. C. (2018). Metagenomic sequencing of environmental DNA reveals marine faunal assemblages from the West Antarctic Peninsula. Mar. Genomics 37, 148–160. doi: 10.1016/j.margen.2017.11.003
Day J. (2008). The need and practice of monitoring, evaluating and adapting marine planning and management—lessons from the Great Barrier Reef. Mar. Policy Role Mar. Spatial Plann. Implementing Ecosystem-based Sea Use Manage. 32, 823–831. doi: 10.1016/j.marpol.2008.03.023
De Vos L., Götz A., Winker H., Attwood C. (2014). Optimal BRUVs (baited remote underwater video system) survey design for reef fish monitoring in the Stilbaai Marine Protected Area. Afr. J. Mar. Sci. 36, 1–10. doi: 10.2989/1814232X.2013.873739
Doi H., Uchii K., Takahara T., Matsuhashi S., Yamanaka H., Minamoto T. (2015). Use of droplet digital PCR for estimation of fish abundance and biomass in environmental DNA surveys. PloS One 10, e0122763. doi: 10.1371/journal.pone.0122763
Egli D. P., Babcock R. C. (2004). Ultrasonic tracking reveals multiple behavioural modes of snapper (Pagrus auratus) in a temperate no-take marine reserve. ICES J. Mar. Sci. 61, 1137–1143. doi: 10.1016/j.icesjms.2004.07.004
Ellis D. M., DeMartini E. E. (1995). Evaluation of a video camera technique for indexing abundances of juvenile pink snapper Pristipomoides filamentosus, and other Hawaiian insular shelf fishes. Fish. Bull. 93, 67–77.
European Commission, Directorate-General for Environment (2021). EU biodiversity strategy for 2030: bringing nature back into our lives (Publications Office of the European Union), 36. doi: 10.2779/677548
Fedra K., Machan R. (1979). A self-contained underwater time-lapse camera for in situ long-term observations. Mar. Biol. 55, 239–246. doi: 10.1007/BF00396824
Garces L. R., Silvestre G. T., Stobutzki I., Gayanilo F. C., Valdez F., Saupi M., et al. (2006). A regional database management system—the fisheries resource information system and tools (FiRST): Its design, utility and future directions. Fish. Res. 78, 119–129. doi: 10.1016/j.fishres.2006.02.003
Garlapati D., Charankumar B., Ramu K., Madeswaran P., Ramana Murthy M. V. (2019). A review on the applications and recent advances in environmental DNA (eDNA) metagenomics. Rev. Environ. Sci. Biotechnol. 18, 389–411. doi: 10.1007/s11157-019-09501-4
Goetze J. S., Bond T., McLean D. L., Saunders B. J., Langlois T. J., Lindfield S., et al. (2019). A field and video analysis guide for diver operated stereo-video. Methods Ecol. Evol. 10, 1083–1090. doi: 10.1111/2041-210X.13189
Gold Z., Sprague J., Kushner D. J., Zerecero Marin E., Barber P. H. (2021). eDNA metabarcoding as a biomonitoring tool for marine protected areas. PloS One 16, e0238557. doi: 10.1371/journal.pone.0238557
Haase S., von Dorrien C., Kaljuste O., Plantener N., Sepp E., Stelzenmüller V., et al. (2023). The rapid expansion of offshore wind farms challenges the reliability of ICES-coordinated fish surveys—insights from the Baltic Sea. ICES J. Mar. Sci fsad124. doi: 10.1093/icesjms/fsad124
Halpern B. S., Lester S. E., McLeod K. L. (2010). Placing marine protected areas onto the ecosystem-based management seascape. Proc. Natl. Acad. Sci. 107, 18312–18317. doi: 10.1073/pnas.0908503107
Hansen B. K., Bekkevold D., Clausen L. W., Nielsen E. E. (2018). The sceptical optimist: challenges and perspectives for the application of environmental DNA in marine fisheries. Fish Fish. 19, 751–768. doi: 10.1111/faf.12286
Harvey E. S., Newman S. J., McLean D. L., Cappo M., Meeuwig J. J., Skepper C. L. (2012). Comparison of the relative efficiencies of stereo-BRUVs and traps for sampling tropical continental shelf demersal fishes. Fish. Res. 125–126, 108–120. doi: 10.1016/j.fishres.2012.01.026
Harvey E., Shortis M., Stadler M., Cappo M. (2002). A comparison of the accuracy and precision of measurements from single and stereo-video systems. Mar. Technol. Soc J. 36, 38–49. doi: 10.4031/002533202787914106
Henseler C., Oesterwind D. (2023). A comparison of fishing methods to sample coastal fish communities in temperate seagrass meadows. Mar. Ecol. Prog. Ser, 715, 91–111. doi: 10.3354/meps14347
Holmes J. A., Cronkite G. M. W., Enzenhofer H. J., Mulligan T. J. (2006). Accuracy and precision of fish-count data from a “dual-frequency identification sonar” (DIDSON) imaging system. ICES J. Mar. Sci. 63, 543–555. doi: 10.1016/j.icesjms.2005.08.015
Hopkins C. C. E. (2000). “Overview of monitoring in the baltic sea,” in Report of the Global Environment Facility/Baltic Sea Regional Project (AquaMarine Advisers), 40.
ICES (2023). Workshop on a Research Roadmap for Offshore and Marine Renewable Energy (WKOMRE) (ICES Scientific Reports). doi: 10.17895/ICES.PUB.23097404
Jan R.-Q., Shao Y.-T., Lin F.-P., Fan T.-Y., Tu Y.-Y., Tsai H.-S., et al. (2007). An underwater camera system for real-time coral reef fish monitoring. Raffles Bull. Zool. 14, 273–279.
Johnson A. F., Gorelli G., Jenkins S. R., Hiddink J. G., Hinz H. (2015). Effects of bottom trawling on fish foraging and feeding. Proc. R. Soc B Biol. Sci. 282, 20142336. doi: 10.1098/rspb.2014.2336
Johnston S. V., Rivera J. A., Rosario A., Timko M. A., Nealson P. A., Kumagai K. K. (2006). Hydroacoustic evaluation of spawning red hind (Epinephelus guttatus) aggregations along the coast of Puerto Rico in 2002 and 2003. Emerg. Technol. Reef Fish. Res. Manage. NOAA Prof. Pap. NMFS 5, 10–17.
Jones E., Tselepides A., Bagley P., Collins M., Priede I. (2003). Bathymetric distribution of some benthic and benthopelagic species attracted to baited cameras and traps in the deep eastern Mediterranean. Mar. Ecol. Prog. Ser. 251, 75–86. doi: 10.3354/meps251075
Jorgensen S., Kaplan D., Klimley A., Morgan S., O’Farrell M., Botsford L. (2006). Limited movement in blue rockfish Sebastes mystinus: internal structure of home range. Mar. Ecol. Prog. Ser. 327, 157–170. doi: 10.3354/meps327157
Kaunda-Arara B., Rose G. A. (2004). Out-migration of tagged fishes from marine reef national parks to fisheries in coastal Kenya. Environ. Biol. Fishes 70, 363–372. doi: 10.1023/B:EBFI.0000035428.59802.af
Kriegl M., Elías Ilosvay X. E., von Dorrien C., Oesterwind D. (2021). Marine protected areas: at the crossroads of nature conservation and fisheries management. Front. Mar. Sci. 8. doi: 10.3389/fmars.2021.676264
Krumme U., Saint-Paul U. (2003). Observations of fish migration in a macrotidal mangrove channel in Northern Brazil using a 200-kHz split-beam sonar. Aquat. Living Resour. 16, 175–184. doi: 10.1016/S0990-7440(03)00046-9
Langlois T., Goetze J., Bond T., Monk J., Abesamis R. A., Asher J., et al. (2020). A field and video annotation guide for baited remote underwater stereo-video surveys of demersal fish assemblages. Methods Ecol. Evol. 11, 1401–1409. doi: 10.1111/2041-210X.13470
Langlois T. J., Newman S. J., Cappo M., Harvey E. S., Rome B. M., Skepper C. L., et al. (2015). Length selectivity of commercial fish traps assessed from in situ comparisons with stereo-video: Is there evidence of sampling bias? Fish. Res. 161, 145–155. doi: 10.1016/j.fishres.2014.06.008
Letessier T. B., Juhel J.-B., Vigliola L., Meeuwig J. J. (2015). Low-cost small action cameras in stereo generates accurate underwater measurements of fish. J. Exp. Mar. Biol. Ecol. 466, 120–126. doi: 10.1016/j.jembe.2015.02.013
Lindeboom H. J., Kouwenhoven H. J., Bergman M. J. N., Bouma S., Brasseur S., Daan R., et al. (2011). Short-term ecological effects of an offshore wind farm in the Dutch coastal zone; a compilation. Environ. Res. Lett. 6, 35101. doi: 10.1088/1748-9326/6/3/035101
Lowe C., Topping D., Cartamil D., Papastamatiou Y. (2003). Movement patterns, home range, and habitat utilization of adult kelp bass Paralabrax clathratus in a temperate no-take marine reserve. Mar. Ecol. Prog. Ser. 256, 205–216. doi: 10.3354/meps256205
Mallet D., Olivry M., Ighiouer S., Kulbicki M., Wantiez L. (2021). Nondestructive monitoring of soft bottom fish and habitats using a standardized, remote and unbaited 360° Video sampling method. Fishes 6, 50. doi: 10.3390/fishes6040050
Mallet D., Pelletier D. (2014). Underwater video techniques for observing coastal marine biodiversity: A review of sixty years of publications, (1952–2012). Elsevier enhanced reader. Fish. Res. 154, 44–62. doi: 10.1016/j.fishres.2014.01.019
Maruyama A., Nakamura K., Yamanaka H., Kondoh M., Minamoto T. (2014). The release rate of environmental DNA from juvenile and adult fish. PloS One 9, e114639. doi: 10.1371/journal.pone.0114639
Maureaud A., Frelat R., Pécuchet L., Shackell N., Mérigot B., Pinsky M. L., et al. (2021). Are we ready to track climate-driven shifts in marine species across international boundaries? - A global survey of scientific bottom trawl data. Glob. Change Biol. 27, 220–236. doi: 10.1111/gcb.15404
McClanahan T. R., Mangi S. (2000). Spillover of exploitable fishes from a marine park and its effect on the adjacent fishery. Ecol. Appl. 10, 1792–1805. doi: 10.1890/1051-0761(2000)010[1792:SOEFFA]2.0.CO;2
McIntyre F. D., Collie N., Stewart M., Scala L., Fernandes P. G. (2013). A visual survey technique for deep-water fishes: estimating anglerfish Lophius spp. abundance in closed areas. J. Fish Biol. 83, 739–753. doi: 10.1111/jfb.12114
McIntyre F. D., Neat F., Collie N., Stewart M., Fernandes P. G. (2015). Visual surveys can reveal rather different “pictures” of fish densities: Comparison of trawl and video camera surveys in the Rockall Bank, NE Atlantic Ocean. Deep Sea Res. Part Oceanogr. Res. Pap. 95, 67–74. doi: 10.1016/j.dsr.2014.09.005
Meyer C. G., Papastamatiou Y. P., Holland K. N. (2007). Seasonal, diel, and tidal movements of green jobfish (Aprion virescens, Lutjanidae) at remote Hawaiian atolls: implications for marine protected area design. Mar. Biol. 151, 2133–2143. doi: 10.1007/s00227-007-0647-7
Miller R. J. (1990). Effectiveness of crab and lobster traps. Can. J. Fish. Aquat. Sci. 47, 1228–1251. doi: 10.1139/f90-143
Moursund R. A., Carlson T. J., Peters R. D. (2003). A fisheries application of a dual-frequency identification sonar acoustic camera. ICES J. Mar. Sci. 60, 678–683. doi: 10.1016/S1054-3139(03)00036-5
Murphy H. M., Jenkins G. P. (2010). Observational methods used in marine spatial monitoring of fishes and associated habitats: a review. Mar. Freshw. Res. 61, 236. doi: 10.1071/MF09068
Oberle F. K. J., Storlazzi C. D., Hanebuth T. J. J. (2016). What a drag: Quantifying the global impact of chronic bottom trawling on continental shelf sediment. J. Mar. Syst. 159, 109–119. doi: 10.1016/j.jmarsys.2015.12.007
O’Donnell J. L., Kelly R. P., Shelton A. O., Samhouri J. F., Lowell N. C., Williams G. D. (2017). Spatial distribution of environmental DNA in a nearshore marine habitat. PeerJ 5, e3044. doi: 10.7717/peerj.3044
Pelletier D., Leleu K., Mallet D., Mou-Tham G., Hervé G., Boureau M., et al. (2012). Remote high-definition rotating video enables fast spatial survey of marine underwater macrofauna and habitats. PloS One 7, e30536. doi: 10.1371/journal.pone.0030536
Pelletier D., Roos D., Bouchoucha M., Schohn T., Roman W., Gonson C., et al. (2021a). A standardized workflow based on the STAVIRO unbaited underwater video system for monitoring fish and habitat essential biodiversity variables in coastal areas. Front. Mar. Sci. 8. doi: 10.3389/fmars.2021.689280
Pelletier D., Rouxel J., Fauvarque O., Hanon D., Gestalin J.-P., Lebot M., et al. (2021b). KOSMOS: an open source underwater video lander for monitoring coastal fishes and habitats. Sensors 21, 7724. doi: 10.3390/s21227724
Pilyugin S. S., Medlock J., De Leenheer P. (2016). The effectiveness of marine protected areas for predator and prey with varying mobility. Theor. Popul. Biol. 110, 63–77. doi: 10.1016/j.tpb.2016.04.005
Priede I. G., Merrett N. R. (1996). Estimation of abundance of abyssal demersal fishes; a comparison of data from trawls and baited cameras. J. Fish Biol. 49, 207–216. doi: 10.1111/j.1095-8649.1996.tb06077.x
Recksiek C. W., Appeldoorn R. S., Turingan R. G. (1991). Studies of fish traps as stock assessment devices on a shallow reef in south-western Puerto Rico. Fish. Res. 10, 177–197. doi: 10.1016/0165-7836(91)90074-P
Reusch T. B. H., Dierking J., Andersson H. C., Bonsdorff E., Carstensen J., Casini M., et al. (2018). The Baltic Sea as a time machine for the future coastal ocean. Sci. Adv. 4, eaar8195. doi: 10.1126/sciadv.aar8195
Rhodes N., Wilms T., Baktoft H., Ramm G., Bertelsen J. L., Flávio H., et al. (2020). Comparing methodologies in marine habitat monitoring research: An assessment of species-habitat relationships as revealed by baited and unbaited remote underwater video systems. J. Exp. Mar. Biol. Ecol. 526, 151315. doi: 10.1016/j.jembe.2020.151315
Rose C. S., Stoner A. W., Matteson K. (2005). Use of high-frequency imaging sonar to observe fish behaviour near baited fishing gears. Fish. Res. 76, 291–304. doi: 10.1016/j.fishres.2005.07.015
Rudershausen P. J., Mitchell W. A., Buckel J. A., Williams E. H., Hazen E. (2010). Developing a two-step fishery-independent design to estimate the relative abundance of deepwater reef fish: Application to a marine protected area off the southeastern United States coast. Fish. Res. 105, 254–260. doi: 10.1016/j.fishres.2010.05.005
Russo T., Maiello G., Talarico L., Baillie C., Colosimo G., D’Andrea L., et al. (2020). All is fish that comes to the net: metabarcoding for rapid fisheries catch assessment. Ecol. Appl. 31, e02273. doi: 10.1101/2020.06.18.159830
Salter I., Joensen M., Kristiansen R., Steingrund P., Vestergaard P. (2019). Environmental DNA concentrations are correlated with regional biomass of Atlantic cod in oceanic waters. Commun. Biol. 2, 1–9. doi: 10.1038/s42003-019-0696-8
Schobernd Z. H., Bacheler N. M., Conn P. B. (2014). Examining the utility of alternative video monitoring metrics for indexing reef fish abundance. Can. J. Fish. Aquat. Sci. 71, 464–471. doi: 10.1139/cjfas-2013-0086
Sheehan E. V., Stevens T. F., Attrill M. J. (2010). A quantitative, non-destructive methodology for habitat characterisation and benthic monitoring at offshore renewable energy developments. PloS One 5, e14461. doi: 10.1371/journal.pone.0014461
Sigsgaard E. E., Nielsen I. B., Carl H., Krag M. A., Knudsen S. W., Xing Y., et al. (2017). Seawater environmental DNA reflects seasonality of a coastal fish community. Mar. Biol. 164, 128. doi: 10.1007/s00227-017-3147-4
Southeast Data Assessment and Review (2011). SEDAR 25: Stock Assessment Report for South Atlantic Black Sea Bass, Southeast Data, Assessment, and Review, North Charleston, South Carolina. North Charleston & SouthEast Data: Assessment and Review.
Spencer M. L., Stoner A. W., Ryer C. H., Munk J. E. (2005). A towed camera sled for estimating abundance of juvenile flatfishes and habitat characteristics: Comparison with beam trawls and divers. Estuar. Coast. Shelf Sci. 64, 497–503. doi: 10.1016/j.ecss.2005.03.012
Starr R. M., Gleason M. G., Marks C. I., Kline D., Rienecke S., Denney C., et al. (2016). Targeting Abundant Fish Stocks while Avoiding Overfished Species: Video and Fishing Surveys to Inform Management after Long-Term Fishery Closures. PloS One 11, e0168645. doi: 10.1371/journal.pone.0168645
Starr R. M., O’Connell V., Ralston S., Breaker L. (2005). Use of acoustic tags to estimate natural mortality, spillover, and movements of lingcod (Ophiodon elongatus) in a marine reserve. Mar. Technol. Soc J. 39, 19–30. doi: 10.4031/002533205787521677
Stelzenmüller V., Letschert J., Gimpel A., Kraan C., Probst W. N., Degraer S., et al. (2022). From plate to plug: The impact of offshore renewables on European fisheries and the role of marine spatial planning. Renew. Sustain. Energy Rev. 158, 112108. doi: 10.1016/j.rser.2022.112108
Stoner A. W. (2004). Effects of environmental variables on fish feeding ecology: implications for the performance of baited fishing gear and stock assessment. J. Fish Biol. 65, 1445–1471. doi: 10.1111/j.0022-1112.2004.00593.x
Stoner A. W., Laurel B. J., Hurst T. P. (2008). Using a baited camera to assess relative abundance of juvenile Pacific cod: Field and laboratory trials. J. Exp. Mar. Biol. Ecol. 354, 202–211. doi: 10.1016/j.jembe.2007.11.008
Strickler K. M., Fremier A. K., Goldberg C. S. (2015). Quantifying effects of UV-B, temperature, and pH on eDNA degradation in aquatic microcosms. Biol. Conserv. 183, 85–92. doi: 10.1016/j.biocon.2014.11.038
Takahara T., Minamoto T., Yamanaka H., Doi H., Kawabata Z. (2012). Estimation of fish biomass using environmental DNA. PloS One 7, e35868. doi: 10.1371/journal.pone.0035868
Tessier A., Descloux S., Lae R., Cottet M., Guedant P., Guillard J. (2016). Fish assemblages in large tropical reservoirs: overview of fish population monitoring methods. Rev. Fish. Sci. Aquac. 24, 160–177. doi: 10.1080/23308249.2015.1112766
Thomsen P. F., Kielgast J., Iversen L. L., Møller P. R., Rasmussen M., Willerslev E. (2012). Detection of a diverse marine fish fauna using environmental DNA from seawater samples. PloS One 7, e41732. doi: 10.1371/journal.pone.0041732
Thrush S., Schultz D., Hewitt J., Talley D. (2002). Habitat structure in soft-sediment environments and abundance of juvenile snapper Pagrus auratus. Mar. Ecol. Prog. Ser. 245, 273–280. doi: 10.3354/meps245273
Trenkel V., Vaz S., Albouy C., Brind’Amour A., Duhamel E., Laffargue P., et al. (2019). We can reduce the impact of scientific trawling on marine ecosystems. Mar. Ecol. Prog. Ser. 609, 277–282. doi: 10.3354/meps12834
Trobbiani G. A., Irigoyen A., Venerus L. A., Fiorda P. M., Parma A. M. (2018). A low-cost towed video camera system for underwater surveys: comparative performance with standard methodology. Environ. Monit. Assess. 190, 1–12. doi: 10.1007/s10661-018-7070-z
Wakefield C. B., Lewis P. D., Coutts T. B., Fairclough D. V., Langlois T. J. (2013). Fish assemblages associated with natural and anthropogenically-modified habitats in a marine embayment: comparison of baited videos and opera-house traps. PloS One 8, e59959. doi: 10.1371/journal.pone.0059959
Watson D. L., Harvey E. S., Anderson M. J., Kendrick G. A. (2005). A comparison of temperate reef fish assemblages recorded by three underwater stereo-video techniques. Mar. Biol. 148, 415–425. doi: 10.1007/s00227-005-0090-6
Wells R. J. D., Boswell K. M., Cowan J. H., Patterson W. F. (2008). Size selectivity of sampling gears targeting red snapper in the northern Gulf of Mexico. Fish. Res. 89, 294–299. doi: 10.1016/j.fishres.2007.10.010
Whitmarsh S. K., Fairweather P. G., Huveneers C. (2017). What is Big BRUVver up to? Methods and uses of baited underwater video. Rev. Fish Biol. Fish. 27, 53–73. doi: 10.1007/s11160-016-9450-1
Willis T. J., Babcock R. C. (2000). A baited underwater video system for the determination of relative density of carnivorous reef fish. Mar. Freshw. Res. 51, 755. doi: 10.1071/MF00010
Willis T., Millar R., Babcock R. (2000). Detection of spatial variability in relative density of fishes:comparison of visual census, angling, and baited underwater video. Mar. Ecol. Prog. Ser. 198, 249–260. doi: 10.3354/meps198249
Wilms T. J. G., Norðfoss P. H., Baktoft H., Støttrup J. G., Kruse B. M., Svendsen J. C. (2021). Restoring marine ecosystems: Spatial reef configuration triggers taxon-specific responses among early colonizers. J. Appl. Ecol. 58, 2936–2950. doi: 10.1111/1365-2664.14014
Zeller D. C. (1998). Spawning aggregations: patterns of movement of the coral trout Plectropomus leopardus (Serranidae) as determined by ultrasonic telemetry. Mar. Ecol. Prog. Ser. 162, 253–263. doi: 10.3354/meps162253
Keywords: underwater video, passive gears, hydroacoustics, marine protected areas, eDNA, offshore wind farms, sustainable fisheries, impact assessment
Citation: Hammerl C, Möllmann C and Oesterwind D (2024) Identifying fit-for purpose methods for monitoring fish communities. Front. Mar. Sci. 10:1322367. doi: 10.3389/fmars.2023.1322367
Received: 16 October 2023; Accepted: 26 December 2023;
Published: 12 January 2024.
Edited by:
Yvonne Schadewell, University of Duisburg-Essen, GermanyReviewed by:
José Lino Vieira De Oliveira Costa, University of Lisbon, PortugalMatteo Zucchetta, National Research Council (CNR), Italy
Copyright © 2024 Hammerl, Möllmann and Oesterwind. This is an open-access article distributed under the terms of the Creative Commons Attribution License (CC BY). The use, distribution or reproduction in other forums is permitted, provided the original author(s) and the copyright owner(s) are credited and that the original publication in this journal is cited, in accordance with accepted academic practice. No use, distribution or reproduction is permitted which does not comply with these terms.
*Correspondence: Constanze Hammerl, constanze.hammerl@thuenen.de