- Institute of Laboratory Medicine, German Center for Lung Research (DZL), Universities of Giessen and Marburg Lung Center (UGMLC), Philipps University Marburg, Marburg, Germany
Myeloid-derived suppressor cells (MDSCs) are a heterogeneous cell population with potent suppressive and regulative properties. MDSCs’ strong immunosuppressive potential creates new possibilities to treat chronic inflammation and autoimmune diseases or induce tolerance towards transplantation. Here, we summarize and critically discuss different pharmacological approaches which modulate the generation, activation, and recruitment of MDSCs in vitro and in vivo, and their potential role in future immunosuppressive therapy.
1. Introduction
Myeloid-derived suppressor cells (MDSCs) are a heterogeneous immature immune cell population, originating from the common myeloid progenitor cell, with potent immunosuppressive effect on T cell proliferation and activity (1). MDSCs were first described in cancer patients and associated with increased tumor growth and T cell dysfunction (2). In this respect, MDSCs are mainly studied in the tumor microenvironment, where they inhibit the anti-tumor immune response and support tumor angiogenesis (1, 3). In the context of the tumor microenvironment, dampening MDSCs may decrease metastatic niche formation (3–5). However, MDSCs not only play a role in cancer but also in a variety of other pathological conditions associated with an inflammatory state, such as, chronic infections, autoimmunity, asthma, or obesity (6–9). In autoimmune diseases, MDSCs can be useful to protect against tissue damage driven by an imbalanced immune reaction. Furthermore, if the immune response needs to be dampened, e.g. after allograft transplantation, or in conditions such as graft-versus-host disease (GVHD), MDSCs’ immunosuppressive potential might be beneficial. So far, the majority of imbalanced immune conditions are treated by corticosteroids and other immunosuppressive drugs which include substantial side effects. Therefore, MDSCs are a promising therapeutic target due to their immunosuppressive properties (10). This review discusses pharmacological approaches involved in the generation, recruitment and activation of MDSCs, providing possible clues for novel cellular immunosuppressive therapies.
1.1 Subsets
Due to the heterogeneity of the MDSC population, most marker proteins are not unique to MDSCs nor universally expressed. Nevertheless, two major subtypes can be distinguished: polymorphonuclear (PMN-) and monocytic (M-) MDSCs based on their similarities to neutrophils and monocytes, respectively. In mice, PMN-MDSCs are defined as CD11b+ Ly-6G+ Ly-6Clow and M-MDSCs as CD11b+ Ly-6G- Ly-6Chigh, while in humans PMN-MDSCs express CD11b+ CD14- CD15+ or CD11b+ CD14- CD66b+ and M-MDSCs CD11b+ CD14+ HLA-DR-/low CD15-. Only by means of these markers, PMN-MDSCs and M-MDSCs are undistinguishable from neutrophils and monocytes, respectively, and functional assays, such as T cell proliferation and cytokine release assays, are needed to evaluate the immunosuppressive activity (11). Unfortunately, MDSC heterogeneity as well as the significant overlap with more ‘conventional’ immune cell populations complicate the refinement of a universal distinctive MDSC signature. Quantitative tools, such as single-cell RNA sequencing and mass cytometry, as well as other advances contributing to the multi-omics approach, are starting to provide more insights into the phenotypic, morphological and functional heterogeneity of MDSCs. Recently, lectin-type oxidized low-density lipoprotein receptor 1 (LOX-1) has been described as a new marker for PMN-MDSCs in humans. LOX-1 can be found on macrophages, endothelial and smooth muscles cells, but importantly is not expressed on neutrophils and therefore presents a surface marker that helps to identify PMN-MDSCs (12). Furthermore, CD84 and JAML were recently identified as potential markers of MDSCs in breast cancer (13). Additionally, two arginase-1-expressing myeloid clusters were identified –Spp1+Apoe+C1qa+ and Gpnmb+Vegfa+Clec4d+Trem2+ – in murine tumors, where Trem2 was found to be associated with immunosuppressive function (14).
1.2 Origin and generation of MDSCs
During normal hematopoiesis common myeloid progenitor cells develop from hematopoietic stem cells and differentiate via immature myeloid cells into red blood cells, granulocytes, monocytes, thrombocytes and mast cells under the influence of growth factors, interleukins and other regulatory molecules. Under certain pathological conditions such as cancer, with chronic stimuli of a relatively low intensity, two signals are required for MDSCs proliferation. The first signal is responsible for stimulation of myelopoiesis with the inhibition of maturation and differentiation of progenitor cells and the expansion of immature myeloid cells while the second signal promotes the transformation into immunosuppressive MDSCs (15). Factors shown to be involved in MDSC expansion and activation include granulocyte-macrophage colony-stimulating factor (GM-CSF), granulocyte colony-stimulating factor (G-CSF), macrophage colony-stimulating factor (M-CSF), stem cell factor (SCF), cyclooxygenase (COX-) 2, prostaglandins (PG), vascular endothelial growth factor (VEGF) and interleukin (IL-) 6 (1). The Janus kinase (JAK) 2/signal transducer and activator of transcription (STAT) 3 pathway appears to be the most important regulator for MDSC expansion (1). For example, GM-CSF, G-CSF, and IL-6 induce the expansion of MDSCs through activation of STAT3 (15–18). The transcription factor interferon regulatory factor (IRF) 8 – downregulated by GM-CSF and G-CSF – acts as a STAT3- and STAT5-dependent negative regulator of MDSC generation (19). In addition, the β2-adrenergic receptor (AR) has been shown to play a role in MDSC generation and activation through STAT3 (20). Further underlining the importance of STAT3, several studies revealed that STAT3 inhibition dampened MDSCs in the tumor microenvironment and proved to be a useful anticancer therapy (21–23). Interferon (IFN-) γ activates the STAT1 pathway and leads to activation of MDSCs (24). Similarly, activation of the STAT6 pathway through IL-4 and IL-13 can induce immunosuppressive properties of MDSCs (15). Pro-inflammatory mediators such as tumor necrosis factor (TNF-) α, IL-1β, IL-12, PGE2 or Toll-like receptor (TLR) ligands enhance the immunosuppressive capacities of MDSCs through the nuclear factor kappa-light-chain-enhancer of activated B cells (NF-κB) pathway (25–30). In detail, activation of PGE2 receptor (EP) 2 and EP4 inhibits receptor-interacting protein kinase 3 (RIPK3), which in turn enhances the NF-κB pathway, as activated RIPK3 is involved in its downregulation (31). Furthermore, the endoplasmic reticulum (ER) stress response pathway ends up in the NF-κB pathway and promotes the activation of immunosuppressive MDSCs. The function of this pathway is to protect the cell from cellular stress like shortage of nutrients, hypoxia, or low pH (12). Furthermore, inhibition of the Notch pathway and activation of the adenosine receptor promote the expansion of MDSCs (32, 33). Those mechanisms create possible targets for in vitro and in vivo generation of MDSCs. MDSCs themselves are considered as immature cells with a high plasticity. Under hypoxic conditions, MDSCs are able to differentiate into tumor-associated macrophages, M2-like macrophages, inflammatory dendritic cells or fibrocytes (34). Hence, some researchers state that MDSCs are not a definitive cell group, but rather cells in transitory states, whose differentiation is ongoing.
1.3 Recruitment
Once generated, MDSCs are recruited to the site of activity by chemokines. The role of several cytokines, chemokines and their receptors in MDSC recruitment is reviewed elsewhere (35). These chemokines have been described to play an important role in MDSC recruitment through their interaction with corresponding G-protein coupled chemokine receptors: C-X-C motif ligand (CXCL)1/CXCL2/CXCL5 with C-X-C motif receptor (CXCR)2, CXCL8 with CXCR1/CXCR2, CXCL17 with CXCR8, C-C motif ligand (CCL)2/CCL12 with CCR2, CCL3/CCL4/CCL5 with CCR5, and CCL15 with CCR1. Specifically, CXCR2 appears to be critical for MDSC recruitment (4, 36–42).
1.4 Mechanisms of immunosuppressive activity
Activated MDSCs carry out their suppressive activity on T cell proliferation by different mechanisms, which were reviewed by Gabrilovich and Nagaraj (1). Upon upregulation of transcription factors like STAT1, 3, 6 and NF-κB, the expression of reactive oxygen species (ROS), arginase-1 (Arg-1), inducible nitric oxide synthase (iNOS), NF-κB and idoleamine 2,3-dioxygenase (IDO) is increased. Production of ROS by nicotiamide adenine dinucleotide phosphate (NADPH) oxidase suppresses T cell function by destroying proteins, lipids and inducing apoptosis among other mechanisms (43, 44). Both Arg-1 and iNOS deprive L-arginine – an amino acid essential for T cell metabolism – from the microenvironment and thus, inhibit T cell proliferation by suppressing T cell cycle progression (45). In addition, the iNOS product nitric oxide (NO) suppresses T cell function and induces apoptosis by itself through different mechanisms (1). Simultaneously, IDO inhibits T cell proliferation by depleting tryptophan from T cell metabolism as well as increasing regulatory T cells (Treg) recruitment (46). Shifting the T cell population towards immunosuppressive forkhead box (Fox) P3+ Tregs presents another effective way of immunosuppression (47, 48). In addition, MDSCs have been shown to suppress B cell responses (49). There are also subset specific differences: M-MDSCs mainly use NO produced by iNOS to suppress T cell function, while PMN-MDSCs express higher levels of ROS and peroxynitrite, a product from the reaction of NO and superoxide anion (50, 51). Both M- and PMN-MDSC secrete Arg-1 (50).
2. Pharmacological approaches to modulate MDSCs
There are in principle two main pharmacological approaches for MDSC generation: Expanding MDSCs ex vivo and adoptively transferring them into patients or stimulating endogenous MDSC expansion/activation. Possible pharmacological approaches for MDSCs generation, activation and recruitment are presented in Table 1 as well as Figure 1.
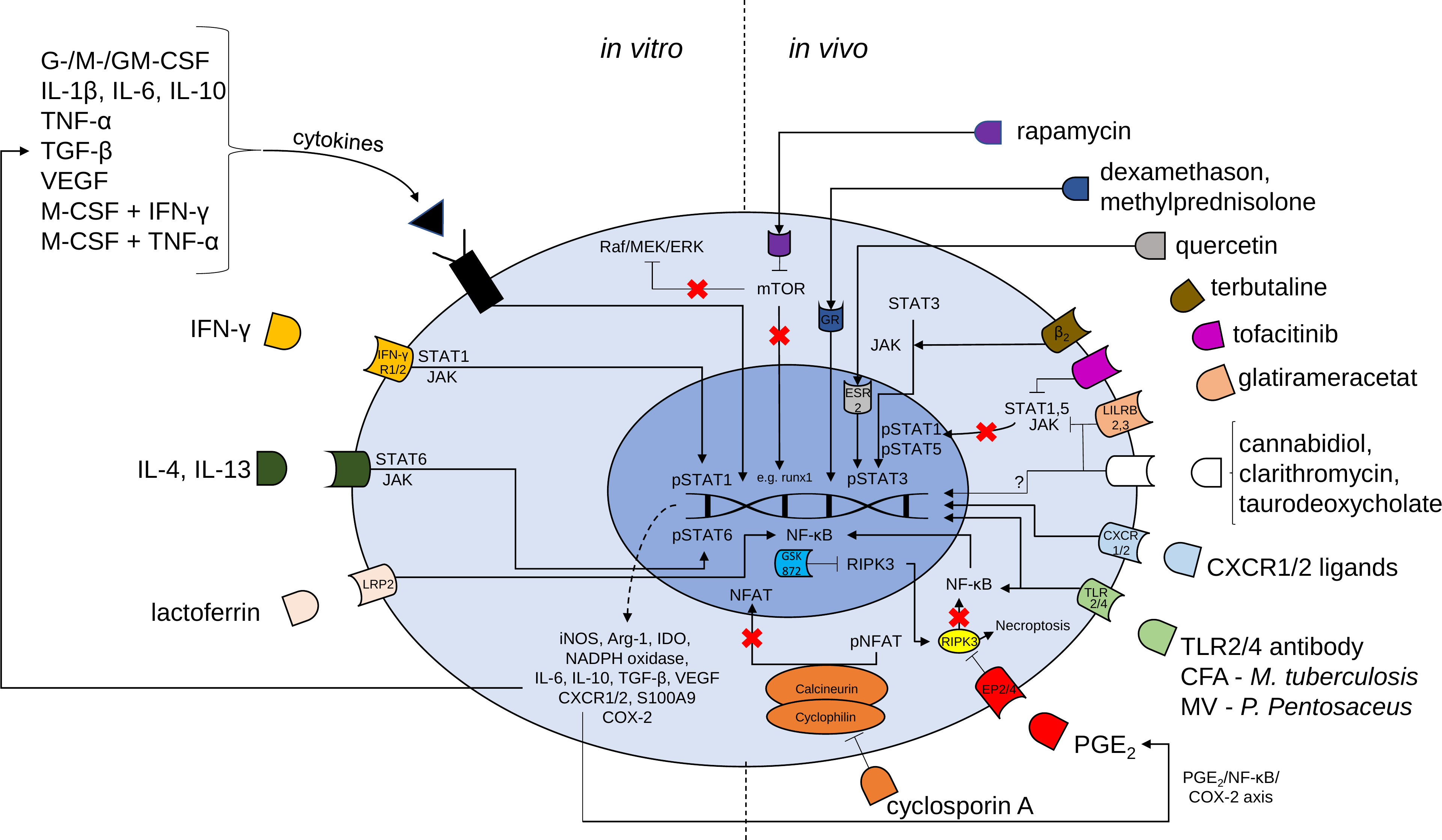
Figure 1 Mechanisms of possible pharmacological targets for the induction of MDSCs. Proliferation and activation of MDSCs is regulated by transcription factors such as signal transducer and activator of transcription (STAT) 3, STAT1, STAT5, STAT6 and nuclear factor kappa-light-chain-enhancer of activated B cells (NF-κB). In vitro generation of MDSCs is induced by a large variety of cytokines and cytokine combinations and lactoferrin. MDSC generation may be followed by adoptive transfer cell therapy to induce the beneficial effects of MDSCs. Various compounds [e.g. rapamycin, glucocorticoids, terbutaline, tofacitinib, glatirameracetat, cannabidiol, clarithromycin, taurodeoxycholate, CXCR1 or CXCR2 ligands, complete Freund’s adjuvant (CFA), membrane vesicles (MV), Toll-like receptor (TLR) 2/4 agonistic antibodies, prostaglandin E2, cyclosporine A and receptor interacting protein kinase (RIPK) 3 inhibitor (GSK 872)] may be used for accumulation of MDSCs in vivo. G-CSF granulocyte colony-stimulating factor, M-CSF macrophage CSF, GM-CSF granulocyte-macrophage CSF, IL Interleukin, TNF tumor necrosis factor, TGF transforming growth factor, VEGF vascular endothelial growth factor, IFN interferon, mTOR mammalian target of rapamycin, JAK Janus kinase, LRP lactoferrin receptor, iNOS inducible nitric oxide synthase, Arg arginase, IDO indoleamine 2,3-dioxygenase, NADPH nicotiamide adenine dinucleotide phosphate, NFAT nuclear factor of activated t cells, p phosphorylated, CXCR CXC chemokine receptors, COX cyclooxygenase.
2.1 Adoptive transfer of in vitro/ex vivo generated/activated MDSCs
Non-stem cell-based cell therapies that use cells such as CAR-T cells, dendritic cells and natural killer cells are promising and rapidly evolving (92). MDSC cell therapy may be another promising strategy in this list, especially considering its potent immunosuppressive capacity and its role in maintaining immune tolerance in transplantation and autoimmunity. Several pre-clinical studies have shown promising therapeutic effects of the adoptive transfer of MDSCs in organ transplantation, autoimmune diseases as well as in a variety of other immune-related disorders, such as cyclosporin A-induced hypertension, heart failure and asthma (63, 69, 93–100). Here, we briefly discuss some of the cytokines and pharmacological compounds that have been identified to promote the generation and/or activation of MDSCs in vitro/ex vivo and were applied as MDSC cell therapy.
2.1.1 Cytokines
Park et al. demonstrated the beneficial role of in vitro generated MDSCs in the context of GVHD (101). Here, the highest efficiency of in vitro production of MDSCs from CD34+ human umbilical cord blood cells was achieved with a combination of GM-CSF and SCF, whereas G-CSF/SCF and M-CSF/SCF were less effective (101). Adoptively transferred MDSCs were shown to ameliorate GVHD and prolong survival in a murine xenogeneic model of GVHD by promoting Tregs and inhibiting the T helper (Th) 1 and Th17-driven inflammatory responses (101). MDSCs generated with GM-CSF/SCF were shown to exhibit increased immunosuppressive activity after the transfer in vivo compared to MDSCs generated in the presence of either G-CSF/SCF or M-CSF/SCF (101). Hsieh et al. also showed beneficial effects of adoptive transfer of MDSCs in renal fibrosis and diabetic neuropathy in diabetic mice (102). Here, murine bone marrow-derived MDSCs were induced with GM-CSF, IL-1β and IL-6 in vitro. In addition, Yang et al. induced M-MDSCs with combinations of M-CSF/IFN-γ and M-CSF/TNF-α, respectively, and demonstrated prolonged skin allograft survival upon adoptive transfer (103, 104). In vitro generated MDSCs, induced by GM-CSF, G-CSF, and IL-6, efficiently ameliorated autoimmune arthritis in mice (105). Recently, another group demonstrated that the adoptive transfer of splenic CD11b+Gr-1+ MDSCs, obtained from G-CSF-treated donor mice, are capable of prolonging heart allograft survival (93).
As already discussed above, there are many cytokines and cytokine combinations identified to play important roles in MDSC generation and activation. Here it is important to consider that certain cytokine signaling is required to induce MDSCs, and the discussed pharmacological compounds are insufficient to induce in vitro/ex vivo MDSCs without additional cytokine signaling.
2.1.2 Glucocorticoids
Zhao et al. induced MDSCs in vitro by means of GM-CSF and dexamethasone (63). Adoptive transfer of dexamethasone-induced MDSCs into mice prolonged heart allograft survival, likely through increased levels of iNOS and increased number of Tregs (63). Indeed, dexamethasone is already an established immunosuppressive drug, and these findings show that a part of its immunosuppressive activity is likely mediated by their effect on MDSCs.
2.1.3 Lactoferrin
Adoptive transfer of in vitro generated murine bone marrow-derived MDSCs treated with lactoferrin (LF) prolonged survival and ameliorated inflammation in necrotizing enterocolitis in newborn mice as well as concanavalin-induced hepatitis and ovalbumin-induced lung inflammation (68). Both in vitro and in vivo LF treatment increased MDSC numbers, likely via NF-κB activation, but only in infant mice due to decreased LF receptor (LRP) 2 expression in adults (68). However, in vivo administration of LF was shown to be much less effective in recruiting and activating MDSCs compared to the adoptive transfer of in vitro generated MDSCs using LF. This study demonstrates the potential of targeting NF-κB and LRP2 to recruit MDSCs (68).
2.1.4 PGE2
PGE2, in combination with GM-CSF and either IL-4 or IL-6, was found to efficiently induce MDSCs ex vivo (55, 100). From the four EP subreceptors (EP1-4) of PGE2, EP2 and EP4, and not EP1 and EP3, were found to induce MDSC development, hinting at an important role of the adenylate cyclase/cAMP/PKA/CREB signaling pathway (55, 100). Furthermore, the adoptive transfer of MDSCs generated in the presence of a selective EP4 receptor agonist dampened airway inflammatory features in a murine model of asthma (100).
2.1.5 Phorbol 12-myristate 13-acetate
The combination of M-CSF and PMA was recently shown to induce MDSCs in vitro (106). The adoptive transfer of PMA-induced MDSCs induced immune tolerance in a mouse skin transplantation model, by inhibiting the T cell response, promotion of cytokine secretion and inducing Tregs (106). PMA significantly upregulated Arg-1 expression in MDSCs, and an Arg-1 inhibitor (nor-NOHA) diminished MDSC activity (106). This study confirms that in vitro induced-MDSCs may be promising targets for adoptive transfer to modulate immunosuppression, such as in organ transplantation.
2.1.6 Rapamycin
The mTOR inhibitor rapamycin is frequently used in immunosuppressive therapy following allograft transplantation in order to prevent allograft rejection. Rapamycin is also applied on drug-eluting stents to decrease stent stenosis, via its anti-proliferative properties. Nakamura et al. performed adoptive transfer of in vitro generated MDSCs, treated with rapamycin, directly into the coronary artery of heart allografts in mice (48). Administration of rapamycin-induced MDSCs prolonged skin allograft survival and demonstrated the possibility of local MDSC therapy. Adoptive transfer of rapamycin-treated MDSCs resulted in improved outcomes concerning acute kidney injury, with increased Tregs number and decreased pro-inflammatory cytokines compared to adoptive transfer of MDSCs not treated with rapamycin (40).
To this end, the in vitro/ex vivo generation and subsequent adoptive transfer of MDSCs has been established in animal models and most studies observe a beneficial effect in the treatment of inflammatory diseases, yet human studies are needed to further confirm the therapeutic concepts. However, at the time of writing this review, no clinical trials investigating MDSC adoptive transfer as a cell therapy have been performed nor were registered in the clinical trial databases of the National Institute of Health1 or the EU clinical trial register2. Nevertheless, the generation/expansion of endogenous MDSC, by administration of the right cytokine combination or potential medications, would be the more elegant way to generate, activate and recruit the MDSCs and dampen inflammatory diseases. We discuss the possibilities in the following chapter of this review.
2.2 In vivo generation, recruitment, and activation of MDSCs
2.2.1 Acetaminophen
Hsu et al. showed that the increase of intrahepatic MDSCs by a sublethal dose of acetaminophen was able to protect mice against subsequent lethal doses of acetaminophen, lipopolysaccharide (LPS)/D-galactosamine or concanavalin A (107). This finding was confirmed by a loss of protection after MDSC depletion (107). The observed protective effect was likely mediated by increased iNOS expression, as iNOS-expressing MDSCs were found to induce the apoptosis of activated neutrophils and decreased the intrahepatic infiltration of elastase-expressing neutrophils (107). Furthermore, the group of Hsu et al. were able to generate human PMBC-derived MDSCs with a similar phenotype (107).
2.2.2 Rapamycin and other mammalian target of rapamycin inhibitors
Besides the immune regulating features of rapamycin and its ability to generate MDSCs in vitro for cell therapy, rapamycin has also been linked to MDSC recruitment and activation in vivo. Nakamura et al. reported prolonged heart allograft survival in mice under Rapamycin administration, which was found to be related to increased number of MDSCs, particularly M-MDSCs, and iNOS expression (48). Zhang et al. showed that rapamycin treatment ameliorated acute kidney injury through the recruitment and activation of mainly PMN-MDSCs (40). A recent study by Scheurer et al. demonstrated that rapamycin administered after bone marrow transplantation promoted immunosuppressive properties of MDSCs and thus prevented GVHD (70). Furthermore, rapamycin treatment ameliorated heart failure in mice, likely through the induction of MDSCs, as MDSC depletion diminished this beneficial effect (69). Wei et al. treated cornea transplanted mice with eye drops containing rapamycin nano-micelles (71). An increased recruitment of MDSCs and expression of Arg-1 and iNOS was observed and was in line with a prolonged allograft survival. Also, in immunological hepatic injury, rapamycin treatment was found to promote MDSC recruitment, generation, and activity, and ameliorated the disease (72). Recently, our group demonstrated the allograft survival prolonging properties of rapamycin in obese mice through increased M-MDSCs number and activity (73). Taken together, rapamycin seems to be an efficient inductor of MDSC generation and activation, with the advantage of already being approved for clinical use for certain diseases. Furthermore, a novel mTOR inhibitor, INK128, was shown to promote wound healing in streptozotocin-induced diabetic mice (74). mTOR deficiency in M-MDSCs was shown to induce tolerance of mouse cardiac allografts (75), while adoptive transfer of PMN-MDSCs lacking mTOR expression was shown to dampen acute GVHD (76), confirming the beneficial role of mTOR inhibition in promoting MDSC immune suppression.
However, the linking mechanism between mTOR and MDSCs is not fully understood. Nakamura et al. stated that mTOR inhibition could lead to increased activation of the Raf/MEK/ERK pathway, which caused MDSC recruitment and activation (48). Another possible mechanism responsible for MDSC activation is the downregulation of runt-related transcription factor 1 (runx1) gene expression (40). A third possible mechanism was recently described by Jia et al. who reported that AKT1, a known downstream target of mTOR, regulates MDSC immunosuppressive activities by suppressing hypoxia-inducible factor 1α-dependent glycolysis (77). Therefore, further research is necessary to further clarify the underlying mechanism.
2.2.3 Glucocorticoids
Evidence emerged showing that glucocorticoids may also carry out their immunosuppressive effect through the induction as well as the recruitment of MDSCs. Mechanistically, the glucocorticoid receptor (GR) is critical for the immunosuppressive activity of MDSCs as GR activation leads to a release of CXCR2, which is one of the main chemokines involved in the recruitment of MDSCs to areas of inflammation (64). Liao et al. demonstrated the same effects and mechanisms of dexamethasone administration in vivo in a mouse model of skin allograft transplantation (64). Another frequently used glucocorticoid is methylprednisolone. Direct correlations between methylprednisolone administration and increases in the number of MDSCs, particularly PMN-MDSCs, have recently been demonstrated in a murine model of multiple sclerosis (MS) and human MS patients by Wang et al. (65). Interestingly, a difference in MDSC immunosuppressive activity was observed in mice compared to human MS patients, whereas MDSCs show higher immunosuppressive activity in the latter upon treatment with methylprednisolone. This was revealed by measuring MDSC activity in experimental autoimmune encephalomyelitis (EAE) mice and MS patients, before and after methylprednisolone treatment (65). In mice, increased number of MDSCs were observed at the onset of EAE, but not after methylprednisolone pulse therapy. In contrast, in MS patients increasing MDSC numbers were observed in PBMCs after methylprednisolone application, and disease remission after treatment was correlated to the increased number of MDSCs (65). This highlights the complexity and importance of analyzing MSDC behavior, not only in mice, but also in humans. Furthermore, the mechanism of MDSC induction by glucocorticoids is linked to inhibition and downregulation of GR-β, which, if activated, antagonizes the effect of glucocorticoids (65). Mice only express one type of GR which might explain the higher MDSC activity observed in humans (63). Methylprednisolone is considered the main drug for chronic inflammatory conditions and is frequently used, not only in MS patients, but also in many other autoimmune or chronic inflammatory diseases like chronic obstructive pulmonary disease (COPD) and rheumatic diseases (108). Hence, methylprednisolone and the GR, with its different subtypes, may provide promising targets for further research.
2.2.4 Chemokines
CXCR1 and CXCR2, among others, are highly expressed on MDSCs and responsible for their recruitment. Blockage of CXCR1 and CXCR2 with selective antagonists was shown to severely decrease the number of MDSC infiltration in pulmonary hypertension and carcinomas (4, 37). Furthermore, intratracheal administration of recombinant mouse protein CXCL17 has been shown to result in the recruitment of MDSCs into the lungs of mice (36). Selective CXCR1, CXCR2 and CXCL17 agonists seem to be promising pharmacological drugs for increasing MDSC migration. However, these agonists need to be further studied in the context of MDSC recruitment. Recently, 2,3,7,8-tetrachlorodibenzo-p-dioxin (TCDD) – an environmental pollutant and aryl hydrocarbon receptor (AhR) agonist – was shown to indirectly recruit MDSCs through strong induction of CXCR2 expression by down-regulating specific miRNAs (39). Upregulation of transcription factors responsible for CXCR2 ligands (CXCL1 and CXCL2) expression, like Snail, which acts through NF-κB pathway, also provides other possible targets related to chemokines (38). In addition, mTOR inhibition with rapamycin was found to result in increased CXCR2, CXCL1 and CXCL2 expression, which was directly linked to MDSC recruitment to the site of acute kidney injury (40). Overall, targeting chemokines and their receptors for MDSC recruitment seems promising, but the involved risks, as previously reported in cancer patients, should be taken into careful consideration (3–5). However, MDSCs primarily are involved in promoting pre-metastatic niche formation and not directly in tumor growth itself (109). Thus, the question can be raised whether it could be beneficial to use locally administered CXCR1 and CXCR2 ligands or CXCL17 only for tumor-free patients in sites of chronic inflammation. As administration of those ligands could increase the risk of pre-metastatic niche formation if tumor cells are present, but not if the patient is tumor-free. Alternatively, coating of allografts with chemokines and cytokines, e.g. GM-CSF and CXCL17, could be used to recruit MDCS to the allograft and locally reduce the immune response.
2.2.5 Endothelin (ET)A receptor antagonist
ET signaling mediates strong vasoconstrictor properties, and the ETA receptor antagonist, BQ123, is an effective therapy for hypertension and obese cardiomyopathy (61). Recently, BQ123 was shown to induce PMN-MDSC-mediated immune suppression in dextran sulfate sodium-induced colitis, papain-induced pneumonia, and concanavalin A-induced hepatitis in mice (62). Both the treatment of BQ123, as well as the transfer of BQ123-induced PMN-MDSCs were effective in dampening inflammation (62). Further analysis showed that BQ123 mediates it effects through the IL13/STAT6/Arg1 signaling pathway (62).
2.2.6 β2-agonists
Recently, one promising approach in endogenous MDSC generation was achieved with the help of β2-AR agonists in a murine model of GVHD (52). Treatment with bambuterol – a prodrug of the selective β2-agonist terbutaline – significantly increased the number of MDSCs and Tregs while effector T cells were reduced and GVHD was ameliorated (52). All other mechanisms of action were excluded, and the central role of β2-AR was confirmed (52). Accordingly, in vitro cultivation of MDSCs is also increased by terbutaline (52). Terbutaline and other β2-agonists are used to treat asthma or COPD due to their bronchodilator effect. However, part of their beneficial role also might originate from MDSC generation.
2.2.7 TLR ligands
TLR4 signaling has been shown to play a crucial role in inducing MDSCs (83). In a study on acute type 1 diabetes using non-obese diabetic (NOD) mice, an agonistic TLR4 monoclonal antibody was found to have a protective effect through the induction of MDSCs (84). Furthermore, the adoptive transfer of ex vivo bone marrow cells, stimulated with TLR4 antibody, into NOD mice suppressed acute type 1 diabetes induction as well (84). TLR4 antibody was shown to alter TLR4 signaling, including NFκB signaling, resulting in the downregulation of inflammatory genes and proteins (84). Furthermore, TLR2 activation by Pam2CSK4 was found to enhance immunosuppressive activity of M-MDSCs by upregulating iNOS and NO production, partly through STAT3 activation (85). Successful in vivo generation of MDSCs was also achieved with administration of complete freund’s adjuvant (CFA) containing heat-killed Mycobacterium (M.) tuberculosis in mice (86). Interestingly, a single dose of CFA increased the number of MDSCs but did not increase MDSC activity. However, a second dose of CFA was shown to increase MDSC activity as well. Here, M-MDSC accumulation and activation was found to be favored. M. tuberculosis was shown to play a pivotal role in the induction of MDSC accumulation by CFA, most likely through activation of TLR pathways such as TLR2 and TLR4 (86). Efficient MDSC boosting is also achieved using isolated membrane vesicles (MVs) of the common human gut bacterium P. pentosaceus via the TLR2 pathway (87). Alpdundar Bulut et al. observed upregulation of MDSC numbers, Arg-1 and IL-10 levels and M2-like macrophage differentiation in vitro as well as in several murine models modelling different inflammatory conditions (87). Isolated MV administration was shown to result in improved disease outcome (87). As the role of TLRs in MDSC induction is well documented, it appears to be a logical pharmacological target with potentially promising effects of TLR2/4 antibodies and/or agonists in promoting MDSCs and dampening inflammation (83).
2.2.8 PGE2/RIPK3 inhibitors
Increased PGE2 levels resulted in elevated activity and production of Arg-1, IL-6, VEGF, S100A9 and NO, while it also further inhibited RIPK3, creating a positive feedback loop for MDSC activation (31). Decreased receptor-interacting protein kinase 3 (RIPK3) expression was found to be correlated with increased MDSC number and activity in colorectal and hepatocellular carcinoma, likely mediated by the NF-κB/COX-2/PGE2 axis (31, 78). Inhibition of RIPK3 with GSK872 was shown to prevent immune-mediated hepatitis (79). The authors showed that RIPK3 inhibition led to an increase in MDSCs number, which likely mediated the observed protective effect, as it was lost after MDSC depletion (79). RIPK3 knockdown resulted in increased MDSC recruitment in a mouse model of hepatocellular carcinoma, which could be inhibited by a CXCR2 antagonist (78). All in all, these findings indicate that RIPK3 inhibition may be another promising pharmacological target to promote MDSC recruitment, likely via the NF-κB/COX-2/PGE2 and CXCR2 chemokine axis. Another possible MDSC activation mechanism linked to the NF-κB/COX-2/PGE2 axis may be the target of the PGE2 receptors EP2 and EP4. Both EP2 and EP4 receptors may be promising targets for research by inducing them via their transcription factors or activating them with agonists. The study of Jontvedt Jorgensen et al. highlighted the potential of using this axis as a therapeutical target, as they demonstrated reduced MDSC numbers in murine models of tuberculosis after COX-2 inhibitor administration (56). Recently, we showed that a selective EP4 agonist could generate and activate MDSCs and dampen airway inflammation in a murine model of asthma (57).
2.2.9 Tofacitinib
Tofacitinib is a JAK inhibitor approved for treatment of rheumatoid arthritis (RA), among others. Sendo et al. showed that tofacitinib promoted MDSC expansion and ameliorated chronic inflammation in a murine model of RA-associated interstitial lung disease (ILD) (81). Previously, the same group showed the increase of the number of MDSCs and the following improvement of RA upon tofacitinib administration (82). Tofacitinib treatment was found to inhibit phosphorylation of STAT1 and STAT5, while STAT3 levels remained constant which underlined the role of STAT3 in MDSC activation. The beneficial effect of tofacitinib in ILD may reveal the potential effect of JAK inhibitors on MDSC recruitment.
2.2.10 Quercetin
Quercetin, a natural substance found in many fruits and seeds, has already been shown to have anti-inflammatory properties in the context of autoimmune diseases such as rheumatoid arthritis and inflammatory bowel disease (110–112). Furthermore, Quercetin has been used as an anti-tumor therapy, due to its potential to induce tumor apoptosis and necrosis (59). Recently, Ma et al. showed a controversial correlation between MDSC regulation through quercetin and its anticancer properties (60). Quercetin treatment promoted PMN-MDSC expansion as well as its immunosuppressive activity in a murine model of prostate cancer (60). Mechanistically, quercetin binds to estrogen signaling receptors (ESR), especially ESR2, and exerts downstream phosphorylation of STAT3 in PMN-MDSCs and increased the expression of iNOS, NADPH oxidase and IDO. However, the same study showed that quercetin induces apoptosis in M-MDSCs through the estrogen signaling pathway (60). Nevertheless, quercetin may be a promising compound for the selective induction and activation of PMN-MDSCs through ESR/STAT3 signaling pathway, further supplementing the anti-inflammatory properties associated with quercetin.
2.2.11 Cannabidiol
Recently, Elliott et al. showed that administration of cannabidiol – a non-psychoactive cannabinoid – ameliorated autoimmune encephalomyelitis in mice through the induction of MDSCs (88). Adoptive transfer of in vitro generated MDSCs, treated with CBD, was similarly found to improve encephalomyelitis, while MDSC depletion had the opposite effect. The same group demonstrated similar effects of CBD treatment in autoimmune hepatitis induced mice (89).
2.2.12 Clarithromycin
One study also revealed clarithromycin as a potential target for MDSC induction in vivo (90). Intraperitoneal and oral treatment with clarithromycin was shown to increase the number of MDSCs and prolong their survival in a murine model of LPS endotoxin shock and post-influenza pneumococcal pneumonia. In healthy humans, clarithromycin intake seems to enhance immunosuppressive activity of MDSCs (90).
2.2.13 Taurodeoxycholate
Chang, S. et al. indicated that TDCA – a taurine-conjugated bile acid – can be used to induce MDSCs (91). Here, administration of TDCA was found to improve survival in a mouse model of sepsis, likely through the generation and activation of MDSCs. Still, further research is needed to better understand the underlying mechanisms.
2.2.14 Cyclosporin A
Calcineurin inhibitors such as cyclosporin A or tacrolimus/FK506 are commonly used to treat autoimmune diseases and are administered to allograft recipients to prevent graft rejection. Calcineurin is released from its auto inhibitory loop upon T cell activation by antigen presentation and then dephosphorylates nuclear factor of activated T cells (NFAT) (53). NFAT migrates to the nucleus where it is responsible for the transcription of many pro-inflammatory genes, e.g. IL-2 (53). Besides NFAT, calcineurin inhibitors also target the mitogen-activated protein kinase (MAPK) pathway, and both these pathways play an important role in the myeloid cell lineage (53). The immunosuppressive properties of CsA could be linked to MDSC recruitment in vitro and in vivo (53). Daily treatment with CsA was found to increase MDSCs number, IDO and CXCR2 expression in a murine skin allograft transplantation model (53). Wang et al. suggested that the MDSC regulating effect of CsA is achieved by downregulation of NFATc1 (53). In addition, in skin grafted mice, the combined administration of GM-CSF and CsA was shown to increase MDSCs number and activity, by means of promoting iNOS expression (54). Therefore, calcineurin and NFAT appear to be interesting targets for MDSC regulation and induction.
2.2.15 Glucosamine
Glucosamine is an essential substrate for the glycosylation of proteins and lipids. Recently, glucosamine was shown to promote the generation of MDSCs from murine bone marrow cells in vitro as well as in mice treated for 14 days with intraperitoneal injections of glucosamine (58). Furthermore, glucosamine also increased MDSC activity confirmed by T cell suppression assays and increased levels of Arg-1 and iNOS expression (58). Further analysis showed this effect was likely mediated via the STAT3 and ERK1/2 pathways (58).
2.2.16 Glatirameracetat
GA has been found capable of promoting Tregs and Th2 lineage, while suppressing CD8+ T cell activity, and is therefore frequently used in relapsing-remitting MS (113). Recently, treatment with GA was demonstrated to increase the number and activity of MDSCs in a murine model of inflammatory bowel disease with improving health condition (66). The underlying mechanism was shown to involve binding of GA to the paired immunoglobulin (Ig) -like receptor B (PIR-B) in mice, or to the human ortholog leukocyte immunoglobulin-like receptor-B (LILRB) in humans (66). This interaction inhibited the STAT1 pathway and resulted in an increased IL-10 and TGF-β secretion (66). The results of inhibiting LILRBs in mice tumor models support this finding, since a decrease in MDSC number and STAT6 phosphorylation, a shift of the macrophage balance towards M2-like macrophages as well as increased STAT1/JAK activity had been observed, which all oppose MDSC promotion (67). As the regulation of the myeloid lineage is a highly complex net of signals in which the role of LILRBs is not fully explained yet, further research is needed.
2.3 Potential pharmacological targets on MDSCs in cancer
MDSCs accumulate in cancer and promote invasion, angiogenesis, metastasis formation and reduce the effectiveness of anti-tumor immunity (1, 3). Considering the negative role of MDSCs in the tumor microenvironment, one of the main goals of cancer research has been to suppress both MDSCs number and activity (1, 3). Many pharmacological targets have already been identified, which were shown to be involved in modulating the number and activity of MDSCs in different types of cancer, and were able to be targeted with different compounds (Table 2). As this review focusses on promoting MDSCs number and activity instead of inhibiting, in order to promote the anti-inflammatory immunity, the suggested MDSC-suppressing drugs are of course not of interest. However, the same targets that are used to suppress MDSCs in the context of the tumor microenvironment, might also be targeted in the complete opposite direction, in order to promote MDSCs in the context of potentially beneficial anti-inflammatory immunity. Several pharmacological targets that were manipulated to reduce the number and/or activity of MDSCs in the context of cancer, such as TLR4, β2-AR, EP4 and CXCR2, have already been described as potential targets for promoting the number and/or activity of MDSCs, as discussed above. For example, a β2-AR blocker, propranolol, was shown to reduce MDSC number and activity (20), while a β2-AR agonist, terbutaline, was shown to have the opposite effect (52). Similarly, the EP4 receptor antagonists, E7046 and YY001, were shown to reduce MDSC number and activity in the context of cancer (181, 182), while a EP4 receptor agonist, L-902,688, was shown to have the opposite effect in a murine model of asthma (57). However, there remain pharmacological targets of MDSC inhibition, which were identified in cancer research, that have so far not been studied in the context of MDSC promotion yet may provide efficient targets in novel anti-inflammatory therapies through generation and activation of these immune suppressive cells.
3 Discussion
Findings on the modulation of the generation, recruitment, and activation of MDSCs open the door to novel pharmacological approaches that can be used to dampen inflammation in a variety of diseases or conditions characterized by excessive and detrimental immune responses (such as autoimmune diseases, chronic inflammatory diseases, transplantation and GVHD). Taking all the mentioned mechanisms and the potential pharmacological targets into consideration, the in vitro generation of MDSCs combined with adoptive transfer (MDSC cell therapy) as well as the in vivo recruitment and activation of endogenous MDSCs seem to both be promising approaches. Nonetheless, cell therapies always bear additional risks, e.g. reaction of the immune system against transferred cells, and adverse side effects, and, as a result, need to be studied carefully before applying it to the clinical setting. Alternatively, the promotion of endogenous MDSCs could create safer, easier, and potentially equally or more efficient MDSC-targeted therapies in the future.
On the one hand, in vitro generation of MDSCs with the help of cytokines – like GM-CSF and IL-6 – or pharmacological compounds – such as PGE2 –, followed by adoptive transfer is an effective and increasingly established treatment in murine models of chronic inflammatory diseases, autoimmune diseases, and allograft transplantation. Here, it is possible to circumvent the potential systemic side effects of unspecific pharmacological compounds and transfer a purified subset of immunosuppressive cells to dampen inflammation. On the other hand, efficient endogenous MDSC generation and activation was achieved with a double CFA injection containing M. tuberculosis or MVs through the TLR pathway, mTOR inhibitor rapamycin, dexamethasone, GA, EP4 receptor agonist as well as with other medications (48, 56, 57, 63, 64, 66, 67, 79, 81, 82, 84, 86, 87). Furthermore, many chemokines, and their receptors, have been identified to be involved in MDSC recruitment, with the most noteworthy chemokine being CXCR2, with its most important ligands CXCL1 and CXCL2. CXCR2 ligands can be used to regulate the migration of generated MDSCs to the site of interest, e.g. the intestines in Crohn’s disease, or the lungs of asthmatic patients. In this context, allografts could be coated with chemokine agonists to promote local MDSC accumulation - similar to vascular stents coated with anticoagulants and anti-proliferative drugs, like rapamycin. However, the in vivo generation of endogenous MDSCs may be a more straightforward therapy compared to MDSC cell therapy, which would ideally be accomplished with a selective drug and pharmacological targets, in order to reduce possible side effects as much as possible.
The safety of adoptively transferred MDSCs is not very well known and remains one of the primary challenges in bringing MDSC therapy to a clinical setting (355). Similarly, the safety of inducing endogenous MDSCs remains largely unknown. One concern may be the immature characteristic of MDSCs, which makes them susceptible to the induction of differentiation into other immune cells, such as macrophages, neutrophils or DCs, depending on specific microenvironmental cues. The negative role of MDSCs in the context of cancer also raises the following questions: Can MDSC inducing therapy only be applied in patients who are “certainly tumor-free”? And if so, how is it possible to identify this patient group? Or is the expected beneficial effect so great that the increased risk of metastasis can be accepted? Is there a way to inhibit MDSCs in undesired parts of the body? As the vast majority of studies on MDSCs are cancer-related and the systemic MDSC induction has been linked to tumor progression, metastasis and impaired survival, a method of local induction of MDSCs would be necessary to reduce the risk of MDSC-induced cancer progression as well as potential other side effects of systemic immunosuppression, e.g. opportunistic infections.
The increasing number of potential pharmacological targets that have been shown to be involved in modulating the number and activity of MDSCs provide significant number of opportunities for novel pharmacological approaches. Especially considering the potential targets, which were previously mainly studied in the context of cancer (Table 2), to inhibit the MDSC response, where this effect may be reversed (e.g. changing from an agonist to antagonist or the other way around), in order to promote the MDSC response. The interest in the modulation of MDSC outside the context of cancer is also increasing, as the importance of MDSCs in many different immune-related pathological conditions is being unraveled (356). Furthermore, transplantation research has also increased its attention towards MDSC immunomodulation as a promising candidate to increase tolerance and improve transplant outcome (357). Thus, the pharmacological approaches which can be applied in MDSC modulation, as discussed in this review, may provide novel opportunities in the future of tolerance-inducing agents in the context of transplantation.
Taken together, there are many different promising pharmacological targets to generate and activate MDSCs, and their beneficial potential in certain pathological conditions has been established in animal studies. Therefore, MDSC therapies may prove to be effective alternatives to other immunosuppressive therapies. The selective harnessing of regulatory immune cells, here expanding suppressive activity of MDSCs on T cells, may bring an advanced possibility to protect, limit or ameliorate the initiation or progression of autoimmunity, inflammation, transplant rejection or GVHD, promote inflammation resolution and transplantation tolerance and pave the way toward precise medical therapy in inflammation/autoimmunity/transplant medicine.
Author contributions
SK conceptualized the review. CG, CH, AD and SK contributed to the original draft. CG and SK contributed to revising and final approval of the manuscript. All authors contributed to the article and approved the submitted version.
Funding
This study was funded by the Universities Giessen Marburg Lung Center (UGMLC) and the German Center for Lung Disease (DZL German Lung Center, no. 82DZL005B2), the Foundation for Pathobiochemistry and Molecular Diagnostics grant and the ƒortüne program of the University of Tuebingen (# 2458-0-0, # 2606-0-0) for SK. Open access was funded by the Open Access Publishing Fund of Philipps-Universität Marburg with support of the Deutsche Forschungsgemeinschaft (DFG, German Research Foundation) and the Open Access Publishing Fund of the University of Tübingen.
Conflict of interest
The authors declare that the research was conducted in the absence of any commercial or financial relationships that could be construed as a potential conflict of interest.
Publisher’s note
All claims expressed in this article are solely those of the authors and do not necessarily represent those of their affiliated organizations, or those of the publisher, the editors and the reviewers. Any product that may be evaluated in this article, or claim that may be made by its manufacturer, is not guaranteed or endorsed by the publisher.
Footnotes
References
1. Gabrilovich DI, Nagaraj S. Myeloid-derived suppressor cells as regulators of the immune system. Nat Rev Immunol (2009) 9(3):162–74. doi: 10.1038/nri2506
2. Bronte V, Apolloni E, Cabrelle A, Ronca R, Serafini P, Zamboni P, et al. Identification of a Cd11b(+)/Gr-1(+)/Cd31(+) myeloid progenitor capable of activating or suppressing Cd8(+) T cells. Blood (2000) 96(12):3838–46. doi: 10.1182/blood.V96.12.3838
3. Talmadge JE, Gabrilovich DI. History of myeloid-derived suppressor cells. Nat Rev Cancer (2013) 13(10):739–52. doi: 10.1038/nrc3581
4. Sun L, Clavijo PE, Robbins Y, Patel P, Friedman J, Greene S, et al. Inhibiting myeloid-derived suppressor cell trafficking enhances T cell immunotherapy. JCI Insight (2019) 4(7):e126853. doi: 10.1172/jci.insight.126853
5. Albeituni SH, Ding C, Yan J. Hampering immune suppressors: Therapeutic targeting of myeloid-derived suppressor cells in cancer. Cancer J (2013) 19(6):490–501. doi: 10.1097/PPO.0000000000000006
6. Dorhoi A, Du Plessis N. Monocytic myeloid-derived suppressor cells in chronic infections. Front Immunol (2017) 8:1895. doi: 10.3389/fimmu.2017.01895
7. Cripps JG, Gorham JD. Mdsc in autoimmunity. Int Immunopharmacol (2011) 11(7):789–93. doi: 10.1016/j.intimp.2011.01.026
8. Deshane JS, Redden DT, Zeng M, Spell ML, Zmijewski JW, Anderson JT, et al. Subsets of airway myeloid-derived regulatory cells distinguish mild asthma from chronic obstructive pulmonary disease. J Allergy Clin Immunol (2015) 135(2):413–24 e15. doi: 10.1016/j.jaci.2014.08.040
9. Clements VK, Long T, Long R, Figley C, Smith DMC, Ostrand-Rosenberg S. Frontline science: High fat diet and leptin promote tumor progression by inducing myeloid-derived suppressor cells. J Leukoc Biol (2018) 103(3):395–407. doi: 10.1002/JLB.4HI0517-210R
10. Pawelec G, Verschoor CP, Ostrand-Rosenberg S. Myeloid-derived suppressor cells: Not only in tumor immunity. Front Immunol (2019) 10:1099. doi: 10.3389/fimmu.2019.01099
11. Bronte V, Brandau S, Chen SH, Colombo MP, Frey AB, Greten TF, et al. Recommendations for myeloid-derived suppressor cell nomenclature and characterization standards. Nat Commun (2016) 7:12150. doi: 10.1038/ncomms12150
12. Condamine T, Dominguez GA, Youn JI, Kossenkov AV, Mony S, Alicea-Torres K, et al. Lectin-type oxidized ldl receptor-1 distinguishes population of human polymorphonuclear myeloid-derived suppressor cells in cancer patients. Sci Immunol (2016) 1(2):aaf8943. doi: 10.1126/sciimmunol.aaf8943
13. Alshetaiwi H, Pervolarakis N, McIntyre LL, Ma D, Nguyen Q, Rath JA, et al. Defining the emergence of myeloid-derived suppressor cells in breast cancer using single-cell transcriptomics. Sci Immunol (2020) 5(44):eaay6017. doi: 10.1126/sciimmunol.aay6017
14. Katzenelenbogen Y, Sheban F, Yalin A, Yofe I, Svetlichnyy D, Jaitin DA, et al. Coupled scrna-seq and intracellular protein activity reveal an immunosuppressive role of Trem2 in cancer. Cell (2020) 182(4):872–85 e19. doi: 10.1016/j.cell.2020.06.032
15. Condamine T, Mastio J, Gabrilovich DI. Transcriptional regulation of myeloid-derived suppressor cells. J Leukoc Biol (2015) 98(6):913–22. doi: 10.1189/jlb.4RI0515-204R
16. Thorn M, Guha P, Cunetta M, Espat NJ, Miller G, Junghans RP, et al. Tumor-associated gm-csf overexpression induces immunoinhibitory molecules Via Stat3 in myeloid-suppressor cells infiltrating liver metastases. Cancer Gene Ther (2016) 23(6):188–98. doi: 10.1038/cgt.2016.19
17. Peng D, Tanikawa T, Li W, Zhao L, Vatan L, Szeliga W, et al. Myeloid-derived suppressor cells endow stem-like qualities to breast cancer cells through Il6/Stat3 and No/Notch cross-talk signaling. Cancer Res (2016) 76(11):3156–65. doi: 10.1158/0008-5472.CAN-15-2528
18. Li W, Zhang X, Chen Y, Xie Y, Liu J, Feng Q, et al. G-Csf is a key modulator of mdsc and could be a potential therapeutic target in colitis-associated colorectal cancers. Protein Cell (2016) 7(2):130–40. doi: 10.1007/s13238-015-0237-2
19. Waight JD, Netherby C, Hensen ML, Miller A, Hu Q, Liu S, et al. Myeloid-derived suppressor cell development is regulated by a Stat/Irf-8 axis. J Clin Invest (2013) 123(10):4464–78. doi: 10.1172/JCI68189
20. Mohammadpour H, MacDonald CR, Qiao G, Chen M, Dong B, Hylander BL, et al. Beta2 adrenergic receptor-mediated signaling regulates the immunosuppressive potential of myeloid-derived suppressor cells. J Clin Invest (2019) 129(12):5537–52. doi: 10.1172/JCI129502
21. Moreira D, Adamus T, Zhao X, Su YL, Zhang Z, White SV, et al. Stat3 inhibition combined with cpg immunostimulation activates antitumor immunity to eradicate genetically distinct castration-resistant prostate cancers. Clin Cancer Res (2018) 24(23):5948–62. doi: 10.1158/1078-0432.CCR-18-1277
22. Hellsten R, Lilljebjorn L, Johansson M, Leandersson K, Bjartell A. The Stat3 inhibitor galiellalactone inhibits the generation of mdsc-like monocytes by prostate cancer cells and decreases immunosuppressive and tumorigenic factors. Prostate (2019) 79(14):1611–21. doi: 10.1002/pros.23885
23. Guha P, Gardell J, Darpolor J, Cunetta M, Lima M, Miller G, et al. Stat3 inhibition induces bax-dependent apoptosis in liver tumor myeloid-derived suppressor cells. Oncogene (2019) 38(4):533–48. doi: 10.1038/s41388-018-0449-z
24. Mundy-Bosse BL, Lesinski GB, Jaime-Ramirez AC, Benninger K, Khan M, Kuppusamy P, et al. Myeloid-derived suppressor cell inhibition of the ifn response in tumor-bearing mice. Cancer Res (2011) 71(15):5101–10. doi: 10.1158/0008-5472.CAN-10-2670
25. Schroder M, Krotschel M, Conrad L, Naumann SK, Bachran C, Rolfe A, et al. Genetic screen in myeloid cells identifies tnf-alpha autocrine secretion as a factor increasing mdsc suppressive activity Via Nos2 up-regulation. Sci Rep (2018) 8(1):13399. doi: 10.1038/s41598-018-31674-1
26. Porta C, Consonni FM, Morlacchi S, Sangaletti S, Bleve A, Totaro MG, et al. Tumor-derived prostaglandin E2 promotes P50 nf-Kappab-Dependent differentiation of monocytic mdscs. Cancer Res (2020) 80(13):2874–88. doi: 10.1158/0008-5472.CAN-19-2843
27. Tu S, Bhagat G, Cui G, Takaishi S, Kurt-Jones EA, Rickman B, et al. Overexpression of interleukin-1beta induces gastric inflammation and cancer and mobilizes myeloid-derived suppressor cells in mice. Cancer Cell (2008) 14(5):408–19. doi: 10.1016/j.ccr.2008.10.011
28. Hu CE, Gan J, Zhang RD, Cheng YR, Huang GJ. Up-regulated myeloid-derived suppressor cell contributes to hepatocellular carcinoma development by impairing dendritic cell function. Scand J Gastroenterol (2011) 46(2):156–64. doi: 10.3109/00365521.2010.516450
29. Tannenbaum CS, Rayman PA, Pavicic PG, Kim JS, Wei W, Polefko A, et al. Mediators of inflammation-driven expansion, trafficking, and function of tumor-infiltrating mdscs. Cancer Immunol Res (2019) 7(10):1687–99. doi: 10.1158/2326-6066.CIR-18-0578
30. Choi JN, Sun EG, Cho SH. Il-12 enhances immune response by modulation of myeloid derived suppressor cells in tumor microenvironment. Chonnam Med J (2019) 55(1):31–9. doi: 10.4068/cmj.2019.55.1.31
31. Yan G, Zhao H, Zhang Q, Zhou Y, Wu L, Lei J, et al. A Ripk3-Pge2 circuit mediates myeloid-derived suppressor cell-potentiated colorectal carcinogenesis. Cancer Res (2018) 78(19):5586–99. doi: 10.1158/0008-5472.CAN-17-3962
32. Cheng P, Kumar V, Liu H, Youn JI, Fishman M, Sherman S, et al. Effects of notch signaling on regulation of myeloid cell differentiation in cancer. Cancer Res (2014) 74(1):141–52. doi: 10.1158/0008-5472.CAN-13-1686
33. Morello S, Miele L. Targeting the adenosine A2b receptor in the tumor microenvironment overcomes local immunosuppression by myeloid-derived suppressor cells. Oncoimmunology (2014) 3:e27989. doi: 10.4161/onci.27989
34. Tcyganov E, Mastio J, Chen E, Gabrilovich DI. Plasticity of myeloid-derived suppressor cells in cancer. Curr Opin Immunol (2018) 51:76–82. doi: 10.1016/j.coi.2018.03.009
35. Li BH, Garstka MA, Li ZF. Chemokines and their receptors promoting the recruitment of myeloid-derived suppressor cells into the tumor. Mol Immunol (2020) 117:201–15. doi: 10.1016/j.molimm.2019.11.014
36. Hsu YL, Yen MC, Chang WA, Tsai PH, Pan YC, Liao SH, et al. Cxcl17-derived Cd11b(+)Gr-1(+) myeloid-derived suppressor cells contribute to lung metastasis of breast cancer through platelet-derived growth factor-bb. Breast Cancer Res (2019) 21(1):23. doi: 10.1186/s13058-019-1114-3
37. Oliveira AC, Fu C, Lu Y, Williams MA, Pi L, Brantly ML, et al. Chemokine signaling axis between endothelial and myeloid cells regulates development of pulmonary hypertension associated with pulmonary fibrosis and hypoxia. Am J Physiol Lung Cell Mol Physiol (2019) 317(4):L434–L44. doi: 10.1152/ajplung.00156.2019
38. Taki M, Abiko K, Baba T, Hamanishi J, Yamaguchi K, Murakami R, et al. Snail promotes ovarian cancer progression by recruiting myeloid-derived suppressor cells Via Cxcr2 ligand upregulation. Nat Commun (2018) 9(1):1685. doi: 10.1038/s41467-018-03966-7
39. Neamah WH, Singh NP, Alghetaa H, Abdulla OA, Chatterjee S, Busbee PB, et al. Ahr activation leads to massive mobilization of myeloid-derived suppressor cells with immunosuppressive activity through regulation of Cxcr2 and microrna mir-150-5p and mir-543-3p that target anti-inflammatory genes. J Immunol (2019) 203(7):1830–44. doi: 10.4049/jimmunol.1900291
40. Zhang C, Wang S, Li J, Zhang W, Zheng L, Yang C, et al. The mtor signal regulates myeloid-derived suppressor cells differentiation and immunosuppressive function in acute kidney injury. Cell Death Dis (2017) 8(3):e2695. doi: 10.1038/cddis.2017.86
41. Katoh H, Wang D, Daikoku T, Sun H, Dey SK, Dubois RN. Cxcr2-expressing myeloid-derived suppressor cells are essential to promote colitis-associated tumorigenesis. Cancer Cell (2013) 24(5):631–44. doi: 10.1016/j.ccr.2013.10.009
42. Han X, Shi H, Sun Y, Shang C, Luan T, Wang D, et al. Cxcr2 expression on granulocyte and macrophage progenitors under tumor conditions contributes to Mo-mdsc generation Via Sap18/Erk/Stat3. Cell Death Dis (2019) 10(8):598. doi: 10.1038/s41419-019-1837-1
43. Ohl K, Tenbrock K. Reactive oxygen species as regulators of mdsc-mediated immune suppression. Front Immunol (2018) 9:2499. doi: 10.3389/fimmu.2018.02499
44. Corzo CA, Cotter MJ, Cheng P, Cheng F, Kusmartsev S, Sotomayor E, et al. Mechanism regulating reactive oxygen species in tumor-induced myeloid-derived suppressor cells. J Immunol (2009) 182(9):5693–701. doi: 10.4049/jimmunol.0900092
45. Rodriguez PC, Quiceno DG, Ochoa AC. L-arginine availability regulates T-lymphocyte cell-cycle progression. Blood (2007) 109(4):1568–73. doi: 10.1182/blood-2006-06-031856
46. Yu J, Du W, Yan F, Wang Y, Li H, Cao S, et al. Myeloid-derived suppressor cells suppress antitumor immune responses through ido expression and correlate with lymph node metastasis in patients with breast cancer. J Immunol (2013) 190(7):3783–97. doi: 10.4049/jimmunol.1201449
47. Huang B, Pan PY, Li Q, Sato AI, Levy DE, Bromberg J, et al. Gr-1+Cd115+ immature myeloid suppressor cells mediate the development of tumor-induced T regulatory cells and T-cell anergy in tumor-bearing host. Cancer Res (2006) 66(2):1123–31. doi: 10.1158/0008-5472.CAN-05-1299
48. Nakamura T, Nakao T, Yoshimura N, Ashihara E. Rapamycin prolongs cardiac allograft survival in a mouse model by inducing myeloid-derived suppressor cells. Am J Transplant (2015) 15(9):2364–77. doi: 10.1111/ajt.13276
49. Wang Y, Schafer CC, Hough KP, Tousif S, Duncan SR, Kearney JF, et al. Myeloid-derived suppressor cells impair b cell responses in lung cancer through il-7 and Stat5. J Immunol (2018) 201(1):278–95. doi: 10.4049/jimmunol.1701069
50. Youn JI, Nagaraj S, Collazo M, Gabrilovich DI. Subsets of myeloid-derived suppressor cells in tumor-bearing mice. J Immunol (2008) 181(8):5791–802. doi: 10.4049/jimmunol.181.8.5791
51. Raber PL, Thevenot P, Sierra R, Wyczechowska D, Halle D, Ramirez ME, et al. Subpopulations of myeloid-derived suppressor cells impair T cell responses through independent nitric oxide-related pathways. Int J Cancer (2014) 134(12):2853–64. doi: 10.1002/ijc.28622
52. Mohammadpour H, Sarow JL, MacDonald CR, Chen GL, Qiu J, Sharma UC, et al. Beta2-adrenergic receptor activation on donor cells ameliorates acute gvhd. JCI Insight (2020) 5(12):e137788. doi: 10.1172/jci.insight.137788
53. Wang X, Bi Y, Xue L, Liao J, Chen X, Lu Y, et al. The calcineurin-nfat axis controls allograft immunity in myeloid-derived suppressor cells through reprogramming T cell differentiation. Mol Cell Biol (2015) 35(3):598–609. doi: 10.1128/MCB.01251-14
54. Han C, Wu T, Na N, Zhao Y, Li W, Zhao Y. The effect of immunosuppressive drug cyclosporine a on myeloid-derived suppressor cells in transplanted mice. Inflamm Res (2016) 65(9):679–88. doi: 10.1007/s00011-016-0949-7
55. Obermajer N, Muthuswamy R, Lesnock J, Edwards RP, Kalinski P. Positive feedback between Pge2 and Cox2 redirects the differentiation of human dendritic cells toward stable myeloid-derived suppressor cells. Blood (2011) 118(20):5498–505. doi: 10.1182/blood-2011-07-365825
56. Jontvedt Jorgensen M, Jenum S, Tonby K, Mortensen R, Walzl G, Du Plessis N, et al. Monocytic myeloid-derived suppressor cells reflect tuberculosis severity and are influenced by cyclooxygenase-2 inhibitors. J Leukoc Biol (2020) 110(1):177–86. doi: 10.1002/JLB.4A0720-409RR
57. van Geffen C, Deißler A, Beer-Hammer S, Nürnberg B, Handgretinger R, Renz H, et al. Myeloid-derived suppressor cells dampen airway inflammation through prostaglandin E2 receptor 4. Front Immunol (2021) 12:695933. doi: 10.3389/fimmu.2021.695933
58. Lin EC, Chen SW, Chen LK, Lin TA, Wu YX, Juan CC, et al. Glucosamine interferes with myelopoiesis and enhances the immunosuppressive activity of myeloid-derived suppressor cells. Front Nutr (2021) 8:762363. doi: 10.3389/fnut.2021.762363
59. Vafadar A, Shabaninejad Z, Movahedpour A, Fallahi F, Taghavipour M, Ghasemi Y, et al. Quercetin and cancer: New insights into its therapeutic effects on ovarian cancer cells. Cell Biosci (2020) 10:32. doi: 10.1186/s13578-020-00397-0
60. Ma Z, Xia Y, Hu C, Yu M, Yi H. Quercetin promotes the survival of granulocytic myeloid-derived suppressor cells via the Esr2/Stat3 signaling pathway. BioMed Pharmacother (2020) 125:109922. doi: 10.1016/j.biopha.2020.109922
61. Davenport AP, Hyndman KA, Dhaun N, Southan C, Kohan DE, Pollock JS, et al. Endothelin. Pharmacol Rev (2016) 68(2):357–418. doi: 10.1124/pr.115.011833
62. Chen Z, Zhang X, Lv S, Xing Z, Shi M, Li X, et al. Treatment with endothelin-a receptor antagonist Bq123 attenuates acute inflammation in mice through T-Cell-Dependent polymorphonuclear myeloid-derived suppressor cell activation. Front Immunol (2021) 12:641874. doi: 10.3389/fimmu.2021.641874
63. Zhao Y, Shen XF, Cao K, Ding J, Kang X, Guan WX, et al. Dexamethasone-induced myeloid-derived suppressor cells prolong allo cardiac graft survival through inos- and glucocorticoid receptor-dependent mechanism. Front Immunol (2018) 9:282. doi: 10.3389/fimmu.2018.00282
64. Liao J, Wang X, Bi Y, Shen B, Shao K, Yang H, et al. Dexamethasone potentiates myeloid-derived suppressor cell function in prolonging allograft survival through nitric oxide. J Leukoc Biol (2014) 96(5):675–84. doi: 10.1189/jlb.2HI1113-611RR
65. Wang Z, Zheng G, Li G, Wang M, Ma Z, Li H, et al. Methylprednisolone alleviates multiple sclerosis by expanding myeloid-derived suppressor cells via glucocorticoid receptor beta and S100a8/9 up-regulation. J Cell Mol Med (2020) 24(23):13703–14. doi: 10.1111/jcmm.15928
66. van der Touw W, Kang K, Luan Y, Ma G, Mai S, Qin L, et al. Glatiramer acetate enhances myeloid-derived suppressor cell function Via recognition of paired ig-like receptor b. J Immunol (2018) 201(6):1727–34. doi: 10.4049/jimmunol.1701450
67. Chen HM, van der Touw W, Wang YS, Kang K, Mai S, Zhang J, et al. Blocking immunoinhibitory receptor Lilrb2 reprograms tumor-associated myeloid cells and promotes antitumor immunity. J Clin Invest (2018) 128(12):5647–62. doi: 10.1172/JCI97570
68. Liu Y, Perego M, Xiao Q, He Y, Fu S, He J, et al. Lactoferrin-induced myeloid-derived suppressor cell therapy attenuates pathologic inflammatory conditions in newborn mice. J Clin Invest (2019) 129(10):4261–75. doi: 10.1172/JCI128164
69. Zhou L, Miao K, Yin B, Li H, Fan J, Zhu Y, et al. Cardioprotective role of myeloid-derived suppressor cells in heart failure. Circulation (2018) 138(2):181–97. doi: 10.1161/CIRCULATIONAHA.117.030811
70. Scheurer J, Reisser T, Leithauser F, Messmann JJ, Holzmann K, Debatin KM, et al. Rapamycin-based graft-Versus-Host disease prophylaxis increases the immunosuppressivity of myeloid-derived suppressor cells without affecting T cells and anti-tumor cytotoxicity. Clin Exp Immunol (2020) 202(3):407–22. doi: 10.1111/cei.13496
71. Wei C, Wang Y, Ma L, Wang X, Chi H, Zhang S, et al. Rapamycin nano-micelle ophthalmic solution reduces corneal allograft rejection by potentiating myeloid-derived suppressor cells' function. Front Immunol (2018) 9:2283. doi: 10.3389/fimmu.2018.02283
72. Zhang Y, Bi Y, Yang H, Chen X, Liu H, Lu Y, et al. Mtor limits the recruitment of Cd11b+Gr1+Ly6chigh myeloid-derived suppressor cells in protecting against murine immunological hepatic injury. J Leukoc Biol (2014) 95(6):961–70. doi: 10.1189/jlb.0913473
73. Deissler A, Della Penna A, van Geffen C, Gonzalez-Menendez I, Quintanilla-Martinez L, Gunther A, et al. Rapamycin delays allograft rejection in obese graft recipients through induction of myeloid-derived suppressor cells. Immunol Lett (2021) 236:1–11. doi: 10.1016/j.imlet.2021.05.003
74. Li Y, Xu Y, Liu X, Yan X, Lin Y, Tan Q, et al. Mtor inhibitor Ink128 promotes wound healing by regulating mdscs. Stem Cell Res Ther (2021) 12(1):170. doi: 10.1186/s13287-021-02206-y
75. Li J, Chen J, Zhang M, Zhang C, Wu R, Yang T, et al. The mtor deficiency in monocytic myeloid-derived suppressor cells protects mouse cardiac allografts by inducing allograft tolerance. Front Immunol (2021) 12:661338. doi: 10.3389/fimmu.2021.661338
76. Li X, Li Y, Yu Q, Xu L, Fu S, Wei C, et al. Mtor signaling regulates the development and therapeutic efficacy of pmn-mdscs in acute gvhd. Front Cell Dev Biol (2021) 9:741911. doi: 10.3389/fcell.2021.741911
77. Jia A, Wang Y, Wang Y, Li Y, Yang Q, Cao Y, et al. The kinase Akt1 potentiates the suppressive functions of myeloid-derived suppressor cells in inflammation and cancer. Cell Mol Immunol (2021) 18(4):1074–76. doi: 10.1038/s41423-020-00610-7
78. Li YM, Liu ZY, Wang JC, Yu JM, Li ZC, Yang HJ, et al. Receptor-interacting protein kinase 3 deficiency recruits myeloid-derived suppressor cells to hepatocellular carcinoma through the chemokine (C-X-C motif) ligand 1-chemokine (C-X-C motif) receptor 2 axis. Hepatology (2019) 70(5):1564–81. doi: 10.1002/hep.30676
79. Liu M, Zhang H, Zhang L, Liu X, Zhou S, Wang X, et al. Rip3 blockade prevents immune-mediated hepatitis through a myeloid-derived suppressor cell dependent mechanism. Int J Biol Sci (2022) 18(1):199–213. doi: 10.7150/ijbs.65402
80. Lusthaus M, Mazkereth N, Donin N, Fishelson Z. Receptor-interacting protein kinases 1 and 3, and mixed lineage kinase domain-like protein are activated by sublytic complement and participate in complement-dependent cytotoxicity. Front Immunol (2018) 9:306. doi: 10.3389/fimmu.2018.00306
81. Sendo S, Saegusa J, Yamada H, Nishimura K, Morinobu A. Tofacitinib facilitates the expansion of myeloid-derived suppressor cells and ameliorates interstitial lung disease in skg mice. Arthritis Res Ther (2019) 21(1):184. doi: 10.1186/s13075-019-1963-2
82. Nishimura K, Saegusa J, Matsuki F, Akashi K, Kageyama G, Morinobu A. Tofacitinib facilitates the expansion of myeloid-derived suppressor cells and ameliorates arthritis in skg mice. Arthritis Rheumatol (2015) 67(4):893–902. doi: 10.1002/art.39007
83. Li J, Yang F, Wei F, Ren X. The role of toll-like receptor 4 in tumor microenvironment. Oncotarget (2017) 8(39):66656–67. doi: 10.18632/oncotarget.19105
84. Locker KCS, Kachapati K, Wu Y, Bednar KJ, Adams D, Patel C, et al. Endosomal sequestration of Tlr4 antibody induces myeloid-derived suppressor cells and reverses acute type 1 diabetes. Diabetes (2022) 71(3):470–82. doi: 10.2337/db21-0426
85. Zhan X, Jiang X, He Q, Zhong L, Wang Y, Huang Y, et al. Pam2 lipopeptides enhance the immunosuppressive activity of monocytic myeloid-derived suppressor cells by Stat3 signal in chronic inflammation. Cent Eur J Immunol (2022) 47(1):30–40. doi: 10.5114/ceji.2022.113086
86. Ribechini E, Eckert I, Beilhack A, Du Plessis N, Walzl G, Schleicher U, et al. Heat-killed mycobacterium tuberculosis prime-boost vaccination induces myeloid-derived suppressor cells with spleen dendritic cell-killing capability. JCI Insight (2019) 5(13):e128664. doi: 10.1172/jci.insight.128664
87. Alpdundar Bulut E, Bayyurt Kocabas B, Yazar V, Aykut G, Guler U, Salih B, et al. Human gut commensal membrane vesicles modulate inflammation by generating M2-like macrophages and myeloid-derived suppressor cells. J Immunol (2020) 205(10):2707–18. doi: 10.4049/jimmunol.2000731
88. Elliott DM, Singh N, Nagarkatti M, Nagarkatti PS. Cannabidiol attenuates experimental autoimmune encephalomyelitis model of multiple sclerosis through induction of myeloid-derived suppressor cells. Front Immunol (2018) 9:1782. doi: 10.3389/fimmu.2018.01782
89. Hegde VL, Nagarkatti PS, Nagarkatti M. Role of myeloid-derived suppressor cells in amelioration of experimental autoimmune hepatitis following activation of Trpv1 receptors by cannabidiol. PloS One (2011) 6(4):e18281. doi: 10.1371/journal.pone.0018281
90. Namkoong H, Ishii M, Fujii H, Yagi K, Asami T, Asakura T, et al. Clarithromycin expands Cd11b+Gr-1+ cells Via the Stat3/Bv8 axis to ameliorate lethal endotoxic shock and post-influenza bacterial pneumonia. PloS Pathog (2018) 14(4):e1006955. doi: 10.1371/journal.ppat.1006955
91. Chang S, Kim YH, Kim YJ, Kim YW, Moon S, Lee YY, et al. Taurodeoxycholate increases the number of myeloid-derived suppressor cells that ameliorate sepsis in mice. Front Immunol (2018) 9:1984. doi: 10.3389/fimmu.2018.01984
92. Bashor CJ, Hilton IB, Bandukwala H, Smith DM, Veiseh O. Engineering the next generation of cell-based therapeutics. Nat Rev Drug Discovery (2022) (online ahead of print):1–21. doi: 10.1038/s41573-022-00476-6
93. Lee YS, Zhang T, Saxena V, Li L, Piao W, Bromberg JS, et al. Myeloid-derived suppressor cells expand after transplantation and their augmentation increases graft survival. Am J Transplant (2020) 20(9):2343–55. doi: 10.1111/ajt.15879
94. Ren Y, Dong X, Zhao H, Feng J, Chen B, Zhou Y, et al. Myeloid-derived suppressor cells improve corneal graft survival through suppressing angiogenesis and lymphangiogenesis. Am J Transplant (2021) 21(2):552–66. doi: 10.1111/ajt.16291
95. Mildner A, Mack M, Schmidt H, Bruck W, Djukic M, Zabel MD, et al. Ccr2+Ly-6chi monocytes are crucial for the effector phase of autoimmunity in the central nervous system. Brain (2009) 132(Pt 9):2487–500. doi: 10.1093/brain/awp144
96. Jeong HJ, Lee HJ, Ko JH, Cho BJ, Park SY, Park JW, et al. Myeloid-derived suppressor cells mediate inflammation resolution in humans and mice with autoimmune uveoretinitis. J Immunol (2018) 200(4):1306–15. doi: 10.4049/jimmunol.1700617
97. Park MJ, Lee SH, Kim EK, Lee EJ, Park SH, Kwok SK, et al. Myeloid-derived suppressor cells induce the expansion of regulatory b cells and ameliorate autoimmunity in the sanroque mouse model of systemic lupus erythematosus. Arthritis Rheumatol (2016) 68(11):2717–27. doi: 10.1002/art.39767
98. Li Y, Tu Z, Qian S, Fung JJ, Markowitz SD, Kusner LL, et al. Myeloid-derived suppressor cells as a potential therapy for experimental autoimmune myasthenia gravis. J Immunol (2014) 193(5):2127–34. doi: 10.4049/jimmunol.1400857
99. Chiasson VL, Bounds KR, Chatterjee P, Manandhar L, Pakanati AR, Hernandez M, et al. Myeloid-derived suppressor cells ameliorate cyclosporine a-induced hypertension in mice. Hypertension (2018) 71(1):199–207. doi: 10.1161/HYPERTENSIONAHA.117.10306
100. van Geffen C, Deissler A, Beer-Hammer S, Nurnberg B, Handgretinger R, Renz H, et al. Myeloid-derived suppressor cells dampen airway inflammation through prostaglandin E2 receptor 4.Front Immunol (2021) 12:695933. doi: 10.3389/fimmu.2021.695933
101. Park MY, Lim BG, Kim SY, Sohn HJ, Kim S, Kim TG. Gm-csf promotes the expansion and differentiation of cord blood myeloid-derived suppressor cells, which attenuate xenogeneic graft-Vs.-Host disease. Front Immunol (2019) 10:183. doi: 10.3389/fimmu.2019.00183
102. Hsieh CC, Lin CL, He JT, Chiang M, Wang Y, Tsai YC, et al. Administration of cytokine-induced myeloid-derived suppressor cells ameliorates renal fibrosis in diabetic mice. Stem Cell Res Ther (2018) 9(1):183. doi: 10.1186/s13287-018-0915-0
103. Yang F, Li Y, Zou W, Xu Y, Wang H, Wang W, et al. Adoptive transfer of ifn-Gamma-Induced m-mdscs promotes immune tolerance to allografts through inos pathway. Inflamm Res (2019) 68(7):545–55. doi: 10.1007/s00011-019-01237-9
104. Yang F, Li Y, Wu T, Na N, Zhao Y, Li W, et al. Tnfalpha-induced m-mdscs promote transplant immune tolerance Via nitric oxide. J Mol Med (Berl) (2016) 94(8):911–20. doi: 10.1007/s00109-016-1398-z
105. Kurko J, Vida A, Ocsko T, Tryniszewska B, Rauch TA, Glant TT, et al. Suppression of proteoglycan-induced autoimmune arthritis by myeloid-derived suppressor cells generated in vitro from murine bone marrow. PloS One (2014) 9(11):e111815. doi: 10.1371/journal.pone.0111815
106. Wang H, Ji J, Zhuang Y, Zhou X, Zhao Y, Zhang X. Pma induces the differentiation of monocytes into immunosuppressive mdscs. Clin Exp Immunol (2021) 206(2):216–25. doi: 10.1111/cei.13657
107. Hsu CY, Lin YC, Chang LY, Huang SK, Huang CH, Yang CK, et al. Therapeutic role of inducible nitric oxide synthase expressing myeloid-derived suppressor cells in acetaminophen-induced murine liver failure. Front Immunol (2020) 11:574839. doi: 10.3389/fimmu.2020.574839
108. Czock D, Keller F, Rasche FM, Haussler U. Pharmacokinetics and Pharmacodynamics of Systemically Administered Glucocorticoids. Clin Pharmacokinet (2005) 44(1):61–98. doi: 10.2165/00003088-200544010-00003.
109. Wang Y, Ding Y, Guo N, Wang S. Mdscs: Key criminals of tumor pre-metastatic niche formation. Front Immunol (2019) 10:172. doi: 10.3389/fimmu.2019.00172
110. Yuan K, Zhu Q, Lu Q, Jiang H, Zhu M, Li X, et al. Quercetin alleviates rheumatoid arthritis by inhibiting neutrophil inflammatory activities. J Nutr Biochem (2020) 84:108454. doi: 10.1016/j.jnutbio.2020.108454
111. Javadi F, Ahmadzadeh A, Eghtesadi S, Aryaeian N, Zabihiyeganeh M, Rahimi Foroushani A, et al. The effect of quercetin on inflammatory factors and clinical symptoms in women with rheumatoid arthritis: A double-blind, randomized controlled trial. J Am Coll Nutr (2017) 36(1):9–15. doi: 10.1080/07315724.2016.1140093
112. Comalada M, Camuesco D, Sierra S, Ballester I, Xaus J, Galvez J, et al. In vivo quercitrin anti-inflammatory effect involves release of quercetin, which inhibits inflammation through down-regulation of the nf-kappab pathway. Eur J Immunol (2005) 35(2):584–92. doi: 10.1002/eji.200425778
113. Lalive PH, Neuhaus O, Benkhoucha M, Burger D, Hohlfeld R, Zamvil SS, et al. Glatiramer acetate in the treatment of multiple sclerosis: Emerging concepts regarding its mechanism of action. CNS Drugs (2011) 25(5):401–14. doi: 10.2165/11588120-000000000-00000
114. Shao B, Wei X, Luo M, Yu J, Tong A, Ma X, et al. Inhibition of A20 expression in tumor microenvironment exerts anti-tumor effect through inducing myeloid-derived suppressor cells apoptosis. Sci Rep (2015) 5:16437. doi: 10.1038/srep16437
115. Trillo-Tinoco J, Sierra RA, Mohamed E, Cao Y, de Mingo-Pulido A, Gilvary DL, et al. Ampk alpha-1 intrinsically regulates the function and differentiation of tumor myeloid-derived suppressor cells. Cancer Res (2019) 79(19):5034–47. doi: 10.1158/0008-5472.CAN-19-0880
116. Qin G, Lian J, Huang L, Zhao Q, Liu S, Zhang Z, et al. Metformin blocks myeloid-derived suppressor cell accumulation through ampk-Dach1-Cxcl1 axis. Oncoimmunology (2018) 7(7):e1442167. doi: 10.1080/2162402X.2018.1442167
117. Li L, Wang L, Li J, Fan Z, Yang L, Zhang Z, et al. Metformin-induced reduction of Cd39 and Cd73 blocks myeloid-derived suppressor cell activity in patients with ovarian cancer. Cancer Res (2018) 78(7):1779–91. doi: 10.1158/0008-5472.CAN-17-2460
118. Xu P, Yin K, Tang X, Tian J, Zhang Y, Ma J, et al. Metformin inhibits the function of granulocytic myeloid-derived suppressor cells in tumor-bearing mice. BioMed Pharmacother (2019) 120:109458. doi: 10.1016/j.biopha.2019.109458
119. Bak SP, Alonso A, Turk MJ, Berwin B. Murine ovarian cancer vascular leukocytes require arginase-1 activity for T cell suppression. Mol Immunol (2008) 46(2):258–68. doi: 10.1016/j.molimm.2008.08.266
120. Ye C, Geng Z, Dominguez D, Chen S, Fan J, Qin L, et al. Targeting ornithine decarboxylase by alpha-difluoromethylornithine inhibits tumor growth by impairing myeloid-derived suppressor cells. J Immunol (2016) 196(2):915–23. doi: 10.4049/jimmunol.1500729
121. Steggerda SM, Bennett MK, Chen J, Emberley E, Huang T, Janes JR, et al. Inhibition of arginase by cb-1158 blocks myeloid cell-mediated immune suppression in the tumor microenvironment. J Immunother Cancer (2017) 5(1):101. doi: 10.1186/s40425-017-0308-4
122. Cao Y, Feng Y, Zhang Y, Zhu X, Jin F. L-arginine supplementation inhibits the growth of breast cancer by enhancing innate and adaptive immune responses mediated by suppression of mdscs in vivo. BMC Cancer (2016) 16:343. doi: 10.1186/s12885-016-2376-0
123. Satoh Y, Kotani H, Iida Y, Taniura T, Notsu Y, Harada M. Supplementation of l-arginine boosts the therapeutic efficacy of anticancer chemoimmunotherapy. Cancer Sci (2020) 111(7):2248–58. doi: 10.1111/cas.14490
124. Yin T, Zhao ZB, Guo J, Wang T, Yang JB, Wang C, et al. Aurora a inhibition eliminates myeloid cell-mediated immunosuppression and enhances the efficacy of anti-Pd-L1 therapy in breast cancer. Cancer Res (2019) 79(13):3431–44. doi: 10.1158/0008-5472.CAN-18-3397
125. Hu X, Bardhan K, Paschall AV, Yang D, Waller JL, Park MA, et al. Deregulation of apoptotic factors bcl-xl and bax confers apoptotic resistance to myeloid-derived suppressor cells and contributes to their persistence in cancer. J Biol Chem (2013) 288(26):19103–15. doi: 10.1074/jbc.M112.434530
126. Fjaestad KY, Romer AMA, Goitea V, Johansen AZ, Thorseth ML, Carretta M, et al. Blockade of beta-adrenergic receptors reduces cancer growth and enhances the response to anti-Ctla4 therapy by modulating the tumor microenvironment. Oncogene (2022) 41(9):1364–75. doi: 10.1038/s41388-021-02170-0
127. Mohammadpour H, MacDonald CR, McCarthy PL, Abrams SI, Repasky EA. Beta2-adrenergic receptor signaling regulates metabolic pathways critical to myeloid-derived suppressor cell function within the tme. Cell Rep (2021) 37(4):109883. doi: 10.1016/j.celrep.2021.109883
128. Calvani M, Bruno G, Dal Monte M, Nassini R, Fontani F, Casini A, et al. Beta3 -adrenoceptor as a potential immuno-suppressor agent in melanoma. Br J Pharmacol (2019) 176(14):2509–24. doi: 10.1111/bph.14660
129. Radwan FF, Hossain A, God JM, Leaphart N, Elvington M, Nagarkatti M, et al. Reduction of myeloid-derived suppressor cells and lymphoma growth by a natural triterpenoid. J Cell Biochem (2015) 116(1):102–14. doi: 10.1002/jcb.24946
130. Terlizzi M, Di Crescenzo VG, Perillo G, Galderisi A, Pinto A, Sorrentino R. Pharmacological inhibition of caspase-8 limits lung tumour outgrowth. Br J Pharmacol (2015) 172(15):3917–28. doi: 10.1111/bph.13176
131. Fan Q, Gu D, Liu H, Yang L, Zhang X, Yoder MC, et al. Defective tgf-beta signaling in bone marrow-derived cells prevents hedgehog-induced skin tumors. Cancer Res (2014) 74(2):471–83. doi: 10.1158/0008-5472.CAN-13-2134-T
132. Mu XY, Wang RJ, Yao ZX, Zheng Z, Jiang JT, Tan MY, et al. Rs 504393 inhibits m-mdscs recruiting in immune microenvironment of bladder cancer after gemcitabine treatment. Mol Immunol (2019) 109:140–8. doi: 10.1016/j.molimm.2019.02.014
133. Wang Y, Zhang X, Yang L, Xue J, Hu G. Blockade of Ccl2 enhances immunotherapeutic effect of anti-Pd1 in lung cancer. J Bone Oncol (2018) 11:27–32. doi: 10.1016/j.jbo.2018.01.002
134. ?>Masuda T, Noda M, Kogawa T, Kitagawa D, Hayashi N, Jomori T, et al. Phase I dose-escalation trial to repurpose propagermanium, an oral Ccl2 inhibitor, in patients with breast cancer. Cancer Sci (2020) 111(3):924–31. doi: 10.1111/cas.14306
135. Schlecker E, Stojanovic A, Eisen C, Quack C, Falk CS, Umansky V, et al. Tumor-infiltrating monocytic myeloid-derived suppressor cells mediate Ccr5-dependent recruitment of regulatory T cells favoring tumor growth. J Immunol (2012) 189(12):5602–11. doi: 10.4049/jimmunol.1201018
136. Zhang Y, Lv D, Kim HJ, Kurt RA, Bu W, Li Y, et al. A novel role of hematopoietic Ccl5 in promoting triple-negative mammary tumor progression by regulating generation of myeloid-derived suppressor cells. Cell Res (2013) 23(3):394–408. doi: 10.1038/cr.2012.178
137. Tang Q, Jiang J, Liu J. Ccr5 blockade suppresses melanoma development through inhibition of il-6-Stat3 pathway Via upregulation of Socs3. Inflammation (2015) 38(6):2049–56. doi: 10.1007/s10753-015-0186-1
138. Ban Y, Mai J, Li X, Mitchell-Flack M, Zhang T, Zhang L, et al. Targeting autocrine Ccl5-Ccr5 axis reprograms immunosuppressive myeloid cells and reinvigorates antitumor immunity. Cancer Res (2017) 77(11):2857–68. doi: 10.1158/0008-5472.CAN-16-2913
139. Zhou J, Liu M, Sun H, Feng Y, Xu L, Chan AWH, et al. Hepatoma-intrinsic ccrk inhibition diminishes myeloid-derived suppressor cell immunosuppression and enhances immune-checkpoint blockade efficacy. Gut (2018) 67(5):931–44. doi: 10.1136/gutjnl-2017-314032
140. Eksioglu EA, Chen X, Heider KH, Rueter B, McGraw KL, Basiorka AA, et al. Novel therapeutic approach to improve hematopoiesis in low risk mds by targeting mdscs with the fc-engineered Cd33 antibody bi 836858. Leukemia (2017) 31(10):2172–80. doi: 10.1038/leu.2017.21
141. Jitschin R, Saul D, Braun M, Tohumeken S, Volkl S, Kischel R, et al. Cd33/Cd3-bispecific T-cell engaging (Bite(R)) antibody construct targets monocytic aml myeloid-derived suppressor cells. J Immunother Cancer (2018) 6(1):116. doi: 10.1186/s40425-018-0432-9
142. Cheng P, Chen X, Dalton R, Calescibetta A, So T, Gilvary D, et al. Immunodepletion of mdsc by Amv564, a novel bivalent, bispecific Cd33/Cd3 T cell engager, ex vivo in mds and melanoma. Mol Ther (2022) 30(6):2315–26. doi: 10.1016/j.ymthe.2022.02.005
143. Pan PY, Ma G, Weber KJ, Ozao-Choy J, Wang G, Yin B, et al. Immune stimulatory receptor Cd40 is required for T-cell suppression and T regulatory cell activation mediated by myeloid-derived suppressor cells in cancer. Cancer Res (2010) 70(1):99–108. doi: 10.1158/0008-5472.CAN-09-1882
144. Shen XZ, Okwan-Duodu D, Blackwell WL, Ong FS, Janjulia T, Bernstein EA, et al. Myeloid expression of angiotensin-converting enzyme facilitates myeloid maturation and inhibits the development of myeloid-derived suppressor cells. Lab Invest (2014) 94(5):536–44. doi: 10.1038/labinvest.2014.41
145. Weiss JM, Subleski JJ, Back T, Chen X, Watkins SK, Yagita H, et al. Regulatory T cells and myeloid-derived suppressor cells in the tumor microenvironment undergo fas-dependent cell death during il-2/Alphacd40 therapy. J Immunol (2014) 192(12):5821–9. doi: 10.4049/jimmunol.1400404
146. Buzzelli JN, Pavlic DI, Chalinor HV, O'Connor L, Menheniott TR, Giraud AS, et al. Il-1rt1 signaling antagonizes il-11 induced Stat3 dependent cardiac and antral stomach tumor development through myeloid cell enrichment. Oncotarget (2015) 6(2):679–95. doi: 10.18632/oncotarget.2707
147. Li T, Li X, Zamani A, Wang W, Lee CN, Li M, et al. C-rel is a myeloid checkpoint for cancer immunotherapy. Nat Cancer (2020) 1(5):507–17. doi: 10.1038/s43018-020-0061-3
148. Garcia AJ, Ruscetti M, Arenzana TL, Tran LM, Bianci-Frias D, Sybert E, et al. Pten null prostate epithelium promotes localized myeloid-derived suppressor cell expansion and immune suppression during tumor initiation and progression. Mol Cell Biol (2014) 34(11):2017–28. doi: 10.1128/MCB.00090-14
149. Mok S, Tsoi J, Koya RC, Hu-Lieskovan S, West BL, Bollag G, et al. Inhibition of colony stimulating factor-1 receptor improves antitumor efficacy of braf inhibition. BMC Cancer (2015) 15:356. doi: 10.1186/s12885-015-1377-8
150. Mao Y, Eissler N, Blanc KL, Johnsen JI, Kogner P, Kiessling R. Targeting suppressive myeloid cells potentiates checkpoint inhibitors to control spontaneous neuroblastoma. Clin Cancer Res (2016) 22(15):3849–59. doi: 10.1158/1078-0432.CCR-15-1912
151. Holmgaard RB, Zamarin D, Lesokhin A, Merghoub T, Wolchok JD. Targeting myeloid-derived suppressor cells with colony stimulating factor-1 receptor blockade can reverse immune resistance to immunotherapy in indoleamine 2,3-Dioxygenase-Expressing tumors. EBioMedicine (2016) 6:50–8. doi: 10.1016/j.ebiom.2016.02.024
152. Yu F, Shi Y, Wang J, Li J, Fan D, Ai W. Deficiency of kruppel-like factor Klf4 in mammary tumor cells inhibits tumor growth and pulmonary metastasis and is accompanied by compromised recruitment of myeloid-derived suppressor cells. Int J Cancer (2013) 133(12):2872–83. doi: 10.1002/ijc.28302
153. Highfill SL, Cui Y, Giles AJ, Smith JP, Zhang H, Morse E, et al. Disruption of Cxcr2-mediated mdsc tumor trafficking enhances anti-Pd1 efficacy. Sci Transl Med (2014) 6(237):237ra67. doi: 10.1126/scitranslmed.3007974
154. Wang G, Lu X, Dey P, Deng P, Wu CC, Jiang S, et al. Targeting yap-dependent mdsc infiltration impairs tumor progression. Cancer Discovery (2016) 6(1):80–95. doi: 10.1158/2159-8290.CD-15-0224
155. Dong Y, Fu R, Chen J, Zhang K, Ji M, Wang M, et al. Discovery of benzocyclic sulfone derivatives as potent Cxcr2 antagonists for cancer immunotherapy. J Med Chem (2021) 64(22):16626–40. doi: 10.1021/acs.jmedchem.1c01219
156. Wang D, Sun H, Wei J, Cen B, DuBois RN. Cxcl1 is critical for premetastatic niche formation and metastasis in colorectal cancer. Cancer Res (2017) 77(13):3655–65. doi: 10.1158/0008-5472.CAN-16-3199
157. Shi H, Han X, Sun Y, Shang C, Wei M, Ba X, et al. Chemokine (C-X-C motif) ligand 1 and Cxcl2 produced by tumor promote the generation of monocytic myeloid-derived suppressor cells. Cancer Sci (2018) 109(12):3826–39. doi: 10.1111/cas.13809
158. Greene S, Robbins Y, Mydlarz WK, Huynh AP, Schmitt NC, Friedman J, et al. Inhibition of mdsc trafficking with sx-682, a Cxcr1/2 inhibitor, enhances nk-cell immunotherapy in head and neck cancer models. Clin Cancer Res (2020) 26(6):1420–31. doi: 10.1158/1078-0432.CCR-19-2625
159. Dubeykovskaya Z, Si Y, Chen X, Worthley DL, Renz BW, Urbanska AM, et al. Neural innervation stimulates splenic Tff2 to arrest myeloid cell expansion and cancer. Nat Commun (2016) 7:10517. doi: 10.1038/ncomms10517
160. Hwang HS, Han AR, Lee JY, Park GS, Min WS, Kim HJ. Enhanced anti-leukemic effects through induction of immunomodulating microenvironment by blocking Cxcr4 and pd-L1 in an aml mouse model. Immunol Invest (2019) 48(1):96–105. doi: 10.1080/08820139.2018.1497057
161. Sun R, Luo H, Su J, Di S, Zhou M, Shi B, et al. Olaparib suppresses mdsc recruitment Via Sdf1alpha/Cxcr4 axis to improve the anti-tumor efficacy of car-T cells on breast cancer in mice. Mol Ther (2021) 29(1):60–74. doi: 10.1016/j.ymthe.2020.09.034
162. Bockorny B, Semenisty V, Macarulla T, Borazanci E, Wolpin BM, Stemmer SM, et al. Bl-8040, a Cxcr4 antagonist, in combination with pembrolizumab and chemotherapy for pancreatic cancer: The combat trial. Nat Med (2020) 26(6):878–85. doi: 10.1038/s41591-020-0880-x
163. Ghonim MA, Ibba SV, Tarhuni AF, Errami Y, Luu HH, Dean MJ, et al. Targeting parp-1 with metronomic therapy modulates mdsc suppressive function and enhances anti-Pd-1 immunotherapy in colon cancer. J Immunother Cancer (2021) 9(1):e001643. doi: 10.1136/jitc-2020-001643
164. Travelli C, Consonni FM, Sangaletti S, Storto M, Morlacchi S, Grolla AA, et al. Nicotinamide phosphoribosyltransferase acts as a metabolic gate for mobilization of myeloid-derived suppressor cells. Cancer Res (2019) 79(8):1938–51. doi: 10.1158/0008-5472.CAN-18-1544
165. Wu J, Zhang R, Tang N, Gong Z, Zhou J, Chen Y, et al. Dopamine inhibits the function of gr-1+Cd115+ myeloid-derived suppressor cells through D1-like receptors and enhances anti-tumor immunity. J Leukoc Biol (2015) 97(1):191–200. doi: 10.1189/jlb.5A1113-626RR
166. Hoeppner LH, Wang Y, Sharma A, Javeed N, Van Keulen VP, Wang E, et al. Dopamine D2 receptor agonists inhibit lung cancer progression by reducing angiogenesis and tumor infiltrating myeloid derived suppressor cells. Mol Oncol (2015) 9(1):270–81. doi: 10.1016/j.molonc.2014.08.008
167. Albeituni SH, Ding C, Liu M, Hu X, Luo F, Kloecker G, et al. Yeast-derived particulate beta-glucan treatment subverts the suppression of myeloid-derived suppressor cells (Mdsc) by inducing polymorphonuclear mdsc apoptosis and monocytic mdsc differentiation to apc in cancer. J Immunol (2016) 196(5):2167–80. doi: 10.4049/jimmunol.1501853
168. D'Amico L, Mahajan S, Capietto AH, Yang Z, Zamani A, Ricci B, et al. Dickkopf-related protein 1 (Dkk1) regulates the accumulation and function of myeloid derived suppressor cells in cancer. J Exp Med (2016) 213(5):827–40. doi: 10.1084/jem.20150950
169. Park MS, Yang AY, Lee JE, Kim SK, Roe JS, Park MS, et al. Galnt3 suppresses lung cancer by inhibiting myeloid-derived suppressor cell infiltration and angiogenesis in a tnfr and c-met pathway-dependent manner. Cancer Lett (2021) 521:294–307. doi: 10.1016/j.canlet.2021.08.015
170. Sinha P, Clements VK, Bunt SK, Albelda SM, Ostrand-Rosenberg S. Cross-talk between myeloid-derived suppressor cells and macrophages subverts tumor immunity toward a type 2 response. J Immunol (2007) 179(2):977–83. doi: 10.4049/jimmunol.179.2.977
171. Ghansah T, Vohra N, Kinney K, Weber A, Kodumudi K, Springett G, et al. Dendritic cell immunotherapy combined with gemcitabine chemotherapy enhances survival in a murine model of pancreatic carcinoma. Cancer Immunol Immunother (2013) 62(6):1083–91. doi: 10.1007/s00262-013-1407-9
172. Sasso MS, Lollo G, Pitorre M, Solito S, Pinton L, Valpione S, et al. Low dose gemcitabine-loaded lipid nanocapsules target monocytic myeloid-derived suppressor cells and potentiate cancer immunotherapy. Biomaterials (2016) 96:47–62. doi: 10.1016/j.biomaterials.2016.04.010
173. Peereboom DM, Alban TJ, Grabowski MM, Alvarado AG, Otvos B, Bayik D, et al. Metronomic capecitabine as an immune modulator in glioblastoma patients reduces myeloid-derived suppressor cells. JCI Insight (2019) 4(22):e130748. doi: 10.1172/jci.insight.130748
174. Yuan SJ, Xu YH, Wang C, An HC, Xu HZ, Li K, et al. Doxorubicin-Polyglycerol-Nanodiamond conjugate is a cytostatic agent that evades chemoresistance and reverses cancer-induced immunosuppression in triple-negative breast cancer. J Nanobiotechnol (2019) 17(1):110. doi: 10.1186/s12951-019-0541-8
175. Vincent J, Mignot G, Chalmin F, Ladoire S, Bruchard M, Chevriaux A, et al. 5-fluorouracil selectively kills tumor-associated myeloid-derived suppressor cells resulting in enhanced T cell-dependent antitumor immunity. Cancer Res (2010) 70(8):3052–61. doi: 10.1158/0008-5472.CAN-09-3690
176. Kuroda H, Mabuchi S, Kozasa K, Yokoi E, Matsumoto Y, Komura N, et al. Pm01183 inhibits myeloid-derived suppressor cells in vitro and in vivo. Immunotherapy (2017) 9(10):805–17. doi: 10.2217/imt-2017-0046
177. Huang X, Cui S, Shu Y. Cisplatin selectively downregulated the frequency and immunoinhibitory function of myeloid-derived suppressor cells in a murine B16 melanoma model. Immunol Res (2016) 64(1):160–70. doi: 10.1007/s12026-015-8734-1
178. Jeanbart L, Kourtis IC, van der Vlies AJ, Swartz MA, Hubbell JA. 6-Thioguanine-Loaded polymeric micelles deplete myeloid-derived suppressor cells and enhance the efficacy of T cell immunotherapy in tumor-bearing mice. Cancer Immunol Immunother (2015) 64(8):1033–46. doi: 10.1007/s00262-015-1702-8
179. Terracina KP, Graham LJ, Payne KK, Manjili MH, Baek A, Damle SR, et al. DNA Methyltransferase inhibition increases efficacy of adoptive cellular immunotherapy of murine breast cancer. Cancer Immunol Immunother (2016) 65(9):1061–73. doi: 10.1007/s00262-016-1868-8
180. Chiu DK, Tse AP, Xu IM, Di Cui J, Lai RK, Li LL, et al. Hypoxia inducible factor hif-1 promotes myeloid-derived suppressor cells accumulation through Entpd2/Cd39l1 in hepatocellular carcinoma. Nat Commun (2017) 8(1):517. doi: 10.1038/s41467-017-00530-7
181. Albu DI, Wang Z, Huang KC, Wu J, Twine N, Leacu S, et al. Ep4 antagonism by E7046 diminishes myeloid immunosuppression and synergizes with treg-reducing il-2-Diphtheria toxin fusion protein in restoring anti-tumor immunity. Oncoimmunology (2017) 6(8):e1338239. doi: 10.1080/2162402x.2017.1338239
182. Peng S, Hu P, Xiao YT, Lu W, Guo D, Hu S, et al. Single-cell analysis reveals Ep4 as a target for restoring T-cell infiltration and sensitizing prostate cancer to immunotherapy. Clin Cancer Res (2022) 28(3):552–67. doi: 10.1158/1078-0432.CCR-21-0299
183. Hossain F, Al-Khami AA, Wyczechowska D, Hernandez C, Zheng L, Reiss K, et al. Inhibition of fatty acid oxidation modulates immunosuppressive functions of myeloid-derived suppressor cells and enhances cancer therapies. Cancer Immunol Res (2015) 3(11):1236–47. doi: 10.1158/2326-6066.CIR-15-0036
184. Li J, Srivastava RM, Ettyreddy A, Ferris RL. Cetuximab ameliorates suppressive phenotypes of myeloid antigen presenting cells in head and neck cancer patients. J Immunother Cancer (2015) 3:54. doi: 10.1186/s40425-015-0097-6
185. Yan J, Kong LY, Hu J, Gabrusiewicz K, Dibra D, Xia X, et al. Fgl2 as a multimodality regulator of tumor-mediated immune suppression and therapeutic target in gliomas. J Natl Cancer Inst (2015) 107(8):djv137. doi: 10.1093/jnci/djv137
186. Waight JD, Hu Q, Miller A, Liu S, Abrams SI. Tumor-derived G-csf facilitates neoplastic growth through a granulocytic myeloid-derived suppressor cell-dependent mechanism. PloS One (2011) 6(11):e27690. doi: 10.1371/journal.pone.0027690
187. Wei WC, Lin SY, Lan CW, Huang YC, Lin CY, Hsiao PW, et al. Inhibiting mdsc differentiation from bone marrow with phytochemical polyacetylenes drastically impairs tumor metastasis. Sci Rep (2016) 6:36663. doi: 10.1038/srep36663
188. Nefedova Y, Fishman M, Sherman S, Wang X, Beg AA, Gabrilovich DI. Mechanism of all-trans retinoic acid effect on tumor-associated myeloid-derived suppressor cells. Cancer Res (2007) 67(22):11021–8. doi: 10.1158/0008-5472.Can-07-2593
189. Chen X, Eksioglu EA, Zhou J, Zhang L, Djeu J, Fortenbery N, et al. Induction of myelodysplasia by myeloid-derived suppressor cells. J Clin Invest (2013) 123(11):4595–611. doi: 10.1172/JCI67580
190. Iclozan C, Antonia S, Chiappori A, Chen DT, Gabrilovich D. Therapeutic regulation of myeloid-derived suppressor cells and immune response to cancer vaccine in patients with extensive stage small cell lung cancer. Cancer Immunol Immunother (2013) 62(5):909–18. doi: 10.1007/s00262-013-1396-8
191. Long AH, Highfill SL, Cui Y, Smith JP, Walker AJ, Ramakrishna S, et al. Reduction of mdscs with all-trans retinoic acid improves car therapy efficacy for sarcomas. Cancer Immunol Res (2016) 4(10):869–80. doi: 10.1158/2326-6066.CIR-15-0230
192. Heine A, Flores C, Gevensleben H, Diehl L, Heikenwalder M, Ringelhan M, et al. Targeting myeloid derived suppressor cells with all-trans retinoic acid is highly time-dependent in therapeutic tumor vaccination. Oncoimmunology (2017) 6(8):e1338995. doi: 10.1080/2162402X.2017.1338995
193. Bauer R, Udonta F, Wroblewski M, Ben-Batalla I, Santos IM, Taverna F, et al. Blockade of myeloid-derived suppressor cell expansion with all-trans retinoic acid increases the efficacy of antiangiogenic therapy. Cancer Res (2018) 78(12):3220–32. doi: 10.1158/0008-5472.CAN-17-3415
194. Tobin RP, Jordan KR, Robinson WA, Davis D, Borges VF, Gonzalez R, et al. Targeting myeloid-derived suppressor cells using all-trans retinoic acid in melanoma patients treated with ipilimumab. Int Immunopharmacol (2018) 63:282–91. doi: 10.1016/j.intimp.2018.08.007
195. Oh MH, Sun IH, Zhao L, Leone RD, Sun IM, Xu W, et al. Targeting glutamine metabolism enhances tumor-specific immunity by modulating suppressive myeloid cells. J Clin Invest (2020) 130(7):3865–84. doi: 10.1172/JCI131859
196. Morales JK, Kmieciak M, Knutson KL, Bear HD, Manjili MH. Gm-csf is one of the main breast tumor-derived soluble factors involved in the differentiation of Cd11b-Gr1- bone marrow progenitor cells into myeloid-derived suppressor cells. Breast Cancer Res Treat (2010) 123(1):39–49. doi: 10.1007/s10549-009-0622-8
197. Kapanadze T, Gamrekelashvili J, Ma C, Chan C, Zhao F, Hewitt S, et al. Regulation of accumulation and function of myeloid derived suppressor cells in different murine models of hepatocellular carcinoma. J Hepatol (2013) 59(5):1007–13. doi: 10.1016/j.jhep.2013.06.010
198. Takeuchi S, Baghdadi M, Tsuchikawa T, Wada H, Nakamura T, Abe H, et al. Chemotherapy-derived inflammatory responses accelerate the formation of immunosuppressive myeloid cells in the tissue microenvironment of human pancreatic cancer. Cancer Res (2015) 75(13):2629–40. doi: 10.1158/0008-5472.CAN-14-2921
199. Wang HF, Ning F, Liu ZC, Wu L, Li ZQ, Qi YF, et al. Histone deacetylase inhibitors deplete myeloid-derived suppressor cells induced by 4t1 mammary tumors in vivo and in vitro. Cancer Immunol Immunother (2017) 66(3):355–66. doi: 10.1007/s00262-016-1935-1
200. Orillion A, Hashimoto A, Damayanti N, Shen L, Adelaiye-Ogala R, Arisa S, et al. Entinostat neutralizes myeloid-derived suppressor cells and enhances the antitumor effect of pd-1 inhibition in murine models of lung and renal cell carcinoma. Clin Cancer Res (2017) 23(17):5187–201. doi: 10.1158/1078-0432.CCR-17-0741
201. Xie Z, Ikegami T, Ago Y, Okada N, Tachibana M. Valproic acid attenuates Ccr2-dependent tumor infiltration of monocytic myeloid-derived suppressor cells, limiting tumor progression. Oncoimmunology (2020) 9(1):1734268. doi: 10.1080/2162402X.2020.1734268
202. Vila-Leahey A, Oldford SA, Marignani PA, Wang J, Haidl ID, Marshall JS. Ranitidine modifies myeloid cell populations and inhibits breast tumor development and spread in mice. Oncoimmunology (2016) 5(7):e1151591. doi: 10.1080/2162402X.2016.1151591
203. Grauers Wiktorin H, Nilsson MS, Kiffin R, Sander FE, Lenox B, Rydstrom A, et al. Histamine targets myeloid-derived suppressor cells and improves the anti-tumor efficacy of pd-1/Pd-L1 checkpoint blockade. Cancer Immunol Immunother (2019) 68(2):163–74. doi: 10.1007/s00262-018-2253-6
204. Parker KH, Sinha P, Horn LA, Clements VK, Yang H, Li J, et al. Hmgb1 enhances immune suppression by facilitating the differentiation and suppressive activity of myeloid-derived suppressor cells. Cancer Res (2014) 74(20):5723–33. doi: 10.1158/0008-5472.CAN-13-2347
205. Tian X, Ma J, Wang T, Tian J, Zhang Y, Mao L, et al. Long non-coding rna hoxa transcript antisense rna myeloid-specific 1-Hoxa1 axis downregulates the immunosuppressive activity of myeloid-derived suppressor cells in lung cancer. Front Immunol (2018) 9:473. doi: 10.3389/fimmu.2018.00473
206. Smith C, Chang MY, Parker KH, Beury DW, DuHadaway JB, Flick HE, et al. Ido is a nodal pathogenic driver of lung cancer and metastasis development. Cancer Discov (2012) 2(8):722–35. doi: 10.1158/2159-8290.CD-12-0014
207. Holmgaard RB, Zamarin D, Li Y, Gasmi B, Munn DH, Allison JP, et al. Tumor-expressed ido recruits and activates mdscs in a treg-dependent manner. Cell Rep (2015) 13(2):412–24. doi: 10.1016/j.celrep.2015.08.077
208. Blair AB, Kleponis J, Thomas DL 2nd, Muth ST, Murphy AG, Kim V, et al. Ido1 inhibition potentiates vaccine-induced immunity against pancreatic adenocarcinoma. J Clin Invest (2019) 129(4):1742–55. doi: 10.1172/JCI124077
209. Schafer CC, Wang Y, Hough KP, Sawant A, Grant SC, Thannickal VJ, et al. Indoleamine 2,3-dioxygenase regulates anti-tumor immunity in lung cancer by metabolic reprogramming of immune cells in the tumor microenvironment. Oncotarget (2016) 7(46):75407–24. doi: 10.18632/oncotarget.12249
210. Li A, Barsoumian HB, Schoenhals JE, Cushman TR, Caetano MS, Wang X, et al. Indoleamine 2,3-dioxygenase 1 inhibition targets anti-Pd1-Resistant lung tumors by blocking myeloid-derived suppressor cells. Cancer Lett (2018) 431:54–63. doi: 10.1016/j.canlet.2018.05.005
211. Shi J, Liu C, Luo S, Cao T, Lin B, Zhou M, et al. Sting agonist and ido inhibitor combination therapy inhibits tumor progression in murine models of colorectal cancer. Cell Immunol (2021) 366:104384. doi: 10.1016/j.cellimm.2021.104384
212. Nandre R, Verma V, Gaur P, Patil V, Yang X, Ramlaoui Z, et al. Ido-vaccine ablates immune-suppressive myeloid populations and enhances anti-tumor effects independent of tumor cell ido status. Cancer Immunol Res (2022) 10(5):571–80. doi: 10.1158/2326-6066.CIR-21-0457
213. Medina-Echeverz J, Haile LA, Zhao F, Gamrekelashvili J, Ma C, Metais JY, et al. Ifn-gamma regulates survival and function of tumor-induced Cd11b+ gr-1high myeloid derived suppressor cells by modulating the anti-apoptotic molecule Bcl2a1. Eur J Immunol (2014) 44(8):2457–67. doi: 10.1002/eji.201444497
214. Lu X, Horner JW, Paul E, Shang X, Troncoso P, Deng P, et al. Effective combinatorial immunotherapy for castration-resistant prostate cancer. Nature (2017) 543(7647):728–32. doi: 10.1038/nature21676
215. Tengesdal IW, Menon DR, Osborne DG, Neff CP, Powers NE, Gamboni F, et al. Targeting tumor-derived Nlrp3 reduces melanoma progression by limiting mdscs expansion. Proc Natl Acad Sci USA (2021) 118(10):e2000915118. doi: 10.1073/pnas.2000915118
216. Jiang J, Wang Z, Li Z, Zhang J, Wang C, Xu X, et al. Early exposure of high-dose interleukin-4 to tumor stroma reverses myeloid cell-mediated T-cell suppression. Gene Ther (2010) 17(8):991–9. doi: 10.1038/gt.2010.54
217. Roth F, de la Fuente AC, Vella JL, Zoso A, Inverardi L, Serafini P. Aptamer-mediated blockade of Il4ralpha triggers apoptosis of mdscs and limits tumor progression. Cancer Res (2012) 72(6):1373–83. doi: 10.1158/0008-5472.CAN-11-2772
218. Chen MF, Hsieh CC, Chen WC, Lai CH. Role of interleukin-6 in the radiation response of liver tumors. Int J Radiat Oncol Biol Phys (2012) 84(5):e621–30. doi: 10.1016/j.ijrobp.2012.07.2360
219. Wu CT, Hsieh CC, Lin CC, Chen WC, Hong JH, Chen MF. Significance of il-6 in the transition of hormone-resistant prostate cancer and the induction of myeloid-derived suppressor cells. J Mol Med (Berl) (2012) 90(11):1343–55. doi: 10.1007/s00109-012-0916-x
220. Wu CT, Chen MF, Chen WC, Hsieh CC. The role of il-6 in the radiation response of prostate cancer. Radiat Oncol (2013) 8:159. doi: 10.1186/1748-717X-8-159
221. Zhang T, Guo W, Yang Y, Liu W, Guo L, Gu Y, et al. Loss of shp-2 activity in Cd4+ T cells promotes melanoma progression and metastasis. Sci Rep (2013) 3:2845. doi: 10.1038/srep02845
222. Caetano MS, Zhang H, Cumpian AM, Gong L, Unver N, Ostrin EJ, et al. Il6 blockade reprograms the lung tumor microenvironment to limit the development and progression of K-Ras-Mutant lung cancer. Cancer Res (2016) 76(11):3189–99. doi: 10.1158/0008-5472.CAN-15-2840
223. Lee BR, Kwon BE, Hong EH, Shim A, Song JH, Kim HM, et al. Interleukin-10 attenuates tumour growth by inhibiting interleukin-6/Signal transducer and activator of transcription 3 signalling in myeloid-derived suppressor cells. Cancer Lett (2016) 381(1):156–64. doi: 10.1016/j.canlet.2016.07.012
224. Dominguez C, McCampbell KK, David JM, Palena C. Neutralization of il-8 decreases tumor pmn-mdscs and reduces mesenchymalization of claudin-low triple-negative breast cancer. JCI Insight (2017) 2(21):e94296. doi: 10.1172/jci.insight.94296
225. Bilusic M, Heery CR, Collins JM, Donahue RN, Palena C, Madan RA, et al. Phase I trial of humax-Il8 (Bms-986253), an anti-Il-8 monoclonal antibody, in patients with metastatic or unresectable solid tumors. J Immunother Cancer (2019) 7(1):240. doi: 10.1186/s40425-019-0706-x
226. Hart KM, Byrne KT, Molloy MJ, Usherwood EM, Berwin B. Il-10 immunomodulation of myeloid cells regulates a murine model of ovarian cancer. Front Immunol (2011) 2:29. doi: 10.3389/fimmu.2011.00029
227. Martinson HA, Jindal S, Durand-Rougely C, Borges VF, Schedin P. Wound healing-like immune program facilitates postpartum mammary gland involution and tumor progression. Int J Cancer (2015) 136(8):1803–13. doi: 10.1002/ijc.29181
228. Medina-Echeverz J, Fioravanti J, Zabala M, Ardaiz N, Prieto J, Berraondo P. Successful colon cancer eradication after chemoimmunotherapy is associated with profound phenotypic change of intratumoral myeloid cells. J Immunol (2011) 186(2):807–15. doi: 10.4049/jimmunol.1001483
229. Steding CE, Wu ST, Zhang Y, Jeng MH, Elzey BD, Kao C. The role of interleukin-12 on modulating myeloid-derived suppressor cells, increasing overall survival and reducing metastasis. Immunology (2011) 133(2):221–38. doi: 10.1111/j.1365-2567.2011.03429.x
230. Hall B, Nakashima H, Sun ZJ, Sato Y, Bian Y, Husain SR, et al. Targeting of interleukin-13 receptor Alpha2 for treatment of head and neck squamous cell carcinoma induced by conditional deletion of tgf-beta and pten signaling. J Transl Med (2013) 11:45. doi: 10.1186/1479-5876-11-45
231. Lim HX, Hong HJ, Cho D, Kim TS. Il-18 enhances immunosuppressive responses by promoting differentiation into monocytic myeloid-derived suppressor cells. J Immunol (2014) 193(11):5453–60. doi: 10.4049/jimmunol.1401282
232. Guan Y, Zhang R, Peng Z, Dong D, Wei G, Wang Y. Inhibition of il-18-Mediated myeloid derived suppressor cell accumulation enhances anti-Pd1 efficacy against osteosarcoma cancer. J Bone Oncol (2017) 9:59–64. doi: 10.1016/j.jbo.2017.10.002
233. Nakamura K, Kassem S, Cleynen A, Chretien ML, Guillerey C, Putz EM, et al. Dysregulated il-18 is a key driver of immunosuppression and a possible therapeutic target in the multiple myeloma microenvironment. Cancer Cell (2018) 33(4):634–48 e5. doi: 10.1016/j.ccell.2018.02.007
234. Lim HX, Choi S, Cho D, Kim TS. Il-33 inhibits the differentiation and immunosuppressive activity of granulocytic myeloid-derived suppressor cells in tumor-bearing mice. Immunol Cell Biol (2017) 95(1):99–107. doi: 10.1038/icb.2016.72
235. Capuano G, Rigamonti N, Grioni M, Freschi M, Bellone M. Modulators of arginine metabolism support cancer immunosurveillance. BMC Immunol (2009) 10:1. doi: 10.1186/1471-2172-10-1
236. Jayaraman P, Parikh F, Lopez-Rivera E, Hailemichael Y, Clark A, Ma G, et al. Tumor-expressed inducible nitric oxide synthase controls induction of functional myeloid-derived suppressor cells through modulation of vascular endothelial growth factor release. J Immunol (2012) 188(11):5365–76. doi: 10.4049/jimmunol.1103553
237. Nam S, Kang K, Cha JS, Kim JW, Lee HG, Kim Y, et al. Interferon regulatory factor 4 (Irf4) controls myeloid-derived suppressor cell (Mdsc) differentiation and function. J Leukoc Biol (2016) 100(6):1273–84. doi: 10.1189/jlb.1A0215-068RR
238. Yang Q, Xie H, Li X, Feng Y, Xie S, Qu J, et al. Interferon regulatory factor 4 regulates the development of polymorphonuclear myeloid-derived suppressor cells through the transcription of c-myc in cancer. Front Immunol (2021) 12:627072. doi: 10.3389/fimmu.2021.627072
239. Parveen S, Siddharth S, Cheung LS, Kumar A, Shen J, Murphy JR, et al. Therapeutic targeting with dabil-4 depletes myeloid suppressor cells in 4t1 triple-negative breast cancer model. Mol Oncol (2021) 15(5):1330–44. doi: 10.1002/1878-0261.12938
240. Sierra RA, Trillo-Tinoco J, Mohamed E, Yu L, Achyut BR, Arbab A, et al. Anti-jagged immunotherapy inhibits mdscs and overcomes tumor-induced tolerance. Cancer Res (2017) 77(20):5628–38. doi: 10.1158/0008-5472.CAN-17-0357
241. Cao M, Xu Y, Youn JI, Cabrera R, Zhang X, Gabrilovich D, et al. Kinase inhibitor sorafenib modulates immunosuppressive cell populations in a murine liver cancer model. Lab Invest (2011) 91(4):598–608. doi: 10.1038/labinvest.2010.205
242. Husain Z, Huang Y, Seth P, Sukhatme VP. Tumor-derived lactate modifies antitumor immune response: Effect on myeloid-derived suppressor cells and nk cells. J Immunol (2013) 191(3):1486–95. doi: 10.4049/jimmunol.1202702
243. Tavazoie MF, Pollack I, Tanqueco R, Ostendorf BN, Reis BS, Gonsalves FC, et al. Lxr/Apoe activation restricts innate immune suppression in cancer. Cell (2018) 172(4):825–40 e18. doi: 10.1016/j.cell.2017.12.026
244. Liang H, Shen X. Lxr activation radiosensitizes non-small cell lung cancer by restricting myeloid-derived suppressor cells. Biochem Biophys Res Commun (2020) 528(2):330–5. doi: 10.1016/j.bbrc.2020.04.137
245. Allegrezza MJ, Rutkowski MR, Stephen TL, Svoronos N, Perales-Puchalt A, Nguyen JM, et al. Trametinib drives T-Cell-Dependent control of kras-mutated tumors by inhibiting pathological myelopoiesis. Cancer Res (2016) 76(21):6253–65. doi: 10.1158/0008-5472.CAN-16-1308
246. Baumann D, Hagele T, Mochayedi J, Drebant J, Vent C, Blobner S, et al. Proimmunogenic impact of mek inhibition synergizes with agonist anti-Cd40 immunostimulatory antibodies in tumor therapy. Nat Commun (2020) 11(1):2176. doi: 10.1038/s41467-020-15979-2
247. Schilling B, Sucker A, Griewank K, Zhao F, Weide B, Gorgens A, et al. Vemurafenib reverses immunosuppression by myeloid derived suppressor cells. Int J Cancer (2013) 133(7):1653–63. doi: 10.1002/ijc.28168
248. Simpson KD, Templeton DJ, Cross JV. Macrophage migration inhibitory factor promotes tumor growth and metastasis by inducing myeloid-derived suppressor cells in the tumor microenvironment. J Immunol (2012) 189(12):5533–40. doi: 10.4049/jimmunol.1201161
249. Zhao T, Du H, Ding X, Walls K, Yan C. Activation of mtor pathway in myeloid-derived suppressor cells stimulates cancer cell proliferation and metastasis in lal(-/-) mice. Oncogene (2015) 34(15):1938–48. doi: 10.1038/onc.2014.143
250. Wu T, Zhao Y, Wang H, Li Y, Shao L, Wang R, et al. Mtor masters monocytic myeloid-derived suppressor cells in mice with allografts or tumors. Sci Rep (2016) 6:20250. doi: 10.1038/srep20250
251. Pi R, Yang Y, Hu X, Li H, Shi H, Liu Y, et al. Dual Mtorc1/2 inhibitor Azd2014 diminishes myeloid-derived suppressor cells accumulation in ovarian cancer and delays tumor growth. Cancer Lett (2021) 523:72–81. doi: 10.1016/j.canlet.2021.09.017
252. Trikha P, Plews RL, Stiff A, Gautam S, Hsu V, Abood D, et al. Targeting myeloid-derived suppressor cells using a novel adenosine monophosphate-activated protein kinase (Ampk) activator. Oncoimmunology (2016) 5(9):e1214787. doi: 10.1080/2162402X.2016.1214787
253. Hong EH, Chang SY, Lee BR, Kim YS, Lee JM, Kang CY, et al. Blockade of Myd88 signaling induces antitumor effects by skewing the immunosuppressive function of myeloid-derived suppressor cells. Int J Cancer (2013) 132(12):2839–48. doi: 10.1002/ijc.27974
254. Wang L, Hu D, Xie B, Xie L. Blockade of Myd88 signaling by a novel Myd88 inhibitor prevents colitis-associated colorectal cancer development by impairing myeloid-derived suppressor cells. Invest New Drugs (2022) 40(3):506–18. doi: 10.1007/s10637-022-01218-6
255. Erin N, Korcum AF, Tanriover G, Kale S, Demir N, Koksoy S. Activation of neuroimmune pathways increases therapeutic effects of radiotherapy on poorly differentiated breast carcinoma. Brain Behav Immun (2015) 48:174–85. doi: 10.1016/j.bbi.2015.02.024
256. Kobayashi M, Chung JS, Beg M, Arriaga Y, Verma U, Courtney K, et al. Blocking monocytic myeloid-derived suppressor cell function via anti-Dc-Hil/Gpnmb antibody restores the in vitro integrity of T cells from cancer patients. Clin Cancer Res (2019) 25(2):828–38. doi: 10.1158/1078-0432.CCR-18-0330
257. Zonneville J, Colligan S, Grant S, Miller A, Wallace P, Abrams SI, et al. Blockade of P38 kinase impedes the mobilization of protumorigenic myeloid populations to impact breast cancer metastasis. Int J Cancer (2020) 147(8):2279–92. doi: 10.1002/ijc.33050
258. Alicea-Torres K, Sanseviero E, Gui J, Chen J, Veglia F, Yu Q, et al. Immune suppressive activity of myeloid-derived suppressor cells in cancer requires inactivation of the type I interferon pathway. Nat Commun (2021) 12(1):1717. doi: 10.1038/s41467-021-22033-2
259. Duraiswamy J, Freeman GJ, Coukos G. Therapeutic pd-1 pathway blockade augments with other modalities of immunotherapy T-cell function to prevent immune decline in ovarian cancer. Cancer Res (2013) 73(23):6900–12. doi: 10.1158/0008-5472.CAN-13-1550
260. John LB, Devaud C, Duong CP, Yong CS, Beavis PA, Haynes NM, et al. Anti-Pd-1 antibody therapy potently enhances the eradication of established tumors by gene-modified T cells. Clin Cancer Res (2013) 19(20):5636–46. doi: 10.1158/1078-0432.CCR-13-0458
261. Noman MZ, Desantis G, Janji B, Hasmim M, Karray S, Dessen P, et al. Pd-L1 is a novel direct target of hif-1alpha, and its blockade under hypoxia enhanced mdsc-mediated T cell activation. J Exp Med (2014) 211(5):781–90. doi: 10.1084/jem.20131916
262. Yu GT, Bu LL, Huang CF, Zhang WF, Chen WJ, Gutkind JS, et al. Pd-1 blockade attenuates immunosuppressive myeloid cells due to inhibition of Cd47/Sirpalpha axis in hpv negative head and neck squamous cell carcinoma. Oncotarget (2015) 6(39):42067–80. doi: 10.18632/oncotarget.5955
263. Davis RJ, Moore EC, Clavijo PE, Friedman J, Cash H, Chen Z, et al. Anti-Pd-L1 efficacy can be enhanced by inhibition of myeloid-derived suppressor cells with a selective inhibitor of Pi3kdelta/Gamma. Cancer Res (2017) 77(10):2607–19. doi: 10.1158/0008-5472.CAN-16-2534
264. Teo ZL, Versaci S, Dushyanthen S, Caramia F, Savas P, Mintoff CP, et al. Combined Cdk4/6 and Pi3kalpha inhibition is synergistic and immunogenic in triple-negative breast cancer. Cancer Res (2017) 77(22):6340–52. doi: 10.1158/0008-5472.CAN-17-2210
265. Lin H, Wu Y, Chen J, Huang S, Wang Y. (-)-4-O-(4-O-Beta-D-Glucopyranosylcaffeoyl) quinic acid inhibits the function of myeloid-derived suppressor cells to enhance the efficacy of anti-Pd1 against colon cancer. Pharm Res (2018) 35(9):183. doi: 10.1007/s11095-018-2459-5
266. Zhang M, Wang L, Liu W, Wang T, De Sanctis F, Zhu L, et al. Targeting inhibition of accumulation and function of myeloid-derived suppressor cells by artemisinin via Pi3k/Akt, mtor, and mapk pathways enhances anti-Pd-L1 immunotherapy in melanoma and liver tumors. J Immunol Res (2022) 2022:2253436. doi: 10.1155/2022/2253436
267. Wu L, Yan C, Czader M, Foreman O, Blum JS, Kapur R, et al. Inhibition of ppargamma in myeloid-lineage cells induces systemic inflammation, immunosuppression, and tumorigenesis. Blood (2012) 119(1):115–26. doi: 10.1182/blood-2011-06-363093
268. Zhao T, Du H, Blum JS, Yan C. Critical role of ppargamma in myeloid-derived suppressor cell-stimulated cancer cell proliferation and metastasis. Oncotarget (2016) 7(2):1529–43. doi: 10.18632/oncotarget.6414
269. Lu T, Ramakrishnan R, Altiok S, Youn JI, Cheng P, Celis E, et al. Tumor-infiltrating myeloid cells induce tumor cell resistance to cytotoxic T cells in mice. J Clin Invest (2011) 121(10):4015–29. doi: 10.1172/JCI45862
270. Yin Y, Huang X, Lynn KD, Thorpe PE. Phosphatidylserine-targeting antibody induces M1 macrophage polarization and promotes myeloid-derived suppressor cell differentiation. Cancer Immunol Res (2013) 1(4):256–68. doi: 10.1158/2326-6066.CIR-13-0073
271. Chalasani P, Marron M, Roe D, Clarke K, Iannone M, Livingston RB, et al. A phase I clinical trial of bavituximab and paclitaxel in patients with Her2 negative metastatic breast cancer. Cancer Med (2015) 4(7):1051–9. doi: 10.1002/cam4.447
272. Meyer C, Sevko A, Ramacher M, Bazhin AV, Falk CS, Osen W, et al. Chronic inflammation promotes myeloid-derived suppressor cell activation blocking antitumor immunity in transgenic mouse melanoma model. Proc Natl Acad Sci U.S.A. (2011) 108(41):17111–6. doi: 10.1073/pnas.1108121108
273. Umansky V, Sevko A. Overcoming immunosuppression in the melanoma microenvironment induced by chronic inflammation. Cancer Immunol Immunother (2012) 61(2):275–82. doi: 10.1007/s00262-011-1164-6
274. Weed DT, Vella JL, Reis IM, de la Fuente AC, Gomez C, Sargi Z, et al. Tadalafil reduces myeloid-derived suppressor cells and regulatory T cells and promotes tumor immunity in patients with head and neck squamous cell carcinoma. Clin Cancer Res (2015) 21(1):39–48. doi: 10.1158/1078-0432.CCR-14-1711
275. Califano JA, Khan Z, Noonan KA, Rudraraju L, Zhang Z, Wang H, et al. Tadalafil augments tumor specific immunity in patients with head and neck squamous cell carcinoma. Clin Cancer Res (2015) 21(1):30–8. doi: 10.1158/1078-0432.CCR-14-1716
276. Lin S, Wang J, Wang L, Wen J, Guo Y, Qiao W, et al. Phosphodiesterase-5 inhibition suppresses colonic inflammation-induced tumorigenesis Via blocking the recruitment of mdsc. Am J Cancer Res (2017) 7(1):41–52.
277. Hassel JC, Jiang H, Bender C, Winkler J, Sevko A, Shevchenko I, et al. Tadalafil has biologic activity in human melanoma. results of a pilot trial with tadalafil in patients with metastatic melanoma (Tame). Oncoimmunology (2017) 6(9):e1326440. doi: 10.1080/2162402X.2017.1326440
278. Sinha P, Clements VK, Fulton AM, Ostrand-Rosenberg S. Prostaglandin E2 promotes tumor progression by inducing myeloid-derived suppressor cells. Cancer Res (2007) 67(9):4507–13. doi: 10.1158/0008-5472.Can-06-4174
279. Zhang Y, Liu Q, Zhang M, Yu Y, Liu X, Cao X. Fas signal promotes lung cancer growth by recruiting myeloid-derived suppressor cells Via cancer cell-derived Pge2. J Immunol (2009) 182(6):3801–8. doi: 10.4049/jimmunol.0801548
280. Obermajer N, Muthuswamy R, Odunsi K, Edwards RP, Kalinski P. Pge(2)-induced Cxcl12 production and Cxcr4 expression controls the accumulation of human mdscs in ovarian cancer environment. Cancer Res (2011) 71(24):7463–70. doi: 10.1158/0008-5472.Can-11-2449
281. Kidiyoor A, Schettini J, Besmer DM, Rego SL, Nath S, Curry JM, et al. Pancreatic cancer cells isolated from Muc1-null tumors favor the generation of a mature less suppressive mdsc population. Front Immunol (2014) 5:67. doi: 10.3389/fimmu.2014.00067
282. Mao Y, Sarhan D, Steven A, Seliger B, Kiessling R, Lundqvist A. Inhibition of tumor-derived prostaglandin-E2 blocks the induction of myeloid-derived suppressor cells and recovers natural killer cell activity. Clin Cancer Res (2014) 20(15):4096–106. doi: 10.1158/1078-0432.Ccr-14-0635
283. Blidner AG, Salatino M, Mascanfroni ID, Diament MJ, Bal de Kier Joffe E, Jasnis MA, et al. Differential response of myeloid-derived suppressor cells to the nonsteroidal anti-inflammatory agent indomethacin in tumor-associated and tumor-free microenvironments. J Immunol (2015) 194(7):3452–62. doi: 10.4049/jimmunol.1401144
284. Guo Y, Xiong J, Wang J, Wen J, Zhi F. Inhibition of rac family protein impairs colitis and colitis-associated cancer in mice. Am J Cancer Res (2018) 8(1):70–80.
285. Strauss L, Sangaletti S, Consonni FM, Szebeni G, Morlacchi S, Totaro MG, et al. Rorc1 regulates tumor-promoting "Emergency" granulo-monocytopoiesis. Cancer Cell (2015) 28(2):253–69. doi: 10.1016/j.ccell.2015.07.006
286. Nagaraj S, Youn JI, Weber H, Iclozan C, Lu L, Cotter MJ, et al. Anti-inflammatory triterpenoid blocks immune suppressive function of mdscs and improves immune response in cancer. Clin Cancer Res (2010) 16(6):1812–23. doi: 10.1158/1078-0432.CCR-09-3272
287. Alizadeh D, Trad M, Hanke NT, Larmonier CB, Janikashvili N, Bonnotte B, et al. Doxorubicin eliminates myeloid-derived suppressor cells and enhances the efficacy of adoptive T-cell transfer in breast cancer. Cancer Res (2014) 74(1):104–18. doi: 10.1158/0008-5472.CAN-13-1545
288. Ozao-Choy J, Ma G, Kao J, Wang GX, Meseck M, Sung M, et al. The novel role of tyrosine kinase inhibitor in the reversal of immune suppression and modulation of tumor microenvironment for immune-based cancer therapies. Cancer Res (2009) 69(6):2514–22. doi: 10.1158/0008-5472.CAN-08-4709
289. Holtzhausen A, Harris W, Ubil E, Hunter DM, Zhao J, Zhang Y, et al. Tam Family receptor kinase inhibition reverses mdsc-mediated suppression and augments anti-Pd-1 therapy in melanoma. Cancer Immunol Res (2019) 7(10):1672–86. doi: 10.1158/2326-6066.CIR-19-0008
290. Apolo AB, Nadal R, Tomita Y, Davarpanah NN, Cordes LM, Steinberg SM, et al. Cabozantinib in patients with platinum-refractory metastatic urothelial carcinoma: An open-label, single-centre, phase 2 trial. Lancet Oncol (2020) 21(8):1099–109. doi: 10.1016/S1470-2045(20)30202-3
291. Aggen DH, Ager CR, Obradovic AZ, Chowdhury N, Ghasemzadeh A, Mao W, et al. Blocking Il1 beta promotes tumor regression and remodeling of the myeloid compartment in a renal cell carcinoma model: Multidimensional analyses. Clin Cancer Res (2021) 27(2):608–21. doi: 10.1158/1078-0432.CCR-20-1610
292. Lu M, Zhang X, Gao X, Sun S, Wei X, Hu X, et al. Lenvatinib enhances T cell immunity and the efficacy of adoptive chimeric antigen receptor-modified T cells by decreasing myeloid-derived suppressor cells in cancer. Pharmacol Res (2021) 174:105829. doi: 10.1016/j.phrs.2021.105829
293. Draghiciu O, Nijman HW, Hoogeboom BN, Meijerhof T, Daemen T. Sunitinib depletes myeloid-derived suppressor cells and synergizes with a cancer vaccine to enhance antigen-specific immune responses and tumor eradication. Oncoimmunology (2015) 4(3):e989764. doi: 10.4161/2162402X.2014.989764
294. Heine A, Schilling J, Grunwald B, Kruger A, Gevensleben H, Held SA, et al. The induction of human myeloid derived suppressor cells through hepatic stellate cells is dose-dependently inhibited by the tyrosine kinase inhibitors nilotinib, dasatinib and sorafenib, but not sunitinib. Cancer Immunol Immunother (2016) 65(3):273–82. doi: 10.1007/s00262-015-1790-5
295. Guislain A, Gadiot J, Kaiser A, Jordanova ES, Broeks A, Sanders J, et al. Sunitinib pretreatment improves tumor-infiltrating lymphocyte expansion by reduction in intratumoral content of myeloid-derived suppressor cells in human renal cell carcinoma. Cancer Immunol Immunother (2015) 64(10):1241–50. doi: 10.1007/s00262-015-1735-z
296. Motoshima T, Komohara Y, Horlad H, Takeuchi A, Maeda Y, Tanoue K, et al. Sorafenib enhances the antitumor effects of anti-Ctla-4 antibody in a murine cancer model by inhibiting myeloid-derived suppressor cells. Oncol Rep (2015) 33(6):2947–53. doi: 10.3892/or.2015.3893
297. Stiff A, Trikha P, Wesolowski R, Kendra K, Hsu V, Uppati S, et al. Myeloid-derived suppressor cells express bruton's tyrosine kinase and can be depleted in tumor-bearing hosts by ibrutinib treatment. Cancer Res (2016) 76(8):2125–36. doi: 10.1158/0008-5472.CAN-15-1490
298. Zhang T, Harrison MR, O'Donnell PH, Alva AS, Hahn NM, Appleman LJ, et al. A randomized phase 2 trial of pembrolizumab versus pembrolizumab and acalabrutinib in patients with platinum-resistant metastatic urothelial cancer. Cancer (2020) 126(20):4485–97. doi: 10.1002/cncr.33067
299. Overman M, Javle M, Davis RE, Vats P, Kumar-Sinha C, Xiao L, et al. Randomized phase ii study of the bruton tyrosine kinase inhibitor acalabrutinib, alone or with pembrolizumab in patients with advanced pancreatic cancer. J Immunother Cancer (2020) 8(1):e000587. doi: 10.1136/jitc-2020-000587
300. Varikuti S, Singh B, Volpedo G, Ahirwar DK, Jha BK, Saljoughian N, et al. Ibrutinib treatment inhibits breast cancer progression and metastasis by inducing conversion of myeloid-derived suppressor cells to dendritic cells. Br J Cancer (2020) 122(7):1005–13. doi: 10.1038/s41416-020-0743-8
301. Mao L, Deng WW, Yu GT, Bu LL, Liu JF, Ma SR, et al. Inhibition of src family kinases reduces myeloid-derived suppressor cells in head and neck cancer. Int J Cancer (2017) 140(5):1173–85. doi: 10.1002/ijc.30493
302. Cheng P, Corzo CA, Luetteke N, Yu B, Nagaraj S, Bui MM, et al. Inhibition of dendritic cell differentiation and accumulation of myeloid-derived suppressor cells in cancer is regulated by S100a9 protein. J Exp Med (2008) 205(10):2235–49. doi: 10.1084/jem.20080132
303. Sinha P, Okoro C, Foell D, Freeze HH, Ostrand-Rosenberg S, Srikrishna G. Proinflammatory S100 proteins regulate the accumulation of myeloid-derived suppressor cells. J Immunol (2008) 181(7):4666–75. doi: 10.4049/jimmunol.181.7.4666
304. Ichikawa M, Williams R, Wang L, Vogl T, Srikrishna G. S100a8/A9 activate key genes and pathways in colon tumor progression. Mol Cancer Res (2011) 9(2):133–48. doi: 10.1158/1541-7786.MCR-10-0394
305. Wang L, Chang EW, Wong SC, Ong SM, Chong DQ, Ling KL. Increased myeloid-derived suppressor cells in gastric cancer correlate with cancer stage and plasma S100a8/A9 proinflammatory proteins. J Immunol (2013) 190(2):794–804. doi: 10.4049/jimmunol.1202088
306. Ortiz ML, Lu L, Ramachandran I, Gabrilovich DI. Myeloid-derived suppressor cells in the development of lung cancer. Cancer Immunol Res (2014) 2(1):50–8. doi: 10.1158/2326-6066.CIR-13-0129
307. Shen L, Sundstedt A, Ciesielski M, Miles KM, Celander M, Adelaiye R, et al. Tasquinimod modulates suppressive myeloid cells and enhances cancer immunotherapies in murine models. Cancer Immunol Res (2015) 3(2):136–48. doi: 10.1158/2326-6066.CIR-14-0036
308. Deguchi A, Tomita T, Ohto U, Takemura K, Kitao A, Akashi-Takamura S, et al. Eritoran inhibits S100a8-mediated Tlr4/Md-2 activation and tumor growth by changing the immune microenvironment. Oncogene (2016) 35(11):1445–56. doi: 10.1038/onc.2015.211
309. Li H, Han Y, Guo Q, Zhang M, Cao X. Cancer-expanded myeloid-derived suppressor cells induce anergy of nk cells through membrane-bound tgf-beta 1. J Immunol (2009) 182(1):240–9. doi: 10.4049/jimmunol.182.1.240
310. Korbelik M, Banath J, Zhang W, Saw KM, Szulc ZM, Bielawska A, et al. Interaction of acid ceramidase inhibitor Lcl521 with tumor response to photodynamic therapy and photodynamic therapy-generated vaccine. Int J Cancer (2016) 139(6):1372–8. doi: 10.1002/ijc.30171
311. Liu F, Li X, Lu C, Bai A, Bielawski J, Bielawska A, et al. Ceramide activates lysosomal cathepsin b and cathepsin d to attenuate autophagy and induces er stress to suppress myeloid-derived suppressor cells. Oncotarget (2016) 7(51):83907–25. doi: 10.18632/oncotarget.13438
312. Plebanek MP, Bhaumik D, Bryce PJ, Thaxton CS. Scavenger receptor type B1 and lipoprotein nanoparticle inhibit myeloid-derived suppressor cells. Mol Cancer Ther (2018) 17(3):686–97. doi: 10.1158/1535-7163.MCT-17-0981
313. Younis RH, Han KL, Webb TJ. Human head and neck squamous cell carcinoma-associated semaphorin 4d induces expansion of myeloid-derived suppressor cells. J Immunol (2016) 196(3):1419–29. doi: 10.4049/jimmunol.1501293
314. Clavijo PE, Friedman J, Robbins Y, Moore EC, Smith E, Zauderer M, et al. Semaphorin4d inhibition improves response to immune-checkpoint blockade Via attenuation of mdsc recruitment and function. Cancer Immunol Res (2019) 7(2):282–91. doi: 10.1158/2326-6066.CIR-18-0156
315. Liu G, Bi Y, Shen B, Yang H, Zhang Y, Wang X, et al. Sirt1 limits the function and fate of myeloid-derived suppressor cells in tumors by orchestrating hif-1alpha-Dependent glycolysis. Cancer Res (2014) 74(3):727–37. doi: 10.1158/0008-5472.CAN-13-2584
316. Xin H, Zhang C, Herrmann A, Du Y, Figlin R, Yu H. Sunitinib inhibition of Stat3 induces renal cell carcinoma tumor cell apoptosis and reduces immunosuppressive cells. Cancer Res (2009) 69(6):2506–13. doi: 10.1158/0008-5472.CAN-08-4323
317. Poschke I, Mougiakakos D, Hansson J, Masucci GV, Kiessling R. Immature immunosuppressive Cd14+Hla-Dr-/Low cells in melanoma patients are Stat3hi and overexpress Cd80, Cd83, and dc-sign. Cancer Res (2010) 70(11):4335–45. doi: 10.1158/0008-5472.CAN-09-3767
318. Tu SP, Jin H, Shi JD, Zhu LM, Suo Y, Lu G, et al. Curcumin induces the differentiation of myeloid-derived suppressor cells and inhibits their interaction with cancer cells and related tumor growth. Cancer Prev Res (Phila) (2012) 5(2):205–15. doi: 10.1158/1940-6207.CAPR-11-0247
319. Vasquez-Dunddel D, Pan F, Zeng Q, Gorbounov M, Albesiano E, Fu J, et al. Stat3 regulates arginase-I in myeloid-derived suppressor cells from cancer patients. J Clin Invest (2013) 123(4):1580–9. doi: 10.1172/JCI60083
320. Panni RZ, Sanford DE, Belt BA, Mitchem JB, Worley LA, Goetz BD, et al. Tumor-induced Stat3 activation in monocytic myeloid-derived suppressor cells enhances stemness and mesenchymal properties in human pancreatic cancer. Cancer Immunol Immunother (2014) 63(5):513–28. doi: 10.1007/s00262-014-1527-x
321. Yu J, Wang Y, Yan F, Zhang P, Li H, Zhao H, et al. Noncanonical nf-kappab activation mediates Stat3-stimulated ido upregulation in myeloid-derived suppressor cells in breast cancer. J Immunol (2014) 193(5):2574–86. doi: 10.4049/jimmunol.1400833
322. Yang F, Hu M, Lei Q, Xia Y, Zhu Y, Song X, et al. Nifuroxazide induces apoptosis and impairs pulmonary metastasis in breast cancer model. Cell Death Dis (2015) 6:e1701. doi: 10.1038/cddis.2015.63
323. Zhu Y, Ye T, Yu X, Lei Q, Yang F, Xia Y, et al. Nifuroxazide exerts potent anti-tumor and anti-metastasis activity in melanoma. Sci Rep (2016) 6:20253. doi: 10.1038/srep20253
324. Ye TH, Yang FF, Zhu YX, Li YL, Lei Q, Song XJ, et al. Inhibition of Stat3 signaling pathway by nifuroxazide improves antitumor immunity and impairs colorectal carcinoma metastasis. Cell Death Dis (2017) 8(1):e2534. doi: 10.1038/cddis.2016.452
325. Al-Khami AA, Zheng L, Del Valle L, Hossain F, Wyczechowska D, Zabaleta J, et al. Exogenous lipid uptake induces metabolic and functional reprogramming of tumor-associated myeloid-derived suppressor cells. Oncoimmunology (2017) 6(10):e1344804. doi: 10.1080/2162402X.2017.1344804
326. Bitsch R, Kurzay A, Ozbay Kurt F, de la Torre C, Lasser S, Lepper A, et al. Stat3 inhibitor napabucasin abrogates mdsc immunosuppressive capacity and prolongs survival of melanoma-bearing mice. J Immunother Cancer (2022) 10(3):e004384. doi: 10.1136/jitc-2021-004384
327. Liu D, You M, Xu Y, Li F, Zhang D, Li X, et al. Inhibition of curcumin on myeloid-derived suppressor cells is requisite for controlling lung cancer. Int Immunopharmacol (2016) 39:265–72. doi: 10.1016/j.intimp.2016.07.035
328. Tian S, Liao L, Zhou Q, Huang X, Zheng P, Guo Y, et al. Curcumin inhibits the growth of liver cancer by impairing myeloid-derived suppressor cells in murine tumor tissues. Oncol Lett (2021) 21(4):286. doi: 10.3892/ol.2021.12547
329. Wang SH, Lu QY, Guo YH, Song YY, Liu PJ, Wang YC. The blockage of notch signalling promoted the generation of polymorphonuclear myeloid-derived suppressor cells with lower immunosuppression. Eur J Cancer (2016) 68:90–105. doi: 10.1016/j.ejca.2016.08.019
330. Mao L, Zhao ZL, Yu GT, Wu L, Deng WW, Li YC, et al. Gamma-secretase inhibitor reduces immunosuppressive cells and enhances tumour immunity in head and neck squamous cell carcinoma. Int J Cancer (2018) 142(5):999–1009. doi: 10.1002/ijc.31115
331. Qiu H, Zmina PM, Huang AY, Askew D, Bedogni B. Inhibiting Notch1 enhances immunotherapy efficacy in melanoma by preventing Notch1 dependent immune suppressive properties. Cancer Lett (2018) 434:144–51. doi: 10.1016/j.canlet.2018.07.024
332. Zhang CX, Ye SB, Ni JJ, Cai TT, Liu YN, Huang DJ, et al. Sting signaling remodels the tumor microenvironment by antagonizing myeloid-derived suppressor cell expansion. Cell Death Differ (2019) 26(11):2314–28. doi: 10.1038/s41418-019-0302-0
333. Wang G, Zhou X, Guo Z, Huang N, Li J, Lv Y, et al. The anti-fibrosis drug pirfenidone modifies the immunosuppressive tumor microenvironment and prevents the progression of renal cell carcinoma by inhibiting tumor autocrine tgf-beta. Cancer Biol Ther (2022) 23(1):150–62. doi: 10.1080/15384047.2022.2035629
334. Gobbo J, Marcion G, Cordonnier M, Dias AMM, Pernet N, Hammann A, et al. Restoring anticancer immune response by targeting tumor-derived exosomes with a Hsp70 peptide aptamer. J Natl Cancer Inst (2016) 108(3):djv330. doi: 10.1093/jnci/djv330
335. Deng Y, Yang J, Qian J, Liu R, Huang E, Wang Y, et al. Tlr1/Tlr2 signaling blocks the suppression of monocytic myeloid-derived suppressor cell by promoting its differentiation into M1-type macrophage. Mol Immunol (2019) 112:266–73. doi: 10.1016/j.molimm.2019.06.006
336. Zhang W, He W, Shi X, Li X, Wang Y, Hu M, et al. An asparagus polysaccharide fraction inhibits mdscs by inducing apoptosis through toll-like receptor 4. Phytother Res (2018) 32(7):1297–303. doi: 10.1002/ptr.6058
337. He W, Zhang W, Zheng Q, Wei Z, Wang Y, Hu M, et al. Cinnamaldehyde causes apoptosis of myeloid-derived suppressor cells through the activation of Tlr4. Oncol Lett (2019) 18(3):2420–6. doi: 10.3892/ol.2019.10544
338. Shayan G, Kansy BA, Gibson SP, Srivastava RM, Bryan JK, Bauman JE, et al. Phase ib study of immune biomarker modulation with neoadjuvant cetuximab and Tlr8 stimulation in head and neck cancer to overcome suppressive myeloid signals. Clin Cancer Res (2018) 24(1):62–72. doi: 10.1158/1078-0432.CCR-17-0357
339. Dang Y, Rutnam ZJ, Dietsch G, Lu H, Yang Y, Hershberg R, et al. Tlr8 ligation induces apoptosis of monocytic myeloid-derived suppressor cells. J Leukoc Biol (2018) 103(1):157–64. doi: 10.1002/JLB.5AB0217-070R
340. Cho JH, Lee HJ, Ko HJ, Yoon BI, Choe J, Kim KC, et al. The Tlr7 agonist imiquimod induces anti-cancer effects via autophagic cell death and enhances anti-tumoral and systemic immunity during radiotherapy for melanoma. Oncotarget (2017) 8(15):24932–48. doi: 10.18632/oncotarget.15326
341. Spinetti T, Spagnuolo L, Mottas I, Secondini C, Treinies M, Ruegg C, et al. Tlr7-based cancer immunotherapy decreases intratumoral myeloid-derived suppressor cells and blocks their immunosuppressive function. Oncoimmunology (2016) 5(11):e1230578. doi: 10.1080/2162402X.2016.1230578
342. Liu Z, Xie Y, Xiong Y, Liu S, Qiu C, Zhu Z, et al. Tlr 7/8 agonist reverses oxaliplatin resistance in colorectal cancer Via directing the myeloid-derived suppressor cells to tumoricidal M1-macrophages. Cancer Lett (2020) 469:173–85. doi: 10.1016/j.canlet.2019.10.020
343. Zoglmeier C, Bauer H, Noerenberg D, Wedekind G, Bittner P, Sandholzer N, et al. Cpg blocks immunosuppression by myeloid-derived suppressor cells in tumor-bearing mice. Clin Cancer Res (2011) 17(7):1765–75. doi: 10.1158/1078-0432.CCR-10-2672
344. Hossain DM, Pal SK, Moreira D, Duttagupta P, Zhang Q, Won H, et al. Tlr9-targeted Stat3 silencing abrogates immunosuppressive activity of myeloid-derived suppressor cells from prostate cancer patients. Clin Cancer Res (2015) 21(16):3771–82. doi: 10.1158/1078-0432.CCR-14-3145
345. Sobo-Vujanovic A, Vujanovic L, DeLeo AB, Concha-Benavente F, Ferris RL, Lin Y, et al. Inhibition of soluble tumor necrosis factor prevents chemically induced carcinogenesis in mice. Cancer Immunol Res (2016) 4(5):441–51. doi: 10.1158/2326-6066.CIR-15-0104
346. Atretkhany KS, Nosenko MA, Gogoleva VS, Zvartsev RV, Qin Z, Nedospasov SA, et al. Tnf neutralization results in the delay of transplantable tumor growth and reduced mdsc accumulation. Front Immunol (2016) 7:147. doi: 10.3389/fimmu.2016.00147
347. Dominguez GA, Condamine T, Mony S, Hashimoto A, Wang F, Liu Q, et al. Selective targeting of myeloid-derived suppressor cells in cancer patients using ds-8273a, an agonistic trail-R2 antibody. Clin Cancer Res (2017) 23(12):2942–50. doi: 10.1158/1078-0432.CCR-16-1784
348. Hartwig T, Montinaro A, von Karstedt S, Sevko A, Surinova S, Chakravarthy A, et al. The trail-induced cancer secretome promotes a tumor-supportive immune microenvironment Via Ccr2. Mol Cell (2017) 65(4):730–42 e5. doi: 10.1016/j.molcel.2017.01.021
349. Hiramoto K, Satoh H, Suzuki T, Moriguchi T, Pi J, Shimosegawa T, et al. Myeloid lineage-specific deletion of antioxidant system enhances tumor metastasis. Cancer Prev Res (Phila) (2014) 7(8):835–44. doi: 10.1158/1940-6207.CAPR-14-0094
350. Espagnolle N, Barron P, Mandron M, Blanc I, Bonnin J, Agnel M, et al. Specific inhibition of the vegfr-3 tyrosine kinase by Sar131675 reduces peripheral and tumor associated immunosuppressive myeloid cells. Cancers (Basel) (2014) 6(1):472–90. doi: 10.3390/cancers6010472
351. Feng PH, Chen KY, Huang YC, Luo CS, Wu SM, Chen TT, et al. Bevacizumab reduces S100a9-positive mdscs linked to intracranial control in patients with egfr-mutant lung adenocarcinoma. J Thorac Oncol (2018) 13(7):958–67. doi: 10.1016/j.jtho.2018.03.032
352. Maughan BL, Pal SK, Gill D, Boucher K, Martin C, Salgia M, et al. Modulation of premetastatic niche by the vascular endothelial growth factor receptor tyrosine kinase inhibitor pazopanib in localized high-risk prostate cancer followed by radical prostatectomy: A phase ii randomized trial. Oncologist (2018) 23(12):1413–e151. doi: 10.1634/theoncologist.2018-0652
353. Horikawa N, Abiko K, Matsumura N, Baba T, Hamanishi J, Yamaguchi K, et al. Anti-vegf therapy resistance in ovarian cancer is caused by gm-Csf-Induced myeloid-derived suppressor cell recruitment. Br J Cancer (2020) 122(6):778–88. doi: 10.1038/s41416-019-0725-x
354. Lacal PM, Atzori MG, Ruffini F, Scimeca M, Bonanno E, Cicconi R, et al. Targeting the vascular endothelial growth factor receptor-1 by the monoclonal antibody D16f7 to increase the activity of immune checkpoint inhibitors against cutaneous melanoma. Pharmacol Res (2020) 159:104957. doi: 10.1016/j.phrs.2020.104957
355. Grover A, Sanseviero E, Timosenko E, Gabrilovich DI. Myeloid-derived suppressor cells: A propitious road to clinic. Cancer Discov (2021) 11(11):2693–706. doi: 10.1158/2159-8290.CD-21-0764
356. Veglia F, Sanseviero E, Gabrilovich DI. Myeloid-derived suppressor cells in the era of increasing myeloid cell diversity. Nat Rev Immunol (2021) 21(8):485–98. doi: 10.1038/s41577-020-00490-y
Keywords: MDSC, pharmacotherapy, immune suppression, immunomodulation, inflammation
Citation: van Geffen C, Heiss C, Deißler A and Kolahian S (2022) Pharmacological modulation of myeloid-derived suppressor cells to dampen inflammation. Front. Immunol. 13:933847. doi: 10.3389/fimmu.2022.933847
Received: 01 May 2022; Accepted: 26 July 2022;
Published: 30 August 2022.
Edited by:
Chih-Chi Andrew Hu, Houston Methodist Research Institute, United StatesReviewed by:
Annalisa Adamo, University of Verona, ItalyAdam William Mailloux, The University of Iowa, United States
Rahul Shinde, Wistar Institute, United States
Copyright © 2022 van Geffen, Heiss, Deißler and Kolahian. This is an open-access article distributed under the terms of the Creative Commons Attribution License (CC BY). The use, distribution or reproduction in other forums is permitted, provided the original author(s) and the copyright owner(s) are credited and that the original publication in this journal is cited, in accordance with accepted academic practice. No use, distribution or reproduction is permitted which does not comply with these terms.
*Correspondence: Saeed Kolahian, saeed.kolahian@uni-marburg.de