Fe mediated alleviation effect of cadmium toxicity in mangrove Avicennia marina (Forssk.) Vierh
- 1School of Emergency Management, School of the Environment and Safety Engineering, Jiangsu University, Zhenjiang, China
- 2State Key Laboratory of Estuarine and Coastal Research, East China Normal University, Shanghai, China
Iron (Fe) supply is one of the key factors in alleviating cadmium (Cd) stress in different plants, including mangroves. However, the effects and mechanisms of Fe in mediating the mangrove response to Cd stress still need to elaborate on the level of plant physiology and metabolism. Herein a 40-day hydroponic study was conducted to evaluate the mediating effect of Fe on Cd tolerance of Avicennia marina (Forssk.) Vierh. Results showed that the Fe supply directly improved the activity of ferric chelate reductase (FCR), increased Fe concentration in tissues, enhanced its competitiveness with Cd, and improved Fe bioavailability, thereby reducing the uptake of Cd by plants. Simultaneously, moderate Fe enhanced the photosynthesis and increased concentrations of total phenolics and tannins in both roots and leaves that play a critical role in chelating and immobilizing Cd, thus restricting the Cd translocation from roots to aboveground tissues. Additionally, the application of Fe significantly upregulated the activity of antioxidant enzymes, including POD and PPO and hence scavenging the reactive oxygen species caused by Cd stress. In conclusion, moderate Fe application improved the growth of A. marina and enhanced its tolerance to Cd at physiological and metabolic levels. Therefore, improving Fe bioavailability will reduce the mangrove degradation caused by Cd contamination, and also this may be a potential way to restore contaminated mangrove ecosystems. Protecting and restoring mangroves is not only essential for preserving biodiversity and supporting coastal ecosystems but also crucial for maintaining their role as crucial carbon sinks (i.e., mangrove blue carbon) combating global warming and climate change.
1 Introduction
Mangroves are critical transitional ecosystems distributed between the marine and terrestrial systems with an irreplaceable role in maintaining the balance of coastal ecosystems. Additionally, they serve as a vital natural carbon sink, absorbing and storing significant amounts of carbon dioxide, thus contributing to climate change mitigation. The global carbon burial (carbon accumulation rates) in mangrove sediments was estimated to be 32.2 Tg yr-1. This accounts for about 0.35% of anthropogenic carbon emissions globally (Jennerjahn, 2020). Although, regarding the total emissions, this number seems negligible, it is still a significant natural carbon sink that needs to be protected to maintain stable and sustainable biogeochemical cycles of various biogenic elements and factors in the ecosystem (Zhu and Yan, 2022). However, mangroves are degenerating and disappearing at a rate between 0.16% and 0.39% per year (Hamilton and Casey, 2016). Other relevant studies showed that the degeneration of mangroves could be attributed to environmental pollutants, especially heavy metal contamination caused by human activities (Li et al., 2016; Jian et al., 2019; Hong et al., 2021a). Due to the special geographic location and unique habitat, a variety of physical and chemical processes occurred (e.g., adsorption, desorption, sedimentation, redox, ligand exchange, and precipitation) in mangrove sediments where heavy metals tended to accumulate (Pan and Wang, 2012; Jian et al., 2017b; Wang et al., 2020). Unlike organic contaminants, heavy metals could not be degraded. Instead, they accumulated and enriched in plants and benthos (Wu et al., 2017; Jian et al., 2019). These heavy metals are then further enriched via food chains and may hence cause risk to ecosystems and human health.
Cadmium (Cd) is one of the most toxic heavy metals that severely threatens the stability and sustainability of coastal ecosystems. For mangroves, Cd causes the overproduction of reactive oxygen species (ROS), which disrupts the intracellular environment and interferes physiological metabolism of plants. On the other hand, Cd affects enzyme activities and inhibits photosynthesis, ultimately leading to retardation and chlorosis of plants (Dai et al., 2017; Jiang et al., 2017). Under the Cd stress, mangrove plants have a series of protective enzymatic and non-enzymatic mechanisms to eliminate the ROS, delay the oxidation of bioactive molecules, alleviate oxidative stress caused by Cd and maintain intracellular homeostasis. In plant tissues, superoxide dismutase (SOD), peroxidases (POD), and polyphenol oxidases (PPO) are ubiquitous enzymes with antioxidant activity (Yan and Tam, 2011; Webb et al., 2014). In contrast, non-enzymatic mechanisms involve glutathione, ascorbate, phenolic compounds, and other low molecular weight antioxidants (Jian et al., 2019). Phenolic compounds, including tannins, are typical secondary metabolites of plants with relatively strong antioxidant capacity due to their redox properties (Yan and Tam, 2011). In mangrove plants, phenolic compounds were reported to comprise up to 40% dry weight in leaves and bark tissues (Wang et al., 2014). Moreover, relevant research has suggested that phenolic compounds play a critical role in the detoxification and immobilization of Cd. For example, in Kandelia obovata, total phenol concentration significantly increased under Cd stress, and a positive correlation was obtained between phenolic metabolism-related enzyme activity and Cd level (Chen et al., 2019). Similarly, another study on Aegiceras corniculatum showed that Cd stress stimulated the synthesis of tannins, which together with other polyphenols restricted the translocation of Cd from roots to leaves (Jiang et al., 2017). POD and PPO were also reported to be associated with phenolic metabolism. POD-mediated polymerization of phenols is enhanced under Cd stress (Lavid et al., 2001a), while PPO participates in the biosynthesis of polyphenols and potentially synthesizes specialized metabolites and responds to environmental factors (Chen et al., 2019).
Iron (Fe) is one of the essential elements for plant metabolism processes and growth, while iron deficiency can cause leaf chlorosis and plant growth retardation (Jian et al., 2017b). Simultaneously, Fe also plays a critical role in plants’ tolerance to Cd stress via different pathways and mechanisms (Table 1), including regulating photosynthesis, antioxidation, and phenolic metabolism systems and affecting the formation of iron plaque, etc. Although the total Fe concentration in mangrove sediments is relatively high, the concentration of bioavailable Fe may not be sufficient for mangrove plant growth due to geochemical constraints (Alongi, 2010). Therefore, the low bioavailability of Fe in sediments is one of the key limitation factors affecting the growth and stress tolerance of mangroves (Jian et al., 2019). In addition to the low bioavailability of Fe in the mangrove sediments, Cd can also cause Fe deficiency in plants due to competing with Fe for ion channels and binding sites, interfering with enzyme activities, inhibiting the biosynthesis of photosynthesis pigments, resulting in the chlorosis, and ultimately caused low biomass and growth retardation. (Yoshihara et al., 2006; Gao et al., 2011; Jian et al., 2019).
Avicennia marina (Forssk.) Vierh, the grey mangrove, is a pioneer mangrove species on the southeast coast of China and a typical Fe reduction strategy plant that acquires Fe through the reduction of ferric mediated by membrane-bound ferric chelate reductase (FCR) (Li et al., 2015; Li et al., 2016). Previous research demonstrated that exogenous Fe convincingly promoted the bioavailability of Fe, decreased the uptake of Cd by plants, upregulated the Cd detoxification, and ultimately alleviated the biotoxicity of Cd (Jian et al., 2019). However, for A. marina, the mechanism of exogenous Fe in regulating Cd stress tolerance and the role of FCR in these processes still needs to be further investigated at physiological and metabolic levels in plants. Thus, in this study, Cd stress and exogenous Fe treatments were introduced to 1) reveal the mechanism of A. marina tolerance to Cd stress from the perspective of plant physiology and metabolism, 2) clarify the regulation mechanisms of exogenous Fe on the Cd detoxification processes, and 3) further explore the effects of Fe in the restoration of cadmium contaminated mangrove ecosystems.
2 Materials and methods
2.1 Plant materials and experimental design
Mature A. marina propagules used in this experiment were collected from the Longhai Mangrove Natural Reserve, Jiulong River Estuary, Fujian province, China (24°24’ N, 117°55’ E). Propagules were disinfected with 1% (w/v) KMnO4 and washed with deionized water. High vitality propagules were chosen for further pre-cultivation in sea sands. Propagules with a comparable diameter (2.5 ± 0.5 cm) were selected and transplanted into black polyethylene seedling pots with a modified Hoagland nutrient solution, the formula was adapted from previous study on A. marina (Li et al., 2015). All seedling pots were placed in a greenhouse with a daily temperature of 25 ± 5 °C (60-80% relative humidity, and 12 h dark/light). The nutrient solution was renewed every 3 days until seedlings had 3-4 fully developed leaves. After that, different treatments were introduced.
The experiment process included two stages (as a cycle), iron plaque induction and Cd stress. Each treatment had three replicates. In the first stage, iron plaque on the root surface was induced by using four different levels of FeSO4·7H2O: no exogenous Fe (0.02 mmol L-1 Fe from the nutrient solution as Fe0), low-dose Fe (0.6 mmol L-1 Fe, as Fe1), medium-does Fe (1.2 mmol L-1 Fe, as Fe2), and high-does Fe (1.8 mmol L-1 Fe, as Fe3). Subsequently, during the Cd stress stage, three levels of Cd (as CdCl2) were introduced (0 mg L-1, 0.5 mg L-1, and 2 mg L-1 as Cd0, Cd1, and Cd2). The applied doses of Cd and Fe were determined based on previous studies on mangrove species (Dai et al., 2017; Jian et al., 2019). The single cycle lasted eight days, including two days in the first stage and six days in the second. During the experiment, those cycles were repeated five times in the total 40 days culture period.
2.2 Harvesting and sampling
After 40 days of cultivation in the greenhouse, all A. marina were harvested and washed gently with deionized water. After washing, plants were separated into three parts: roots, stems, and leaves, biomass was measured and partial samples were dried at 80 °C to constant weights for metal element analysis. The remaining leaves and roots samples were used for the biochemical analysis.
2.3 Sample analysis
2.3.1 Plant biochemical analysis
Fresh leaf samples weighing 0.5g were ground in 95% alcohol and filtered in darkness. The extract was diluted with 95% alcohol to 25 ml, and the absorbance of the mixture at 649 and 665 nm was measured by an ultraviolet-visible spectrophotometer (UV-2600, Shimadzu, Japan). Total chlorophyll concentration was calculated using the formula of (Lichtenthaler and Wellburn, 1983):
Where C a, C b, and C t are chlorophyll a, chlorophyll b, and total chlorophyll concentrations (mg g-1 FW). A649 and A665 refer to the absorbance of the sample at 649 and 665 nm, respectively.
Peroxidase (POD) and polyphenol oxidase (PPO) levels were determined by the method described by (Dörnenburg and Knorr, 1997). In brief, fresh samples were homogenized with phosphate buffer and centrifuged, all above extraction procedures were carried out at 4 °C and enzyme extracts were diluted with buffer. After that, hydrogen peroxide and pyrogallol were used as substrates and mixed with enzyme extracts to measure the activity of POD. Similarly, to determine the level of PPO, catechol solution was mixed with enzyme extracts. The enzyme assay was performed at 25°C, the absorbance of these mixtures was measured at 420 nm, an increase in absorbance of 0.001 min-1 was taken as one unit (U) of enzyme activity.
Fresh tissues were used to extract phenolics by the 70% (v/v) acetone method described by (Guangqiu et al., 2007). Subsequently, the Folin-Ciocalteu reagent was introduced to determine the total phenolics. The absorbance of the mixture was measured at 725 nm (Makkar, 2003). Simultaneously, to measure the total tannin concentration, polyvinylpolypyrrolidone (PVPP) was introduced to bind tannins in the extracts. In brief, 100 mg PVPP was added to 1.0 ml ultrapure water (18 MΩ), and a 1.0 ml extract sample was added. The mixture was then stirred and centrifugated at 4 °C. After that, the concentration of non-tannin phenolics in the supernatant was measured as mentioned above. The total tannin concentration was then calculated as follows:
Ferric chelate reductase (FCR) activity of roots and leaves was measured according to (Jin et al., 2007) with slightly modified. Briefly, 1 g of excised sample was put into 50 ml of assay solution with 0.5mM CaSO4, 0.1mM 4,7-diphenyl-1,10-phenanthroline-disulfonic acid, 0.1 mM 2-morpholinoethanesulfonic acid (MES), and 0.1mM of Fe(III)-EDTA at a pH of 5.5 adjusted by 1M NaOH. The flask was kept in the dark in a shaking water bath for 1 h at 25°C, 50 rpm. Then, the absorbance was measured at 535 nm. The concentration of Fe(II) was quantified using a molar extinction coefficient of 22.14 mM-1 cm-1.
2.3.2 Determination of Cd and Fe concentration in plant tissues
Approximately 0.5 g of samples were weighed into the polytetrafluoroethylene digestion tube and digested as described by (Soto-Jiménez and Páez-Osuna, 2001). The concentration of Cd and Fe was determined by flame atomic absorption spectrometry (FL-AAS, AA-6800, Shimadzu, Japan). Reagent blanks and standard references (GBW-07603, from the National Research Center for Standards in China) were introduced for quality assurance and control in the determination process. The recovery rate was 99% - 103% for Fe and 98% - 101% for Cd. All of the above reagents were analytical grade or guaranteed grade bought from Sinopharm Chemical Reagent Co., Ltd. Glassware was acid-cleaned (14% (v/v) HNO3) and rinsed with ultrapure water (18 MΩ) before use.
The translocation factor (TF) was introduced to describe the translocation of Cd from plant roots to stems and leaves (Jian et al., 2017a). TF was calculated as follows:
Where TF R to S is the translocation factor of Cd from roots to stems and TF S to L is the translocation factor of Cd from stems to leaves. C leaves, C stems, and C roots are Cd concentrations in leaves, stems, and roots of A. marina, respectively (mg kg-1 DW).
2.4 Statistical analysis
Statistical analyses were performed using SPSS Statistics 24 (IBM SPSS Statistics for Windows, IBM Corporation, Armonk, NY, USA). Cd and Fe concentrations were considered fixed effects, with replicates considered random effects. All graphs were plotted using OriginPro 2021b (OriginLab, MA, USA). Differences between data from the various treatments were determined using Duncan’s and LSD multiple comparison tests. Treatments were considered significant when F-test P values were< 0.05. The means reported are the least-squares means ± standard deviation of three replicates.
3 Results
3.1 Plant biomass and chlorophyll concentration in leaves
The biomass of A. marina treated by different Fe supply and Cd stress are shown in Table 2. Fe treatments alone (without Cd stress) significantly increased all of aboveground biomass (P< 0.05), belowground biomass (P< 0.01), and total biomass (P< 0.01). Cd1 treatment alone (without Fe supply) did not significantly affect the biomass of A. marina. Conversely, for Cd2 treatment alone the aboveground biomass (P< 0.01), belowground biomass (P< 0.05), and total biomass (P< 0.01) all significantly decreased. Significant negative correlations were observed between different Cd treatments and aboveground biomass (r = -0.682, P< 0.01), and total biomass (r = -0.598, P< 0.01) regardless of different Fe supplies. Furthermore, Fe and Cd treatment interactions were significant on belowground and total biomass (P< 0.01, P< 0.01, respectively, Table 2). Within the same Cd treatment (Cd0, Cd1, or Cd2), the Fe supply generally increased plant biomass (belowground biomass and total biomass) relative to the Fe0 treatments, and Fe2 or Fe3 supply resulted in either similar or greater plant biomass compared with the Fe1 treated plants. For example, under Cd2 treatment, the addition of Fe1, Fe2, and Fe3 supply increased belowground biomass by 34.95%, 73.66%, and 51.61%, and total biomass increased by 23.26%, 30.56%, and 29.34%, respectively.
Compared with Cd0 treated plants, Cd1 treatment alone had no significant influence on chlorophyll concentration in leaves, and Cd2 treatment caused a considerable decrease in chlorophyll concentration (Figure 1). Fe1 (P< 0.05) and Fe2 (P< 0.05) treatment alone significantly increased chlorophyll concentration in plant leaves, whereas under Fe3 treatment, the concentration was similar to Fe0 treated plants. Moreover, under the same Cd stress (Cd1 or Cd2), the addition of Fe1 and Fe2 treatments increased the chlorophyll concentration, while there was no difference between Fe0 and Fe3 treated plants. For example, chlorophyll concentration was lowest in Cd2 combined with Fe0 treatment, and with the introduction of Fe1 and Fe2 treatments, the chlorophyll concentration in leaves of A. marina increased by 16.27% and 29.93%, respectively. However, Fe3 treatment decreased chlorophyll concentration to a level similar to that of the Cd2Fe0.
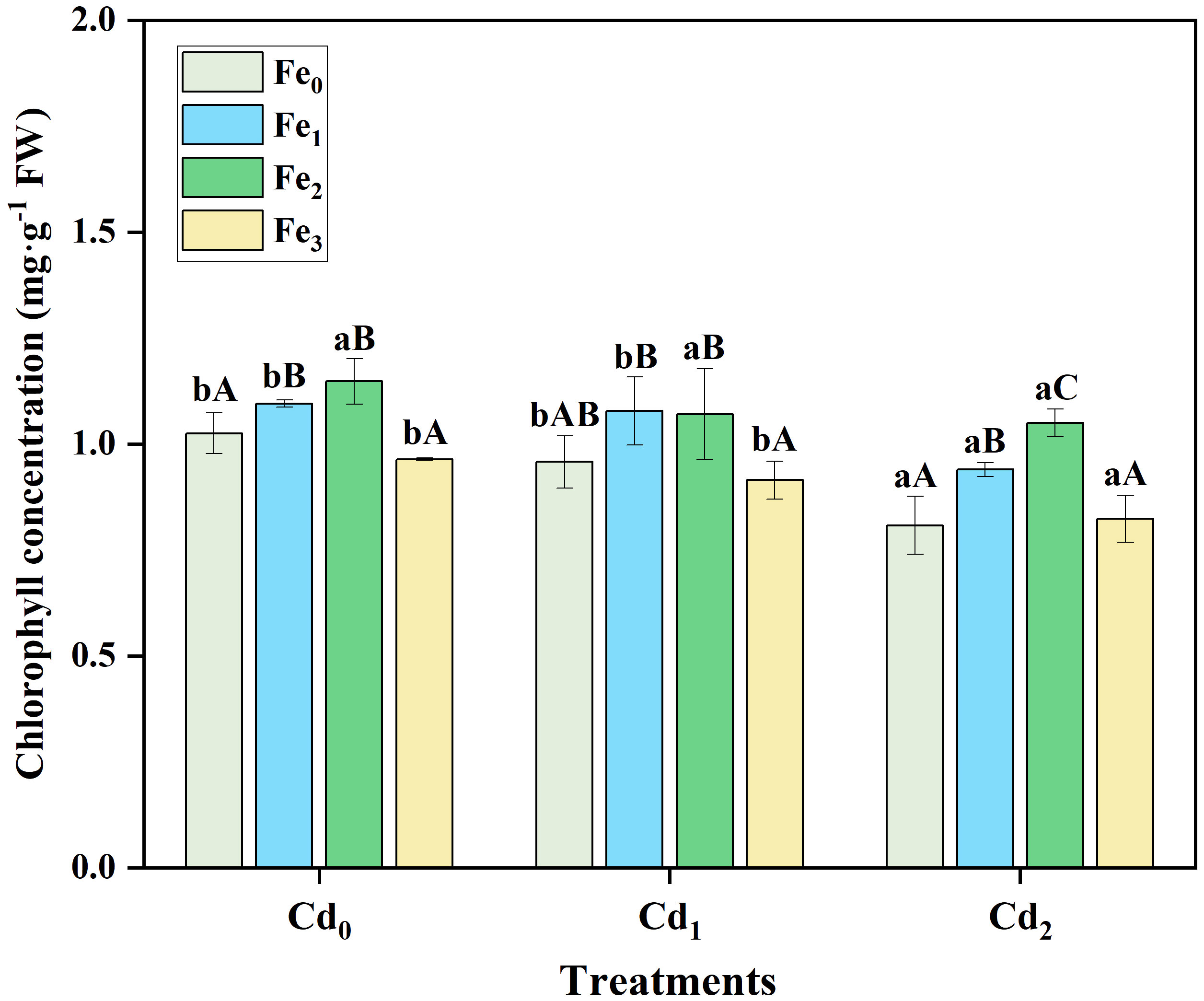
Figure 1 Chlorophyll concentration in leaves of A. marina under different Cd and Fe treatments. Different lowercase letters (a and b) indicate significant differences across different Cd treatments under the same Fe treatment (P< 0.05, n = 3). Different uppercase letters (A, B, and C) indicate significant differences across different Fe treatments under the same Cd treatment (P< 0.05, n = 3).
3.2 Cd and Fe distribution in plant tissues
Cd was mainly distributed in roots, and only part of Cd migrated to stems and leaves irrespective of different treatments (Figures 2A, B), except for the Cd2Fe0 treatment, in which Cd concentration was higher in stems than in roots and leaves. Significant two-way interactions from Fe and Cd treatments in TF R to S and TF S to L were observed (P< 0.01, P< 0.01 respectively, Figures 2A, B). Under Cd1 (P< 0.01) and Cd2 (P< 0.01) stress, the addition of Fe remarkably decreased roots to stems translocation factor of Cd, and no significant difference was observed among Fe1, Fe2, and Fe3 treatments under the same Cd stress. Similarly, under Cd2 stress, the addition of Fe significantly (P< 0.01) decreased the stems to leaves translocation factor of Cd, and the TF S to L further decreased with the increase of Fe supply (Figure 2B). However, under Cd1 treatment combined with Fe1 and Fe2 supply, TF S to L did not significantly change, and with Fe3 supply, TF S to L considerably (P< 0.01) decreased.
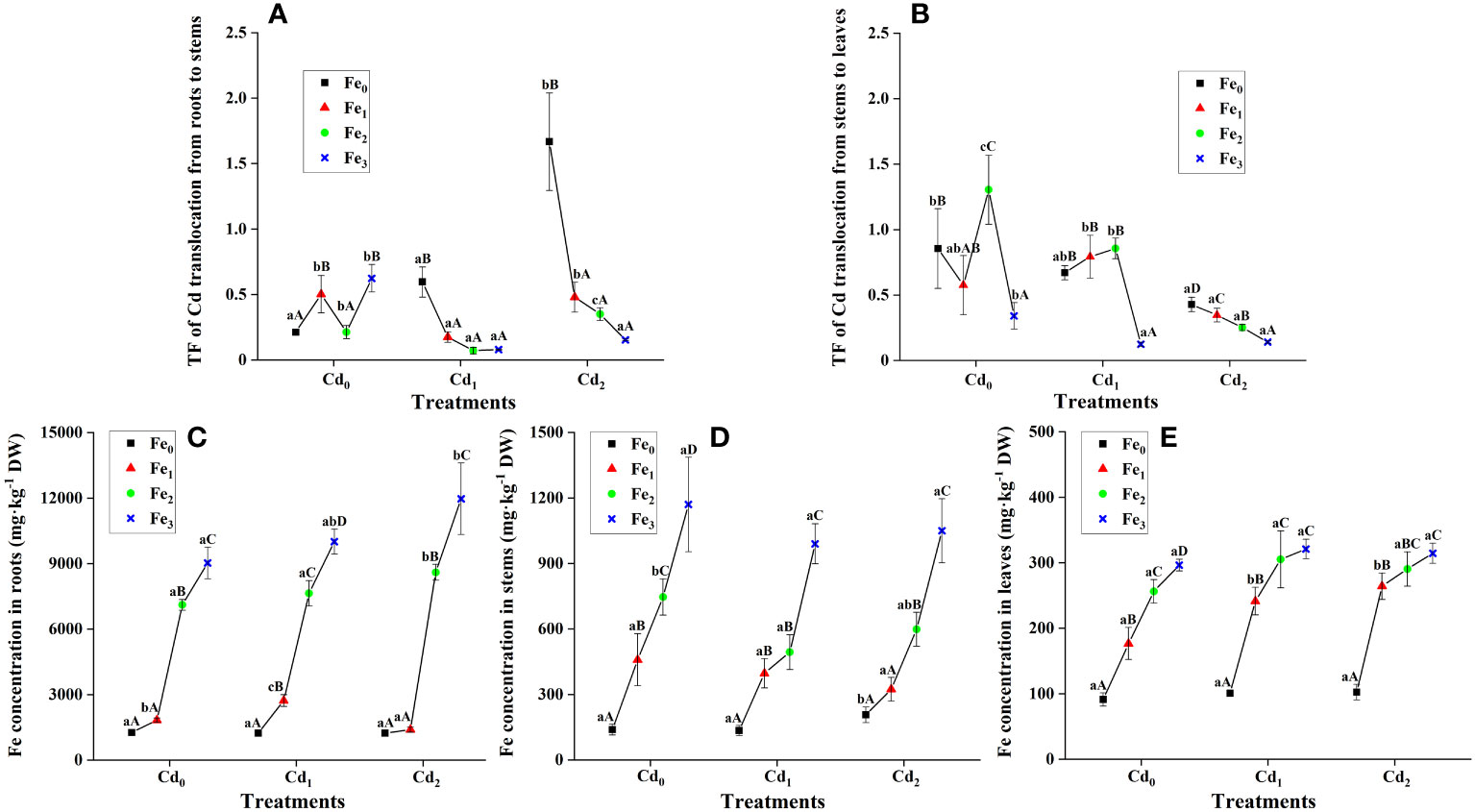
Figure 2 Translocation factor (TF) of Cd from roots to stems (A) and from stems to leaves (B) under different Cd and Fe treatments. Fe concentration in roots (C), stems (D), and leaves (E). Different lowercase letters (a, b, and c) indicate significant differences across different Cd treatments under the same Fe treatment (P< 0.05, n = 3). Different uppercase letters (A, B, C, and D) indicate significant differences across different Fe treatments under the same Cd treatment (P< 0.05, n = 3).
Cd treatments alone did not significantly affect Fe concentrations in roots and leaves of A. marina. In contrast, the addition of Fe significantly increased Fe concentrations in all of the roots, stems, and leaves regardless of different Cd treatments (P< 0.01, P< 0.01, and P< 0.01, respectively, Figures 2C–E), and significant positive correlations were observed between different Fe treatments and Fe concentrations in roots and leaves (Figure 3).
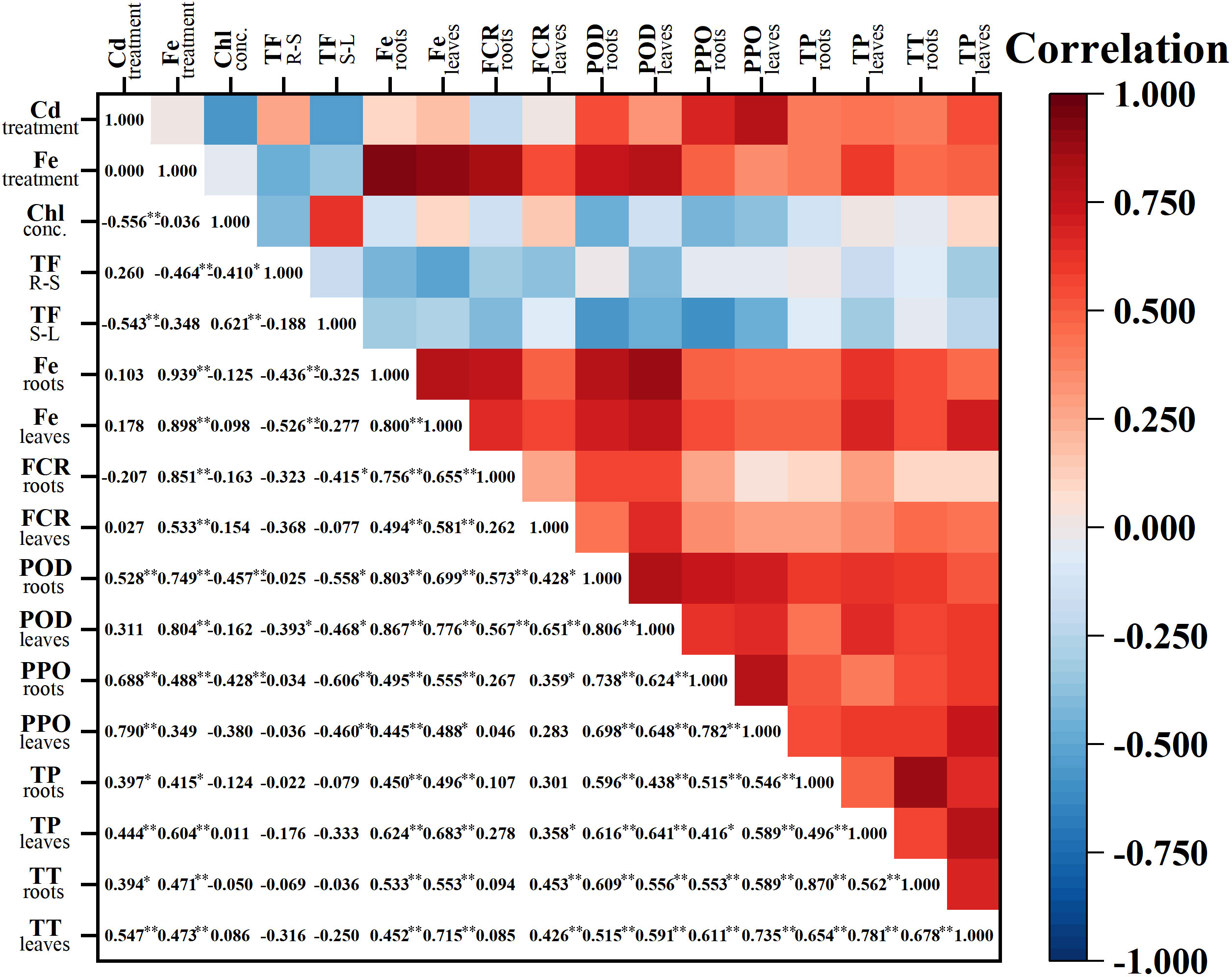
Figure 3 Pearson correlation coefficient among different treatments and determined parameters. P< 0.05; **: P< 0.01; Red indicates a positive correlation, blue indicates a negative correlation. Chl, chlorophyll; TF R-S, translocation factor of Cd from roots to stems; TF S-L, translocation factor of Cd from stems to leaves; TP, total phenolics; TT, total tannin.
3.3 Effects of Fe on antioxidative enzymes and ferric chelate reductase activities under Cd stress
Cd treatment alone significantly affected FCR activity in leaves (P< 0.05) and roots (P< 0.05) of A. marina (Figure 4). In leaves, compared with Cd0Fe0, Cd1Fe0 treatment resulted in a significant (P< 0.05) higher FCR activity, while under a higher Cd2 stress, the FCR activity decreased to a similar level to Cd0Fe0 treated plants. Fe1 and Fe2 treatments alone did not significantly affect the FCR activity in leaves, whereas Fe3 supply caused a significant (P< 0.05) higher FCR activity. Moreover, there was a significant (P< 0.01) effect of Fe and Cd interaction on FCR activity in leaves. In Fe treated (Fe1, Fe2, and Fe3) plants, Cd1 stress generally led to higher FCR activity. However, FCR activity in leaves generally decreased under Cd2 stress compared with Cd1 treated plants. In roots, Cd1 stress alone did not significantly affect FCR activity, whereas Cd2 stress alone resulted in a significant decrease in FCR activity (Figure 4). Fe supply alone significantly (P< 0.01) increased FCR activity, and there was a positive correlation between Fe treatments and roots FCR activity (r = 0.881, P< 0.01). Additionally, the application of Cd stress caused either similar or lower FCR activity in the root of Fe treated plants. For example, roots FCR activity decreased by 19.46% and 19.55% in Cd1Fe3 and Cd2Fe3 treatments, respectively, compared with Cd0Fe3 treatment.
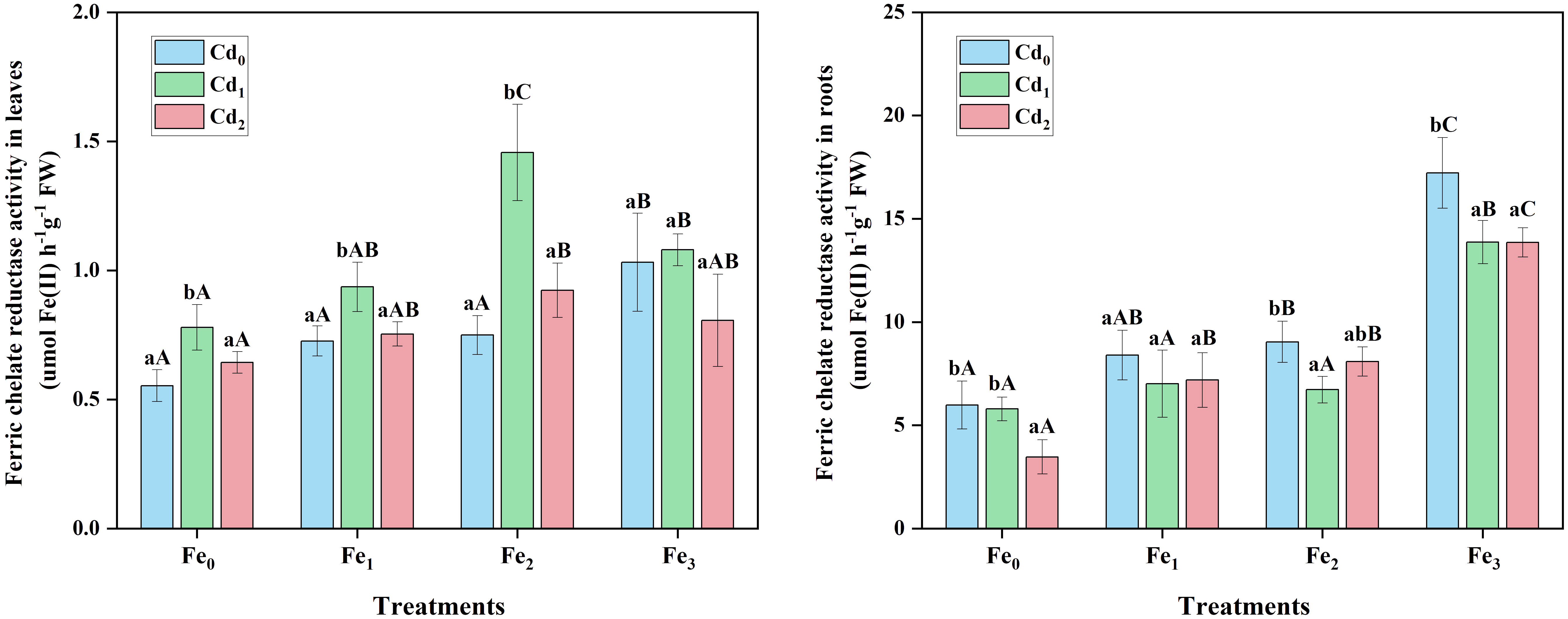
Figure 4 Ferric chelate reductase (FCR) activity in leaves and roots of A. marina under different Cd and Fe treatments. Different lowercase letters (a and b) indicate significant differences across different Cd treatments under the same Fe treatment (P< 0.05, n = 3). Different uppercase letters (A, B, and C) indicate significant differences across different Fe treatments under the same Cd treatment (P< 0.05, n = 3).
In both leaves (P< 0.05) and roots (P< 0.05) of A. marina, POD activity significantly increased under Cd1 stress (Figure 5). And compared with Cd1 treated plants, Cd2 resulted in a higher POD activity in plant tissues. Fe supply alone led to either similar or greater POD activity, and significant positive correlations were obtained between Fe treatments and POD activity in both leaves (r = 0.872, P< 0.01) and roots (r = 0.942, P< 0.01). Moreover, Fe supplied to Cd treated plants further increased POD activity in leaves. For example, compared with Cd2Fe0 treatment, POD activity in leaves increased by 21.25%, 477.47%, and 449.13% in Cd2Fe1, Cd2Fe2, and Cd2Fe3 treatments, respectively, and the POD activity in Cd2Fe2 treated plants was the highest (165.73 ± 20.70 U g-1 FW, Figure 5). In roots, Fe supply also increased POD activity, and the highest POD activity was observed in Cd2 combined with Fe3 treatment (833.80 ± 29.30 U g-1 FW, Figure 5).
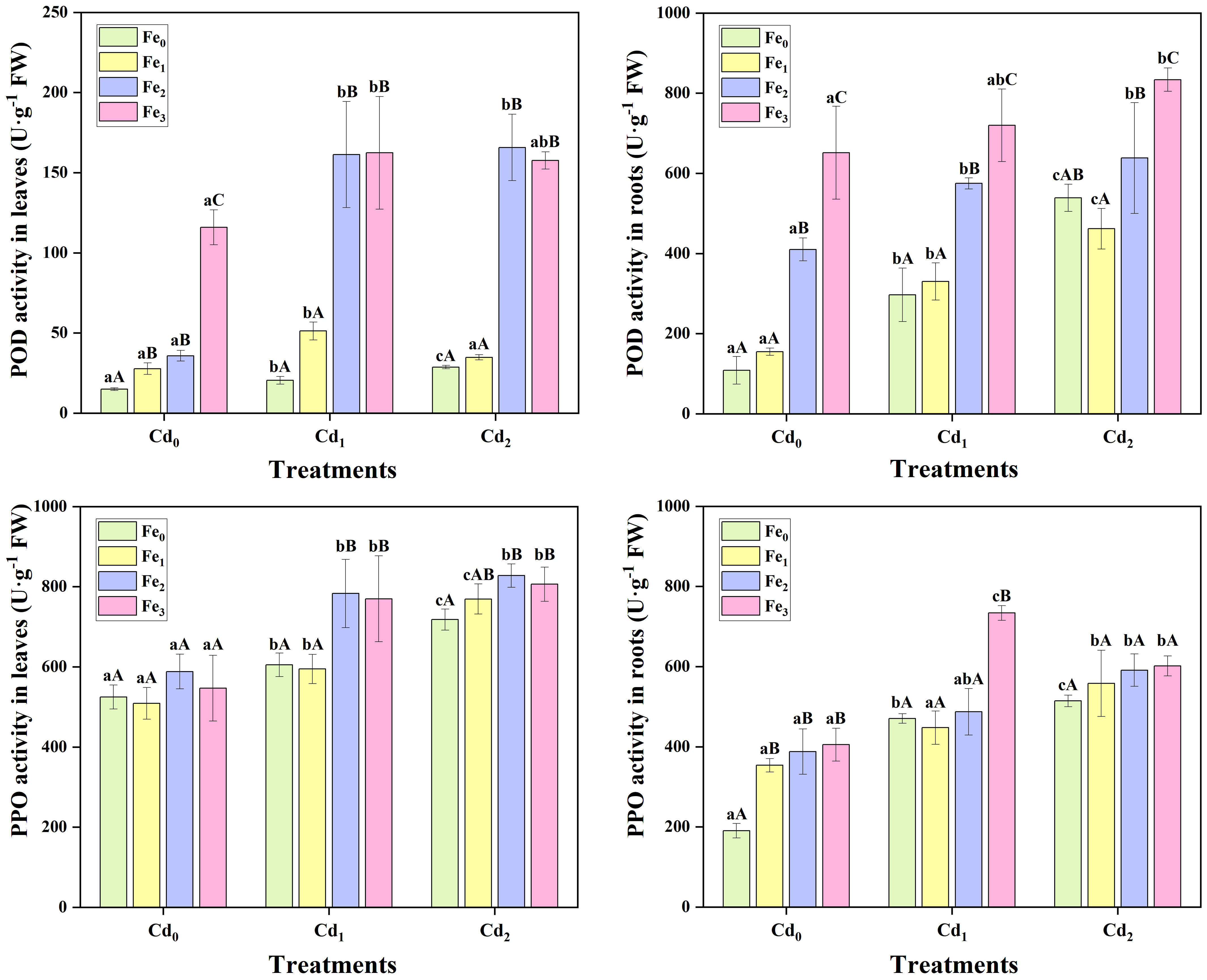
Figure 5 Peroxidase (POD) and polyphenol oxidase (PPO) activity in leaves and roots of A. marina under different Cd and Fe treatments. Different lowercase letters (a, b, and c) indicate significant differences across different Cd treatments under the same Fe treatment (P< 0.05, n = 3). Different uppercase letters (A, B, and C) indicate significant differences across different Fe treatments under the same Cd treatment (P< 0.05, n = 3).
Similar to POD activity, Cd stress caused a considerable increase in leaves (P< 0.01) and roots (P< 0.01) PPO activity. In contrast, Fe supply alone only caused higher PPO activity in roots of A. marina, while PPO activity of leaves was not significantly affected. When under Cd stress, the introduction of Fe supply generally increased the activity of PPO in leaves, while PPO activity in leaves was not significantly affected, except for some increases in Cd1Fe3 treated plants. The highest PPO activities in leaves and roots were obtained in Cd2Fe2 (827.72 ± 29.12 U g-1 FW) and Cd1Fe3 (733.88 ± 18.29 U g-1 FW) treatments, respectively.
3.4 Effects of Fe and Cd on total phenolics and total tannin concentrations
Cd1 treatment alone did not result in a considerable difference in total phenolics in leaves and roots, while Cd2 treatment significantly increased total phenolics in different tissues (Figure 6). In contrast, Fe1 supply alone significantly (P< 0.05) increased total phenolics in leaves, while no further increase in total phenolics concentration was evident at higher Fe2 or Fe3 supply. As for roots, only Fe2 and Fe3 treatments generally increased total phenolics. When under Cd1 (P< 0.05) and Cd2 (P< 0.01) stress, the application of Fe1 treatment significantly increased total phenolics in leaves of A. marina, and higher total phenolics concentrations were observed under Fe2 supply. However, compared with Cd1Fe2 and Cd2Fe2, total phenolics in Cd1Fe3 and Cd2Fe3 treated plants significantly (P< 0.05) decreased. The total phenolics concentration in leaves was highest in Cd2 combined with Fe2 treatment (77.81 ± 1.51 mg g-1 DW, Figure 6), which accounts for 128.99% of Cd2Fe0, 116.00% of Cd2Fe1, or 108.31% of Cd2Fe3. Additionally, under Cd1 stress, Fe1 supply did not significantly affect roots total phenolics, while a higher Fe2 (P< 0.05) or Fe3 (P< 0.05) supply significantly increased the concentration. When the level of Cd stress further increased, total phenolics concentrations in roots were not significantly affected by different Fe treatments.
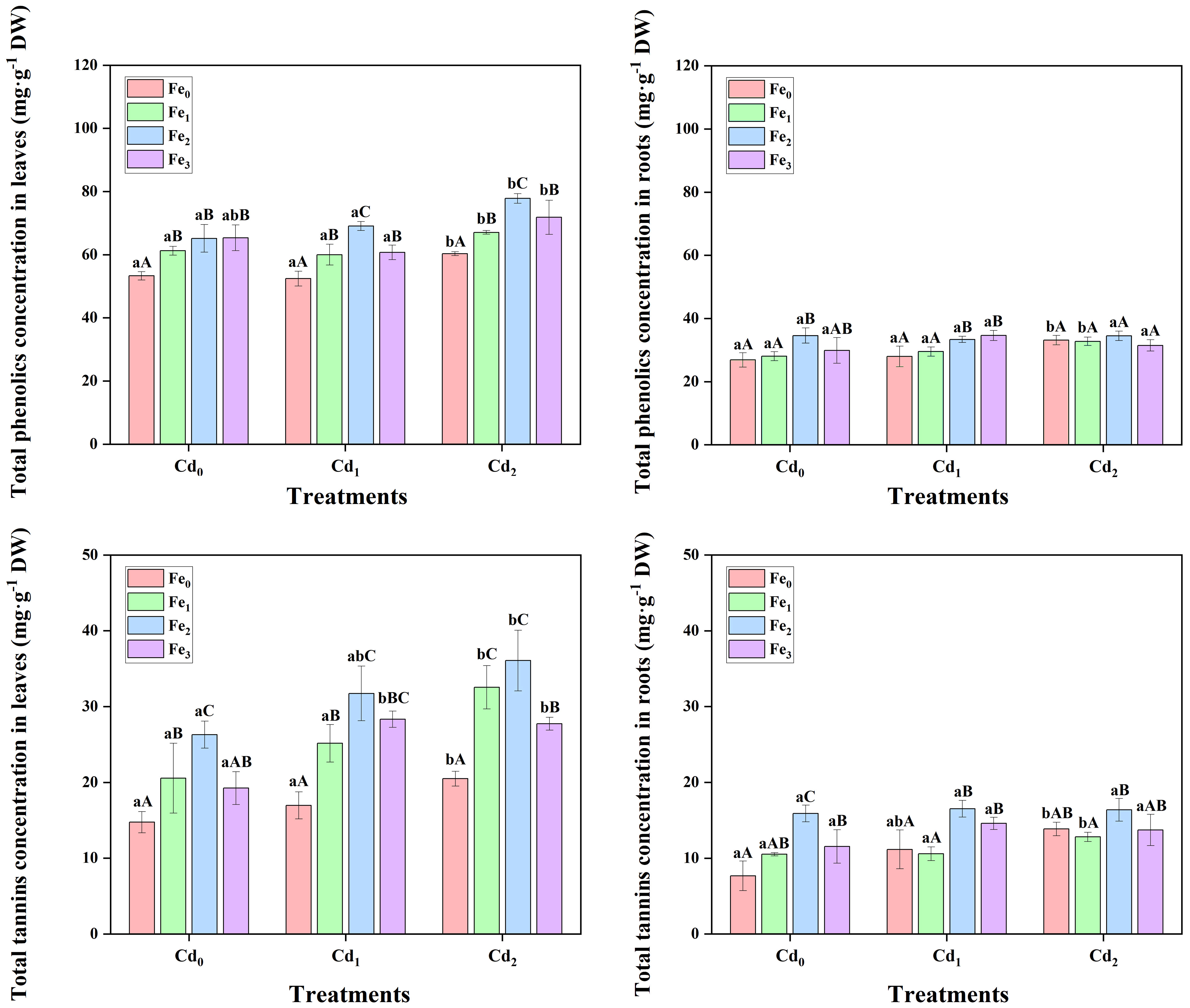
Figure 6 Total phenolics and total tannin concentrations in leaves and roots of A. marina under different Cd and Fe treatments. Different lowercase letters (a and b) indicate significant differences across different Cd treatments under the same Fe treatment (P< 0.05, n = 3). Different uppercase letters (A, B, and C) indicate significant differences across different Fe treatments under the same Cd treatment (P< 0.05, n = 3).
Cd1 stress alone did not affect total tannin in both leaves and roots, whereas Cd2 stress resulted in significantly higher total tannin concentrations (Figure 6). Interestingly, with the application of Fe1 or Fe2 supply alone, total tannin in tissues exhibited a general increasing trend (Figure 6), but with a higher Fe3 supply, total tannin in leaves and roots decreased remarkably (P< 0.05, n = 3). Similarly, in leaves treated with Cd, the application of Fe1 or Fe2 supply led to a general upward trend in total tannin concentration (Figure 6), whereas Fe3 supply caused either similar or lower total tannin concentration compared with Fe2 treated plants. For example, the total tannin concentration in Cd2Fe0 treated leaves was 20.49 ± 0.97 mg g-1 DW, with the application of Fe1 and Fe2 supply, the concentration increased to 32.54 ± 2.86 mg g-1 DW, and 36.07 ± 4.01 mg g-1 DW, respectively, while in Cd1Fe3 the concentration was only 27.74 ± 0.85 mg g-1 DW. In roots, Fe1 supply combined with different Cd stress did not affect the total tannin concentration. In contrast, a higher Fe2 or Fe3 supply generally increased total tannin concentration in roots under Cd stress (Figure 6).
3.5 Correlation analysis
As shown in Figure 3, a significant negative correlation was found between Cd treatment levels and chlorophyll concentration in leaves, while positive correlations were found between Cd treatments and POD activity in leaves, PPO activity in all tissues, and phenolic compounds (both total phenolics and tannins) in different tissues. Moreover, positive correlations were also observed between Fe treatment levels and FCR activity, POD activity, and phenolic compounds in both roots and leaves, whereas a negative correlation was observed between Fe treatment levels and TF R to S.
4 Discussion
4.1 Fe supply alleviated biomass reduction and photosynthesis inhibition caused by Cd stress
Numerous studies have shown that heavy metals such as Cd could result in considerable growth retardation and biomass reduction in different wetland plants (Guangqiu et al., 2007; Dai et al., 2018; Jian et al., 2019). In the present study, Cd2 treatment alone resulted in significant biomass reduction (Table 2), while the addition of Cd1 treatment alone did not significantly affect the biomass of A. marina due to the heavy metal tolerance and self-detoxification mechanisms, and Cd1 treatment did not reach the threshold of causing biotoxicity (Wu et al., 2022a). In contrast, Fe is a critical nutrient element for plant growth and biomass accumulation and is involved in chlorophyll synthesis, chloroplast development, and other metabolism processes (Yoshihara et al., 2006). Therefore, plant growth and biomass accumulation of A. marina were significantly promoted by Fe supply (Table 2). Moreover, in the current study, increasing the Fe supply under Cd stress significantly alleviated biomass reduction caused by Cd toxicity, and it is particularly evident under Fe1 and Fe2 treatments (Table 2). This could be attributed to the following aspects: first, the addition of Fe directly facilitated photosynthesis and other biosynthetic processes related to nutrient assimilation and biomass accumulation (Liu et al., 2017; Sági-Kazár et al., 2021). Second, Fe mediated a series of heavy metal immobilization and detoxification processes in copes such as Oryza sativa (Liu et al., 2017) and Vigna radiata (Biyani et al., 2019) under Cd stress, reduced the accumulation of Pb in roots of Spartina alterniflora, a typical salt marsh plant (Zhang et al., 2020), and ultimately reduced heavy metal stress in plant tissues. In contrast, excess Fe may be unfavorable to plants under heavy metal stress (Jian et al., 2019). To demonstrate these aspects, the Fe-mediated chlorophyll concentration, Cd translocation, and detoxification mechanisms were further studied and discussed.
Photosynthesis is the basis of biomass accumulation and plant growth (Figure 1). However, when under Cd stress, it becomes one of the main targets of Cd toxicity (Dai et al., 2017). Cd stress could damage the ultrastructure of membranes, reduce the activity of protochlorophyllide reductase, inhibit the biosynthesis of chlorophyll, and eventually cause the disturbance of photosynthesis (Stobart et al., 1985; Benavides et al., 2005). In this study, Cd1 treatment had no significant effect on the chlorophyll concentration due to most of Cd accumulated in roots, while the accumulation of Cd in leaves did not reach the level of causing severe damage. However, a higher Cd2 treatment resulted in a significantly decreased chlorophyll concentration. Furthermore, in plant tissues, Cd competes with Fe for ion channels and enzyme active sites and leads to the disruption of intracellular metabolism (Chang et al., 2003; Liu et al., 2020). The introduction of Fe supply not only directly increased the Fe bioavailability in leaves (Figure 2E) and enhanced its competitiveness with Cd, but also restricted the translocation of Cd (Figures 2A, B), therefore restoring the synthesis of chlorophyll under Cd stress, and inducing an increase in chlorophyll concentration. On the contrary, excess Fe may not be favorable to plant metabolism and growth (Müller et al., 2017; Mehrabanjoubani et al., 2019). Thus, Fe1 and Fe2 treatments significantly increased chlorophyll concentration under different Cd treatments, whereas compared with Fe2 treated plants, Fe3 treatment resulted in a decreased chlorophyll concentration (Figure 1).
4.2 Fe supply affected the translocation of Cd and the accumulation of Fe in A. marina tissues
The present study showed that under Cd1 stress, both TF R to S and TFS to L were lower than 1 (Figures 2A, B), which indicated most Cd was accumulated in roots. In comparison, under Cd2 stress, most Cd was accumulated in stems. Nevertheless, Cd concentration in leaves was the lowest among all tissues, regardless of different treatments. This could be partly attributed to the redistribution strategy of plant tissues, which allows plants to fix most heavy metals in roots and stems to protect the sensitive leaves (Weng et al., 2012; Wu et al., 2022b). Li et al. (Li et al., 2019a) found that in Aegiceras corniculatum roots, Cd tended to accumulate in xylem and pith, which have a high affinity for Cd and restrict the further translocation of Cd from roots to other tissues. Moreover, under Cd1 and Cd2 stress, Fe supply remarkably decreased the translocation of Cd from roots to other tissues (Figures 2A, B), and negative correlations between Fe supply and TF R to S, and TF S to L were observed in A. marina (r = -0.695, P< 0.01, and r = -0.513, P< 0.05 respectively), which is comparable to previous research that showed the application of exogenous Fe strongly suppressed the translocation of Cd in different plant species (Sharma et al., 2004; Jian et al., 2019; Liu et al., 2020). These results could be interpreted from three aspects: 1) higher Fe supply significantly enhanced the formation of iron plaque on the root surface of wetland plants, especially mangroves (Liu et al., 2017; Lin et al., 2018). The iron plaque might be a “barrier” inhibiting the uptake and accumulation of heavy metals by roots and hence decrease the amount of heavy metals being translocated to other tissues (Du et al., 2013; Mei et al., 2021). 2) Fe supply convincingly promoted the uptake of Fe by plants and enhanced the accumulation of Fe in different tissues (Figures 2C–E), which can cause competitive effects on Cd uptake and translocation (Jian et al., 2019; Wu et al., 2022b). 3) the exogenous Fe supply could not only alleviate the photosynthesis inhibition caused by Cd toxicity (Figure 1) but also help maintain the stable intracellular environment via inducing the upregulating of antioxidant enzymes activities and stimulating the biosynthesis of antioxidant and chelating compounds, which include tannins and other phenolic compounds (Yan and Tam, 2011; Wang et al., 2014; Chen et al., 2019). The chelate effect mediated by tannins and other phenolic compounds play a critical role in restricting the translocation of Cd in plant tissues (Lavid et al., 2001a; Guangqiu et al., 2007; Jiang et al., 2017). Therefore, to further demonstrate the restriction and detoxification effects mediated by Fe, the Fe-induced activities of FCR and typical antioxidant enzymes, as well as the concentrations of total tannin and phenolics were determined and further discussed.
4.3 Fe supply enhanced activity of FCR and antioxidant enzymes and promoted biosynthesis of phenolic compounds
For plants that obtain Fe via the strategy I (also known as the reducing strategy) mechanism, the FCR, which reduces ferric (Fe3+) to ferrous (Fe2+), plays a critical role in Fe uptake and assimilation by roots (Jeong and Connolly, 2009; Li et al., 2019b). Then, Fe is transported from roots to aboveground tissues via xylem as Fe3+-citrate and other complexes (Brüggemann et al., 1993). Subsequently, Fe3+ can be reduced by membrane-bound FCR and utilized by leaf cells, which may have high iron requirements due to the biosynthesis of Fe-containing enzymes associated with photosynthesis (Hell and Stephan, 2003). In the present study, the addition of Fe supply promoted the activity of FCR in both roots and leaves. This may be because, in the current study, the first stage is iron plaque induction, in which the Fe supply enhanced the formation of iron plaque and stimulated the activity of FCR (Figure 4). This iron oxide/hydroxide structure can not only adsorb or co-precipitate heavy metals but also be an irrefutable Fe source for plant growth (Sebastian and Prasad, 2016). Moreover, significant positive correlations between FCR activity and Fe concentration were observed in both roots and leaves of A. marina (Figure 3), this further demonstrated the key role of FCR in the uptake of Fe. Additionally, Cd stress had different effects on FCR activity, depending mainly on stress level and plant species. For example, in the study by Bari et al. (Bari et al., 2019), under hydroponic conditions, the addition of 10 uM CdSO4 significantly increased the FCR activity in roots of O. sativa due to the Cd-caused Fe deficiency. In contrast, for Cucumis sativus, 10 uM or higher level of Cd(NO3)2 significantly decreased the FCR activity due to Cd inhibited the biosynthesis of FCR or occupied active binding sites of enzymes (Chang et al., 2003; Kovács et al., 2010). In the present study, Cd concentration in leaves under Cd1 stress was relatively low due to most Cd was immobilized in roots, these low-level Cd may stimulate the FCR activity, while Cd2 treatment caused a higher Cd accumulation and biotoxicity in leaves, and hence the FCR activity was inhibited compared to Cd1 treated leaves. In comparison, for roots, both Cd1 and Cd2 treatments negatively impacted FCR activity (Figure 4), this can be attributed to roots directly exposed to Cd solution, and the high toxicity excessed the tolerance threshold of roots and resulted in a decrease in FCR activity.
POD and PPO are typical antioxidant enzymes that respond rapidly under heavy metal stress (Yan and Tam, 2011). The introduction of Cd stress can cause the overproduction and accumulation of ROS, including hydrogen peroxide, superoxide, hydroxyl radicals, and peroxyl radicals (Jiang et al., 2017), and may trigger the antioxidation and detoxification mechanisms in plants. Thus, in this study, Cd led to a higher POD and PPO activity in different tissues compared with Cd0 treated plants (Figure 5). Additionally, Fe is a well-known cofactor of different antioxidant enzymes (Liu et al., 2020). Therefore, although under Cd stress, the moderate (Fe1 and Fe2) Fe supply could maintain the stability of antioxidative systems and scavenge the overgenerated ROS (Figure 5). However, a high or excess Fe can motivate the generation and accumulation of ROS and stimulate a dramatic increase in POD activity (Liu et al., 2017). Similar results were obtained in the present study that Fe3 supply resulted in a several-fold increase in POD activity in both leaves and roots of A. marina (Figure 5). Besides, the addition of Cd resulted in a significantly higher PPO activity (Figure 5), which can also be attributed to the fact that PPO is associated with phenolic metabolism in different plants, hence further affecting the fate of heavy metals in plant tissues. For example, PPO was involved in the polymerization of phenol that may trap and immobilize Cd by forming Cd-Ca crystals and restricting it in special epidermal structures (Lavid et al., 2001a; Lavid et al., 2001b).
In plant tissues, phenolic compounds, especially simple phenols and tannins that present within symplast and apoplast, can efficiently scavenge ROS to protect the vulnerable membrane structures and maintain photosynthesis, biosynthesis of proteins, and other metabolisms (Wang et al., 2014; Jiang et al., 2017). Numerous groups of phenolic compounds are united by a common path of biosynthesis, and therefore their content varies interconnected. Changes in the metabolism of phenols are the most important mechanism for plant adaptation to different stresses (Naikoo et al., 2019; Sharma et al., 2019). In mangrove plants like Aegiceras corniculatum, total phenolics and tannins concentration markedly increased with increasing Cd stress indicating that phenolic compounds play an important role in responding to Cd stress and act as antioxidants (Guangqiu et al., 2007). This was consistent with the current study that Cd stress generally increased the concentration of total phenolics and tannins in A. marina (Figure 6). Simultaneously, correlations between Cd level and phenolic compounds also suggested the effectiveness of phenolic compounds in the tolerance and detoxification of plants under Cd stress (Figure 3). More importantly, tannins and other phenolic compounds have a strong ability to chelate heavy metals, which have profound effects on the bioavailability, translocation and accumulation of Cd (Wang et al., 2014). Lavid et al. (Lavid et al., 2001b) showed that soluble phenols and tannins can chelate heavy metal ions with hydroxyl and carboxyl function groups. Similarly, Jiang et al. (Jiang et al., 2017) evidenced that phenolic compounds mediated the fate of Cd in both symplast and apoplast. Phenolic compounds negatively affect the translocation of Cd through adsorption, chelation, etc., and transfer those complexes to the “inactive” cell walls and vacuoles to reduce the biotoxicity and restrict Cd translocation from roots to leaves. In this study, Cd1 stress did not significantly impact the concentration of total phenolics and tannins, while Cd2 caused a significant increase in phenolic compounds of different tissues (Figure 6). This may be due to the accumulation of Cd under higher Cd stress reaching the threshold of inducing the response of phenolic metabolism-associated tolerance and detoxification systems. Similarly, in K. obovata, antioxidant enzymes were reported to be more sensitive and respond more rapidly than phenolic compounds (Yan and Tam, 2011). Notably, the increase of phenolic compounds in different tissues under Fe1 and Fe2 supply can be attributed to the moderate Fe supply promoted photosynthesis in which plants assimilate inorganic carbons and further enhanced the biosynthesis of phenolic compounds (Figures 1, 6). However, relative to Fe2, a higher Fe3 supply inhibited photosynthesis (Figure 1), thus resulting in an equal or lower phenolic compounds concentration in different tissues (Figure 6), again demonstrating that excess Fe supply could also be a stress factor. Interestingly, under Cd2 stress, total phenolics in roots were not significantly affected by different Fe treatments (Figure 6), this is probably because the synthesis of phenolic compounds in roots relied mainly on carbon allocation from leaves to roots, and under Cd2 stress, the downward translocation of phenolic compounds and other organic matters were inhibited, due to heavy metal stress (Kosola et al., 2006).
4.4 Interaction of Fe and Cd on the growth of A. marina
There have been studies on Fe supply alleviating phytotoxicity induced by Cd stress in different plants (mainly on terrestrial plants and corps, Table 1). The mechanisms include promoting photosynthesis, regulating antioxidant systems, and directly or indirectly affecting the uptake and transportation of metal ions, thus maintaining intracellular homeostasis and plant growth under Cd stress. However, there are few similar studies on intertidal zone ecosystems and coastal wetland plants, particularly mangroves, and focus on the activities of FCR, which play an unreplaceable role in Fe uptake by strategy I plant species. Intertidal zones are critical transition areas between the terrestrial and marine ecosystems. Unlike the “relatively stable” terrestrial habitats, numerous unique biogeochemical cycles of different nutrient elements and heavy metals occur or operate at different levels in the intertidal zone, a “crossroads” for the flow and exchange of materials (Zhou et al., 2011; Thomas et al., 2014; Jian et al., 2017b; Hong et al., 2021b). This could be attributed to the alternate cycles between aerobic and anaerobic conditions in sediments caused by intermittent tidal inundation (Wu et al., 2022b). In addition to controlling the mineralization of organic matter and the speciation, transformation, and migration of heavy metals, the natural tidal also deeply affected the speciation and bioavailability of Fe and contributed to the limitation of bioavailable Fe in the intertidal zone (Alongi, 2010). Simultaneously, as a prominent form of permanent pollutants, heavy metals are one of the notable threats to mangroves that connect and mediate a range of biotic and abiotic processes in intertidal habitats (Gao et al., 2016; Steinmuller et al., 2020; Wu et al., 2022b). Furthermore, the limitation of bioavailable Fe in sediments may further exacerbate the threat of heavy metal contamination to mangroves (Jian et al., 2019). The current study suggested that in the Fe-limited intertidal zones, for mangrove plants, in addition to mediating and regulating plants’ tolerance and detoxification mechanisms through the above pathways, Fe supply also regulates phenolic metabolism that played an irreplaceable role in fixing heavy metals and antioxidation (Yan and Tam, 2011; Wang et al., 2014). Simultaneously, the Cd translocation factor tended to decrease with increasing phenolic compound concentrations in different tissues. Therefore, Fe-regulated phenolic metabolism and other mechanisms are key factors in decelerating the translocation of Cd, decreasing damage caused by Cd toxicity, and maintaining the growth of A. marina under Cd stress. Moreover, FCR serves as the core component/mechanism of the Fe uptake by mangroves. When exposed to Cd stress, the increased FCR activity, along with the application of a moderate amount of Fe, could enhance the assimilation of Fe by plant tissues. This, in turn, enhances the detoxification mechanisms mentioned above, alleviates Cd toxicity, and ultimately improves the growth of A. marina. Thus, this process may establish “positive feedback” and emphasize the important role of FCR in the uptake of Fe by plants.
5 Conclusion
This work studied the response of A. marina to Fe and Cd interactions from the perspective of Cd translocation and physiological mechanisms. Results revealed that a certain dose of Fe supply (0.6 - 1.8 mmol L-1 Fe in the current study) could restrict the translocation and promote the detoxification of Cd, hence alleviating the impact of Cd stress on A. marina growth. Meanwhile, the most significant immobilization and detoxification effects were observed under a 1.2 mmol L-1 Fe supply. The mechanisms can be separated into three aspects. First, moderate Fe supply directly improved the Fe bioavailability and inhibited the uptake of Cd by plant roots. When under Cd stress, a moderate Fe supply could significantly improve the accumulation of Fe in different tissues and decrease the TF R to S and TF S to L of Cd by up to 90.76% and 81.52%, from 1.667 ± 0.373 (Cd2Fe0) to 0.154 ± 0.006 (Cd2Fe3) and 0.671 ± 0.054 (Cd1Fe0) to 0.124 ± 0.016 (Cd1Fe3) respectively. Second, Fe supply increased chlorophyll concentration in leaves and enhanced photosynthesis, thereby further facilitating nutrient assimilation and plant growth under Cd stress. Subsequently, the organic matter-depends phenolic metabolism was upregulated, and phenolic compounds, including tannins, were synthesized. Fe supply resulted in the synthesis and accumulation of up to 123.67% of total phenolics and 130.73% of tannins in roots, while 119.10% and 166.97% for leaves. In plant tissues, phenolic compounds directly participated in the chelation and immobilization of Cd, decreased the biotoxicity, and restricted the translocation of Cd. Meanwhile, phenolic compounds can also act as antioxidants to scavenge ROS and maintain intracellular homeostasis. Finally, the Fe supply upregulated the activity of antioxidant enzymes and FCR in both roots and leaves of A. marina, which further enhanced plant tolerance to Cd, improved Fe bioavailability, and alleviated Cd toxicity and competition between Cd and Fe. In summary, a moderate Fe supply enhanced Cd tolerance and improved the growth of A. marina under Cd stress. To reduce mangrove degradation caused by heavy metal contamination and maintain the natural carbon sink in coastal wetlands (mangrove blue carbon), improving Fe bioavailability appears to be one of the optional approaches.
Data availability statement
The raw data supporting the conclusions of this article will be made available by the authors, without undue reservation.
Author contributions
JL: Conceptualization, Data curation, Funding acquisition, Project administration, Supervision, Writing – original draft, Writing – review & editing. YW: Conceptualization, Data curation, Investigation, Methodology, Writing – original draft, Writing – review & editing. XG: Writing – review & editing. SJ: Writing – review & editing. ZL: Conceptualization, Investigation, Writing – review & editing. JX: Writing – review & editing. CZ: Writing – review & editing. HJ: Writing – review & editing. DD: Writing – review & editing.
Funding
The author(s) declare financial support was received for the research, authorship, and/or publication of this article. This work was supported by the Open Research Fund of State Key Laboratory of Estuarine and Coastal Research (Grant number SKLEC-KF202306), National Natural Science Foundation of China (Grant Nos. 31800429), the Natural Science Foundation of Jiangsu Province (Grant Nos. BK20210751), Carbon Peak and Carbon Neutrality Technology Innovation Special Foundation of Jiangsu Province (BK20220030), and Jiangsu Collaborative Innovation Center of Technology and Material of Water Treatment, China.
Conflict of interest
The authors declare that the research was conducted in the absence of any commercial or financial relationships that could be construed as a potential conflict of interest.
Publisher’s note
All claims expressed in this article are solely those of the authors and do not necessarily represent those of their affiliated organizations, or those of the publisher, the editors and the reviewers. Any product that may be evaluated in this article, or claim that may be made by its manufacturer, is not guaranteed or endorsed by the publisher.
References
Adrees M., Khan Z. S., Ali S., Hafeez M., Khalid S., ur Rehman M. Z., et al. (2020). Simultaneous mitigation of cadmium and drought stress in wheat by soil application of iron nanoparticles. Chemosphere 238, 124681. doi: 10.1016/j.chemosphere.2019.124681
Alongi D. M. (2010). Dissolved iron supply limits early growth of estuarine mangroves. Ecology 91, 3229–3241. doi: 10.1890/09-2142.1
Bari M. A., Akther M. S., Reza M. A., Kabir A. H. (2019). Cadmium tolerance is associated with the root-driven coordination of cadmium sequestration, iron regulation, and ROS scavenging in rice. Plant Physiol. Biochem. 136, 22–33. doi: 10.1016/j.plaphy.2019.01.007
Benavides M. P., Gallego S. M., Tomaro M. L. (2005). Cadmium toxicity in plants. Braz. J. Plant Physiol. 17, 21–34. doi: 10.1590/S1677-04202005000100003
Biyani K., Tripathi D. K., Lee J. H., Muneer S. (2019). Dynamic role of iron supply in amelioration of cadmium stress by modulating antioxidative pathways and peroxidase enzymes in mungbean. AoB Plants 11, plz005. doi: 10.1093/aobpla/plz005
Brüggemann W., Maas-Kantel K., Moog P. R. (1993). Iron uptake by leaf mesophyll cells: the role of the plasma membrane-bound ferric-chelate reductase. Planta 190, 151–155. doi: 10.1007/BF00196606
Chang Y.-C., Zouari M., Gogorcena Y., Lucena J. J., Abadía J. (2003). Effects of cadmium and lead on ferric chelate reductase activities in sugar beet roots. Plant Physiol. Biochem. 41, 999–1005. doi: 10.1016/j.plaphy.2003.07.007
Chen S., Wang Q., Lu H., Li J., Yang D., Liu J., et al. (2019). Phenolic metabolism and related heavy metal tolerance mechanism in Kandelia Obovata under Cd and Zn stress. Ecotoxicology Environ. Saf. 169, 134–143. doi: 10.1016/j.ecoenv.2018.11.004
Dai M., Liu W., Hong H., Lu H., Liu J., Jia H., et al. (2018). Exogenous phosphorus enhances cadmium tolerance by affecting cell wall polysaccharides in two mangrove seedlings Avicennia marina (Forsk.) Vierh and Kandelia obovata (S., L.) Yong differing in cadmium accumulation. Mar. pollut. Bull. 126, 86–92. doi: 10.1016/j.marpolbul.2017.10.083
Dai M., Lu H., Liu W., Jia H., Hong H., Liu J., et al. (2017). Phosphorus mediation of cadmium stress in two mangrove seedlings Avicennia marina and Kandelia obovata differing in cadmium accumulation. Ecotoxicology Environ. Saf. 139, 272–279. doi: 10.1016/j.ecoenv.2017.01.017
Dörnenburg H., Knorr D. (1997). Evaluation of elicitor- and high-pressure-induced enzymatic browning utilizing potato (Solanum tuberosum) suspension cultures as a model system for plant tissues. J. Agric. Food Chem. 45, 4173–4177. doi: 10.1021/jf9701603
Du J., Yan C., Li Z. (2013). Formation of iron plaque on mangrove Kandalar. Obovata (SL) root surfaces and its role in cadmium uptake and translocation. Mar. pollut. Bull. 74, 105–109. doi: 10.1016/j.marpolbul.2013.07.023
Elazab D. S., Abdel-Wahab D. A., El-Mahdy M. T. (2021). Iron and zinc supplies mitigate cadmium toxicity in micropropagated banana (Musa spp.). Plant Cell Tissue Organ Culture (PCTOC) 145, 367–377. doi: 10.1007/s11240-021-02013-6
Gao W., Du Y., Gao S., Ingels J., Wang D. (2016). Heavy metal accumulation reflecting natural sedimentary processes and anthropogenic activities in two contrasting coastal wetland ecosystems, eastern China. J. Soils Sediments 16, 1093–1108. doi: 10.1007/s11368-015-1314-0
Gao C., Wang Y., Xiao D.-S., Qiu C.-P., Han D.-G., Zhang X.-Z., et al. (2011). Comparison of cadmium-induced iron-deficiency responses and genuine iron-deficiency responses in Malus xiaojinensis. Plant Sci. 181, 269–274. doi: 10.1016/j.plantsci.2011.05.014
Guangqiu Q., Chongling Y., Haoliang L. (2007). Influence of heavy metals on the carbohydrate and phenolics in mangrove, Aegiceras corniculatum L., seedlings. Bull. Environ. contamination Toxicol. 78, 440–444. doi: 10.1007/s00128-007-9204-9
Hamilton S. E., Casey D. (2016). Creation of a high spatio-temporal resolution global database of continuous mangrove forest cover for the 21st century (CGMFC-21). Global Ecol. Biogeography 25, 729–738. doi: 10.1111/geb.12449
Hell R., Stephan U. W. (2003). Iron uptake, trafficking and homeostasis in plants. Planta 216, 541–551. doi: 10.1007/s00425-002-0920-4
Hong H., Wu S., Wang Q., Qian L., Lu H., Liu J., et al. (2021a). Trace metal pollution risk assessment in urban mangrove patches: Potential linkage with the spectral characteristics of chromophoric dissolved organic matter. Environ. pollut. 272, 115996. doi: 10.1016/j.envpol.2020.115996
Hong H., Zhang B., Lu H. (2021b). Seasonal variation and ecological risk assessment of heavy metal in an estuarine mangrove wetland. Water 13, 2064. doi: 10.3390/w13152064
Jennerjahn T. C. (2020). Relevance and magnitude of ‘Blue Carbon’ storage in mangrove sediments: Carbon accumulation rates vs. stocks, sources vs. sinks. Estuarine Coast. Shelf Sci. 247, 107027. doi: 10.1016/j.ecss.2020.107027
Jeong J., Connolly E. L. (2009). Iron uptake mechanisms in plants: functions of the FRO family of ferric reductases. Plant Sci. 176, 709–714. doi: 10.1016/j.plantsci.2009.02.011
Jian L., Chongling Y., Daolin D., Haoliang L., Jingchun L. (2017a). Accumulation and speciation of Cd in Avicennia marina tissues. Int. J. Phytoremediation 19, 1000–1006. doi: 10.1080/15226514.2017.1303817
Jian L., Jingchun L., Chongling Y., Daolin D., Haoliang L. (2019). The alleviation effect of iron on cadmium phytotoxicity in mangrove A. marina. Alleviation effect of iron on cadmium phytotoxicity in mangrove Avicennia marina (Forsk.) Vierh. Chemosphere 226, 413–420. doi: 10.1016/j.chemosphere.2019.03.172
Jian L., Junyi Y., Jingchun L., Chongling Y., Haoliang L., Spencer K. L. (2017b). The effects of sulfur amendments on the geochemistry of sulfur, phosphorus and iron in the mangrove plant (Kandelia obovata (SL)) rhizosphere. Mar. pollut. Bull. 114, 733–741. doi: 10.1016/j.marpolbul.2016.10.070
Jiang S., Weng B., Liu T., Su Y., Liu J., Lu H., et al. (2017). Response of phenolic metabolism to cadmium and phenanthrene and its influence on pollutant translocations in the mangrove plant Aegiceras corniculatum (L.) Blanco (Ac). Ecotoxicology Environ. Saf. 141, 290–297. doi: 10.1016/j.ecoenv.2017.03.041
Jin C. W., You G. Y., He Y. F., Tang C., Wu P., Zheng S. J. (2007). Iron deficiency-induced secretion of phenolics facilitates the reutilization of root apoplastic iron in red clover. Plant Physiol. 144, 278–285. doi: 10.1104/pp.107.095794
Kosola K. R., Parry D., Workmaster B. A. A. (2006). Responses of condensed tannins in poplar roots to fertilization and gypsy moth defoliation. Tree Physiol. 26, 1607–1611. doi: 10.1093/treephys/26.12.1607
Kovács K., Kuzmann E., Vértes A., Lévai L., Cseh E., Fodor F. (2010). Effect of cadmium on iron uptake in cucumber roots: a Mössbauer-spectroscopic study. Plant Soil 327, 49–56. doi: 10.1007/s11104-009-0030-1
Lavid N., Schwartz A., Lewinsohn E., Tel-Or E. (2001a). Phenols and phenol oxidases are involved in cadmium accumulation in the water plants Nymphoides peltata (Menyanthaceae) and Nymphaeae (Nymphaeaceae). Planta 214, 189–195. doi: 10.1007/s004250100610
Lavid N., Schwartz A., Yarden O., Tel-Or E. (2001b). The involvement of polyphenols and peroxidase activities in heavy-metal accumulation by epidermal glands of the waterlily (Nymphaeaceae). Planta 212, 323–331. doi: 10.1007/s004250000400
Li J., Liu J., Lu H., Jia H., Yu J., Hong H., et al. (2016). Influence of the phenols on the biogeochemical behavior of cadmium in the mangrove sediment. Chemosphere 144, 2206–2213. doi: 10.1016/j.chemosphere.2015.10.128
Li J., Lu H., Liu J., Hong H., Yan C. (2015). The influence of flavonoid amendment on the absorption of cadmium in Avicennia marina roots. Ecotoxicology Environ. Saf. 120, 1–6. doi: 10.1016/j.ecoenv.2015.05.004
Li L., Ye L., Kong Q., Shou H. (2019b). A vacuolar membrane ferric-chelate reductase, OsFRO1, alleviates Fe toxicity in rice (Oryza sativa L.). Front. Plant Sci. 10, 700. doi: 10.3389/fpls.2019.00700
Li J., Yu J., Du D., Liu J., Lu H., Yan C. (2019a). Analysis of anatomical changes and cadmium distribution in Aegiceras corniculatum (L.) Blanco roots under cadmium stress. Mar. pollut. Bull. 149, 110536. doi: 10.1016/j.marpolbul.2019.110536
Lichtenthaler H. K., Wellburn A. R. (1983). Determinations of total carotenoids and chlorophylls a and b of leaf extracts in different solvents (Portland Press Ltd). doi: 10.1042/bst0110591
Lin Y., Fan J., Yu J., Jiang S., Yan C., Liu J. (2018). Root activities and arsenic translocation of Avicennia marina (Forsk.) Vierh seedlings influenced by sulfur and iron amendments. Mar. pollut. Bull. 135, 1174–1182. doi: 10.1016/j.marpolbul.2018.08.040
Liu H., Yang L., Li N., Zhou C., Feng H., Yang J., et al. (2020). Cadmium toxicity reduction in rice (Oryza sativa L.) through iron addition during primary reaction of photosynthesis. Ecotoxicology Environ. Saf. 200, 110746. doi: 10.1016/j.ecoenv.2020.110746
Liu H., Zhang C., Wang J., Zhou C., Feng H., Mahajan M. D., et al. (2017). Influence and interaction of iron and cadmium on photosynthesis and antioxidative enzymes in two rice cultivars. Chemosphere 171, 240–247. doi: 10.1016/j.chemosphere.2016.12.081
Makkar H. P. (2003). Quantification of tannins in tree and shrub foliage: a laboratory manual (Springer Science & Business Media). https://link.springer.com/book/10.1007/978-94-017-0273-7
Mehrabanjoubani P., Abdolzadeh A., Sadeghipour H. R., Aghdasi M., Bagherieh-Najjar M. B., Barzegargolchini B. (2019). Silicon increases cell wall thickening and lignification in rice (Oryza sativa) root tip under excess Fe nutrition. Plant Physiol. Biochem. 144, 264–273. doi: 10.1016/j.plaphy.2019.09.047
Mei K., Liu J., Fan J., Guo X., Wu J., Zhou Y., et al. (2021). Low-level arsenite boosts rhizospheric exudation of low-molecular-weight organic acids from mangrove seedlings (Avicennia marina): Arsenic phytoextraction, removal, and detoxification. Sci. Total Environ. 775, 145685. doi: 10.1016/j.scitotenv.2021.145685
Müller C., da Silveira Silveira S. F., de Menezes Daloso D., Mendes G. C., Merchant A., Kuki K. N., et al. (2017). Ecophysiological responses to excess iron in lowland and upland rice cultivars. Chemosphere 189, 123–133. doi: 10.1016/j.chemosphere.2017.09.033
Naikoo M. I., Dar M. I., Raghib F., Jaleel H., Ahmad B., Raina A., et al. (2019). Role and regulation of plants phenolics in abiotic stress tolerance: An overview. In: Plant Signaling molecules, 157–168. doi: 10.1016/B978-0-12-816451-8.00009-5
Pan K., Wang W.-X. (2012). Trace metal contamination in estuarine and coastal environments in China. Sci. total Environ. 421, 3–16. doi: 10.1016/j.scitotenv.2011.03.013
Sági-Kazár M., Zelenyánszki H., Müller B., Cseh B., Gyuris B., Farkas S. Z., et al. (2021). Supraoptimal iron nutrition of Brassica napus plants suppresses the iron uptake of chloroplasts by down-regulating chloroplast ferric chelate reductase. Front. Plant Sci. 12, 748. doi: 10.3389/fpls.2021.658987
Sebastian A., Prasad M. (2016). Iron plaque decreases cadmium accumulation in Oryza sativa L. and serves as a source of iron. Plant Biol. 18, 1008–1015. doi: 10.1111/plb.12484
Sharma S. S., Kaul S., Metwally A., Goyal K. C., Finkemeier I., Dietz K.-J. (2004). Cadmium toxicity to barley (Hordeum vulgare) as affected by varying Fe nutritional status. Plant Sci. 166, 1287–1295. doi: 10.1016/j.plantsci.2004.01.006
Sharma A., Shahzad B., Rehman A., Bhardwaj R., Landi M., Zheng B. (2019). Response of phenylpropanoid pathway and the role of polyphenols in plants under abiotic stress. Molecules 24 (13), 2452. doi: 10.3390/molecules24132452
Soto-Jiménez M., Páez-Osuna F. (2001). Distribution and normalization of heavy metal concentrations in mangrove and lagoonal sediments from Mazatlan Harbor (SE Gulf of California). Estuarine Coast. Shelf Sci. 53, 259–274. doi: 10.1006/ecss.2000.0814
Steinmuller H. E., Foster T. E., Boudreau P., Ross Hinkle C., Chambers L. G. (2020). Tipping points in the mangrove March: characterization of biogeochemical cycling along the mangrove–salt marsh ecotone. Ecosystems 23, 417–434. doi: 10.1007/s10021-019-00411-8
Stobart A. K., Griffiths W. T., Ameen-Bukhari I., Sherwood R. P. (1985). The effect of Cd2+ on the biosynthesis of chlorophyll in leaves of barley. Physiologia plantarum 63, 293–298. doi: 10.1111/j.1399-3054.1985.tb04268.x
Thomas F., Giblin A. E., Cardon Z. G., Sievert S. M. (2014). Rhizosphere heterogeneity shapes abundance and activity of sulfur-oxidizing bacteria in vegetated salt marsh sediments. Front. Microbiol. 5, 309. doi: 10.3389/fmicb.2014.00309
Wang Q., Hong H., Yang D., Li J., Chen S., Pan C., et al. (2020). Health risk assessment of heavy metal and its mitigation by glomalin-related soil protein in sediments along the South China coast. Environ. pollut. 263, 114565. doi: 10.1016/j.envpol.2020.114565
Wang Y., Zhu H., Tam N. F. Y. (2014). Polyphenols, tannins and antioxidant activities of eight true mangrove plant species in South China. Plant Soil 374, 549–563. doi: 10.1007/s11104-013-1912-9
Webb K. J., Cookson A., Allison G., Sullivan M. L., Winters A. L. (2014). Polyphenol oxidase affects normal nodule development in red clover (Trifolium pratense L.). Front. Plant Sci. 5, 700. doi: 10.3389/fpls.2014.00700
Weng B., Xie X., Weiss D. J., Liu J., Lu H., Yan C. (2012). Kandelia obovata (S., L.) Yong tolerance mechanisms to cadmium: subcellular distribution, chemical forms and thiol pools. Mar. pollut. Bull. 64, 2453–2460. doi: 10.1016/j.marpolbul.2012.07.047
Wu Y., Leng Z., Li J., Jia H., Yan C., Hong H., et al. (2022a). Increased fluctuation of sulfur alleviates cadmium toxicity and exacerbates the expansion of Spartina alterniflora in coastal wetlands. Environ. pollut. 292, 118399. doi: 10.1016/j.envpol.2021.118399
Wu Y., Leng Z., Li J., Yan C., Wang X., Jia H., et al. (2022b). Sulfur mediated heavy metal biogeochemical cycles in coastal wetlands: From sediments, rhizosphere to vegetation. Front. Environ. Sci. Eng. 16, 1–18. doi: 10.1007/s11783-022-1523-x
Wu H., Liu J., Bi X., Lin G., Feng C. C., Li Z., et al. (2017). Trace metals in sediments and benthic animals from aquaculture ponds near a mangrove wetland in Southern China. Mar. pollut. Bull. 117, 486–491. doi: 10.1016/j.marpolbul.2017.01.026
Yan Z. Z., Tam N. F. Y. (2011). Temporal changes of polyphenols and enzyme activities in seedlings of Kandelia obovata under lead and manganese stresses. Mar. pollut. Bull. 63, 438–444. doi: 10.1016/j.marpolbul.2011.04.027
Yoshihara T., Hodoshima H., Miyano Y., Shoji K., Shimada H., Goto F. (2006). Cadmium inducible Fe deficiency responses observed from macro and molecular views in tobacco plants. Plant Cell Rep. 25, 365–373. doi: 10.1007/s00299-005-0092-3
Zhang Q., Yan Z., Li X. (2020). Ferrous iron facilitates the formation of iron plaque and enhances the tolerance of Spartina alterniflora to artificial sewage stress. Mar. pollut. Bull. 157, 111379. doi: 10.1016/j.marpolbul.2020.111379
Zhou Y.-w., Peng Y.-s., Li X.-l., Chen G.-z. (2011). Accumulation and partitioning of heavy metals in mangrove rhizosphere sediments. Environ. Earth Sci. 64, 799–807. doi: 10.1007/s12665-011-0904-4
Keywords: mangrove, Avicennia marina, cadmium, iron, phenolic compounds
Citation: Li J, Wu Y, Guo X, Jiang S, Leng Z, Xia J, Zhuo C, Jia H and Du D (2023) Fe mediated alleviation effect of cadmium toxicity in mangrove Avicennia marina (Forssk.) Vierh. Front. Mar. Sci. 10:1269550. doi: 10.3389/fmars.2023.1269550
Received: 30 July 2023; Accepted: 20 November 2023;
Published: 05 December 2023.
Edited by:
Vitor H. Paiva, University of Coimbra, PortugalReviewed by:
Meilin Wu, Chinese Academy of Sciences (CAS), ChinaNatalya Vinogradova, Donetsk National Medical University, Ukraine
Copyright © 2023 Li, Wu, Guo, Jiang, Leng, Xia, Zhuo, Jia and Du. This is an open-access article distributed under the terms of the Creative Commons Attribution License (CC BY). The use, distribution or reproduction in other forums is permitted, provided the original author(s) and the copyright owner(s) are credited and that the original publication in this journal is cited, in accordance with accepted academic practice. No use, distribution or reproduction is permitted which does not comply with these terms.
*Correspondence: Jian Li, jianli@ujs.edu.cn
†These authors have contributed equally to this work