- 1Instituto de Farmacología y Morfofisiología, Facultad de Ciencias Veterinarias, Universidad Austral de Chile, Valdivia, Chile
- 2Instituto de Ciencias Clínicas Veterinarias, Facultad de Ciencias Veterinarias, Universidad Austral de Chile, Valdivia, Chile
- 3Escuela de Graduados, Facultad de Ciencias Veterinarias, Universidad Austral de Chile, Valdivia, Chile
Neutrophil extracellular traps (NETs) are a recently described mechanism of neutrophils that play an important role in health and disease. NETs are an innate defense mechanism that participate in clearance of pathogens, but they may also cause collateral damage in unrelated host tissues. Neutrophil dysregulation and NETosis occur in multiple lung diseases, such as pathogen-induced acute lung injury, pneumonia, chronic obstructive pulmonary disease (COPD), severe asthma, cystic fibrosis, and recently, the novel coronavirus SARS-CoV-2. More recently, research into immunometabolism has surged due to the possibility of reprogramming metabolism in order to modulate immune functions. The present review analyzes the different metabolic pathways associated with NETs formation, and how these impact on pathologies of the airways.
1 Introduction
Innate immunity is a primary respiratory defense line against pathogens, allergens and environmental pollutants (1); neutrophils are a major presence in respiratory organs (2). These cells are the first responder cell type for combating pathological insults (3, 4) or sterile lesions, in which they contribute to healing and recovery besides participating in immune responses (5, 6). These cells can release protease and microbicide peptide-containing granules, they produce intra- and extracellular reactive oxygen species (ROS), and they can phagocyte microorganisms or release chromatin traps with cytoplasmic and granular proteins which include myeloperoxidase and elastase (7, 8). Chromatin traps, described in 2004 by using bacteria as stimuli, are termed neutrophil extracellular traps (NETs) (9). However, there is evidence to suggest that other microorganisms besides bacteria (viruses, fungi, protozoa) and non-microbial molecules can also induce NETs release by human neutrophils (8, 10). Unfortunately, pulmonary dysregulation of neutrophil NETosis occur in multipe diseases such as chronic obstructive pulmonary disease (COPD), severe asthma, cystic fibrosis (CF), and the novel coronavirus SARS-CoV-2. In this review, we discuss metabolic reprogramming regarding dysregulation of innate immune responses, focusing on NETs formation in asthma, COPD, CF and SARS-CoV-2.
2 Role of Metabolic Reprogramming in Cells
Cellular metabolism can be defined as a set of fundamental biochemical processes through which cells maintain their energy homeostasis and receive the necessary components for the biosynthesis of macromolecules (11). Immune cell metabolism directly influences their differentiation and function, which affects immunity, tolerance, and the inflammatory response (12). Inflammation entails dramatic changes in the metabolism of the affected tissue, including nutrient depletion, increased consumption of molecular oxygen (O2), and generation of ROS and reactive nitrogen species (13). These changes in tissue metabolism result, at least partially, from the massive inflammatory cell influx, especially of the myeloid type such as monocytes and neutrophils, which dramatically modify their functional activity in response to proinflammatory agents (5, 14). These environmental and functional alterations represent a major metabolic stress, which is usually efficiently managed due to cells ability to dynamically reprogram their metabolism (15, 16). How cells modify their metabolic patterns to adapt to the inflammatory environment and respond in the face of insult determines the course and prognosis of these diseases (11, 17). If these metabolic processes are dysregulated, the innate immune function can be significantly impaired. Immunometabolism emerges as a new field of research at the interface between immunology and metabolism, historically separated disciplines (17, 18).
3 Metabolic Requirements for NET Formation
Formation of NETs, or NETosis, is a cell death mechanism (different from necrosis or apoptosis) which seems to be essential to the innate immune response, permitting capture and degradation of pathogens and their virulence factors (19–21). During NETosis the neutrophil nucleus grows, its chromatin decondenses and long DNA strands are secreted towards the extracellular medium along with cytoplasmic granular proteins (which also disintegrate as the nucleus dissolves) and from chromatin (histones) (9, 22, 23). Bactericidal proteins associated with NETs are mainly cationic and therefore with high affinity to DNA: histones, defensins, proteinase 3, lactoferrin, cathepsin G, neutrophil elastase (NE) and myeloperoxidase (MPO), among others (24). Pentraxin 3 (25) and S100A8/A9 (calprotectin complex, which constitutes 40% of the cytosolic proteins of the neutrophil) are also associated to NETs (24).
NET formation (Figure 1) was initially described and characterized through stimulation of human and murine neutrophils with PMA (26). This classic NETosis mechanism involves NADPH oxidase activation via protein kinase C (PKC) and RAF-MEK-ERK pathways, which lead to ROS production and activation of calcium dependent enzyme peptidil arginine deiminase (PAD4), which is particularly abundant in mature neutrophils (27–30). Upon activation, PAD4 translocates to the nucleus and induces histone hypercitrullination, converting positively charged arginine side chains into uncharged histone citrulline side chains, which reduces electrostatic force between histones and DNA and causes chromatin decondensation (31–33). Additionally, MPO converts hydrogen peroxide into hypochlorous acid and other oxidants, which release NE from azurosome in azurophilic granules, allowing its nuclear translocation where it favors chromatin unfolding and nuclear membrane breakdown, releasing chromatin into the cytosol (34–36). NE also cleaves and activates gasdermin D (GSDMD), which leads to pore formation in the granular and plasma membrane, enhancing release of NE and other granular proteases into the cytoplasm, as well as the NE nuclear translocation and GSDMD cleavage (37, 38). Finally, after associating with cytosolic and granular proteins, chromatin is secreted extracellularly (23). Other stimuli apart from PMA can trigger the NETs formation depending on the respiratory burst, including some classical pathogens as bacteria and its products (28, 39–41), viruses (42–44), fungi (45–47) and parasites (48–51).
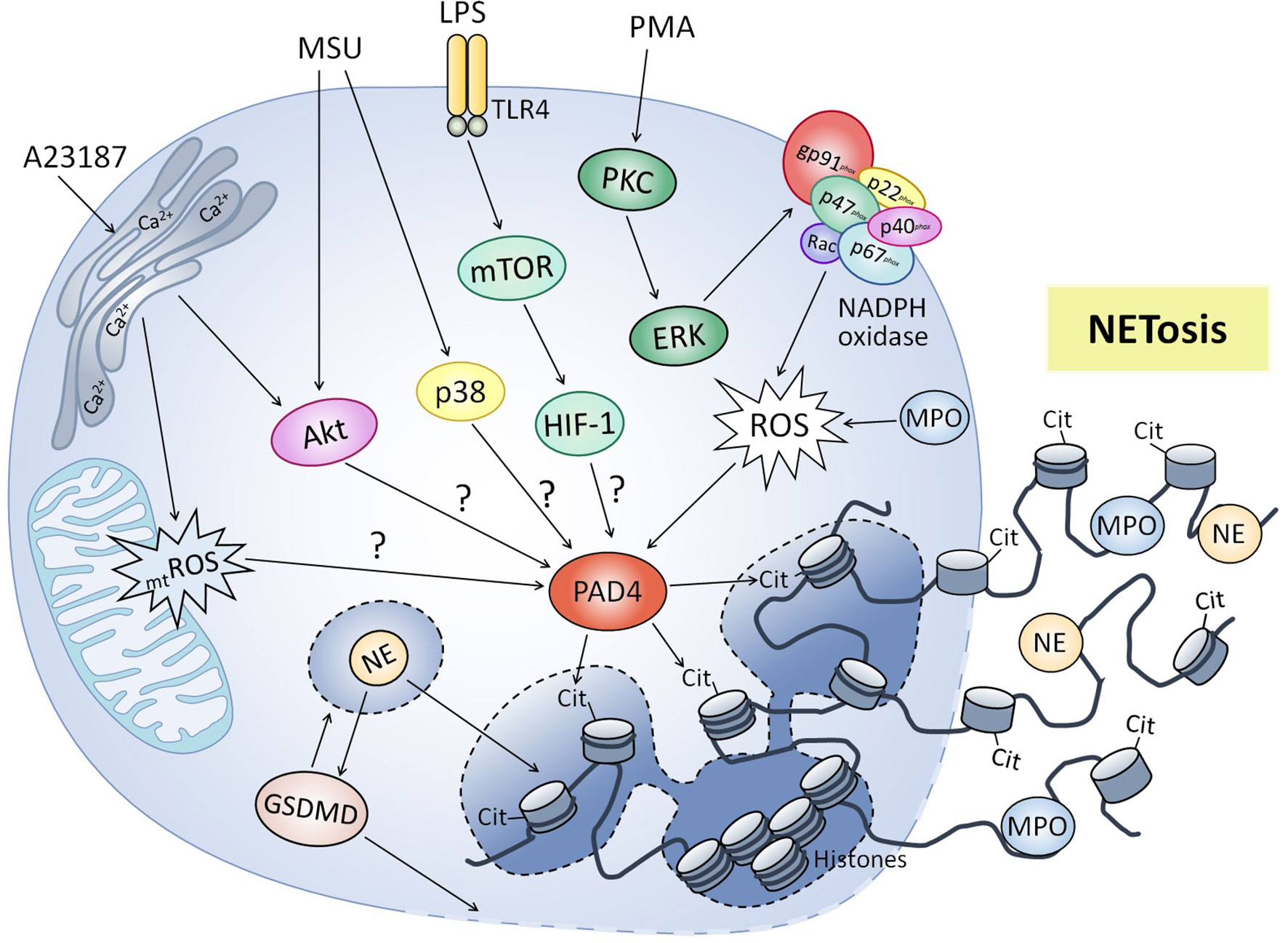
Figure 1 Molecular mechanisms regulating neutrophil extracellular traps formation. Classic NETosis induced by phorbol 12-myristate 13-acetate (PMA) involved activation of NADPH oxidase via PKC, production of reactive oxygen species (ROS) and activation of peptidyl arginine deiminase 4 (PAD4), which results in hypercitrullination (cit) of histones and chromatin decondensation. Lipopolysaccharide (LPS) induces NET formation by mTOR/HIF-1 activation, although molecular mechanisms have not been elucidated (?). Other stimuli like calcium ionophore A23187 and monosodium urate (MSU) crystals also trigger NET formation independent of NADPH oxidase, involving signaling pathways like PI3K/Akt and p38 MAPK. Mitochondrial ROS (mtROS) production has been also involved in NADPH-independent NET release. Myeloperoxidase (MPO) participates in NETosis by generating oxidative compounds necessary for the release of neutrophilic elastase (NE). NE also helps chromatin decondensation and activates gasdermin D (GSDMD), which forms pores in the granular and plasma membrane facilitating the release of NETs.
Otherwise, NET release has been also described independently of NADPH activation after stimulation with agonists as calcium ionophores (52, 53), non-esterified fatty acids (NEFAs) (54), uric acid (55), monosodium-urate (MSU) crystals (56) and pathogen agents as Staphylococcus aureus (S. aureus) (57), Candida albicans (58), Entamoeba histolytica (E. histolytica) (30) and Dengue virus (59). Interestingly, NET release induced by MSU crystals in human neutrophils (56) and D-lactate in bovine neutrophils (60) was independent of respiratory burst but dependent on PAD4 activity, suggesting that the latter can be also activated by mechanisms independent of NADPH oxidase. NET formation independently of NADPH oxidase and PAD4 induced by E. histolytica has also been described in human neutrophils (61), as well as the independently of PAD4 in a murine model of pneumonia caused by Klebsiella pneumoniae (62). Neutrophil mitochondria also appear to play a role in NETosis mechanisms. In this respect, NET release induced by the calcium ionophore A23187 in human neutrophils was triggered through a mechanism independent of NADPH oxidase activity but dependent on mitochondrial ROS production (52). Likewise, mitochondria participate in NET formation induced by platelet-activating factor (PAF) in bovine neutrophils by producing ATP necessary to activate purinergic signaling mechanism (63). Supporting the above, ATP contributes to classical PMA-induced NETosis, and NADPH oxidase independent NET formation triggered by A23187 in murine neutrophils (64). Furthermore, some studies have suggested that extracellular DNA strands could have a mitochondrial origin, which would be also compatible with the functional activity of the neutrophil without involving its death (65–69).
In addition to the involvement of RAF-MEK-ERK signaling pathways for NETosis, contribution of the PI3K/Akt axis has also been reported with several stimuli capable of inducing NET formation through the classical pathway (52, 70–72) and NADPH oxidase-independent pathway (30, 52, 56). The PI3K/Akt signaling pathway regulates classic NETosis induced by PMA (52, 70) and immune complexes (71), as well as NADPH oxidase-independent NETosis induced by E. histolytica (30), A23187 (52) and MSU crystals (56). In addition, contribution of p38 MAPK in NETosis induced by PMA, MSU crystals, histamine, bacteria and parasites has been demonstrated (28, 29, 49, 56, 72), while TAK1 and Syk pathways in NET formation induced by MSU crystals have also been observed (56). Interestingly, mammalian target of rapamycin (mTOR) mediates LPS-triggered NET formation in murine and human neutrophils by post-transcriptional control of expression of hypoxia-inducible factor 1´s alpha subunit (HIF-1α) (73). Although the mechanisms through which HIF-1 participates in NETs formation aren´t fully known, HIF-1´s role in regulating the expression of enzymes associated with glycolytic metabolism is widely understood (74, 75), suggesting an association between NETosis and metabolism.
Metabolic requirements (Figure 2) for NET formation have been an object of study in the past years, with glycolysis identified as a pivotal metabolic pathway for its development. Initially, Rodríguez-Espinosa et al. demonstrated that NETosis induced by PMA in human neutrophils depends on exogenous glucose and glutamine (26). Furthermore, glycolysis inhibition through 2-deoxy-D-glucose (2-DG) blocks PMA-triggered NET release, while the ATP synthase inhibitor oligomycin only partially reduces it (26). Similar relevance to exogenous glucose and glycolysis was demonstrated by Azevedo et al. in classical NETosis triggered by PMA and amyloid fibrils in humans, further identifying a key role of the pentose phosphate pathway, since glucose-6-phosphate dehydrogenase provides NADPH to NADPH oxidase, for ROS production and NETs release (76). Amini et al. also reported a decrease in NETosis induced by granulocyte-macrophage colony-stimulating factor (GM-CSF) + C5a due to glycolysis inhibition by 2-DG in human and murine neutrophils (77). However, mitochondrial complex I activity was also involved in NET release (77). More recently, Quiroga et al. also showed that blocking glycolysis with 2-DG inhibited NETosis triggered by PAF in bovine neutrophils (63). In addition, blocking mitochondrial complex I activity and oxidative phosphorylation with rotenone and carbonyl cyanide 3-chlorophenylhydrazone (CCCP), respectively, prevented extracellular ATP release and NETosis, and pharmacological inhibition of P2X1 purinergic receptor inhibited NET formation, suggesting the role of purinergic signaling in NETosis mechanism (63). In contrast to the above, Zhou et al. observed an increase in glucose consumption after Besnoitia besnoiti tachyzoite exposure in bovine neutrophils; however, glycolysis blocking by 2-fluor-2-deoxy-D-glucose (FDG) didn´t influence NET release (78). Interestingly, these authors also observed a relevant role of mitochondrial ATP and P2X1 receptor-dependent purinergic signaling for tachyzoite-triggered NETosis (78). In agreement with previous authors, Alarcón et al. recently showed that NET formation induced by NEFAs in bovine neutrophils was strongly dependent on purinergic signaling, since pharmacological inhibition of pannexin 1 channels and P2X1 receptors reduced NET release partially and totally, respectively (54). In addition, inhibition of β-oxidation with etomoxir partially reduced NETosis induced by NEFAs, suggesting some degree of involvement of this metabolic pathway (54).
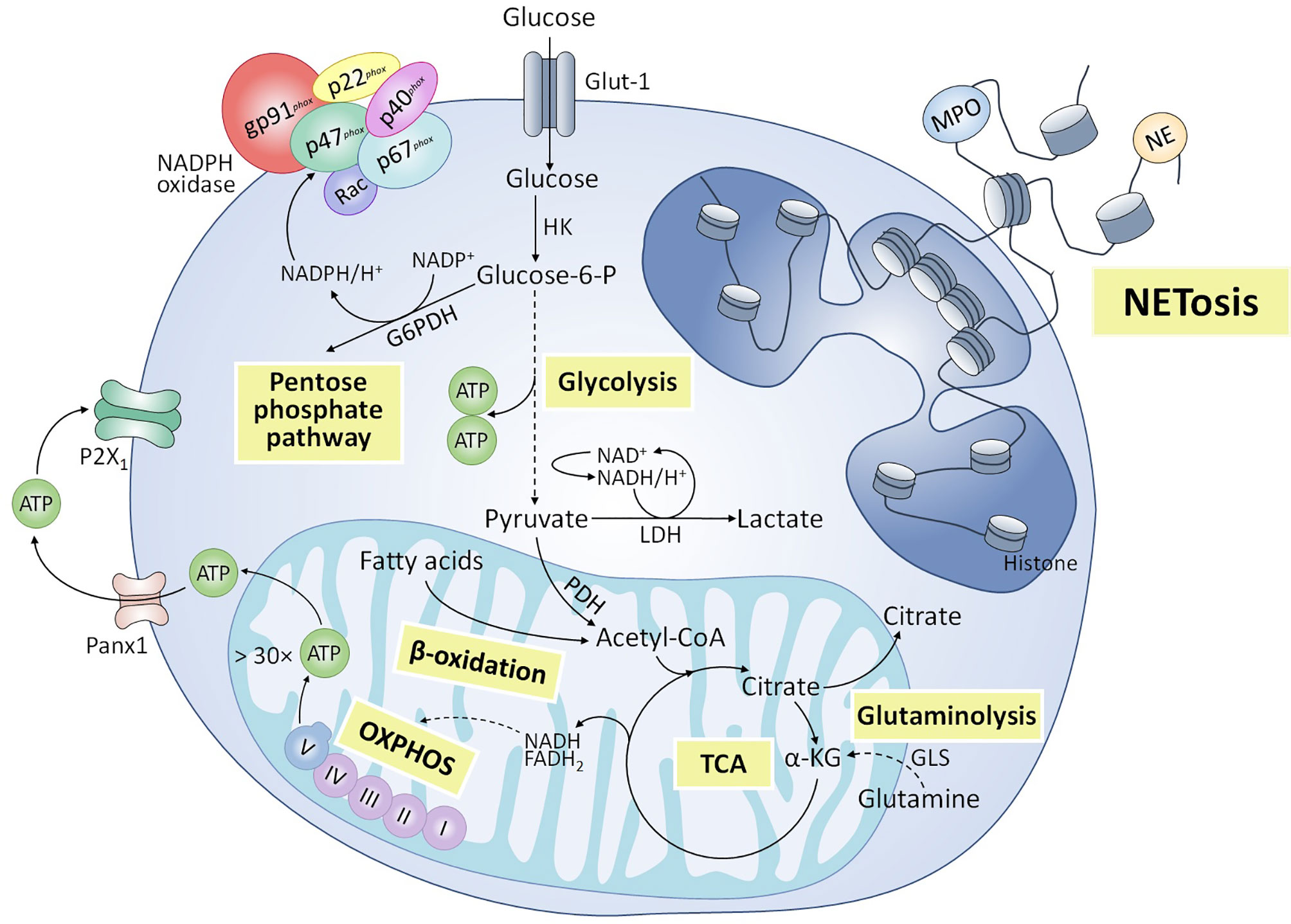
Figure 2 Metabolic pathways involved in NETosis. Various metabolic routes have been implicated in the release of NETs. Glycolysis is an essential metabolic pathway for NADPH oxidase-dependent and -independent NETosis triggered by various stimuli. Pentose phosphate pathway has also been shown to participate in classical NETosis, by providing the NADPH necessary to produce reactive oxygen species (ROS) by the NADPH oxidase complex. Other metabolic pathways associated with mitochondrial activity also appear to be partially involved in NET formation, including hydrolysis of glutamine by glutaminase (GLS) and subsequent anaplerotic reactions to form α-ketoglutarate (α-KG) that enters the tricarboxylic acid (TCA) cycle, and β-oxidation of fatty acids to supply acetyl-coenzyme A (CoA) to the TCA cycle. In addition, the adenosine triphosphate (ATP) synthesized in mitochondria as a product of oxidative phosphorylation (OXPHOS) would be released into the extracellular space through pannexin 1 (Panx1) channels and would enhance NET formation through purinergic signaling mechanisms dependent on P2X1 receptors.
The molecular mechanisms involved in the NET formation, as well as the metabolic pathways on which it depends, seem to be mainly dependent on the inducing stimulus and the inflammatory context. However, they are not yet fully understood and are still being studied.
4 Metabolic Adaptations in Neutrophils During Airways Pathological Conditions
4.1 Cystic Fibrosis (CF)
CF is a monogenic multiorganic disease that affects epithelial organs, with mortality often occurring from airway disease (79). Pulmonary damage is a consequence of chronic airway inflammation associated with bacterial colonization (80). In the disease, the CF transmembrane conductance regulator (CFTR) gene, encoding a chloride-bicarbonate transmembrane anion channel, suffers autosomal recessive mutations (81). In this disease, NETs have a deleterious effect in inflammation and lung destruction instead of working in their antimicrobial capacity. Some studies have shown that extracellular DNA concentration correlates with lung neutrophil concentration, and therefore can be used to measure pulmonary inflammation and severity of disease (82, 83). Neutrophils fail to clear infections in cases of CF, due to reduction of CD16-CD14 receptors and functional changes (84). The same authors observed an increase in lipid raft assembly, granule mobilization and CD11b and CD66 expression. Studies on metabolic reprogramming in CF airway neutrophils may explain how such dysfunctional phenotype develops. The mTOR pathway is activated in CF airway neutrophils (85), and glucose transporter 1 (Glut1) and inorganic phosphate transporter 1 (PiT1) expression both increase (86), all of which promotes glucose utilization in the airways (87). Other authors have also shown that airway neutrophils in CF patients respond to excess free glucose and amino acids, concomitantly increasing Glut1 expression. CF cases also increase production of resistin, a regulatory protein (88) closely related to insulin resistance (89). While insulin resistance impairs glucose uptake by cells, anabolic reprogramming of CF airway neutrophils allows them to efficiently uptake and utilize glucose, fueling their pro-survival pathways (84, 85). Unfortunately, resistin also diminishes neutrophils´ bactericidal ability because it inhibits actin polymerization and ROS production, as has been observed for the pathogens Pseudomonas aeruginosa and S. aureus which are closely associated to CF (90). On the other hand, the mechanisms that regulate NET formation, especially during chronic lung inflammation, as occurs in CF, are not well understood. One study showed that the G protein-coupled receptor (GPCR) CXCR2 mediates NET formation (91) independently of NADPH oxidase, but with involvement of Src family kinases. Pulmonary blockade of CXCR2 inhibits NET formation and ameliorated lung function in vivo, with no effect on neutrophil recruitment, proteolytic activity or antibacterial defense of the host. Those authors concluded that CXCR2 is a receptor that mediates NET formation independently of NADPH oxidase (91).
4.2 Chronic Obstructive Pulmonary Disease (COPD)
Chronic obstructive pulmonary disease (COPD) is a leading cause of worldwide morbidity and mortality (92). Chronic exposure to cigarette smoke, environmental pollutants or other inhaled irritants, is its primary cause (93). Neutrophils correlate directly with severity and inflammation and seem to be key effectors in the pathophysiology of the disease (94, 95). Progressive destruction of lung parenchyma, as well as poor responses in infective exacerbations, are thought to be due to dysregulated functions of neutrophils in COPD patients, which has been proven in vitro (96). NETs formation is increased in the sputum of stable COPD patients, which correlates with airway neutrophil numbers and extracellular DNA concentration, limited lung function and disease severity (97–99). Likewise, expression of PAD4 gene is up-regulated in neutrophilic COPD cases, compared to non-neutrophilic patients (100). Increased expression of PAD4 has been described in lung samples from COPD patients (101), which leads some authors to support that NETs formation could be a feature of the NET-COPD phenotype (102). Furthermore, peripheral blood neutrophils from e-cigarette users had a high susceptibility to NETosis induction, and there were more NETs-related proteins in these subjects´ sputum compared to nonsmokers (103).
Although glycolysis is the primary energy source for neutrophils, their few mitochondria are functional and contribute to ATP and ROS production, which are necessary for migration (104, 105). Some authors suggest that alterations of mitochondrial function and impaired glucose metabolism both may lead to defects in neutrophil migration and function in cases of COPD (1). Of the latter, one study demonstrated elevated glucose levels in the sputum of COPD patients, and this glucose increased further in response to exacerbations or experimental rhinovirus infection. Furthermore, they showed that increased glucose concentration in sputum samples could support bacterial growth, suggesting that increased glucose in the airways in response to viral infection could in turn facilitate secondary bacterial infections (106). However, in this study the production of NETs was not quantified, so we could not make an association between sputum glucose and NET formation, but as glycolysis is important in the production of this phenomenon, we could infer that there is an alteration of neutrophil cellular metabolism in COPD patients. Ultimately, the mechanisms of NET formation in COPD are still being explored and more studies are needed to understand this phenomenon. Some authors claim that it is unclear whether neutrophils undergo NETosis after migration into the lungs, or rather, whether they are constitutively primed to undergo this response during COPD-related inflammation while still in circulation (107). Cellular metabolic changes could also be important in defective innate immune responses in COPD, and unraveling these neutrophilic metabolic changes could lead to novel unconventional therapies for this pathology.
4.3 Severe Asthma
Asthma is a chronic inflammatory airway disease of enormous importance, with approximately 350 million cases around the world (108). It represents 1.1% of total global disease and produces loss of 26.2 million disability-adjusted life years according to WHO (109). The disease is generally characterized by airway inflammation, hyperresponsiveness, and reversible airflow obstruction (110, 111). The type and degree of airway inflammation and airway remodeling are heterogeneous, as are symptoms. Thus, various phenotype models have been developed (112), representing observable clinical characteristics which include age of onset (early or late onset), possible comorbidities (obesity and others), exacerbating factors (infections, allergens, and exercise among others), and response to treatment (for example, response to steroids) (113). Airway infiltration with eosinophils and mast cells -and their role in disease progression- is well described, and neutrophil infiltration has also been assessed in many clinical studies and is associated with disease severity (114). Marked relative neutrophilia in the sputum (more than 60%) is a more frequent finding in older adults. These patients are predominantly male, develop late-onset asthma, have more severe lung disease, resistance to treatment with corticosteroids, and a higher risk of hospitalization (115). They also had more comorbidities, including hypertension, osteoporosis, gastro-esophageal reflux disease, smoking, and obesity (115–118).
Neutrophils and NET secretion are fundamental in the pathogenesis of early asthma according to murine models of asthma (119–122). However, evidence for NETs in the pathogenesis of early asthma in human patients is still scarce. Peripheral blood NETs concentration is higher in children with asthma, particularly during episodes of symptom exacerbation, and studies showed that asthmatic children´s neutrophils can produce more NETs than healthy controls in vitro (123). In addition, extracellular DNA was higher in severe asthma cases´ sputum samples than in those of mild to moderate asthma (100). In that study, NETs levels were not only higher in asthmatic patients than in healthy controls: extracellular DNA concentration also correlated with asthma severity. IL-1β, IL-8 and gene expression levels of inflammasome components (such as NLRP3) sputum concentrations were also higher in patients with elevated extracellular DNA concentrations. Other authors also reported high concentrations of extracellular DNA in the sputum of severely asthmatic patients, with airway NETs and markers of inflammasome activation (124). However, as demonstrated in another study, circulating NETs seem to be better indicators of asthma severity than alveolar NETs concentration (125). Therefore, further clinical investigations are needed to elucidate the role of NETs in the progression of asthma. Nevertheless, studies have shown a cytotoxic effect of NETs in lung epithelial and endothelial cells (126–128). NETs can also impair lung epithelial barrier functions (129), induce release of proinflammatory mediators from dendritic cells, and of proteases from neutrophils (130). Authors suggest that NETs may act directly on airway epithelial cells, inducing secretion of inflammatory factors, aggravating airway inflammation and worsening respiratory symptoms (124, 126). Other studies have also shown that the protease contained in NETs activates proinflammatory cytokines, thus aggravating the inflammatory response (131). Thus, all these studies suggest that NETs destroy the integrity of the airway epithelium, increase cytokine secretion, and lead to asthma progression.
Several investigations relate metabolomic profiles to human asthma, focusing on metabolites as systemic biomarkers (132–135). Metabolites have also been studied in an equine model of asthma (136, 137), with neutrophilic phenotypic characteristics (138, 139). Previous studies have characterized the metabolic profile of circulating neutrophils, but those of pulmonary neutrophils are few. However, similar to severe asthma in humans, airway neutrophilia is typical of asthmatic horses (138, 139). NETs and evidence of oxidative stress have been described in bronchoalveolar lavage fluid (BALF) of asthma-affected horses (140). Albornoz et al. (137) found increased lactate, citrate and arabitol in BALF of asthmatic horses, suggesting elevated energy demand during exacerbation of the disease. This lactate increase has also been previously reported both in asthmatic children´s urine (141) and in BALF from patients with CF (142), demonstrating that those respiratory diseases course with similar energetic metabolic changes. In turn, an elevated citrate concentration was observed in horses with equine asthma (137), which could be due to an increase in glycolysis energy flow and alterations in Krebs cycle function, which can occur in activated inflammatory cells (143). Similarly, an increase in arabitol accumulation was observed in equine asthma (137). These same authors argue that this increase in arabitol could be explained by an increase in the oxidative phase of pentose phosphate pathway originating from large quantities of neutrophils recruited to the inflamed airways. However, the contribution of other cell types to metabolomic changes in BALF cannot be ruled out.
4.4 Severe Acute Respiratory Syndrome Coronavirus 2 (SARS-CoV-2)
Severe acute respiratory syndrome coronavirus (SARS-CoV)-2 was first found in Wuhan, China (144). The worldwide pandemic of coronavirus disease 19 (COVID-19) has affected all of humanity, with millions of deaths. Although SARS-CoV-2 can extend to many organs, including the heart, kidneys, gut, blood vessels and brain, it is initially a pulmonary disease (145). Viral replication and its subsequent immune response both contribute to COVID-19´s severity: in some patients, this entails a cytokine storm and severe inflammatory response syndrome (SIRS), secondary sepsis, multiorganic failure, and eventually death (146). Since the start of the COVID-19 pandemic, progressive pathophysiologic evidence shows that neutrophils play an important role in the disease, especially in severe patients (147). Neutrophil defense mechanisms (phagocytosis, ROS, NETosis among others) are differentially active in neutrophil subsets. The capacity of these mechanisms increases with maturation phase (148, 149). In circulation, progressive granulation in aging neutrophils impairs their functions and NET formation (150). The importance of this last sentence lies in the fact that in severe SARS-CoV-2 infection it is marked by altered abundance, phenotype and functionality of neutrophils. High numbers of neutrophils have been found in the nasopharyngeal epithelium, distal lung and in blood counts (151–153). Likewise, studies show changes in gene expression in blood neutrophils with pre-mature phenotypic markers in severe cases of COVID-19 (154, 155).
Analysis of COVID-19 patient lung samples showed neutrophilic mucositis, neutrophil infiltration of pulmonary capillaries, fibrin deposition, and neutrophil extravasation of neutrophils towards the alveolar space (156). Fox et al. (157) observed neutrophils and platelets trapped in a fibrin mesh within the alveolar capillaries of patients with COVID-19, which suggests the presence of NETs (157). In turn, other authors have detected augmented serum cell-free DNA concentration, DNA-MPO complexes, and citrullinated histone H3 (Cit-H3), all of which correlate with disease severity (158). In fact, through unknown mechanisms, viable SARS-CoV-2 can directly induce NET formation in healthy neutrophils (159). Likewise, NET formation and alveolar epithelial necrotic cell death both release damage-associated molecular patterns which entail production of proinflammatory cytokines, and vice versa, establishing a necroinflammatory loop that is responsible for cytokine storm and sepsis (160). There are still no studies of alterations in neutrophil cellular metabolism for the induction of NETs, but there are metabolomic studies showing that increased glucose concentration and glycolysis promote cytokine production by monocytes and replication of SARS-CoV-2 (CoV-2) via a mitochondrial ROS/HIF-1α dependent mechanism, which lead to T-cell and epithelial cell death. Therefore, people with hyperglycemia (for example, diabetics) have an increased risk of developing severe COVID-19 disease, which suggests that metabolism plays an important role in the disease progression of COVID-19 infection (161, 162). Of the latter, it is known that the tricarboxylic acids (TCA) cycle produces metabolites used in the synthesis of amino acids, lipids and nucleotides, all of which viruses need to replicate (163). In addition, mTOR complex 1 (mTORC1) has been shown to regulate mitochondrial activity and the anabolic metabolism, while on the contrary, mTORC1 inhibitors can decrease TCA cycle metabolite concentrations (164). Mullen et al. (165) suggest that SARS-CoV-2 readjusts carbon input into the TCA cycle, which results in a reduction of the oxidative metabolism of glutamine and increases the input of pyruvate, via pyruvate carboxylase. These same authors demonstrated that inhibition of mTORC1 produces a decrease in SARS-CoV-2 infection, which could justify a potential therapeutic option for treating patients with COVID-19; however, further studies are needed to understand the mechanism of inhibition and its potential applications and efficacy in patients.
5 Conclusion
Recent advances in the field of immunometabolism emphasize the plasticity of neutrophil biology in health and disease. In addition, neutrophil-mediated innate immunity has been redefined since the identification of NETs. NETosis plays an important role in physiological and pathological conditions, and their metabolic implications (Table 1) must be understood in order to enable exploration of possible therapeutic interventions. Although formation of NETs is in essence a useful antimicrobial defense strategy, dysregulation of the process may entail tissular adverse effects and hence contribute to NETopathic lung inflammation. Novel therapeutic strategies targeted at neutrophils, such as inhibitors of neutrophil recruitment or NET formation, may help reduce the severity of multiple pulmonary diseases, including asthma, COPD, CF and COVID-19.
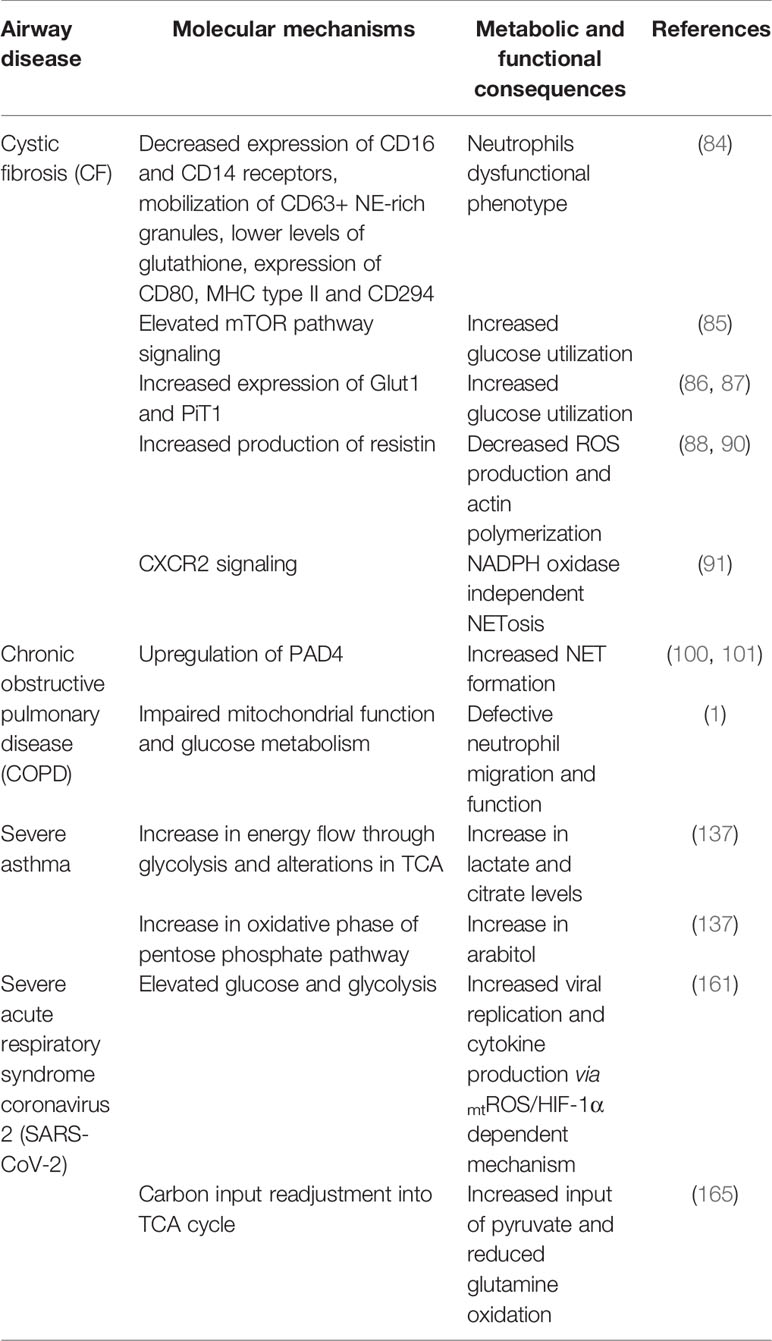
Table 1 Molecular mechanisms and metabolic pathways possibly altered in leucocytes in airway diseases.
Author Contributions
All listed authors have made a direct and substantial contribution to this work and approved the final manuscript.
Funding
This work was partially supported by FONDECYT grant 1190369.
Conflict of Interest
The authors declare that the research was conducted in the absence of any commercial or financial relationships that could be construed as a potential conflict of interest.
Publisher’s Note
All claims expressed in this article are solely those of the authors and do not necessarily represent those of their affiliated organizations, or those of the publisher, the editors and the reviewers. Any product that may be evaluated in this article, or claim that may be made by its manufacturer, is not guaranteed or endorsed by the publisher.
References
1. Michaeloudes C, Bhavsar PK, Mumby S, Xu B, Hui CKM, Chung KF, et al. Role of Metabolic Reprogramming in Pulmonary Innate Immunity and Its Impact on Lung Diseases. J Innate Immun (2020) 12:31–46. doi: 10.1159/000504344
2. Giacalone VD, Margaroli C, Mall MA, Tirouvanziam R. Neutrophil Adaptations Upon Recruitment to the Lung: New Concepts and Implications for Homeostasis and Disease. Int J Mol Sci (2020) 21:851. doi: 10.3390/ijms21030851
3. Kovach MA, Standiford TJ. The Function of Neutrophils in Sepsis. Curr Opin Infect Dis (2012) 25:321–7. doi: 10.1097/QCO.0b013e3283528c9b
4. Mócsai A. Diverse Novel Functions of Neutrophils in Immunity, Inflammation, and Beyond. J Exp Med (2013) 210:1283–99. doi: 10.1084/jem.20122220
5. Kolaczkowska E, Kubes P. Neutrophil Recruitment and Function in Health and Inflammation. Nat Rev Immunol (2013) 13:159–75. doi: 10.1038/nri3399
6. Szabady RL, McCormick BA. Control of Neutrophil Inflammation at Mucosal Surfaces by Secreted Epithelial Products. Front Immunol (2013) 4:220. doi: 10.3389/fimmu.2013.00220
7. Nathan C. Neutrophils and Immunity: Challenges and Opportunities. Nat Rev Immunol (2006) 6:173–82. doi: 10.1038/nri1785
8. Guimarães-Costa AB, Nascimento MTC, Wardini AB, Pinto-da-Silva LH, Saraiva EM. ETosis: A Microbicidal Mechanism Beyond Cell Death. J Parasitol Res (2012) 2012:929743. doi: 10.1155/2012/929743
9. Brinkmann V, Reichard U, Goosmann C, Fauler B, Uhlemann Y, Weiss DS, et al. Neutrophil Extracellular Traps Kill Bacteria. Science (80-) (2004) 303:1532–5. doi: 10.1126/science.1092385
10. Branzk N, Papayannopoulos V. Molecular Mechanisms Regulating NETosis in Infection and Disease. Semin Immunopathol (2013) 35:513–30. doi: 10.1007/s00281-013-0384-6
11. Gaber T, Strehl C, Buttgereit F. Metabolic Regulation of Inflammation. Nat Rev Rheumatol (2017) 13:267–79. doi: 10.1038/nrrheum.2017.37
12. Kotas ME, Medzhitov R. Homeostasis, Inflammation, and Disease Susceptibility. Cell (2015) 160:816–27. doi: 10.1016/j.cell.2015.02.010
13. Kominsky DJ, Campbell EL, Colgan SP. Metabolic Shifts in Immunity and Inflammation. J Immunol (2010) 184:4062–8. doi: 10.4049/jimmunol.0903002
14. Campbell EL, Bruyninckx WJ, Kelly CJ, Glover LE, McNamee EN, Bowers BE, et al. Transmigrating Neutrophils Shape the Mucosal Microenvironment Through Localized Oxygen Depletion to Influence Resolution of Inflammation. Immunity (2014) 40:66–77. doi: 10.1016/j.immuni.2013.11.020
15. Loftus RM, Finlay DK. Immunometabolism: Cellular Metabolism Turns Immune Regulator. J Biol Chem (2016) 291:1–10. doi: 10.1074/jbc.R115.693903
16. O’Neill LAJ, Pearce EJ. Immunometabolism Governs Dendritic Cell and Macrophage Function. J Exp Med (2016) 213:15–23. doi: 10.1084/jem.20151570
17. O’Neill LAJ, Kishton RJ. Rathmell J. A Guide to Immunometabolism for Immunologists. Nat Rev Immunol (2016) 16:553–65. doi: 10.1038/nri.2016.70
18. Mathis D, Shoelson SE, Lugus JJ, Walsh K, Shoelson SE, Cantrell DA, et al. Immunometabolism: An Emerging Frontier. Nat Rev Immunol (2011) 11:81–3. doi: 10.1038/nri2922
19. Arazna M, Pruchniak MP, Demkow U. Neutrophil Extracellular Traps in Bacterial Infections: Strategies for Escaping From Killing. Respir Physiol Neurobiol (2013) 187:74–7. doi: 10.1016/j.resp.2013.03.002
20. Aulik NA, Hellenbrand KM, Klos H, Czuprynski CJ. Mannheimia Haemolytica and Its Leukotoxin Cause Neutrophil Extracellular Trap Formation by Bovine Neutrophils. Infect Immun (2010) 78:4454–66. doi: 10.1128/IAI.00840-10
21. Hellenbrand KM, Forsythe KM, Rivera-Rivas JJ, Czuprynski CJ, Aulik NA. Histophilus Somni Causes Extracellular Trap Formation by Bovine Neutrophils and Macrophages. Microb Pathog (2013) 54:67–75. doi: 10.1016/j.micpath.2012.09.007
22. Brinkmann V, Zychlinsky A. Beneficial Suicide: Why Neutrophils Die to Make NETs. Nat Rev Microbiol (2007) 5:577–82. doi: 10.1038/nrmicro1710
23. Fuchs TA, Abed U, Goosmann C, Hurwitz R, Schulze I, Wahn V, et al. Novel Cell Death Program Leads to Neutrophil Extracellular Traps. J Cell Biol (2007) 176:231–41. doi: 10.1083/jcb.200606027
24. Urban CF, Ermert D, Schmid M, Abu-Abed U, Goosmann C, Nacken W, et al. Neutrophil Extracellular Traps Contain Calprotectin, a Cytosolic Protein Complex Involved in Host Defense Against Candida Albicans. PloS Pathog (2009) 5:e1000639. doi: 10.1371/journal.ppat.1000639
25. Jaillon S, Peri G, Delneste Y, Frémaux I, Doni A, Moalli F, et al. The Humoral Pattern Recognition Receptor PTX3 Is Stored in Neutrophil Granules and Localizes in Extracellular Traps. J Exp Med (2007) 204:793–804. doi: 10.1084/jem.20061301
26. Rodríguez-Espinosa O, Rojas-Espinosa O, Moreno-Altamirano MMB, López-Villegas EO, Sánchez-García FJ. Metabolic Requirements for Neutrophil Extracellular Traps Formation. Immunology (2015) 145:213–24. doi: 10.1111/imm.12437
27. Hakkim A, Fuchs TA, Martinez NE, Hess S, Prinz H, Zychlinsky A, et al. Activation of the Raf-MEK-ERK Pathway Is Required for Neutrophil Extracellular Trap Formation. Nat Chem Biol (2011) 7:75–7. doi: 10.1038/nchembio.496
28. Ma F, Chang X, Wang G, Zhou H, Ma Z, Lin H, et al. Streptococcus Suis Serotype 2 Stimulates Neutrophil Extracellular Traps Formation via Activation of P38 MAPK and ERK1/2. Front Immunol (2018) 9:2854. doi: 10.3389/fimmu.2018.02854
29. Zhou E, Wu Z, Zhu X, Li P, Wang J, Yang Z. Histamine Triggers the Formation of Neutrophil Extracellular Traps via NADPH Oxidase, ERK and P38 Pathways. Vet Immunol Immunopathol (2021) 235:110234. doi: 10.1016/j.vetimm.2021.110234
30. Fonseca Z, Díaz-Godínez C, Mora N, Alemán OR, Uribe-Querol E, Carrero JC, et al. Entamoeba Histolytica Induce Signaling via Raf/MEK/ERK for Neutrophil Extracellular Trap (NET) Formation. Front Cell Infect Microbiol (2018) 8:226. doi: 10.3389/fcimb.2018.00226
31. Leshner M, Wang S, Lewis C, Zheng H, Chen XA, Santy L, et al. PAD4 Mediated Histone Hypercitrullination Induces Heterochromatin Decondensation and Chromatin Unfolding to Form Neutrophil Extracellular Trap-Like Structures. Front Immunol (2012) 3:307. doi: 10.3389/fimmu.2012.00307
32. Nakashima K, Hagiwara T, Yamada M. Nuclear Localization of Peptidylarginine Deiminase V and Histone Deimination in Granulocytes. J Biol Chem (2002) 277:49562–8. doi: 10.1074/jbc.M208795200
33. Wang Y, Li M, Stadler S, Correll S, Li P, Wang D, et al. Histone Hypercitrullination Mediates Chromatin Decondensation and Neutrophil Extracellular Trap Formation. J Cell Biol (2009) 184:205–13. doi: 10.1083/jcb.200806072
34. Metzler KD, Goosmann C, Lubojemska A, Zychlinsky A. Papayannopoulos V. A Myeloperoxidase-Containing Complex Regulates Neutrophil Elastase Release and Actin Dynamics During NETosis. Cell Rep (2014) 8:883–96. doi: 10.1016/j.celrep.2014.06.044
35. Papayannopoulos V, Metzler KD, Hakkim A, Zychlinsky A. Neutrophil Elastase and Myeloperoxidase Regulate the Formation of Neutrophil Extracellular Traps. J Cell Biol (2010) 191:677–91. doi: 10.1083/jcb.201006052
36. Metzler KD, Fuchs TA, Nauseef WM, Reumaux D, Roesler J, Schulze I, et al. Myeloperoxidase Is Required for Neutrophil Extracellular Trap Formation : Implications for Innate Immunity. Blood (2011) 117:953–60. doi: 10.1182/blood-2010-06-290171
37. Sollberger G, Choidas A, Burn GL, Habenberger P, Di Lucrezia R, Kordes S, et al. Gasdermin D Plays a Vital Role in the Generation of Neutrophil Extracellular Traps. Sci Immunol (2018) 3:eaar6689. doi: 10.1126/sciimmunol.aar6689
38. Chen KW, Monteleone M, Boucher D, Sollberger G, Ramnath D, Condon ND, et al. Noncanonical Inflammasome Signaling Elicits Gasdermin D-Dependent Neutrophil Extracellular Traps. Sci Immunol (2018) 3:eaar6676. doi: 10.1126/sciimmunol.aar6676
39. Pieterse E, Rother N, Yanginlar C, Hilbrands LB, van der Vlag J. Neutrophils Discriminate Between Lipopolysaccharides of Different Bacterial Sources and Selectively Release Neutrophil Extracellular Traps. Front Immunol (2016) 7:484. doi: 10.3389/fimmu.2016.00484
40. Yuen J, Pluthero FG, Douda DN, Riedl M, Cherry A, Ulanova M, et al. NETosing Neutrophils Activate Complement Both on Their Own NETs and Bacteria via Alternative and Non-Alternative Pathways. Front Immunol (2016) 7:137. doi: 10.3389/fimmu.2016.00137
41. Skopelja-Gardner S, Theprungsirikul J, Lewis KA, Hammond JH, Carlson KM, Hazlett HF, et al. Regulation of Pseudomonas Aeruginosa-Mediated Neutrophil Extracellular Traps. Front Immunol (2019) 10:1670. doi: 10.3389/fimmu.2019.01670
42. Saitoh T, Komano J, Saitoh Y, Misawa T, Takahama M, Kozaki T, et al. Neutrophil Extracellular Traps Mediate a Host Defense Response to Human Immunodeficiency Virus-1. Cell Host Microbe (2012) 12:109–16. doi: 10.1016/j.chom.2012.05.015
43. Hu S, Liu X, Gao Y, Zhou R, Wei M, Dong J, et al. Hepatitis B Virus Inhibits Neutrophil Extracellular Trap Release by Modulating Reactive Oxygen Species Production and Autophagy. J Immunol (2019) 202:805–15. doi: 10.4049/jimmunol.1800871
44. Hiroki CH, Toller-Kawahisa JE, Fumagalli MJ, Colon DF, Figueiredo LTM, Fonseca BALD, et al. Neutrophil Extracellular Traps Effectively Control Acute Chikungunya Virus Infection. Front Immunol (2020) 10:3108. doi: 10.3389/fimmu.2019.03108
45. Rocha JDB, Nascimento MTC, Decote-Ricardo D, Côrte-Real S, Morrot A, Heise N, et al. Capsular Polysaccharides From Cryptococcus Neoformans Modulate Production of Neutrophil Extracellular Traps (NETs) by Human Neutrophils. Sci Rep (2015) 5:8008. doi: 10.1038/srep08008
46. Mejía SP, Cano LE, López JA, Hernandez O, González Á. Human Neutrophils Produce Extracellular Traps Against Paracoccidioides Brasiliensis. Microbiol (United Kingdom) (2015) 161:1008–17. doi: 10.1099/mic.0.000059
47. Röhm M, Grimm MJ, D’Auria AC, Almyroudis NG, Segal BH, Urban CF. NADPH Oxidase Promotes Neutrophil Extracellular Trap Formation in Pulmonary Aspergillosis. Infect Immun (2014) 82:1766–77. doi: 10.1128/IAI.00096-14
48. Mendez J, Sun D, Tuo W, Xiao Z. Bovine Neutrophils Form Extracellular Traps in Response to the Gastrointestinal Parasite Ostertagia Ostertagi. Sci Rep (2018) 8:17598. doi: 10.1038/s41598-018-36070-3
49. Muñoz-Caro T, Lendner M, Daugschies A, Hermosilla C, Taubert A. NADPH Oxidase, MPO, NE, ERK1/2, P38 MAPK and Ca2+influx Are Essential for Cryptosporidium Parvum-Induced NET Formation. Dev Comp Immunol (2015) 52:245–54. doi: 10.1016/j.dci.2015.05.007
50. Muñoz-Caro T, Huertas SJM, Conejeros I, Alarcón P, Hidalgo MA, Burgos RA, et al. Eimeria Bovis-Triggered Neutrophil Extracellular Trap Formation Is Cd11b-, ERK 1/2-, P38 MAP Kinase- and Soce-Dependent. Vet Res (2015) 46:23. doi: 10.1186/s13567-015-0155-6
51. Muñoz-Caro T, Rubio R MC, Silva LMR, Magdowski G, Gärtner U, McNeilly TN, et al. Leucocyte-Derived Extracellular Trap Formation Significantly Contributes to Haemonchus Contortus Larval Entrapment. Parasites Vectors (2015) 8:607. doi: 10.1186/s13071-015-1219-1
52. Douda DN, Khan MA, Grasemann H, Palaniyar N. SK3 Channel and Mitochondrial ROS Mediate NADPH Oxidase-Independent NETosis Induced by Calcium Influx. Proc Natl Acad Sci (2015) 112:2817–22. doi: 10.1073/pnas.1414055112
53. Parker H, Dragunow M, Hampton MB, Kettle AJ, Winterbourn CC. Requirements for NADPH Oxidase and Myeloperoxidase in Neutrophil Extracellular Trap Formation Differ Depending on the Stimulus. J Leukoc Biol (2012) 92:841–9. doi: 10.1189/jlb.1211601
54. Alarcón P, Manosalva C, Quiroga J, Belmar I, Álvarez K, Díaz G, et al. Oleic and Linoleic Acids Induce the Release of Neutrophil Extracellular Traps via Pannexin 1-Dependent ATP Release and P2X1 Receptor Activation. Front Vet Sci (2020) 7:260. doi: 10.3389/fvets.2020.00260
55. Arai Y, Nishinaka Y, Arai T, Morita M, Mizugishi K, Adachi S, et al. Uric Acid Induces NADPH Oxidase-Independent Neutrophil Extracellular Trap Formation. Biochem Biophys Res Commun (2014) 443:556–61. doi: 10.1016/j.bbrc.2013.12.007
56. Tatsiy O, Mayer TZ, de Carvalho Oliveira V, Sylvain-Prévost S, Isabel M, Dubois CM, et al. Cytokine Production and NET Formation by Monosodium Urate-Activated Human Neutrophils Involves Early and Late Events, and Requires Upstream TAK1 and Syk. Front Immunol (2019) 10:2996. doi: 10.3389/fimmu.2019.02996
57. Pilsczek FH, Salina D, Poon KKH, Fahey C, Yipp BG, Sibley CD, et al. A Novel Mechanism of Rapid Nuclear Neutrophil Extracellular Trap Formation in Response to Staphylococcus Aureus. J Immunol (2010) 185:7413–25. doi: 10.4049/jimmunol.1000675
58. Byrd AS, O’Brien XM, Johnson CM, Lavigne LM, Reichner JS. An Extracellular Matrix–Based Mechanism of Rapid Neutrophil Extracellular Trap Formation in Response to Candida Albicans. J Immunol (2013) 190:4136–48. doi: 10.4049/jimmunol.1202671
59. Garishah FM, Rother N, Riswari SF, Alisjahbana B, Overheul GJ, van Rij RP, et al. Neutrophil Extracellular Traps in Dengue Are Mainly Generated NOX-Independently. Front Immunol (2021) 12:629167. doi: 10.3389/fimmu.2021.629167
60. Alarcón P, Manosalva C, Conejeros I, Carretta MD, Muñoz-Caro T, Silva LMR, et al. D(-) Lactic Acid-Induced Adhesion of Bovine Neutrophils Onto Endothelial Cells Is Dependent on Neutrophils Extracellular Traps Formation and CD11b Expression. Front Immunol (2017) 8:975. doi: 10.3389/fimmu.2017.00975
61. Díaz-Godínez C, Fonseca Z, Néquiz M, Laclette JP, Rosales C, Carrero JC. Entamoeba Histolytica Trophozoites Induce a Rapid Non-Classical NETosis Mechanism Independent of NOX2-Derived Reactive Oxygen Species and PAD4 Activity. Front Cell Infect Microbiol (2018) 8:184. doi: 10.3389/fcimb.2018.00184
62. Claushuis TAM, van der Donk LEH, Luitse AL, van Veen HA, van der Wel NN, van Vught LA, et al. Role of Peptidylarginine Deiminase 4 in Neutrophil Extracellular Trap Formation and Host Defense During Klebsiella Pneumoniae– Induced Pneumonia-Derived Sepsis. J Immunol (2018) 201:1241–52. doi: 10.4049/jimmunol.1800314
63. Quiroga J, Alarcón P, Manosalva C, Taubert A, Hermosilla C, Hidalgo MA, et al. Mitochondria-Derived ATP Participates in the Formation of Neutrophil Extracellular Traps Induced by Platelet-Activating Factor Through Purinergic Signaling in Cows. Dev Comp Immunol (2020) 113:103768. doi: 10.1016/j.dci.2020.103768
64. Sofoluwe A, Bacchetta M, Badaoui M, Kwak BR, Chanson M. ATP Amplifies NADPH-Dependent and -Independent Neutrophil Extracellular Trap Formation. Sci Rep (2019) 9:16556. doi: 10.1038/s41598-019-53058-9
65. Keshari RS, Jyoti A, Kumar S, Dubey M, Verma A, Srinag BS, et al. Neutrophil Extracellular Traps Contain Mitochondrial as Well as Nuclear DNA and Exhibit Inflammatory Potential. Cytom Part A (2012) 81:A:238–47. doi: 10.1002/cyto.a.21178
66. McIlroy DJ, Jarnicki AG, Au GG, Lott N, Smith DW, Hansbro PM, et al. Mitochondrial DNA Neutrophil Extracellular Traps Are Formed After Trauma and Subsequent Surgery. J Crit Care (2014) 29:1133.e1–1133.e5. doi: 10.1016/j.jcrc.2014.07.013
67. Stojkov D, Amini P, Oberson K, Sokollik C, Duppenthaler A, Simon HU, et al. ROS and Glutathionylation Balance Cytoskeletal Dynamics in Neutrophil Extracellular Trap Formation. J Cell Biol (2017) 216:4073–90. doi: 10.1083/jcb.201611168
68. Yang H, Biermann MH, Brauner JM, Liu Y, Zhao Y, Herrmann M. New Insights Into Neutrophil Extracellular Traps: Mechanisms of Formation and Role in Inflammation. Front Immunol (2016) 7:302. doi: 10.3389/fimmu.2016.00302
69. Yousefi S, Mihalache C, Kozlowski E, Schmid I, Simon HU. Viable Neutrophils Release Mitochondrial DNA to Form Neutrophil Extracellular Traps. Cell Death Differ (2009) 16:1438–44. doi: 10.1038/cdd.2009.96
70. Douda DN, Yip L, Khan MA, Grasemann H, Palaniyar N. To the Editor: Akt Is Essential to Induce NADPH-Dependent NETosis and to Switch the Neutrophil Death to Apoptosis. Blood (2014) 123:597–600. doi: 10.1182/blood-2013-09-526707
71. Behnen M, Leschczyk C, Möller S, Batel T, Klinger M, Solbach W, et al. Immobilized Immune Complexes Induce Neutrophil Extracellular Trap Release by Human Neutrophil Granulocytes via FcγRIIIB and Mac-1. J Immunol (2014) 193:1954–65. doi: 10.4049/jimmunol.1400478
72. Keshari RS, Verma A, Barthwal MK, Dikshit M. Reactive Oxygen Species-Induced Activation of ERK and P38 MAPK Mediates PMA-Induced NETs Release From Human Neutrophils. J Cell Biochem (2013) 114:532–40. doi: 10.1002/jcb.24391
73. McInturff AM, Cody MJ, Elliott EA, Glenn JW, Rowley JW, Rondina MT, et al. Mammalian Target of Rapamycin Regulates Neutrophil Extracellular Trap Formation via Induction of Hypoxia-Inducible Factor 1 α. Blood (2012) 120:3118–25. doi: 10.1182/blood-2012-01-405993
74. Kim J, Tchernyshyov I, Semenza GL, Dang CV. HIF-1-Mediated Expression of Pyruvate Dehydrogenase Kinase: A Metabolic Switch Required for Cellular Adaptation to Hypoxia. Cell Metab (2006) 3:177–85. doi: 10.1016/j.cmet.2006.02.002
75. Semenza GL, Roth PH, Fang HM, Wang GL. Transcriptional Regulation of Genes Encoding Glycolytic Enzymes by Hypoxia-Inducible Factor 1. J Biol Chem (1994) 269:23757–63. doi: 10.1016/S0021-9258(17)31580-6
76. Azevedo EP, Rochael NC, Guimarães-Costa AB, De Souza-Vieira TS, Ganilho J, Saraiva EM, et al. A Metabolic Shift Toward Pentose Phosphate Pathway Is Necessary for Amyloid Fibril- and Phorbol 12-Myristate 13-Acetate-Induced Neutrophil Extracellular Trap (NET) Formation. J Biol Chem (2015) 290:22174–83. doi: 10.1074/jbc.M115.640094
77. Amini P, Stojkov D, Felser A, Jackson CB, Courage C, Schaller A, et al. Neutrophil Extracellular Trap Formation Requires OPA1-Dependent Glycolytic ATP Production. Nat Commun (2018) 9:2958. doi: 10.1038/s41467-018-05387-y
78. Zhou E, Conejeros I, Gärtner U, Mazurek S, Hermosilla C, Taubert A. Metabolic Requirements of Besnoitia Besnoiti Tachyzoite-Triggered NETosis. Parasitol Res (2020) 119:545–57. doi: 10.1007/s00436-019-06543-z
79. Guglani L. Changing the Paradigm - Treating the Basic Defect in Cystic Fibrosis. Indian J Pediatr (2015) 82:727–36. doi: 10.1007/s12098-015-1786-3
80. Law SM, Gray RD. Neutrophil Extracellular Traps and the Dysfunctional Innate Immune Response of Cystic Fibrosis Lung Disease: A Review. J Inflamm (2017) 14:29. doi: 10.1186/s12950-017-0176-1
81. Martínez-Alemán SR, Campos-García L, Palma-Nicolas JP, Hernández-Bello R, González GM, Sánchez-González A. Understanding the Entanglement: Neutrophil Extracellular Traps (NETs) in Cystic Fibrosis. Front Cell Infect Microbiol (2017) 7:104. doi: 10.3389/fcimb.2017.00104
82. Kirchner KK, Wagener JS, Khan TZ, Copenhaver SC, Accurso FJ. Increased DNA Levels in Bronchoalveolar Lavage Fluid Obtained From Infants With Cystic Fibrosis. Am J Respir Crit Care Med (1996) 154:1426–9. doi: 10.1164/ajrccm.154.5.8912759
83. Ratjen F, Paul K, van Koningsbruggen S, Breitenstein S, Rietschel E, Nikolaizik W. DNA Concentrations in BAL Fluid of Cystic Fibrosis Patients With Early Lung Disease: Influence of Treatment With Dornase Alpha. Pediatr Pulmonol (2005) 39:1–4. doi: 10.1002/ppul.20134
84. Tirouvanziam R, Gernez Y, Conrad CK, Moss RB, Schrijver I, Dunn CE, et al. Profound Functional and Signaling Changes in Viable Inflammatory Neutrophils Homing to Cystic Fibrosis Airways. Proc Natl Acad Sci USA (2008) 105:4335–9. doi: 10.1073/pnas.0712386105
85. Makam M, Diaz D, Laval J, Gernez Y, Conrad CK, Dunn CE, et al. Activation of Critical, Host-Induced, Metabolic and Stress Pathways Marks Neutrophil Entry Into Cystic Fibrosis Lungs. Proc Natl Acad Sci (2009) 106:5779–83. doi: 10.1073/pnas.0813410106
86. Laval J, Touhami J, Herzenberg LA, Conrad C, Taylor N, Battini J-L, et al. Metabolic Adaptation of Neutrophils in Cystic Fibrosis Airways Involves Distinct Shifts in Nutrient Transporter Expression. J Immunol (2013) 190:6043–50. doi: 10.4049/jimmunol.1201755
87. Baker EH, Clark N, Brennan AL, Fisher DA, Gyi KM, Hodson ME, et al. Hyperglycemia and Cystic Fibrosis Alter Respiratory Fluid Glucose Concentrations Estimated by Breath Condensate Analysis. J Appl Physiol (2007) 102:1969–75. doi: 10.1152/japplphysiol.01425.2006
88. Forrest OA, Chopyk DM, Gernez Y, Brown MR, Conrad CK, Moss RB, et al. Resistin Is Elevated in Cystic Fibrosis Sputum and Correlates Negatively With Lung Function. J Cyst Fibros (2019) 18:64–70. doi: 10.1016/j.jcf.2018.05.018
89. Park HK, Kwak MK, Kim HJ, Ahima RS. Linking Resistin, Inflammation, and Cardiometabolic Diseases. Korean J Intern Med (2017) 32:239–47. doi: 10.3904/kjim.2016.229
90. Miller L, Singbartl K, Chroneos ZC, Ruiz-Velasco V, Lang CH, Bonavia A. Resistin Directly Inhibits Bacterial Killing in Neutrophils. Intensive Care Med Exp (2019) 7:30. doi: 10.1186/s40635-019-0257-y
91. Marcos V, Zhou Z, Yildirim AÖ, Bohla A, Hector A, Vitkov L, et al. CXCR2 Mediates NADPH Oxidase–Independent Neutrophil Extracellular Trap Formation in Cystic Fibrosis Airway Inflammation. Nat Med (2010) 16:1018–23. doi: 10.1038/nm.2209
92. Singh D, Agusti A, Anzueto A, Barnes PJ, Bourbeau J, Celli BR, et al. Global Strategy for the Diagnosis, Management, and Prevention of Chronic Obstructive Lung Disease: The GOLD Science Committee Report 2019. Eur Respir J (2019) 53:1900164. doi: 10.1183/13993003.00164-2019
93. Barnes PJ, Burney PGJ, Silverman EK, Celli BR, Vestbo J, Wedzicha JA, et al. Chronic Obstructive Pulmonary Disease. Nat Rev Dis Prim (2015) 1:15076. doi: 10.1038/nrdp.2015.76
94. Quint JK, Wedzicha JA. The Neutrophil in Chronic Obstructive Pulmonary Disease. J Allergy Clin Immunol (2007) 119:1065–71. doi: 10.1016/j.jaci.2006.12.640
95. Di Stefano A, Caramori G, Gnemmi I, Contoli M, Bristot L, Capelli A, et al. Association of Increased CCL5 and CXCL7 Chemokine Expression With Neutrophil Activation in Severe Stable COPD. Thorax (2009) 64:968–75. doi: 10.1136/thx.2009.113647
96. Walton G, Stockley J, Griffiths D, Sadhra C, Purvis T, Sapey E. Repurposing Treatments to Enhance Innate Immunity. Can Statins Improve Neutrophil Functions and Clinical Outcomes in COPD? J Clin Med (2016) 5:89. doi: 10.3390/jcm5100089
97. Pedersen F, Marwitz S, Holz O, Kirsten A, Bahmer T, Waschki B, et al. Neutrophil Extracellular Trap Formation and Extracellular DNA in Sputum of Stable COPD Patients. Respir Med (2015) 109:1360–2. doi: 10.1016/j.rmed.2015.08.008
98. Grabcanovic-Musija F, Obermayer A, Stoiber W, Krautgartner W-D, Steinbacher P, Winterberg N, et al. Neutrophil Extracellular Trap (NET) Formation Characterises Stable and Exacerbated COPD and Correlates With Airflow Limitation. Respir Res (2015) 16:59. doi: 10.1186/s12931-015-0221-7
99. Dicker AJ, Crichton ML, Pumphrey EG, Cassidy AJ, Suarez-Cuartin G, Sibila O, et al. Neutrophil Extracellular Traps Are Associated With Disease Severity and Microbiota Diversity in Patients With Chronic Obstructive Pulmonary Disease. J Allergy Clin Immunol (2018) 141:117–27. doi: 10.1016/j.jaci.2017.04.022
100. Wright TK, Gibson PG, Simpson JL, McDonald VM, Wood LG, Baines KJ. Neutrophil Extracellular Traps Are Associated With Inflammation in Chronic Airway Disease. Respirology (2016) 21:467–75. doi: 10.1111/resp.12730
101. Lugli EB, Correia RESM, Fischer R, Lundberg K, Bracke KR, Montgomery AB, et al. Expression of Citrulline and Homocitrulline Residues in the Lungs of Non-Smokers and Smokers: Implications for Autoimmunity in Rheumatoid Arthritis. Arthritis Res Ther (2015) 17:9. doi: 10.1186/s13075-015-0520-x
102. Uddin M, Watz H, Malmgren A, Pedersen F. NETopathic Inflammation in Chronic Obstructive Pulmonary Disease and Severe Asthma. Front Immunol (2019) 10:47. doi: 10.3389/fimmu.2019.00047
103. Reidel B, Radicioni G, Clapp PW, Ford AA, Abdelwahab S, Rebuli ME, et al. Kesimer M. E-Cigarette Use Causes a Unique Innate Immune Response in the Lung, Involving Increased Neutrophilic Activation and Altered Mucin Secretion. Am J Respir Crit Care Med (2018) 197:492–501. doi: 10.1164/rccm.201708-1590OC
104. Zhou W, Cao L, Jeffries J, Zhu X, Staiger CJ, Deng Q. Neutrophil-Specific Knockout Demonstrates a Role for Mitochondria in Regulating Neutrophil Motility in Zebrafish. Dis Model Mech (2018) 11:dmm033027. doi: 10.1242/dmm.033027
105. Bao Y, Ledderose C, Graf AF, Brix B, Birsak T, Lee A, et al. mTOR and Differential Activation of Mitochondria Orchestrate Neutrophil Chemotaxis. J Cell Biol (2015) 210:1153–64. doi: 10.1083/jcb.201503066
106. Mallia P, Webber J, Gill SK, Trujillo-Torralbo M-B, Calderazzo MA, Finney L, et al. Role of Airway Glucose in Bacterial Infections in Patients With Chronic Obstructive Pulmonary Disease. J Allergy Clin Immunol (2018) 142:815–23.e6. doi: 10.1016/j.jaci.2017.10.017
107. Trivedi A, Khan MA, Bade G, Talwar A. Orchestration of Neutrophil Extracellular Traps (Nets), a Unique Innate Immune Function During Chronic Obstructive Pulmonary Disease (COPD) Development. Biomedicines (2021) 9:1–25. doi: 10.3390/biomedicines9010053
108. Chen F, Yu M, Zhong Y, Wang L, Huang H. Characteristics and Role of Neutrophil Extracellular Traps in Asthma. Inflammation (2022) 45:6–13. doi: 10.1007/s10753-021-01526-8
109. Soriano JB, Abajobir AA, Abate KH, Abera SF, Agrawal A, Ahmed MB, et al. Global, Regional, and National Deaths, Prevalence, Disability-Adjusted Life Years, and Years Lived With Disability for Chronic Obstructive Pulmonary Disease and Asthma, 1990-2015: A Systematic Analysis for the Global Burden of Disease Study 2015. Lancet Respir Med (2017) 5:691–706. doi: 10.1016/S2213-2600(17)30293-X
110. Porto BN, Stein RT. Neutrophil Extracellular Traps in Pulmonary Diseases: Too Much of a Good Thing? Front Immunol (2016) 7:311. doi: 10.3389/fimmu.2016.00311
111. Bateman ED, Hurd SS, Barnes PJ, Bousquet J, Drazen JM, FitzGeralde M, et al. Global Strategy for Asthma Management and Prevention: GINA Executive Summary. Eur Respir J (2008) 31:143–78. doi: 10.1183/09031936.00138707
112. Wenzel SE. Asthma Phenotypes: The Evolution From Clinical to Molecular Approaches. Nat Med (2012) 18:716–25. doi: 10.1038/nm.2678
113. Kuruvilla ME, Lee FEH, Lee GB. Understanding Asthma Phenotypes, Endotypes, and Mechanisms of Disease. Clin Rev Allergy Immunol (2019) 56:219. doi: 10.1007/S12016-018-8712-1
114. Ray A, Kolls JK. Neutrophilic Inflammation in Asthma and Association With Disease Severity. Trends Immunol (2017) 38:942–54. doi: 10.1016/j.it.2017.07.003
115. Moore WC, Hastie AT, Li X, Li H, Busse WW, Jarjour NN, et al. Sputum Neutrophil Counts Are Associated With More Severe Asthma Phenotypes Using Cluster Analysis. J Allergy Clin Immunol (2014) 133:1557. doi: 10.1016/j.jaci.2013.10.011
116. Sur S, Crotty TB, Kephart GM, Hyma BA, Colby TV, Reed CE, et al. Sudden-Onset Fatal Asthma. A Distinct Entity With Few Eosinophils and Relatively More Neutrophils in the Airway Submucosa? Am Rev Respir Dis (1993) 148:713–9. doi: 10.1164/ajrccm/148.3.713
117. Bantulà M, Roca-Ferrer J, Arismendi E, Picado C. Asthma and Obesity: Two Diseases on the Rise and Bridged by Inflammation. J Clin Med (2021) 10:169. doi: 10.3390/jcm10020169
118. Tiotiu A, Ioan I, Wirth N, Romero-Fernandez R, González-Barcala F-J. The Impact of Tobacco Smoking on Adult Asthma Outcomes. Int J Environ Res Public Health (2021) 18:992. doi: 10.3390/ijerph18030992
119. Fernandes PD, Landgraf RG, Britto LRG, Jancar S. Production of Nitric Oxide by Airways Neutrophils in the Initial Phase of Murine Asthma. Int Immunopharmacol (2007) 7:96–102. doi: 10.1016/j.intimp.2006.09.004
120. Toussaint M, Jackson DJ, Swieboda D, Guedán A, Tsourouktsoglou T-D, Ching YM, et al. Host DNA Released by NETosis Promotes Rhinovirus-Induced Type-2 Allergic Asthma Exacerbation. Nat Med (2017) 23:681–91. doi: 10.1038/nm.4332
121. Radermecker C, Sabatel C, Vanwinge C, Ruscitti C, Maréchal P, Perin F, et al. Locally Instructed CXCR4hi Neutrophils Trigger Environment-Driven Allergic Asthma Through the Release of Neutrophil Extracellular Traps. Nat Immunol (2019) 20:1444. doi: 10.1038/S41590-019-0496-9
122. Weng Q, Zhu C, Zheng K, Wu Y, Dong L, Wu Y, et al. Early Recruited Neutrophils Promote Asthmatic Inflammation Exacerbation by Release of Neutrophil Elastase. Cell Immunol (2020) 352:104101. doi: 10.1016/j.cellimm.2020.104101
123. Li W-X, Wang F, Zhu Y-Q, Zhang L-M, Zhang Z-H, Wang X-M. Inhibitors of Nitric Oxide Synthase Can Reduce Extracellular Traps From Neutrophils in Asthmatic Children In Vitro. Pediatr Pulmonol (2020) 55:68–75. doi: 10.1002/ppul.24520
124. Lachowicz-Scroggins ME, Dunican EM, Charbit AR, Raymond W, Looney MR, Peters MC, et al. Extracellular DNA, Neutrophil Extracellular Traps, and Inflammasome Activation in Severe Asthma. Am J Respir Crit Care Med (2019) 199:1076–85. doi: 10.1164/rccm.201810-1869OC
125. Granger V, Taillé C, Roach D, Letuvé S, Dupin C, Hamidi F, et al. Circulating Neutrophil and Eosinophil Extracellular Traps Are Markers of Severe Asthma. Allergy (2020) 75:699–702. doi: 10.1111/all.14059
126. Pham DL, Ban G-Y, Kim S-H, Shin YS, Ye Y-M, Chwae Y-J, et al. Neutrophil Autophagy and Extracellular DNA Traps Contribute to Airway Inflammation in Severe Asthma. Clin Exp Allergy (2017) 47:57–70. doi: 10.1111/cea.12859
127. Saffarzadeh M, Juenemann C, Queisser MA, Lochnit G, Barreto G, Galuska SP, et al. Neutrophil Extracellular Traps Directly Induce Epithelial and Endothelial Cell Death: A Predominant Role of Histones. PloS One (2012) 7:e32366. doi: 10.1371/journal.pone.0032366
128. Li Y, Yang Y, Gan T, Zhou J, Hu F, Hao N, et al. Extracellular RNAs From Lung Cancer Cells Activate Epithelial Cells and Induce Neutrophil Extracellular Traps. Int J Oncol (2019) 55:69–80. doi: 10.3892/ijo.2019.4808
129. Narasaraju T, Yang E, Samy RP, Ng HH, Poh WP, Liew AA, et al. Excessive Neutrophils and Neutrophil Extracellular Traps Contribute to Acute Lung Injury of Influenza Pneumonitis. Am J Pathol (2011) 179:199–210. doi: 10.1016/j.ajpath.2011.03.013
130. Twaddell SH, Baines KJ, Grainge C, Gibson PG. The Emerging Role of Neutrophil Extracellular Traps in Respiratory Disease. Chest (2019) 156:774–82. doi: 10.1016/j.chest.2019.06.012
131. Fu Z, Akula S, Thorpe M, Hellman L. Potent and Broad But Not Unselective Cleavage of Cytokines and Chemokines by Human Neutrophil Elastase and Proteinase 3. Int J Mol Sci (2020) 21:651. doi: 10.3390/ijms21020651
132. Kelly RS, Dahlin A, McGeachie MJ, Qiu W, Sordillo J, Wan ES, et al. Asthma Metabolomics and the Potential for Integrative Omics in Research and the Clinic. Chest (2017) 151:262–77. doi: 10.1016/j.chest.2016.10.008
133. Licari A, Castagnoli R, Brambilla I, Marseglia A, Tosca MA, Marseglia GL, et al. Asthma Endotyping and Biomarkers in Childhood Asthma. Pediatr Allergy Immunol Pulmonol (2018) 31:44–55. doi: 10.1089/ped.2018.0886
134. Pité H, Morais-Almeida M, Rocha SM. Metabolomics in Asthma. Curr Opin Pulm Med (2018) 24:94–103. doi: 10.1097/MCP.0000000000000437
135. Snowden S, Dahlén S-E, Wheelock CE. Application of Metabolomics Approaches to the Study of Respiratory Diseases. Bioanalysis (2012) 4:2265–90. doi: 10.4155/bio.12.218
136. Bazzano M, Laghi L, Zhu C, Magi GE, Serri E, Spaterna A, et al. Metabolomics of Tracheal Wash Samples and Exhaled Breath Condensates in Healthy Horses and Horses Affected by Equine Asthma. J Breath Res (2018) 12:046015. doi: 10.1088/1752-7163/aade13
137. Albornoz A, Alarcon P, Morales N, Uberti B, Henriquez C, Manosalva C, et al. Metabolomics Analysis of Bronchoalveolar Lavage Fluid Samples in Horses With Naturally-Occurring Asthma and Experimentally-Induced Airway Inflammation. Res Vet Sci (2020) 133:276–82. doi: 10.1016/j.rvsc.2020.09.033
138. Uberti B, Morán G. Role of Neutrophils in Equine Asthma. Anim Heal Res Rev (2018) 19:65–73. doi: 10.1017/S146625231800004X
139. Davis KU, Sheats MK. The Role of Neutrophils in the Pathophysiology of Asthma in Humans and Horses. Inflammation (2021) 44:450–65. doi: 10.1007/s10753-020-01362-2
140. Vargas A, Boivin R, Cano P, Murcia Y, Bazin I, Lavoie JP. Neutrophil Extracellular Traps Are Downregulated by Glucocorticosteroids in Lungs in an Equine Model of Asthma. Respir Res (2017) 18:1–11. doi: 10.1186/s12931-017-0689-4
141. Saude EJ, Skappak CD, Regush S, Cook K, Ben-Zvi A, Becker A, et al. Metabolomic Profiling of Asthma: Diagnostic Utility of Urine Nuclear Magnetic Resonance Spectroscopy. J Allergy Clin Immunol (2011) 127:757–764.e6. doi: 10.1016/j.jaci.2010.12.1077
142. Wolak JE, Esther CR, O’Connell TM. Metabolomic Analysis of Bronchoalveolar Lavage Fluid From Cystic Fibrosis Patients. Biomarkers (2009) 14:55–60. doi: 10.1080/13547500802688194
143. Williams NC. O’neill LAJ. A Role for the Krebs Cycle Intermediate Citrate in Metabolic Reprogramming in Innate Immunity and Inflammation. Front Immunol (2018) 9:141. doi: 10.3389/fimmu.2018.00141
144. Zhou P, Yang XL, Wang XG, Hu B, Zhang L, Zhang W, et al. A Pneumonia Outbreak Associated With a New Coronavirus of Probable Bat Origin. Nature (2020) 579:270–3. doi: 10.1038/s41586-020-2012-7
145. Wadman M, Couzin-Frankel J, Kaiser J, Matacic C. A Rampage Through the Body. Science (2020) 368:356–60. doi: 10.1126/science.368.6489.356
146. Huang C, Wang Y, Li X, Ren L, Zhao J, Hu Y, et al. Clinical Features of Patients Infected With 2019 Novel Coronavirus in Wuhan, China. Lancet (2020) 395:497–506. doi: 10.1016/S0140-6736(20)30183-5
147. Reusch N, De Domenico E, Bonaguro L, Schulte-Schrepping J, Baßler K, Schultze JL, et al. Neutrophils in COVID-19. Front Immunol (2021) 12:652470. doi: 10.3389/fimmu.2021.652470
148. Xie X, Shi Q, Wu P, Zhang X, Kambara H, Su J, et al. Single-Cell Transcriptome Profiling Reveals Neutrophil Heterogeneity in Homeostasis and Infection. Nat Immunol (2020) 21:1119. doi: 10.1038/S41590-020-0736-Z
149. Evrard M, Kwok IWH, Chong SZ, Teng KWW, Becht E, Chen J, et al. Developmental Analysis of Bone Marrow Neutrophils Reveals Populations Specialized in Expansion, Trafficking, and Effector Functions. Immunity (2018) 48:364–79.e8. doi: 10.1016/j.immuni.2018.02.002
150. Adrover JM, Aroca-Crevillén A, Crainiciuc G, Ostos F, Rojas-Vega Y, Rubio-Ponce A, et al. Programmed ‘Disarming’ of the Neutrophil Proteome Reduces the Magnitude of Inflammation. Nat Immunol (2020) 21:135–44. doi: 10.1038/s41590-019-0571-2
151. Chua RL, Lukassen S, Trump S, Hennig BP, Wendisch D, Pott F, et al. COVID-19 Severity Correlates With Airway Epithelium-Immune Cell Interactions Identified by Single-Cell Analysis. Nat Biotechnol (2020) 38:970–9. doi: 10.1038/s41587-020-0602-4
152. Liao M, Liu Y, Yuan J, Wen Y, Xu G, Zhao J, et al. Single-Cell Landscape of Bronchoalveolar Immune Cells in Patients With COVID-19. Nat Med (2020) 26:842–4. doi: 10.1038/S41591-020-0901-9
153. Guan W, Ni Z, Hu Y, Liang W-H, Ou C, He J, et al. Clinical Characteristics of Coronavirus Disease 2019 in China. N Engl J Med (2020) 382:1708–20. doi: 10.1056/NEJMoa2002032
154. Aschenbrenner AC, Mouktaroudi M, Krämer B, Oestreich M, Antonakos N, Nuesch-Germano M, et al. Disease Severity-Specific Neutrophil Signatures in Blood Transcriptomes Stratify COVID-19 Patients. Genome Med (2021) 13:7. doi: 10.1186/s13073-020-00823-5
155. Meizlish ML, Pine AB, Bishai JD, Goshua G, Nadelmann ER, Simonov M, et al. A Neutrophil Activation Signature Predicts Critical Illness and Mortality in COVID-19. Blood Adv (2021) 5:1164–77. doi: 10.1182/bloodadvances.2020003568
156. Barnes BJ, Adrover JM, Baxter-Stoltzfus A, Borczuk A, Cools-Lartigue J, Crawford JM, et al. Targeting Potential Drivers of COVID-19: Neutrophil Extracellular Traps. J Exp Med (2020) 217:e20200652. doi: 10.1084/jem.20200652
157. Fox SE, Akmatbekov A, Harbert JL, Li G, Quincy Brown J, Vander Heide RS. Pulmonary and Cardiac Pathology in African American Patients With COVID-19: An Autopsy Series From New Orleans. Lancet Respir Med (2020) 8:681–6. doi: 10.1016/S2213-2600(20)30243-5
158. Zuo Y, Yalavarthi S, Shi H, Gockman K, Zuo M, Madison JA, et al. Neutrophil Extracellular Traps in COVID-19. JCI Insight (2020) 5:e138999. doi: 10.1172/jci.insight.138999
159. Veras FP, Pontelli MC, Silva CM, Toller-Kawahisa JE, de Lima M, Nascimento DC, et al. SARS-CoV-2–Triggered Neutrophil Extracellular Traps Mediate COVID-19 Pathology. J Exp Med (2020) 217:e20201129. doi: 10.1084/jem.20201129
160. Tomar B, Anders H-J, Desai J, Mulay SR. Neutrophils and Neutrophil Extracellular Traps Drive Necroinflammation in COVID-19. Cells (2020) 9:1383. doi: 10.3390/cells9061383
161. Codo AC, Davanzo GG, Monteiro L de B, de Souza GF, Muraro SP, Virgilio-da-Silva JV, et al. Elevated Glucose Levels Favor SARS-CoV-2 Infection and Monocyte Response Through a HIF-1α/Glycolysis-Dependent Axis. Cell Metab (2020) 32:437–446.e5. doi: 10.1016/j.cmet.2020.07.007
162. Ayres JS. A Metabolic Handbook for the COVID-19 Pandemic. Nat Metab (2020) 2:572–85. doi: 10.1038/s42255-020-0237-2
163. Martínez-Reyes I, Chandel NS. Mitochondrial TCA Cycle Metabolites Control Physiology and Disease. Nat Commun (2020) 11:102. doi: 10.1038/s41467-019-13668-3
164. Morita M, Gravel S-P, Chénard V, Sikström K, Zheng L, Alain T, et al. Mtorc1 Controls Mitochondrial Activity and Biogenesis Through 4E-BP-Dependent Translational Regulation. Cell Metab (2013) 18:698–711. doi: 10.1016/j.cmet.2013.10.001
Keywords: neutrophils, NETs, innate immunity, cellular metabolism, airway inflammation
Citation: Morán G, Uberti B and Quiroga J (2022) Role of Cellular Metabolism in the Formation of Neutrophil Extracellular Traps in Airway Diseases. Front. Immunol. 13:850416. doi: 10.3389/fimmu.2022.850416
Received: 07 January 2022; Accepted: 18 March 2022;
Published: 12 April 2022.
Edited by:
Carlos Rodrigo Hermosilla, University of Giessen, GermanyReviewed by:
Meraj Alam Khan, University of Toronto, CanadaKim Maree O’Sullivan, Monash University, Australia
Ahmed Yaqinuddin, Alfaisal University, Saudi Arabia
Copyright © 2022 Morán, Uberti and Quiroga. This is an open-access article distributed under the terms of the Creative Commons Attribution License (CC BY). The use, distribution or reproduction in other forums is permitted, provided the original author(s) and the copyright owner(s) are credited and that the original publication in this journal is cited, in accordance with accepted academic practice. No use, distribution or reproduction is permitted which does not comply with these terms.
*Correspondence: Gabriel Morán, gmoran@uach.cl