- 1Department of Endocrinology, the First Affiliated Hospital of USTC, Division of Life Sciences and Medicine, University of Science and Technology of China, Hefei, Anhui, China
- 2Department of Ophthalmology, the First Affiliated Hospital of USTC, Division of Life Sciences and Medicine, University of Science and Technology of China, Hefei, Anhui, China
Diabetic retinopathy is one of the most common complications of diabetes mellitus and the leading cause of low vision and blindness worldwide. Mounting evidence demonstrates that inflammation is a key mechanism driving diabetes-associated retinal disturbance, yet the pathophysiological process and molecular mechanisms of inflammation underlying diabetic retinopathy are not fully understood. Cytokines, chemokines, and adhesion molecules interact with each other to form a complex molecular network that propagates the inflammatory and pathological cascade of diabetic retinopathy. Therefore, it is important to understand and elucidate inflammation-related mechanisms behind diabetic retinopathy progression. Here, we review the current understanding of the pathology and pathogenesis of inflammation in diabetic retinopathy. In addition, we also summarize the relevant clinical trials to further suggest inflammation-targeted therapeutics for prevention and management of diabetic retinopathy.
Introduction
Diabetic retinopathy (DR) is a common microvascular complication of type 1 and type 2 diabetes. DR is the leading cause of low vision and blindness in patients with diabetes and can severely affect people of all ages worldwide, with a prevalence of 34.6% (93 million) in adults aged 40 years and over (1). A systematic review focused on population-based studies estimated that DR has an annual incidence ranging from 2.2% to 12.7% (2). Epidemiological evidence suggests that DR not only increases the risk of vision impairment and blindness in diabetic patients but also increases the risk of all-cause and cardiovascular disease (CVD) mortality in a multi-ethnic Asian population (3, 4).
DR is classified as nonproliferative diabetic retinopathy (NPDR) and proliferative diabetic retinopathy (PDR) according to the modified Airlie House Classification used in the Early Treatment Diabetic Retinopathy Study (ETDRS) (5). The earliest morphological sign of NPDR is the formation of microaneurysms, in which the capillary wall expands outwards, detected by ophthalmoscopy with blot hemorrhages (6). Further signs of NPDR are changes in retinal blood flow and vascular permeability, thickening of the basement membrane, loss of pericytes, and formation of the acellular capillary. As the severity of ischemia increases, it may develop into PDR. PDR is characterized by the hallmark feature of pathologic retinal neovascularization, with vitreous hemorrhage, vitreous new blood vessels, and retinal traction detachment, which will lead to blindness (7).
Diabetic macular edema (DME) is an important additional classification in DR that is associated with ischemia because of the increased permeability of retinal capillaries and microaneurysms, resulting in extracellular fluid accumulation and normal dense macular tissue thickening. DME can arise at any stage of NPDR and PDR and threatens visual acuity (8). Overall, T1DM patients tended to develop diabetic retinopathy and PDR, while T2DM patients treated with insulin were more likely to develop DME.
The pathophysiology of DR is driven by the interaction of many factors, among which long-term episodes of hyperglycemia (elevated blood glucose levels) are an important factor in diabetic patients (9). In DR patients, elevated blood glucose levels lead to abnormal regulation of many biochemical pathways, hyperglycemia-induced increases in the flux of advanced glycation end products/receptors (AGE/RAGE), the polyol pathway, protein kinase C (PKC) activation, and the hexosamine pathway. These modifications also result in mitochondrial failure, inflammation, and hypoxia-driven vascular endothelial growth factor (VEGF) secretion, leading to vascular and neuronal apoptosis, neovascularization, and vascular permeability, respectively (10, 11). The complex aetiology of DR reflects the various treatments currently available, including laser photocoagulation, glucocorticoids, vitrectomy, and drugs that neutralize VEGF. Due to painful patient administration and long-term adverse effects, the use of argon laser and intravitreal injection therapeutic approaches is limited (12). There is interest in developing pharmacological therapies for DR management conditions, such as nonsteroidal anti-inflammatory drugs (NSAIDs) that inhibit or delete proinflammatory molecules, anti-VEGF agents, and antitumour necrosis factor α (TNF-α) agents (13). Therefore, a deeper comprehension of fundamental processes and innovative treatments in DR is needed.
In this review, we concentrate on the involvement of inflammation in the pathophysiology of DR and summarize the recent advances in current and emerging treatments for DR.
Inflammation in diabetes and diabetic retinopathy
For many years, it has been proposed that chronic tissue inflammation may play a role in metabolic illness. Chronic inflammatory problems in the peripheral and central nervous systems are caused by diabetes. A substantial amount of evidence from both patients and animal models demonstrates that DR is a chronic low-grade inflammatory illness involving inflammatory mediators.
Inflammation in diabetes
Type 1 diabetes (T1D) is a condition caused by autoimmune damage or loss of functional β cell mass. Low insulin secretion capability, self-antigen presentation, and immune-mediated destruction are hypothesized to be the results of cytokine-driven inflammation and other stress factors (14). Both CD4+ and CD8+ T cells are involved in the development of T1D and play a role in the different stages of T1D to promote the destruction of pancreatic β cells and the pathogenesis of the disease (15). These adaptive immune cells regulate the inflammatory response and destroy insulin-producing β cells by secreting proinflammatory cytokines such as TNF-α, interferon γ (IFN-γ), and interleukin-1 (IL-1) (16).
In addition to causing oxidative stress, oxygen species (ROS) can stimulate the growth of macrophages and dendritic cells by activating the nuclear factor kappa light chain enhancer (NF-κb) pathway, activator protein-1 (AP-1), and mitogen-activated protein kinase (MAPK) (17). Type I interferon (IFN) is a cytokine essential for innate and adaptive immune responses. Levels of type I interferon as well as mediated signaling were also found to be upregulated in children at high risk for T1D and in new-onset T1D patients (18). In β cells, activation of type I IFN signaling leads to high expression of major histocompatibility complex (MHC) class I, epigenetic changes, endoplasmic reticulum (ER) stress, and induction of post-transcription and post-translation modifications (19). This may result in the persistent presentation of neoantigens to the immune system and apoptosis of β cells. Innate immunity and inflammatory mediators can impact T1D, contribute to destroying pancreatic β cells and cause peripheral insulin resistance (20). The study of blocking inflammatory factors such as the IFN inhibitor golimumab in youth with new-onset T1D has achieved some success (21).
The pathogenesis of type 2 diabetes (T2D) is closely related to obesity and insulin resistance, which leads to a burden on beta cells, which eventually depletes them, leading to hyperglycemia (22). Studies have shown that people with prediabetes have an increase in several inflammatory markers, such as resistin, interleukin 6 (IL-6), TNF-α, interleukin 1β (IL-1β), and monocyte chemoattractant protein-1 (MCP-1), in their serum and fasting glucose levels (23). As the adipose tissue increases and as various metabolic pressures build up, the cytokines released by the adipose tissue “Spill over”, which can create an imbalance between cytokines that promote insulin sensitivity, including adiponectin, leptin, and proinflammatory cytokines (24). T2D frequently exhibits low-grade inflammation, and the maturation of local macrophages is crucial in controlling this process. T2D-related inflammation is characterized by an increase in macrophages in different tissues and the simultaneous production of TNF-α, IL-6, IL-1β, and interleukin 8 (IL-8) cytokines (25). The underlying mechanism of insulin resistance is also related to the inflammatory response, with activation of inflammasomes in islet inflammatory cells impairing islet function and viability. Additionally, it appears that the development of diabetic ophthalmological problems involves an inflammatory process. Inflammation is a nonspecific response to injury or stress, including various functional and molecular mediators, leukocyte recruitment, and/or activation (26). An acute inflammatory response can eliminate infectious agents, but if it persists for a long time, it may have an adverse effect.
Inflammation, immune cell modulation, survival, and proliferation are all impacted by toll-like receptors (TLRs), which are the first line of defense against pathogen invasion and identify various pathogen-associated chemical patterns (27). The hallmark of chronic inflammation is tissue filled with macrophages, lymphocytes, and mature B cells. As a result of the long-term release of inflammatory factors in the tissue, these white blood cells continue to exude from the blood vessels and eventually accumulate in the tissue (28). Inflammatory dysregulation can also lead to tissue and organ damage, which promotes disease. Inflammation is involved in the development of DR, so understanding the inflammatory process may provide new strategies for DR therapy.
Inflammation in DR
The pathophysiology of DR is complicated, and the disease’s fundamental processes are not fully understood. Figure 1 summarizes the key diabetes-related factors associated with the development of T1D, T2D, and DR. Elevated intracellular glucose levels in diabetic individuals trigger the polyol pathway, which metabolizes glucose (29). This leads to the deposition of AGEs, the activation of PKC, and the upregulation of AGE receptors and the hexokinase pathway (30). It triggers oxidative stress, which causes a rise in intracellular reactive oxygen species (ROS) and irreparable cellular damage. A hyperglycemic environment leads to metabolic dysfunction, oxidative stress, and the production of ROS, such as superoxide radicals (31). Apoptosis may be caused by mitochondrial abnormalities, hypoxia-mediated VEGF production may be increased by inflammatory stimuli, and VEGF is a crucial angiogenesis mediator (32). Studies have shown that circulating mitochondrial DNA (mtDNA) levels are associated with diabetic retinopathy, and high blood glucose-induced mtDNA changes in early diabetes may contribute to inflammation and diabetic retinopathy progression (33). Chronic inflammation can result from chronic hyperglycemia and oxidative stress, as well as other molecular mediators. Increased levels of chemokines, including MCP-1, CCL2 and CCL5, as well as proinflammatory cytokines, such as TNF-α, IL-1β, and IL-6, were found in DR (34). Activated cytokines secrete intracellular adhesion molecules, such as ICAM-1 and VCAM-1, which attract monocytes and leukocytes and promote a continuous inflammatory response (35). With the accumulation of chronic inflammation, inflammatory cells infiltrate and destroy tissues, further aggravating retinal vascular permeability, vasodilation, and retinal thickening in DR patients.
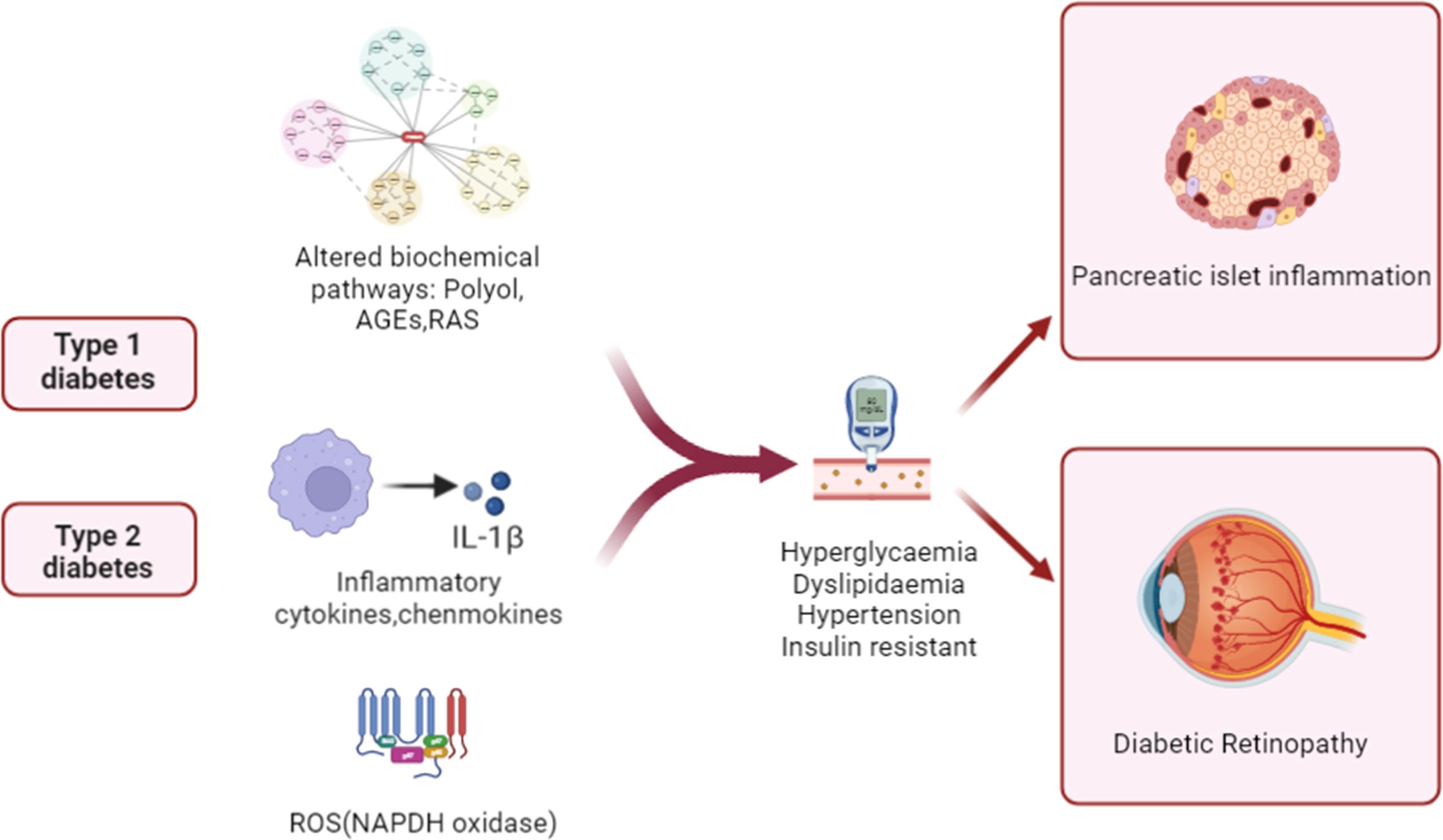
Figure 1 Schematic illustration of pathogenic mechanisms leading to pancreatic β cell damage and sight-threatening endpoints of diabetic retinopathy (DR). In patients with diabetes, inflammation, aberrant signaling of trophic factors, and biochemical pathways are upregulated. The alterations then enter the systemic circulation and contribute to diabetic pathology and islet inflammation by increasing levels of blood glucose and lipids and insulin resistance.
Inflammation plays an important role in the pathogenesis of DR. In animal models and patients with diabetes, chronic low-grade inflammation is widely found at different stages of DR (36). It has been established that leukocytosis is a crucial step in the early stages of DR and is related to adhesion molecule-mediated leucocyte-endothelial adhesion. PDR vitreous bodies also cause proinflammatory activation of endothelial cells. The nuclear translocation of the proinflammatory transcription factors NF-κB and pCREB, ROS production, disruption of endothelial barrier integrity, E-selectin, the upregulation of VCAM-1 and ICAM-1 and the increase in leukocyte adhesion were observed. Studies have found that both soluble E-selectin and SVCAM-1 levels are elevated in diabetic retinopathy patients and that CCL17, CCL19, and TGF β are significantly upregulated (37, 38). It has been reported that chemokines that regulate the attraction and activation of leukocytes have a role in the development of DR. Patients with DR had higher levels of chemokines such as MCP-1, macrophage inflammatory protein-1 alpha (MIP-1 α) and MIP-1 beta (39). In addition, retinal neuroglia dysfunction is also associated with the development and expansion of retinal inflammation in DR (40). In general, chronic inflammation in diabetes leads to the response of inflammatory cells in the body, which further affects capillary dysfunction and ultimately leads to DR. Therefore, inflammation as a fundamental cause of DR still needs further understanding to solve this problem. Figures 2A, B summarize the changes caused by chronic inflammation of DR.
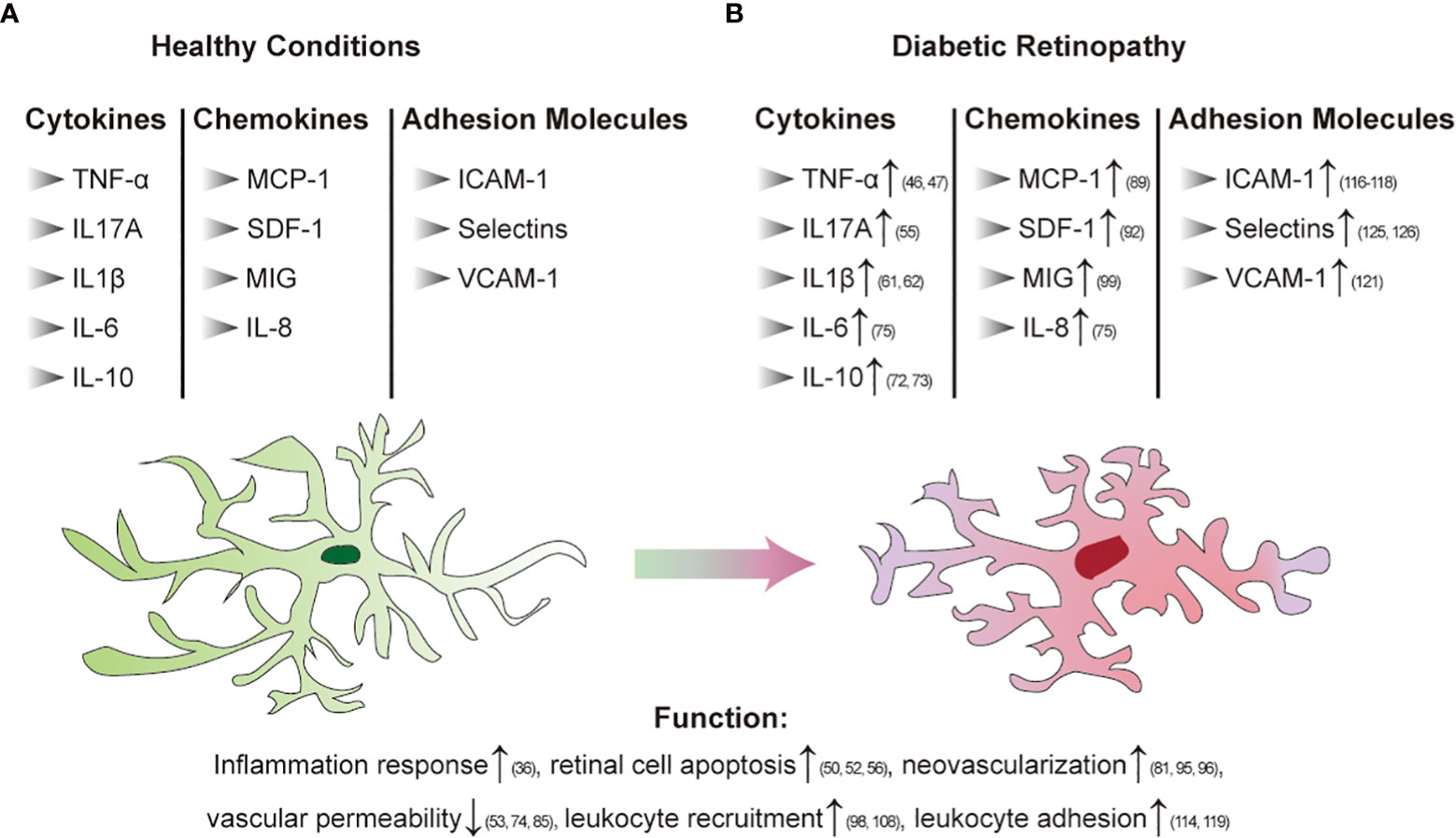
Figure 2 Immune regulation in diabetic retinopathy [(A): Healthy conditions; (B) DR]. Arrows indicate elevated levels or increased activity.
Inflammatory cytokines
Cytokines are a wide range of molecular families with different structures and individual proteins known for their many roles in the immune system. Some cytokines mediate downstream responses through the JAK/STAT signaling pathway, such as IL-6, and others activate the NF-κB signaling pathway, such as IL-1 and IL-17 (41). Some cytokines have a clear role in promoting inflammation, such as IL-1 and TNF, known as proinflammatory cytokines, and some cytokines, such as IL-4 and IL-10, suppress proinflammatory activity, called anti-inflammatory cytokines. Proinflammatory cytokines upregulate the expression of proinflammatory genes encoding enzymes that synthesize leukotrienes, platelet-activating factors, NO, and prostanoids. They also participate in inducing endothelial adhesion molecules, which are crucial for leukocyte adhesion to the surface of endothelial cells. Thus, proinflammatory cytokines induce inflammation, tissue damage, and dysfunction, while anti-inflammatory factors block this process or suppress the intensity of the inflammatory response (42).
TNF-α is a proinflammatory cytokine produced by macrophages, natural killer cells, or T cells. It acts as an inflammatory marker closely related to diabetes, which is linked to metabolic disorders, including obesity and insulin resistance (43). Diabetes causes damage to a variety of tissues associated with this cytokine. For example, in a mouse model of type 2 diabetes, the interaction between TNF-α and IL-6 led to cardiac endothelial dysfunction (44); TNF-α participates in the recruitment of monocytes and macrophages, reduces the glomerular filtration rate through hemodynamic changes, and promotes the progression of diabetic nephritis (45). TNF-α also plays an important role in diabetic retinopathy. Elevated concentrations of TNF-α have been reported in the serum, vitreous, and aqueous humor of patients with DR (46, 47), while the level of TNF-α in the serum is positively correlated with the severity of the disease (48). It can increase the expression levels of endothelial nitric oxide synthase gene and intercellular cell adhesion molecule-1 (ICAM-1) and activate nuclear actor kappa B (NF-κB).These molecules all play a role in promoting the inflammatory response in DR. Additionally, it has been reported that TNF-α inhibitors reduce the inflammatory response in DR (49). However, TNF-α induces apoptosis, thus disrupting the normal function of the blood vessel wall and affecting the vascular permeability of the retina. TNF-α, which is released from Müller cells, causes apoptosis of retinal pigment epithelial cells by activating the EGFR/p38/NF-κB/p62 pathway. In diabetic mice, blockage of the TNF-a/EGFR axis relieves blood-retina barrier breakdown (50). Moreover, TNF-α causes the loss of retinal microvascular cells and promotes DR progression (51). In general, TNF-α participates in the inflammatory response, neovascularization and vascular reactivity.
IL-17A is a proinflammatory factor produced primarily by T cells. IL-17A knockout reduces the levels of TNF-a, IFN-c and IL-1b in Akita mice, suggesting that IL-17A is strongly associated with proinflammatory cytokine-driven inflammatory responses in diabetes progression (52). IL-17A plays a major role in increasing the intensity of retinal inflammation, oxidative stress, and vascular permeability in retinal disease (53). Blocking IL-17A alleviates diabetic retinopathy in rodents (54). In in vitro culture, retinal Müller cells display elevated expression of IL-17A and its receptor IL-17RA, along with increased secretion of IL-17A under hyperglycemic conditions. Meanwhile, IL-17A induced apoptosis of Müller cells through the Act1 pathway (55). Diabetic mice with IL-17A knockout display reduced retinal microvascular damage, retinal Müller cell abnormalities, and retinal ganglion cell apoptosis, which suggests that IL-7A is positively involved in DR pathophysiology (56). Furthermore, through IL-17A/IL-17R to the Act1/FADD signaling cascade, IL-17A causes degeneration of retinal capillaries and induces apoptosis of retinal endothelial cells (52). IL-17A acts as a proinflammatory cytokine that is primarily involved in retinal cell apoptosis and is expected to be a potential clinical target for the treatment of diabetic retinopathy in the future.
IL-1β is a multifunctional cytokine that promotes inflammation. It is rarely present in the cells of healthy individuals. Some cytokines, such as TNF-α, IL-18, and IL-1, including IL-1β itself, induce the production of IL-1β (57). Unlike TNF-α-induced insulin resistance, IL-1β has a direct killing effect on islet β cells (58). The findings are that IL-1β released by macrophages after a meal can synergize with insulin to activate the inflammasome, which can promote inflammation (59). In addition, IL-1β is a major trigger of the neuroinflammatory cascade (60). IL-1β was found to be increased in the retina of rats with diabetes as well as in the serum of proliferative diabetic retinopathy patients (61, 62). Studies have shown that the inflammatory status of DR is associated with a decreased degree of tyrosine nitrosylation of IL-1β in the vitreous (63). IL-1β damages retinal capillary endothelial cells by activating NF-κB and increasing oxidative stress, which can mediate mitochondrial damage, and it accelerates this damaging process in the case of hyperglycemia (64–66). Many studies have reported the effects of blocking IL-1β: pituitary adenylate cyclase-activating peptide administration reduces levels of IL-1β in rats with DR, thus protecting retinal tissue (67); blockade of IL-1β restores islet beta cell function over a short period or even allows some islet beta cells to regenerate (57); IL-1 blockers are effective in many autoinflammatory syndromes (68), suggesting their anti-inflammatory effects; and IL-1 receptor antagonists are effective in treating many eye diseases, such as uveitis and scleritis (69). These findings may start a new avenue for the treatment of DR.
IL-10 is an anti-inflammatory cytokine that plays a protective role in DR progression. Decreased IL-10 levels led to accelerated DR development in retinas of CX3CR1-deficient mice model (70). IL-10 improves the formation of subretinal fibrosis under the induction of exogenous HSP70, which helps avoid severe vision loss (71). While IL-10 acts as an anti-inflammatory factor, several studies have reported increased levels of IL-10 in the aqueous humor and vitreous of DR patients (72, 73). In addition, studies have reported that IL-1β is inversely correlated with IL-10 in the healthy population; however, when diabetes mellitus occurs, the balance between anti-inflammatory IL-10 and proinflammatory IL-1β is broken (72). One possible reason for the increase in IL-10 levels is that when inflammation occurs, IL-10 will display higher secretory activity to offset the rise in proinflammatory cytokine levels and prevent the development of inflammation.
IL-6 acts as a multifunctional inflammatory factor whose role is associated with immunomodulation, increased vascular permeability, and stimulation of angiogenesis (74). Higher concentrations of IL-6 were found in aqueous humor and serum in DR patients (75). IL-6 plays a role through classic and trans-signaling. Classic signaling exerts an anti-inflammatory effect and has regenerative activities, while trans-signaling is thought to be associated with proinflammatory activities (76). It has been discovered that inhibiting IL-6 trans-signaling lessens the oxidative damage that diabetes mellitus causes to the retina (77) and helps to minimize vascular inflammation and endothelial barrier issues (78). Following the activation of the IL-6 trans signaling pathway, the expression of adhesion molecules such as ICAM-1, VCAM-1 and selectins is increased (79). By rearranging actin filaments and altering the morphology of endothelial cells, IL-6 improves the permeability of endothelial cells in vitro (74). In addition, IL-6 stimulates the Jak/STAT3 pathway in the eyes, thus inducing apoptosis by the downstream receptor NO (80). Another important downstream effector of the STAT3 pathway is vascular endothelial growth factor (VEGF). It mediates pathological angiogenesis and increased vascular permeability. IL-6 can support angiogenesis by inducing VEGF indirectly in the state of DR (81). Inhibition of IL-6 and selective inhibition of IL-6 trans-signaling have entered clinical trials for treating a variety of inflammatory diseases and are expected to be a therapeutic target for DR (79).
Chemokines
Chemokines are small heparin-binding proteins that can induce circulating leukocytes to move to inflammation or injury sites. According to their different structures and functions, chemokines segregate into four families, namely, CC chemokines, CXC chemokines, CX3C chemokines, and XC chemokines. The binding of chemokines to receptors activates the cascade of signals, which eventually leads to the rearrangement, shape change, and cell movement of actin (82).
MCP-1, which belongs to the CC family of chemokines, participates in the progression of vascular inflammation in DR and acts as a powerful chemokine for recruiting monocytes and macrophages (83). In the hyperglycemic state, MCP-1 is upregulated after NF-B activation, and diabetes patients may produce a significant amount of MCP-1 from Müller cells into the vitreous cavity and anterior chamber (84). By attracting monocytes, higher levels of MCP-1 in the diabetic retina affect the blood-retinal barrier and the permeability of retinal blood vessels (85). Retinal vascular ischemia is brought on by capillary blockage brought on by recruited leukocytes and macrophages adhering more strongly to the vascular endothelium (86). MCP-1 is one of the dominant causes of blindness in patients with DR. MCP-1 also exerts angiogenesis by inducing VEGF and activating RhoA (87). In addition, MCP-1 induces the activation of microglia, which release inflammatory factors, leading to the breakdown of optic vessels and damage to retinal neurons (88). Higher concentrations of MCP-1 have been found in vitreous samples of patients with DR (89); although MCP-1 primarily has an indirect impact on the progression of the illness, its importance cannot be understated.
C-X-C motif chemokine 12 (CXCL12), also known as stromal cell-derived factor 1 (SDF-1), is intimately linked to the development of type 2 diabetes and associated consequences. Patients with type 2 diabetes mellitus have increased serum levels of SDF-1, according to reports (90). Inhibition of SDF-1 in mice with diabetic nephritis reduces the severity of glomerular sclerosis and prevents proteinuria (91). Higher levels of SDF-1 have also been found in the vitreous of patients with DR, suggesting its pathogenic effect on eye lesions (92). SDF-1 promotes a firm adhesion of endothelial cells to the endothelium of the vasculature by increasing the expression of VCAM on endothelial cells; it also promotes the migration and homing of endothelial progenitor cells (93). It also acts as angiogenesis (94) with higher levels in ischemic and hypoxic retinas. It recruits endothelial progenitor cells to ischemic regions and synergize with VEGF and its receptor CXCR4 to participate in angiogenesis events (95, 96), which is one of the primary reasons why DR patients become blind. However, recent studies have reported that the SCF-1/CXCR4 pathway may improve DR by increasing cell activity, and further clinical studies are still needed to confirm this hypothesis (97).
Monokine induced by interferon-γ (MIG) is a CXC chemokine expressed in multiple cell types exposed to interferon-γ. It is usually connected to the Th1 response and directs the migration of activated lymphocytes, with an activity that inhibits angiogenesis (98). The levels of MIG increase in the vitreous of DR patients and are significantly related to VEGF (99). Compared to inactive proliferative diabetic retinopathy patients, the levels of MIG are significantly elevated in proliferative diabetic retinopathy patients with active neovascularization (100). The mechanism of action of MIG in DR has not yet been elucidated, and some studies believe that there is a positive regulatory feedback loop between MIG and VEGF, which may facilitate a regulatory angiostatic function (100). Another hypothesis is that MIG plays a role in the chemotaxis of leukocytes, rather than as an angiogenic inhibitor (74). MIG is known to have higher expression in other inflammatory diseases. MIG blockade has been described as a prospective therapeutic target for Crohn’s disease, and serum MIG levels represent the activity of the disease (101); in patients with rheumatoid arthritis, the expression of MIG is observed in the serum, synovial fluid, and synovial tissues (102). The inhibition of MIG in inflammatory diseases may present a potential therapeutic target for DR, but the feasibility remains to be checked.
IL-8 is the most well-known CXC chemokine, which has powerful proinflammatory properties resulting in its strict regulation, with low or no expression in normal tissues. Activated macrophages and monocytes release IL-8, which encourages the directed migration of basophils, neutrophils, and T cells. The eye effects of IL-8 vary depending on the site of action and the source of production, and one of its surprising effects is that it shows angiogenic activity in any part of the eye (103). Through angiogenesis and the proinflammatory response, IL-8 actively contributes to DR (104). Patients with proliferative diabetic retinopathy have significantly higher vitreous and aqueous fluid levels of IL-8 (75), which are linked to a greater amount of large-vessel gliotic obliteration in these patients (105). The increase in IL-8 in patients with poor visual prognosis after vitrectomy may damage the retina by recruiting ischemic inflammatory cells (104). Endothelial cell proliferation and inhibition of apoptosis can both be directly induced by IL-8 (106). In response to hypoxia, periretinal cells, ciliary epithelium, and glial cells release VEGF and/or IL-8, which stimulates the proliferation of endothelial cells and results in intraocular neovascularization (103). Diabetes mellitus can lead to retinopathy and hypoxia, while activation of NF-κB under hypoxia regulation increases the expression of IL-8 mRNA (107), which worsens retinopathy. Overall, elevated levels of IL-8 promote DR progression.
Fractalkine, also known as CX3CL1, is a CX3C chemokine that interacts with the specific CX3CR1 receptor on peripheral leukocytes such as microglia (108). Fractalkine has angiogenic activity both in vitro and in vivo and is substantially expressed in the vitreous of patients with proliferative diabetic retinopathy, suggesting that it may be a key factor in the progression of the disease (109). However, it has been shown recently that the absence of CX3CR1 in the DR mouse model of systemic inflammation leads to substantial perivascular clustering of proliferating microglia in regions of fibrinogen extravasation and increases the level of the proinflammatory factor IL-1β (110). Additionally, retinal ganglion cell layer neuronal cell counts in CX3CR1 deletion diabetic mice were lower, whereas microglial cell counts were higher and the microglia were more active (111). Microglia are resident monocytes in the retina. Activated microglia in DR patients release various proinflammatory mediators, including cytokines, chemokines, glutamate, and caspases, and enhance the expansion and migration of these mediators. These changes cause damage to retinal neurons, leading to blindness in patients with DR (112). While fractalkine activates the Nrf2 pathway and inhibits the NF-B pathway to deactivate microglia, this reduces the production of ROS and proinflammatory cytokines (113). In summary, the fractalkine/CX3CR1 signaling pathway plays a protective role in the diabetic retina.
Adhesion molecules
White blood cell adherence to the microvasculature is one of the early events of diabetic retinal inflammation. Leukocyte adhesion accelerates the loss of endothelial cells and disrupts the blood-retinal barrier by releasing inflammatory cytokines, growth cytokines and vascular permeability factors (114). High expression of cell adhesion molecules promotes the effect of leukocyte adhesion.
Interccellular adhesion molecule 1 (ICAM-1), which is increased in disorders such uveitis, diabetes, and age-related macular degeneration, is crucial for the migration of white blood cells (115). It is also the main adhesion molecule involved in DR. Several studies have reported high expression of ICAM-1 in the vitreous of DR patients (116–118). ICAM-1, which binds to integrins on leukocytes, is induced by TNF-α. It can regulate the adherence and migration of leukocytes, causing retinal leukostasis (119). By interacting with a number of cytokines to breakdown the blood-retinal barrier, ICAM mediates the migration of white blood cells. Serum sICAM-1, which improves the adhesion between white blood cells and the vascular endothelium, is released from the outer segment of ICAM-1 (120).
Vascular cell adhesion molecule-1 (VCAM-1), which binds to integrin, is expressed on endothelial cells (119). It is overexpressed in the diabetic fiber vascular membrane (118) and is raised in conditions of hyperglycemia or hyperlipidemia (121). TNF-α regulates the expression of VCAM-1 but has a dual effect. It reduces the level of VCAM-1 under basal conditions but promotes retinal endothelial activation in response to diabetes (121). The antiangiogenic drug conbercept has a significant inhibitory effect on VCAM-1 expression in the retina of mice with proliferative diabetic retinopathy, which prevents retinal endothelial cell proliferation (122). Although it is currently known that VCAM-1 is related to endothelial function, its function in DR is not well understood.
Elevated levels of selectin induce the aggregation of leukocytes into the endothelial wall and then lead to retinal leukostasis. It can be divided into three classes depending on the expressed cell type. L-selectin, which is primarily responsible for moving leukocytes to inflamed tissues, is expressed on circulating leukocytes (123). Only endothelial cells express E-selectin, and its expression is increased when cytokines or ROS are present (124). According to reports (125), the presence of retinopathy is associated with higher levels of soluble E-selectin. P-Selectin (126) is expressed in endothelial cells as well as platelets, and it is expressed more frequently in DR patients. This process involves endothelial barrier alteration (48).
Integrins are a large group of membrane-binding proteins with 18 α subunits and 8 β subunits in vertebrates that can form different heterodimers. The function of integrins is to allow white blood cells to pass through the blood vessel walls, and they are receptors for cell adhesion during DR development. On the membrane of leukocytes, β2 integrin binds to ICAM-1, while α4β1 and α4β7 bind to VCAM-1 on endothelial cells (127, 128). Integrins make a difference in the progression of eye diseases. As an example, integrins αVβ1 and α3β1 are involved in the infectious process of corneal tissue in allergic eye disease (129). The pathogenic process underlying glaucoma is significantly influenced by αVβ3 integrin (130, 131). For DR, it has been noted that patients with proliferative diabetic retinopathy have higher amounts of αvβ3-, α5- and αvβ5-integrins in their fibrovascular membranes (132). In the human eye, angiogenesis can be induced through two pathways of integrins: first, αvβ3 mediates angiogenesis in models of corneal or chorioallantoic angiogenesis involving TNF- and basic fibroblast growth factor; second, VEGF or transforming growth factor is mostly mediated by αvβ5 during angiogenesis (133). Additionally, diabetic retinopathy in an animal model is induced by α4 integrin/CD49d, which also facilitates leukocyte adhesion. By inhibiting the NF-B pathway, blockade of α4 integrin/CD49d can reduce vascular leakage and leukocyte adhesions (134). As mentioned before, β2 integrin binds to ICAM-1 to participate in adhesion to the blood vessel wall, induces downstream leukocyte activation and promotes inflammation. After the activation of white blood cells, it reacts to the increase in the expression of β2 integrin (135). In conclusion, integrins promote the function of other adhering molecules and cause angiogenesis in the development of DR.
Although DR is considered a microvascular disease, there is increasing evidence that a low-grade inflammatory state of the retina is an early manifestation of DR. Increased levels of multiple cytokines, chemokines and adhesion molecules are found in eye tissue of DR patients. Proinflammatory cytokines mediate the downstream inflammatory signaling pathways, directly promote the progress of DR. Chemokines recruit leukocytes that secrete cytokines to inflammatory sites, making cytokines in functional spatial position; adhesion molecules then bind leukocytes to inflammatory sites, prolonging the action time of functional cells. Chemokines and adhesion molecules help cytokines to enhance inflammation indirectly. The long-term inflammatory state makes the blood-retinal barrier damage, retinal cell apoptosis, and ultimately leads to DR patients’ visual loss.
Therapies targeting inflammation in DR
Over the past decade, advances in drugs and therapies have improved the prevention and treatment of patients with DR. The DR preferred practice pattern recommended that regular follow-up and necessary and appropriate retinal photocoagulation and vitrectomy can prevent severe visual loss in 90% of patients (136). Retinal laser photocoagulation is an important method for the treatment of DR and can be divided into panretinal photocoagulation (PRP) and macular laser treatment. Frequent laser treatment and vitreous surgery can have adverse effects on patients, such as apoptosis of retinal pigment epithelium and other retinal cell types and reduced vision (126).
Optical Coherence Tomography (OCT) is a non-invasive fundus imaging device, which has great clinical significance in the screening, diagnosis, follow-up, and evaluation of therapeutic effect of DR. Serous retinal detachment (SRD) and high reflectivity points (HRDs) on OCT may be related to the anti-inflammatory effect of DME. One of the early events in the pathogenesis of DME is microglia activation. The microglia is an intrinsic macrophage that sits around blood vessels in the inner layer of the retina and is involved in the maintenance of BRB. It is the sentinel cell of the retina. The activated microglia in OCT are seen as high reflectivity points between the retinal layers. OCT showed an increase in interlaminar hyperreflectivity in all diabetic patients, especially in diabetic retinopathy patients (137). The more the number of high reflex points, the worse the effect of anti-VEGF therapy, and the better the effect of hormone therapy (138). SD-OCT can help to predict the visual prognosis of DME patients. Serous retinal detachment is more common in more severe DME, and about 30% of DME is associated with serous retinal detachment (139). Serous retinal detachment suggests an inflammatory factor. Hormones are more effective against serous retinal detachment than anti-VEGF drugs (140).
Although clinicians can use many therapeutic strategies to treat DR, no treatment can completely attenuate clinical progression to reverse retinal damage. More current strategies for the treatment of DR aim to improve therapeutic efficacy, as well as noninvasive or alternative delivery mechanisms that provide a longer duration of action. Table 1 summarizes existing clinical trials that have been completed to treat DR.
Control of metabolic disorders
The fluctuation of blood glucose and hypoglycemia can aggravate ocular fundus changes, and intensive glycemic control can prevent and delay the occurrence and progression of DR. The Diabetes Control and Complications Trial (DCCT) proved that intensive treatment reduced the risks of DR (148). The burden of diabetic retinopathy may be lessened by renin-angiotensin system (RAS) blockers. The Diabetes Retinopathy Candesartan Trials (DIRECT) Programme (NCT00252733, NCT00252720) evaluated whether candesartan might slow the development and progression of retinopathy in T1D patients (142). Although the development of retinopathy is unaffected, candesartan may lower the incidence of retinopathy. In transgenic (mRen-2)27 rats, aliskiren, as a renin inhibitor, reduced intercellular adhesion molecule-1 to control levels and provided the same protection as ACE inhibition against proliferative neovascularization of NPDR and oxygen-induced retinopathy (149). RAS blockers are recommended as the first choice for diabetic patients with hypertension, but RAS blockers are not recommended for the prevention of retinopathy in normotensive diabetic patients. The Action to Control Cardiovascular Risk in Diabetes (ACCORD) study (NCT00542178) has investigated whether intensive glycemic control, combined therapy for dyslipidemia, and intensive blood pressure control limit diabetic retinopathy progression in patients with type 2 diabetes (141). This 10-year duration study found that intensive glucose control and intensive combination therapy for dyslipidaemia reduced the rate of diabetic retinopathy progression.
Anti-angiogenic therapies
VEGF is an important factor involved in the pathophysiological process of DR and DME. Hypoxia and hyperglycemia may lead to the upregulation of VEGF, which may lead to leakage and vascular proliferation. There is a large amount of evidence showing the efficacy of anti-VEGF therapy in DME. Currently, bevacizumab, aflibercept, conbercept, and pegaptanib sodium have been studied in clinical studies as anti-VEGF medications for the treatment of DR (Table 1). Several large randomized trials have expanded on the initial findings of the Diabetic Retinopathy Clinical Research Network (DRCRnet) to show that other VEGF agents (bevacizumab, ranibizumab, and aflibercept) are also superior to laser therapy (150). Pegaptanib sodium, an RNA aptamer targeting VEGF-165, was approved by the Food and Drug Administration (FDA) in 2006 and is the first VEGFA inhibitor in ophthalmology. Pegaptanib sodium can be used in DME and appears to be well tolerated with evidence of efficacy, but vision is still declining in most patients; now, it is rarely used in clinics (151).
In 2004, the FDA authorized bevacizumab as the first antiangiogenic medication for use in the first-line treatment of metastatic colorectal cancer (152). It is a recombinant human IgG-1 monoclonal antibody against VEGF that prevents VEGF from binding to VEGFR by binding to VEGF, and inhibition of endothelial proliferation and activation leads to antiangiogenic and antitumour effects. In large clinical trials, ranibizumab has been shown to be effective and safe in DR treatment, improving DR severity in both NPDR and PDR (153). The researchers investigated the relative effectiveness and safety of glass injections of aflibercept, bevacizumab, and ranibizumab in the treatment of DME. In patients with central involvement of DME, it was discovered that vitreous injections of aflibercept, bevacizumab, and ranibizumab enhanced visual acuity and decreased retinal thickness, although the proportionate benefit depended on baseline eyesight (154).. At a lower initial level of vision, aflibercept was more effective at improving vision. In the DRCR Retina Network trial, which involved moderate vision loss due to DME, the team did not find that over a two-year period, there was a significant difference in visual outcome between aflibercept monotherapy and bevacizumab treatment, and in the event of a poor response, aflibercept may be preferred (155).
Conbercept is the first new biological class I drug with independent intellectual property rights in China and has been given the World Health Organization’s (WHO) international generic name. As a new generation of anti-VEGF fusion proteins, conbercept can inhibit choroidal angiogenesis and reduce the leakage of new blood vessels (156). At the time of the phase III trial, subjects were unable to schedule treatment every 8 weeks or 12 weeks because of the COVID-19 outbreak, and several studies are still recruiting patients. However, the limitations and adverse effects of anti-VEGF therapy have also received much attention. Because anti-VEGF drugs have a short half-life, they need to be injected monthly or every two months to ensure efficacy. In patients receiving anti-VEGF, conjunctival hemorrhage, eye discomfort, cataract, vitreous detachment, vitreous floaters, and elevated intraocular pressure were the most frequently reported side effects (5%) (157). Endophthalmitis and retinal detachment may occur after intravitreal injection. There are also studies to improve injection methods, such as the Port Delivery System (PDS), which is a permanent, repeatable, and small-size eye implant that can deliver custom-formulated ranibizumab over several months. There is potential to reduce the treatment burden of frequent eye injections. In phase 2 trials, PDS was generally well tolerated, reducing the burden of therapy for individuals with neovascular age-related macular degeneration (nAMD) while maintaining visual acuity (158).
Anti-inflammatory therapies
Several studies have demonstrated that inflammation is involved in the pathogenesis of DR. Therefore, anti-inflammation is not only an effective supplement to DR therapy but also an important treatment for some patients who are ineffective or resistant to anti-VEGF therapy. Before the use of VEGF drugs, intravitreal glucocorticoid therapy was popular among treating physicians. Steroids reduce neutrophil migration, restrict access to inflammatory sites, and reduce cytokine production (159, 160). In the nonproliferative phase of DR, DME is the main cause of visual impairment. Dexamethasone vitreous implants have been effective in treating DME indications since the FDA was approved in 2014 (161). The Chinese phase III study of dexamethasone intravitreal implants (DEX-I) for DME patients enrolled 284 Asian patients from 18 centers, and the visual acuity, macular edema thickness, and leakage area of patients treated with DEX-I were better than those treated with laser photocoagulation. Although glucocorticoids such as triamcinolone acetonide and dexamethasone implants have also been shown to reduce retinal thickening and improve vision, the use of glucocorticoids for intravitreal treatment increases the risk of cataract surgery and can lead to elevated intraocular pressure and glaucoma (162). Therefore, the complications of high intraocular pressure and cataract formation should be considered in vitreous glucocorticoids.
Non-steroidal anti-inflammatory drugs (NSAIDs) can reduce damage to the DR retina by inhibiting the expression of inflammatory factors and nonsteroidal anti-inflammatory drug mediators to control the retinal inflammatory reaction. Several studies assessing the effect of localized NSAIDs on diabetic retinopathy have reported improvements in fovea thickness and vision at approximately four to six months (163). Nepafenac ophthalmic (Nevanac, Alcon), the first eye NSAIDS product authorized by the FDA in 2005, is used to relieve pain and inflammation associated with cataract surgery. After being given to the eye, nepafenac can pass through the cornea quickly and transform into aminophenic acid under the influence of ocular tissue hydrolase, which can quickly reach the target location to suppress the activation of caspase-3 and -6 in the retina (164, 165). Nepafenac 0.3% showed superior clinical outcomes than vehicles in two prospective, randomized, multicenter, double-masked, phase 3 trials in patients with DR, with better BCVA after cataract surgery and no unexpected adverse events (145). NSAIDS has definite clinical efficacy and high safety. The adverse reactions disappear automatically after discontinuation of NSAIDS and do not affect the efficacy. However, NSAIDS can cause corneal melting and even perforation, which should be given more attention. The use of NSAIDS in patients with autoimmune diseases, eye diseases, and other diseases should be done with caution.
IL-6 has been investigated as a viable target for anti-inflammatory treatment of DR since it is one of the most significant proinflammatory cytokines in the vitreous of patients with DR. Some clinical trials have led to the development of antibodies against IL-6 (EBI-031) and the IL-6 receptor (tocilizumab). The safety, tolerability, and effectiveness of tocilizumab, a recombinant humanized anti-human interleukin-6 (IL-6) receptor monoclonal antibody, have been examined in clinical studies in eyes with DME (NCT02511067). Clinical research (NCT02842541) is evaluating the safety, tolerability, immunogenicity, and pharmacokinetics of up to three dosage levels of EBI-031 administered intravitreally to participants with diabetic macular edema (NCT02842541). The interventional study found that ketorolac (Acuvail®) concentrations (0.45%) significantly reduced the levels of aqueous IL-8, vitreous IL-8, and platelet-derived growth factor (PDGF) AA in 20 eyes from 20 patients, which suggests that it may induce significant inhibition of inflammatory cytokines involved in the pathogenesis of DR (146).
Vascular adhesion protein-1 (VAP-1) regulates leukocyte adhesion and has semicarbazide-sensitive amine oxidase (SSAO) functions to affect oxidase activity, catalyzing oxidative deamination to produce hydrogen peroxide and aldehydes, leading to the production of AGEs and ALEs (166). PXS-4728A is mainly used in the treatment of cardiometabolic diseases. In acute lung inflammation, PXS-4728A, as a recently reported SSAO inhibitor, was used in the treatment of cardiometabolic diseases. In acute lung inflammation, PXS-4728A reduced CXCL1/KC-induced leukocyte rolling and adhesion (167). In arteriosclerosis, PXS-4728A reduced oxidative stress and the expression of adhesion molecules, chemoattractant proteins and proinflammatory cytokines in the aorta, and it also inhibited the adhesion and migration of monocytes in human umbilical vein endothelial cells (168). Currently, a clinical study that tested the safety and efficacy of BI 1467335 (PXS-4728A) has shown some improvement in patients with DR, with no increase in adverse events (NCT03238963).
Montelukast, a leukotriene receptor antagonist, revealed a protective impact on vision in a streptozotocin-induced diabetes mouse model by inhibiting diabetes-induced capillary and neuronal degeneration (169). Drug blockade of the leukotriene pathway holds the potential for new therapies to prevent or slow diabetic retinopathy development. At present, there is no clinical evaluation in the treatment of DR.
Connexin43 translucency plays a role in the pathogenesis of chronic inflammatory diseases, including activation of the inflammasome pathway, and sanguinarine blockade has been shown to alleviate vascular leakage and inflammation (170, 171). Hyperglycemia and inflammation increased Connexin43 expression in both the Akimba (DR) mouse retinas and the donor retina with confirmed DR (172). Tonabersat, a connexin hemichannel blocker, can inhibit NLRP3 and lysed Caspase-1 complex formation with hyperglycemia and cytokine activation while preventing the release of the proinflammatory cytokines IL-1β, VEGF, and IL-6 (173). Tonabersat reduced retinal inflammation by modulating the assembly of the inflammasome (NLRP3) through Connexin43 translucent blockade while preserving retinal photoreceptor function and restoring vascular integrity (174). Tonabersat has the potential to improve some functional outcomes in diabetic retinopathy and is a potential therapeutic agent.
AKST4290, an inhibitor of CC chemokine receptor-3 (CCR3), is a natural receptor for eotaxin. Wet age-related macular degeneration (wet AMD), as well as other neurological and immunological illnesses, is mostly a result of the pathophysiology of inflammation, immune cell recruitment, and neovascularization, which is regulated by CCR3 (175). The oral medication AKST4290, which works well in preventing eotaxin from attaching to its G-protein coupled receptor (GPCR) CCR3, increased the age-related macular degeneration BCVA score (176). The effectiveness of oral AKST4290 in patients with moderate to severe diabetic retinopathy (CAPRI) is currently being studied (NCT05038020).
Other therapeutic agents
At present, there are a number of drugs being studied for the treatment of DR. First, Runcaciguat, which has been studied from diabetes-related diseases. Runcaciguat (BAY1101042), as a sGC activator, may be an expansive therapeutic option for the prevention of CKD associated with hypertension, diabetes, and obesity (177, 178), which is currently in a phase II clinical trial (NCT04722991).
To develop some visual mechanisms, a nonretinoic acid small molecule called emixustat hydrochloride inhibits RPE65, also known as retinol isomeric hydrolase, which is a 65 kDa protein found in the retina (179). In 23 PDR patients with or without DME, the effects of oral emixustat hydrochloride on proangiogenic and inflammatory cytokines (levels of IL-1, IL-6, IL-8, TGF-1, and VEGF) were assessed. VEGF levels were marginally lower in the emixustat hydrochloride group, although this preliminary investigation did not demonstrate a statistically significant difference in changes in aqueous humor cytokine levels between the emixustat hydrochloride group and the placebo group(NCT02753400) (147). OTT166 is a novel small-molecule-selective integrin inhibitor specifically designed by OcuTerra Therapeutics, Inc. to have the required physicochemical properties to reach the retina from eye drops (180). OTT166 is currently being given in a phase II clinical trial in diabetic retinopathy patients (NCT05409235).
In addition, fenofibrate is a peroxisome proliferator-activated receptor alpha activator (PPARα) involved in the regulation of lipid metabolism disorder, inflammation, oxidative stress, angiogenesis and apoptosis, which reduces the progression of DR (181). It was found that PPARα was downregulated in diabetic retinas, which may be partly due to the overexpression of microRNA-21 (miR-21). In db/db mice, knockdown of miR-21 prevented PPARα downregulation, alleviated microvascular damage, and improved neovascularization and inflammation of the retina (182).
Concluding remarks and future perspectives
Anti-inflammatory approaches targeting certain molecular markers might be viable treatment options for DR since inflammation is now recognized as a significant contributor to the onset and progression of DR. Cytokines, chemokines and adhesion molecules participate in the inflammatory process of diabetic retinopathy. Three types of molecules interact and are inseparable, forming a complex molecular network that promotes the pathological process of diabetic retinopathy(Figure 3). Cytokines are divided into proinflammatory and anti-inflammatory cytokines according to their function. When inflammation occurs, the levels of proinflammatory cytokines are upregulated, promoting inflammatory responses through a variety of pathways, such as retinal cell apoptosis and angiogenesis. Anti-inflammatory cytokines may also reach a higher level to counteract the protective effect of the inflammatory response. Chemokines, as the transport intermediary of cells, induce circulating leukocytes to reach inflammatory sites. Adhesion molecules help white blood cells adhere to inflammatory sites. Three types of molecules perform their own functions, while an increase in the level of one type of molecule also induces the expression of another type of molecule in an inflammatory state; for example, the cytokine TNF-α upregulates the level of the adhesion molecule VCAM-1. The complex pathological mechanism has not yet been elucidated and needs further study in the future. In the future, the immune inflammation mechanism of DR can be further studied to provide insight into the biological function of DR and related targeted therapies. Therefore, these findings imply the clinical importance of new therapies targeting inflammatory responses in the management of DR and facilitate the transfer of recent research findings from ‘bench to bedside’ in the future.
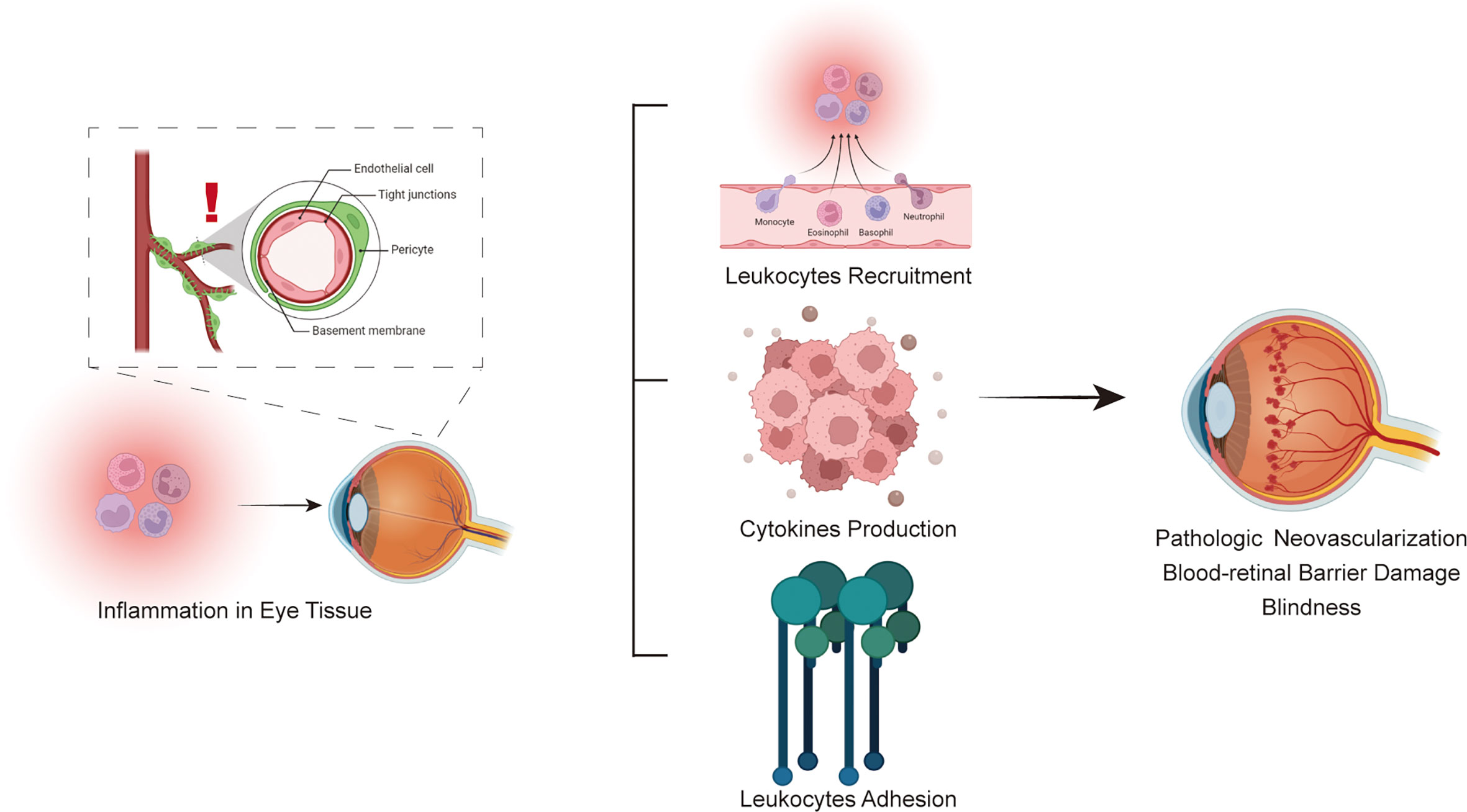
Figure 3 Schematic flowchart for the immune system involved in the pathophysiology of diabetic retinopathy.
Author contributions
Conceptualization; writing-original draft and editing: TY, YS. Writing-review and editing: SL. Writing-review and editing: JW, YW, XZ. All authors contributed to the article and approved the submitted version.
Funding
This study was supported by National Natural Science Foundation of China (Grant No. 81941022, No. 82100857).
Acknowledgments
We thank Suowen Xu for proofreading the manuscript.
Conflict of interest
The authors declare that the research was conducted in the absence of any commercial or financial relationships that could be construed as a potential conflict of interest.
Publisher’s note
All claims expressed in this article are solely those of the authors and do not necessarily represent those of their affiliated organizations, or those of the publisher, the editors and the reviewers. Any product that may be evaluated in this article, or claim that may be made by its manufacturer, is not guaranteed or endorsed by the publisher.
References
1. Cheung N, Mitchell P, Wong TY. Diabetic retinopathy. Lancet (2010) 376(9735):124–36. doi: 10.1016/s0140-6736(09)62124-3
2. Sabanayagam C, Banu R, Chee ML, Lee R, Wang YX, Tan G, et al. Incidence and progression of diabetic retinopathy: A systematic review. Lancet Diabetes Endocrinol (2019) 7(2):140–9. doi: 10.1016/s2213-8587(18)30128-1
3. Sabanayagam C, Chee ML, Banu R, Cheng CY, Lim SC, Tai ES, et al. Association of diabetic retinopathy and diabetic kidney disease with all-cause and cardiovascular mortality in a multiethnic Asian population. JAMA Netw Open (2019) 2(3):e191540. doi: 10.1001/jamanetworkopen.2019.1540
4. Cao K, Wang B, Friedman DS, Hao J, Zhang Y, Hu A, et al. Diabetic retinopathy, visual impairment, and the risk of six-year death: A cohort study of a rural population in China. Ophthalmic Res (2021) 64(6):983–90. doi: 10.1159/000512667
5. Early Treatment Diabetic Retinopathy Study Research Group, Grading diabetic retinopathy from stereoscopic color fundus photographs - an extension of the modified airlie house classification: Etdrs report number 10. Ophthalmology (2020) 127(4s):S99–s119. doi: 10.1016/j.ophtha.2020.01.030
6. Kollias AN, Ulbig MW. Diabetic retinopathy: Early diagnosis and effective treatment. Dtsch Arztebl Int (2010) 107(5):75–83. doi: 10.3238/arztebl.2010.0075
7. Duh EJ, Sun JK, Stitt AW. Diabetic retinopathy: Current understanding, mechanisms, and treatment strategies. JCI Insight (2017) 2(14):e93751. doi: 10.1172/jci.insight.93751
8. Stitt AW, Curtis TM, Chen M, Medina RJ, McKay GJ, Jenkins A, et al. The progress in understanding and treatment of diabetic retinopathy. Prog Retin Eye Res (2016) 51:156–86. doi: 10.1016/j.preteyeres.2015.08.001
9. Aiello LP. Diabetic retinopathy and other ocular findings in the diabetes control and complications Trial/Epidemiology of diabetes interventions and complications study. Diabetes Care (2014) 37(1):17–23. doi: 10.2337/dc13-2251
10. Zhong Q, Kowluru RA. Epigenetic changes in mitochondrial superoxide dismutase in the retina and the development of diabetic retinopathy. Diabetes (2011) 60(4):1304–13. doi: 10.2337/db10-0133
11. Friedrichs P, Schlotterer A, Sticht C, Kolibabka M, Wohlfart P, Dietrich A, et al. Hyperglycaemic memory affects the neurovascular unit of the retina in a diabetic mouse model. Diabetologia (2017) 60(7):1354–8. doi: 10.1007/s00125-017-4254-y
12. Wong TY, Sun J, Kawasaki R, Ruamviboonsuk P, Gupta N, Lansingh VC, et al. Guidelines on diabetic eye care: The international council of ophthalmology recommendations for screening, follow-up, referral, and treatment based on resource settings. Ophthalmology (2018) 125(10):1608–22. doi: 10.1016/j.ophtha.2018.04.007
13. Rodríguez ML, Pérez S, Mena-Mollá S, Desco MC, Ortega ÁL. Oxidative stress and microvascular alterations in diabetic retinopathy: Future therapies. Oxid Med Cell Longev (2019) 2019:4940825. doi: 10.1155/2019/4940825
14. von Scholten BJ, Kreiner FF, Gough SCL, von Herrath M. Current and future therapies for type 1 diabetes. Diabetologia (2021) 64(5):1037–48. doi: 10.1007/s00125-021-05398-3
15. Bluestone JA, Buckner JH, Herold KC. Immunotherapy: Building a bridge to a cure for type 1 diabetes. Science (2021) 373(6554):510–6. doi: 10.1126/science.abh1654
16. Padgett LE, Broniowska KA, Hansen PA, Corbett JA, Tse HM. The role of reactive oxygen species and proinflammatory cytokines in type 1 diabetes pathogenesis. Ann N Y Acad Sci (2013) 1281(1):16–35. doi: 10.1111/j.1749-6632.2012.06826.x
17. Tse HM, Milton MJ, Schreiner S, Profozich JL, Trucco M, Piganelli JD. Disruption of innate-mediated proinflammatory cytokine and reactive oxygen species third signal leads to antigen-specific hyporesponsiveness. J Immunol (2007) 178(2):908–17. doi: 10.4049/jimmunol.178.2.908
18. Vecchio F, Lo Buono N, Stabilini A, Nigi L, Dufort MJ, Geyer S, et al. Abnormal neutrophil signature in the blood and pancreas of presymptomatic and symptomatic type 1 diabetes. JCI Insight (2018) 3(18):e122146. doi: 10.1172/jci.insight.122146
19. Marroqui L, Perez-Serna AA, Babiloni-Chust I, Dos Santos RS. Type I interferons as key players in pancreatic B-cell dysfunction in type 1 diabetes. Int Rev Cell Mol Biol (2021) 359:1–80. doi: 10.1016/bs.ircmb.2021.02.011
20. Eizirik DL, Colli ML, Ortis F. The role of inflammation in insulitis and beta-cell loss in type 1 diabetes. Nat Rev Endocrinol (2009) 5(4):219–26. doi: 10.1038/nrendo.2009.21
21. Quattrin T, Haller MJ, Steck AK, Felner EI, Li Y, Xia Y, et al. Golimumab and beta-cell function in youth with new-onset type 1 diabetes. N Engl J Med (2020) 383(21):2007–17. doi: 10.1056/NEJMoa2006136
22. Donath MY, Shoelson SE. Type 2 diabetes as an inflammatory disease. Nat Rev Immunol (2011) 11(2):98–107. doi: 10.1038/nri2925
23. Weaver JR, Odanga JJ, Breathwaite EK, Treadwell ML, Murchinson AC, Walters G, et al. An increase in inflammation and islet dysfunction is a feature of prediabetes. Diabetes Metab Res Rev (2021) 37(6):e3405. doi: 10.1002/dmrr.3405
24. Roden M, Shulman GI. The integrative biology of type 2 diabetes. Nature (2019) 576(7785):51–60. doi: 10.1038/s41586-019-1797-8
25. Eguchi K, Nagai R. Islet inflammation in type 2 diabetes and physiology. J Clin Invest (2017) 127(1):14–23. doi: 10.1172/jci88877
26. Medzhitov R. Origin and physiological roles of inflammation. Nature (2008) 454(7203):428–35. doi: 10.1038/nature07201
27. Jialal I, Kaur H. The role of toll-like receptors in diabetes-induced inflammation: Implications for vascular complications. Curr Diabetes Rep (2012) 12:172–9. doi: 10.1007/s11892-012-0258-7
28. Rübsam A, Parikh S, Fort PE. Role of inflammation in diabetic retinopathy. Int J Mol Sci (2018) 19(4):942. doi: 10.3390/ijms19040942
29. Tarr JM, Kaul K, Chopra M, Kohner EM, Chibber R. Pathophysiology of diabetic retinopathy. ISRN Ophthalmol (2013) 2013:343560. doi: 10.1155/2013/343560
30. Giacco F, Brownlee M. Oxidative stress and diabetic complications. Circ Res (2010) 107(9):1058–70. doi: 10.1161/circresaha.110.223545
31. Lin WJ, Kuang HY. Oxidative stress induces autophagy in response to multiple noxious stimuli in retinal ganglion cells. Autophagy (2014) 10(10):1692–701. doi: 10.4161/auto.36076
32. Fogli S, Mogavero S, Egan CG, Del Re M, Danesi R. Pathophysiology and pharmacological targets of vegf in diabetic macular edema. Pharmacol Res (2016) 103:149–57. doi: 10.1016/j.phrs.2015.11.003
33. Malik AN, Parsade CK, Ajaz S, Crosby-Nwaobi R, Gnudi L, Czajka A, et al. Altered circulating mitochondrial DNA and increased inflammation in patients with diabetic retinopathy. Diabetes Res Clin Pract (2015) 110(3):257–65. doi: 10.1016/j.diabres.2015.10.006
34. Ellis MP, Lent-Schochet D, Lo T, Yiu G. Emerging concepts in the treatment of diabetic retinopathy. Curr Diabetes Rep (2019) 19(11):137. doi: 10.1007/s11892-019-1276-5
35. Chen W, Esselman WJ, Jump DB, Busik JV. Anti-inflammatory effect of docosahexaenoic acid on cytokine-induced adhesion molecule expression in human retinal vascular endothelial cells. Invest Ophthalmol Vis Sci (2005) 46(11):4342–7. doi: 10.1167/iovs.05-0601
36. Soto I, Krebs MP, Reagan AM, Howell GR. Vascular inflammation risk factors in retinal disease. Annu Rev Vis Sci (2019) 5:99–122. doi: 10.1146/annurev-vision-091517-034416
37. Olson JA, Whitelaw CM, McHardy KC, Pearson DW, Forrester JV. Soluble leucocyte adhesion molecules in diabetic retinopathy stimulate retinal capillary endothelial cell migration. Diabetologia (1997) 40(10):1166–71. doi: 10.1007/s001250050802
38. Dai Y, Wu Z, Wang F, Zhang Z, Yu M. Identification of chemokines and growth factors in proliferative diabetic retinopathy vitreous. BioMed Res Int (2014) 2014:486386. doi: 10.1155/2014/486386
39. Suzuki Y, Nakazawa M, Suzuki K, Yamazaki H, Miyagawa Y. Expression profiles of cytokines and chemokines in vitreous fluid in diabetic retinopathy and central retinal vein occlusion. Jpn J Ophthalmol (2011) 55(3):256–63. doi: 10.1007/s10384-011-0004-8
40. Sohn EH, van Dijk HW, Jiao C, Kok PH, Jeong W, Demirkaya N, et al. Retinal neurodegeneration may precede microvascular changes characteristic of diabetic retinopathy in diabetes mellitus. Proc Natl Acad Sci U.S.A. (2016) 113(19):E2655–64. doi: 10.1073/pnas.1522014113
41. Benveniste EN. Cytokines. Encyclopedia Neurol Sci (2014) 1:921–5. doi: 10.1016/B978-0-12-385157-4.00175-5
42. Dinarello CA. Proinflammatory cytokines. Chest (2000) 118(2):503–8. doi: 10.1378/chest.118.2.503
43. Calle MC, Fernandez ML. Inflammation and type 2 diabetes. Diabetes Metab (2012) 38(3):183–91. doi: 10.1016/j.diabet.2011.11.006
44. Lee J, Lee S, Zhang H, Hill MA, Zhang C, Park Y. Interaction of il-6 and tnf-alpha contributes to endothelial dysfunction in type 2 diabetic mouse hearts. PloS One (2017) 12(11):e0187189. doi: 10.1371/journal.pone.0187189
45. Lim AK, Tesch GH. Inflammation in diabetic nephropathy. Mediators Inflammation (2012) 2012:146154. doi: 10.1155/2012/146154
46. Adamiec-Mroczek J, Oficjalska-Mlynczak J. Assessment of selected adhesion molecule and proinflammatory cytokine levels in the vitreous body of patients with type 2 diabetes–role of the inflammatory-immune process in the pathogenesis of proliferative diabetic retinopathy. Graefes Arch Clin Exp Ophthalmol (2008) 246(12):1665–70. doi: 10.1007/s00417-008-0868-6
47. Kocabora MS, Telli ME, Fazil K, Erdur SK, Ozsutcu M, Cekic O, et al. Serum and aqueous concentrations of inflammatory markers in diabetic macular edema. Ocul Immunol Inflammation (2016) 24(5):549–54. doi: 10.3109/09273948.2015.1034804
48. Rangasamy S, McGuire PG, Das A. Diabetic retinopathy and inflammation: Novel therapeutic targets. Middle East Afr J Ophthalmol (2012) 19(1):52–9. doi: 10.4103/0974-9233.92116
49. Joussen AM, Poulaki V, Mitsiades N, Kirchhof B, Koizumi K, Dohmen S, et al. Nonsteroidal anti-inflammatory drugs prevent early diabetic retinopathy Via tnf-A suppression. FASEB J (2002) 16:438–40. doi: 10.1096/fj.01-0707fje
50. Liu Y, Li L, Pan N, Gu J, Qiu Z, Cao G, et al. Tnf-alpha released from retinal Muller cells aggravates retinal pigment epithelium cell apoptosis by upregulating mitophagy during diabetic retinopathy. Biochem Biophys Res Commun (2021) 561:143–50. doi: 10.1016/j.bbrc.2021.05.027
51. Behl Y, Krothapalli P, Desta T, DiPiazza A, Roy S, Graves DT. Diabetes-enhanced tumor necrosis factor-alpha production promotes apoptosis and the loss of retinal microvascular cells in type 1 and type 2 models of diabetic retinopathy. Am J Pathol (2008) 172(5):1411–8. doi: 10.2353/ajpath.2008.071070
52. Qiu AW, Cao X, Zhang WW, Liu QH. Il-17a is involved in diabetic inflammatory pathogenesis by its receptor il-17ra. Exp Biol Med (Maywood) (2021) 246(1):57–65. doi: 10.1177/1535370220956943
53. Sigurdardottir S, Zapadka TE, Lindstrom SI, Liu H, Taylor BE, Lee CA, et al. Diabetes-mediated il-17a enhances retinal inflammation, oxidative stress, and vascular permeability. Cell Immunol (2019) 341:103921. doi: 10.1016/j.cellimm.2019.04.009
54. Qiu AW, Liu QH, Wang JL. Blocking il-17a alleviates diabetic retinopathy in rodents. Cell Physiol Biochem (2017) 41(3):960–72. doi: 10.1159/000460514
55. Qiu AW, Bian Z, Mao PA, Liu QH. Il-17a exacerbates diabetic retinopathy by impairing Muller cell function Via Act1 signaling. Exp Mol Med (2016) 48(12):e280. doi: 10.1038/emm.2016.117
56. Qiu AW, Huang DR, Li B, Fang Y, Zhang WW, Liu QH. Il-17a injury to retinal ganglion cells is mediated by retinal Muller cells in diabetic retinopathy. Cell Death Dis (2021) 12(11):1057. doi: 10.1038/s41419-021-04350-y
57. Dinarello CA, Simon A, van der Meer JW. Treating inflammation by blocking interleukin-1 in a broad spectrum of diseases. Nat Rev Drug Discovery (2012) 11(8):633–52. doi: 10.1038/nrd3800
58. Karstoft K, Pedersen BK. Exercise and type 2 diabetes: Focus on metabolism and inflammation. Immunol Cell Biol (2016) 94(2):146–50. doi: 10.1038/icb.2015.101
59. Dror E, Dalmas E, Meier DT, Wueest S, Thevenet J, Thienel C, et al. Postprandial macrophage-derived il-1beta stimulates insulin, and both synergistically promote glucose disposal and inflammation. Nat Immunol (2017) 18(3):283–92. doi: 10.1038/ni.3659
60. Maedler K, Liu Y, Biarnés Costa M, Gerhardinger C. Il-1β is upregulated in the diabetic retina and retinal vessels: Cell-specific effect of high glucose and il-1β autostimulation. PloS One (2012) 7(5):e36949. doi: 10.1371/journal.pone.0036949
61. Vincent JA, Mohr S. Inhibition of caspase-1/Interleukin-1beta signaling prevents degeneration of retinal capillaries in diabetes and galactosemia. Diabetes (2007) 56(1):224–30. doi: 10.2337/db06-0427
62. Koleva-Georgieva DN, Sivkova NP, Terzieva D. Serum inflammatory cytokines il-1beta, il-6, tnf-alpha and vegf have influence on the development of diabetic retinopathy. Folia Med (Plovdiv) (2011) 53(2):44–50. doi: 10.2478/v10153-010-0036-8
63. Reverter JL, Nadal J, Ballester J, Ramio-Lluch L, Rivera MM, Fernandez-Novell JM, et al. Diabetic retinopathy is associated with decreased tyrosine nitrosylation of vitreous interleukins il-1alpha, il-1beta, and il-7. Ophthalmic Res (2011) 46(4):169–74. doi: 10.1159/000323812
64. Kowluru RA, Odenbach S. Role of interleukin-1beta in the development of retinopathy in rats: Effect of antioxidants. Invest Ophthalmol Vis Sci (2004) 45(11):4161–6. doi: 10.1167/iovs.04-0633
65. Kowluru RA, Odenbach S. Role of interleukin-1beta in the pathogenesis of diabetic retinopathy. Br J Ophthalmol (2004) 88(10):1343–7. doi: 10.1136/bjo.2003.038133
66. Kowluru RA, Mohammad G, Santos JM, Tewari S, Zhong Q. Interleukin-1beta and mitochondria damage, and the development of diabetic retinopathy. J Ocul Biol Dis Infor (2011) 4(1-2):3–9. doi: 10.1007/s12177-011-9074-6
67. D'Amico AG, Maugeri G, Rasa DM, Bucolo C, Saccone S, Federico C, et al. Modulation of il-1beta and vegf expression in rat diabetic retinopathy after pacap administration. Peptides (2017) 97:64–9. doi: 10.1016/j.peptides.2017.09.014
68. Jesus AA, Goldbach-Mansky R. Il-1 blockade in autoinflammatory syndromes. Annu Rev Med (2014) 65:223–44. doi: 10.1146/annurev-med-061512-150641
69. Fabiani C, Sota J, Tosi GM, Franceschini R, Frediani B, Galeazzi M, et al. The emerging role of interleukin (Il)-1 in the pathogenesis and treatment of inflammatory and degenerative eye diseases. Clin Rheumatol (2017) 36(10):2307–18. doi: 10.1007/s10067-016-3527-z
70. Beli E, Dominguez JM 2nd, Hu P, Thinschmidt JS, Caballero S, Li Calzi S, et al. Cx3cr1 deficiency accelerates the development of retinopathy in a rodent model of type 1 diabetes. J Mol Med (Berl) (2016) 94(11):1255–65. doi: 10.1007/s00109-016-1433-0
71. Yang Y, Takeda A, Yoshimura T, Oshima Y, Sonoda K-H, Ishibashi T. Il-10 is significantly involved in Hsp70-regulation of experimental subretinal fibrosis. PloS One (2013) 8(12):e80288. doi: 10.1371/journal.pone.0080288
72. Mao C, Yan H. Roles of elevated intravitreal il-1beta and il-10 levels in proliferative diabetic retinopathy. Indian J Ophthalmol (2014) 62(6):699–701. doi: 10.4103/0301-4738.136220
73. Song S, Yu X, Zhang P, Dai H. Increased levels of cytokines in the aqueous humor correlate with the severity of diabetic retinopathy. J Diabetes Complications (2020) 34(9):107641. doi: 10.1016/j.jdiacomp.2020.107641
74. Semeraro F, Cancarini A, dell'Omo R, Rezzola S, Romano MR, Costagliola C. Diabetic retinopathy: Vascular and inflammatory disease. J Diabetes Res (2015) 2015:582060. doi: 10.1155/2015/582060
75. Wu F, Phone A, Lamy R, Ma D, Laotaweerungsawat S, Chen Y, et al. Correlation of aqueous, vitreous, and plasma cytokine levels in patients with proliferative diabetic retinopathy. Invest Ophthalmol Vis Sci (2020) 61(2):26. doi: 10.1167/iovs.61.2.26
76. Akbari M, Hassan-Zadeh V. Il-6 signalling pathways and the development of type 2 diabetes. Inflammopharmacology (2018) 26(3):685–98. doi: 10.1007/s10787-018-0458-0
77. Robinson R, Srinivasan M, Shanmugam A, Ward A, Ganapathy V, Bloom J, et al. Interleukin-6 trans-signaling inhibition prevents oxidative stress in a mouse model of early diabetic retinopathy. Redox Biol (2020) 34:101574. doi: 10.1016/j.redox.2020.101574
78. Valle ML, Dworshak J, Sharma A, Ibrahim AS, Al-Shabrawey M, Sharma S. Inhibition of interleukin-6 trans-signaling prevents inflammation and endothelial barrier disruption in retinal endothelial cells. Exp Eye Res (2019) 178:27–36. doi: 10.1016/j.exer.2018.09.009
79. Sharma S. Interleukin-6 trans-signaling: A pathway with therapeutic potential for diabetic retinopathy. Front Physiol (2021) 12:689429. doi: 10.3389/fphys.2021.689429
80. Elsaeidi F, Bemben MA, Zhao XF, Goldman D. Jak/Stat signaling stimulates zebrafish optic nerve regeneration and overcomes the inhibitory actions of Socs3 and sfpq. J Neurosci (2014) 34(7):2632–44. doi: 10.1523/JNEUROSCI.3898-13.2014
81. Ye EA, Steinle JJ. Mir-146a suppresses Stat3/Vegf pathways and reduces apoptosis through il-6 signaling in primary human retinal microvascular endothelial cells in high glucose conditions. Vision Res (2017) 139:15–22. doi: 10.1016/j.visres.2017.03.009
82. Charo IF, Ransohoff RM. The many roles of chemokines and chemokine receptors in inflammation. N Engl J Med (2006) 354(6):610–21. doi: 10.1056/NEJMra052723
83. Taghavi Y, Hassanshahi G, Kounis NG, Koniari I, Khorramdelazad H. Monocyte chemoattractant protein-1 (Mcp-1/Ccl2) in diabetic retinopathy: Latest evidence and clinical considerations. J Cell Commun Signal (2019) 13(4):451–62. doi: 10.1007/s12079-018-00500-8
84. Harada C, Okumura A, Namekata K, Nakamura K, Mitamura Y, Ohguro H, et al. Role of monocyte chemotactic protein-1 and nuclear factor kappa b in the pathogenesis of proliferative diabetic retinopathy. Diabetes Res Clin Pract (2006) 74(3):249–56. doi: 10.1016/j.diabres.2006.04.017
85. Rangasamy S, McGuire PG, Franco Nitta C, Monickaraj F, Oruganti SR, Das A. Chemokine mediated monocyte trafficking into the retina: Role of inflammation in alteration of the blood-retinal barrier in diabetic retinopathy. PloS One (2014) 9(10):e108508. doi: 10.1371/journal.pone.0108508
86. Knott R, Robertson M, Muckersie E, Folefac V, Fairhurst F. A model system for the study of human retinal angiogenesis: Activation of monocytes and endothelial cells and the association with the expression of the monocarboxylate transporter type 1 (Mct-1). Diabetologia (1999) 42(7):870–7. doi: 10.1007/s001250051240
87. Hong KH, Ryu J, Han KH. Monocyte chemoattractant protein-1-Induced angiogenesis is mediated by vascular endothelial growth factor-a. Blood (2005) 105(4):1405–7. doi: 10.1182/blood-2004-08-3178
88. Dong N, Li X, Xiao L, Yu W, Wang B, Chu L. Upregulation of retinal neuronal mcp-1 in the rodent model of diabetic retinopathy and its function in vitro. Invest Ophthalmol Vis Sci (2012) 53(12):7567–75. doi: 10.1167/iovs.12-9446
89. El-Asrar AMA, Nawaz MI, Kangave D, Geboes K, Ola MS, Ahmad S, et al. High-mobility group box-1 and biomarkers of inflammation in the vitreous from patients with proliferative dia betic retinopathy. Mol Vis (2011) 17:1829.
90. Karimabad MN, Hassanshahi G. Significance of Cxcl12 in type 2 diabetes mellitus and its associated complications. Inflammation (2015) 38(2):710–7. doi: 10.1007/s10753-014-9981-3
91. Sayyed SG, Hagele H, Kulkarni OP, Endlich K, Segerer S, Eulberg D, et al. Podocytes produce homeostatic chemokine stromal cell-derived factor-1/Cxcl12, which contributes to glomerulosclerosis, podocyte loss and albuminuria in a mouse model of type 2 diabetes. Diabetologia (2009) 52(11):2445–54. doi: 10.1007/s00125-009-1493-6
92. Keles A, Sonmez K, Erol YO, Ayyildiz SN, Ogus E. Vitreous levels of vascular endothelial growth factor, stromal cell-derived factor-1alpha, and angiopoietin-like protein 2 in patients with active proliferative diabetic retinopathy. Graefes Arch Clin Exp Ophthalmol (2021) 259(1):53–60. doi: 10.1007/s00417-020-04889-0
93. Butler JM, Guthrie SM, Koc M, Afzal A, Caballero S, Brooks HL, et al. Sdf-1 is both necessary and sufficient to promote proliferative retinopathy. J Clin Invest (2005) 115(1):86–93. doi: 10.1172/JCI22869
94. Salcedo R, Oppenheim JJ. Role of chemokines in angiogenesis: Cxcl12/Sdf-1 and Cxcr4 interaction, a key regulator of endothelial cell responses. Microcirculation (2003) 10(3-4):359–70. doi: 10.1038/sj.mn.7800200
95. Segal MS, Shah R, Afzal A, Perrault CM, Chang KH, Schuler A, et al. Nitric oxide cytoskeletal-induced alterations reverse the endothelial progenitor cell migratory defect associated with diabetes. Diabetes (2006) 55:102–9. doi: 10.2337/diabetes.55.01.06.db05-0803
96. Silva RLE, Shen J, Hackett SF, Kachi S, Akiyama H, Kiuchi K, et al. The sdf-1/Cxcr4 Ligand/Receptor pair is an important contributor to several types of ocular neovascularization. FASEB J (2007) 21(12):3219–30. doi: 10.1096/fj.06-7359com
97. Deng L, Jia J, Yao J, Xu Z. Stromal cell-derived factor 1 (Sdf-1) and its receptor Cxcr4 improves diabetic retinopathy. Biosci Biotechnol Biochem (2019) 83(6):1072–6. doi: 10.1080/09168451.2019.1588095
98. Xia YJ, Zeng D, Xia LM, Yu F, Lin HH, Zhou L, et al. Role of monokine induced by interferon-gamma in liver injury induced by hepatitis b virus in mice. J Viral Hepat (2012) 19(7):509–18. doi: 10.1111/j.1365-2893.2011.01581.x
99. Wakabayashi Y, Usui Y, Okunuki Y, Takeuchi M, Kezuka T, Iwasaki T, et al. Increased levels of monokine induced by interferon-gamma (Mig) in the vitreous of patients with diabetic retinopathy. Diabetes Med (2008) 25(7):875–7. doi: 10.1111/j.1464-5491.2008.02466.x
100. Nawaz MI, Van Raemdonck K, Mohammad G, Kangave D, Van Damme J, Abu El-Asrar AM, et al. Autocrine Ccl2, Cxcl4, Cxcl9 and Cxcl10 signal in retinal endothelial cells and are enhanced in diabetic retinopathy. Exp Eye Res (2013) 109:67–76. doi: 10.1016/j.exer.2013.01.008
102. Paparo SR. Rheumatoid arthritis and the Th1 chemokine mig. Clin Ter (2019) 170(6):e472–e7. doi: 10.7417/CT.2019.2178
103. Ghasemi H, Ghazanfari T, Yaraee R, Faghihzadeh S, Hassan ZM. Roles of il-8 in ocular inflammations: A review. Ocular Immunol Inflammation (2011) 19(6):401–12. doi: 10.3109/09273948.2011.618902
104. Petrovič MG, Korošec P, Košnik M, Hawlina M. Association of preoperative vitreous il-8 and vegf levels with visual acuity after vitrectomy in proliferative diabetic retinopathy. Acta Ophthalmol (2010) 88(8):e311–e6. doi: 10.1111/j.1755-3768.2010.02030.x
105. Petrovic MG, Korosec P, Kosnik M, Hawlina M. Vitreous levels of interleukin-8 in patients with proliferative diabetic retinopathy. Am J Ophthalmol (2007) 143(1):175–6. doi: 10.1016/j.ajo.2006.07.032
106. Li A, Dubey S, Varney ML, Dave BJ, Singh RK. Il-8 directly enhanced endothelial cell survival, proliferation, and matrix metalloproteinases production and regulated angiogenesis. J Immunol (2003) 170(6):3369–76. doi: 10.4049/jimmunol.170.6.3369
107. Cicik E, Tekin H, Akar S, Ekmekçi ÖB, Donma O, Koldaş L, et al. Interleukin-8, nitric oxide and glutathione status in proliferative vitreoretinopathy and proliferative diabetic retinopathy. Ophthalmic Res (2003) 35(5):251–5. doi: 10.1159/000072145
108. Cook DN, Chen SC, Sullivan LM, Manfra DJ, Wiekowski MT, Prosser DM, et al. Generation and analysis of mice lacking the chemokine fractalkine. Mol Cell Biol (2001) 21(9):3159–65. doi: 10.1128/mcb.21.9.3159-3165.2001
109. You JJ, Yang CH, Huang JS, Chen MS, Yang CM. Fractalkine, a Cx3c chemokine, as a mediator of ocular angiogenesis. Invest Opthalmol Visual Sci (2007) 48(11):5290–8. doi: 10.1167/iovs.07-0187
110. Mendiola AS, Garza R, Cardona SM, Mythen SA, Lira SA, Akassoglou K, et al. Fractalkine signaling attenuates perivascular clustering of microglia and fibrinogen leakage during systemic inflammation in mouse models of diabetic retinopathy. Front Cell Neurosci (2017) 10:303. doi: 10.3389/fncel.2016.00303
111. Cardona SM, Mendiola AS, Yang Y-C, Adkins SL, Torres V, Cardona AE. Disruption of fractalkine signaling leads to microglial activation and neuronal damage in the diabetic retina. ASN Neuro (2015) 7(5):1759091415608204. doi: 10.1177/1759091415608204
112. Altmann C, Schmidt MHH. The role of microglia in diabetic retinopathy: Inflammation, microvasculature defects and neurodegeneration. Int J Mol Sci (2018) 19(1):110. doi: 10.3390/ijms19010110
113. Jiang M, Xie H, Zhang C, Wang T, Tian H, Lu L, et al. Enhancing Fractalkine/Cx3cr1 signalling pathway can reduce neuroinflammation by attenuating microglia activation in experimental diabetic retinopathy. J Cell Mol Med (2022) 26(4):1229–44. doi: 10.1111/jcmm.17179
114. Joussen AM, Murata T, Tsujikawa A, Kirchhof B, Bursell S-E, Adamis AP. Leukocyte-mediated endothelial cell injury and death in the diabetic retina. Am J Pathol (2001) 158(1):147–52. doi: 10.1016/s0002-9440(10)63952-1
115. Hirano Y, Sakurai E, Matsubara A, Ogura Y. Suppression of icam-1 in retinal and choroidal endothelial cells by plasmid small-interfering rnas in vivo. Invest Ophthalmol Vis Sci (2010) 51(1):508–15. doi: 10.1167/iovs.09-3457
116. Loporchio DF, Tam EK, Cho J, Chung J, Jun GR, Xia W, et al. Cytokine levels in human vitreous in proliferative diabetic retinopathy. Cells (2021) 10(5):1069. doi: 10.3390/cells10051069
117. Turan M, Turan G. Immunoreactivity of icam-1, mmp-2, and nesfatin-1 in lens epithelial cells of patients with diabetes mellitus with or without diabetic retinopathy. Eur J Ophthalmol (2022) 32(1):255–62. doi: 10.1177/1120672120966559
118. Khalfaoui T, Lizard G, Beltaief O, Colin D, Ben Hamida J, Errais K, et al. Immunohistochemical analysis of cellular adhesion molecules (Icam-1, vcam-1) and vegf in fibrovascular membranes of patients with proliferative diabetic retinopathy: Preliminary study. Pathol Biol (Paris) (2009) 57(7-8):513–7. doi: 10.1016/j.patbio.2008.07.021
119. Joy SS, Siddiqui K. Molecular and pathophysiological mechanisms of diabetic retinopathy in relation to adhesion molecules. Curr Diabetes Rev (2019) 15(5):363–71. doi: 10.2174/1573399814666181017103844
120. Yao Y, Du J, Li R, Zhao L, Luo N, Zhai JY, et al. Association between icam-1 level and diabetic retinopathy: A review and meta-analysis. Postgrad Med J (2019) 95(1121):162–8. doi: 10.1136/postgradmedj-2018-136102
121. Gustavsson C, Agardh CD, Zetterqvist AV, Nilsson J, Agardh E, Gomez MF. Vascular cellular adhesion molecule-1 (Vcam-1) expression in mice retinal vessels is affected by both hyperglycemia and hyperlipidemia. PloS One (2010) 5(9):e12699. doi: 10.1371/journal.pone.0012699
122. Xia JP, Liu SQ, Wang S. Intravitreal conbercept improves outcome of proliferative diabetic retinopathy through inhibiting inflammation and oxidative stress. Life Sci (2021) 265:118795. doi: 10.1016/j.lfs.2020.118795
123. Karadayi K, Top C, Gülecek O. The relationship between soluble l-selectin and the development of diabetic retinopathy. Ocul Immunol Inflammation (2003) 11(2):123–9. doi: 10.1076/ocii.11.2.123.15920
124. Yun MR, Im DS, Lee JS, Son SM, Sung SM, Bae SS, et al. Nad(P)H oxidase-stimulating activity of serum from type 2 diabetic patients with retinopathy mediates enhanced endothelial expression of e-selectin. Life Sci (2006) 78(22):2608–14. doi: 10.1016/j.lfs.2005.10.044
125. Kasza M, Meleg J, Vardai J, Nagy B Jr., Szalai E, Damjanovich J, et al. Plasma e-selectin levels can play a role in the development of diabetic retinopathy. Graefes Arch Clin Exp Ophthalmol (2017) 255(1):25–30. doi: 10.1007/s00417-016-3411-1
126. Distefano LN, Garcia-Arumi J, Martinez-Castillo V, Boixadera A. Combination of anti-vegf and laser photocoagulation for diabetic macular edema: A review. J Ophthalmol (2017) 2017:2407037. doi: 10.1155/2017/2407037
127. Gorina R, Lyck R, Vestweber D, Engelhardt B. Beta2 integrin-mediated crawling on endothelial icam-1 and icam-2 is a prerequisite for transcellular neutrophil diapedesis across the inflamed blood-brain barrier. J Immunol (2014) 192(1):324–37. doi: 10.4049/jimmunol.1300858
128. Chang AC, Chen PC, Lin YF, Su CM, Liu JF, Lin TH, et al. Osteoblast-secreted wisp-1 promotes adherence of prostate cancer cells to bone Via the vcam-1/Integrin Alpha4beta1 system. Cancer Lett (2018) 426:47–56. doi: 10.1016/j.canlet.2018.03.050
129. Storm RJ, Persson BD, Skalman LN, Frangsmyr L, Lindstrom M, Rankin G, et al. Human adenovirus type 37 uses Alphavbeta1 and Alpha3beta1 integrins for infection of human corneal cells. J Virol (2017) 91(5):e02019-16. doi: 10.1128/JVI.02019-16
130. Faralli JA, Gagen D, Filla MS, Crotti TN, Peters DM. Dexamethasone increases Alphavbeta3 integrin expression and affinity through a Calcineurin/Nfat pathway. Biochim Biophys Acta (2013) 1833(12):3306–13. doi: 10.1016/j.bbamcr.2013.09.020
131. Wang Y, Yan W, Lu X, Qian C, Zhang J, Li P, et al. Overexpression of osteopontin induces angiogenesis of endothelial progenitor cells Via the Avbeta3/Pi3k/Akt/Enos/No signaling pathway in glioma cells. Eur J Cell Biol (2011) 90(8):642–8. doi: 10.1016/j.ejcb.2011.03.005
132. Friedlander M, Theesfeld CL, Sugita M, Fruttiger M, Thomas MA, Chang S, et al. Involvement of integrins Alpha(V)Beta(3) and Alpha(V)Beta(5) in ocular neovascular diseases. Proc Natl Acad Sci USA (1996) 93(18):9764–9. doi: 10.1073/pnas.93.18.9764
133. Bhatwadekar AD, Kansara V, Luo Q, Ciulla T. Anti-integrin therapy for retinovascular diseases. Expert Opin Investig Drugs (2020) 29(9):935–45. doi: 10.1080/13543784.2020.1795639
134. Matsubara Y, Murata M, Maruyama T, Handa M, Yamagata N, Watanabe G, et al. Association between diabetic retinopathy and genetic variations in A2β1 integrin, a platelet receptor for collagen. Blood (2000) 95(5):1560–4. doi: 10.1182/blood.V95.5.1560.005k43_1560_1564
135. Mrugacz M, Bryl A, Falkowski M, Zorena K. Integrins: An important link between angiogenesis, inflammation and eye diseases. Cells (2021) 10(7):1703. doi: 10.3390/cells10071703
136. Jampol LM, Glassman AR, Sun J. Evaluation and care of patients with diabetic retinopathy. N Engl J Med (2020) 382(17):1629–37. doi: 10.1056/NEJMra1909637
137. Vujosevic S, Midena E. Retinal layers changes in human preclinical and early clinical diabetic retinopathy support early retinal neuronal and müller cells alterations. J Diabetes Res (2013) 2013:905058. doi: 10.1155/2013/905058
138. Hwang HS, Chae JB, Kim JY, Kim DY. Association between hyperreflective dots on spectral-domain optical coherence tomography in macular edema and response to treatment. Invest Ophthalmol Vis Sci (2017) 58(13):5958–67. doi: 10.1167/iovs.17-22725
139. de Smet MD. Insights into the physiopathology of inflammatory macular edema. Dev Ophthalmol (2017) 58:168–77. doi: 10.1159/000455279
140. Vujosevic S, Torresin T, Bini S, Convento E, Pilotto E, Parrozzani R, et al. Imaging retinal inflammatory biomarkers after intravitreal steroid and anti-vegf treatment in diabetic macular oedema. Acta Ophthalmol (2017) 95(5):464–71. doi: 10.1111/aos.13294
141. Chew EY, Ambrosius WT, Davis MD, Danis RP, Gangaputra S, Greven CM, et al. Effects of medical therapies on retinopathy progression in type 2 diabetes. N Engl J Med (2010) 363(3):233–44. doi: 10.1056/NEJMoa1001288
142. Chaturvedi N, Porta M, Klein R, Orchard T, Fuller J, Parving HH, et al. Effect of candesartan on prevention (Direct-prevent 1) and progression (Direct-protect 1) of retinopathy in type 1 diabetes: Randomised, placebo-controlled trials. Lancet (2008) 372(9647):1394–402. doi: 10.1016/s0140-6736(08)61412-9
143. Davis MD, Sheetz MJ, Aiello LP, Milton RC, Danis RP, Zhi X, et al. Effect of ruboxistaurin on the visual acuity decline associated with long-standing diabetic macular edema. Invest Ophthalmol Vis Sci (2009) 50(1):1–4. doi: 10.1167/iovs.08-2473
144. Brown DM, Wykoff CC, Boyer D, Heier JS, Clark WL, Emanuelli A, et al. Evaluation of intravitreal aflibercept for the treatment of severe nonproliferative diabetic retinopathy: Results from the PANORAMA randomized clinical trial. JAMA Ophthalmol (2021) 139(9):946–55. doi: 10.1001/jamaophthalmol.2021.2809
145. Singh RP, Lehmann R, Martel J, Jong K, Pollack A, Tsorbatzoglou A, et al. Nepafenac 0.3% after cataract surgery in patients with diabetic retinopathy: Results of 2 randomized phase 3 studies. Ophthalmology (2017) 124(6):776–85. doi: 10.1016/j.ophtha.2017.01.036
146. Schoenberger SD, Kim SJ, Shah R, Sheng J, Cherney E. Reduction of interleukin 8 and platelet-derived growth factor levels by topical ketorolac, 0.45%, in patients with diabetic retinopathy. JAMA Ophthalmol (2014) 132(1):32–7. doi: 10.1001/jamaophthalmol.2013.6203
147. Kubota R, Jhaveri C, Koester JM, Gregory JK. Effects of emixustat hydrochloride in patients with proliferative diabetic retinopathy: A randomized, placebo-controlled phase 2 study. Graefes Arch Clin Exp Ophthalmol (2021) 259(2):369–78. doi: 10.1007/s00417-020-04899-y
148. Lachin JM, Genuth S, Cleary P, Davis MD, Nathan DM. Retinopathy and nephropathy in patients with type 1 diabetes four years after a trial of intensive therapy. N Engl J Med (2000) 342(6):381–9. doi: 10.1056/nejm200002103420603
149. Wilkinson-Berka JL, Tan G, Binger KJ, Sutton L, McMaster K, Deliyanti D, et al. Aliskiren reduces vascular pathology in diabetic retinopathy and oxygen-induced retinopathy in the transgenic (Mren-2)27 rat. Diabetologia (2011) 54(10):2724–35. doi: 10.1007/s00125-011-2239-9
150. Baker CW, Jiang Y, Stone T. Recent advancements in diabetic retinopathy treatment from the diabetic retinopathy clinical research network. Curr Opin Ophthalmol (2016) 27(3):210–6. doi: 10.1097/icu.0000000000000262
151. Sivaprasad S, Browning RC, Starita C. An open-label, one-year, noncomparative study to evaluate the safety and tolerability of intravitreal pegaptanib sodium in patients with diabetic macular edema. Clin Ophthalmol (2014) 8:1565–71. doi: 10.2147/opth.S68498
152. Huang M, Lin Y, Wang C, Deng L, Chen M, Assaraf YG, et al. New insights into antiangiogenic therapy resistance in cancer: Mechanisms and therapeutic aspects. Drug Resist Update (2022) 64:100849. doi: 10.1016/j.drup.2022.100849
153. Chatziralli I. Ranibizumab for the treatment of diabetic retinopathy. Expert Opin Biol Ther (2021) 21(8):991–7. doi: 10.1080/14712598.2021.1928629
154. Wells JA, Glassman AR, Ayala AR, Jampol LM, Aiello LP, Antoszyk AN, et al. Aflibercept, bevacizumab, or ranibizumab for diabetic macular edema. N Engl J Med (2015) 372(13):1193–203. doi: 10.1056/NEJMoa1414264
155. Jhaveri CD, Glassman AR, Ferris FL 3rd, Liu D, Maguire MG, Allen JB, et al. Aflibercept monotherapy or bevacizumab first for diabetic macular edema. N Engl J Med (2022) 387(8):692–703. doi: 10.1056/NEJMoa2204225
156. Zhang X, Wu J, Wu C, Bian AL, Geng S, Dai RP. Comparison of aqueous humor levels of plgf and vegf in proliferative diabetic retinopathy before and after intravitreal conbercept injection. Diabetes Res Clin Pract (2020) 162:108083. doi: 10.1016/j.diabres.2020.108083
157. Zhang B, Zhou Z, Zhang B, Wang D. Efficacy and safety of various treatments for proliferative diabetic retinopathy: A systematic review and network meta-analysis. Front Pharmacol (2021) 12:709501. doi: 10.3389/fphar.2021.709501
158. Campochiaro PA, Marcus DM, Awh CC, Regillo C, Adamis AP, Bantseev V, et al. The port delivery system with ranibizumab for neovascular age-related macular degeneration: Results from the randomized phase 2 ladder clinical trial. Ophthalmology (2019) 126(8):1141–54. doi: 10.1016/j.ophtha.2019.03.036
159. Martidis A, Duker JS, Greenberg PB, Rogers AH, Puliafito CA, Reichel E, et al. Intravitreal triamcinolone for refractory diabetic macular edema. Ophthalmology (2002) 109(5):920–7. doi: 10.1016/s0161-6420(02)00975-2
160. Gillies MC, Sutter FK, Simpson JM, Larsson J, Ali H, Zhu M. Intravitreal triamcinolone for refractory diabetic macular edema: Two-year results of a double-masked, placebo-controlled, randomized clinical trial. Ophthalmology (2006) 113(9):1533–8. doi: 10.1016/j.ophtha.2006.02.065
161. García-Layana A, Figueroa MS, Arias L, Adán A, Cabrera F, Abraldes M, et al. Clinical decision-making when treating diabetic macular edema patients with dexamethasone intravitreal implants. Ophthalmologica (2018) 240(2):61–72. doi: 10.1159/000486800
162. Rittiphairoj T, Mir TA, Li T, Virgili G. Intravitreal steroids for macular edema in diabetes. Cochrane Database Syst Rev (2020) 11(11):Cd005656. doi: 10.1002/14651858.CD005656.pub3
163. Sahoo S, Barua A, Myint KT, Haq A, Abas AB, Nair NS. Topical non-steroidal anti-inflammatory agents for diabetic cystoid macular oedema. Cochrane Database Syst Rev (2015) 2):Cd010009. doi: 10.1002/14651858.CD010009.pub2
164. Kern TS, Miller CM, Du Y, Zheng L, Mohr S, Ball SL, et al. Topical administration of nepafenac inhibits diabetes-induced retinal microvascular disease and underlying abnormalities of retinal metabolism and physiology. Diabetes (2007) 56(2):373–9. doi: 10.2337/db05-1621
165. Gardiner TA, Anderson HR, Degenhardt T, Thorpe SR, Baynes JW, Archer DB, et al. Prevention of retinal capillary basement membrane thickening in diabetic dogs by a non-steroidal anti-inflammatory drug. Diabetologia (2003) 46(9):1269–75. doi: 10.1007/s00125-003-1147-z
166. Kubota R, Reid MJ, Lieu KL, Orme M, Diamond C, Tulberg N, et al. Comparison of inhibitor and substrate selectivity between rodent and human vascular adhesion protein-1. Mediators Inflammation (2020) 2020:3270513. doi: 10.1155/2020/3270513
167. Schilter HC, Collison A, Russo RC, Foot JS, Yow TT, Vieira AT, et al. Effects of an anti-inflammatory vap-1/Ssao inhibitor, pxs-4728a, on pulmonary neutrophil migration. Respir Res (2015) 16(1):42. doi: 10.1186/s12931-015-0200-z
168. Wang SH, Yu TY, Tsai FC, Weston CJ, Lin MS, Hung CS, et al. Inhibition of semicarbazide-sensitive amine oxidase reduces atherosclerosis in apolipoprotein e-deficient mice. Transl Res (2018) 197:12–31. doi: 10.1016/j.trsl.2018.03.001
169. Bapputty R, Talahalli R, Zarini S, Samuels I, Murphy R, Gubitosi-Klug R. Montelukast prevents early diabetic retinopathy in mice. Diabetes (2019) 68(10):2004–15. doi: 10.2337/db19-0026
170. Roy S, Jiang JX, Li AF, Kim D. Connexin channel and its role in diabetic retinopathy. Prog Retin Eye Res (2017) 61:35–59. doi: 10.1016/j.preteyeres.2017.06.001
171. Mugisho OO, Green CR, Zhang J, Acosta ML, Rupenthal ID. Connexin43 hemichannels: A potential drug target for the treatment of diabetic retinopathy. Drug Discovery Today (2019) 24(8):1627–36. doi: 10.1016/j.drudis.2019.01.011
172. Mugisho OO, Green CR, Zhang J, Binz N, Acosta ML, Rakoczy E, et al. Immunohistochemical characterization of Connexin43 expression in a mouse model of diabetic retinopathy and in human donor retinas. Int J Mol Sci (2017) 18(12):2567. doi: 10.3390/ijms18122567
173. Lyon H, Shome A, Rupenthal ID, Green CR, Mugisho OO. Tonabersat inhibits Connexin43 hemichannel opening and inflammasome activation in an in vitro retinal epithelial cell model of diabetic retinopathy. Int J Mol Sci (2020) 22(1):298. doi: 10.3390/ijms22010298
174. Mat Nor MN, Rupenthal ID, Green CR, Acosta ML. Connexin hemichannel block using orally delivered tonabersat improves outcomes in animal models of retinal disease. Neurotherapeutics (2020) 17(1):371–87. doi: 10.1007/s13311-019-00786-5
175. Hirahara S, Nozaki M, Ohbayashi M, Hasegawa N, Ozone D, Ogura Y. Suppression of retinal neovascularization by anti-Ccr3 treatment in an oxygen-induced retinopathy model in mice. Ophthalmic Res (2017) 58(1):56–66. doi: 10.1159/000463238
176. Stewart MW, Garg S, Newman EM, Jeffords E, Konopińska J, Jackson S, et al. Safety and therapeutic effects of orally administered Akst4290 in newly diagnosed neovascular age-related macular degeneration. Retina (2022) 42(6):1038–46. doi: 10.1097/iae.0000000000003446
177. Hahn MG, Lampe T, El Sheikh S, Griebenow N, Woltering E, Schlemmer KH, et al. Discovery of the soluble guanylate cyclase activator runcaciguat (Bay 1101042). J Med Chem (2021) 64(9):5323–44. doi: 10.1021/acs.jmedchem.0c02154
178. Sandner P, Follmann M, Becker-Pelster E, Hahn MG, Meier C, Freitas C, et al. Soluble gc stimulators and activators: Past, present and future. Br J Pharmacol (2021) 179(11):2321–7. doi: 10.1111/bph.15698
179. Bavik C, Henry SH, Zhang Y, Mitts K, McGinn T, Budzynski E, et al. Visual cycle modulation as an approach toward preservation of retinal integrity. PloS One (2015) 10(5):e0124940. doi: 10.1371/journal.pone.0124940
180. Askew BC, Furuya T, Edwards DS. Ocular distribution and pharmacodynamics of Sf0166, a topically administered A(V)B(3) integrin antagonist, for the treatment of retinal diseases. J Pharmacol Exp Ther (2018) 366(2):244–50. doi: 10.1124/jpet.118.248427
181. Persistent effects of intensive glycemic control on retinopathy in type 2 diabetes in the action to control cardiovascular risk in diabetes (Accord) follow-on study. Diabetes Care (2016) 39(7):1089–100. doi: 10.2337/dc16-0024
Keywords: diabetic retinopathy, immune inflammation, therapy, cytokines, chemokines
Citation: Yue T, Shi Y, Luo S, Weng J, Wu Y and Zheng X (2022) The role of inflammation in immune system of diabetic retinopathy: Molecular mechanisms, pathogenetic role and therapeutic implications. Front. Immunol. 13:1055087. doi: 10.3389/fimmu.2022.1055087
Received: 27 September 2022; Accepted: 25 November 2022;
Published: 13 December 2022.
Edited by:
Jialin Gao, First Affiliated Hospital of Wannan Medical College, ChinaReviewed by:
Manuela Bartoli, Augusta University, United StatesJohn Vincent Forrester, University of Aberdeen, United Kingdom
José Carlos Rivera, University of Montreal, Canada
Copyright © 2022 Yue, Shi, Luo, Weng, Wu and Zheng. This is an open-access article distributed under the terms of the Creative Commons Attribution License (CC BY). The use, distribution or reproduction in other forums is permitted, provided the original author(s) and the copyright owner(s) are credited and that the original publication in this journal is cited, in accordance with accepted academic practice. No use, distribution or reproduction is permitted which does not comply with these terms.
*Correspondence: Yali Wu, wuyali@ustc.edu.cn; Xueying Zheng, lxyzheng@ustc.edu.cn
†These authors have contributed equally to this work and share first authorship