Sediment-Derived Dissolved Organic Matter Stimulates Heterotrophic Prokaryotes Metabolic Activity in Overlying Deep Sea in the Ulleung Basin, East Sea
- 1Department of Marine Science and Convergence Technology, Hanyang University, Ansan, South Korea
- 2Division of Polar Ocean Environment, Korea Polar Research Institute, Incheon, South Korea
- 3School of Earth and Environmental Sciences/Research Institute of Oceanography, Seoul National University, Seoul, South Korea
- 4Oceanic Climate and Ecology Research Division, National Institute of Fisheries Science, Busan, South Korea
The effects of benthic dissolved organic carbon (DOC) flux on the dynamics of DOC in the deep continental margins (200 – 2000 m depth) is poorly understood. We investigated heterotrophic prokaryotes (hereafter bacteria) production (BP) and the bio-reactive properties of sediment-derived dissolved organic matter (SDOM) to elucidate microbially mediated cause-effect relationships regarding the rapid consumption of dissolved oxygen (DO) and accumulation of humic-like fluorescent DOM (FDOMH) in the deep-water column (750 – 2000 m depth range) of the Ulleung Basin (UB) in the East Sea. BP in the deep water (2.2 μmol C m-3 d-1) of the UB was among the highest reported for various deep-sea sites. The high DOC concentration (55 μM) likely supported the high BP seen in the deep-water column of the UB. Concentrations of DOC and C1 component of the FDOMH, which is indicative of microbial metabolic by-products, were 13-fold and 20-fold greater, respectively, in pore water than in the overlying bottom water, indicating that the sediment in the continental margins is a significant source of DOM in the overlying water column. Fine-scale water sampling revealed that BP near the sediment (0 – 30 m above the seafloor; 2.78 μmol C m-3 d-1) was 1.67 times higher than that measured in the water column above (30 – 100 m above the seafloor; 1.67 μmol C m-3 d-1). In addition, BP increased in the bottom water incubation amended with SDOM-containing pore water (PW). The results demonstrated that SDOM contains bio-reactive forms of DOM that stimulate heterotrophic microbial metabolism at the expense of oxygen in the bottom water layer. The accumulation of C1 component in both PW-amended and unamended bottom water incubation (i.e., without an extra DOM supply from sediment) further indicated that refractory DOM is produced autochthonously in the water column via heterotrophic metabolic activity. This explains in part the microbially mediated accumulation of excess FDOMH in the deep-water column of the UB. Overall results suggest that the benthic release of bio-reactive DOM may be of widespread significance in controlling microbial processes in the deep-water layer of marginal seas.
Introduction
Due to the large quantity of dissolved organic carbon (DOC) in the ocean (~ 660 Pg), which is comparable to the amount of CO2 in the atmosphere, and its wide spectrum of reactivity, oceanic DOC is considered an important component of biogeochemical carbon cycles regulating ocean carbon storage (Church et al., 2002; Hansell, 2013; Hansell and Carlson, 2015). Several biotic and abiotic processes associated with production, transformation, and removal of DOC in the ocean have been proposed (see Jiao et al., 2010; Arrieta et al., 2015; Carlson and Hansell, 2015; Jiao et al., 2018; Shen and Benner, 2018). However, relatively little is known about the role of benthic DOC flux on the dynamics of DOC in the deep ocean, especially in the continental margins (200 - 2000 m depth), where integrated benthic DOC flux (89 Tg C yr-1) accounts for 45% of riverine DOC flux (160 Tg C yr-1) (see Burdige, 2002).
In general, a large fraction of the DOC produced during the early diagenetic process in sediments is considered recalcitrant (Weston and Joye, 2005; Chipman et al., 2010; Robador et al., 2010; Komada et al., 2012; Fox et al., 2018). However, most recent studies revealed that a certain fraction of sediment-derived DOM (SDOM) has the potential to be bio-reactive in the overlying water column (Rossel et al., 2016; Jessen et al., 2017; Cai et al., 2019; Tobias-Hünefeldt et al., 2021). The impact of benthic DOM flux on the concentration and properties of DOM depends on the reactivity of SDOM in the water column (Burdige and Komada, 2015). Since heterotrophic prokaryotes (hereafter bacteria, as a traditional ecological term) are the only biological component responsible for consumption and transformation of particulate organic carbon (POC) and DOC in the deep ocean (Nagata et al., 2000; Reinthaler et al., 2006; Arístegui et al., 2009; Liu et al., 2020), it is particularly important to investigate direct interactions between microbial metabolic activities and SDOM, in order to better understand the dynamics (i.e., distribution and transformation) of DOC in deep sea environments (Jiao et al., 2010; Arrieta et al., 2015; Shen and Benner, 2018).
The East Sea (often referred to as the Sea of Japan), which is located in the far eastern region of the Eurasian continental margin, consists of three major deep basins: the Japan Basin, Yamato Basin and Ulleung Basin (Figure 1). Compared to the other two basins, the Ulleung Basin (UB) is characterized by the highest primary production (Yamada et al., 2005; Hyun et al., 2009; Yoo and Park, 2009; Kim et al., 2012; Kwak et al., 2013; Joo et al., 2014). Owing to the high export flux of phytoplankton-derived labile organic carbon (Corg) (Kim et al., 2011) and lateral transport of resuspended material along the continental slope (Lee et al., 2019), the surface sediment of the UB is characterized by high Corg content (> 2.5% dry wt.) and Corg mineralization rate that is the highest among deep sea sediments with a similar depth range (Lee et al., 2008; Hyun et al., 2017; Lee et al., 2019). Therefore, the water column and sediment of the UB is regarded as a biological and biogeochemical hot spot where a significant turnover of organic matter and nutrient regeneration occurs (Joo et al., 2014; Hyun et al., 2017).
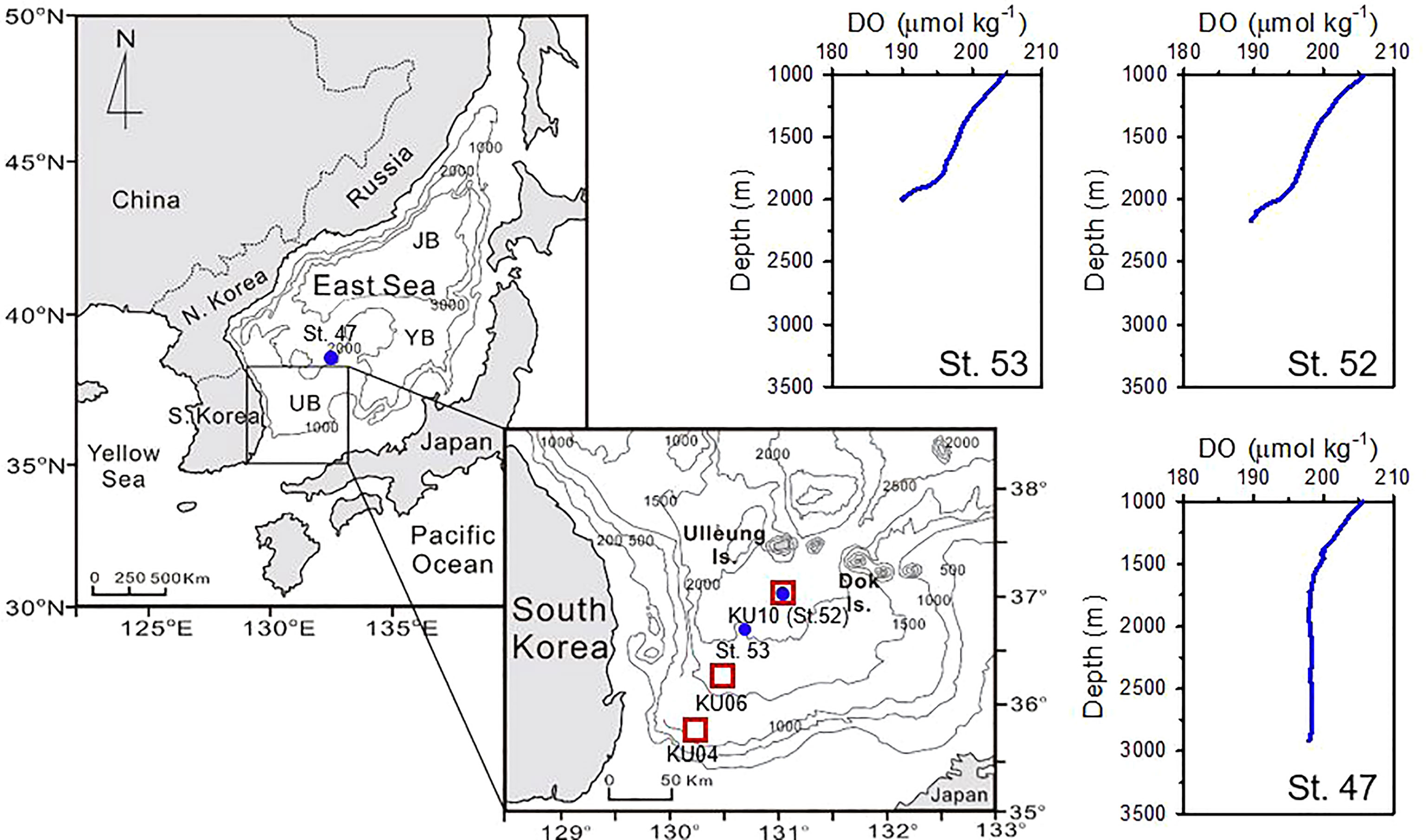
Figure 1 Maps of sampling sites in the upper continental slope (KU04; 35° 49’ 48” N; 130° 9’ 36” E), lower slope (KU06; 36° 14’ 24” N; 130° 27’ 36” E) and rise (KU10; 37° 01’ 12” N; 131° 03’ 00” E) of the Ulleung Basin (UB). Vertical profiles of dissolved oxygen (DO) concentrations (St. 47, St. 52 and St. 53) were re-drawn from Kang et al. (2010). Unlike DO that measured outside the UB (St. 47), DO concentrations in the UB region (St. 52 and 53) show a sharp decrease in the bottom water layer.
In addition to research on the surface water column and sediments, several intriguing oceanographic observations on the distribution and reactivity of DOC have been reported in the deep-water column of the East Sea, including the UB (Kim et al., 2015; Kim and Kim, 2015; Kim and Kim, 2016; Kim T. H. et al., 2017b). In particular, in the deep-water layer (1000 – 3500 m depth), Kim and Kim (2016) found a surprisingly large excess of humic-like fluorescent DOM (FDOMH) that exceeded the production of FDOMH estimated from the apparent oxygen utilization (AOU). Benthic release of refractory DOM produced by anaerobic microbial metabolism in sediments was proposed as potential source of this excess FDOMH. However, in their earlier oceanographic observations, Kang et al. (2010) found a drastic decrease of dissolved oxygen (DO) in the deep-water layer (i.e., 20 – 200 m above the seafloor) of the UB (Figure 1). This rapid consumption of DO, which is typically observed only in the bottom water layer of the UB, suggests that benthic release of bio-reactive DOM leads to consumption of oxygen. To better explain these intriguing but somewhat conflicting observations and interpretations on the decrease of DO and accumulation of excess refractory FDOMH in the deep-water column, we need to elucidate whether DOM in sediment has bio-reactive properties that support aerobic bacterial metabolic activity in the overlying water column.
Based on the high labile Corg content and benthic Corg mineralization rates (Lee et al., 2008; Hyun et al., 2017; Lee et al., 2019), in combination with the high primary production and export flux of labile Corg in the UB (Kim et al., 2009; Joo et al., 2014), we hypothesized that the DOM in pore water would contain bio-reactive DOM. Then, the benthic release of this bio-reactive DOM would stimulate microbial metabolic activity in overlying deep water, which is ultimately responsible for oxygen consumption and transformation of bio-reactive SDOM to a further recalcitrant form that accumulates as excess FDOMH in the deep-water column of the UB. The primary objective of this paper was to demonstrate the bio-reactive properties of SDOM to elucidate microbially mediated cause-effect relationships associated with the consumption of DO and accumulation of FDOMH in the bottom water column of the UB. We also addressed bacterial parameters (i.e., abundance and production) in the deep-water column of the UB.
Materials and Methods
Study Area
The UB located in the southwestern part of the East Sea is a bowl-shaped deep basin (2000 – 3000 m depth) (Figure 1) surrounded by the continental slope of the Korean peninsula and the southwestern Japanese archipelago to the west and south, respectively. It is delimited by the Korea Plateau and the Oki Bank on the north and east, respectively (Chough et al., 2000). The northern and eastern margins of the UB are characterized by a steep slope (> 10°) compared to those of the southern and western margins (1 – 2°) (Lee et al., 2016).
Annual mean primary production (PP) in the UB (280 g C m-2 yr-1, Joo et al., 2014) is higher than that measured in other regions of the East Sea (Yamada et al., 2005). High PP has been ascribed to various physical forces such as wind-driven upwelling along the southeast coast of the Korean peninsula (Hyun et al., 2009; Yoo and Park, 2009), upward nutrient flux via shallowing of the pycnocline (Kwak et al., 2013) and formation of an intrathermocline eddies (Kim et al., 2012). High export flux of phytoplankton-derived labile Corg that accounts for 7 – 56% of PP (average 34% of PP, Kim et al., 2011) and lateral transport of the POC along the continental slope (Lee et al., 2019) are likely to be responsible for the high Corg content and rapid Corg mineralization rate (average 6.2 mmol C m-2 d-1) in surface sediment (Hyun et al., 2017), which is the highest among other deep sea sediments with a similar depth range and comparable to the sediment of Chilean and Peruvian upwelling regions.
In addition to the high Corg content, the sediment of the UB is characterized by very high Mn oxide (> 200 µmol cm−3) and Fe oxide (up to 100 µmol cm−3) contents (Cha et al., 2005; Hyun et al., 2017). As oxygen penetrates less than 4 mm into the sediments, manganese reduction and iron reduction were the dominant Corg mineralization pathways, accounting for 45% and 20%, respectively, of total microbial respiration in the center of the UB. In contrast, sulfate reduction was responsible for 50% of the total microbial mineralization of Corg in the sediment of the continental slope (Hyun et al., 2017).
Sampling
Shipboard microbiological incubation experiments together with measurements of physico-chemical oceanographic parameters were carried out from November 4 to 11, 2020. Seawater and sediment samples were collected aboard the R/V Onnuri of the Korea Institute of Ocean Science and Technology (KIOST) along the southwestern continental slope of the UB (Figure 1). Sampling sites were selected from the upper slope (KU04), lower slope (KU06) and at the center (KU10) of the UB with water depths of 1238 m, 1759 m, and 2176 m, respectively (Figure 1). The bottom layer of the KU04 and KU06 is characterized by lateral transport of resuspended sediments along the continental slope (Lee et al., 2019), whereas near-bottom current fluctuations prevail in the center of the UB (KU10) (Chang et al., 2009; Kim et al., 2013).
Seawater samples for chemical and microbiological analyses were collected at the 3 stations (KU04, KU06 and KU10) using Niskin bottles attached to a rosette sampler. The sample bottles were first washed with 10% HCl and rinsed with Milli-Q water. The hydrographic conditions including temperature, salinity, DO (SBE 43), and turbidity (C-star transmissometer) were measured using a CTD sensor (SBE 911 Plus, Seabird Electronics). Water samples were collected at 10 – 12 designated water depths based on the vertical temperature profiling including the bottom water layer, approximately 15 – 30 m above the seafloor (Table 1). Sediment samples for extracting pore water were collected at 2 stations (KU04 and KU10) using a box corer. Onboard, pore water was extracted from acrylic sub-cores (7.5 cm in diameter) using Rhizon soil moisture samplers (Rizosphere Research Products, Wageningen, The Netherlands) that were washed with acid (10% HCl) and rinsed with Milli-Q water.

Table 1 Mean (± 1 standard deviation) of bacterial production (BP), bacterial abundance (BA), and concentrations of dissolved organic carbon (DOC) and C1 component (C1) and C3 component (C3) of humic-like DOM (FDOMH) in the water column at a designated depth range from the seafloor.
DOC and FDOM in Sea Water and Pore Water
Samples for DOC analysis were filtered through a pre-combusted (500°C for 5 h) GF/F filter (0.7-μm pore size; Whatman) and acidified using 6M HCl (pH ~2) to prevent any bacterial activity, followed by hermetic sealing in pre-combusted (500°C for 5 h) 20 mL glass ampoules and preserved at room temperature until analysis in the laboratory. DOC concentrations were determined via a high-temperature catalytic oxidation (HTCO) using a total organic carbon (TOC) analyzer (TOC-L, Shimadzu Co.). Measurements were verified using deep sea reference (DSR) material (~ 43 μM for DOC; University of Miami; Hansell, 2005). The instrument signal and the signal linearity were calibrated using potassium hydrogen phthalate (KHP) standard solutions every day. The precision of DOC measurement was about ± 2 μM based on multiple analyses of DSR material.
Fluorescent dissolved organic matter (FDOM) samples were filtered through a pre-combusted (500°C for 5 h) GF/F filter (0.7 µm pore size; Whatman) and stored in pre-combusted dark amber vials at 4°C until analysis. Excitation-emission matrix spectroscopy (EEMs) analysis of FDOM components was performed using a spectrofluorometer (Aqualog, Horiba). Emission and excitation wavelengths were set as 250 to 600 nm and 250 to 500 nm, respectively, with 5 nm intervals and an integration time of 3 sec. The parallel factor analysis (PARAFAC) model was applied to characterize major FDOM components of all collected samples (Bro, 1997; Stedmon and Bro, 2008). Raman and Rayleigh scattering signals, inner-filter effect, and blank subtraction were corrected using Solo software (Eigenvector, 108 Inc.). All FDOM components were validated using split-half analysis by constraining non-negativity for all components. Fluorescence intensities were normalized with the Raman peak area of Milli-Q water (18.2 MΩcm) and expressed as Raman units (R.U.) (Lawaetz and Stedmon, 2009). Spectral shapes of FDOM components were compared with previous results based on the OpenFluor database (https://openfluor.lablicate.com) (Murphy et al., 2014). All FDOM components were well matched with the major components from 12, 11, and 2 previous studies, respectively, with ≥ 95% of similarity.
Microbiological Parameters
Samples for enumerating bacterial abundance (BA) were preserved with 25% glutaraldehyde (final concentration, 1%) and stored in a freezer at -20°C (Hyun and Yang, 2003). The samples were stained with 4’ 6-diamidino-2-phenyl-indole (DAPI, Porter and Feig, 1980), filtered through a Nuclepore filter (0.2-µm pore size, black), and mounted on slide glass with immersion oil (Cargille type A). Bacterial cells were counted using an epifluorescence microscope (Nikon, Eclipse 80i, Tokyo, Japan) equipped with a mercury lamp (HB-10101 AF), an ultraviolet (UV) excitation filter, and a BA 420 Barrier filter.
Heterotrophic bacterial production (BP) was estimated using the microcentrifuge method for 3H-leucine (3H-leu) incorporation into protein (Smith and Azam, 1992). Duplicate 1.5 mL water samples were incubated in the dark with 3H-leu (final concentration, 10 nM leucine, Perkin Elmer, NET1166005) at in situ water temperature for one hour in 2 ml sterilized microcentrifuge tubes. Incubation was terminated by the addition of 50% cold trichloroacetic acid (TCA), and the samples stood at room temperature for 30 min prior to centrifugation. Killed control samples were treated with 50% cold TCA before the addition of 3H-leu, and incubated for one hour in the dark. The tubes were centrifuged for 10 min at 14,000 rpm and aspirated. Samples were extracted with 80% ethanol after precipitating and washing with 5% cold TCA. Liquid scintillation cocktail (1.5 ml; Lumagel Safe, Lumac-LSC) was added directly to the microcentrifuge tube, and the activity of the cold TCA insoluble macromolecules was determined using a liquid scintillation counter (LKB, Rack Beta II). Rates of incorporated leucine (pmol leu L-1 h-1) were converted to BP (μmol C m-3 d-1) using a standard conversion factor (CF = 1.5 kg C mol leucine-1; Kirchman, 1993).
Pore Water Amended Incubation Experiments
To directly determine the effect of SDOM on bacterial metabolic activity in deep water (i.e., to demonstrate the bioavailability of SDOM), an incubation experiment was performed by mixing the pore water with overlying bottom water. Bottom water samples were collected at a depth of 1,224 m (KU04) and 2,147 m (KU10), approximately 15 m and 30 m above the seafloor, respectively. Pore water extracted from surface sediment (0 – 5 cm depth) was mixed with the bottom water in a 4 L carboy (pore water to bottom water ratio of 1:9, v/v). Since the accumulation of fresh marine detrital organic matter would be limited to surface sediment (Rossel et al., 2016), we collected pore water within 5 cm of the sediment. Mixed waters were then divided evenly into 2 L polycarbonate bottles for duplicate treatments. Unamended bottom water was also placed into 2 L polycarbonate bottles. Incubation was performed in the dark at in situ temperature for 5 days. Duplicate sub-samples for measuring bacterial production and variation in FDOMH concentrations were collected daily.
Results
Physical Settings of the Deep-Water Column
In the water column with a depth >1000 m, temperature ranged from 0.22 to 0.36°C, and salinity was distributed consistently (34.07 psu) with depth (Figures S1A, C, E). In contrast to the temperature and salinity, concentrations of DO and beam attenuation coefficient (i.e., a proxy for turbidity) showed notable variations in the bottom layer (Figures S1B, D, F). DO was distributed relatively consistently at depths below 750 m but decreased abruptly in the bottom water column (50 – 100 m above the seafloor) (Figures S1B1, D1, F1). In contrast, the beam attenuation coefficient increased in the bottom water layer, which coincided with a rapid DO decrease in the bottom water column.
DOC and Humic-Like FDOM
Average DOC concentrations in the surface (0 – 100 m depth) and subsurface water column (200 – 500 m depth) were 66 ± 6 μM and 58 ± 3 μM, respectively (Figure 2). DOC in the deep-water column (i.e., 750 – 2000 m water depth), approximately 30 – 500 m above the seafloor, ranged from 51 to 63 μM (average 55 μM, Table 1). DOC near the bottom layer appeared to be highly variable: it showed a slight decrease with increasing depth at KU04 and KU10, but increased in the bottom layer at KU06 (Figures 2A1, C1, E1).
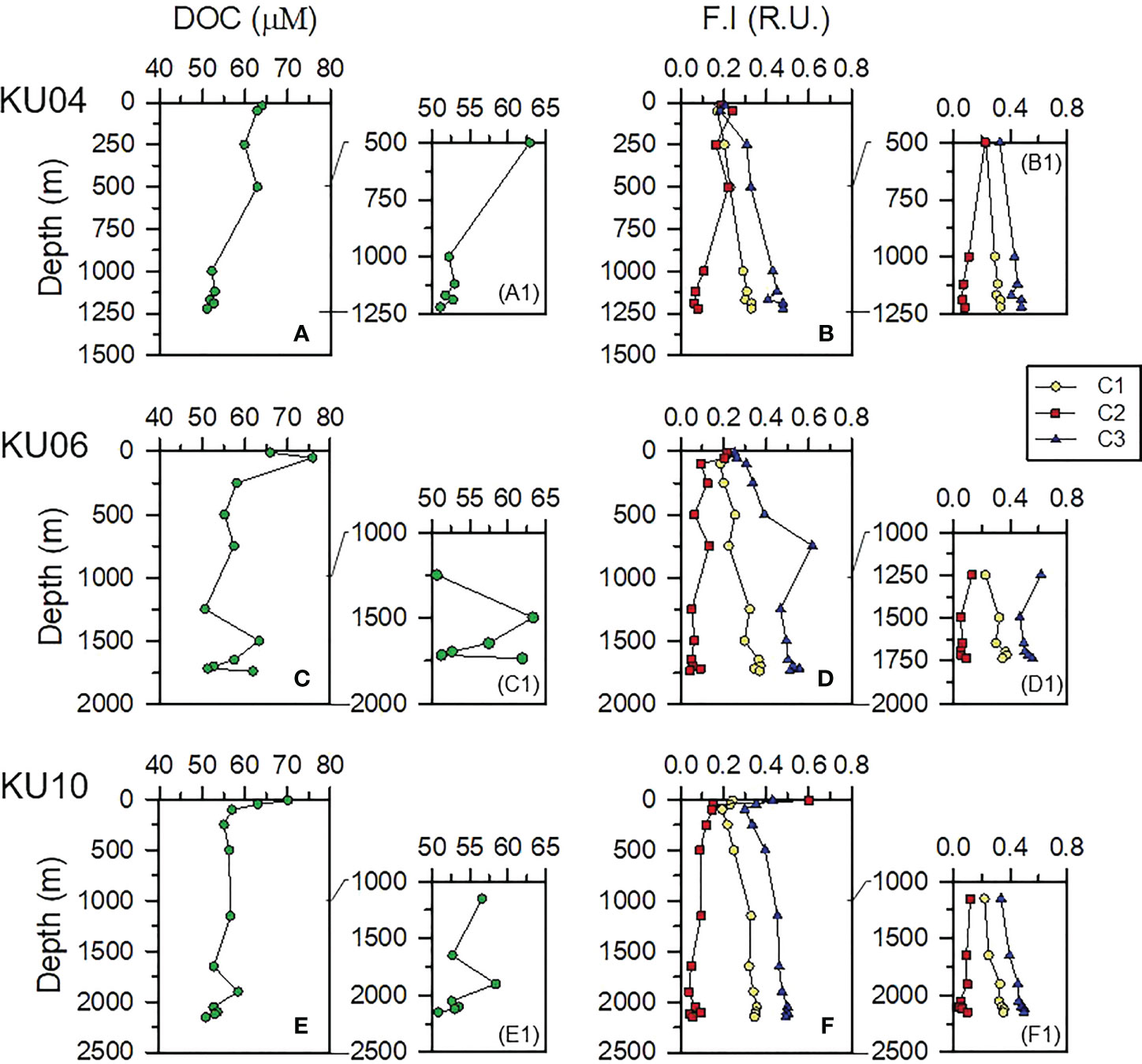
Figure 2 Vertical distribution of dissolved organic carbon (DOC; A–E1) and fluorescence intensity (F.I., B–F1) of the three components (C1, C2 and C3) defined as fluorescent dissolved organic matter (FDOM) in the water column of the UB.
The PARAFAC model identified three components of FDOM (Figure 3): Components 1, 2, and 3 showed excitation and emission spectra (Ex/Em) at 375/442 nm, 260/298 nm, and 280, 345/337 nm, respectively (Figure 3). Component 1 (C1; Ex/Em = 375/442 nm) is characterized as a terrestrial humic-like component, which not only originates from terrestrial environments, but is also produced in situ by phytoplankton (Fukuzaki et al., 2014; Osburn et al., 2015). Component 2 (C2; Ex/Em = 260/298 nm) is identified as the tryptophan-like component, which is mainly derived from freshly produced organic matter (Yamashita and Tanoue, 2003). Component 3 (Ex/Em = 280,345/337 nm) is also produced from microbial degradation of plankton-origin organic matter in the ocean (Yamashita et al., 2008). The concentrations of C1 and C3 components increased with increasing depth at both sites, whereas C2 component decreased with increasing depth (Figures 2B, D, F).
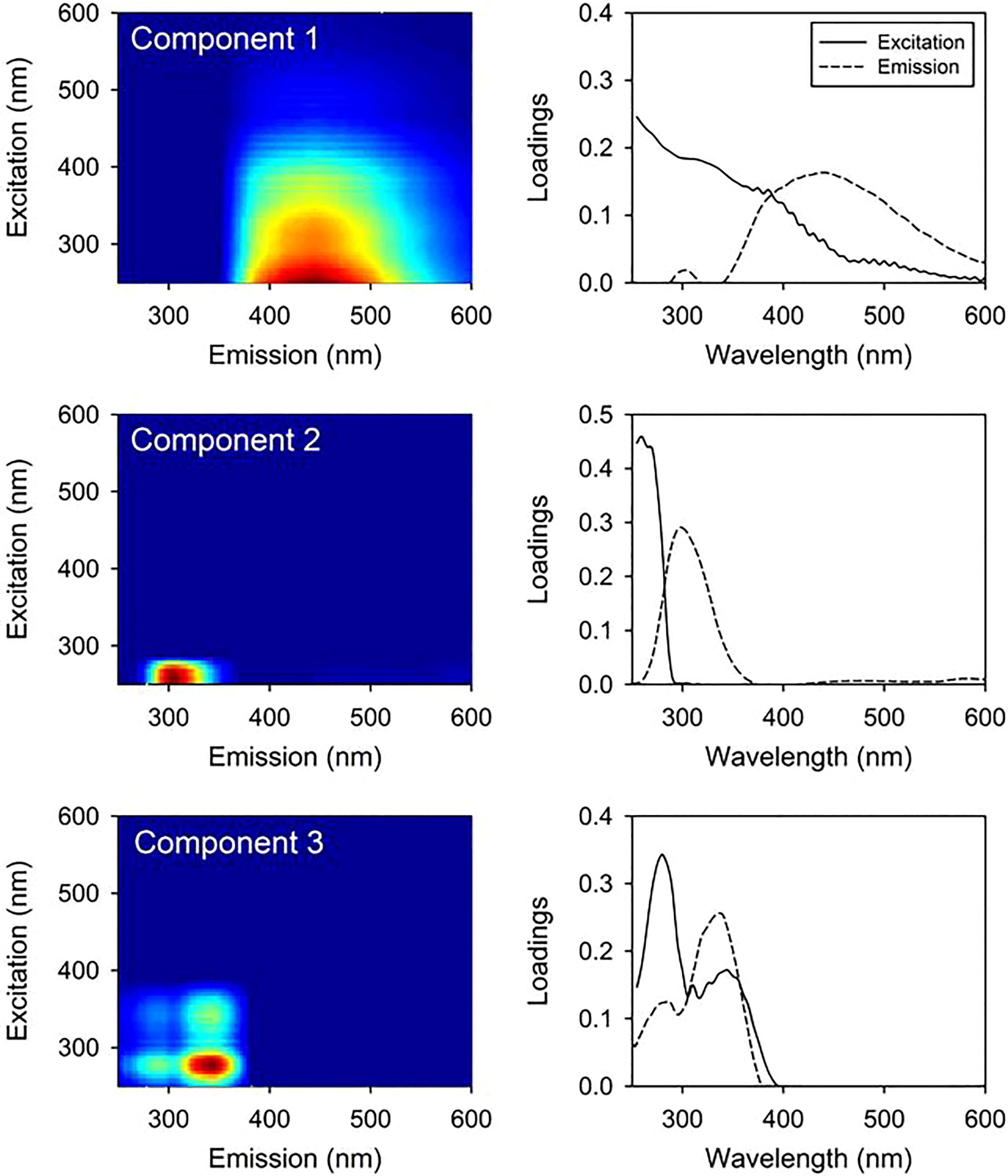
Figure 3 Excitation–emission matrix spectroscopy (EEMs) contour plots and loadings of three components (Component 1, Component 2, and Component 3) identified using the Parallel Factor Analysis (PARAFAC) model. The solid line indicates the emission wavelength, and the dotted line indicates the excitation wavelength.
In pore water, concentrations of DOC (710 ± 314 μM) in the surface sediment (0 – 4 cm; Table S1) were more than 13-fold greater than the DOC in overlying bottom water (55 μM, Table 1). The PARAFAC model on pore water identified C1 and C3 components in the FDOMH. The fluorescence intensity of C1 (7.2 ± 2.7 R.U.) and C3 (3.8 ± 3.3 R.U.) components in pore water of the surface sediment (0 – 4 cm) (Figure 4 and Table S1) was 20-fold and 8-fold, respectively, higher than that measured in the bottom water layer (Table 1).
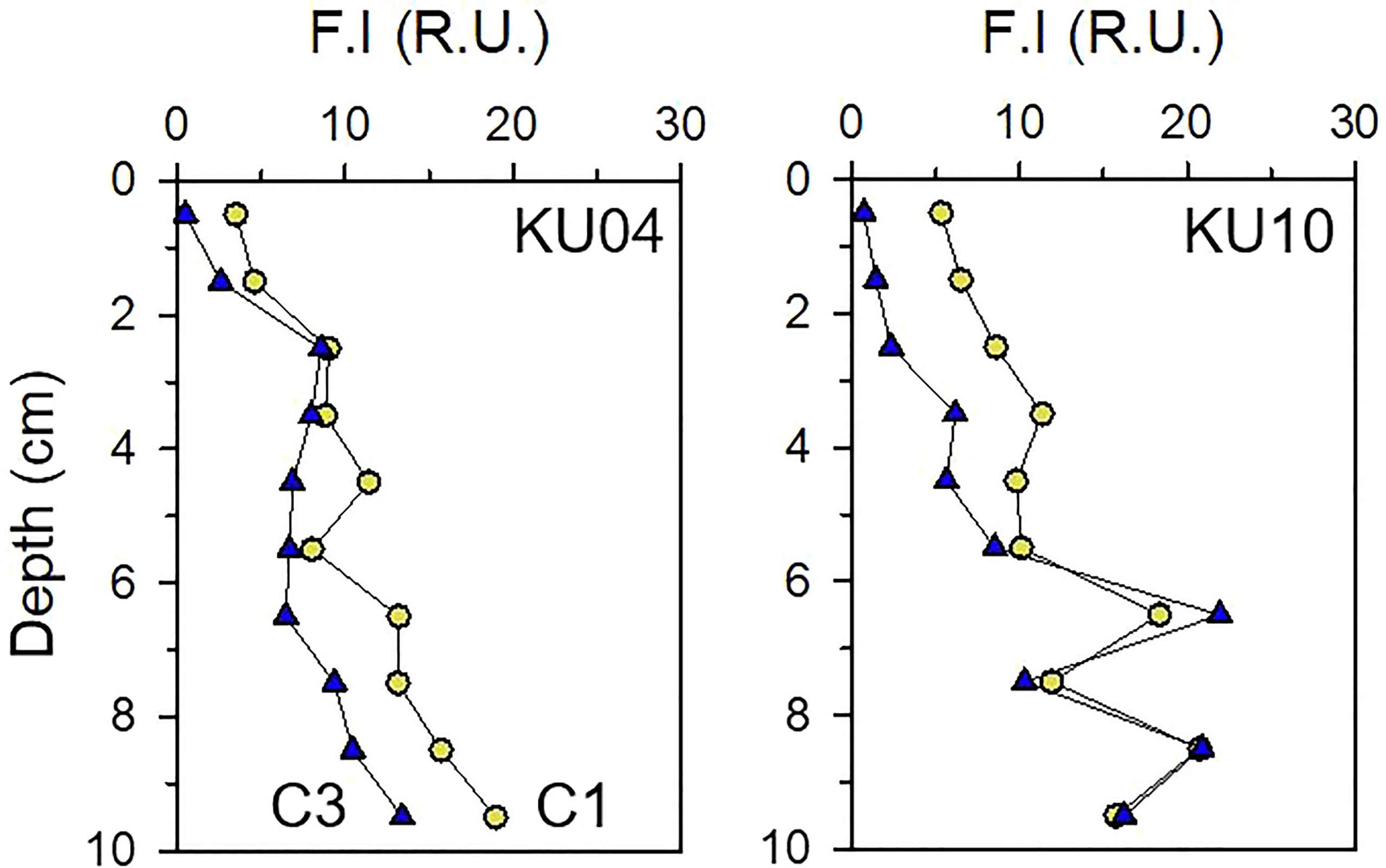
Figure 4 Vertical profiles of fluorescence intensity of FDOMH (C1 and C3 components) in pore water at the slope site (KU04) and basin site (KU10).
BA and BP in the Deep-Water Column
Heterotrophic bacterial abundance (BA) and bacterial production (BP) below the surface mixed layer (< 200 m depth) decreased exponentially with depth (Figure 5). The BA approximately 30 – 500 m above the seafloor (i.e., 750 – 2000 m water depth) ranged from 0.5 – 1.8 × 105 cells mL-1 (average 1.1 × 105 cells mL-1). The BP in the same deep-water column ranged from 1.67 – 3.33 μmol C m-3 d-1 (average 2.2 μmol C m-3 d-1). Interestingly, enhanced BA and BP were observed in the bottom water layer (Table 1 and Figure 5). Mean BP and BA in bottom water near the sediment (i.e., 0 – 30 m above the seafloor; BP = 2.78 μmol C m-3 d-1; BA = 1.50 × 105 cells mL-1) was 1.67 and 1.77 times, respectively, higher than that measured above that (i.e., 30 – 100 m above the seafloor; BP = 1.67 μmol C m-3 d-1; BA = 0.85 × 105 cells mL-1) (Table 1 and Figure 5).
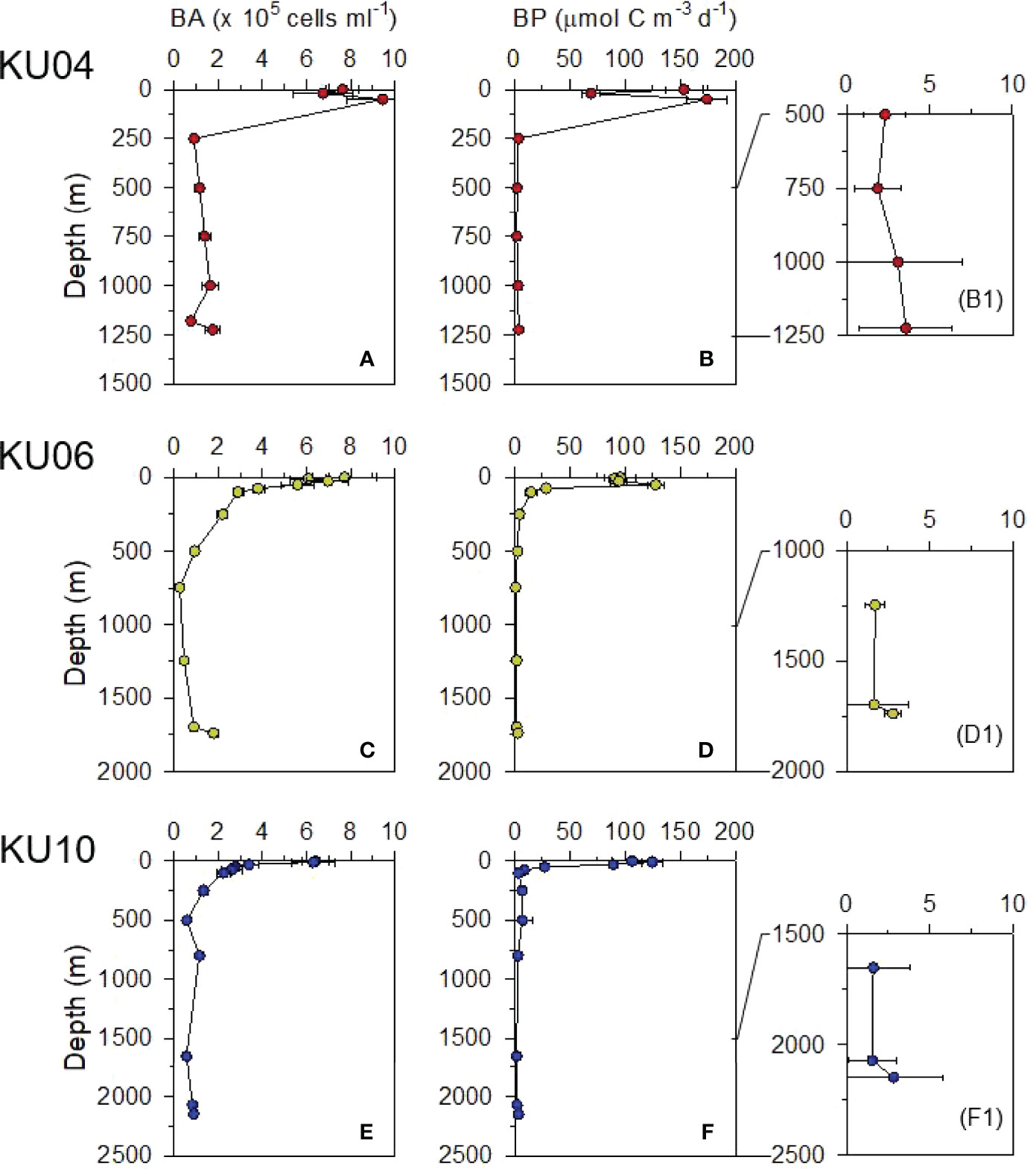
Figure 5 Vertical profiles of bacterial abundance (BA; A, C and E) and bacterial production (BP; B, D and F and B1, D1 and F1) in the water column.
Effects of Sediment-Derived DOM on BP and FDOMH
In the bottom water incubation experiment that was amended with SDOM containing pore water (PW), changes in BP and fluorescence intensity of the C1 component were measured with time. Initial concentrations of DOC (89 – 106 μM) and the C1 component (1.17 – 1.32 R.U.) in PW-amended samples were 1.5-fold and 2-fold, respectively, greater than those measured in unamended bottom water samples (DOC = 63 μM; C1 = 0.52 – 0.61 R.U.), which confirmed that substantial amounts of natural SDOC and FDOMH were added to the bottom water (Table S2).
BP in the PW-amended samples increased from day 4 of incubation, whereas BP in unamended control treatment was invariant during the entire incubation period (Figure 6A). After 5 days of incubation, BP in PW-amended samples reached 8-fold and 6-fold higher than that of the unamended control of the KU04 and KU10, respectively. The results indicated that SDOM contains bio-reactive materials stimulating BP (Figures 5B1, D1, F1) and enhancing BA (Figures 5A, C, E) in the deep-water column near the sediment of the UB. Unlike BP, the C1 component of the FDOMH increased in both PW-amended samples and unamended controls (Figure 6B), which indicated that new FDOM is produced during microbial degradation of DOM derived not only from sediment, but also from various sources in the water column. The increase in C1 component was greatest in PW-amended samples of the slope site (KU04) that received greater DOM from PW (Table S2).
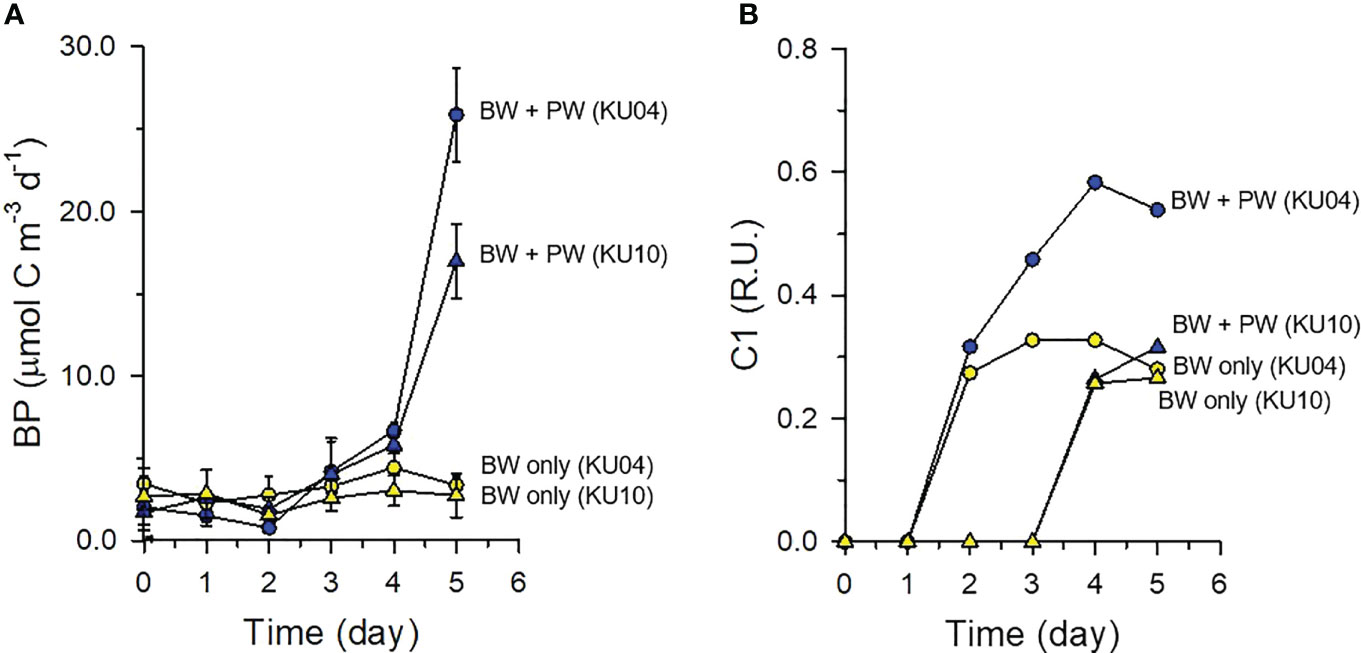
Figure 6 Heterotrophic bacterial production (BP) (A), and normalized fluorescence intensity of component 1 (C1) of humic-like FDOM during the incubation experiment conducted at the slope site (KU4) and basin site (KU10) (B). Normalized fluorescence intensity was calculated by subtracting the fluorescence intensity at each time point from that of time zero.
Discussion
High DOC and BP in Deep-Water Column of the UB
The DOC concentrations in deep-water layer of the UB (55 μM in average, Table 1) were consistent with previous observations reported in the East Sea (58 ± 4 μM; Kim et al., 2015), which is higher than that measured in other major oceans (45 – 50 μM) including N. Pacific, N. Atlantic and the Mediterranean Sea (Santinelli et al., 2010). In general, DOM concentration in the subsurface water column correlates with net community production and convective transport via vertical mixing (Hansell, 2013; Carlson and Hansell, 2015). In the UB, a combination of high water column primary production (280 mg C m-2 yr-1; Joo et al., 2014) and export flux (average 34% of primary production; Kim et al., 2011), as well as the formation of the Ulleung warm eddy that efficiently delivers the POM and DOM to subsurface layer (Hyun et al., 2009; Kim et al., 2012), is likely to be responsible for the accumulation of DOC in the deep-water column.
The BP values (1.67 – 3.33 μmol C m-3 d-1; average 2.2 μmol C m-3 d-1) observed in the deep-water column (> 750 m) (Figure 5) were among the highest reported for various deep-water columns using the same conversion factor of leucine to carbon (see Nagata et al., 2010), including the North Atlantic Basin (0.45 – 1.05 μmol C m-3 d-1; 1000 – 3870 m depth; Reinthaler et al., 2006), the NW African upwelling site (0.25 – 1.85 μmol C m-3 d-1; 2000 m depth; Baltar et al., 2007), and the Mediterranean Sea (0.15 – 0.5 μmol C m-3 d-1; 1000 – 2000 m depth; Tamburini et al., 2002). This high BP in the deep-water column of the UB is likely to be associated with the high DOC concentrations (ca. 55 μM) (Figure 2 and Table 1). Based on the stable isotope analysis, Kim et al. (2015) revealed that DOC in the deep-water column of the East Sea consists of the labile form primarily derived from phytoplankton. In general, temperature and availability of DOC are considered the two major factors controlling heterotrophic bacterial metabolism in the ocean and sediments (Kirchman et al., 2009; Arndt et al., 2013). The high BP despite the low temperature of the UB (< 1 °C) indicated that the DOC inventory is of labile nature to stimulate the metabolic activity of heterotrophic bacteria even at low water temperature.
Enhanced fluorescence intensity of C1 and C3 component with depth (Figure 2) is also likely to be resulted from the microbial degradation of labile DOM in deep-water column. In the aphotic ocean, based on strong correlations with oxygen utilization and nutrient mineralization, this C1 component emitting a wavelength of 400 nm and 440 nm was considered a by-product of heterotrophic bacterial metabolic processes (Kramer and Herndl, 2004; Nieto-Cid et al., 2006; Yamashita and Tanoue, 2008; Jørgensen et al., 2011). Component 3 (Ex/Em = 280,345/337 nm) is also produced from microbial degradation of plankton-origin organic matter in the ocean (Yamashita et al., 2008). In the present study, scatter plots between FDOMH and AOU (Figure S2) revealed that the increase of FDOMH was significantly correlated with the AOU (Figure S2A, C). The results indicated that the C1 and C3 components of the FDOMH in the deep water of the UB is produced by in situ microbial remineralization of relatively labile organic matter in the water column (Jørgensen et al., 2011; Catalá et al., 2015; Kim and Kim, 2016). Spatially, BA and BP were also higher at the upper slope site (KU04; Figure 5B1) than at the lower slope (KU06; Figure 5D1) and the center of the UB (KU10; Figure 5F1), which also suggested that Corg supply via lateral transport along the continental slope is a significant factor supporting heterotrophic bacterial metabolic activity in the deep-water column of the UB.
DOC and FDOMH in Pore Water
High DOC concentration in pore water (710 μM, Table S1) compared to that observed in bottom water (55 μM, Table 1) is largely attributed to the accumulation of low-molecular-weight (LMW) DOC resulting from benthic mineralization of organic matter delivered from the productive overlying water column (Alperin et al., 1999; Burdige and Komada, 2015; Rossel et al., 2016). The higher fluorescence intensity in pore water (Table S1) than that of the overlying water column (Table 1) is mostly associated with the higher concentration of DOC (and hence FDOM) in sediments (Chen and Bada, 1994; Komada et al., 2004; Marchand et al., 2006; Rossel et al., 2016). As the sediment of the UB is characterized by high Corg content (> 2.5% dry wt.) resulting from the high export flux of phytoplankton-derived labile organic matter (Lee et al., 2008) and lateral transport along the continental slope (Lee et al., 2019), substantial amounts of DOC and FDOMH are accumulated in pore water during the degradation of POC to LMW-DOM (Alperin et al., 1999; Chen et al., 2016).
In addition to the vertical and lateral source, high metal reductions are also likely to be responsible for the high DOC concentration in pore water of the UB where surface sediment is enriched with high Mn oxide (> 200 µmol cm−3) and Fe oxide (up to 100 µmol cm−3) (Cha et al., 2005; Hyun et al., 2017). While these hydrous forms of Mn and Fe have a strong capacity to sorb DOM, the DOM-metal oxide complexes are highly vulnerable to reductive dissolution during microbial reduction of Mn and Fe oxides, which ultimately results in a release of DOM into pore water (Chin et al., 1998; Eusterhues et al., 2014; Stuckey et al., 2018). Indeed, Mn and Fe reduction accounted for the 45% and 20%, respectively, of total microbial respiration in basin sediment, and microbial Fe reduction was also responsible for 12% of the total microbial mineralization of Corg in the slope sediment (Hyun et al., 2017). Therefore, the DOM released during the reduction of hydrous Fe and Mn in the Fe- and Mn oxide rich sediment in the UB is likely to be associated with the high DOC concentration in pore water (Chin et al., 1998; Lalonde et al., 2012; Stuckey et al., 2018; Ma et al., 2020; Trainer et al., 2021). Quantitative contribution of the DOM desorbed during the reduction of metal oxides to DOM inventory in pore water remains to be estimated in the UB.
Enhanced BP Near the Sediment Surface and Benthic DOC Flux
Mean BP and BA in bottom water near the sediment (i.e., 0 – 30 m above the seafloor; BP = 2.78 μmol C m-3 d-1; BA = 1.50 × 105 cells mL-1) was 1.67 and 1.77 times, respectively, higher than that measured above that (i.e., 30 – 100 m above the seafloor; BP = 1.67 μmol C m-3 d-1; BA = 0.85 × 105 cells mL-1) (Table 1 and Figure 5). Based on the DOC and C1 and C3 components of the FDOMH in pore water that was 13-, 20- and 8-fold, respectively, higher than that of the bottom water (Figure 2 and Table 1), it was speculated that substantial amounts of DOM produced in sediment were released into the overlying water, and that certain fractions of the SDOM stimulated the BP (Skoog et al., 1996; Cai et al., 2019; Tobias-Hünefeldt et al., 2021).
In terms of benthic release of DOC, Burdige et al. (1999) derived an empirical equation for the relationship between benthic DOC flux (BDF) and benthic Corg mineralization rate (BDF = 0.36 × Cox0.29; Cox = benthic Corg oxidation rate), and reported that BDF accounts for approximately 9.5% of benthic Corg mineralization in continental margins. In the UB, the benthic Corg mineralization rate reported at the same sampling sites as the present study site was 8700 and 3660 μmol C m-2 d-1 at the slope site (KU04) and the basin site (KU10), respectively (Hyun et al., 2017). Therefore, using an average benthic mineralization rate of 6180 μmol C m-2 d-1, the BDF calculated according to Burdige et al. (1999) was 600 μmol C m-2 d-1. If we adopt the bacterial growth efficiency (BGE) of 2% (Reinthaler et al., 2006) that was derived from the deep water of the North Atlantic Basin, depth integrated bacterial carbon demand (BCD = BP/BGE = 2.78 μmol C m-3 d-1/0.02) near the sediment surface (Table 1) was estimated to be 138 μmol C m-2 d-1. Therefore, benthic DOC flux in the UB (average 600 μmol C m-2 d-1) accounted for 434% of the BCD near the surface sediment. Lateral transport along the slope (Lee et al., 2019) and near-bottom current prevailing in the center of the UB (Chang et al., 2009; Kim et al., 2013) are likely to stimulate sediment resuspension (Kim et al., 2017), which ultimately supply DOM in sediment into overlying bottom water. Quantification and/or fractionation of bio-available DOM in the sediment is not feasible at this moment. However, high BP and BA in bottom water near the sediment surface (Figure 5), together with high benthic DOC flux, strongly indicated that the SDOC contains certain fractions of bio-reactive DOC, thereby stimulating heterotrophic bacterial metabolism and rapid oxygen consumption in bottom water near the sediment. The bioavailability of SDOM is further discussed in next section.
Bioavailability of Sediment-Derived DOM and Production of New FDOM
Heterotrophic microbes act as both consumers and producers of DOC in the ocean carbon cycle, in which most of the DOC taken up by bacteria is respired to CO2, but a certain fraction is transformed into refractory DOC with low molecular weight (Ogawa et al., 2001; Jiao et al., 2010; Benner and Amon, 2015; Wear et al., 2020). In the present study, the scatter plot between FDOMH and AOU further revealed that excess FDOMH beyond what is supported by the estimated AOU was observed in the deep-water column (i.e., 750 m – bottom) (Figure S2B, D). Excess FDOMH is often observed in various deep waters such as the Central Pacific Ocean, the Southern Ocean, and the East Sea (Hayase and Shinozuka, 1995; Yamashita et al., 2007; Tanaka et al., 2014; Kim and Kim, 2016). The accumulation of this excess FDOMH in the deep layer of the UB was consistent with the previous observation reported for the entire East Sea (Kim and Kim, 2016). Kim and Kim (2016) proposed that excess FDOMH with refractory properties was produced anaerobically in the sediment. In the present study, however, the high DOC and FDOMH concentrations in pore water (Figure 4) and the enhanced BA and BP (Figure 5) that accompanied a rapid decrease of DO (Figure S1) and accumulation of excess FDOMH in the turbid bottom water layer (Figure S2) implied that bio-reactive DOM released by the sediment resuspension stimulated heterotrophic bacterial metabolism at the expense of oxygen, producing new recalcitrant DOM (i.e., FDOMH) in the deep-water column of the UB.
To explore this hypothesis, we conducted an incubation experiment in which the DOM extracted from surface sediment was amended with the overlying bottom water to elucidate: (1) whether SDOM directly stimulates bacterial production in overlying deep water, and (2) whether new FDOMH is produced during the bacterial utilization of SDOM. Several lines of evidence obtained from the PW-amended incubation experiment support our hypothesis (Figure 6). First, the increase in BP in the PW-amended incubations (Figure 6A) clearly indicated that SDOM contains bio-reactive materials stimulating BP (Figures 5B1, D1, F1) and enhancing BA (Figures 5A, C, E) in the deep-water column near the sediment of the UB. Although the detailed composition of DOM in pore water and bottom water remains unclear (Arrieta et al., 2015; Burdige and Komada, 2015; Jiao et al., 2018), several previous research on deep sea sediments revealed that peptides, unsaturated aliphatics, and saturated fatty acids are prevalent in the sediment underlying productive water columns (Arndt et al., 2013; Rossel et al., 2016; Niggemann et al., 2017). These results may provide an example of the priming effect (Guenet et al., 2010; Shen and Benner, 2018; Wear et al., 2020), in which input of labile DOM tends to increase the mineralization of the more recalcitrant DOM in continental margins. Our results of enhanced heterotrophic BP also indicated that the benthic release of bio-reactive SDOM is presumably responsible for the rapid consumption of oxygen in the bottom water column (Figures 1, S1) by stimulating aerobic microbial respiration in the overlying deep-water column.
Secondly, the C1 component of the FDOMH increased in both PW-amended samples and unamended controls, although the increase was greater in PW-amended samples (Figure 6B). The production of the C1 component, by-product of microbial degradation of SDOM (Nieto-Cid et al., 2006; Yamashita and Tanoue, 2008; Jørgensen et al., 2011), in the PW-amended bottom water (Figure 6B) confirmed that benthic release of semi-labile DOM and its subsequent microbial degradation may play a significant role in re-generating DOM with different lability (i.e., more recalcitrant DOM) in the bottom water of the UB. An accumulation of C1 component even in unamended control incubations (i.e., without PW addition) (Figure 6B) further indicated that refractory DOM is produced autochthonously during microbial oxidation of DOM derived from various sources in the deep-water column (Yamashita and Tanoue, 2008; Jiao et al., 2010) even without a supply of SDOM. The persistence of newly produced FDOMH until the end of incubation (Figure 6B) also suggested that some FDOMH is resistant to microbial degradation, and thus accumulates in the water column. These results explain microbially mediated accumulation of excess FDOMH in the deep-water column of the UB (Figure S2, Kim and Kim, 2016). This microbially mediated production of new FDOM (Figure 6B) is consistent with previous incubation experiments amended with various organic resources (Nelson et al., 2004; Benner and Amon, 2015; Wear et al., 2020), and likely to support the formation of RDOC via microbial carbon pump in deep ocean (Jiao et al., 2010).
Interestingly, in PW-amended samples, the increase of the C1 component began after 1 day of incubation (i.e., 3 days earlier than the enhancement of BP), and this increase was observed even in unamended samples (i.e., without an increase in BP) (Figure 6B). These results imply that new FDOMH is generated during bacterial respiration (i.e., energy yielding catabolic activity). Although bacteria cannot produce new cells using the SDOM at the early time of incubation, they still metabolize SDOM to generate the energy necessary to maintain the cell (Carlson and Hansell, 2015). The increase of both BP and C1 component was less conspicuous at the basin site (KU10) than at the slope site (KU04) (Figure 6), which suggested a greater supply of organic matter along the continental slope and its subsequent accumulation in the sediment. Accordingly, benthic mineralization rates at the slope site (17.1 mmol C m-2 d-1) were approximately two-fold higher than those measured at the basin site (9.2 mmol C m-2 d-1) (Hyun et al., 2017). Overall, the results of the PW-amended incubation experiment suggested that the SDOM containing bio-reactive forms supports high BP in overlying deep water, which ultimately plays a significant role in microbially mediated consumption of DO and regeneration of new FDOMH with refractory properties in the deep-water column of the UB. Our results also indicated that the sediment along the continental slope is a significant source of bio-reactive DOC that stimulates heterotrophic bacterial metabolism and controls the DOM inventory in the ocean.
Microbially Mediated Dynamics of DOM in Deep-Water Column of the UB
In the present study, fine-scale water sampling in the deep water revealed that: (1) BP in the deep-water column of the UB was among the highest values reported in similar water depth ranges of various oceanographic sites, and (2) BP in the deep bottom layer (i.e., near the sediment surface) was notably higher than that of the water column above. By combining these major results obtained from the present study with previously reported oceanographic observations such as rapid DO consumption and high DOC concentrations, and the accumulation of excess FDOMH (i.e., RDOM) in the deep-water column, we propose a schematic diagram of microbially mediated biogeochemical cycles of DOM in the deep-water column of the UB (Figure 7), which addresses a cause-effect relationship for the distribution of DO, DOC and FDOMH in the deep-water column of the UB.
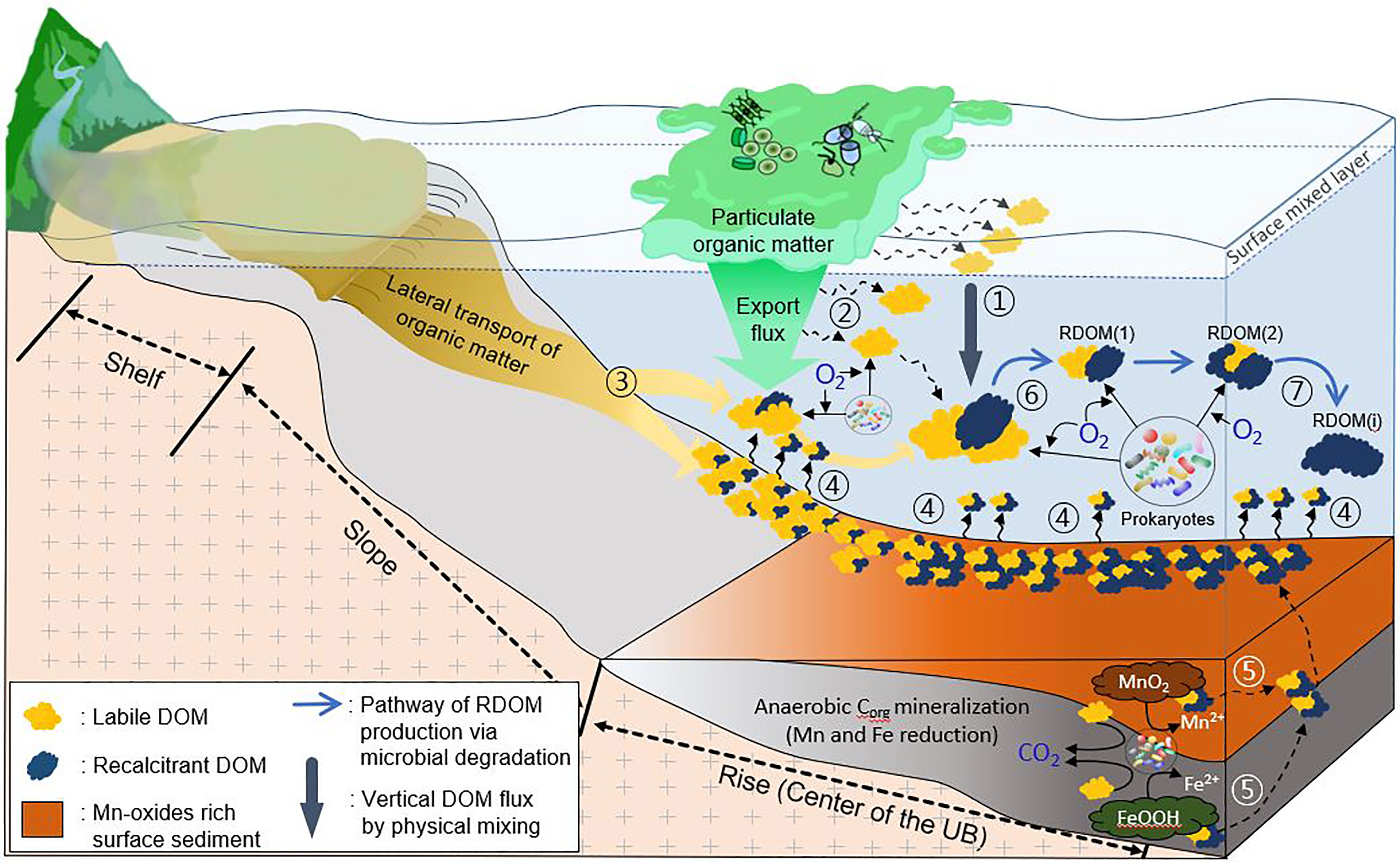
Figure 7 Schematic summary of microbially mediated cycles of DOM in the deep-water column of the UB. Each step (circled number) is described in the text. The present study deals with steps 4, 6 and 7, and also proposes the potential significance of step 5.
Regarding the high DOC concentrations in the deep-water column, the labile DOM released from the POM in the surface water column is delivered to the deep layer via vertical mixing (Kim et al., 2015) (step 1). DOM is also released into the deep-water column from the POM during export flux of POM to the subsurface water column (Kim et al., 2009; Kim et al., 2011) (step 2). Additional DOM is supplied to the deep water via lateral transport of POM along the continental slope (Lee et al., 2019) (step 3). In the present study, high DOC and FDOMH concentrations in pore water (Figure 4) also indicated that a substantial amount of DOM is released from the sediment to the overlying deep water (step 4). DOM released by reduction of Fe and Mn in Fe- and Mn-oxide rich sediment (Hyun et al., 2017) is a potentially significant source of bio-reactive DOM in the overlying water column (step 5), which remains to be estimated in the UB.
The DOM supplied from diverse sources (steps 1, 2, 3, 4 and 5 in Figure 7) ultimately stimulates heterotrophic bacterial metabolism in the deep-water column including the bottom water layer near the sediment surface (Figure 5). In particular, the SDOM containing bio-reactive forms is responsible for the enhanced BP (Figure 6A) and rapid consumption of DO in deep bottom water (Figures 1, S1B, D, F) observed in the UB (step 6). On the other hand, the accumulation of the C1 component, a by-product of microbial degradation of DOM, in both PW-amended and unamended bottom water incubation experiments (Figure 6B) implied that new FDOM with more recalcitrant properties is produced during microbial oxidation of Corg derived from sediment and various sources in the water column, and then accumulates as excess FDOMH in the deep-water layer of the UB (Figure S2) (step 7). The relative contribution of sediment-derived recalcitrant DOM (step 4) and the recalcitrant DOM autochthonously produced by microbial metabolism in deep water (step 7) to excess FDOMH in the deep-water column of the UB remains to be evaluated.
Conclusion
We addressed the effect of SDOM on the microbially mediated dynamics of DOM in overlying deep-water column of the UB. A combination of oceanographic survey and bottom water incubation experiment amended with pore water revealed that the sediment along the continental margins is a significant source of DOM for the overlying water column, and the SDOM contains bio-reactive forms of DOM that stimulate heterotrophic microbial metabolism at the expense of oxygen in the bottom water of the UB. Consequently, the results suggest that the benthic release of bio-reactive DOM may be of widespread significance in controlling microbial processes in the deep-water column of marginal seas. To better understand carbon cycles in highly productive marginal seas, in addition to the quantification and fractionation of bio-available DOM in the sediment and water column, comprehensive studies are needed on the microbial communities that are directly responsible for the consumption of labile DOC and production of recalcitrant DOC with diverse reactivity in the sediment and water column.
Data Availability Statement
The original contributions presented in the study are included in the article/Supplementary Material. Further inquiries can be directed to the corresponding author.
Author Contributions
J-HH and BK designed the study. BK, HH, Y-JB, HL and HC collected the samples and performed most laboratory analysis. JH-H wrote the manuscript with contributions and comments from all co-authors. S-HY and GK significantly improved earlier version of the manuscript. All authors contributed to the article and approved the submitted version.
Funding
This work was supported by the projects titled “Deep-Water Circulation and Material Cycling in the East Sea” and "Predictable Effects of Climate Change on Marine Ecosystem in The Tsushima Warm Current System of Korean Waters" funded by the Korean Ministry of Oceans and Fisheries, and partly by the National Research Foundation (NRF) grant funded by the Korean Ministry of Science and ICT (NRF-2018R1A2B2006340) and Development of Assessment Technology on the Structure Variations in Marine Ecosystem (R2021068).
Conflict of Interest
The authors declare that the research was conducted in the absence of any commercial or financial relationships that could be construed as a potential conflict of interest.
Publisher’s Note
All claims expressed in this article are solely those of the authors and do not necessarily represent those of their affiliated organizations, or those of the publisher, the editors and the reviewers. Any product that may be evaluated in this article, or claim that may be made by its manufacturer, is not guaranteed or endorsed by the publisher.
Supplementary Material
The Supplementary Material for this article can be found online at: https://www.frontiersin.org/articles/10.3389/fmars.2022.826592/full#supplementary-material
Supplementary Figure 1 | Vertical distribution of temperature (T), salinity (S), dissolved oxygen (DO) and turbidity measured by beam attenuation (B). Note that the DO concentrations in the profile represent instrumental readings that were not corrected with the concentration measured by the Winkler titration method. Nonetheless, contrasting patterns showing a steep decrease and increase in DO and turbidity, respectively, are still evident in the bottom water column.
Supplementary Figure 2 | Scatterplots of FDOMH fluorescence against apparent oxygen utilization (AOU) at the slope site (KU04 and KU06) and basin site (KU10) below 120 m depth. Note the excess FDOMH in the deep-water column (the small box in panels A, C) that exceeds the production of FDOMH estimated from AOU.
References
Alperin M. J., Martens C. S., Albert D. B., Suayah I. B., Benninger L. K., Blair N. E., et al. (1999). Benthic Fluxes and Porewater Concentration Profiles of Dissolved Organic Carbon in Sediments From the North Carolina Continental Slope. Geochim. Cosmochim. Acta 63 (3-4), 427–448. doi: 10.1016/S0016-7037(99)00032-0
Arístegui J., Gasol J. M., Duarte C. M., Herndld G. J. (2009). Microbial Oceanography of the Dark Ocean's Pelagic Realm. Limnol. Oceanogr. 54 (5), 1501–1529. doi: 10.4319/lo.2009.54.5.1501
Arndt S., Jørgensen B. B., Larowe D. E., Middelburg J. J., Pancost R. D., Regnier P. (2013). Quantifying the Degradation of Organic Matter in Marine Sediments: A Review and Synthesis. Earth Sci. Rev. 123 (4), 53–86. doi: 10.1016/j.earscirev.2013.02.008
Arrieta J. M., Mayol E., Hansman R. L., Herndl G. J., Dittmar T., Duarte C. M. (2015). Dilution Limits Dissolved Organic Carbon Utilization in the Deep Ocean. Sci. J. 348 (6232), 331–333. doi: 10.1126/science.1258955
Baltar F., Arístegui J., Gasol J. M., Hernández-León S., Herndl G. J. (2007). Strong Coast–Ocean and Surface–Depth Gradients in Prokaryotic Assemblage Structure and Activity in a Coastal Transition Zone Region. Aquat. Microb. Ecol. 50 (1), 63–74. doi: 10.3354/ame01156
Benner R., Amon R. M. (2015). The Size-Reactivity Continuum of Major Bioelements in the Ocean. Annu. Rev. Mar. Science 7, 185–205. doi: 10.1146/annurev-marine-010213-135126
Bro R. (1997). PARAFAC. Tutorial and Applications. Chemometr Intell. Lab. 38, 149–171. doi: 10.1016/S0169-7439(97)00032-4
Burdige D. J. (2002). ““Sediment Pore Waters,”,” in Biogeochemistry of Marine Dissolved Organic Matter. Eds. Hansell D. A., Carlson C. A. (Amsterdam: Academic Press), 612–653.
Burdige D. J., Berelson W. M., Coale K. H., McManus J., Johnson K. S. (1999). Fluxes of Dissolved Organic Carbon From California Continental Margin Sediments. Geochim. Cosmochim. Acta 63 (10), 1507–1515. doi: 10.1016/S0016-7037(99)00066-6
Burdige D. J., Komada T. (2015). ““Sediment Pore Waters,”,” in Biogeochemistry of Marine Dissolved Organic Matter, 2nd Edn. Eds. Hansell D. A., Carlson C. A. (Amsterdam: Academic Press), 535–577. doi: 10.1016/B978-0-12-405940-5.00012-1
Cai R., Zhou W., He C., Tang K., Guo W., Shi Q., et al. (2019). Microbial Processing of Sediment-Derived Dissolved Organic Matter: Implications for Its Subsequent Biogeochemical Cycling in Overlying Seawater. J. Geophys. Res. G: Biogeosci. 124 (11), 3479–3490. doi: 10.1029/2019jg005212
Carlson C. A., Hansell D. A. (2015). ““DOM Sources, Sinks, Reactivity, and Budgets”,” in Biogeochemistry of Marine Dissolved Organic Matter, 2nd Edn. Eds. Hansell D. A., Carlson C. A. (Amsterdam: Academic Press), 65–126. doi: 10.1016/B978-0-12-405940-5.00003-0
Catalá T. S., Reche I., Fuentes-Lema A., Romera-Castillo C., Nieto-Cid M., Ortega-Retuerta E., et al. (2015). Turnover Time of Fluorescent Dissolved Organic Matter in the Dark Global Ocean. Nat. Commun. 6 (1), 1–9. doi: 10.1038/ncomms6986
Cha H. J., Lee C. B., Kim B. S., Choi M. S., Ruttenberg K. C. (2005). Early Diagenetic Redistribution and Burial of Phosphorus in the Sediments of the Southwestern East Sea (Japan Sea). Mar. Geol. 216 (3), 127–143. doi: 10.1016/j.margeo.2005.02.001
Chang K. I., Kim K., Kim Y. B., Teague W. J., Lee J. C., Lee J. H. (2009). Deep Flow and Transport Through the Ulleung Interplain Gap in the Southwestern East/Japan Sea. Deep Sea Res. Part I. 56 (1), 61–72. doi: 10.1016/j.dsr.2008.07.015
Chen R. F., Bada J. L. (1994). The Fluorescence of Dissolved Organic Matter in Porewaters of Marine Sediments. Mar. Chem. 45 (1-2), 31–42. doi: 10.1016/0304-4203(94)90089-2
Chen M., Kim J. H., Nam S. I., Niessen F., Hong W. L., Kang M. H., et al. (2016). Production of Fluorescent Dissolved Organic Matter in Arctic Ocean Sediments. Sci. Rep. 6 (1), 1–10. doi: 10.1038/srep39213
Chin Y. P., Traina S. J., Swank C. R., Backhus D. (1998). Abundance and Properties of Dissolved Organic Matter in Pore Waters of a Freshwater Wetland. Limnol. Oceanogr. 43 (6), 1287–1296. doi: 10.4319/lo.1998.43.6.1287
Chipman L., Podgorski D., Green S., Kostka J., Cooper W., Huettel M. (2010). Decomposition of Plankton-Derived Dissolved Organic Matter in Permeable Coastal Sediments. Limnol. Oceanogr. 55 (2), 857–871. doi: 10.4319/lo.2010.55.2.0857
Chough S. K., Lee H. J., Yoon S. H. (2000). Marine Geology of Korean Seas. 2nd Edn (Amsterdam: Elsevier).
Church M. J., Ducklow H. W., Karl D. M. (2002). Multiyear Increases in Dissolved Organic Matter Inventories at Station ALOHA in the North Pacific Subtropical Gyre. Limnol. Oceanogr. 47 (1), 1–10. doi: 10.4319/lo.2002.47.1.0001
Eusterhues K., Neidhardt J., Hädrich A., Küsel K., Totsche K. U. (2014). Biodegradation of Ferrihydrite-Associated Organic Matter. Biogeochem. 119 (1), 45–50. doi: 10.1007/s10533-013-9943-0
Fox C. A., Abdulla H. A., Burdige D. J., Lewicki J. P., Komada T. (2018). Composition of Dissolved Organic Matter in Pore Waters of Anoxic Marine Sediments Analyzed by 1H Nuclear Magnetic Resonance Spectroscopy. Front. Mar. Sci. 5. doi: 10.3389/fmars.2018.00172
Fukuzaki K., Imai I., Fukushima K., Ishii K. I., Sawayama S., Yoshioka T. (2014). Fluorescent Characteristics of Dissolved Organic Matter Produced by Bloom-Forming Coastal Phytoplankton. J. Plankton Res. 36 (3), 685–694. doi: 10.1093/plankt/fbu015
Guenet B., Danger M., Abbadie L., Lacroix G. (2010). Priming Effect: Bridging the Gap Between Terrestrial and Aquatic Ecology. J. Ecol. 91 (10), 2850–2861. doi: 10.1890/09-1968.1
Hansell D. A. (2005). Dissolved Organic Carbon Reference Material Program. EOS Trans. Am. Geophysical Union. 86, 318. doi: 10.1029/2005EO350003
Hansell D. A. (2013). Recalcitrant Dissolved Organic Carbon Fractions. Annu. Rev. Mar. Science 5, 421–445. doi: 10.1146/annurev-marine-120710-100757
Hansell D. A., Carlson C. A. (2015). Biogeochemistry of Marine Dissolved Organic Matter. 2nd Edn (Amsterdam: Academic Press).
Hayase K., Shinozuka N. (1995). Vertical Distribution of Fluorescent Organic Matter Along With AOU and Nutrients in the Equatorial Central Pacific. Mar. Chem. 48 (3-4), 283–290. doi: 10.1016/0304-4203(94)00051-E
Hyun J. H., Kim S. H., Mok J. S., Cho H., Lee T., Vandieken V., et al. (2017). Manganese and Iron Reduction Dominate Organic Carbon Oxidation in Surface Sediments of the Deep Ulleung Basin, East Sea. Biogeosci. 14 (4), 941–958. doi: 10.5194/bg-14-941-2017
Hyun J. H., Kim D., Shin C. W., Noh J. H., Yang E. J., Mok J. S., et al. (2009). Enhanced Phytoplankton and Bacterioplankton Production Coupled to Coastal Upwelling and an Anticyclonic Eddy in the Ulleung Basin, East Sea. Aquat. Microb. Ecol. 54 (1), 45–54. doi: 10.3354/ame01280
Hyun J. H., Yang E. J. (2003). Freezing Seawater for the Long-Term Storage of Bacterial Cells for Microscopic Enumeration. J. Microbiol. 41 (3), 262–265.
Jørgensen L., Stedmon C. A., Kragh T., Markager S., Middelboe M., Søndergaard M. (2011). Global Trends in the Fluorescence Characteristics and Distribution of Marine Dissolved Organic Matter. Mar. Chem. 126, 139–148. doi: 10.1016/j.marchem.2011.05.002
Jessen G. L., Lichtschlag A., Ramette A., Pantoja S., Rossel P. E., Schubert C. J., et al. (2017). Hypoxia Causes Preservation of Labile Organic Matter and Changes Seafloor Microbial Community Composition (Black Sea). Sci. Adv. 3 (2), e1601897. doi: 10.1126/sciadv.1601897
Jiao N., Cai R., Zheng Q., Tang K., Liu J., Jiao F., et al. (2018). Unveiling the Enigma of Refractory Carbon in the Ocean. Natl. Sci. Rev. 5 (4), 459–463. doi: 10.1093/nsr/nwy020
Jiao N., Herndl G. J., Hansell D. A., Benner R., Kattner G., Wilhelm S. W., et al. (2010). Microbial Production of Recalcitrant Dissolved Organic Matter: Long-Term Carbon Storage in the Global Ocean. Nat. Rev. Microbiol. 8 (8), 593–599. doi: 10.1038/nrmicro2386
Joo H., Park J. W., Son S., Noh J. H., Jeong J. Y., Kwak J. H., et al. (2014). Long-Term Annual Primary Production in the Ulleung Basin as a Biological Hot Spot in the East/Japan Sea. J. Geophys. Res.: Oceans. 119 (5), 3002–3011. doi: 10.1002/2014JC009862
Kang D. J., Kim B. Y., Kim K. R. (2010). Dissolved Oxygen at the Bottom Boundary Layer of the Ulleung Basin, East Sea. Ocean Polar Res. 32 (4), 439–448. doi: 10.4217/OPR.2010.32.4.439
Kim Y. B., Chang K. I., Park J. H., Park J. J. (2013). Variability of the Dokdo Abyssal Current Observed in the Ulleung Interplain Gap of the East/Japan Sea. Acta Oceanolog. Sin. 32 (1), 12–23. doi: 10.1007/s13131-013-0263-y
Kim D., Choi M. S., Oh H. Y., Kim K. H., Noh J. H. (2009). Estimate of Particulate Organic Carbon Export Flux Using 234th/238u Disequilibrium in the Southwestern East Sea During Summer. Sea: J. Korean Soc. Oceanogr. 14 (1), 1–9.
Kim D., Choi M. S., Oh H. Y., Song Y. H., Noh J. H., Kim K. H. (2011). Seasonal Export Fluxes of Particulate Organic Carbon From 234th/238u Disequilibrium Measurements in the Ulleung Basin1 (Tsushima Basin) of the East Sea1 (Sea of Japan). J. Oceanogr. 67 (5), 577–588. doi: 10.1007/s10872-011-0058-8
Kim M., Hwang J., Rho T., Lee T., Kang D. J., Chang K. I., et al. (2017). Biogeochemical Properties of Sinking Particles in the Southwestern Part of the East Sea (Japan Sea). J. Mar. Syst. 167, 33–42. doi: 10.1016/j.jmarsys.2016.11.001
Kim J., Kim G. (2015). Importance of Colored Dissolved Organic Matter (CDOM) Inputs From the Deep Sea to the Euphotic Zone: Results From the East (Japan) Sea. Mar. Chem. 169, 33–40. doi: 10.1016/j.marchem.2014.12.010
Kim J., Kim G. (2016). Significant Anaerobic Production of Fluorescent Dissolved Organic Matter in the Deep East Sea (Sea of Japan). Geophys. Res. Lett. 43, 7609–7616. doi: 10.1002/2016GL069335
Kim T. H., Kim G., Lee S. A., Dittmar T. (2015). Extraordinary Slow Degradation of Dissolved Organic Carbon (DOC) in a Cold Marginal Sea. Sci. Rep. 5 (1), 1–6. doi: 10.1038/srep13808
Kim T. H., Kim G., Shen Y., Benner R. (2017). Strong Linkages Between Surface and Deep-Water Dissolved Organic Matter in the East/Japan Sea. Biogeosci. 14 (9), 2561–2570. doi: 10.5194/bg-14-2561-2017
Kim D., Yang E. J., Kim K. H., Shin C. W., Park J., Yoo S., et al. (2012). Impact of an Anticyclonic Eddy on the Summer Nutrient and Chlorophyll a Distributions in the Ulleung Basin, East Sea (Japan Sea). ICES J. Mar. Sci. 69 (1), 23–29. doi: 10.1093/icesjms/fsr178
Kirchman D. L., et al. (1993). Leucine Incorporation as a Measure of Biomass Production by Heterotrophic Bacteriam, “in Handbook of Methods in Aquatic Microbial Ecology, ed. Kemp et al., (Boca Raton, Lewis, CRC press), 509–512.
Kirchman D. L., Morán X. A. G., Ducklow H. (2009). Microbial Growth in the Polar Oceans—Role of Temperature and Potential Impact of Climate Change. Nat. Rev. Microbiol. 7 (6), 451–459. doi: 10.1038/nrmicro2115
Komada T., Polly J. A., Johnson L. (2012). Transformations of Carbon in Anoxic Marine Sediments: Implications From Δ14 C and δ 13 C Signatures. Limnol. Oceanogr. 57 (2), 567–581. doi: 10.4319/lo.2012.57.2.0567
Komada T., Reimers C. E., Luther G. W. III, Burdige D. J. (2004). Factors Affecting Dissolved Organic Matter Dynamics in Mixed-Redox to Anoxic Coastal Sediments. Geochim. Cosmochim. Acta 68 (20), 4099–4111. doi: 10.1016/j.gca.2004.04.005
Kramer G. D., Herndl G. J. (2004). Photo-And Bioreactivity of Chromophoric Dissolved Organic Matter Produced by Marine Bacterioplankton. Aquat. Microb. Ecol. 36 (3), 239–246. doi: 10.3354/ame036239
Kwak J. H., Hwang J., Choy E. J., Park H. J., Kang D. J., Lee T., et al. (2013). High Primary Productivity and F-Ratio in Summer in the Ulleung Basin of the East/Japan Sea. Deep Sea Res. Part I. 79, 74–85. doi: 10.1016/j.dsr.2013.05.011
Lalonde K., Mucci A., Ouellet A., Gelinas Y. (2012). Preservation of Organic Matter in Sediments Promoted by Iron. Nat. 483(7388), 198–200. doi: 10.1038/nature10855
Lawaetz A. J., Stedmon C. A. (2009). Fluorescence Intensity Calibration Using the Raman Scatter Peak of Water. Appl. Spectrosc. 63 (8), 936–940. doi: 10.1366/000370209788964548
Lee S. H., Bahk J. J., Kim S. P., Park J. Y. (2016). “Physiography and Late Quaternary Sedimentation,” in Oceanography of the East Sea (Japan Sea). Eds. Chang K.-I., Zhang C.-I., Park C., Kang D.-J., Ju S.-J., Lee S.-H., Wimbush M. (Switzerland: Springer), 389–414. doi: 10.1007/978-3-319-22720-7_16
Lee J. S., Han J. H., An S. U., Kim S. H., Lim D., Kim D., et al. (2019). Sedimentary Organic Carbon Budget Across the Slope to the Basin in the Southwestern Ulleung (Tsushima) Basin of the East (Japan) Sea. J. Geophys. Res.: Biogeosci. 124 (9), 2804–2822. doi: 10.1029/2019JG005138
Lee T., Hyun J. H., Mok J. S., Kim D. (2008). Organic Carbon Accumulation and Sulfate Reduction Rates in Slope and Basin Sediments of the Ulleung Basin, East/Japan Sea. Geo-Mar. Lett. 28 (3), 153–159. doi: 10.1007/s00367-007-0097-8
Liu S., Parsons R., Opalk K., Baetge N., Giovannoni S., Bolaños L. M., et al. (2020). Different Carboxyl-Rich Alicyclic Molecules Proxy Compounds Select Distinct Bacterioplankton for Oxidation of Dissolved Organic Matter in the Mesopelagic Sargasso Sea. Limnol. Oceanogr. 65 (7), 1532–1553. doi: 10.1002/lno.11405
Marchand C., Albéric P., Lallier-Vergès E., Baltzer F. (2006). Distribution and Characteristics of Dissolved Organic Matter in Mangrove Sediment Pore Waters Along the Coastline of French Guiana. Biogeochem. 81 (1), 59–75. doi: 10.1007/s10533-006-9030-x
Ma D., Wu J., Yang P., Zhu M. (2020). Coupled Manganese Redox Cycling and Organic Carbon Degradation on Mineral Surfaces. Environ. Sci. Technol. 54 (14), 8801–8810. doi: 10.1021/acs.est.0c02065
Murphy K. R., Stedmon C. A., Wenig P., Bro R. (2014). OpenFluor-An Online Spectral Library of Auto-Fluorescence by Organic Compounds in the Environment. Anal. Meth. 6, 658–661. doi: 10.1039/C3AY41935E
Nagata T., Fukuda H., Fukuda R., Koike I. (2000). Bacterioplankton Distribution and Production in Deep Pacific Waters: Large–Scale Geographic Variations and Possible Coupling With Sinking Particle Fluxes. Limnol. Oceanogr. 45 (2), 426–435. doi: 10.4319/lo.2000.45.2.0426
Nagata T., Tamburini C., Arístegui J., Baltar F., Bochdansky A. B., Fonda-Umani S., et al. (2010). Emerging Concepts on Microbial Processes in the Bathypelagic Ocean–Ecology, Biogeochemistry, and Genomics. Deep Sea Res. Part II. 57 (16), 1519–1536. doi: 10.1016/j.dsr2.2010.02.019
Nelson N. B., Carlson C. A., Steinberg D. K. (2004). Production of Chromophoric Dissolved Organic Matter by Sargasso Sea Microbes. Mar. Chem. 89 (1-4), 273–287. doi: 10.1016/j.marchem.2004.02.017
Nieto-Cid M., Álvarez-Salgado X. A., Pérez F. F. (2006). Microbial and Photochemical Reactivity of Fluorescent Dissolved Organic Matter in a Coastal Upwelling System. Limnol. Oceanogr. 51 (3), 1391–1400. doi: 10.4319/lo.2006.51.3.1391
Niggemann J., Lomstein B. A., Schubert C. J. (2017). Diagenesis of Amino Compounds in Water Column and Sediment of Lake Baikal. Org. Geochem. 115, 67–77. doi: 10.1016/j.orggeochem.2017.10.008
Ogawa H., Amagai Y., Koike I., Kaiser K., Benner R. (2001). Production of Refractory Dissolved Organic Matter by Bacteria. Science. 292(5518), 917–920. doi: 10.1126/science.1057627
Osburn C. L., Mikan M. P., Etheridge J. R., Burchell M. R., Birgand F. (2015). Seasonal Variation in the Quality of Dissolved and Particulate Organic Matter Exchanged Between a Salt Marsh and its Adjacent Estuary. J. Geophys. Res. 120, 1430–1449. doi: 10.1002/2014JG002897
Porter K. G., Feig Y. S. (1980). The Use of DAPI for Identifying and Counting Aquatic Microflora 1. Limnol. Oceanogr. 25 (5), 943–948. doi: 10.4319/lo.1980.25.5.0943
Reinthaler T., Van Aken H., Veth C., Arístegui J., Robinson C., Williams P. J. L. B., et al. (2006). Prokaryotic Respiration and Production in the Meso-And Bathypelagic Realm of the Eastern and Western North Atlantic Basin. Limnol. Oceanogr. 51 (3), 1262–1273. doi: 10.4319/lo.2006.51.3.1262
Robador A., Brüchert V., Steen A. D., Arnosti C. (2010). Temperature Induced Decoupling of Enzymatic Hydrolysis and Carbon Remineralization in Long-Term Incubations of Arctic and Temperate Sediments. Geochim. Cosmochim. Acta 74 (8), 2316–2326. doi: 10.1016/j.gca.2010.01.022
Rossel P. E., Bienhold C., Boetius A., Dittmar T. (2016). Dissolved Organic Matter in Pore Water of Arctic Ocean Sediments: Environmental Influence on Molecular Composition. Org. Geochem. 97, 41–52. doi: 10.1016/j.orggeochem.2016.04.003
Santinelli C., Nannicini L., Seritti A. (2010). DOC Dynamics in the Meso and Bathypelagic Layers of the Mediterranean Sea. Deep Sea Res. Part ІІ. 57, 1446–1459. doi: 10.1016/j.dsr2.2010.02.014
Shen Y., Benner R. (2018). Mixing It Up in the Ocean Carbon Cycle and the Removal of Refractory Dissolved Organic Carbon. Sci. Rep. 8 (1), 1–9. doi: 10.1038/s41598-018-20857-5
Skoog A., Hall P. O., Hulth S., Paxéus N., van der Loeff M. R., Westerlund S. (1996). Early Diagenetic Production and Sediment-Water Exchange of Fluorescent Dissolved Organic Matter in the Coastal Environment. Geochim. Cosmochim. Acta 60 (19), 3619–3629. doi: 10.1016/0016-7037(96)83275-3
Smith D. C., Azam F. (1992). A Simple, Economical Method for Measuring Bacterial Protein Synthesis Rates in Seawater Using 3h-Leucine. Mar. Microb. Food Webs. 6 (2), 107–114. doi: 10.1037//0893-164X.6.2.107
Stedmon C. A., Bro R. (2008). Characterizing Dissolved Organic Matter Fluorescence With Parallel Factor Analysis: A Tutorial. Limnol. Oceanogr. Meth. 6 (11), 572–579. doi: 10.4319/lom.2008.6.572
Stuckey J. W., Goodwin C., Wang J., Kaplan L. A., Vidal-Esquivel P., Beebe T. P., et al. (2018). Impacts of Hydrous Manganese Oxide on the Retention and Lability of Dissolved Organic Matter. Geochem. Trans. 19 (1), 6. doi: 10.1186/s12932-018-0051-x
Tamburini C., Garcin J., Ragot M., Bianchi A. (2002). Biopolymer Hydrolysis and Bacterial Production Under Ambient Hydrostatic Pressure Through a 2000 M Water Column in the NW Mediterranean. Deep Sea Res. Part II. 49 (11), 2109–2123. doi: 10.1016/S0967-0645(02)00030-9
Tanaka K., Kuma K., Hamasaki K., Yamashita Y. (2014). Accumulation of Humic-Like Fluorescent Dissolved Organic Matter in the Japan Sea. Sci. Rep. 4 (1), 1–7. doi: 10.1038/srep05292
Tobias-Hünefeldt S. P., Wing S. R., Baltar F., Morales S. E. (2021). Changes in Microbial Community Phylogeny and Metabolic Activity Along the Water Column Uncouple at Near Sediment Aphotic Layers in Fjords. Sci. Rep. 11, 19303. doi: 10.21203/rs.3.rs-334470/v1
Trainer E. L., Ginder-Vogel M., Remucal C. K. (2021). Selective Reactivity and Oxidation of Dissolved Organic Matter by Manganese Oxides. Environ. Sci. Technol. 55 (17), 12084–12094. doi: 10.1021/acs.est.1c03972
Wear E. K., Carlson C. A., Church M. J. (2020). Bacterioplankton Metabolism of Phytoplankton Lysates Across a Cyclone-Anticyclone Eddy Dipole Impacts the Cycling of Semi-Labile Organic Matter in the Photic Zone. Limnol. Oceanogr. 65 (7), 1608–1622. doi: 10.1002/lno.11409
Weston N. B., Joye S. B. (2005). Temperature-Driven Decoupling of Key Phases of Organic Matter Degradation in Marine Sediments. PNAS. 102 (47), 17036–17040. doi: 10.1073/pnas.0508798102
Yamada K., Ishizaka J., Nagata H. (2005). Spatial and Temporal Variability of Satellite Primary Production in the Japan Sea From 1998 to 2002. J. Oceanogr. 61 (5), 857–869. doi: 10.1007/s10872-006-0005-2
Yamashita Y., Jaffé R., Maie N., Tanoue E. (2008). Assessing the Dynamics of Dissolved Organic Matter (DOM) in Coastal Environments by Excitation Emission Matrix Fluorescence and Parallel Factor Analysis (EEM-PARAFAC). Limnol. Oceanogr. 53 (5), 1900–1908. doi: 10.4319/lo.2008.53.5.1900
Yamashita Y., Tanoue E. (2003). Chemical Characterization of Protein-Like Fluorophores in DOM in Relation to Aromatic Amino Acids. Mar. Chem. 82, 255–271. doi: 10.1016/S0304-4203(03)00073-2
Yamashita Y., Tanoue E. (2008). Production of Bio-Refractory fluorescent Dissolved Organic Matter in the Ocean Interior. Nat. Geosci. 1, 579–582. doi: 10.1038/ngeo279
Yamashita Y., Tsukasaki A., Nishida T., Tanoue E. (2007). Vertical and Horizontal Distribution of Fluorescent Dissolved Organic Matter in the Southern Ocean. Mar. Chem. 106 (3-4), 498–509. doi: 10.1016/j.marchem.2007.05.004
Keywords: marginal seas, dissolved organic carbon (DOC), bacterial production, sediment-derived DOM, Ulleung Basin, East Sea
Citation: Hyun J-H, Kim B, Han H, Baek Y-J, Lee H, Cho H, Yoon S-H and Kim G (2022) Sediment-Derived Dissolved Organic Matter Stimulates Heterotrophic Prokaryotes Metabolic Activity in Overlying Deep Sea in the Ulleung Basin, East Sea. Front. Mar. Sci. 9:826592. doi: 10.3389/fmars.2022.826592
Received: 01 December 2021; Accepted: 11 March 2022;
Published: 22 April 2022.
Edited by:
X. Antón Álvarez-Salgado, Spanish National Research Council (CSIC), SpainReviewed by:
Christina Zeri, Hellenic Centre for Marine Research (HCMR), GreeceWei Xie, Sun Yat-sen University, Zhuhai Campus, China
Copyright © 2022 Hyun, Kim, Han, Baek, Lee, Cho, Yoon and Kim. This is an open-access article distributed under the terms of the Creative Commons Attribution License (CC BY). The use, distribution or reproduction in other forums is permitted, provided the original author(s) and the copyright owner(s) are credited and that the original publication in this journal is cited, in accordance with accepted academic practice. No use, distribution or reproduction is permitted which does not comply with these terms.
*Correspondence: Jung-Ho Hyun, hyunjh@hanyang.ac.kr