Soil carbon dynamics are linked to tree species growth strategy in a naturally regenerating tropical forest
- 1Lancaster Environment Centre, Lancaster University, Lancaster, United Kingdom
- 2UK Centre for Ecology & Hydrology, Penicuik, United Kingdom
- 3Smithsonian Tropical Research Institute, Panama City, Panama
- 4Max Planck Institute of Animal Behavior, ETH Zürich, Zürich, Switzerland
- 5Department of Environmental Systems Science, ETH Zürich, Zürich, Switzerland
Secondary tropical forests are increasingly important for their role in the global carbon (C) balance as they can rapidly accumulate aboveground biomass C during regrowth. Substantial amounts of plant-derived carbon are also incorporated into the soil through decomposition processes, but our understanding of soil C dynamics during forest regrowth is limited. Secondary succession is characterised by a shift in tree functional groups from light-demanding to shade-tolerant species over time, which can influence rates of C turnover via differences in litter quality and by modifying the decomposition environment. Changes in decomposition processes in turn affect the amount of organic C stored in the soil or released to the atmosphere as CO2. Consequently, understanding how tree functional composition influences C turnover during decomposition could help us predict soil C storage during tropical forest regrowth. We experimentally explored the relationship between tree functional groups and soil C dynamics (decomposition and respiration) by conducting a litter decomposition experiment across a successional gradient of naturally regenerating tropical forest. We created litter mixtures representing tree communities differing in their shade tolerance, as well as a functionally diverse litter mixture, and observed litter mass loss and soil respiration as measures of C turnover over a 6 month period. Litter from light-demanding species decomposed faster than litter from shade-tolerant species, which was reflected in the pattern of soil respiration. There were no clear patterns of increasing or decreasing rates of litter decay or soil respiration with increasing forest age, but there was an interaction between stand age and litter type which influenced both decomposition and soil respiration rates. Interestingly, soil respiration from the functionally diverse litter mixture was significantly higher in the younger than older forest stands, and the functionally diverse litter mixture decayed more rapidly than expected in one of the younger stands. Our findings highlight the potential importance of functionally diverse plant inputs, as well as the interaction between local environmental attributes and litter type, for soil C dynamics in tropical forests.
1. Introduction
Secondary tropical forests now make up over half of all tropical forests globally, and are expected to increase in area as a result of agricultural abandonment and recovery from selective logging (Chazdon, 2014). Regenerating tropical forests rapidly accumulate C in aboveground biomass (Pan et al., 2011) and thus, secondary or “regrowth” forests are becoming increasingly important for climate regulation (Poorter et al., 2016). Importantly, over half of the C stock in tropical forests is stored in soils (Don et al., 2011) but our understanding of soil C dynamics during secondary forest regrowth is hampered by the complexity of biogeochemical processes and inconsistent patterns of soil C gains during forest recovery (Yang et al., 2011; Li et al., 2012; Martin et al., 2013; Marín-Spiotta and Sharma, 2013; Wallwork et al., 2022; van der Sande et al., 2023). Recent work demonstrated that shifts in tree community composition during secondary forest regrowth influence soil C storage via differences in the quality of plant inputs to the soil (Wallwork et al., 2022). Litter quality is usually defined by specific leaf traits, such as foliar concentrations of nitrogen (N), lignin and secondary metabolites, which determine the availability of plant-derived C compounds to decomposer organisms (Santiago, 2007; Cornwell et al., 2008; Bakker et al., 2011; Szefer et al., 2017). Easily degradable compounds such as sugars and amino acids are more efficiently used by soil microbes compared to complex structural C compounds and thus contribute to the microbial stabilisation of C in soil organic matter (Cotrufo et al., 2013). However, a large proportion of plant-derived C is released to the atmosphere as CO2 through microbial respiration (Kuzyakov, 2006). Consequently, microbial activity is strongly related to C turnover (Prescott and Grayston, 2013) and changes in soil C dynamics during secondary succession are likely to be linked to shifts in litter quality.
Changes in litter quality arise during secondary succession because the characteristic shift from light-demanding to shade-tolerant species (Dent et al., 2013; Chazdon, 2014; Whitfeld et al., 2014) is reflected in a set of coordinated leaf traits (Conti and Díaz, 2013). Light-demanding species that invest in fast growth often produce “high-quality” litter with high nutrient concentrations, high specific leaf area, and low content of structural compounds such as lignin (Wright et al., 2004; Chazdon, 2014). Conversely, shade-tolerant species are more likely to have “low quality” litter characterised by low nutrient concentrations, high fibre and lignin content and greater concentrations of foliar defence compounds such as tannins and phenols (Wright et al., 2004; Ostertag et al., 2008). These marked changes in leaf traits and the overall quality of litter inputs that accompany the shift from light-demanding to shade-tolerant tree functional groups during secondary succession (Cortez et al., 2007; Kröber et al., 2012) are likely to influence decomposition processes (Cornelissen et al., 1999; Jewell et al., 2017) and thus determine the amount of C stored as soil organic matter or released as CO2 to the atmosphere (Kutsch et al., 2009). Soil respiration in tropical forests represents one of the largest natural sources of atmospheric CO2, for example, mature tropical broadleaf forests are estimated to release around 14.31 Mg C ha–1 year–1 to the atmosphere (Anderson-Teixeira et al., 2021), of which c. 24–62% is derived from the decomposition of plant litter (Sayer and Tanner, 2010; Wang et al., 2017). Litter quality is a dominant control of both decomposition (Cornwell et al., 2008; Hattenschwiler et al., 2011) and soil respiration (Fanin et al., 2011; Bréchet et al., 2017) in tropical forests because, although moisture and temperature are strong predictors of decay rates (Coûteaux et al., 1995; Powers et al., 2009) and soil CO2 efflux (Raich and Schlesinger, 1992) globally, neither tend to be limiting in moist lowland tropical environments. The combination of litter decay and soil respiration rates may provide a more complete picture of soil carbon turnover by capturing both the early response of physical fragmentation as well as the turnover of finer particles and leachate in later stage decomposition (Jewell et al., 2017). A mechanistic understanding of the links between plant traits, decomposition, and soil C dynamics during secondary tropical forest succession could therefore provide crucial information for forest management to maximise soil C storage and help parameterise ecosystem models (e.g., Pugh et al., 2019). However, linking litter quality to soil C dynamics is particularly complex in tropical forests, due to the extraordinarily high plant species diversity (Wright, 2002; Fanin et al., 2011).
Naturally occurring litter comprises a mixture of senesced leaves from numerous tree species with distinct leaf traits, which can provide a wide range of resources to microbial decomposers, modify decomposer interactions (Hättenschwiler et al., 2005), or supress microbial activity (Chomel et al., 2016). As a result of this “functional complementarity” of leaf traits, the decomposition of mixed species litter cannot necessarily be predicted from the decay rates of the component species (Gartner and Cardon, 2004; Gessner et al., 2010; Jewell et al., 2017). Instead, decay rates of litter mixtures are often “non-additive,” whereby mixed litter decomposes more rapidly (synergistic effects) or more slowly (antagonistic effects) than would be expected based on the decay rates of individual component species (Kou et al., 2020). A recent meta-analysis demonstrated that synergistic effects of litter mixtures on mass loss predominate (Kou et al., 2020), and thus, species diversity could promote soil carbon storage by enhancing the availability of litter-derived compounds to microbial decomposers (Chen et al., 2020). Studies in temperate systems have also shown that synergistic effects of litter mixtures can also manifest as differences in CO2 release from decomposing litter (Wang et al., 2014; Mao et al., 2017). Consequently, it is conceivable that the functional diversity of the tree community will determine C turnover rates. Unfortunately, much of our knowledge around the role of plant diversity in driving soil C dynamics is derived from temperate studies focussing on a few dominant species, which may not apply to highly diverse tropical forests (Laird-Hopkins et al., 2017). However, previous work demonstrated that simple litter mixtures representing tree functional groups can represent highly diverse natural litter in decomposition experiments (Laird-Hopkins et al., 2017) and that differences in broad tree functional groups influence soil C storage during secondary tropical forest succession (Wallwork et al., 2022). Although the changes in litter inputs that accompany shifts in tree functional groups are also likely to influence soil respiration rates, the linkages between tree functional diversity, decomposition, and soil respiration have yet to be established in tropical forests. Consequently, experimental studies using multi-species litter mixtures representing tree functional groups could help us elucidate the influence of shifts in tree community composition on soil C dynamics during secondary succession (Laird-Hopkins et al., 2017; Getaneh et al., 2022).
Studies assessing the relationship between changes in tree community composition and biogeochemical cycling during secondary succession over long time periods are rare; instead most substitute space-for-time using chronosequences of forest stands to represent a gradient of successional stages (Walker et al., 2010; Powers and Marín-Spiotta, 2017). An important caveat of chronosequence studies is that they rely on the assumption that variation among stands is determined solely by forest age and that all other factors are identical (Johnson and Kiyoko, 2008). However, even fine-scale spatial heterogeneity in environmental conditions relevant to decomposition processes, such as soil physical and chemical properties, would likely play an important role in soil C turnover and contribute to variation between stands (van der Sande et al., 2023). Consequently, differences in stand environmental conditions and soil properties, as well as tree functional composition, need to be taken into account to identify potential mechanisms underpinning changes in soil C dynamics during forest succession. Here, we used litter mixtures from tree species differing in their shade tolerance as a key functional characteristic, and measured litter mass loss, soil respiration, and soil properties in four stands representing a tropical forest age gradient to test the following hypotheses:
H1. Litter from light-demanding tree species will have high nutrient but low structural fibre content and will therefore decompose faster and result in higher respiration rates than litter from slow-growing shade-tolerant trees.
H2. Litter containing a mixture of both light-demanding and shade-tolerant species will result in faster decomposition and higher respiration rates than predicted rates based on the constituent litter mixtures of functionally similar tree species.
H3. Consequently, C turnover rates (rates of litter decay and soil respiration) will be higher in young stands dominated by light-demanding species compared to old-growth stands dominated by slow-growing shade-tolerant trees, but C turnover rates will be overall highest in mid-successional stand characterised by a mixture of tree functional groups.
We further expected that differences in soil properties would contribute to variation in the decomposition rates of the litter mixtures among stands.
2. Materials and methods
2.1. Study site and litter mixtures
The experiment was conducted in four stands of naturally regenerating forest within the Barro Colorado National Monument (BCNM) Panama, Central America which are part of an established chronosequence of forest plots (Dent et al., 2013). The climate is classified as moist tropical with a mean annual temperature of c. 27°C and a distinct dry season from January to April. The average annual rainfall is 2600 mm, of which 90% falls in the rainy season (Windsor, 1990). The soils are described as Cambisols and Ferralsols on sedimentary and volcanic parent materials (Baillie et al., 2007) that do not differ significantly in soil C or nutrient concentrations (Yavitt, 2000; Grimm et al., 2008). To represent a gradient of secondary regrowth forest succession, we selected three secondary forest (SF) stands aged 40, 60, and 90 years old, and an old-growth (OG) stand aged at >500 years old (henceforth 40Y, 60Y, 90Y, and OG, respectively; Table 1). Although tree community shade-tolerance does not always increase linearly with forest age, there was an increase in the relative influence (RI) of shade-tolerant species and a decrease in light-demanding species with age across our four stands (Table 1; Wallwork et al., 2022) thus allowing us to use forest age as a descriptor for successional stage. Each stand was ≥5 ha in size, within which we established five replicate blocks per stand. Replicate blocks were ≥4 m2 in size and spaced at least 20 m apart on level terrain, avoiding obvious disturbances (e.g., footpaths, canopy gaps, animal activity).
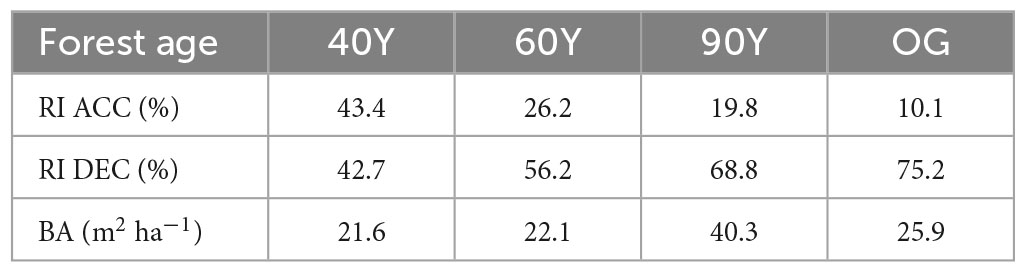
Table 1. Stand characteristics for four secondary forest stands (40, 60, 90 years old and OG) in Panama, Central America; where RI ACC is relative influence of accelerating growth tree species, RI DEC is relative influence of decelerating growth tree species, BA is total tree basal area (Wallwork et al., 2022).
We previously characterised the tree functional composition of the four stands using species-specific data on tree growth response to increasing light (Rüger et al., 2009; Table 1) to represent tree community shade tolerance (Wallwork et al., 2022) and we used the same classification to create functionally distinct litter mixtures. Briefly, species were assigned to one of two growth-response categories: “accelerating” or “decelerating” growth with increasing light based on a light effect parameter ranging from −0.6 to 3.3 (Rüger et al., 2009). Accelerating species (ACC; light effect >1) represent light-demanding, resource-acquisitive “pioneer” species, whereas decelerating species (DEC; light effect <1) represent shade-tolerant, resource-conservative species (Rüger et al., 2009).
To determine whether changes in tree functional groups during secondary succession influence decomposition rates and soil respiration, and to test non-additive effects of functionally diverse litter mixtures, we created three distinct “functional litter treatments”: (1) litter from ACC species, representing a young, light-demanding, secondary forest community; (2) litter from DEC species, representing a shade-tolerant, old secondary or old-growth forest community; and (3) a mixture of ACC and DEC species (MIX), representing a more functionally diverse, intermediate successional stage community. Henceforth, the ACC, DEC and MIX litters are referred to collectively as “functional litter treatments.” We also used a standard litter treatment comprising natural mixed litter from each forest stand (NAT) to assess whether our litter mixtures were representative of natural litter. To ensure that the litter treatments represented changes in tree functional groups during succession, while reflecting species composition at the study site, we chose species within each growth response category that: (a) showed a clear trend (positive or negative) in relative abundance with forest age; (b) were representative of earlier or later stages of succession based on other studies, and (c) had leaf traits that are considered broadly representative of successional stage. Based on the above criteria, the ACC treatment comprised litter from Luehea seemannii Triana and Planch and Miconia argentea (Sw.) DC., and the DEC treatment comprised litter from Protium panamense (Rose) I.M.Johnst. and Tetragastris panamensis (Engl.) Kuntze (Table 2). The MIX treatment included all four species. The three functional litter treatments (ACC, DEC, MIX) contained an equal mass of litter from the constituent species.
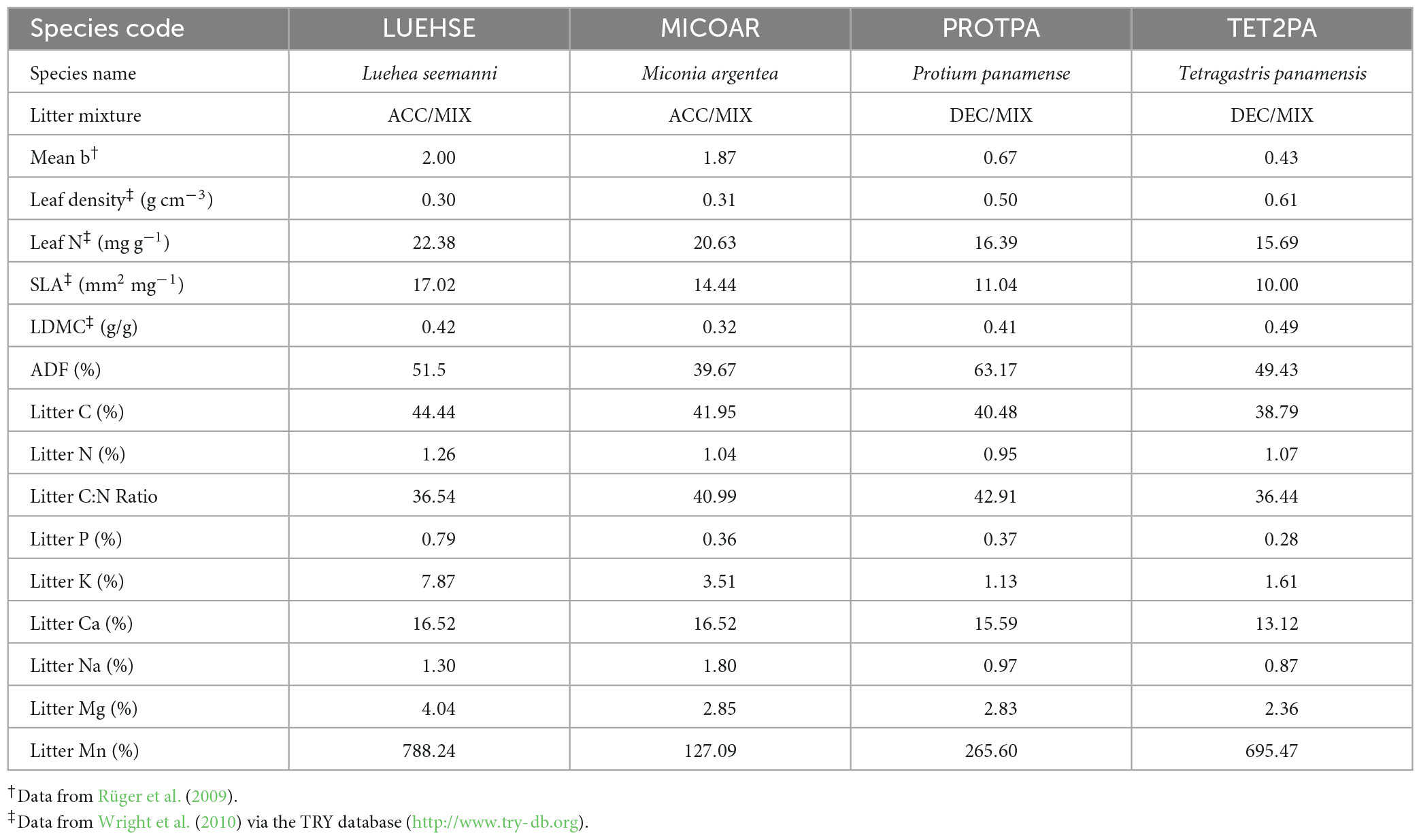
Table 2. Selected characteristics of four tree species used to create three functionally distinct litter treatments (ACC, DEC, and MIX) for a litter decomposition experiment in lowland tropical forest in Panama, Central America, where: mean b is the species-specific light effect parameter, leaf N is foliar nitrogen concentration, SLA is specific leaf area, LDMC is leaf dry matter content, ADF is acid dissolvable fibre from litter, and litter C, N, P, K, Ca, Na, Mg, and Mn are litter carbon, nitrogen, phosphorous, potassium, calcium, sodium, magnesium, and manganese concentration, respectively.
2.2. Litter collection and chemical analysis
We collected freshly fallen litter for the four tree species during the dry season from February to April 2017, which coincided with the period of highest litterfall and minimised decomposition prior to collection. Litter was collected from the forest floor at least once a week to ensure fresh samples and avoiding specimens with visual signs of decay or disease. To obtain representative samples, litter was collected from beneath c. 10 individuals of each species within the age class of forest stand they represented. We also collected stand-specific natural mixed litter (NAT) from the five replicate blocks in each stand which were homogenised across the stand to create a representative composite sample. To determine the functional characteristics of the litter treatments, we collected c. 40 g (dry weight) of additional litter samples from five individuals per species (or groups of individuals, where it was not possible to distinguish the litter among closely grouped individuals). All litter samples were oven-dried separately at 65°C to constant weight directly after collection. To standardise the size of litter fragments we removed petioles and cut larger leaves into pieces of <10 cm length.
We determined litter nutrient, carbon and fibre concentrations as a measure of litter quality. The nutrient and carbon concentrations of the functional litter treatments were determined using ground, air-dried litter of each constituent species. Foliar phosphorus (P), potassium (K), calcium (Ca), magnesium (Mg) concentrations were determined on three replicate samples per species by Inductively Coupled Plasma-Optical Emission Spectrometry (ICP-OES) after HNO3 digestion. Total litter C and N were analysed for five replicates per species using high temperature combustion gas chromatography on a Vario El III C/N analyser (Elementar, Stockport, UK).
Three replicate samples per species were analysed for acid detergent fibre (ADF) following Van Soest et al. (1992). Briefly, 1 g of ground sample was placed in a crucible with 1 g of acetanilide and boiled for 1 h with 100 ml of acid detergent solution and four drops of n-Octanol using a FOSS fibertec™ 8000 fibre analysis system (FOSS, Hilleroed Denmark). Samples were rinsed with distilled water until acid-free and soaked with reagent-grade acetone, then dried overnight at 105°C before weighing. Weighed samples were then soaked in 25 ml H2SO4 (72%) and stirred every hour for 3 h, before being rinsed with hot distilled water and dried overnight at 105°C. The samples were then placed in a furnace at 525°C for 3 h then left to cool in desiccators at room temperature before weighing. To calculate total extracted fibre content (ADF), the weight of the processed sample was subtracted from the original sample weight and corrected using blanks.
2.3. Field experiment and respiration measurements
To link measurements of litter decomposition and soil respiration, we used in situ mesocosms to delimit the experimental area from surrounding soil and litter. Mesocosms are an effective method to measure both litter decomposition and soil respiration in a single system; they provide comparable litter mass loss measurements to litter-bags while minimising disturbance and maintaining natural environmental conditions (Laird-Hopkins et al., 2017). The mesocosms were PVC tubes (20-cm diameter and c. 15-cm height) sunk into the soil to a depth of 2 cm, which were installed in April 2017, allowing the soil to recover from initial disturbance for at least 2 weeks before the start of measurements. We installed a total of five mesocosms in each of the five replicate blocks per stand, with one mesocosm for each of the four litter treatments (ACC, DEC, MIX and NAT) and an additional mesocosm without litter (bare soil controls; CTL), which allowed us to assess the influence of baseline belowground stand characteristics on soil respiration. Hence, we installed a total of 100 mesocosms (four stands, five replicate blocks, five treatments), giving n = 5 replicate values for each measurement and treatment. Natural litter was removed from the mesocosms, and mesh baskets (10-mm plastic mesh) were installed in each mesocosm to contain the litter treatments (Laird-Hopkins et al., 2017). Using “baskets” instead of litterbags provided a natural decomposition environment with maximum contact between litter and soil during decomposition but also allowed us to collect the litter remaining at the end of the experiment to determine mass loss. In May 2017, each basket received 12 g dry weight of litter, based on the average standing litter dry weight measured across the chronosequence. The mesocosms were covered with mesh (10-mm) to prevent additional inputs of natural litter.
To investigate the effects of functionally distinct litter treatments on soil respiration, we took monthly measurements of soil CO2 efflux (SR) over each mesocosm for 6 months from May to October 2017 using an infrared gas analyser attached to a 20-cm diameter soil survey chamber (Li-8100; LI-COR Biosciences, Lincoln, NE, USA). Total soil respiration measured above decaying litter is henceforth referred to as SRTOT, whereas soil respiration from bare soil controls is referred to as SRB. To account for differences in soil moisture and temperature among stands, we measured soil water content at 0–6 cm depth using a Thetaprobe (Delta-T Devices, Cambridge, UK) at three points within 1 m of each mesocosm, and soil temperature at a depth of 0–10 cm in the same area using a temperature probe (Fisher Scientific, Leicestershire, UK).
2.4. Soil sampling and litter mass loss
To assess litter mass loss, we collected the remaining litter from each mesocosm at the end of the study in October 2018. Samples were sealed in individual plastic bags, transported to the laboratory within 2 h, stored at 4°C and processed within 2 days. To calculate litter mass loss and decay rate we carefully removed any large soil particles and extraneous material from fresh samples before weighing, we then washed a pre-weighed subsample for 2 min to remove any remaining soil particles before drying at 60°C for 48 h and weighing again to calculate litter dry mass (g).
We characterised the soil of the forest stands by collecting three soil cores (0–5 cm depth) from each replicate block using a 4.8-cm diameter punch corer and homogenised the cores to make one composite sample per block. Fresh subsamples were taken from each composite sample to determine soil water content and pH. Total soil C and N content was determined by high temperature combustion gas chromatography on a Vario El III C/N analyser (Elementar, Stockport, UK) using 30 mg subsamples of homogenised, ground, air-dried soil. Extractable P and K were determined using the modified Morgan’s method at a commercial laboratory (Central Analytical Laboratory, SRUC Veterinary Services, Midlothian UK). Soil pH was measured on a 1:3 mixture of fresh soil and deionised water using a bench pH metre (STARTER 2100, OHAUS, NJ, USA, and Mettler Toledo® Seven Compact®, Leicester, UK).
2.5. Data analyses
The litter decay rate k for each mesocosm was calculated from the dry mass of litter remaining at the end of the experiment following Olson (1963; Eq. 1):
Where t is time in years, X is litter dry mass at collection, and X0 is the initial litter dry mass (12 g). Decay rates were used for statistical analyses, but we show litter mass loss as the more intuitive measure of decomposition in figures.
Non-additive effects of the mixed litter treatment on litter decay and soil respiration rates were assessed for each replicate block by comparing the observed rates of litter decay or SRTOT of the MIX treatment to the predicted rates calculated from the means of the two component (ACC and DEC) litter treatments (Eq. 2).
Where EXPi is the expected value of decay (k) or respiration rate (SRTOT) for the MIX treatment and ACCi and DECi are the observed values for k or SRTOT for the ACC and DEC litter treatments, respectively.
Data analyses were performed using R version 3.5.2 (R Core Team, 2018) using the vegan package for multivariate analyses (Oksanen et al., 2008) and the lme4 (Bates et al., 2015) and lmerTest (Kuznetsova et al., 2017) packages for mixed effects models. Data were log-transformed where necessary to meet model assumptions.
To test our first hypothesis, we assessed differences in litter traits between ACC and DEC species using the non-parametric Wilcoxon test (wilcox.test function) to account for non-normally distributed data, which did not improve with transformation. We then tested the influence of the forest environment on soil respiration from the bare soil controls (SRB), using linear mixed effects models (lmer function) to model SRB as a function of stand age, with month and replicate block as random effects. We then evaluated whether decay rates or SRTOT differed among functional litter mixtures, and whether stand age influenced decomposition of litter mixtures using linear mixed effects models. We modelled decay rate as a function of treatment, stand age, and their interaction with replicate block as a random effect, including the decay rates for NAT litter as reference values. We assessed the influence of functional litter mixture and stand age on SRTOT using linear mixed effects models with treatment, stand and their interaction as fixed effects and month and replicate block as random effects, including SRTOT over NAT litter as reference values.
Significance of all models was determined by sequentially dropping terms until a minimum adequate model was reached, using the Akaike Information Criterion corrected for small sample sizes (AICc) and p-values to check for model improvement. The final model fit was assessed using diagnostic plots (Bates et al., 2015).
To test our second hypothesis, we compared observed and predicted values under the MIX litter treatment in each stand for: (a) decay rate using linear models (lm function) and (b) SRTOT using linear mixed effects models with the predicted/observed factor, stand and their interaction as fixed effects and month and replicate block as random effects.
Finally, to aid interpretation of stand age effects on C turnover in addition to the influence of tree functional composition (our third hypothesis), we assessed whether differences in soil properties among stands might contribute to distinct rates of litter decay or SRB. We compared soil properties (total C and N concentrations, extractable P and K, pH, water content, and temperature; Table 3) among stands using linear models. We subsequently reduced the multivariate soil data to two dimensions using principal components analysis (PCA, rda function) based on the soil properties measured in each block. All soil values were centred and scaled before analysis and fitted to the ordination as vectors to aid interpretation (envfit function). We assessed whether stands were separated in ordination space with a permutational multivariate analysis of variance (perMANOVA, adonis function; Anderson, 2017) based on Euclidean distance and 9999 permutations. We then extracted the scores for the first two principal components (PC1 and PC2) to represent differences in soil properties among stands and tested whether PC1 or PC2 explained variation in SRB or litter decay rates for each litter mixture separately using linear models (lm function).
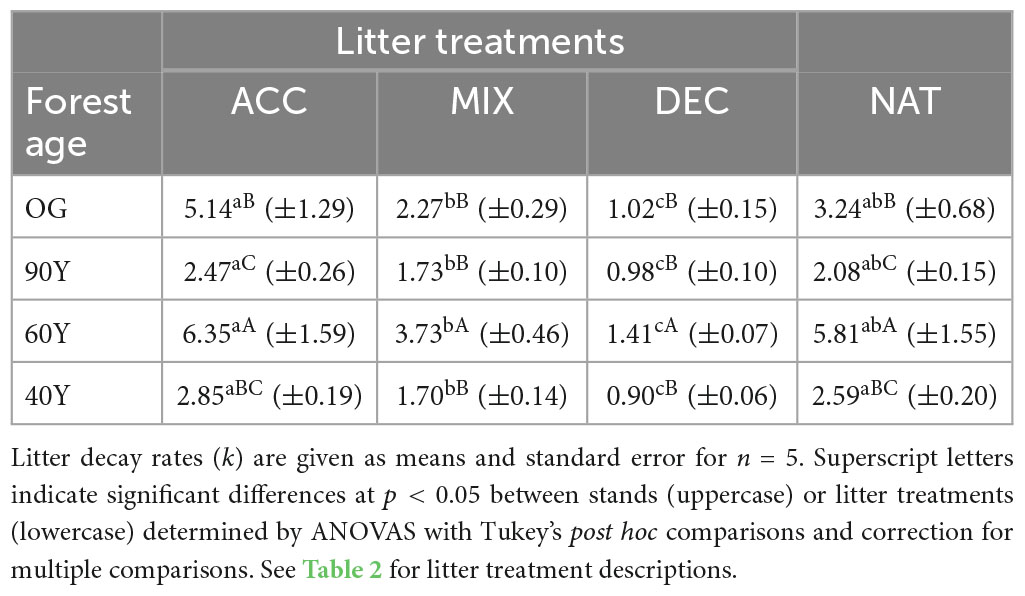
Table 3. Litter decay rates (k) for three functional litter treatments and stand-specific natural litter across an age gradient of forest stands after 5 months of decomposition in Panama, Central America; the stands are 40Y = 40-year old, 60Y = 60-year old, 90Y = 90 year-old, OG, old-growth; the functional litter treatments are: DEC, shade-tolerant (decelerating growth) species litter; ACC, light-demanding (accelerating growth) species litter; MIX, mixture of decelerating and accelerating species litter, and natural litter; NAT, stand-specific natural litter treatments.
Results are reported as significant at p < 0.05 and as a non-significant trend at p < 0.1. For all linear mixed effects models, the significance of terms was determined using Satterthwaite’s approximation to estimate degrees of freedom. Full model terms and statistics are provided in Supplementary Table 1.
3. Results
3.1. Litter traits and decay rates
The ACC and DEC litter mixtures were characterised by distinct leaf traits. Total litter C concentrations were higher in the ACC litter than in the DEC litter, but ACC litter had slightly lower concentrations of ADF than DEC litter (Table 2). Surprisingly, total N concentrations and the litter C:N ratio were similar between ACC and DEC species, as were Mn concentrations (Table 2). Nonetheless, the ACC species litter had significantly higher K, Ca, and Na concentrations, and there was a trend toward higher P and Mg in ACC compared to DEC litter (Table 2).
Litter decay rates differed notably among stands and treatments (stand + treatment effect; p < 0.001; Supplementary Table 1 and Figure 1) but there was no linear relationship between litter decay rate and stand age. Across stands, ACC litter decayed slightly faster than NAT litter, whereas DEC and MIX litter decayed more slowly (treatment effect: p < 0.001; Figure 1). Thus, the mass loss of litter treatments across stands occurred consistently in the order ACC > NAT > MIX >> DEC (Table 3 and Figure 1) resulting in significant differences among all functional litter treatments. Nonetheless, the stand environment also influenced decomposition, as the decay rates of all litter treatments were lower in the 90Y and 40Y stands compared to the 60Y and OG stands (stand effect: p = 0.0007; Supplementary Table 1). Notably, the decay rates of NAT litter and all functional litter treatments were higher in the 60Y compared to all other stands (p < 0.001; Supplementary Table 1 and Table 3).
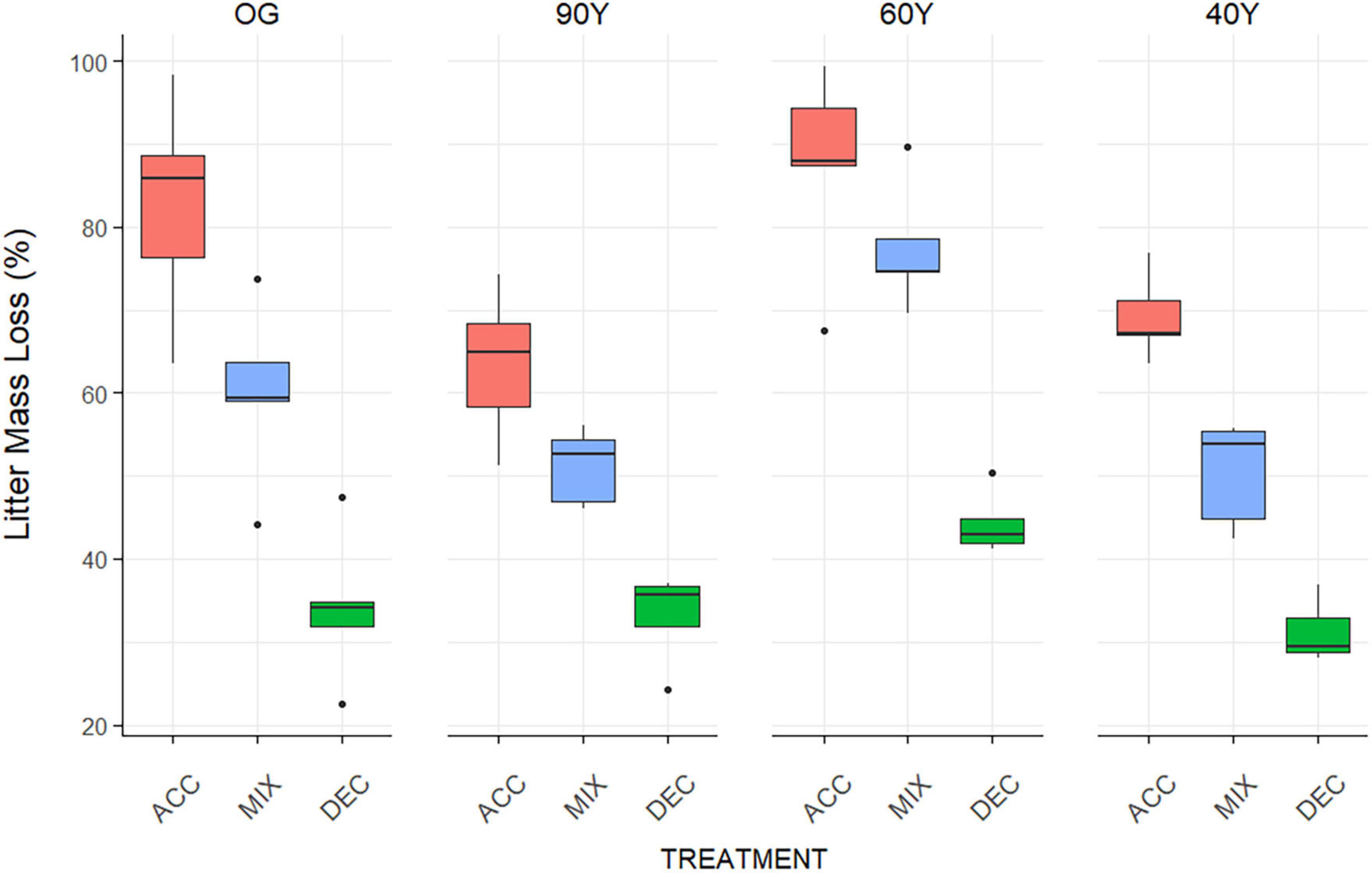
Figure 1. Litter mass loss of three functional litter mixtures and stand-specific natural litter after 5 months of decomposition across an age gradient of tropical forest in Panama, Central America; ACC = light-demanding, accelerating growth species (red), DEC = shade-tolerant, decelerating growth species (green), MIX = a mixture of light-demanding and shade-tolerant species (blue) and NAT = stand-specific natural litter (light grey). 40Y, 60Y, and 90Y refer to stand age in years, and OG is old-growth forest. Boxes denote the 25th and 75th percentiles and median lines are given for n = 5, whiskers indicate values up to 1.5× the interquartile range, and dots indicate outliers.
3.2. Differences in soil respiration among stands and litter mixtures
Measurements of respiration over bare soil revealed significantly lower belowground respiration (SRB) in the 90Y compared to all other stands, but there were no differences among the others (χ2 = 32.54, p < 0.001; Supplementary Figure 1). However, total soil respiration (SRTOT) differed markedly among stands and litter mixtures (treatment × stand interaction, p < 0.001; Supplementary Table 1). Across litter mixtures, there was no difference in SRTOT between OG and 60Y or 40Y stands but SRTOT was higher in the 60Y stand compared to the 90Y stand (p = 0.022). Across stands, respiration rates for the ACC treatment were highest in the early stages of decomposition (June–July) and declined toward the end of the experiment. By contrast, respiration rates for the DEC treatment gradually increased throughout the experiment and were highest in late-stage decomposition (October–September, Figure 2). SRTOT from the MIX treatment first tracked that of the ACC litter during early decomposition, and then matched the increasing SRTOT from the DEC treatment in late-stage decomposition (Figure 2). As a result, SRTOT under NAT and MIX litter was higher than under ACC litter (p = 0.001 and p < 0.001, respectively), and DEC litter (p = 0.031 and 0.003, respectively; Figure 3). The litter × age interaction was largely due to differences in SRTOT under MIX litter, which was substantially higher in 60Y and 40Y stands than in 90Y or OG stands (p < 0.001 for all contrasts; Figure 3).
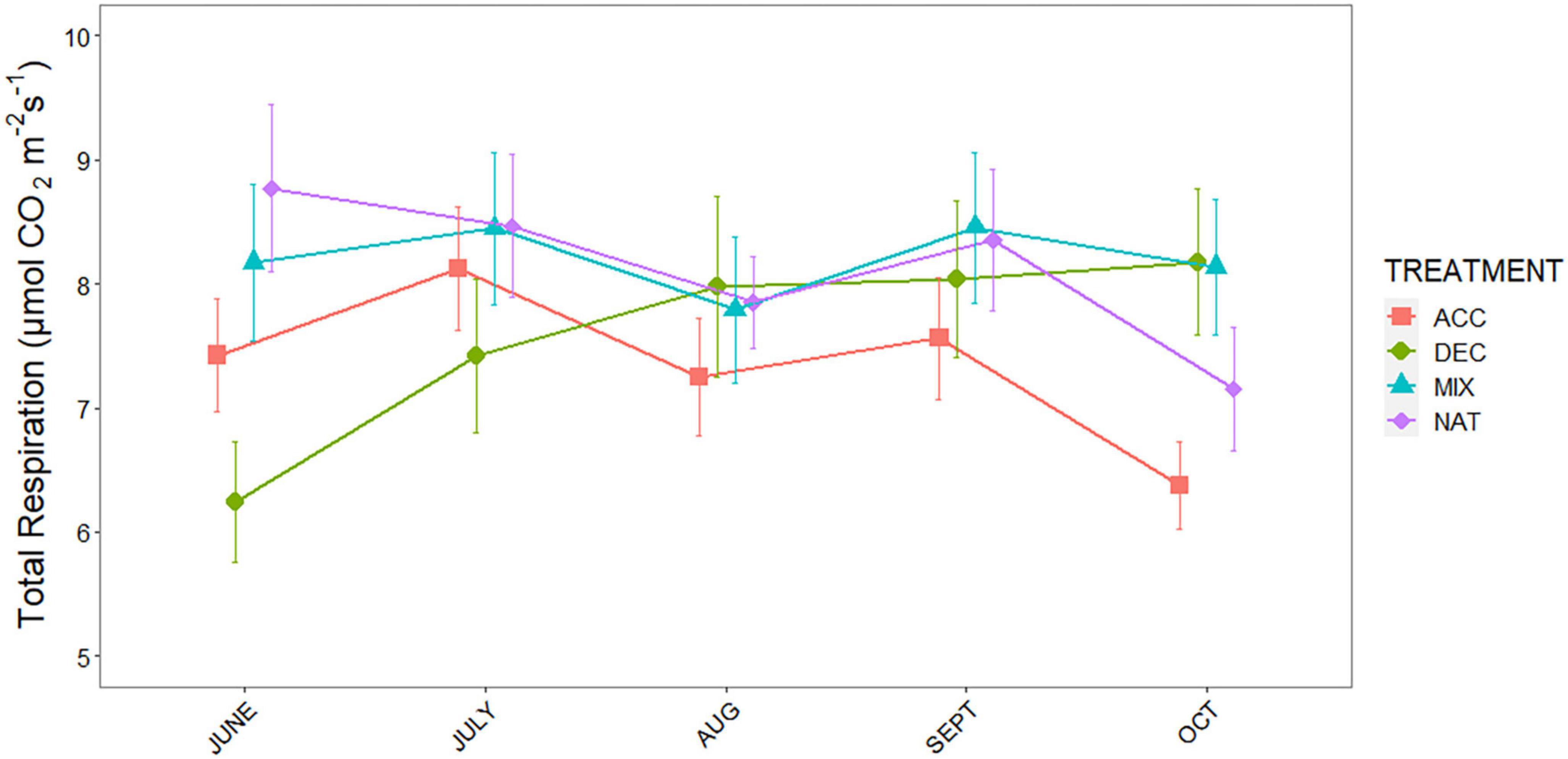
Figure 2. Total soil respiration (SRTOT) of three functional litter mixtures and stand specific natural litter in four forest stands measured during a litter decomposition experiment in Panama, Central America: light-demanding, accelerating growth species (ACC) = red squares; shade-tolerant, decelerating growth species (DEC) = green circles; a mixture of light-demanding and shade-tolerant species (MIX) = blue triangles, and stand-specific natural litter (NAT) = purple diamonds. Means and standard errors are shown for n = 5 per time point and treatment.
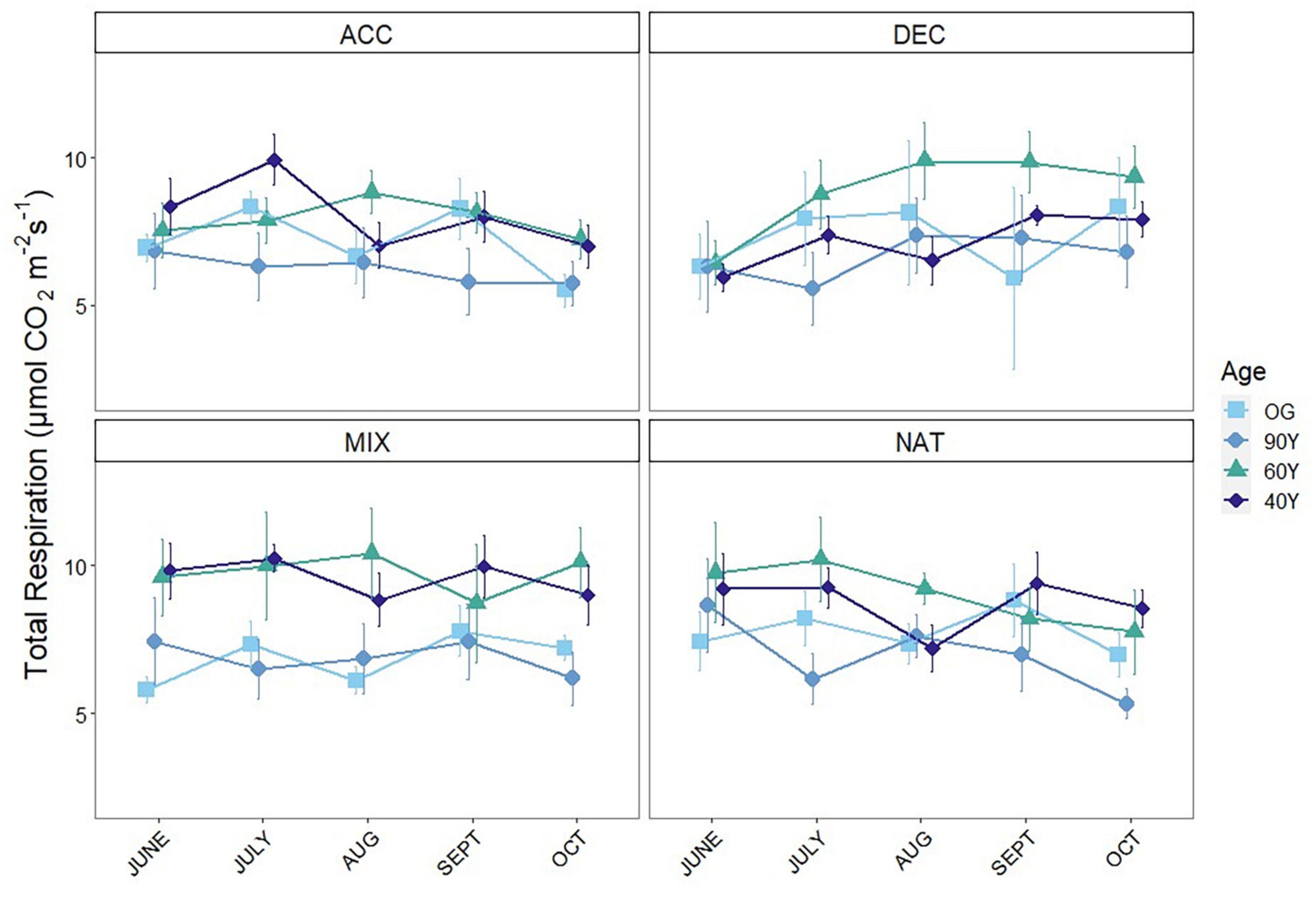
Figure 3. Comparison of total soil respiration (SRTOT) of three functional litter mixtures and stand specific natural litter in four forest stands measured during a litter decomposition experiment in Panama, Central America. Where: ACC = light-demanding accelerating growth species; DEC = shade-tolerant, decelerating growth species; MIX = a mixture of light-demanding and shade-tolerant species; and NAT = stand-specific natural litter. 40Y (diamonds), 60Y (triangles), and 90Y (circles) refer to stand age in years, and OG is old-growth forest (squares). Separate panels show means, and standard errors for n = 5 measurements per time point.
3.3. Non-additive effects of functionally diverse litter
Overall, litter decay rates of the MIX treatment were close to predicted rates but the relationship between predicted and observed mass loss of MIX litter differed among stands [F(7,11) = 8.39, p = 0.001; Figure 4]. Specifically, there was a significant synergistic effect on litter decomposition in the 60Y stand, where the MIX treatment decayed c. 16% faster than predicted (p = 0.04; Figure 4) but effects were purely additive in the other stands.
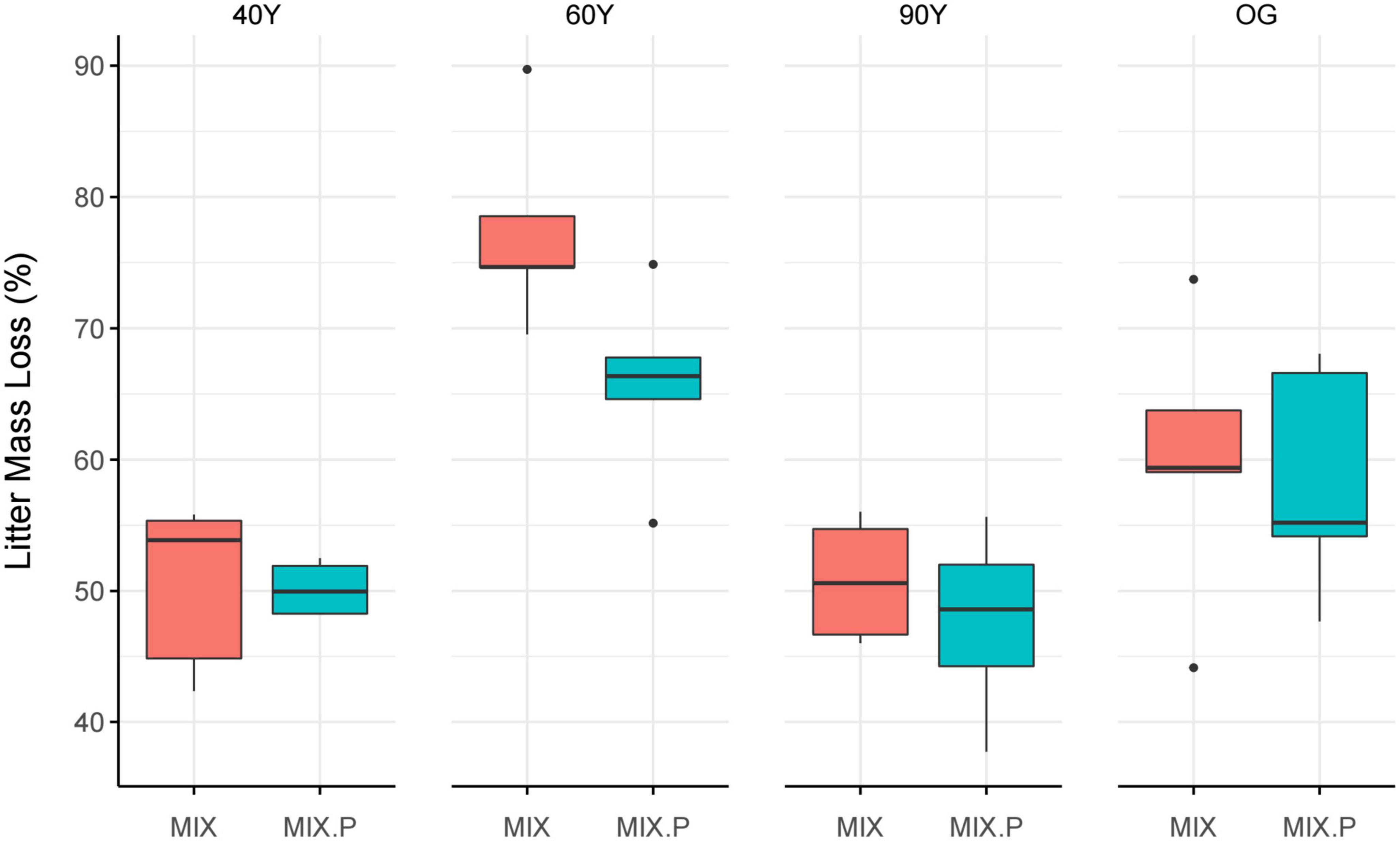
Figure 4. Comparison of observed vs. predicted mass loss of the mixed (MIX) litter treatment, after 5 months of decomposition across an age gradient of tropical forest in Panama, Central America. MIX (red) is the measured mass loss of litter treatments containing a mixture of light-demanding and shade-tolerant species, and MIX.P (blue) the predicted mass loss, i.e., the mean of the measured mass loss of light-demanding (ACC) species litter, and shade-tolerant (DEC) species litter treatments (ACC + DEC/2). 40Y, 60Y, and 90Y refer to stand age in years, and OG is old-growth forest. Boxes denote the 25th and 75th percentiles and median lines are given for n = 5, whiskers indicate values up to 1.5× the interquartile range, and dots indicate outliers.
Across all four stands, we observed an overall significant synergistic effect of the functionally diverse MIX litter treatment on SRTOT, and there was a significant interaction with forest stand (χ2 = 48.97, p < 0.001). This was explained by significantly higher observed SRTOT compared to predicted SRTOT in the 40Y and 60Y stands (Figure 5) and significantly higher observed SRTOT in the two younger compared to the two older stands. However, there were no significant non-additive effects for SRTOT in the two older forest stands. Analysis of non-additive effects in individual stands revealed that SRTOT under the MIX treatment was c. 19% higher than predicted in the 40Y stand (χ2 = 18.22, p < 0.001; Figure 5), and c. 15% higher in the 60Y stand (χ2 = 12.30, p < 0.001; Figure 5).
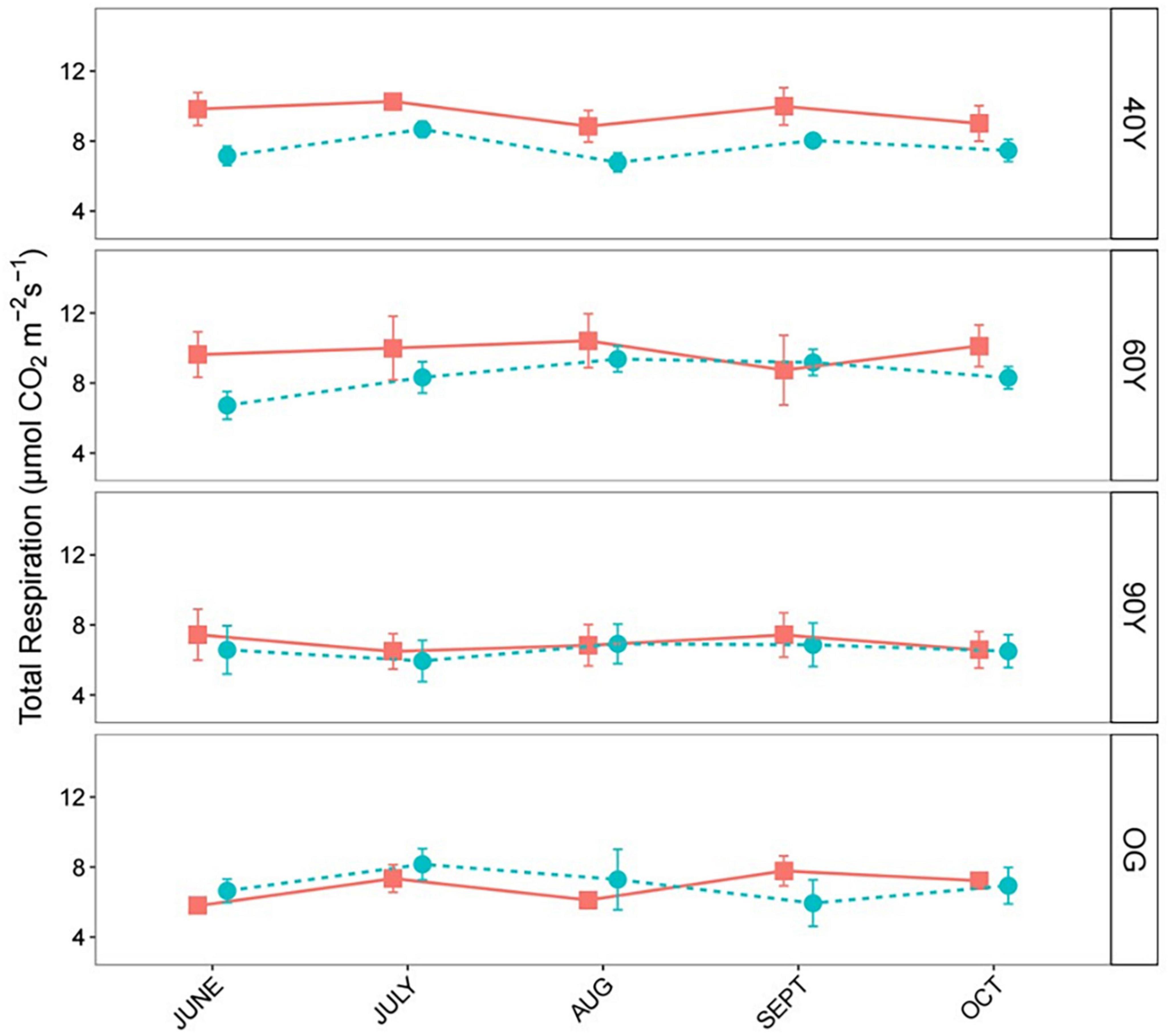
Figure 5. Comparison of observed vs. predicted total soil respiration (SRTOT) of the mixed (MIX) litter treatment, during 5 months of decomposition across an age gradient of tropical forest in Panama, Central America. MIX (red squares) is the observed mass loss of litter treatments (containing a mixture of light-demanding and shade-tolerant species), and MIX.P (blue circles) is the predicted mass loss, i.e., the mean from the measured mass loss of light-demanding (ACC) species litter, and shade-tolerant (DEC) species litter treatments (ACC + DEC/2). 40Y, 60Y, and 90Y refer to stand age in years, and OG is old-growth forest. Separate panels show means, and standard errors for n = 5 measurements per time point.
3.4. Influence of forest stand characteristics on decay rates and soil respiration
Linear models indicated differences among stands in total soil C [F(3,16) = 4.04, p = 0.026] and N concentrations [F(3,16) = 4.14, p = 0.024], with higher values in the two younger stands than the two older stands, however, post hoc contrasts revealed no significant differences between pairs of individual stands (see Table 4). Soil pH was significantly higher in the 60Y compared to 90Y stand [F(3,16) = 5.17, p = 0.011] but did not differ among the other stands. Soil extractable P did not differ among stands, but extractable K was slightly higher in the 60Y than 40Y stand [marginally significant trend, F(3,16) = 3.11, p = 0.056; Table 4]. Soil water content was lower in the 40Y than 60Y stand but there were no significant differences between other stands [F(3,16) = 3.72, p = 0.033; Table 4]. Finally, soil temperature was around 1°C lower in the OG than the other stands [F(3,16) = 30.16, p < 0.001; Table 4] but there was no difference in temperature among the 40Y, 60Y, and 90Y stands.
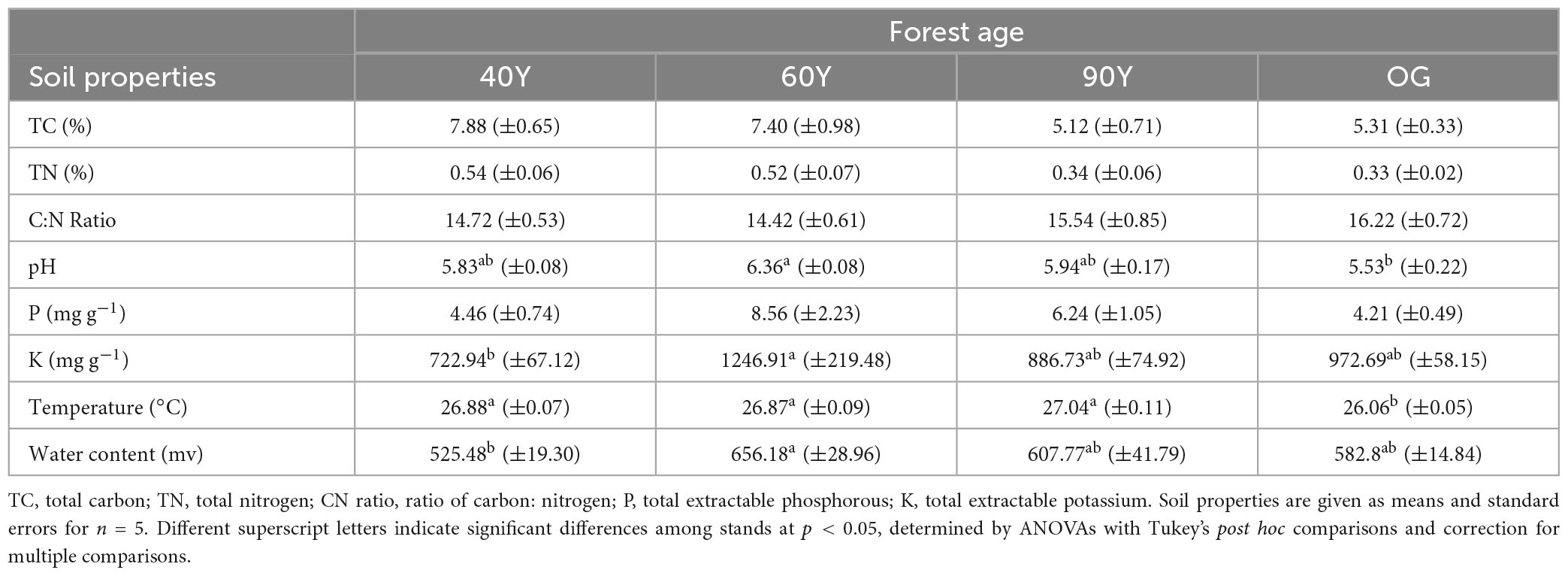
Table 4. Soil properties at 0–5 cm depth across an age gradient of forest stands in Panama, Central America, where 40Y is 40 year-old, 60Y is 60 year-old, 90Y is 90 year-old, OG is old-growth.
Multivariate analysis of soil properties demonstrated separation of stands in ordination space (perMANOVA R2 = 0.47, p < 0.001; Figure 6) and the first two ordination axes explained 49.2% of the total variation among stands (32.1 and 17.1% for PC1 and PC2, respectively). Total soil C and N, extractable P and soil temperature were strongly correlated with PC1, which explained the separation between the 60Y and 90Y stands, and between 40Y or 60Y and OG stands. Extractable K, water content and, to a lesser extent, pH were correlated with PC2, explaining the separation between 40Y, 90Y, and 60Y stands (Figure 6).
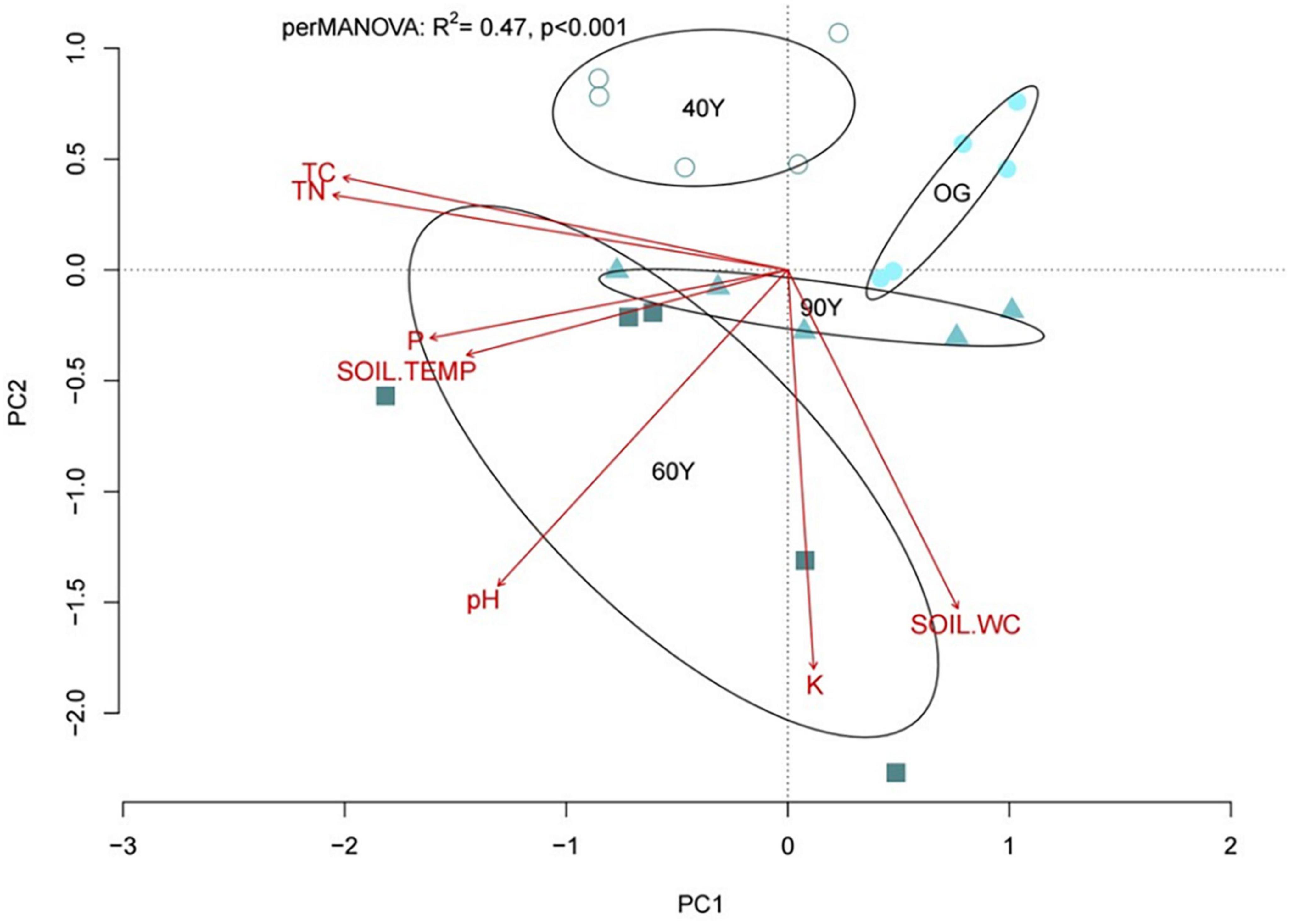
Figure 6. Ordination plot (principal component analysis) based on soil properties for 40-year old (40Y), 60-year old (60Y) and 90-year old (90Y) secondary forest stands and old-growth (OG) lowland tropical forest in Panama Central America. Ellipses show the separation of forest stands in ordination space based on 99% confidence limits based on standard errors; soil properties that explain the separation of stands in ordination space are shown as vectors, where P is soil extractable phosphorus, K is soil extractable potassium, SOIL.WC is soil water content, SOIL.TEMP is soil temperature, and TC and TN are total carbon and nitrogen concentration, respectively.
Linear regression of decay rates against the PC axis scores revealed that PC2 explained 22.4, 23.8, 44.1, and 48.7% of the variation in the decay rates of ACC [F(1,18) = 6.47, p = 0.002], DEC [F(1,18) = 6.93, p = 0.017], MIX [F(1,18) = 15.98, p < 0.001], and NAT [F(1,18) = 18.95, p < 0.001] litter treatments, respectively, indicating that litter decomposition was facilitated by high soil extractable K concentrations and high soil water content. By contrast, no variation in SRB was explained by soil properties.
4. Discussion
Our study aimed to elucidate how differences in tree functional composition influence soil C dynamics during secondary succession. We found higher nutrient content, decomposition rate and higher rates of respiration in the early stages of decomposition for the ACC species compared to DEC species litter, largely supporting our first hypothesis. However, we found limited evidence that C turnover rates in functionally diverse litter mixtures are higher than expected (H2), or that C turnover rates are higher in younger than in older forest stands (H3). Instead, we found a strong influence of forest stand on both litter decomposition and respiration rates, and whilst soil properties explained separation among stands, they only partially explained variation in decay rates. This suggests that soil C dynamics are determined by both soil properties and tree species traits.
4.1. Tree growth strategies influence litter decay via distinct foliar traits
The composition and turnover of functionally distinct litter mixtures largely conformed to expectations based on the differences in growth strategies between tree functional groups. As hypothesised, the ACC litter treatment representing early successional light-demanding species had higher nutrient concentrations and decomposed more rapidly than the DEC treatment which represented late-successional shade-tolerant trees (Table 2). The decay rates of ACC and DEC species litter in this study are comparable with the decay rates of “pioneer” and “old growth” species litter mixtures at a nearby study site (Laird-Hopkins et al., 2017), which supports the assumption that the ACC and DEC litter mixtures are largely representative of early and late successional stages, respectively.
Despite the overall differences in litter quality among treatments, there is nonetheless considerable variation in litter traits among species within broad functional groups (Hättenschwiler et al., 2008; Table 4). Although litter decay rates are often strongly related to foliar N (Wright et al., 2010), litter C:N ratios, or lignin content (Zhang et al., 2008; Hattenschwiler et al., 2011), our analyses revealed no differences in litter N or C:N ratios and only a small difference in ADF between ACC and DEC species. Although litter traits often correlate with living “green leaf” traits and both have been used successfully to predict decomposition, fresh leaf traits may better reflect plant growth strategies (Bakker et al., 2011), as nutrient reabsorption during leaf senescence can result in lower nutrient contents in litter compared to fresh leaves (Vergutz et al., 2012). Indeed, there were clear differences in the N content of fresh leaves between the ACC and DEC species we used in our study (Wright et al., 2010; Table 2). Since the experiment used a relatively small species pool, and because traits tend to be highly coordinated (e.g., Wright et al., 2004), it was not possible to formally test the influence of specific litter traits on decomposition rates. However, a fertilisation experiment conducted close to our study sites demonstrated that litter decomposition was enhanced by P addition, and the decomposition of cellulose (the primary constituent of leaf litter) increased with the addition of both P and K (Kaspari et al., 2008). Similarly, litter P and K content were shown to be more important than N in explaining the decomposition of natural mixed litter in a nearby forest stand (Kerdraon et al., 2020). Thus, the marked differences in litter P and K concentrations between ACC and DEC species could explain their distinct decay rates in our study. Our multivariate analyses also indicated that soil K concentrations were important for explaining differences in decay rates among stands. Potassium availability could influence decay rates because fungi actively accumulate K (Tyler, 2005), and K addition can stimulate fungal activity (Nottingham et al., 2018). Hence, the high soil K concentrations in the 60Y stands likely contributed to faster decay of leaf litter compared to the other stands.
Despite inter-specific variation in the litter traits of light-demanding and shade-tolerant species, the observed differences in litter quality clearly reflect the shift in resource investment of trees during secondary succession, from fast-growing acquisitive to slow-growing conservative strategies (Chazdon, 2014; Reich, 2014) and this was reflected in the faster decay rates of ACC litter and slower decomposition of DEC litter. Hence, in support of our first hypothesis, the litter properties and decay rates in the present study suggest that leaf traits related to the life-history strategy of tree functional groups have a considerable influence on litter decomposition.
4.2. Quality and decay rate of litter from different tree functional groups is reflected in distinct patterns of soil respiration during decomposition
Our study revealed a clear link between litter decay rates and soil respiration for functionally distinct litter treatments, but these become most apparent when comparing patterns of mass loss over time. Whereas in natural conditions there is a simultaneous accumulation and breakdown of plant material on the forest floor, we measured the decay rate and respiration rates for a representative mass of leaf litter without additional inputs. This not only allowed us to directly link decay rate to respiration, but also to compare patterns of respiration among functional litter treatments during distinct decomposition stages. The differences in respiration among the functional litter treatments during early- (June and July) and late- (September and October) stage decomposition suggest a strong influence of different litter types on soil microbial activity via the accessibility and availability of labile compounds from decaying plant material (e.g., Berg and McClaugherty, 2007). Hence, we propose that early nutrient release from the high-quality ACC litter boosted microbial activity in the first 2 months of decomposition.
Higher SRTOT from ACC species litter during the first 2 months of decomposition also indicates rapid loss of highly soluble compounds (Kutsch et al., 2009). Conversely, higher rates of SRTOT over DEC litter during the last 2 months of the study likely reflect the greater amount of substrate remaining, coupled with greater activity of slow-growing microbes capable of degrading tougher C compounds (Waldrop et al., 2000; Kutsch et al., 2009). Hence, the differences in SRTOT from ACC and DEC litter during early- and late-stage decomposition likely reflect the sequential breakdown and processing of labile C during decomposition (Kutsch et al., 2009; Powers et al., 2009). These processes are supported by the pattern of SRTOT observed from the MIX treatment, which first tracked that of the ACC litter during the first 2 months, and then matched the increasing SR from the DEC treatment in late-stage decomposition (Figure 3). Thus, in support of our first hypothesis, SRTOT was significantly higher from the ACC litter than from the DEC litter treatment in early decomposition, suggesting that light-demanding species litter provide a high-quality resource to microbial decomposers via the release of soluble C and nutrients.
Few studies have measured soil C turnover during secondary succession in tropical forests and they often report contrasting or no clear trends with stand age. For example, higher respiration rates were observed in older compared to younger secondary tropical forest in southern Mexico (Aryal et al., 2017) but this was only observed during a single season and across a relatively narrow age range of secondary forest (5–35 years old). Other studies report no clear relationships between respiration and forest age (e.g., Adachi et al., 2006; Schedlbauer and Kavanagh, 2008), although a global study found that respiration declined with increasing age in temperate forests (Pregitzer and Euskirchen, 2004). However, there was insufficient data to test the relationship across tropical forests, and while the authors attribute higher respiration in the younger temperate forests to disturbance in early succession, it is conceivable that changes in species traits likely contribute to these patterns (Pregitzer and Euskirchen, 2004).
4.3. Non-additive effects of the mixed litter treatment
To help resolve some of the uncertainty regarding the influence of functionally diverse litter mixtures on soil C dynamics, due in part to the paucity of data in tropical forests (Kou et al., 2020), we assessed non-additive effects of a mixed litter treatment on rates of litter decay and respiration in comparison to the component, functionally similar litter mixtures. Other studies which have assessed non-additive effects of functionally diverse litter mixtures on decomposition processes in tropical forests have variously reported synergistic (e.g., Trogisch et al., 2016; Getaneh et al., 2022), antagonistic (Laird-Hopkins et al., 2017), and mixed responses (Lin and Zeng, 2018), as well as purely additive effects (Scherer-Lorenzen et al., 2007). In our study, we hypothesised that the observed decay and respiration rates for the MIX litter treatment would be higher than predicted rates, but we found limited evidence for non-additive effects of the MIX litter treatment on litter decay rates. However, we observed a synergistic effect on respiration rates in the two youngest stands (Figure 5), suggesting that both stand environment and litter traits could influence non-additive effects during decomposition (e.g., Scherer-Lorenzen et al., 2007; Barantal et al., 2011). Interactions between litter mixture and stand environment could contribute to the large discrepancies among studies investigating non-additive effects, and the potential mechanisms merit further investigation.
We also investigated the potential for non-additive effects of litter mixtures on soil respiration rates, which has hitherto only been assessed in temperate systems (Wang et al., 2014; Mao et al., 2017). Synergistic non-additive effects of mixed species litter are expected to occur due to the transfer of nutrients between different litter types which can promote decomposition of more recalcitrant litter compounds, or as a result of greater variation in the physical microhabitat and decomposer interactions compared to single species litter (Hättenschwiler et al., 2005; Keiser et al., 2013). Differences in foliar nutrients (P, K, and Mg) between the constituent treatments in the MIX treatment (ACC and DEC; Table 2), and also between species in the ACC litter treatment (Table 2), suggest that multiple nutrient transfers between litter types may occur during decomposition, and thus may help explain non-additive effects of the MIX treatment on respiration rates. Interestingly there was a clear positive, non-additive effect of the mixed litter treatment on SRTOT in the two younger (40Y and 60Y) stands, a slight positive non-additive effect in the 90Y stand, but there was no effect in the OG stand. Our findings thus provide further evidence that the wider forest stand influenced the non-additive effects of the functionally diverse litter on the decomposer community (e.g., Scherer-Lorenzen et al., 2007; Barantal et al., 2011), and suggest that differences in soil vs. litter nutrient concentrations as a potential underlying mechanism.
Surprisingly, the synergistic effect of the MIX litter treatment on SRTOT appeared to be largely uncoupled with litter decay rates, as only the 60Y stand showed a clear synergistic effect of MIX litter on both decay rates and respiration (Figures 4, 5). The synergistic effect of the MIX treatment on decomposition in the 60Y stand could be partly explained by the overall high C turnover rates in this stand, demonstrated by comparably fast litter decomposition and high respiration rates (e.g., for the MIX and DEC treatments). Interestingly, a study across a chronosequence of secondary (10–80 years old) and old growth tropical forest in Puerto Rica reported that decomposition of a common litter was generally fastest in the 60 and 80 year old stands, which was attributed to more favourable microsites for decomposition (Ostertag et al., 2008). Thus, the high C turnover and strong synergistic effects we observed in the 60Y site likely arise from favourable microclimate as well as higher soil concentrations of potentially limiting elements such as K.
It is conceivable that non-additive effects from a functionally diverse litter mixture may be more pronounced in stands with higher microbial activity as a result of a more diverse and complex decomposer community, adapted to consume a wide range of C compounds (Van Der Heijden et al., 2008). Another potential explanation is that soil K was significantly higher in the 60Y than 40Y stand (Table 4) perhaps suggesting that nutrient exchange between the soil and litter enhanced the positive non-additive effect in this stand. It is noteworthy that the influence of soil properties (represented by PC2 in our study) on litter decomposition was strongest for the MIX and NAT litter treatments, which could indicate stronger environmental controls on decomposition for functionally diverse leaf litter. Such interactions between soil nutrient availability and litter diversity could explain why experiments investigating non-additive effects of litter mixtures have produced such variable results (Kou et al., 2020). We therefore suggest that to fully understand how diversity influences decomposition, future experiments will need to assess the combined influence of soil properties and species diversity on decomposition processes.
Interestingly, although we observed a clear temporal response of SRTOT to rates of litter decomposition between early and late stage decomposition, along with higher rates of decomposition in the 60Y stand compared to the 40Y and 90Y stands, the non-additive effects on litter decomposition in our study did not confirm to recent findings from Kou et al. (2020), whereby synergistic effects were observed more strongly in early decomposition (from 10 to 40% mass loss) and declined as decomposition progressed. By contrast, we observed the largest synergistic effect in litter at the end of the experiment (> 60% mass loss, Figure 4). Stronger synergistic effects in the earlier stages of decomposition are expected to occur when mass loss is dominated by leaching rather than microbial processes (Kou et al., 2020). However, in our study, the synergistic effect observed in the 60Y stand in the later stages of decomposition instead suggests that the ability of the microbial community to process functionally diverse compounds in litter shapes decomposition in this forest.
Our results reveal that there were clear non-additive effects on decomposition of the mixed litter treatment, suggesting that measurements comparing litter from a single species or from functionally similar species do not fully capture the complex interactions between plant litter traits and decomposer communities which drive soil C dynamics. Instead, we propose that assessing the decay rate and respiration rates of a standardised, functionally mixed litter treatment containing litter from a range of tree functional groups would more closely represent natural conditions and better reveal the influence of tree functional characteristics on soil C dynamics in highly diverse tropical forest stands.
4.4. Strong influence of forest stand on soil carbon dynamics
We hypothesised that soil carbon turnover would be higher in the younger than older forest stands due to the greater relative influence of light-demanding tree species providing a high-quality resource to microbial decomposers. Although there was a clear separation in SRTOT between the two younger and two older stands measured over the functionally diverse MIX litter treatment, we found limited evidence for declining soil C turnover with stand age. A study in a subtropical forest in China found that litter decomposition of an abundant canopy species decreased significantly with increasing stand age (Trogisch et al., 2016), although the pattern was not observed for mixed species litter. The authors propose this was driven by stand and microclimate characteristics related to succession. In our study, the strong influence of forest stand on both litter decomposition and soil respiration was partially explained by measured soil characteristics.
Soil C turnover is strongly linked to plant growth rates and the quality of plant inputs (Schlessinger and Andrews, 2000; De Deyn et al., 2008). The relative influence of shade-tolerant (DEC) species increased with forest age across the four stands (Table 1), and previous work revealed that soil C stocks at 0–10 cm increased with the increasing influence of light-demanding (ACC) species in these forest stands (Wallwork et al., 2022). As such, we expected that C turnover would be fastest in younger stands, resulting in higher rates of litter decay and total respiration compared to the older forest stands. However, in contrast to our hypothesis, variation in decay rates and SR was not explained by shifts in tree functional groups among forest age classes, as the highest rates of decay and SRTOT were recorded in the 60Y stand, and the lowest in the 90Y stand. Although we did not find a clear relationship between C turnover and the increasing relative influence of shade-tolerant tree species at a stand level, it is possible that rates of litter decomposition and respiration were influenced by dominant species at a more local scale (e.g., Joly et al., 2017). For example, the “homefield advantage” theory stipulates that litter decomposes more rapidly in proximity to the tree species it derives from due to specialisation of the soil microbial community (Ayres et al., 2009; Kerdraon et al., 2019). While we did not formally assess the influence of trees in direct proximity to the mesocosms in each block, the relative influence of our four target tree species within a 20-m radius of soil mesocosms was highest in the 60Y stand and lowest in the 90Y stand, potentially explaining some of the variation in C turnover between these stands. Additionally, small-scale heterogeneity of forest structure and soil properties can help explain variation in litter decomposition due to variations in microhabitat, although the direction of the relationship can vary among studies. For example, litter decomposition increased with tree density, DBH and basal area at one an old-growth tropical forest site (de Godoy Fernandes et al., 2021), whereas decomposition declined with tree density but increased with soil nutrient concentrations (including P, K, Ca, and Mg) at another site (Oliveira et al., 2019).
Although there were no clear relationships between individual soil properties and rates of litter decomposition or soil respiration in our study, there was a clear separation of stands based on multiple soil properties and these helped explain some of the variation in rates of litter decomposition among stands. Although our analyses indicated no difference among stands in soil C and N concentration at 0–5 cm depth, previous studies revealed that soil C and N stocks at 0–10 cm depth were higher in the 40Y and 60Y stands (Jones et al., 2019; Wallwork et al., 2022), which could contribute to the higher rates of SR by sustaining greater microbial biomass (Fierer et al., 2009). Correspondingly, the lower rates of decay and SR in the 90Y stand coincide with lower total soil N content compared to all other stands (Jones et al., 2019; Wallwork et al., 2022). In addition, soil pH exerts a strong influence on soil microbial community composition (Fierer and Jackson, 2006; Rousk et al., 2009), which in turn controls rates of C turnover (Schimel and Schaeffer, 2012). Although we found only a weak relationship between soil pH and litter decomposition, soil pH was significantly higher in the 60Y compared to 90Y stand, and, coupled with greater soil water content in during dry periods could also explain the high rates of litter decay and SR in the 60Y stand.
Given the strong links between soil properties and tree functional composition at our study sites (Wallwork et al., 2022) it is not possible to disentangle the influence of tree functional composition on soil C turnover from the influence of soil properties, however, it is clear that both act in concert to shape decomposition processes during secondary forest succession.
5. Conclusion
Our study presents an integrated assessment of soil C dynamics during litter decomposition in regenerating tropical forests, measuring both the decay rate and derived respiration rate of functionally different litter mixtures across a successional gradient of tropical forest. We found that multiple, interacting factors influence patterns of soil C turnover during decomposition. Although rates of litter decay and soil respiration did not follow the expected trajectory of forest successional age, there was a clear separation in C turnover between functionally different litter treatments, whereby litter from light-demanding “ACC” species decomposed more rapidly than litter from shade-tolerant “DEC” species, and these distinct decay rates influenced soil respiration at different stages of decomposition. Surprisingly, despite stands being of the same climate, ecosystem, and relative proximity to one another, both litter decay rates and soil respiration were strongly influenced by forest stand, which was only partially explained by soil properties.
Our study thus demonstrates that changes in tree species composition can influence soil carbon dynamics in regenerating tropical forests via differences in leaf litter quality and decay rates. We also highlight the potential importance of functionally diverse plant inputs for soil microbial activity in tropical forests and that local stand-level characteristics may be of equal importance in explaining variation in rates of carbon turnover in these forests. We suggest that future work on the links between litter traits, soil properties and soil microbial communities could further clarify the role of functional diversity in soil C dynamics and storage during secondary tropical forest succession.
Data availability statement
The raw data supporting the conclusions of this article will be made available by the authors, without undue reservation.
Author contributions
ES, LB, DD, US, and AW conceived the ideas and designed methodology. AW, BC-T, and DK collected the data. AW and ES analysed the data. AW led the writing of the manuscript. AW, ES, LB, DD, US, and DK contributed critically to the drafts and gave final approval for publication. All authors contributed to the article and approved the submitted version.
Funding
AW was funded by a NERC ENVISION DTP Studentship, and ES was funded by a European Research Council Starting Grant under the European Union’s Seventh Framework Programme (FP/2007-2013), ERC Grant Agreement No. 307888.
Acknowledgments
We would like to thank the staff at the Smithsonian Tropical Research Institute (STRI) in Panama for their valuable assistance, Laetitia Bréchet for her advice in the early stages of the study, and Annette Ryan for her support in the laboratory.
Conflict of interest
The authors declare that the research was conducted in the absence of any commercial or financial relationships that could be construed as a potential conflict of interest.
Publisher’s note
All claims expressed in this article are solely those of the authors and do not necessarily represent those of their affiliated organizations, or those of the publisher, the editors and the reviewers. Any product that may be evaluated in this article, or claim that may be made by its manufacturer, is not guaranteed or endorsed by the publisher.
Supplementary material
The Supplementary Material for this article can be found online at: https://www.frontiersin.org/articles/10.3389/ffgc.2023.1232694/full#supplementary-material
References
Adachi, M., Bekku, Y., Rashidah, W., Okuda, T., and Koizumi, H. (2006). Differences in soil respiration between different tropical ecosystems. Appl. Soil Ecol. 34, 258–265. doi: 10.1016/j.apsoil.2006.01.006
Anderson, M. J. (2017). “Permutational multivariate analysis of variance (PERMANOVA),” in Wiley StatsRef: Statistics reference online, eds W. W. Piegorsch, R. A. Levine, H. Zhang, and T. Lee (Hoboken, NJ: Wiley), 1–15. doi: 10.1002/9781118445112.stat07841
Anderson-Teixeira, K. J., Herrmann, V., Morgan, R., Bond-Lamberty, B., Cook-Patton, S., and Ferson, A. (2021). Carbon cycling in mature and regrowth forests globally. Environ. Res. Lett. 16:053009. doi: 10.1088/1748-9326/abed01
Aryal, D. R., De Jong, B., Ochoa-Gaona, S., Esparza-Olguin, L., and Mendoza-Vega, J. (2017). “Soil organic carbon stocks and soil respiration in tropical secondary forests in Southern Mexico,” in Global soil security. Progress in soil science, eds D. J. Field, C. L. S. Morgan, and A. B. McBratney (Cham: Springer), 153–165. doi: 10.1007/978-3-319-43394-3_14
Ayres, E., Steltzer, H., Simmons, B., Simpson, R., Steinweg, J., Wallenstein, M., et al. (2009). Home-field advantage accelerates leaf litter decomposition in forests. Soil Biol. Biochem. 41, 606–610. doi: 10.1016/j.soilbio.2008.12.022
Baillie, I., Elsenbeer, H., Barthold, F., Grimm, R., and Stallard, R. (2007). Semi-detailed soil survey of Barro Colorado Island, Panama. Panama City: Smithsonian Tropical Research Institute.
Bakker, M. A., Carreño-Rocabado, G., and Poorter, L. (2011). Leaf economics traits predict litter decomposition of tropical plants and differ among land use types. Funct. Ecol. 25, 473–483. doi: 10.1111/j.1365-2435.2010.01802.x
Barantal, S., Roy, J., Fromin, N., Schimann, H., and Hättenschwiler, S. (2011). Long-term presence of tree species but not chemical diversity affect litter mixture effects on decomposition in a neotropical rainforest. Oecologia 167, 241–252. doi: 10.1007/s00442-011-1966-4
Bates, D., Mächler, M., Bolker, B., and Walker, S. (2015). Fitting linear mixed-effects models using lme4. J. Stat. Softw. 67, 1–48. doi: 10.18637/jss.v067.i01
Berg, B., and McClaugherty, C. (2007). Plant litter: Decomposition, humus formation, carbon sequestration, 2nd Edn. Berlin: Springer Berlin Heidelberg.
Bréchet, L., Le Dantec, V., Ponton, S., Goret, J., Sayer, E., and Bonal, D. (2017). Short- and long-term influence of litter quality and quantity on simulated heterotrophic soil respiration in a lowland tropical forest. Ecosystems 20, 1190–1204. doi: 10.1007/s10021-016-0104-x
Chazdon, R. L. (2014). Second growth: The promise of tropical forest regeneration in an age of deforestation. Chicago: The University of Chicago Press, doi: 10.1017/CBO9781107415324.004
Chen, X., Chen, H., Chen, C., Ma, Z., Searle, E., and Yu, Z. (2020). Effects of plant diversity on soil carbon in diverse ecosystems: A global meta-analysis. Biol. Rev. 95, 167–183. doi: 10.1111/brv.12554
Chomel, M., Guittonny, M., Fernandez, C., Gallet, C., Desrochers, A., and Paré, D. (2016). Plant secondary metabolites: A key driver of litter decomposition and soil nutrient cycling. J. Ecol. 104, 1527–1541. doi: 10.1111/1365-2745.12644
Conti, G., and Díaz, S. (2013). Plant functional diversity and carbon storage - an empirical test in semi-arid forest ecosystems. J. Ecol. 101, 18–28. doi: 10.1111/1365-2745.12012
Cornelissen, J. H. C., Pérez Harguindeguy, N., Díaz, S., Grime, N., Marzano, B., and Cabido, M. (1999). Leaf structure and defence control litter decomposition rate across species and life forms in regional floras on two continents. New Phytol. 143, 191–200. doi: 10.1046/j.1469-8137.1999.00430.x
Cornwell, W. K., Cornelissen, J., Amatangelo, K., Dorrepaal, E., Eviner, V., and Godoy, O. (2008). Plant species traits are the predominant control on litter decomposition rates within biomes worldwide. Ecol. Lett. 11, 1065–1071. doi: 10.1111/j.1461-0248.2008.01219.x
Cortez, J., Garnier, E., Pérez-Harguindeguy, N., Debussche, M., and Gillon, D. (2007). Plant traits, litter quality and decomposition in a Mediterranean old-field succession. Plant Soil 296, 19–34. doi: 10.1007/s11104-007-9285-6
Cotrufo, M. F., Wallenstein, M. D., and Boot, C. M. (2013). The microbial efficiency-matrix stabilization (MEMS) framework integrates plant litter decomposition with soil organic matter stabilization: Do labile plant inputs form stable soil organic matter? Glob. Change Biol. 19, 988–995. doi: 10.1111/gcb.12113
Coûteaux, M. M., Bottner, P., and Berg, B. (1995). Litter decomposition, climate and liter quality. Trends Ecol. Evol. 10, 63–66. doi: 10.1016/S0169-5347(00)88978-8
De Deyn, G. B., Cornelissen, J. H. C., and Bardgett, R. D. (2008). Plant functional traits and soil carbon sequestration in contrasting biomes. Ecol. Lett. 11, 516–531. doi: 10.1111/j.1461-0248.2008.01164.x
de Godoy Fernandes, P. H., de Souza, A. L. T., Tanaka, M. O., and Sebastiani, R. (2021). Decomposition and stabilization of organic matter in an old-growth tropical riparian forest: Effects of soil properties and vegetation structure. For. Ecosyst. 8:13. doi: 10.1186/s40663-021-00293-0
Dent, D. H., DeWalt, S. J., and Denslow, J. S. (2013). Secondary forests of central Panama increase in similarity to old-growth forest over time in shade tolerance but not species composition. J. Veg. Sci. 24, 530–542. doi: 10.1111/j.1654-1103.2012.01482.x
Don, A., Schumacher, J., and Freibauer, A. (2011). Impact of tropical land-use change on soil organic carbon stocks – a meta-analysis. Glob. Change Biol. 17, 1658–1670. doi: 10.1111/j.1365-2486.2010.02336.x
Fanin, N., Hättenschwiler, S., Barantal, S., Schimann, H., and Fromin, N. (2011). Does variability in litter quality determine soil microbial respiration in an Amazonian rainforest? Soil Biol. Biochem. 43, 1014–1022. doi: 10.1016/j.soilbio.2011.01.018
Fierer, N., and Jackson, R. B. (2006). The diversity and biogeography of soil bacterial communities. Proc. Natl. Acad. Sci. U.S.A. 103, 626–631.
Fierer, N., Strickland, M., Liptzin, D., Bradford, M., and Cleveland, C. (2009). Global patterns in belowground communities. Ecol. Lett. 12, 1238–1249. doi: 10.1111/j.1461-0248.2009.01360.x
Gartner, T. B., and Cardon, Z. G. (2004). Decomposition dynamics in mixed-species leaf litter. Oikos 2, 230–246.
Gessner, M. O., Swan, C., Dang, C., McKie, B., Bardgett, R., and Wall, D. (2010). Diversity meets decomposition. Trends Ecol. Evol. 25, 372–380. doi: 10.1016/j.tree.2010.01.010
Getaneh, S., Honnay, O., Desie, E., Helsen, K., Couck, L., and Shibru, S. (2022). Impact of tree litter identity, litter diversity and habitat quality on litter decomposition rates in tropical moist evergreen forest. For. Ecosyst. 9:100023. doi: 10.1016/j.fecs.2022.100023
Grimm, R., Behrens, T., Märker, M., and Elsenbeer, H. (2008). Soil organic carbon concentrations and stocks on Barro Colorado Island — Digital soil mapping using random forests analysis. Geoderma 146, 102–113. doi: 10.1016/j.geoderma.2008.05.008
Hättenschwiler, S., Aeschlimann, B., Coûteaux, M., Roy, J., and Bonal, D. (2008). High variation in foliage and leaf litter chemistry among 45 tree species of a neotropical rainforest community. New Phytol. 179, 165–175. doi: 10.1111/j.1469-8137.2008.02438.x
Hattenschwiler, S., Coq, S., Barantal, S., and Handa, I. (2011). Leaf traits and decomposition in tropical rainforests: Revisiting some commonly held views and towards a new hypothesis. New Phytol. 189, 950–965. doi: 10.1111/j.1469-8137.2010.03483.x
Hättenschwiler, S., Tiunov, A. V., and Scheu, S. (2005). Biodiversity and litter decomposition in terrestrial ecosystems. Annu. Rev. Ecol. Evol. Syst. 36, 191–218. doi: 10.1146/annurev.ecolsys.36.112904.151932
Jewell, M. D., Shipley, B., Low-Decarie, E., and Tobner, C. (2017). Partitioning the effect of composition and diversity of tree communities on leaf litter decomposition and soil respiration. Oikos 126, 959–971. doi: 10.1111/oik.03868
Johnson, E., and Kiyoko, M. (2008). Testing the assumptions of chronosequences in succession. Ecol. Lett. 11, 419–431. doi: 10.1111/j.1461-0248.2008.01173.x
Joly, F. X., Milcu, A., Scherer-Lorenzen, M., Jean, L., Bussotti, F., and Dawud, S. (2017). Tree species diversity affects decomposition through modified micro-environmental conditions across European forests. New Phytol. 214, 1281–1293. doi: 10.1111/nph.14452
Jones, I. L., DeWalt, S., Lopez, O., Bunnefeld, L., Pattison, Z., and Dent, D. (2019). Above- and belowground carbon stocks are decoupled in secondary tropical forests and are positively related to forest age and soil nutrients respectively. Sci. Total Environ. 697:133987. doi: 10.1016/j.scitotenv.2019.133987
Kaspari, M., Garcia, M., Harms, K., Santana, M., Wright, S., and Yavitt, J. (2008). Multiple nutrients limit litterfall and decomposition in a tropical forest. Ecol. Lett. 11, 35–43.
Keiser, A. D., Knoepp, J. D., and Bradford, M. A. (2013). Microbial communities may modify how litter quality affects potential decomposition rates as tree species migrate. Plant Soil 372, 167–176. doi: 10.1007/s11104-013-1730-0
Kerdraon, D., Drewer, J., Castro, B., Wallwork, A., Hall, J., and Sayer, E. (2019). Litter traits of native and non-native tropical trees influence soil carbon dynamics in timber plantations in Panama. Forests 10:209. doi: 10.3390/f10030209
Kerdraon, D., Drewer, J., Chung, A., Majalap, N., Slade, E., Bréchet, L., et al. (2020). Litter inputs, but not litter diversity, maintain soil processes in degraded tropical forests—A cross-continental comparison. Front. For. Glob. Change 2:90. doi: 10.3389/ffgc.2019.00090
Kou, L., Jiang, L., Hättenschwiler, S., Zhang, M., Niu, S., and Fu, X. (2020). Diversity-decomposition relationships in forests worldwide. eLife 9, 1–51. doi: 10.7554/eLife.55813
Kröber, W., Böhnke, M., Welk, E., Wirth, C., and Bruelheide, H. (2012). Leaf trait-environment relationships in a subtropical broadleaved forest in South-East China. PLoS One 7:e0035742. doi: 10.1371/journal.pone.0035742
Kutsch, W. L., Bahn, M., and Heinemeyer, A. (eds) (2009). Soil carbon dynamics: An integrated methodology. Cambridge, MA: Cambridge University Press, doi: 10.1017/CBO9780511711794
Kuznetsova, A., Brockhoff, P. B., and Christensen, R. H. B. (2017). lmerTest Package: Tests in linear mixed effects models. J. Stat. Softw. 82, 1–26. doi: 10.18637/jss.v082.i13
Kuzyakov, Y. (2006). Sources of CO2 efflux from soil and review of partitioning methods. Soil Biol. Biochem. 38, 425–448. doi: 10.1016/j.soilbio.2005.08.020
Laird-Hopkins, B. C., Brechet, L., Trujillo, B., and Sayer, E. (2017). Tree functional diversity affects litter decomposition and arthropod community composition in a tropical forest. Biotropica 49, 903–911. doi: 10.1111/btp.12477
Li, D., Niu, S., and Luo, Y. (2012). Global patterns of the dynamics of soil carbon and nitrogen stocks following afforestation: A meta-analysis. New Phytol. 195, 172–181. doi: 10.1111/j.1469-8137.2012.04150.x
Lin, G., and Zeng, D. H. (2018). Functional identity rather than functional diversity or species richness controls litter mixture decomposition in a subtropical forest. Plant Soil 428, 179–193. doi: 10.1007/s11104-018-3669-7
Mao, B., Mao, R., and Zeng, D. (2017). Species diversity and chemical properties of litter influence non-additive effects of litter mixtures on soil carbon and nitrogen cycling. PLoS One 12:e0180422. doi: 10.1371/journal.pone.0180422
Marín-Spiotta, E., and Sharma, S. (2013). Carbon storage in successional and plantation forest soils: A tropical analysis. Glob. Ecol. Biogeogr. 22, 105–117. doi: 10.1111/j.1466-8238.2012.00788.x
Martin, P., Bullock, J., and Newton, A. (2013). Carbon pools recover more rapidly than plant biodiversity in secondary tropical forests. Philos. Trans. R. Soc. B 280, 1–8. doi: 10.1098/rspb.2013.2236
Nottingham, A., Hicks, L., Ccahuana, A., Salinas, N., Bååth, E., Meir, P., et al. (2018). Nutrient limitations to bacterial and fungal growth during cellulose decomposition in tropical forest soils. Biol. Fertil. Soils 54, 219–228. doi: 10.1007/s00374-017-1247-4
Oksanen, J., Simpson, G., Blanchet, F., Kind, R., Legendre, P., and Minchin, P. (2008). The vegan package. Community ecology package. 190. Available online at: https://cran.r-project.org/web/packages/vegan/vegan.pdf (accessed November 11, 2016).
Oliveira, R. A. C., Marques, R., and Marques, M. C. M. (2019). Plant diversity and local environmental conditions indirectly affect litter decomposition in a tropical forest. Appl. Ecol. 134, 45–53. doi: 10.1016/j.apsoil.2018.09.016
Olson, J. S. (1963). Energy storage and the balance of producers and decomposers in ecological systems. Ecology 44, 322–331. doi: 10.2307/1932179
Ostertag, R., Marín-Spiotta, E., Silver, W., and Schulten, J. (2008). Litterfall and decomposition in relation to soil carbon pools along a secondary forest chronosequence in Puerto Rico. Ecosystems 11, 701–714. doi: 10.1007/s10021-008-9152-1
Pan, Y., Birdsey, R., Fang, J., Houghton, R., Kauppi, P., Kurz, W., et al. (2011). A large and persistent carbon sink in the world’s forests. Science 333, 988–993. doi: 10.1126/science.1201609
Poorter, L., Bongers, F., Aide, T., Zambrano, A., Balvanera, P., Becknell, J., et al. (2016). Biomass resilience of Neotropical secondary forests. Nature 530, 211–214. doi: 10.1038/nature16512
Powers, J., Montgomery, R., Adair, E., Brearley, F., DeWalt, S., Castanho, C., et al. (2009). Decomposition in tropical forests: A pan-tropical study of the effects of litter type, litter placement and mesofaunal exclusion across a precipitation gradient. J. Ecol. 97, 801–811. doi: 10.1111/j.1365-2745.2009.01515.x
Powers, J. S., and Marín-Spiotta, E. (2017). Ecosystem processes and biogeochemical cycles in secondary tropical forest succession. Annu. Rev. Ecol. Evol. Syst. 48, 497–519. doi: 10.1146/annurev-ecolsys-110316-022944
Pregitzer, K. S., and Euskirchen, E. S. (2004). Carbon cycling and storage in world forests: Biome patterns related to forest age. Glob. Change Biol. 10, 2052–2077. doi: 10.1111/j.1365-2486.2004.00866.x
Prescott, C. E., and Grayston, S. J. (2013). Forest ecology and management tree species influence on microbial communities in litter and soil: Current knowledge and research needs. For. Ecol. Manag. 309, 19–27. doi: 10.1016/j.foreco.2013.02.034
Pugh, T., Lindeskog, M., Smith, B., Poulter, B., Arneth, A., Haverd, V., et al. (2019). Role of forest regrowth in global carbon sink dynamics. Proc. Natl. Acad. Sci. U.S.A. 116, 4382–4387. doi: 10.1073/pnas.1810512116
R Core Team (2018). R: A language and environment for statistical computing. Vienna: R Foundation for Statistical Computing.
Raich, J. W., and Schlesinger, W. H. (1992). The global carbon dioxide flux in soil respiration and its relationship to vegetation and climate. Tellus 44, 81–99. doi: 10.1034/j.1600-0889.1992.t01-1-00001.x
Reich, P. B. (2014). The world-wide “fast-slow” plant economics spectrum: A traits manifesto. J. Ecol. 102, 275–301. doi: 10.1111/1365-2745.12211
Rousk, J., Brookes, P. C., and Bååth, E. (2009). Contrasting soil pH effects on fungal and bacterial growth suggest functional redundancy in carbon mineralization. Appl. Environ. Microbiol. 75, 1589–1596. doi: 10.1128/AEM.02775-08
Rüger, N., Huth, A., Hubbell, S., and Condit, R. (2009). Response of recruitment to light availability across a tropical lowland rain forest community. J. Ecol. 97, 1360–1368. doi: 10.1111/j.1365-2745.2009.01552.x
Santiago, L. S. (2007). Extending the leaf economics spectrum to decomposition: Evidence from a tropical forest. Ecology 88, 1126–1131. doi: 10.1890/06-1841
Sayer, E. J., and Tanner, E. V. J. (2010). Experimental investigation of the importance of litterfall in lowland semi-evergreen tropical forest nutrient cycling. J. Ecol. 98, 1052–1062. doi: 10.1111/j.1365-2745.2010.01680.x
Schedlbauer, J. L., and Kavanagh, K. L. (2008). Soil carbon dynamics in a chronosequence of secondary forests in northeastern Costa Rica. For. Ecol. Manag. 255, 1326–1335.
Scherer-Lorenzen, M., Bonilla, J. L., and Potvin, C. (2007). Tree species richness affects litter production and decomposition rates in a tropical biodiversity experiment. Oikos 116, 2108–2124. doi: 10.1111/j.2007.0030-1299.16065.x
Schimel, J. P., and Schaeffer, S. M. (2012). Microbial control over carbon cycling in soil. Front. Microbiol. 3:348. doi: 10.3389/fmicb.2012.00348
Schlessinger, W., and Andrews, J. A. (2000). Soil respiration and the global carbon cycle. Biogeochemistry 48, 7–20. doi: 10.1023/A:1006247623877
Szefer, P., Carmona, C., Chmel, K., Konečná, M., Libra, M., Molem, K., et al. (2017). Determinants of litter decomposition rates in a tropical forest: Functional traits, phylogeny and ecological succession. Oikos 126, 1101–1111. doi: 10.1111/oik.03670
Trogisch, S., He, J., Hector, A., and Scherer-Lorenzen, M. (2016). Impact of species diversity, stand age and environmental factors on leaf litter decomposition in subtropical forests in China. Plant Soil 400, 337–350. doi: 10.1007/s11104-015-2737-5
Tyler, G. (2005). Changes in the concentrations of major, minor and rare-earth elements during leaf senescence and decomposition in a Fagus sylvatica forest. For. Ecol. Manag. 206, 167–177. doi: 10.1016/j.foreco.2004.10.065
Van Der Heijden, M. G. A., Bardgett, R. D., and Van Straalen, N. M. (2008). The unseen majority: Soil microbes as drivers of plant diversity and productivity in terrestrial ecosystems. Ecol. Lett. 11, 296–310. doi: 10.1111/j.1461-0248.2007.01139.x
van der Sande, M., Powers, J., Kuyper, T., Norden, N., Negret, B., Almeida, J., et al. (2023). Soil resistance and recovery during neotropical forest succession. Philos. Trans. R. Soc. B Biol. Sci. 378:20210074. doi: 10.1098/rstb.2021.0074
Van Soest, P. J., Robertson, J. B., and Lewis, B. A. (1992). Methods for dietary fiber, neutral detergent fiber, and nonstarch polysaccharides in relation to animal nutrition. J. Dairy Sci. 74, 3583–3597. doi: 10.3168/jds.S0022-0302(91)78551-2
Vergutz, L., Manzoni, S., Porporato, A., Novais, R., and Jackson, R. (2012). Global resorption efficiencies and concentrations of carbon and nutrients in leaves of terrestrial plants. Ecol. Monogr. 82, 205–220. doi: 10.1890/11-0416.1
Waldrop, M. P., Balser, T. C., and Firestone, M. K. (2000). Linking microbial community composition to function in a tropical soil. Soil Biol. Biochem. 32, 1837–1846. doi: 10.1016/S0038-0717(00)00157-7
Walker, L., Wardle, D., Bardgett, R., and Clarkson, B. (2010). The use of chronosequences in studies of ecological succession and soil development. J. Ecol. 98, 725–736. doi: 10.1111/j.1365-2745.2010.01664.x
Wallwork, A., Banin, L., Dent, D., Skiba, U., and Sayer, E. (2022). Soil carbon storage is related to tree functional composition in naturally regenerating tropical forests. Funct. Ecol. 36, 3175–3187. doi: 10.1111/1365-2435.14221
Wang, Q., Yu, Y., He, T., and Wang, Y. (2017). Aboveground and belowground litter have equal contributions to soil CO2 emission: An evidence from a 4-year measurement in a subtropical forest. Plant Soil 421, 7–17. doi: 10.1007/s11104-017-3422-7
Wang, Y., Fang, S., Chang, S., and Tian, Y. (2014). Non-additive effects of litter-mixing on soil carbon dioxide efflux from poplar-based agroforestry systems in the warm temperate region of China. Agrofor. Sys. 88, 193–203. doi: 10.1007/s10457-013-9665-2
Whitfeld, T., Lasky, J., Damas, K., Sosanika, G., Molem, K., Montgomery, R., et al. (2014). Species richness, forest structure, and functional diversity during succession in the New Guinea lowlands. Biotropica 46, 538–548. doi: 10.1111/btp.12136
Windsor, D. M. (1990). Climate and moisture variability in a tropical forest: Long-term Records from BCI. Available online at: https://repository.si.edu/handle/10088/811 (accessed October 23, 2015).
Wright, I., Reich, P., Westoby, M., Ackerly, D., Baruch, Z., Bongers, F., et al. (2004). The worldwide leaf economics spectrum. Nature 12, 821–827.
Wright, S., Kitajima, K., Kraft, N., Reich, P., Wright, I., Bunker, D., et al. (2010). Functional traits and the growth – mortality trade-off in tropical trees. Ecology 91, 3664–3674.
Wright, S. J. (2002). Plant diversity in tropical forests: A review of mechanisms of species coexistence. Oecologia 130, 1–14. doi: 10.1007/s004420100809
Yang, Y., Luo, Y., and Finzi, A. C. (2011). Carbon and nitrogen dynamics during forest stand development: A global synthesis. New Phytol. 190, 977–989. doi: 10.1111/j.1469-8137.2011.03645.x
Yavitt, J. B. (2000). Nutrient dynamics of soil derived from different parent material on Barro Colorado Island. Panama’. Biotropica 32, 198–207.
Keywords: light-demanding species, litter decomposition, secondary tropical forests, secondary succession, shade-tolerant species, soil carbon, soil respiration, tree functional groups
Citation: Wallwork A, Castro-Trujillo B, Banin LF, Dent DH, Skiba U, Kerdraon D and Sayer EJ (2023) Soil carbon dynamics are linked to tree species growth strategy in a naturally regenerating tropical forest. Front. For. Glob. Change 6:1232694. doi: 10.3389/ffgc.2023.1232694
Received: 01 June 2023; Accepted: 21 July 2023;
Published: 08 August 2023.
Edited by:
Shalom D. Addo-Danso, Forestry Research Institute of Ghana, GhanaReviewed by:
Akwasi Duah-Gyamfi, Council for Scientific and Industrial Research (CSIR), GhanaNing Chen, Lanzhou University, China
Copyright © 2023 Wallwork, Castro-Trujillo, Banin, Dent, Skiba, Kerdraon and Sayer. This is an open-access article distributed under the terms of the Creative Commons Attribution License (CC BY). The use, distribution or reproduction in other forums is permitted, provided the original author(s) and the copyright owner(s) are credited and that the original publication in this journal is cited, in accordance with accepted academic practice. No use, distribution or reproduction is permitted which does not comply with these terms.
*Correspondence: Abby Wallwork, a.wallwork@lancaster.ac.uk