Substantial seagrass blue carbon pools in the southwestern Baltic Sea include relics of terrestrial peatlands
- Marine Evolutionary Ecology, GEOMAR Helmholtz Centre for Ocean Research Kiel, Wischhofstraße, Kiel, Germany
Seagrass meadows have a disproportionally high organic carbon (Corg) storage potential within their sediments and thus can play a role in climate change mitigation via their conservation and restoration. However, high spatial heterogeneity is observed in Corg, with wide differences seen globally, regionally, and even locally (within a seagrass meadow). Consequently, it is difficult to determine their contributions to the national remaining carbon dioxide (CO2) budget without introducing a large degree of uncertainty. To address this spatial heterogeneity, we sampled 20 locations across the German Baltic Sea to quantify Corg stocks and sources in Zostera marina seagrass-vegetated and adjacent unvegetated sediments. To predict and integrate the Corg inventory in space, we measured the physical (seawater depth, sediment grain size, current velocity at the seafloor, anthropogenic inputs) and biological (seagrass complexity) environment to determine regional and local drivers of Corg variation. Here we show that seagrass meadows in Germany constitute a significant Corg stock, storing on average 1,920 g C/m2, three times greater than meadows from other parts of the Baltic Sea, and three-fold richer than adjacent unvegetated sediments. Stocks were highly heterogenous; they differed widely between (by 22-fold) and even within (by 1.5 to 31-fold) sites. Regionally, Corg was controlled by seagrass complexity, fine sediment fraction, and seawater depth. Autochthonous material contributed to 12% of the total Corg in seagrass-vegetated sediments and the remaining 88% originated from allochthonous sources (phytoplankton and macroalgae). However, relics of terrestrial peatland material, deposited approximately 6,000 years BP during the last deglaciation, was an unexpected and significant source of Corg. Collectively, German seagrasses in the Baltic Sea are preventing 2.01 Mt of future CO2 emissions. Because Corg is dependent on high seagrass complexity, the richness of this pool may be contingent on seagrass habitat health. Disturbance of this Corg stock could act as a source of CO2 emissions. However, the high spatial heterogeneity warrant site-specific investigations to obtain accurate estimates of blue carbon, and a need to consider millennial timescale deposits of Corg beneath seagrass meadows in Germany and potentially other parts of the southwestern Baltic Sea.
Introduction
The oceans have absorbed approximately one-third of anthropogenic carbon dioxide (CO2) emissions to date (Mcleod et al., 2011), making the marine biome one of the largest carbon stores on Earth and thus an integral part of the climate change mitigation strategy. Despite having a relatively small global extent (0.5% of total ocean seafloor; Macreadie et al., 2021), coastal vegetated ecosystems, like seagrass meadows, mangrove forests, and tidal salt marshes, account for almost half of the total organic carbon (Corg) buried in marine sediments (Duarte et al., 2005; Mcleod et al., 2011; Krause-Jensen and Duarte, 2016), collectively estimated to mitigate approx. 3% of global CO2 emissions (Macreadie et al., 2021). Seagrass meadows alone have been estimated to contribute to 10% of the total buried Corg in ocean sediments (Duarte et al., 2005). Extraordinary rates of Corg accumulation and long-term storage in seagrass meadows have been attributed to high primary productivity (Hendriks et al., 2008; Duarte et al., 2013; Macreadie et al., 2014), efficient ability to capture particles from outside meadow boundaries (Kennedy et al., 2010; Miyajima et al., 2015; Oreska et al., 2018), a heavy network of roots and rhizomes that stabilize sediments and the carbon accumulated within them (Duarte et al., 2013), and formation of muddy anoxic sediments that prevent decomposition of the Corg, which can thus be stored for centuries to millenia (Fourqurean et al., 2012; Duarte et al., 2013; Greiner et al., 2016).
Seagrass meadows are found in tropical and temperate bioregions, on the coasts of all continents (except Antarctica) and thus occur within the exclusive economic zones (EEZ) of many coastal nations, including Germany (Hemminga and Duarte, 2000; Short et al., 2007). Through careful management of seagrass habitats, such nations can use this natural carbon sink as a way to sequester part of their CO2 emissions. However, high spatial heterogeneity is observed in soil Corg storage potential, with wide differences seen globally (i.e. tropical vs. temperate environments), regionally, and even locally (within a seagrass meadow) (e.g. Kennedy et al., 2010; Röhr et al., 2018; Prentice et al., 2020; Mazarrasa et al., 2021). Consequently, it is difficult to apply ecological economic approaches to provide accurate economic valuations and determine seagrass habitat contributions to the national remaining CO2 budget without introducing a large degree of uncertainty.
Regional estimates are contingent on site-specific evaluations because the mechanism involved in Corg storage is largely based on local environmental factors, such as (1) local hydrodynamic regimes (e.g. seawater depth, Lavery et al., 2013; Serrano et al., 2014; Mazarrasa et al., 2017a; decreased water motion, Prentice et al., 2019; lower wave height and exposure, Samper-Villarreal et al., 2016), (2) anthropogenic inputs (Macreadie et al., 2012; Mazarrasa et al., 2017a; Mazarrasa et al., 2017b; Ricart et al., 2020), (3) seagrass properties (e.g. species composition, Serrano et al., 2014; Serrano et al., 2019; increased seagrass complexity, Jankowska et al., 2016; Samper-Villarreal et al., 2016; Mazarrasa et al., 2018; Mazarrasa et al., 2021), and (4) sediment characteristics (e.g. Dahl et al., 2016; Röhr et al., 2016; Miyajima et al., 2017; Gullström et al., 2018).
The Baltic Sea coast of Germany is home to lush Zostera marina seagrass meadows, where seagrasses span a total area of approximately 285 km2 between 1-8 m seawater depth (Schubert and Steinhardt, 2014; Schubert et al., 2015; Schubert and Schygulla, 2016). Provided that the ambitious nutrient abatement targets of the Baltic Sea Action Plan are met, there is the potential that seagrass meadows could expand by at least 57 km2 by the year 2066 (Bobsien et al., 2021) and restoration activities could increase the existing area, leaving a large potential to gain negative emissions via restoration or improved growing conditions of these habitats.
The objectives of the present study were to (i) provide a detailed assessment of the regional (between sites) heterogeneity of blue carbon stocks along 350 km of German Baltic Sea coastline; (ii) compare Corg content between seagrass-vegetated and adjacent unvegetated sediments to understand local (within site) Corg variation; (iii) determine the source of Corg contributing to these stocks (autochthonous vs allochthonous); (iv) combine biophysical parameters such as seawater depth, sediment grain size, current velocity at the seafloor, and seagrass complexity with Corg content into a predictive model to understand regional drivers of Corg variation; (v) scale up measurements and convert to CO2 equivalent units to determine the role of seagrass conservation in the total CO2 budget of Germany.
Materials and methods
Study area
Sampling took place in 20 seagrass meadows in the western part of the Baltic Sea, along the coasts of Schleswig-Holstein (n = 17) and Mecklenburg-Vorpommern (n = 3) in northern Germany (Figure 1). The German Baltic Sea coast consists of shallow bays and fjords that experience weak water currents and low wave heights (Petterson et al., 2018). Like the whole Baltic Sea region, German coastal waters were geologically shaped by the last glacial periods (Schmölcke et al., 2006; Andrén, 2012). As a consequence of the last deglaciation, a conglomerate of differently sized stones, sand, and clay settled in the southwestern part of the Baltic Sea basin. The seabed consists of shallow sandy and muddy layers with consolidated marl underneath. A maximum water depth of 40 m is reached in the western part of the Baltic Sea. However, seagrasses here are rarely observed deeper than 8 m seawater depth (Schubert et al., 2015). The Baltic Sea is the largest brackish water basin in the world and because of the narrow Danish Straits connecting the Baltic Sea to the North Sea, low rates of water exchange are observed (residence time of 35-40 years) resulting in high eutrophication from nutrient discharge by the nine Baltic Sea nations that enclose it (HELCOM, 2018). However, eutrophication, which negatively impacts seagrass health and bathymetric range, is most pronounced in Germany, Russia and Poland (Thorsøe et al., 2022).
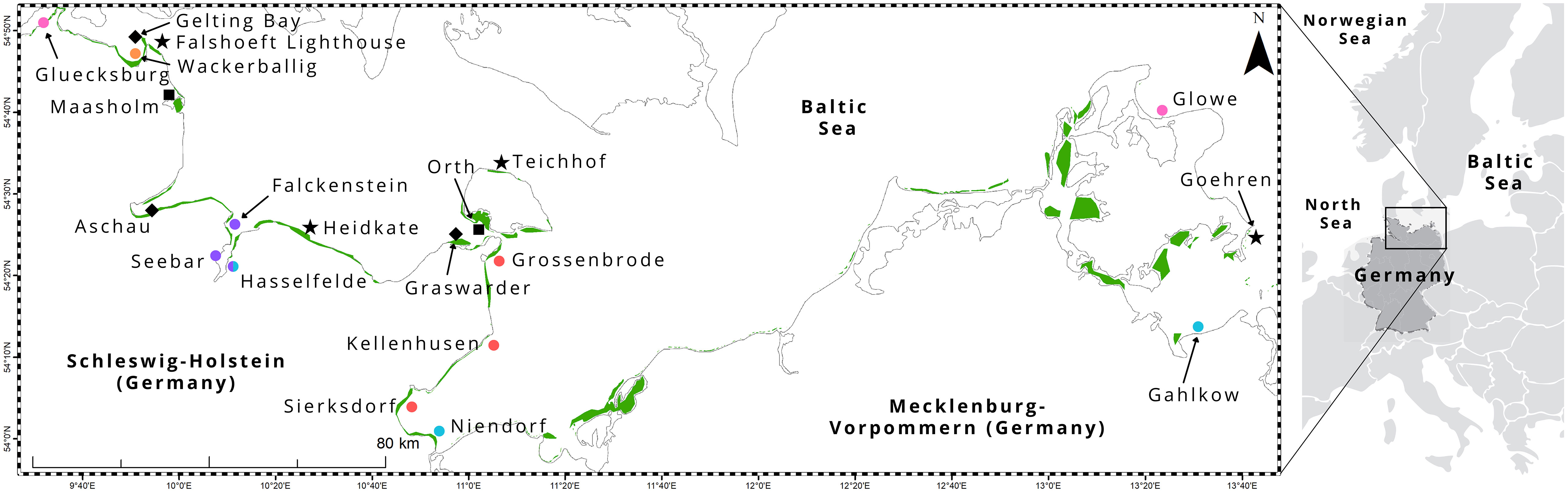
Figure 1 Study area, including 20 sampling locations, in the Baltic Sea coast of northern Germany. Seagrass area (green) was extracted from Schubert and Steinhardt (2014); Schubert et al. (2015); Schubert and Schygulla (2016). Shapes: stars - wave exposed, squares – sheltered, diamond - relatively pristine (nature reserve or some degree of protection from human impact), circle - anthropogenic inputs (pink = adjacent to a marina, purple = heavy ship traffic or near urban area, orange = agricultural land, red = tourist area, blue = proximity to river influx).
Sites in the study area represent the greatest environmental gradient, ranging from wave exposed (e.g. Heidkate, Falshoeft lighthouse, Teichhof, Goehren; Figure 1 star shape) to sheltered (e.g. Orth, Maasholm; Figure 1 square shape), relatively pristine (nature reserve e.g. Gelting Bay and Graswarder; Aschau is protected from human impact due to military controlled access to the area; Figure 1 diamond shape) to varying degrees of anthropogenic inputs (Figure 1 circle shape), such as adjacent to a marina (e.g. Gluecksburg, Glowe; Figure 1 pink circles); heavy ship traffic and near urban areas (e.g. Kiel Fjord, including Falckenstein, Seebar, Hasselfelde; Figure 1 purple circles); agricultural land (e.g. Wackerballig; Figure 1 orange circles); tourist areas (e.g. Grossenbrode, Kellenhusen, Sierksdorf; Figure 1 red circles), and proximity to river influx (e.g. Gahlkow, Hasselfelde, Niendorf; Figure 1 blue circles).
Sample collection and core subsampling
A total of 169 sediment cores (30 cm length; 5.5 cm inner diameter) were sampled in seagrass meadows (n = 110 cores) and nearby unvegetated sediments (n = 59 cores). Nine cores (with some exceptions) were collected at each site, from three sublocations: (1) in the high-density part of the meadow (‘dense seagrass’ hereafter), (2) low density or fringe of the meadow (‘sparse seagrass’ hereafter), and (3) adjacent unvegetated sediments at least 5 m from seagrass (‘unvegetated’). Dense and sparse seagrass sublocations are collectively referred to as ‘seagrass-vegetated’ sediments or sublocations. Sediment cores taken within the seagrass meadow were sampled at least 10 m apart from each other. Seawater depth was measured from a diver computer. Seagrass shoot density was counted using a 0.04 m2 frame, and the leaf lengths of five randomly selected plants were measured next to the location where the core was extracted. Seagrass density and leaf length vary between sites and at meadow stage of maturity. The measure of “seagrass complexity” was defined as the product of seagrass canopy height and shoot density, to obtain the sum of leaf heights within a unit of area (in m/m2) (Prentice et al., 2019). Seagrass canopy height and shoot density exhibit seasonal variations, and so it must be noted that all sampling took place between July 15th and September 1st of 2019 or 2020, at the peak of the seagrass growing season for Z. marina in Germany, except for the samples in Gelting Bay and Falshoeft lighthouse which were collected in late November (23rd and 24th of 2020) due to permitting and accessibility issues.
Cores were collected between 1-5 m seawater depth manually via SCUBA divers (self-contained underwater breathing apparatus) pounding impact resistant PVC tubes into the sediment with a rubber mallet. Cores were capped at both ends by the divers and stored upright for transport to shore, and in a cooler thereafter for transport to the lab. Cores were stored at 0°C until further processing.
Sediments are known to shift during the coring process, so the degree of compression was measured by divers once the core was fully inserted. Compaction was calculated as the distance (in cm) from the top of the core to the sediment surface outside of the core, divided by the sample depth (cm of compression/cm core depth). A compression correction factor was calculated by dividing the length of the sample recovered by the length of core penetration (Howard et al., 2014). Cores were subsectioned into 5 cm depth intervals, and depth intervals were adjusted based on compaction.
Sediments from each depth interval were homogenized, measured for dry-bulk density and subsampled for chemical analyses (total Corg, δ13C, δ15N, 14C, each described in detail in subsections below). A total of 394 sections underwent total Corg analyses: all top (0-5 cm) layers of all cores were processed for total Corg, but the selection of subsequent core depth intervals depended on color changes throughout the core (color changes indicate potential changes in Corg content) (Nederbragt et al., 2006, and e.g. Klingler et al., 2020). If no color change was apparent, the mid (10-15 cm) and bottom sections of the core were processed (typically 20-25 cm, except where cores were shorter due to marl or difficult sediments, such as large rocks or bolder field beneath the surface of the sediment). If a color change occurred, the respective section was instead examined. It must be noted that the total Corg was measured in 0-5 cm, 10-15 cm and 15-20 cm sections of all sites, but not all cores as described above. The 5-10 cm depth interval of all cores was used for grain size analysis (described below, under ‘grain size analysis’). Visible plant material (seagrass roots and rhizomes) and infauna (lug worm Arenicola marina, and, in some cases, soft tissue of clams and mussels) were removed from the sediment prior to chemical analyses (hereafter referred to as ‘visible organic’), but all other materials (e.g. such as wood) were left in the sediment (referred to as ‘sediment organic carbon’ or ‘SOC’). Shells, were also left in the sediment after the organic tissue was removed.
Maximum orbital velocity
These values were represented as maximum wave-generated orbital velocity (MOV) at the seafloor, modelled for the year 2021. Due to the limited availability of simulated wave data, MOVs were calculated only for cores taken at the outer coast sites of Schleswig-Holstein, not for the Schlei fjord site (Maasholm) and also not for any of the sites in Mecklenburg-Vorpommern (Gahlkow, Glowe, Goehren). MOV was calculated as a function of wave height, mean wave period, mean wave length (all simulated values), and seawater depth for each site according to linear wave theory as per the procedure described in Bobsien et al. (2021). MOV averages (‘avg. MOV’) and maximum MOV (‘max MOV’) were based on MOV calculations for simulated hourly seastate values within the year 2021 (n = 365 days x 24 hours). Average maximum MOV (‘avg. max MOV’) at each site was obtained by averaging the highest MOV observed at the depth and location of each core.
Grain size distribution
The 5-10 cm depth interval of each core was processed for grain size distribution. Visible organics were first removed, then the sediments were oven dried at 60°C for a minimum of 48 hrs. Dry sieving was conducted with stainless steel Test Sieve ISO 3310-1 and sieve shaker (Fritsch Analysette 3 Spartan Pulverisette 0) set to amplitude 2 mm for 15 min. The fractions of sediment in 2 mm, 1 mm, 500 μm, 250 μm, 125 μm, 63 μm, and <63 μm (herein referred to as ‘fine sediment’ fraction) size classes were determined to the nearest 0.01 g, and the percent amount of each size class was calculated using the total sample mass obtained from the sum of each fraction.
Corg stock quantification
Sediment total Corg was determined using an Elemental Analyzer (EURO EA Elemental Analyzer). Sediments were first dried at 60°C for 48 h and then ground to a homogeneous fine powder using a mechanical agate ball mill (Fritsch Pulveisette 5) set at rotational speed 240-300 rpm for 15-20 minutes. A subsample of this homogenized sediment was acidified to remove inorganic carbon by adding 1 M HCl drop-by-drop until gas evolution ceased. Samples were observed under a dissecting microscope to ensure CO2 had fully evolved. These acidified samples were re-dried at 60°C for 48 h, ground again (with mortar and pestle), and encapsulated into silver capsules. Total organic carbon concentrations were calculated based on a linear regression. Acetanilide and sediments were used as standards to measure data accuracy and analytical uncertainty. For sections that were not measured by EA, the section carbon content was taken from the quantify produced in the layer above (with similar coloration, see core subsampling method above for further details).
Stable isotope analyses
To decipher the source material of the remaining (non-visible) organic fraction in the sediments (referred to as SOC), four sites (Falckenstein, Graswarder, Grossenbrode, Wackerballig) underwent further examination using biotracers of δ13C and δ15N. Samples were pre-treated as outlined above (see ‘Corg stock quantification’), and then combusted in an elemental analyzer system (NA 1110, Thermo) coupled to a temperature-controlled gas chromatography oven (SRI 9300, SRI Instruments), connected to an isotope ratio mass spectrometer (DeltaPlus Advantage, Thermo Fisher Scientific) as described by Hansen et al. (2009). δ15N, δ13C ratios were reported in delta notation relative to the international Air-N2 and VPDB scale following the equation:
where R = 15N/14N or 13C/12C. N2 and CO2 gases were used as reference gases and calibrated against International Atomic Energy Agency (IAEA) reference standards (N1-,N2-, NO3-) and National Institute of Standards and Technology (NBS-22 and NBS-600) compounds. To measure analytical uncertainty, acetanilide and caffeine were used as internal standards after every sixth sample to test if the analytical setup was working properly. Precision for Caffein was ± 0.13 ‰ for δ15N and ± 0.09 ‰ for δ13C; and ± 0.20 ‰ for δ15N and ± 0.27 ‰ for δ13C for Acetanilide.
A two biotracer (δ13C and δ15N), five-source Bayesian mixing model using R package (R Core Team, 2022) MixSIAR (Stock and Semmens, 2016) was used to determine the contribution of Corg to seagrass-vegetated and unvegetated sediments in Falckenstein, Grossenbrode, Graswarder, and Wackerballig. Signatures of five potential sources were compiled from previous studies in Kiel Fjord, Germany and Gulf of Gdansk, Poland, and consisted of: (1) Pilayella littoralis, a filamentous brown algae known to form thick drifting mats accumulating in seagrass meadows in Germany (Kruk-Dowgiallo, 1991; Kiirikki and Lehvo, 1997), (2) other macroalgae (e.g. Ulva intestinalis and Cladophora fracta) extracted from Table 2 in Maksymowska et al., 2000; and (3) phytoplankton (collected by seston), (4) epiphytes attached to seagrass leaves, (5) seagrass leaves (there is little difference between above- and below-ground biomass signatures, see Röhr et al., 2016) extracted from Table 1 in Mittermayr et al. (2014). Sampling location (four locations) and vegetation state (two states: seagrass-vegetated vs unvegetated) were included as random effects in the stable isotope mixing model. Vegetation state was nested within sampling location for the random structure. We specified an uninformative, generalist prior and discrimination factor of 0 as no further fractionation is expected in stored Corg (Greiner et al., 2016; Miyajima et al., 2017). Note that visible seagrass roots and rhizomes were omitted from this analysis, which may lead to a lower contribution of autochthonous organic carbon sources.
Radiocarbon dating
Large amounts of exceptionally well-preserved wood pieces were discovered in sediment cores extracted from the seagrass meadow in Sierksdorf, Luebeck Bay (Figure 2). Of those, eleven pieces from a depth of 5 to 24 cm in four sediment cores were radiocarbon dated. The samples were visually inspected under a microscope and an appropriate amount of wood material was selected for dating. A standard decontamination procedure was subsequently applied to remove carbonates and soil humic contaminants, consisting of 1% HCl, 1% NaOH at 60°C and again 1% HCl. All radiocarbon measurements were conducted at the Leibniz-Labor, using the type HVE 3MV Tandetron 4130 accelerator mass spectrometer (AMS). Following standard procedures, the 14C/12C and 13C/12C isotope ratios were simultaneously measured by AMS, compared to the CO2 measurement standards (oxalic acid II), and corrected for effects of exposure to foreign carbon during the sample pretreatment. The resulting 14C-content was corrected for isotope fractionation, related to the hypothetical atmospheric value of 1950, and reported in pMC (percent Modern Carbon). This value was used to calculate the radiocarbon age according to Stuiver and Polach (1977). The reported uncertainty of the 14C result takes into account the uncertainty of the measured 14C/12C ratios of sample and measurement standard, as well as the uncertainty of the fractionation correction and the uncertainty of the applied blank correction. The radiocarbon ages were translated to calendar ages using the software package OxCal4 (Ramsey and Lee, 2013) and the Intcal20 dataset (Reimer et al., 2020). Note that this is not an observation unique to Sierksdorf as our diver and drop camera surveys showcase old wood material that has become visible on the fringes of seagrass meadows where erosion has occurred: in other parts of Sierksdorf that were not cored in this study, Neustadt, Fehmarnsund, Kellenhusen, Heidkate, wendtorf, Dollerupholz (Table 1).
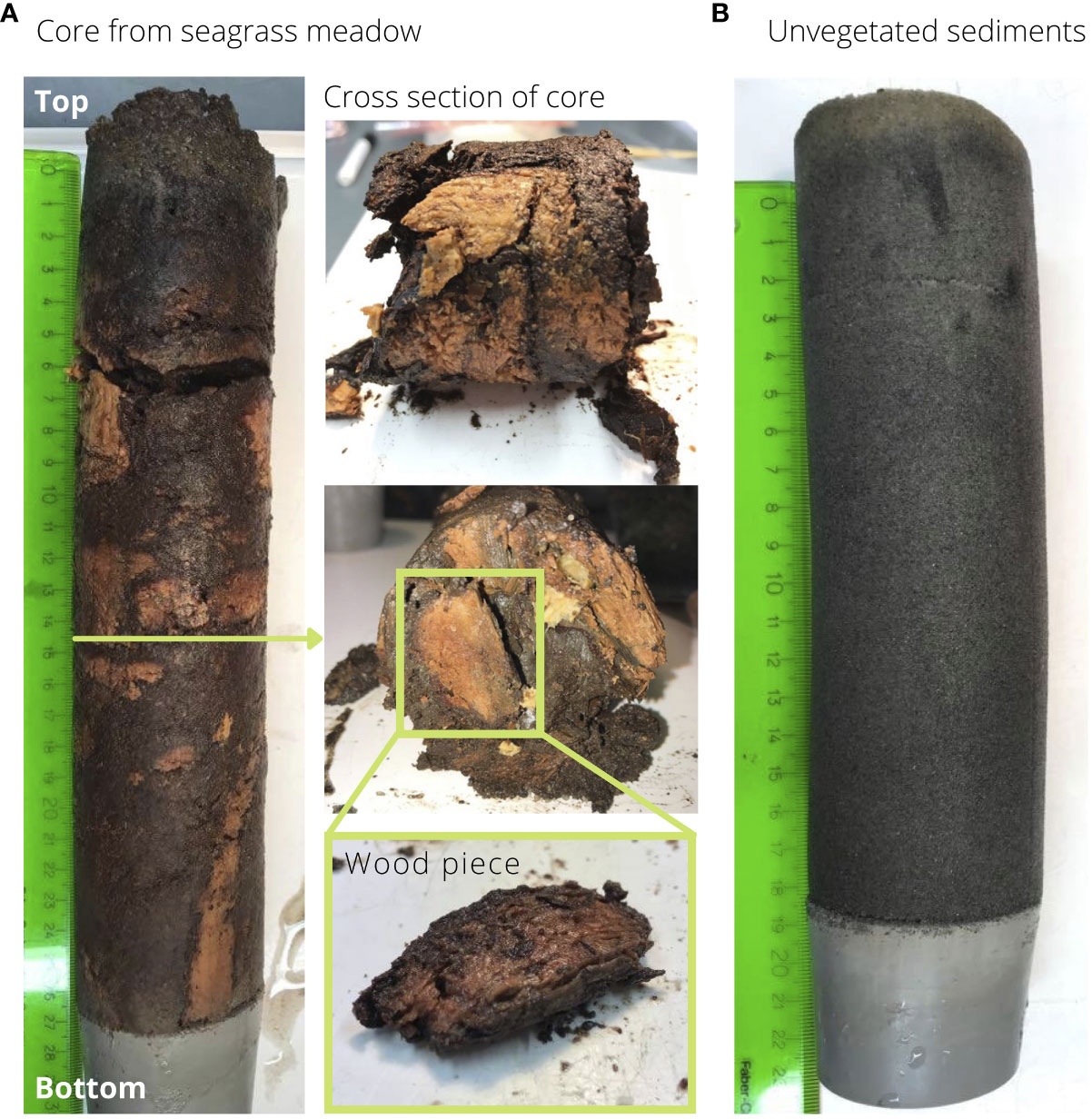
Figure 2 Sediment cores from Zostera marina seagrass-vegetated (A) and adjacent unvegetated (B) sublocations in Sierksdorf, Luebeck Bay, where unexpected large amounts of exceptionally well-preserved wood pieces were discovered.
Statistical analyses
Statistical analyses were performed in R version 4.1.3 (R Core Team, 2022) for Mac OS X, and all mixed models were implemented with package “lme4” (Bates et al., 2015). For all tests, significance was determined at p < 0.05. Visual inspection of standard model validation graphs was used to verify model assumptions: residuals versus fitted values were used to verify homogeneity; a histogram or Quantile–Quantile (q–q) plot of the residuals for normality; and residuals versus each explanatory variable to check independence.
Generalized linear mixed models (GLMM) with a Gamma distribution and log link function were used to test: (1) core position effect on Corg between sublocations (dense seagrass, sparse seagrass, unvegetated) within each site (locally), with a random intercept of sublocation nested in sampling location (site), and (2) biophysical factors influencing regional differences in stocks within seagrass-vegetated sediments, with sampling location as a random intercept. In the local model (1), a posthoc test using Tukey contrasts was performed with package “multcomp” to examine multiple comparisons between sublocations of each site. A multi-model inference approach based on AICc was used to determine the strongest predictors of regional Corg (2). Selection criteria AICc was used (instead of AIC) due to a small sample size (n/k < 40; n = total number of observations; k = total number of parameters in the most saturated model, including both fixed and random effects). The saturated model included four factors: seagrass complexity, average MOV, seawater depth, percent fine sediments, and their interactions. However, it is important to consider multicollinearity when interpreting model outputs as coefficient estimates (i.e. beta coefficients) and p-values become very sensitive to any small changes in the model. Multicollinearity of parameters in the dataset and among saturated model terms were examined using the Pearson correlation coefficient (ρ) and variance inflation factor (VIF). Correlation coefficients < 0.6 and VIFs approx. < 5 indicate an acceptable level of collinearity, VIFs > 10 warrant further investigation (Montgomery and Peck, 1992). Low collinearity was observed in the data itself (ρ = -0.43 to 0. 35), but structural multicollinearity (between some model terms) was observed in the saturated model (VIF 1.876 to 14.051), so all terms with VIF >10 were removed from the global model. Hence, the final, global model included four factors: seagrass complexity, average MOV, seawater depth, percent fine sediments and two-way interactions between all variables except average MOV and fine sediment fraction, and all three- and four-way interactions were excluded (excluded VIFs: 10.055 to 14.051). After model selection, statistically indistinguishable (ΔAICc <2) candidate models were averaged using package ‘MuMIn’ (Bartoń, 2022). To estimate the importance of each variable, the RVI (relative variable importance) value was computed by summing Akaike weights across all averaged models where a particular variable appeared. Model-averaged coefficients and p-values were obtained from the ‘full average’ (rather than the ‘conditional average’).
Results
Corg stocks
Averaged across all sediment cores in seagrass-vegetated sublocations, including dense and sparse seagrass sublocations (n = 110 cores total) and integrated to 25 cm core length, Corg stocks averaged at 1,920 ± 402 g C/m2, and varied 22-fold between sites, ranging from 475 ± 61 and 10,577 ± 6,178 g C/m2 (Figure 3). For seagrass-vegetated sediments, the highest average Corg stocks were found in Maasholm (Schlei Fjord) and Sierksdorf (Luebeck Bay), while the lowest values were observed in Heidkate (Kiel Fjord) and Grossenbrode (Luebeck Bay).
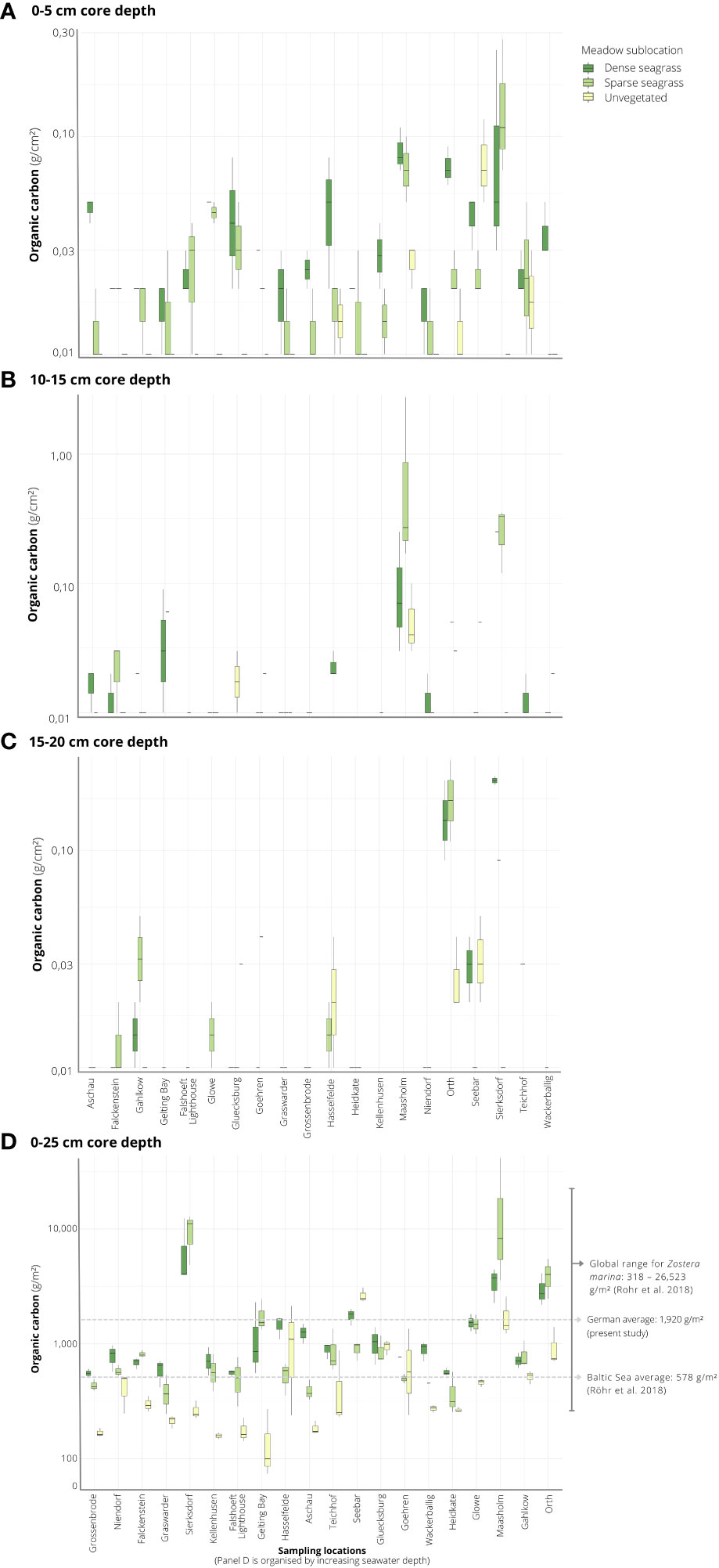
Figure 3 Overview of the organic carbon (Corg) stocks for core sections 0-5 cm (A), 10-15 cm (B), 15-20 cm (C) measured by elemental analyzer, and integrated to 25 cm sediment depth (D) across 20 sampling locations situated in the Baltic Sea coast of northern Germany. Box and whiskers show the median as a line, first and third quartiles as hinges, and the highest and lowest values within 1.5 times the inter-quartile range as whiskers.
An examination of the local (within site) variation in Corg content across all sites showed significantly greater (by three-fold) stocks in seagrass-vegetated sediments (both dense and sparse seagrass) compared to nearby unvegetated sediments (dense seagrass: average 1,523 ± 244 g C/m2; sparse seagrass: 2,316 ± 795 g C/m2; unvegetated: 611 ± 93 g C/m2) (Table 2; Figure 3). However, considering sites individually, within site comparisons ranged widely: in all but four cases (Gluecksburg, Gohren, Hasselfelde, Seebar), seagrass-vegetated sediments had 1.5 to 31 times more Corg than nearby unvegetated sediments, with Sierksdorf and Maasholm showing the greatest discrepancies (10 and 31 times that of unvegetated sediments). Gluecksburg and Hasselfelde had the same; Gohren, and Seebar less Corg than nearby unvegetated sediments. Seebar had the highest average Corg stocks, twice as much as in nearby seagrass-vegetated areas. Corg content did not differ significantly between sparse and dense seagrass sublocations.
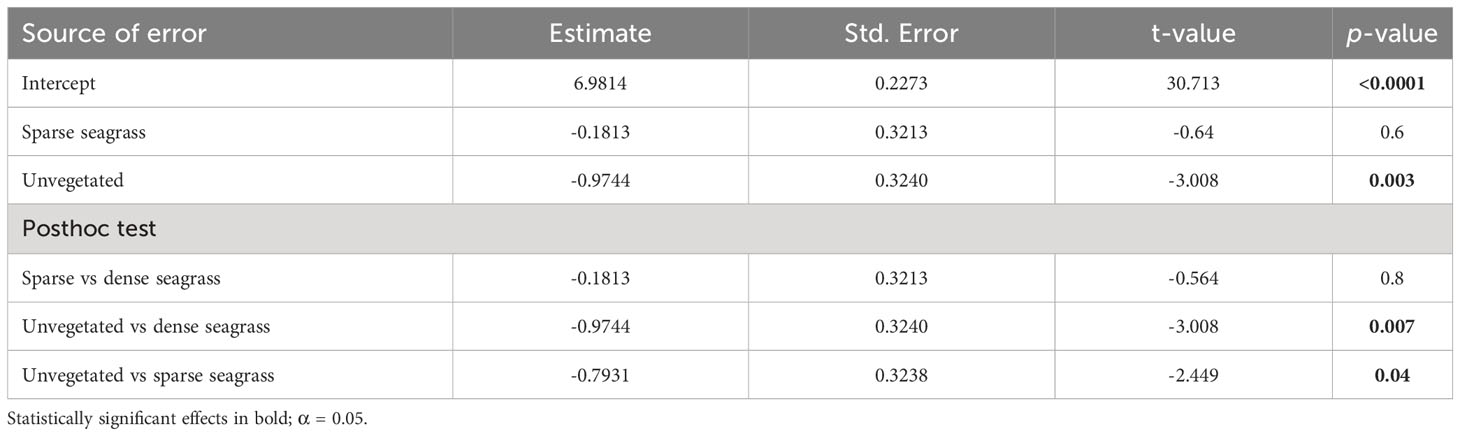
Table 2 Results of the Generalized Linear Mixed Model (Gamma, log link function), testing for the effect of sublocation on local (within site) sediment organic carbon (Corg) content of seagrass meadows in the German Baltic Sea.
Sources of sediment Corg (δ13C, δ15N, 14C)
Overall, the stable isotope signatures of the SOC fraction of seagrass-vegetated sediments (including dense and sparse seagrass sublocations along the entire core depth) were near identical to those of unvegetated sediments (δ15N 5.2 ± 0.3‰, δ13C -21.9 ± 0.2‰ vs δ15N 5.3 ± 0.2‰, δ13C -21.6 ± 0.2‰). In seagrass-vegetated sediments, signatures ranged between δ15N 4.1 ± 0.2 (Grossenbrode) to 7.3 ± 0.3 (Falckenstein), and δ13C -23.1 ± 0.4 (Falckenstein) to -19.4 ± 0.5 (Wackerballig) (Figure 4B). δ13C and δ15N signatures were also homogenous across core depth; top sediments (0 to 5 cm) averaged across all seagrass cores were 15N depleted by 0.48‰ and 13C enriched by 0.68‰ compared to the 10 to 15 cm and 15 to 20 cm sections.
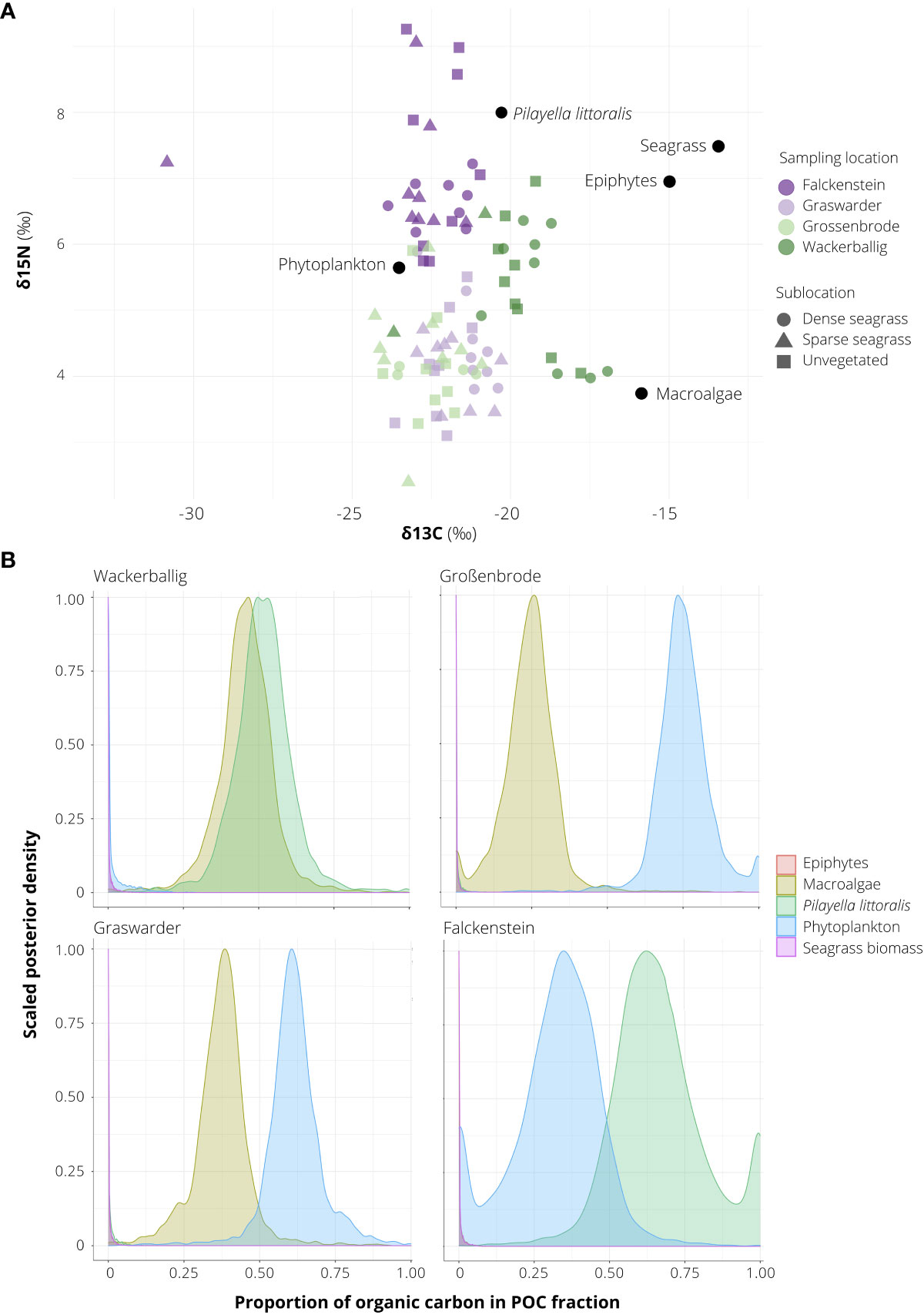
Figure 4 δ13C, δ15N stable isotope signatures (A) and posterior estimates of the proportion of organic carbon (Corg) sources (B) of sediments sampled in seagrass-vegetated and unvegetated sublocations off the coasts of Falckenstein (FS), Wackerballig (WB), Grossenbrode (GB), Graswarder (GW), in northern Germany. Sources (black circles) were obtained from Table 1 in Mittermayr et al. (2014) and Table 2 in Maksymowska et al. (2000).
Averaged across all sites, visible organic material such as invertebrates and seagrass root, shoot, rhizome material on average contributed to 12% of the average total Corg in seagrass-vegetated sediments and 9% in unvegetated sediments. Visible organics were responsible for 65% of the Corg in unvegetated sediments in Hasselfelde. Results from the five-source two-biotracer (δ13C, δ15N) mixed model of the remaining organic fraction (the non-visible fraction, called SOC) in the sediments of Falckenstein (SOC fraction = 94%), Wackerballig (SOC = 56%), Grossenbrode (SOC = 78%), Graswarder (SOC = 55%), showed that phytoplankton (24%), P. littoralis (18%) and other macroalgae (22%), made the largest contribution to Corg overall, not seagrass biomass or its epiphytes, and a combination of two of these three dominant carbon sources could be seen at each site (Figure 4A). Sampling location accounted for most of the variation in the mixing model (50th percentile σ = 6.311), while vegetation coverage (seagrass-vegetated vs unvegetated) had a negligible effect (50th percentile σ = 0.105).
Radiocarbon dating of wood pieces found in Sierksdorf cores revealed no age-depth correlation within a single core, but all dates fell in two well separated time intervals, averaging at 5,806 years BP and 5,095 years BP (before present), i.e. a hiatus of approx. 700 years between these time intervals (Table 3).
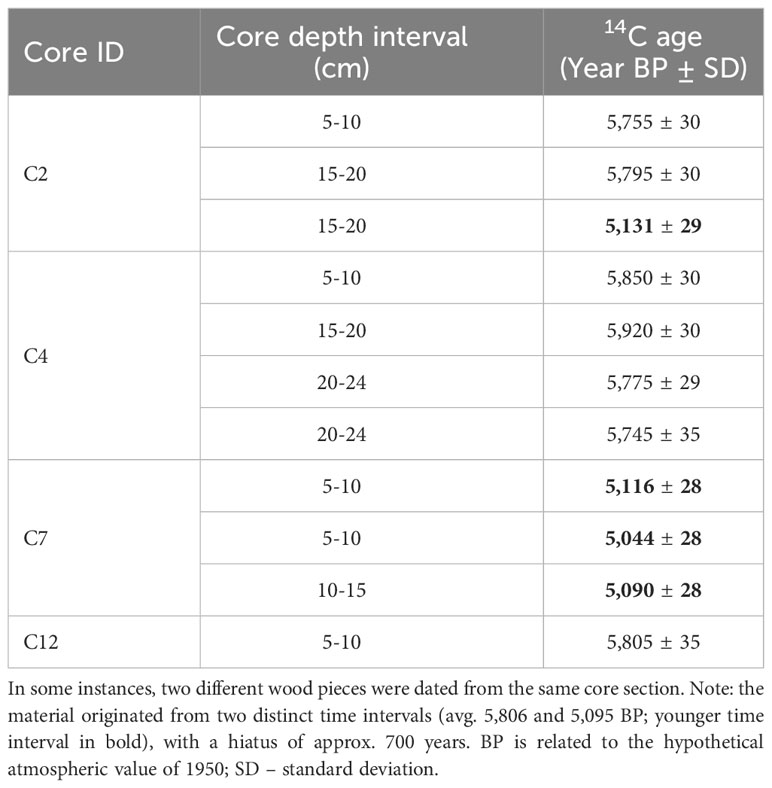
Table 3 Radiocarbon ages (in years before present, BP) of wood material collected from Zostera marina seagrass-vegetated sediment cores in Sierksdorf, Luebeck Bay, in northern Germany.
Biophysical predictors of Corg
The percent fine sediment fraction of seagrass-vegetated sediments varied greatly between sites, from 0.09 ± 0.04% (Gahlkow) to 7 ± 3% (Goehren). All unvegetated sediments and 17 of 20 seagrass-vegetated sites had a low fine sediment fraction (<3%) (Table 4). The unvegetated sediments of Orth and Seebar had the highest fine sediment fraction (2.5 and 3%), and of the seagrass-vegetated sites Glowe, Goehren, Niendorf, Orth, Sierksdorf had 2.7 to 7% fine sediments. Seagrass-vegetated sediments contained similar or more (by 1-31 times) fine-grained sediments than adjacent unvegetated sublocations, except in Graswarder, Gluecksburg, Seebar, where unvegetated sublocations contained 2-10 times more fine-grained sediments than their vegetated counterparts. For seagrass complexity, Maasholm exhibited the lowest (92 ± 21 m/m2) and Wackerballig the highest (539 ± 132 m/m2) average seagrass complexity of all sites (including sparse and dense seagrass sublocations) (Table 4). Dense seagrass sublocations were one to five times more complex than sparse seagrass sublocations, with the greatest difference observed in Teichhof and Aschau, and smallest difference seen in Sierksdorf and Grossenbrode. Average MOV varied six-fold, ranging from 0.155 ± 0.007 m/s (Niendorf) and 0.91 ± 0.03 m/s (Teichhof) between sties, but some (Falshoeft lighthouse, Heidkate, Kellenhusen, Teichhof, Wackerballig) experienced strong peak MOVs (> 3 m/s) (Table 4).
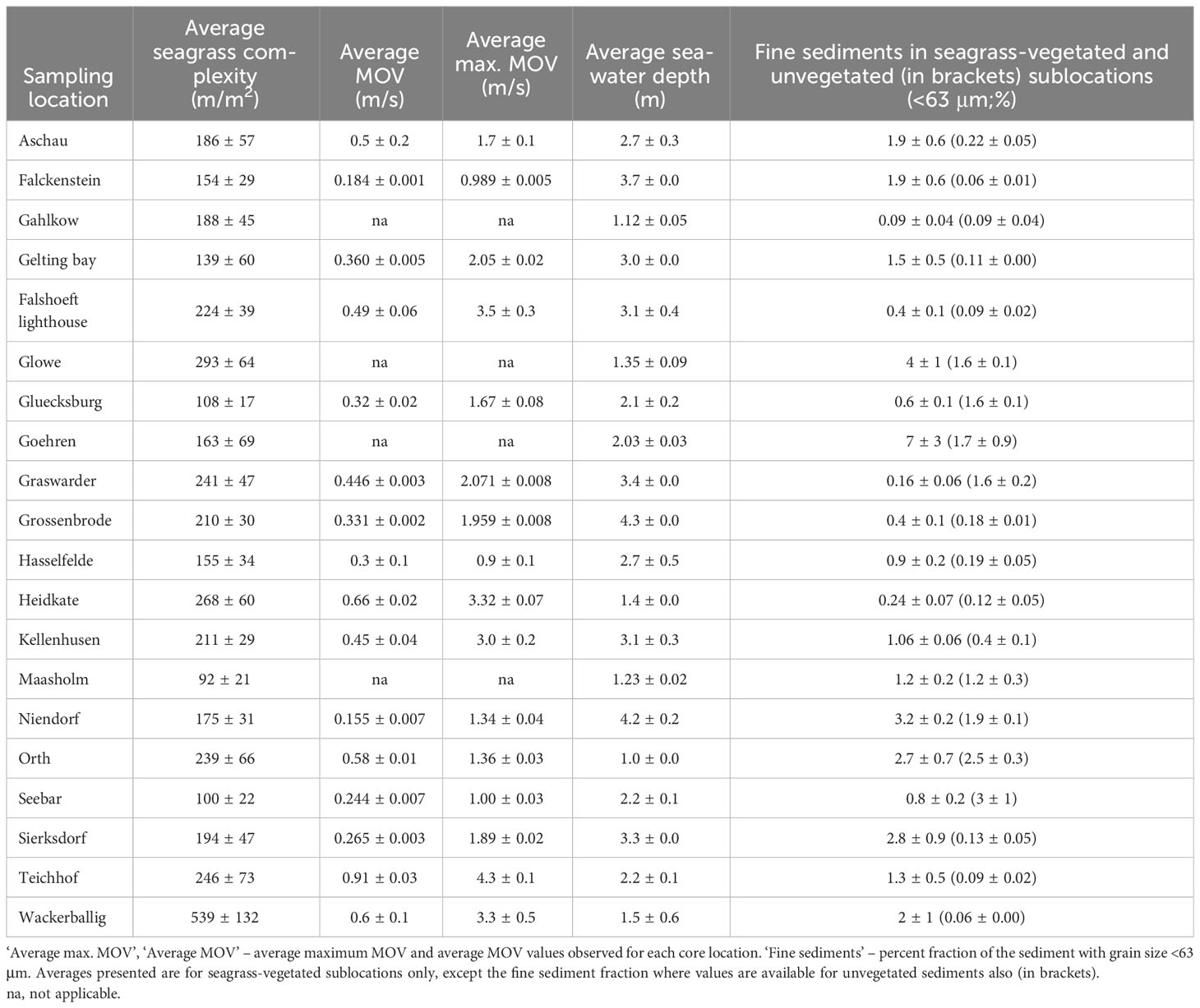
Table 4 Summary of average (± SE) of each biophysical variable calculated (seagrass complexity), modelled (average and maximum Maximum Orbital Velocity; MOV), or measured (seawater depth, % fine sediments) in seagrass-vegetated sediments of 20 seagrass meadows along the Baltic Sea coast of Germany.
Six alternative candidate models were deemed statistically indistinguishable (ΔAICc < 2) from each other (summarized in Table 5A). Meaning, there was no strong support for one particular model. In the averaged model, seawater depth, seagrass complexity, and fine sediment fraction were similarly important in predicting Corg within seagrass-vegetated sediments (RVI 1.00 for each, Table 5B), and they had a significant negative (seawater depth) or positive (fine sediment fraction, seagrass complexity) effect on Corg stocks (see ‘Estimate’ in Table 5B). Avg. MOV had a weaker (by approx. 2.5 times) and insignificant effect in predicting Corg. There were no significant interactive effects between variables, except for that of seawater depth and seagrass complexity, but it had a weak (by approx. 5 times) predictive effect on Corg stocks.
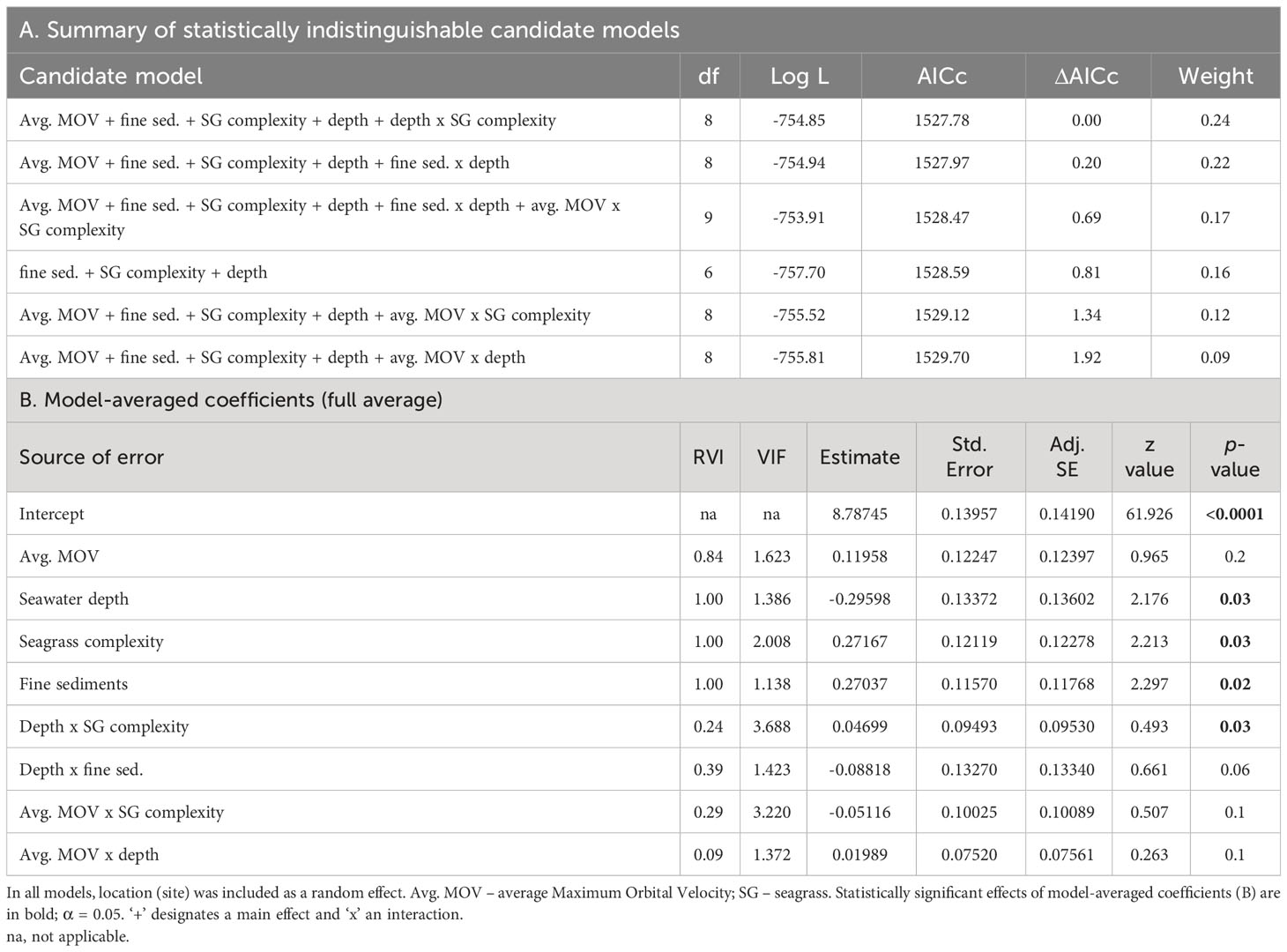
Table 5 Summary of alternative candidate Generalized Linear Mixed Models (Gamma, log link function) with ΔAICc < 2 (A) and their model-averaged coefficients (B) for biophysical predictors of regional sediment organic carbon (Corg) content of seagrass-vegetated sublocations along the Baltic Sea coast of Germany.
Discussion
Spatial heterogeneity of blue carbon stocks in Germany
Corg stocks in the top 25 cm of seagrass-vegetated sediments in the German Baltic Sea were high (average 1,920 ± 402 g C/m2), and richer than adjacent unvegetated sediments, but differed widely between and even within sites. Regional heterogeneity observed here was comparable to previous regional evaluations of blue carbon in Z. marina meadows (e.g. Röhr et al., 2016; Prentice et al., 2019), but local heterogeneity was much greater, with previous measurements reporting <10 times more Corg content in seagrass-vegetated vs unvegetated sediments (Table 6), or similar carbon content between these sublocations (Prentice et al., 2019; Prentice et al., 2020; Mazarrasa et al., 2021; Krause et al., 2022). Overall, average Corg stocks in the German Baltic Sea fit within the global range reported for those of Z. marina (318 ± 10 to 26,523 ± 667 g C/m2), but more closely resembled Z. marina Corg stocks in the Mediterranean Sea (8,793 + 2,248 g C/m2), Funen area of Denmark, and Skagerrak coast of Sweden, than Corg stocks in other parts of the Baltic Sea (Table 6). In fact, German blue carbon stocks were on average richer (by three times) than the Baltic Sea average (578 ± 43 g C/m2, excluding Germany, Röhr et al., 2018). A similar geographical trend was also reported for sites along the coast of Sweden, where percent Corg in top sediments were 10 to 25 times higher along the west (in Skagerrak) vs south and east coasts (in the Baltic Sea) of Sweden (Jephson et al., 2008). Consistent with the present study, wide regional variation in Corg stocks were observed in eastern Jutland of Denmark, which included “carbon hot spots” (stocks as high as 26,523 ± 667 g C/m2 in Thurøbund) that were comparable to those observed in Germany (10,577 ± 6,178 g C/m2). Two biophysical parameters were thought to be responsible for the Corg hotspot found in Thurøbund: low wave exposure and high seagrass productivity (420 ± 98 shoots/m2) – seagrass densities and exposures that were similar in magnitude to those measured in the sheltered bay of Orth (467 ± 98 shoots/m2) where the third highest Corg was found in Germany. It is worthwhile contemplating explanations for the higher Corg stocks reported in Skagerrak-Kattegat and southwestern Baltic Sea relative to other parts of this basin (Table 6). The rich geological history that shaped the Baltic Sea, including northward retreat of the Scandinavian ice sheet that caused land uplift in the south and changed coastal landscapes as a consequence of sea-level rise during the Littorina Transgression, but also shaped the geology that enhanced its ability to sequester Corg. For instance, hard-bottom and rocky shores dominate in the northern coasts, whereas till material and sandy/muddy beaches are common in the south (Schiewer, 2008).
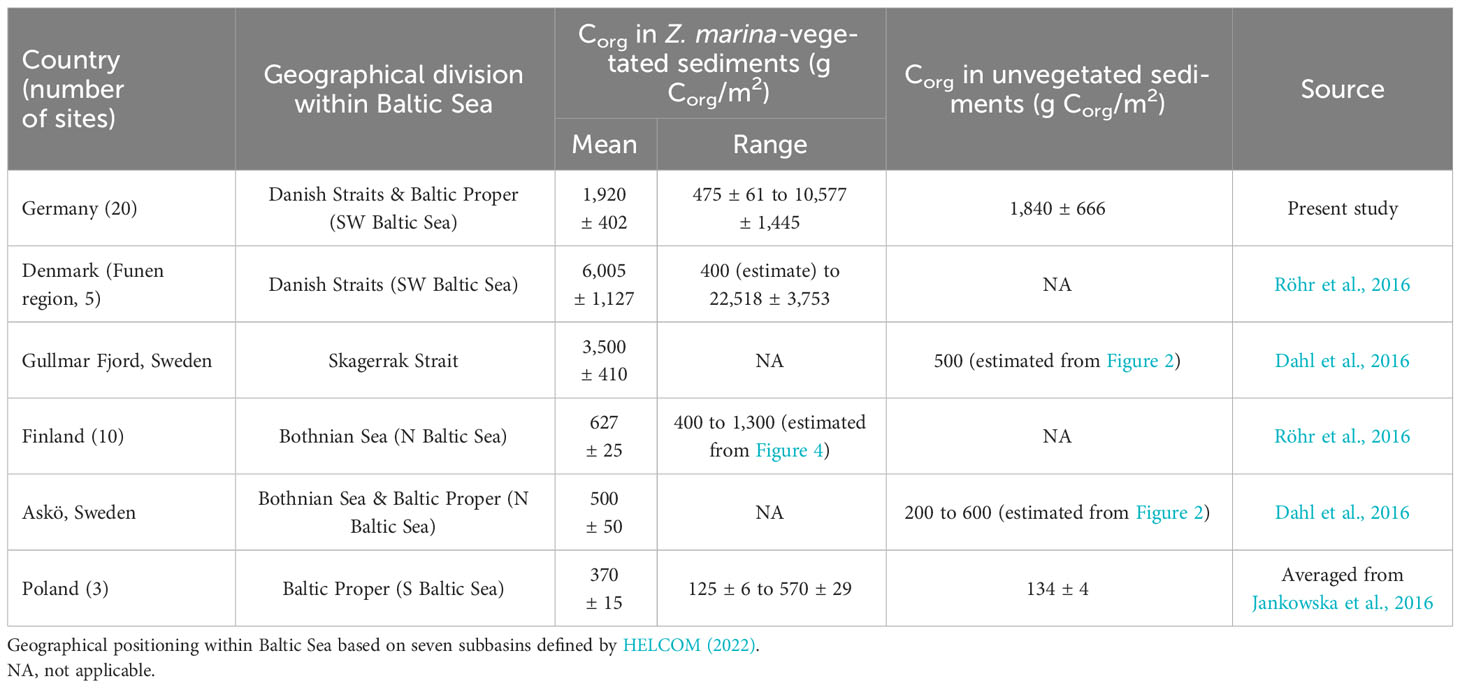
Table 6 Spatial heterogeneity (average, min, max) of Corg (g Corg/m2) in the upper 25 cm sediments of Zostera marina-vegetated and unvegetated sublocations of the Baltic Sea.
Sources of Corg
Our results suggest that the Corg accumulating in seagrass meadows in the German Baltic Sea is primarily (88%) originating from allochthonous sources, material originating from outside the seagrass meadow, thus, most of the Corg making up stocks here are imported from outside the boundaries of the meadow. Of the five Corg sources tested, this material was predominantly derived from a combination of phytoplankton, drift algae P. littoralis, and other macroalgae. It must be noted that the determination of the source materials in the SOC fraction was derived from stable isotope analyses of materials from sites in Schleswig-Holstein only, and so the source material for Mecklenburg-Vorpommern may differ from these. Nonetheless, these findings are consistent with those of neighboring Baltic Sea nations, like Finland, where phytoplankton material was the primary source of Corg (43 – 86%), while seagrass made a relatively small contribution to the overall sediment Corg pool (1.5 – 32%) (Röhr et al., 2016), and along the northwest Pacific coast, Z. marina meadows primarily sequestered allochthonous Corg, originating from plankton, terrestrial, and kelp sources rather than seagrass material (Prentice et al., 2019; Krause et al., 2022). The latter contributed less than 25.3% to the Corg pool. However, in Denmark and Poland, seagrass biomass was found to be the biggest contributor to sediment Corg pools (13 – 81%, Röhr et al., 2016; 40 – 45%, Jankowska et al., 2016). A country-wide analysis of blue carbon stocks in Australia revealed that coastal meadows in temperate regions were dominated by autochthonous Corg sources (72% was derived from seagrass), whereas allochthonous material prevailed in tropical meadows (64%) (Mazarrasa et al., 2021). In a global analysis (across 88 locations and multiple seagrass species) of seagrass blue carbon stocks, seagrass biomass accounted for approximately 50% of the Corg stocks, while the remainder originated from allochthonous sources (Kennedy et al., 2010).
In the mixing model, sampling location (not vegetation coverage) was the main driver of the variation in Corg sources (for the non-visible fraction of Corg; SOC). Coastal landscape and differences in inputs to marine foodwebs, including currents, upwelling, as well as point (e.g. sewage outfall, rivers) and diffuse (e.g. atmospheric, rainwater runoff) sources of nutrients may help explain some of the dissimilarity observed between locations. For example, phytoplankton contribution was abundant in the seagrass meadow in Graswarder, but absent in Wackerballig. The former is situated near a point source contamination of sewage outflow (Schubert et al., 2013), and the latter is adjacent to a large marine national park. Phytoplankton composition and abundance are often used as bioindicators of enhanced nutrient concentrations (2000/60/EC, EU, 2000). For the drift algae P. littoralis, large mats can become entrapped in narrow fjords (Falckenstein) and bays (Wackerballig), whereas they may be carried away from sites along open coasts (Grasswarder, Grossenbrode), which fits with the pattern seen in the sediment sources of the present study.
While Corg content was consistently higher in seagrass-vegetated vs unvegetated sediments, two cases had similar (Gluecksburg and Hasselfelde) or more (Gohren and Seebar) Corg in their unvegetated sediments. A similar phenomenon was previously reported for Z. marina meadows and other temperate seagrass species (see Mazarrasa et al., 2017a; Mazarrasa et al., 2017b; Prentice et al., 2019; Prentice et al., 2020; Mazarrasa et al., 2021; Krause et al., 2022). Some of this discrepancy was attributed to the export of organic material originating from seagrass habitats to adjacent unvegetated sediments (Duarte and Krause-Jensen, 2017). A similar spillover effect of Corg from adjacent seagrass meadows may be contributing to the high Corg accumulating in nearby unvegetated sediments in Hasselfelde, where visible Corg (not SOC, but also not seagrass biomass) were the main source of Corg in unvegetated sublocations. Also, there is a dense community (several cm thick) of bivalve Cerastoderma edule populated unvegetated sediments at this site (but not in seagrass-vegetated sediments) and their soft tissue would have contributed to Corg sinks (or CO2 sinks) measured in these sediments. Autochthonous export is not a valid justification for Gohren, Seebar and Gluecksburg as bare sediments were dominated by non-visible Corg (100% SOC) and the latter two had a sludge-like consistency with the top (Seebar) and sixth (Gluecksburg) highest fine (muddy) particle fraction of all sites and sublocations in the study. Heavy anthropogenic pressure at these sites may be contributing to the excess nutrient inputs that would elevate baseline Corg in the sediments (Nixon, 1995; Short and Burdick, 1996; Bowen and Valiela, 2001; Nedwell et al., 2002). Also, Seebar was not populated by seagrass only 8 years prior to the study, which could have contributed to these findings as well (pers. obs. P. Schubert). It must be noted that visible seagrass roots and rhizomes were omitted from this analysis, which may lead to a lower contribution of autochthonous organic carbon sources, as observed in the present study.
Unexpected large amounts of well-preserved wood pieces were found in one location: Sierksdorf, Luebeck Bay. Radiocarbon dating of this wood suggests that it was deposited here during two distinct time intervals, averaging at 5,806 BP and 5,095 BP, that coincide well with the second phase of the Littorina Transgression (dated approx. 6,000 to 3,800 BP) following the last deglaciation (Schmölcke et al., 2006; Kostecki et al., 2021). In this period, rapid changes occurred especially in the southwestern part of the Baltic Sea, where fast sea level rise combined with a flat landscape led to the widespread die off of forests situated along the coast. These events caused the demise of coastal alder woodlands that subsequently produced peat containing abundant alder-wood remains (Schmölcke et al., 2006). Indeed, the lack of age (14C) correlation across core depth is typical of natural wood pieces (rather than man-made artefacts) because younger wood can be found in deeper parts of the core due to the roots of the living tree. The two distinct time intervals occurring 700 years apart may suggest a recolonization event took place at this site. Interestingly, the two-time intervals are situated on either side of a brief cooling period (the Subboreal period), which started 5,650 BP and caused widespread natural environmental changes in the southwestern part of the Baltic Sea (Schmölcke et al., 2006). Note that while only one site was radiocarbon dated, we report old wood co-occurring with seagrass meadows in other parts of Schleswig-Holestein, Germany (see Table 1).
The oldest known submarine peatlands globally are dated 5,616 ± 46 years BP and correspond to the thick matte formed by Posidonia oceanica, a long-lived Mediterranean seagrass, which constitute deep and significant Corg stocks (Lo Iacono et al., 2008). Z. marina does not form similarly thick and old sedimentary deposits, but our investigation confirms that it too can store millennia of carbon by protecting former (and now submerged) forested peatlands, acting as a protective covering for these rich carbon deposits (Krause-Jensen et al., 2019). Even well-preserved prehistoric settlements have been previously discovered beneath Z. marina meadows in Denmark (Fischer, 2011; Andersen, 2013; Pedersen et al., 2017) and Germany (Goldhammer and Hartz, 2017). Submerged wood artefacts are also present in the Baltic Sea coast of Germany, including a site in Neustadt near Sierksdorf (Klooß, 2014), and they too overlap with seagrass habitats. Recognition that some seagrass meadows in Germany are sitting atop ancient terrestrial carbon deposits that are potentially several meters thick has important consequences for avoiding the re-emission of CO2 (via Corg) stored on a millennial timescale. However, our understanding of submerged coastlines is incomplete, so it is likely that many more submarine peatlands, like that found in Sierksdorf, await discovery near the German Baltic Sea coast.
Predicting blue carbon stocks across Germany
A fourth objective of the study was to use the identified relationships between seagrass meadow attributes and environmental parameters on Corg to extrapolate stocks for the entire German Baltic Sea region. This regional variation in Corg was best explained by seawater depth, seagrass complexity, and the fraction of fine particles in the sediment, not average MOV or interactions between parameters. The latter two had a positive effect on the Corg content in the sediment, whereas stocks decreased with seawater depth, suggesting that the highest stocks were found in shallow locations with high seagrass complexity and the ability to accumulate fine-grained particles, regardless of MOV at the seafloor. However, these parameters do not demonstrate a clear ability to predict the regional distribution of Corg (Figure 5). A combination of these three parameters, along with water motion, is consistently found to be the main biotic and abiotic driver of Corg in the literature, but its influence varies widely across regions and seems principally coupled to local hydrodynamic regimes. Because sediment erosion and detritus export rates are typically heavily influenced by water motion (e.g. lower wave height and exposure, fetch, and currents), sediment Corg content is typically lower in dynamic systems compared to more static ones (e.g. Samper-Villarreal et al., 2016; Mazarrasa et al., 2017a; Prentice et al., 2019; Novak et al., 2020; Mazarrasa et al., 2021). But in the absence of strong water movement, like in the German Baltic Sea where tides are negligible, water currents are weak, and wave heights are low, other aspects of the systems can shape Corg stocks. The lack of a trend observed in our data in spite of sampling extremes in hydrodynamics of our system, is a testament to this hypothesis, whereby the sites that experienced weakest (Orth, Maasholm, Sierksdorf) maximum current speeds at the seafloor both had the highest Corg pools of all sites examined in this study. However, sites with the strongest (Teichhof, Wackerballig) currents did not have low Corg pools, in fact they had the 10th and 11th highest Corg of all sites. Reduced hydrodynamics may also mean enhanced particle trapping from the water column, which may help explain the mainly allochthonous provenance of the Corg and overall high stocks in our study.
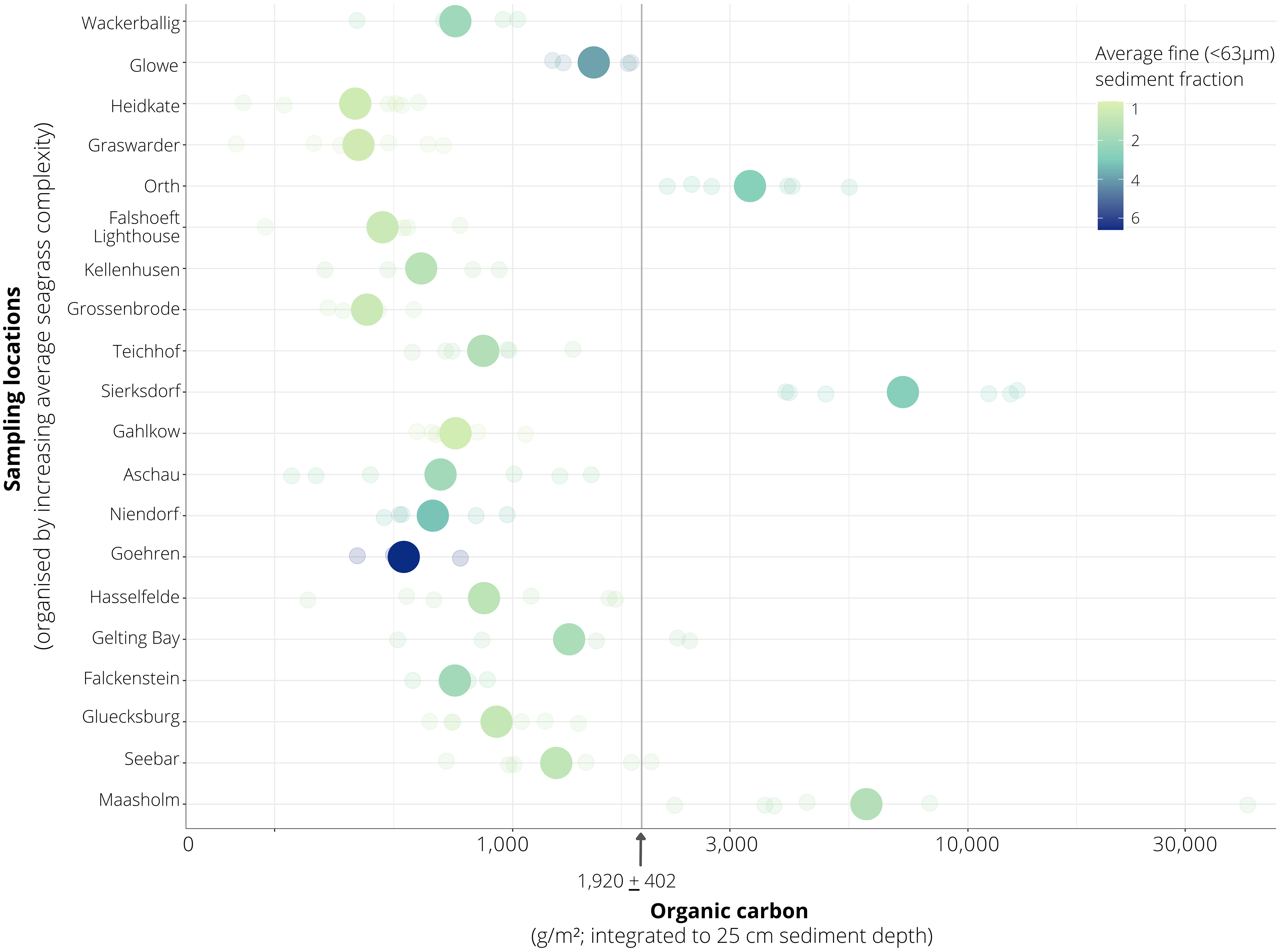
Figure 5 Interplay between seagrass complexity and fine grain (<63 μm) sediment predictors of organic carbon (Corg) content in seagrass-vegetated sediments along the Baltic Sea coast of Germany.
The positive relationship observed between the amount of fine particles in the sediment and Corg in seagrass-vegetated sublocations is not surprising as it is well known that more Corg is associated with finer mineral particles in soils and sediments (Calvert et al., 1995; Lin et al., 2002). These fine particles nurture anoxic conditions in the uppermost layer of the soil that protect organic particles from remineralization (Schrameyer et al., 2018; Brodersen et al., 2019). Our findings are consistent with previous findings for Z. marina meadows in other than the German parts of the Baltic Sea (e.g. Sweden, Dahl et al., 2016; Denmark and Finland, Röhr et al., 2016) and elsewhere (Z. marina outside Baltic Sea: Miyajima et al., 2015; Miyajima et al., 2017; Prentice et al., 2019; Krause et al., 2022; multiple species, including Z. marina: Kennedy et al., 2010; P. oceanica: Gacia et al., 2002; Hendriks et al., 2008). For example, in Denmark and Finland, more than 40% of the variation in Corg between sites could be explained by sediment characteristics, including the fine sediment content.
The thick canopy of seagrass leaves is known to effectively intercept particles in the water column, as well as decrease sediment erosion and seagrass detritus export (Ward et al., 1984; Fonseca and Cahalan, 1992; Madsen et al., 2001; Christianen et al., 2013). While seagrass complexity is a strong positive predictor of the regional differences in Corg in our study, and that of others (Jankowska et al., 2016; Samper-Villarreal et al., 2016; Serrano et al., 2016), this was not the case for Z. marina meadows off the coast of British Columbia, in Canada, where no clear relationship (negative or positive) was observed between the two parameters (Prentice et al., 2019). Here, water motion was the strongest predictor of Corg.
The dampening effect of seawater depth on surface water motion is correlated to the trend of increasing Corg content with deeper depths (Lavery et al., 2013; Mazarrasa et al., 2017a). While we also observed a dampened effect of water currents (but at the seafloor, rather than surface waters) by increasing seawater depth (ρ = -0.43), an opposite relationship was seen between Corg content and depth. A fourfold decrease in Corg was also observed with increasing seawater depth (from 2–4 m to 6–8 m) in P. oceanica seagrass, in the Mediterranean Sea (Serrano et al., 2014). Regarding the Baltic Sea, seagrass was historically present at deeper depths (e.g. observed beyond 8 m in the past ten years), but today it is rarely observed beyond 5 m seawater depth in Germany. The realization of the Helsinki Commission’s Baltic Sea Action Plan (BSAP) goals, which implicate a considerable nutrient abatement, would result in seagrass expansion into deeper waters, as was shown by Bobsien et al., 2021 for the German part of the Baltic Sea. This expansion would lead to an enhancement of the CO2 storage potential by these habitats, but it is important to consider that this potential is diminished at deeper depths (relative shallow depths) – an important consideration for carbon accounting when including seagrass blue carbon contributions to the German national CO2 budget.
Scaling up for CO2 accounting and further considerations
Our measurements confirm that seagrass meadows in the Baltic Sea coast of Germany store a large Corg pool. The high spatial heterogeneity seen across the region warrant site-specific investigations to obtain accurate estimates of blue carbon. However, localities with high seagrass complexity, high fine sediment fraction, and low seawater depth could help select localities with more favorable Corg accumulation potential. An unexpected and significant relic terrestrial Corg pool was found beneath the seagrass meadow in Sierksdorf (Luebeck Bay), and also confirmed in other locations (see Table 1). It is likely that many more submarine peatlands await discovery along the southwestern Baltic Sea region, and that they hold millennial timescale Corg deposits similar to those found in Germany.
Based on a conservative scaling up of measurements (integrated to 25 cm sediment depth), collectively (total of approx. 285 km2, Schubert et al., 2015) seagrass meadows in the German Baltic Sea are preventing 2.01 Mt of future CO2 emissions from being released into the atmosphere. However, it must be noted that in some locations (Gelting Bay, Teichhof) the sediment has been eroded to the marl layer, which seagrass roots cannot penetrate, while in other locations the sediment is known to extend to 7 m sediment thickness below the seafloor, e.g. within the inner areas of Mecklenburg Bay (Lemke, 1998), such as Sierksdorf, Niendorf, Kellenhusen, Grossenbrode in the present study.
Because Corg is dependent on high seagrass complexity, accumulation of blue carbon in Germany may be contingent on healthy seagrass habitats. Furthermore, loss of these habitats will have negative consequences for the German remaining CO2 budget because the Corg stored beneath meadows may be rereleased into the water column and later to the atmosphere. Their loss would also impact their many co-benefits (see Heckwolf et al., 2021). Given the pressing need to offset and prevent future CO2 emissions, more stringent and concerted efforts are urgently needed to enhance the Corg storage potential (via habitat restoration and improving growing conditions) and prevent further degradation (via conservation) of seagrass habitats along the Baltic Sea coast of Germany. Our study provides urgently needed knowledge and constitutes a further incentive to enhance efforts in protecting existing seagrass meadows in Germany, and restore them where natural recolonization is likely slow, such as in enclosed embayments or areas that have seen a loss in seagrass habitat, especially where the distance to the next seagrass-vegetated site is high.
Data availability statement
The datasets presented in this study can be found in online repositories. The names of the repository/repositories and accession number(s) can be found below: https://doi.pangaea.de/10.1594/PANGAEA.947704.
Author contributions
AS: Conceptualization, Data curation, Formal analysis, Investigation, Methodology, Visualization, Writing – original draft, Writing – review & editing. TÓC: Investigation, Methodology, Writing – review & editing. WH: Data curation, Formal analysis, Methodology, Software, Writing – review & editing. PS: Conceptualization, Funding acquisition, Methodology, Resources, Writing – review & editing. TR: Conceptualization, Funding acquisition, Resources, Supervision, Writing – review & editing.
Funding
The author(s) declare financial support was received for the research, authorship, and/or publication of this article. The Helmholtz-Climate-Initiative (HI-CAM) is funded by the Helmholtz Associations Initiative and Networking Fund. The authors are responsible for the content of this publication. BMBF-funded project SeaStore within program MARE:N.
Acknowledgments
Many thanks are due to Ainara Zander, Christian Howe, Dr. Florian Huber, Marlene Beer, Nasif Bin Said, Philipp Suessle, Roxanna Timm for their help in the field and/or lab. Dr. Christian Hamann, Dr. Jan Dierking, and Dr. Tomas Hansen for their insights on the stable isotope analyses.
Conflict of interest
The authors declare that the research was conducted in the absence of any commercial or financial relationships that could be construed as a potential conflict of interest.
Publisher’s note
All claims expressed in this article are solely those of the authors and do not necessarily represent those of their affiliated organizations, or those of the publisher, the editors and the reviewers. Any product that may be evaluated in this article, or claim that may be made by its manufacturer, is not guaranteed or endorsed by the publisher.
References
Andersen S. H. (2013). Tybrind Vig: submerged mesolithic settlements in Denmark. Jutland Archaeological Society/Moesgård Museum: Højbjerg, 527.
Andrén T. (2012). “‘Baltic Sea Basin, since the latest deglaciation’,” in Encyclopedia of Lakes and Reservoirs. Eds. Bengtsson L., Herschy R. W., Fairbridge R. W. (Dordrecht: Springer Science+Business Media B.V), 95–102.
Bartoń K. (2022) MuMIn: Multi-Model Inference. R package version 1.46.0. Available at: https://CRAN.R-project.org/package=MuMIn.
Bates D., Mächler M., Bolker B., Walker S. (2015). Fitting linear mixed-effects models using lme4. J. Stat. Software 67 (1), 1–48. doi: 10.48550/ arXiv.1406.5823
Bobsien I. C., Hukriede W., Schlamkow C., Friedland R., Dreier N., Schubert P. R., et al. (2021). Modelling eelgrass spatial response to nutrient abatement measures in a changing climate. Ambio 50, 400–412. doi: 10.1007/s13280-020-01364-2
Bowen J. L., Valiela I. (2001). The ecological effects of urbanization of coastal watersheds: Historical increases in nitrogen loads and eutrophication of Waquoit Bay estuaries. Can. J. Fish. Aquat. Sci. 58, 1489–1500. doi: 10.1139/f01-094
Brodersen K. E., Trevathan-Tackett S. M., Nielsen D. A., Connolly R. M., Lovelock C. E., Atwood T. B., et al. (2019). Oxygen consumption and sulfate reduction in vegetated coastal habitats: effects of physical disturbance. Front. Mar. Sci. 6, 14. doi: 10.3389/fmars.2019.00014
Calvert S. E., Pedersen T. F., Naidu P. D., Von Stackelberg U. (1995). On the organic carbon maximum on the continental slope of the eastern Arabian Sea. J. Mar. Res. 53, 269–296. doi: 10.1357/0022240953213232
Christianen M. J., van Belzen J., Herman P. M., van Katwijk M. M., Lamers L. P., van Leent P. J., et al. (2013). Low-canopy seagrass beds still provide important coastal protection services. PLoS One 8 (5), e62413. doi: 10.1371/journal.pone.0062413
Dahl M., Deyanova D., Gütschow S., Asplund M. E., Lyimo L. D., Karamfilov V., et al. (2016). Sediment properties as important predictors of carbon storage in Zostera marina meadows: a comparison of four European areas. PLoS One 11 (12), e0167493. doi: 10.1371/journal.pone.0167493
Duarte C. M., Kennedy H., Marbaá N., Hendriks I. (2013). Assessing the capacity of seagrass meadows for carbon burial: Current limitations and future strategies. Ocean Coast. Manage. 83, 32–38. doi: 10.1016/j.ocecoaman.2011.09.001
Duarte C. M., Krause-Jensen D. (2017). Export from seagrass meadows contributes to marine carbon sequestration. Front. Mar. Sci. 4, 1–7. doi: 10.3389/fmars.2017.00013
Duarte C. M., Middelburg J. J., Caraco N. (2005). Major role of marine vegetation on the oceanic carbon cycle. Biogeosciences 2 (1), 1–8. doi: 10.5194/bg-2-1-2005
Fischer A. (2011). ““Stone age on the continental shelf: An eroding resource,”,” in Submerged Prehistory. Eds. Benjamin J., Bonsall C., Pickard C., Fischer A. (Oxford: Oxbow Books), 298–310.
Fonseca M. S., Cahalan J. A. (1992). A preliminary evaluation of wave attenuation by four species of seagrass. Estuarine Coast. Shelf Sci. 35 (6), 565–576. doi: 10.1016/S0272-7714(05)80039-3
Fourqurean J. W., Duarte C. M., Kennedy H., Marba N., Holmer M., Mateo A. M. (2012). Seagrass ecosystems as a globally significant carbon stock. Nat. Geosci. 5, 505–509. doi: 10.1038/ngeo1477
Gacia E., Duarte C. M., Middelburg J. J. (2002). Carbon and nutrient deposition in a Mediterranean seagrass (Posidonia oceanica) meadow. Limnol. Oceanogr. 47, 23–32. doi: 10.4319/lo.2002.47.1.0023
Goldhammer J., Hartz S. (2017). “Fished up from the Baltic Sea: A New Ertebølle Site near Stohl Cliff, Kiel Bay, Germany,” in Under the Sea: Archaeology and Palaeolandscapes of the Continental Shelf. Coastal Research Library, vol. 20 . Eds. Bailey G., Harff J., Sakellariou D. (Cham: Springer). doi: 10.1007/978-3-319-53160-1_9
Greiner J. T., Wilkinson G. M., McGlathery K. J., Emery K. A. (2016). Sources of sediment carbon sequestered in restored seagrass meadows. Mar. Ecol. Prog. Ser. 551, 95–105. doi: 10.3354/meps11722
Gullström M., Lyimo L. D., Dahl M., Samuelsson G. S., Eggertsen M., Anderberg E., et al. (2018). Blue carbon storage in tropical seagrass meadows relates to carbonate stock dynamics, plant–sediment processes, and landscape context: insights from the western Indian Ocean. Ecosystems 21 (3), 551–566. doi: 10.1007/s10021-017-0170-8
Hansen T., Burmeister A., Sommer U. (2009). Simultaneous δ15N, δ13C and δ34S measurements of low-biomass samples using a technically advanced high sensitivity elemental analyzer connected to an isotope ratio mass spectrometer. Rapid Commun. Mass Spectrom. 23, 3387–3393. doi: 10.1002/rcm.4267
Heckwolf M. J., Peterson A., Jänes H., Horne P., Künne J., Liversage K., et al. (2021). From ecosystems to socio-economic benefits: A systematic review of coastal ecosystem services in the Baltic Sea. Sci. Total Environ. 755, 142565. doi: 10.1016/j.scitotenv.2020.142565
HELCOM (2018). State of the Baltic Sea - Second HELCOM holistic assessment 2011-2016, in Baltic Sea Environment Proceedings 155. (Helsinki, Finland: Baltic Marine Environment Protection Commission)
HELCOM (2022). HELCOM Guidelines for the annual and periodical compilation and reporting of waterborne pollution inputs to the Baltic Sea (PLC-Water). (Helsinki, Finland: PLC-Water)
Hendriks I. E., Sintes T., Bouma T. J., Duarte C. M. (2008). Experimental assessment and modelling evaluation of the effects of the seagrass Posidonia oceanica on flow and particle trapping. Mar. Ecol. Prog. Ser. 356, 163–173. doi: 10.3354/meps07316
Howard J., Hoyt S., Isensee K., Pidgeon E., Telszewski M. (2014). Methods for assessing carbon stocks and emissions factors in mangroves, tidal salt marshes, and seagrass meadows (Arlington, Virginia, USA: Conservation International, Intergovernmental Oceanographic Commission of UNESCO, International Union for Conservation of Nature).
Jankowska E., Michel L. N., Zaborska A., Włodarska-Kowalczuk M. (2016). Sediment carbon sink in low-density temperate eelgrass meadows (Baltic Sea). J. Geophys. Res-Biogeo. 121 (12), 2918–2934. doi: 10.1002/2016JG003424
Jephson T., Nyström P., Moksnes P. O., Baden S. P. (2008). Trophic interactions in Zostera marina beds along the Swedish coast. Mar. Ecol. Prog. Ser. 369, 63–76. doi: 10.3354/meps07646
Kennedy H., Beggins J., Duarte C. M., Fourqurean J. W., Holmer M., Marba N. (2010). Seagrass sediments as a global carbon sink: Isotopic constraints. Global Biogeochem. Cy 24, GB4026. doi: 10.1029/2010GB003848
Kiirikki M., Lehvo A. (1997). Life strategies of filamentous algae in the northern Baltic Proper. Sarsia 82, 259–268. doi: 10.1080/00364827.1997.10413653
Klingler S., Cirpka O. A., Werban U., Leven C., Dietrich P. (2020). Direct-push color logging images spatial heterogeneity of organic carbon in floodplain sediments. J. Geophysical Research: Biogeosciences 125 (12), e2020JG005887.
Klooß S. (2014). They were fishing in the sea and coppicing the forest. Bericht der Römisch-Germanischen Kommission 92, 251–274.
Kostecki R., Janczak-Kostecka B., Endler M. (2021). Littorina and post-Littorina sedimentological processes in the Odra Channel in light of multidisciplinary investigations of a sediment core, Pomeranian Bay, southern Baltic Sea. Quat. Int. 602, 131–142. doi: 10.1016/j.quaint.2020.10.044
Krause J. R., Hinojosa-Corona A., Gray A. B., Herguera J. C., McDonnell J., Schaefer M. V., et al. (2022). Beyond habitat boundaries: Organic matter cycling requires a system-wide approach for accurate blue carbon accounting. Limnol. Oceanogr. 9999, 1–13. doi: 10.1002/lno.12071
Krause-Jensen D., Duarte C. M. (2016). Substantial role of macroalgae in marine carbon sequestration. Nat. Geosci 9, 737–742. doi: 10.1038/ngeo2790
Krause-Jensen D., Serrano O., Apostolaki E. T., Gregory D. J., Duarte C. M. (2019). Seagrass sedimentary deposits as security vaults and time capsules of the human past. Ambio 48 (4), 325–335. doi: 10.1007/s13280-018-1083-2
Kruk-Dowgiallo L. (1991). Long-term changes in the structure of underwater meadows of the Puck lagoon. Acta Ichthyol. Piscat. Suppl. 22, 77–84. doi: 10.3750/AIP1991.21.S.09
Lavery P. S., Mateo M.-Á., Serrano O., Rozaimi M. (2013). Variability in the carbon storage of seagrass habitats and its implications for global estimates of blue carbon ecosystem service. PLoS One 8, e73748. doi: 10.1371/journal.pone.0073748
Lemke W. (1998). Sedimentation und paläogeographische Entwicklung im westlichen Ostseeraum (Mecklenburger Bucht bis Arkonabecken) vom Ende der Weichselvereisung bis zur Litorinatransgression. Meereswissenschtliche Berichte, vol. 31 . In: Marine science reports. (Warnemünde: Baltic Sea Research Institute).
Lin S., Hsieh I.-J., Huang K.-M., Wang C.-H. (2002). Influence of the Yangtze River and grain size on the spatial variations of heavy metals and organic carbon in the East China Sea continental shelf sediments. Chem. Geol. 182, 377–394. doi: 10.1016/S0009-2541(01)00331-X
Lo Iacono C., Mateo M. A., Gracia E., Guasch L., Carbonell R., Serrano L., et al. (2008). Very high-resolution seismo-acoustic imaging of seagrass meadows (Mediterranean Sea): Implications for carbon sink estimates. Geophys. Res. Lett. 35 (18), 18601. doi: 10.1029/2008GL034773
Macreadie P. I., Allen K., Kelaher B. P., Ralph P. J., Skilbeck. C. G. (2012). Paleoreconstruction of estuarine sediments reveal human-induced weakening of coastal carbon sinks. Glob. Change Biol. 18, 891–901. doi: 10.1111/j.1365-2486.2011.02582.x
Macreadie P. I., Baird M. E., Trevathan-Tackett S. M., Larkum A. W. D., Ralph P. J. (2014). Quantifying and modelling the carbon sequestration capacity of seagrass meadows - a critical assessment. Mar. pollut. Bull. 83, 430–439. doi: 10.1016/j.marpolbul.2013.07.038
Macreadie P. I., Costa M. D., Atwood T. B., Friess D. A., Kelleway J. J., Kennedy H., et al. (2021). Blue carbon as a natural climate solution. Nat. Rev. Earth Environ. 2 (12), 826–839. doi: 10.1038/s43017-021-00224-1
Madsen J. D., Chambers P. A., James W. F., Koch E. W., Westlake D. F. (2001). The interaction between water movement, sediment dynamics and submersed macrophytes. Hydrobiologia 444 (1-3), 71–84. doi: 10.1023/A:1017520800568
Maksymowska D., Richard P., Piekarek-Jankowska H., Riera P. (2000). Chemical and isotopic composition of the organic matter sources in the Gulf of Gdansk (Southern Baltic Sea). Estuar. Coast. Shelf S. 51 (5), 585–598. doi: 10.1006/ecss.2000.0701
Mazarrasa I., Marbaá N., Garcia-Orellana J., Masqué P., Arias-Ortiz A., Duarte C. M. (2017b). Dynamics of carbon sources supporting burial in seagrass sediments under increasing anthropogenic pressure. Limnol. Oceanogr. 62, 1451–1465. doi: 10.1002/lno.10509
Mazarrasa I., Lavery P., Duarte C. M., Lafratta A., Lovelock C. E., Macreadie P. I., et al. (2021). Factors determining seagrass Blue Carbon across bioregions and geomorphologies. Global Biogeochem. Cy 35 (6), e2021GB006935. doi: 10.1029/2021GB006935
Mazarrasa I., Marbá N., Garcia-Orellana J., Masqué P., Arias-Ortiz A., Duarte C. M. (2017a). Effect of environmental factors (wave exposure and depth) and anthropogenic pressure in the C sink capacity of Posidonia oceanica meadows. Limnol. Oceanogr. 62, 1436–1450. doi: 10.1002/lno.10510
Mazarrasa I., Samper-Villarreal J., Serrano O., Lavery P. S., Lovelock C. E., Duarte C. M., et al. (2018). Habitat characteristics provide insights of carbon storage in seagrass meadows. Mar. pollut. Bull. 134, 106–117. doi: 10.1016/j.marpolbul.2018.01.059
Mcleod E., Chmura G. L., Bouillon S., Salm R., Björk M., Duarte C. M., et al. (2011). A blueprint for blue carbon: toward an improved understanding of the role of vegetated coastal habitats in sequestering CO2. Front. Ecol. Environ. 9 (10), 552–560. doi: 10.1890/110004
Mittermayr A., Fox S. E., Sommer U. (2014). Temporal variation in stable isotope composition (δ13C, δ15N and δ34S) of a temperate Zostera marina food web. Mar. Ecol. Prog. Ser. 505, 95–105. doi: 10.3354/meps10797
Miyajima T., Hori M., Hamaguchi M., Shimabukuro H., Adachi H., Yamano H., et al. (2015). Geographic variability in organic carbon stock and accumulation rate in sediments of East and Southeast Asian seagrass meadows. Global Biogeochem. Cy. 29, 397–415. doi: 10.1002/2014GB004979
Miyajima T., Hori M., Hamaguchi M., Shimabukuro H., Yoshida G. (2017). Geophysical constraints for organic carbon sequestration capacity of Zostera marina seagrass meadows and surrounding habitats. Limnol. Oceanogr. 62, 954–972. doi: 10.1002/lno.10478
Nederbragt A. J., Dunbar R. B., Osborn A. T., Palmer A., Thurow J. W., Wagner T. (2006). “Sediment colour analysis from digital images and correlation with sediment composition,” in Geological Society, vol. 267. (London: Special Publications), 113–128.
Nedwell D. B., Dong L. F., Sage A., Underwood G. J. C. (2002). Variations of the nutrients loads to the mainland UK estuaries: Correlation with catchment areas, urbanization and coastal eutrophication. Estuar. Coast. Shelf Sci. 54, 951–970. doi: 10.1006/ecss.2001.0867
Nixon S. W. (1995). Coastal marine eutrophication: A definition, social causes, and future concerns. Ophelia 41, 199–219. doi: 10.1080/00785236.1995.10422044
Novak A. B., Pelletier M. C., Colarusso P., Simpson J., Gutierrez M. N., Arias-Ortiz A., et al. (2020). Factors influencing carbon stocks and accumulation rates in eelgrass meadows across New England, USA. Estuaries Coasts 43 (8), 2076–2091. doi: 10.1007/s12237-020-00754-9
Oreska M. P. J., Wilkinson G. M., McGlathery K. J., Bost M., McKee B. A. (2018). Non‐seagrass carbon contributions to seagrass sediment blue carbon. Limnol. Oceanogr. 63 (S1), 53–518. doi: 10.1002/lno.10718
Pedersen L., Fischer A., Gregory D. J. (2017). Fletværket ved Nekselø – skovdrift og storstilet fiskeri i bondestenalderen. Nationalmuseets Arbejdsmark 63, 134–145.
Petterson H., Ola K., Brüning T. (2018). “Wave climate in the Baltic Sea in 2017,” in HELCOM Baltic Sea Environment Fact Sheets. Available at: http://www.helcom.fi/baltic-sea-trends/environment-fact-sheets/.
Prentice C., Hessing-Lewis M., Sanders-Smith R., Salomon A. K. (2019). Reduced water motion enhances organic carbon stocks in temperate eelgrass meadows. Limnol. Oceanogr. 64 (6), 2389–2404. doi: 10.1002/lno.11191
Prentice C., Poppe K. L., Lutz M., Murray E., Stephens T. A., Spooner A., et al. (2020). A synthesis of blue carbon stocks, sources, and accumulation rates in eelgrass (Zostera marina) meadows in the Northeast Pacific. Global Biogeochem Cy 34 (2), e2019GB006345. doi: 10.1029/2019GB006345
Ramsey C. B., Lee S. (2013). Recent and planned developments of the program OxCal. Radiocarbon 55, 720–730. doi: 10.1017/S0033822200057878
R Core Team (2022). R: A language and environment for statistical computing (Vienna, Austria: R Foundation for Statistical Computing). Available at: https://www.R-project.org/.
Reimer P. J., Austin W. E., Bard E., Bayliss A., Blackwell P. G., Ramsey C. B., et al. (2020). The IntCal20 Northern Hemisphere radiocarbon age calibration curve (0–55 cal kBP). Radiocarbon 62 (4), 725–757. doi: 10.1017/RDC.2020.41
Ricart A. M., York P. H., Bryant C. V., Rasheed M. A., Ierodiaconou D., Macreadie P. I. (2020). High variability of blue carbon storage in seagrass meadows at the estuary scale. Sci. Rep. 10, 5865. doi: 10.1038/s41598-020-62639-y
Röhr M. E., Bostrom C., Canal-Verges P., Holmer M. (2016). Blue carbon stocks in Baltic Sea eelgrass (Zostera marina) meadows. Biogeosciences 13 (22), 6139–6153. doi: 10.5194/bg-13-6139-2016
Röhr M. E., Holmer M., Baum J. K., Björk M., Boyer K., Chin D., et al. (2018). Blue carbon storage capacity of temperate eelgrass (Zostera marina) meadows. Global Biogeochem Cy. 32 (10), 1457–1475. doi: 10.1029/2018GB005941
Samper-Villarreal J., Lovelock C. E., Saunders M. I., Roelfsema C., Mumby P. J. (2016). Organic carbon in seagrass sediments is influenced by seagrass canopy complexity, turbidity, wave height, and water depth. Limnol. Oceanogr. 61, 938–952. doi: 10.1002/lno.10262
Schiewer U. (2008). “The Baltic coastal zones” in Ecology of Baltic Coastal Waters. Ed. Schiewer U. (Berlin: Springer), 23–33.
Schmölcke U., Endtmann E., Klooss S., Meyer M., Michaelis D., Rickert B. H., et al. (2006). Changes of sea level, landscape and culture: a review of the south-western Baltic area between 8800 and 4000 BC. Palaeogeogr. Palaeocl 240 (3-4), 423–438. doi: 10.1016/j.palaeo.2006.02.009
Schrameyer V., York P. H., Chartrand K., Ralph P. J., Kühl M., Brodersen K. E., et al. (2018). Contrasting impacts of light reduction on sediment biogeochemistry in deep-and shallow-water tropical seagrass assemblages (Green Island, Great Barrier Reef). Mar. Environ. Res. 136, 38–47. doi: 10.1016/j.marenvres.2018.02.008
Schubert P. R., Hukriede W., Karez R., Reusch T. B. (2015). Mapping and modelling eelgrass Zostera marina distribution in the western Baltic Sea. Mar. Ecol. Prog. Ser. 522, 79–95. doi: 10.3354/meps11133
Schubert P. R., Karez R., Reusch T. B., Dierking J. (2013). Isotopic signatures of eelgrass (Zostera marina L.) as bioindicator of anthropogenic nutrient input in the western Baltic Sea. Mar. pollut. Bull. 72 (1), 64–70. doi: 10.1016/j.marpolbul.2013.04.029
Schubert H., Schygulla C. (2016). Die Erfassung rezenter Zosfera-Bestände und weiterer Makrophyten in den Küstengewässern MV“ (ZOSINF), Im Auftrag des Landesamtes für Umwelt (Rostock, Germany: Naturschutz und Geologie Mecklenburg-Vorpommern) (LUNG 100G-30.15/16). pp. 76.
Schubert H., Steinhardt T. (2014). Monitoring Makrophytobenthos – Dokumentation von historischen und rezenten Seegrasvorkommen für die Bewertung nach WRRL und MSRL entlang der Ostseeküste Mecklenburg-Vorpommerns (Güstrow: Landesamt für Umwelt, Naturschutz und Geologie Mecklenburg-Vorpommern), Postfach 1338, 18263. pp. 43.
Serrano O., Lavery P. S., Rozaimi M., Mateo M. Á. (2014). Influence of water depth on the carbon sequestration capacity of seagrasses. Global Biogeochem. Cy. 28, 950–961. doi: 10.1002/2014GB004872
Serrano O., Lovelock C. E., B Atwood T., Macreadie P. I., Canto R., Phinn S., et al. (2019). Australian vegetated coastal ecosystems as global hotspots for climate change mitigation. Nat. Commun. 10 (1), 1–10. doi: 10.1038/s41467-019-12176-8
Serrano O., Ricart A. M., Lavery P. S., Mateo M. A., Arias-Ortiz A., Masque P., et al. (2016). Key biogeochemical factors affecting soil carbon storage in Posidonia meadows. Biogeosciences 13 (15), 4581–4594. doi: 10.5194/bg-13-4581-2016
Short F. T., Burdick D. M. (1996). Quantifying eelgrass habitat loss in relation to housing development and nitrogen loading in Waquoit Bay, Massachusetts. Estuaries 19, 730. doi: 10.2307/1352532
Short F., Carruthers T., Dennison W., Waycott M. (2007). Global seagrass distribution and diversity: a bioregional model. J. Exp. Mar. Biol. Ecol. 350 (1-2), 3–20. doi: 10.1016/j.jembe.2007.06.012
Stock B. C., Semmens B. X. (2016) MixSIAR GUI User Manual. Version 3.1. Available at: https://github.com/brianstock/MixSIAR.
Stuiver M., Polach H. A. (1977). Discussion: reporting of 14C data. Radiocarbon 19, 355–363. doi: 10.1017/S0033822200003672
Thorsøe M. H., Andersen M. S., Brady M. V., Graversgaard M., Kilis E., Pedersen A. B., et al. (2022). Promise and performance of agricultural nutrient management policy: Lessons from the Baltic Sea. Ambio 51 (1), 36–50. doi: 10.1007/s13280-021-01549-3
Keywords: climate change, Germany, nature-based solution, radiocarbon dating, submarine peatland, underwater archaeology, Zostera marina, carbon dioxide removal
Citation: Stevenson A, Ó Corcora TC, Hukriede W, Schubert PR and Reusch TBH (2023) Substantial seagrass blue carbon pools in the southwestern Baltic Sea include relics of terrestrial peatlands. Front. Mar. Sci. 10:1266663. doi: 10.3389/fmars.2023.1266663
Received: 25 July 2023; Accepted: 23 November 2023;
Published: 15 December 2023.
Edited by:
Stelios Katsanevakis, University of the Aegean, GreeceReviewed by:
Stefania Klayn, Bulgarian Academy of Sciences, BulgariaKasper Elgetti Brodersen, University of Copenhagen, Denmark
Kun-Seop Lee, Pusan National University, Republic of Korea
Copyright © 2023 Stevenson, Ó Corcora, Hukriede, Schubert and Reusch. This is an open-access article distributed under the terms of the Creative Commons Attribution License (CC BY). The use, distribution or reproduction in other forums is permitted, provided the original author(s) and the copyright owner(s) are credited and that the original publication in this journal is cited, in accordance with accepted academic practice. No use, distribution or reproduction is permitted which does not comply with these terms.
*Correspondence: Angela Stevenson, astevenson@geomar.de