Hybridization in heliothine moths: impacts on reproduction, pheromone communication, and pest management
- 1Department of Biology, Acadia University, Wolfville, NS, Canada
- 2Bio-Agriculture Laboratory, The Verschuren Centre for Sustainability in Energy and The Environment, Sydney, NS, Canada
Heliothine moths (Family Noctuidae : Subfamily Heliothinae) are ubiquitous crop pests with three documented species combinations known to hybridize: Helicoverpa zea x Helicoverpa armigera, H. armigera x Helicoverpa assulta, and Heliothis virescens x Heliothis subflexa. Hybrids can have advantageous traits, such as increased host range, resistance to insecticides, and increased mating success, posing serious threats to agriculture. However, deleterious traits of hybrids, such as developmental abnormalities or sterility, can be exploited for pest management. In this review, the characteristics of F1 hybrids and backcrosses are examined through a historical lens. Topics reviewed include developmental characteristics, sex pheromone synthesis and perception, mating and calling behavior, sperm morphology, gene expression, electrophysiological responses, structures of the central and peripheral nervous systems, hybrid sterility, and applications in pest control. Recommendations for future studies based on existing gaps of knowledge are given, as are proposed pest management strategies.
Introduction
Moths of the subfamily Heliothinae, often referred to as heliothine moths or heliothines, are highly cosmopolitan, with nearly 400 species identified across Africa, Asia, Europe, Oceania, and the Americas to date (Cho et al., 2008). Multiple species exist in overlapping geographic ranges, and where sympatry occurs, both prezygotic and postzygotic mating barriers may be present, resulting in reproductive isolation (Hardwick, 1965; Proshold and Lachance, 1974). While prezygotic mechanisms prevent interspecific mating, post-zygotic mechanisms such as hybrid inviability or sterility are only effective after mating has occurred. The species Helicoverpa zea and Heliothis virescens, which coexist in North America, exhibit irreversible locking of the genitalia upon mating resulting in death of the mating pair – an example of prezygotic mechanical isolation (Hardwick, 1965). Although H. virescens can successfully mate with its congener Heliothis subflexa, which also resides in North America, their male offspring are sterile – an example of postzygotic isolation (Proshold and Lachance, 1974). In some cases, the fitness costs associated with interspecific mating have reinforced the evolution of prezygotic mechanisms to distinguish between different species before mating occurs (Hardwick, 1965). In heliothines (and many other insects), the emission and detection of specific volatile chemicals, such as pheromones and synomones, can perform this function. For example, the pheromone blend of H. virescens females contains the compound Z-9-tetradecenal (Z9-14:Ald), which repels male H. zea (Table 1; Figure 1; Vickers et al., 1991). When Z9-14:Ald is at a proportion of 15% relative to the principal component, Z-11-hexadecenal (Z11-16:Ald), upwind flight of males is significantly reduced, an effect referred to as “behavioral antagonism” (Table 1; Figure 1; Vickers et al., 1991; Baker, 2008). Therefore, Z9-14:Ald is said to be a behavioral antagonist of male H. zea (Baker, 2008). Similar heterospecific antagonism has been demonstrated between other sympatric species, such as antagonism of H. virescens males to Z-11-hexadecanyl acetate (Z11-16:OAc) produced by H. subflexa, or antagonism of H. armigera males when exposed to pheromone blends with abnormally high titres of Z9-14:Ald (Hillier and Baker, 2016). Pheromone-detecting olfactory sensory neurons (OSNs) are organized by “sensillar types”, with Heliothis spp. typically exhibiting three predominant types (A, B, C) and Helicoverpa spp. exhibiting two (A and C) (Figure 1; Hillier and Baker, 2016). OSNs in type-A sensilla detect primary pheromone components (most often Z11-16:Ald), type-B OSNs detect secondary compounds, and type-C often house OSNs which are involved in heterospecific antagonism (Figure 1; Hillier and Baker, 2016).
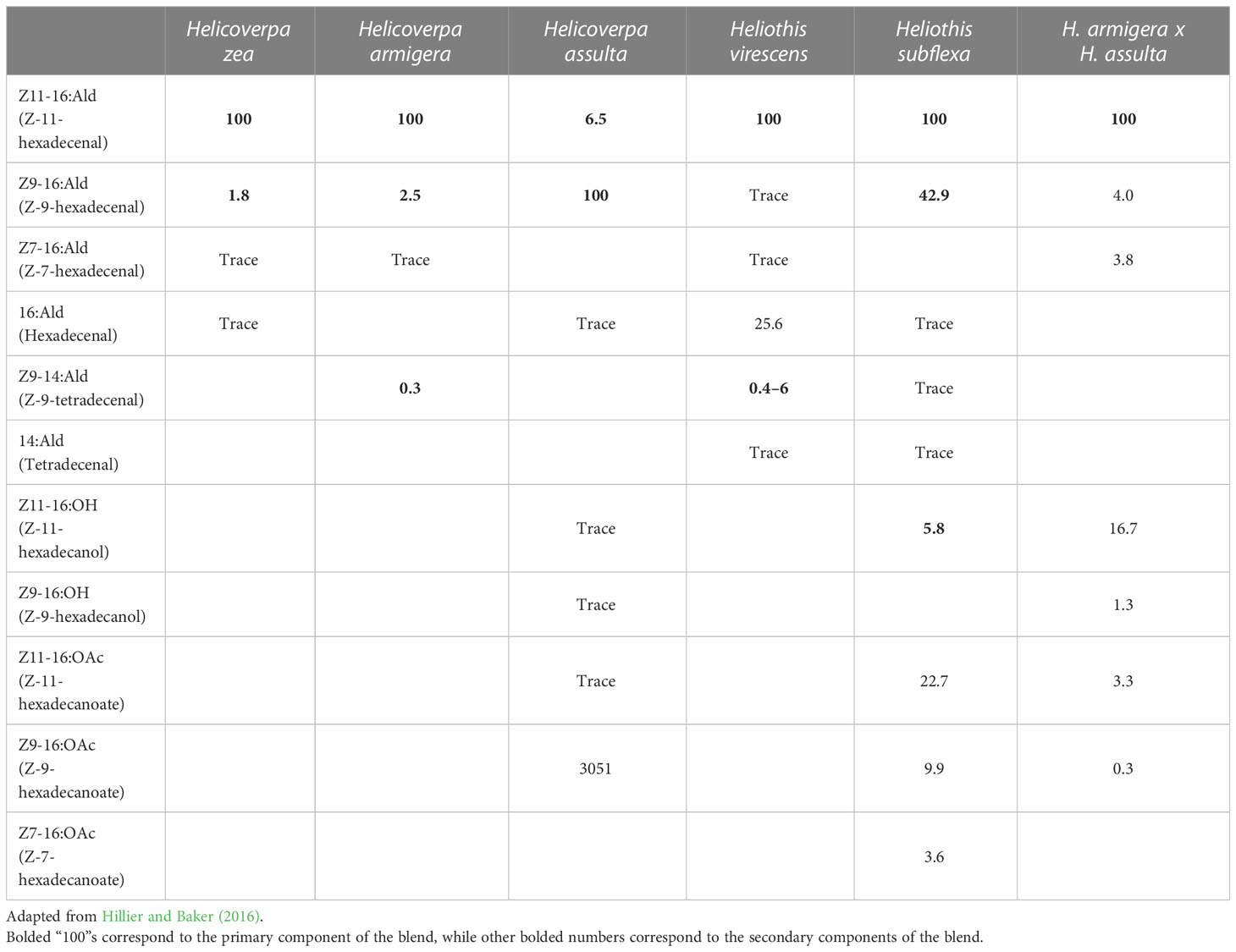
Table 1 Female pheromone blends of major heliothine species (Helicoverpa zea, Helicoverpa armigera, Helicoverpa assulta, Heliothis virescens, and Heliothis subflexa) and the hybrid of H. armigera x H. assulta with percentage of compounds produced by females relative to the primary component (Z11-16:Ald for all except H. assulta, which has Z916:Ald as the primary component) and trace components.
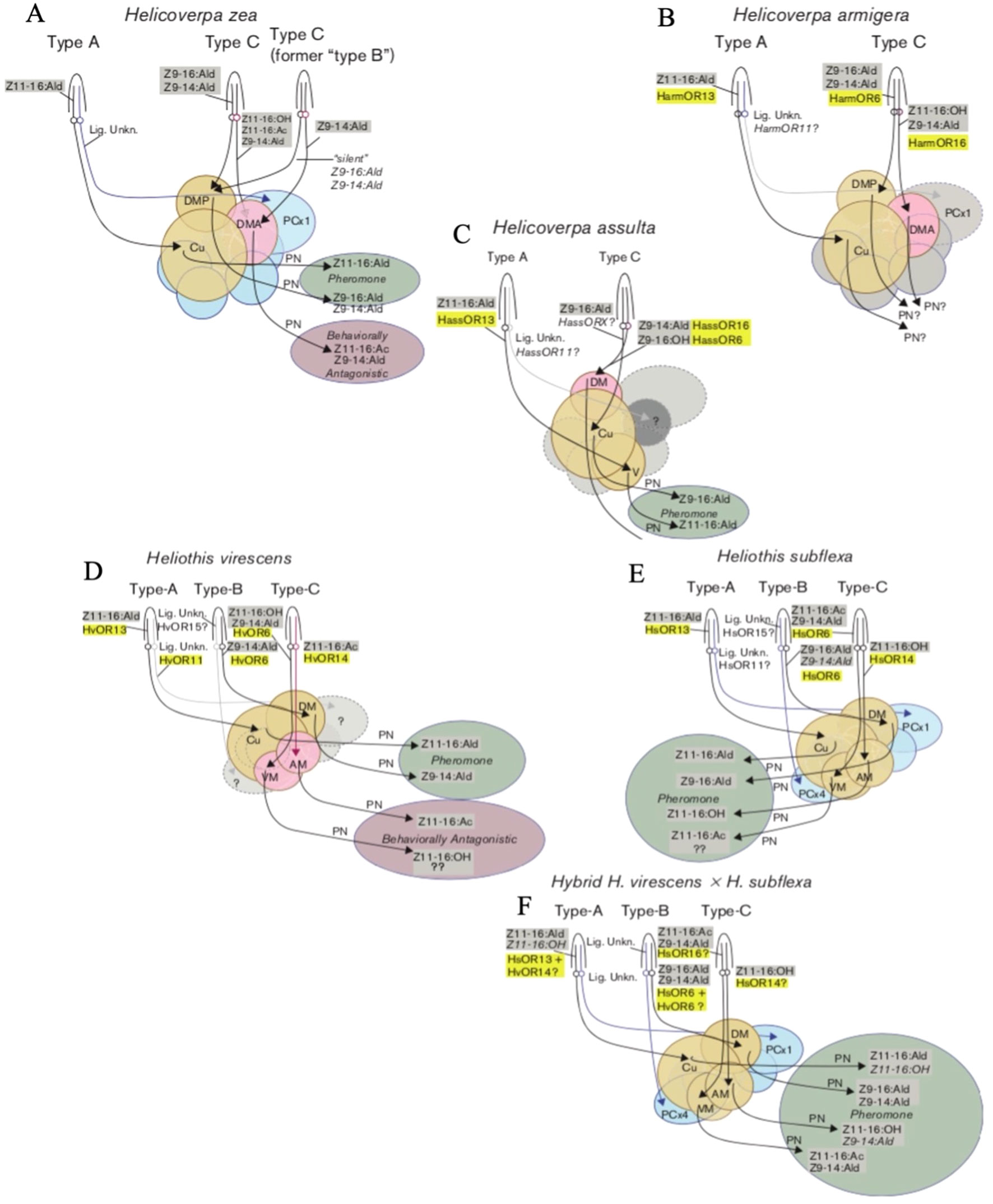
Figure 1 Diagrams of the olfactory pathways of male H.zea (A), H. armigera (B), H. assulta (C), H. virescens (D), H. subflexa (E), and hybrid H.virescens x H. subflexa (F). Sensilla are represented as conical structures labeled Type-A, Type-B, or Type-C. Olfactory sensory neurons (OSNs) are represented as lines inside the sensilla. The ligands known to produce responses within each OSN are highlighted in grey. The olfactory receptors (ORs) to which these ligands bind are highlighted in yellow. The projections of each OSN from neuronal cell bodies to areas of the brain are represented by arrows projecting from circles. Areas of the brain are represented by circular or ovoid shapes where Cu = cumulus, DM = dorsomedial glomeruli, AM = anteromedial glomeruli, VM = ventromedial glomeruli, DMA = anterior dorsomedial glomeruli, DMP = posterior dorsomedial glomeruli, PCx1 = posterior complex glomerulus 1, PCx4 = posterior complex glomerulus 4. The projections of projection interneurons (PN) are represented by black arrows. The behavioural responses elicited by particular pheromone components are represented by circular or ovoid shapes, where green = behavioral agonists (attraction) and red = behavioral antagonists (repulsion). Adapted from Hillier and Baker (2016).
Given the high fitness costs of some interspecific mating, prezygotic mating isolation via sex pheromone communication is under high selective pressure. Stabilizing selection would theoretically be favored in heliothine sex pheromone communication systems, with species becoming finely-tuned to the blends of conspecifics and blends remaining within tight parameters (Cardé & Baker, 1984; Baker, 1989). However, with random genetic mutations and epigenetic changes occurring in individuals within a population, and asymmetric sexual selection taking place, modifications in blend production and/or detection can occur (Löfstedt et al., 1989; Phelan, 1992; Löfstedt, 1993; Jurenka et al., 1994; Haynes, 1997; Phelan, 1997a; Phelan, 1997b; Baker, 2002; Roelofs et al., 2002). Thus, despite the evolution of sex pheromones as prezygotic isolating mechanisms, hybridization of various heliothine species has been well-documented both in the wild (Anderson et al., 2016; Anderson et al., 2018) and under laboratory conditions (Hardwick, 1965; Laster, 1972; Wang and Dong, 2001). Heliothine hybrids were first extensively documented by Hardwick in 1965 in “The Corn Earworm Complex”, primarily by attempting crosses between H. zea, Helicoverpa armigera, and Helicoverpa punctigera for the purpose of investigating the potential for hybrid species to be used for pest control strategies (Hardwick, 1965). For each pairing, a female of one species was placed in a jar with two males of another species, and attempted and completed copulations were recorded (Hardwick, 1965). The only successful mating resulting in viable eggs and offspring occurred between a male H. zea and female H. armigera, although these species sometimes had locked genitalia leading to death without reproduction (Figure 2; Hardwick, 1965). In addition to H. zea and H. armigera, two other major heliothine hybrids would be identified in the following decades: those between crosses of H. virescens and H. subflexa (Laster, 1972) and between crosses of Helicoverpa assulta and H. armigera (Figures 3, 4; Wang and Dong, 2001). The implications of these hybridizations on pheromone production and detection are important for understanding the history of speciation events in heliothine moths and for developing new pest management techniques as these hybrids emerge in the wild.
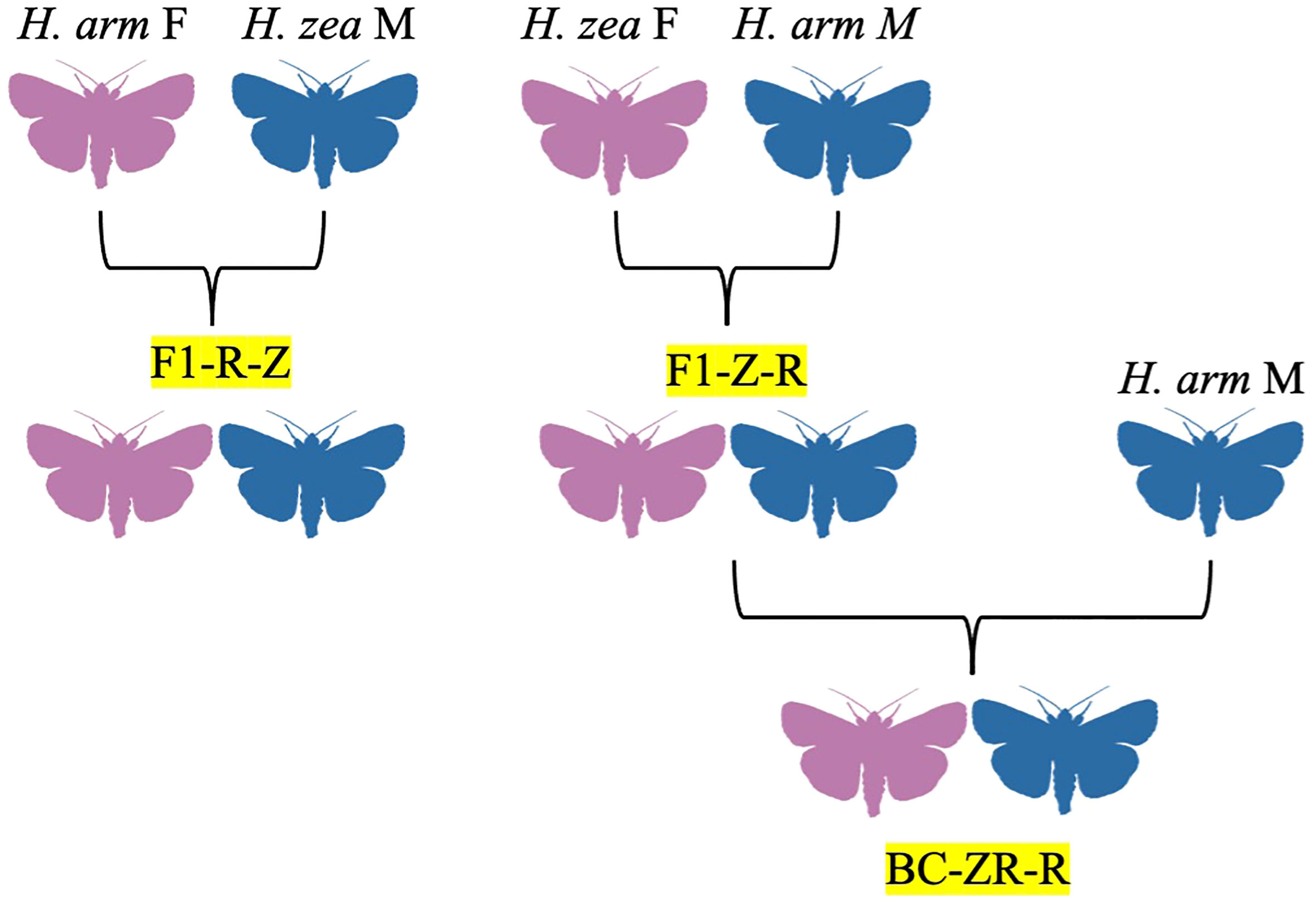
Figure 2 Hybrid family trees for H. zea x H. armigera crosses, identified by codes denoting generation, female parent, and male parent, in order. F1 = filial generation, BC = backcross generations, Z = H. zea, R = H. armigera.
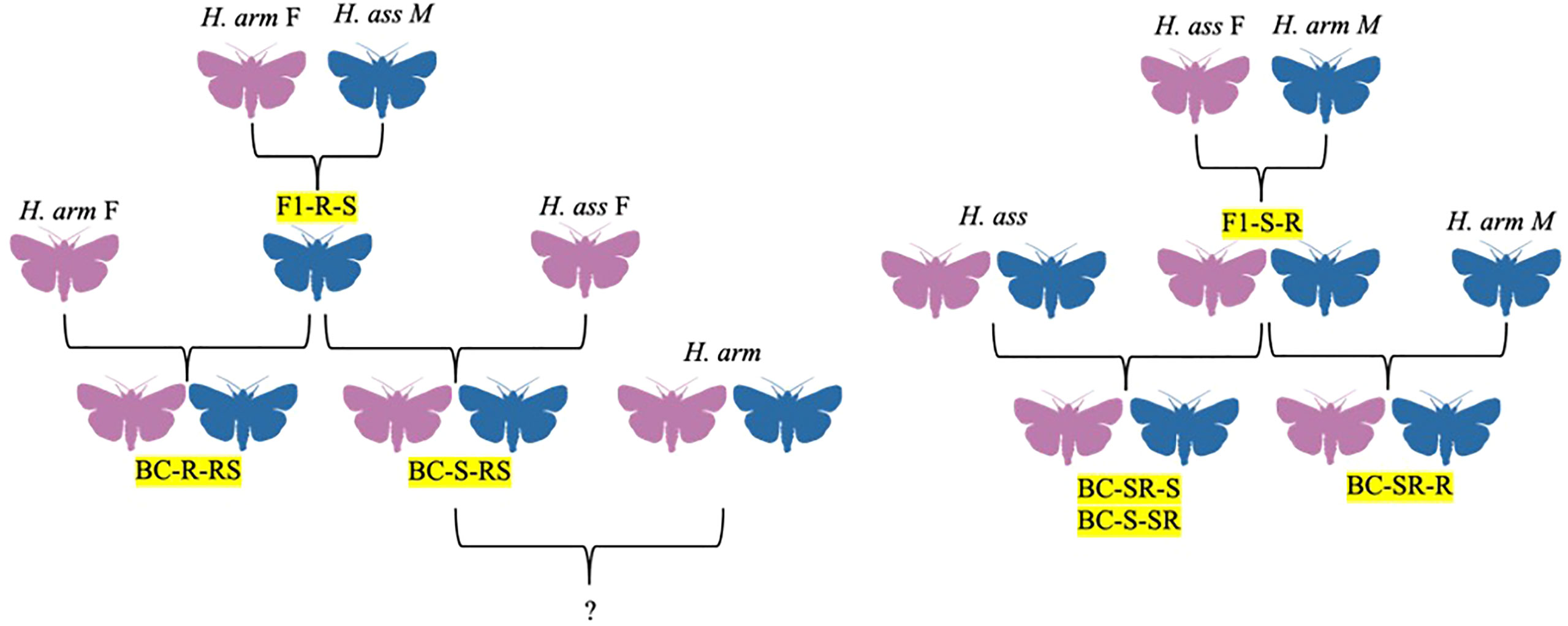
Figure 3 Hybrid family trees for H. assulta x H. armigera crosses, identified by codes denoting generation, female parent, and male parent, in order. F1 = filial generation, BC = backcross generations, S = H. assulta, R = H. armigera.
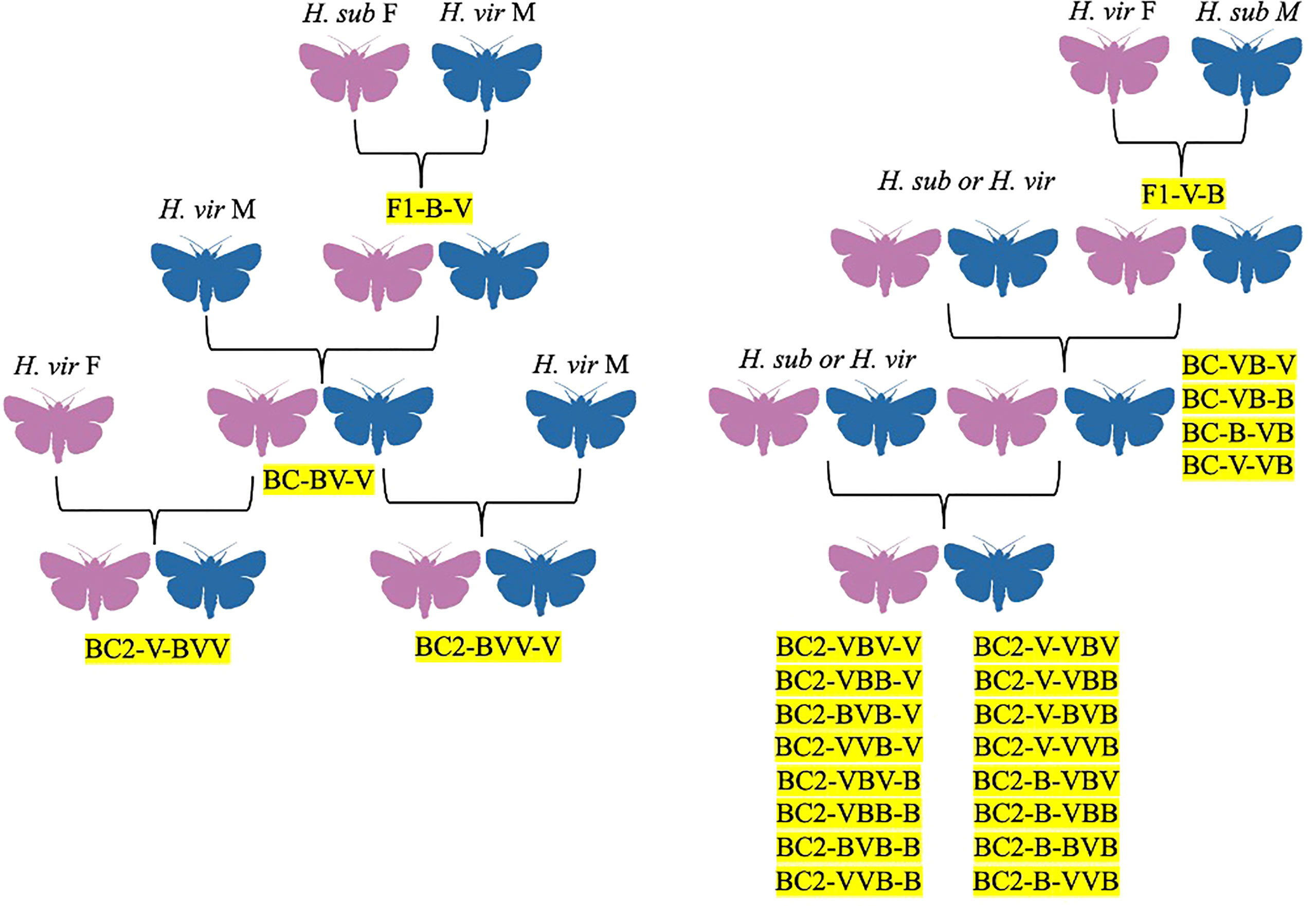
Figure 4 Hybrid family trees for H. virescens x H. subflexa crosses, identified by codes denoting generation, female parent, and male parent, in order. F1 = filial generation, BC = backcross generations, V = H. virescens, B = H. subflexa.
Furthermore, studying hybridization can provide insight into how changes in pheromone detection systems may be driven by phenotypic plasticity (Anderson, 2003; Andersson et al., 2007; Bailey and Zuk, 2008; Groot et al., 2009a; Groot et al., 2010). Studies in various insects, including heliothines, have shown that individuals can change their pheromone blends in response to the social environment (Bashir et al., 2003; Petfield et al., 2005; Kent et al., 2008; Krupp et al., 2008; Groot et al., 2009a; Groot et al., 2010). In the presence of hybrid species in hybrid zones, plastic non-hybrid individuals could potentially change their blends to prevent interspecific mating, allowing them to colonize new habitats and forcing pheromone detection in conspecifics to shift in response to the new blends (Crispo, 2008; Groot et al., 2010). Numerous studies on the three known heliothine hybrids (H. zea x H. armigera, H. assulta x H. armigera, and H. subflexa x H. virescens) have explored their genetics, physiology, and behavior, and provide insight into the plasticity of pheromone communication systems in wild populations.
Helicoverpa zea and Helicoverpa armigera
First laboratory studies of H. zea x H. armigera hybrids
The species H. zea and H. armigera are recently sympatric in regions of the Americas, particularly South America and the southern USA (Hillier and Baker, 2016). Following the discovery of the first H. armigera x H. zea crosses in the lab by Hardwick, Laster and colleagues would attempt a formal study on their intermating compatibility in 1985, inspired by previous research on H. subflexa x H. virescens (Laster, 1972; Laster et al., 1985). In their study, wild H. armigera females imported from Australia were crossed with laboratory-reared male H. zea to see if sterile male offspring could be obtained, allowing for pest control via the sterile insect technique (Figure 2; Laster, 1972; Laster et al., 1985). Some evidence of male sterility was found in later filial generations, leading to further investigation in a 1995 study using wild H. armigera from Russia and laboratory-bred H. zea populations (Laster and Hardee, 1995). However, no backcross sterility was detected despite some differences in mating incidence, life-stage development, and pupal weights between certain crosses and some incidences of genital locking (Laster and Hardee, 1995). A similar study of wild H. armigera from China and lab-reared H. zea also found no evidence of sterility between any crosses (Laster and Sheng, 1995).
The viability of reciprocal crosses of H. zea x H. armigera was evaluated by Rios and colleagues in 2021, with the authors detecting hybrid vigor in some F1 hybrids and hybrid sterility in intercrosses of offspring from H. armigera females and H. zea males not previously documented by Laster, Sheng, and Hardee (Figure 2; Laster and Hardee, 1995; Laster and Sheng, 1995; Rios et al., 2021). Although the fecundity and egg survival of parental species was higher, most hybrids showed greater survival rates in the larval, pupal, and pre-pupal stages (Rios et al., 2021). Some hybrid crosses also showed unbalanced sex ratios; H. armigera male x H. zea female hybrids were 38.3% female, while backcrosses of these hybrid females and H. armigera males were 63.6% female (Rios et al., 2021). Female hybrids also tended to produce fewer females (Rios et al., 2021). The increased survival rates of hybrids in immature stages of development were not enough to overcome their lack of fecundity, a weakness which could counteract other benefits hybrids may have, such as shorter development time (Rios et al., 2021). The authors suggest that such limitations would prevent hybrids from flourishing in the wild, although a greater number of mating events over a longer time period could produce enough hybrids with advantageous traits (i.e., pesticide and disease resistance, polyphagy, thermal tolerance, high mobility) to cause issues (Rios et al., 2021). To prevent this, numbers of each parental species should ideally be maintained in equal numbers, and other IPM strategies employed, including: usage of predators, parasitoids, or microorganisms for population control; usage of genetically-modified crops; pheromone trapping and mating disruption with special attention to geographic variations in pheromone blend; use of insecticides against H. zea but not H. armigera, which exhibit some resistance; population monitoring particularly at the egg and larval stages; monitoring of resistance to pesticides and xenobiotics; and strategic crop rotation involving staggering of crops (Rios et al., 2021). For example, corn is the preferred host of H. zea, but soybeans, tomatoes, and cotton for H. armigera, and thus sowing of these crops could be alternated depending on geography and seasonality (Rios et al., 2021).
Discovery of wild H. zea x H. armigera hybrids
Although previous laboratory studies showed that H. armigera and H. zea could produce viable offspring, it was not until 2013 that a potential H. armigera and H. zea hybrid was found in the wild in Brazil by Anderson et al. (2016). Heliothines were collected from sixteen different countries between 2004–2014 and identified at the species level using mitochondrial gene sequencing. Some H. zea were collected from Brazil before 2013 (the year of the first H. armigera invasion to the Americas), and some after the invasion (Anderson et al., 2016). Genotyping-by-sequencing analysis identified one H. zea individual from Brazil as falling between the main cluster of H. zea and two clusters of H. armigera, making it a possible hybrid (Anderson et al., 2016). Additionally, H. armigera from Brazil were found to be more similar to populations of H. zea from Brazil than to other populations of H. armigera collected from Australia, China, India, or Uganda, denoting either potential hybridization or similar populations of origin between the two species (Anderson et al., 2016). In contrast, H. zea sampled both before and after the H. armigera invasion in 2013 did not differ from one another (Anderson et al., 2016). The only significant admixture between populations was found between Brazilian H. zea and USA H. zea, evidence of the founder effect caused by the initial foundation of the H. zea population when H. armigera first came to the Americas 1.5–2 million years ago (Behere et al., 2007). There was no evidence of greater gene flow from Brazilian H. armigera into Brazilian H. zea (Anderson et al., 2016). The researchers hypothesized that the genetic distinctions between H. armigera and H. zea could become more difficult to parse if H. armigera were to spread and hybridize in the Americas (Anderson et al., 2016). They also suggest that H. zea may have facilitated the arrival of H. armigera into the Americas by exchanging genetic material that would lessen the effects of a bottleneck or provide a source for advantageous traits (Anderson et al., 2016). One such advantageous trait could be resistance to the pyrethroid insecticide fenvalerate conferred by the CYP337B3 gene, which was found to be under selection in the study (Joußen et al., 2012; Anderson et al., 2016). The CYP337B3 gene codes for the P450 enzyme capable of converting pyrethroid insecticides into nontoxic compounds, and its presence has been shown to give a 42-fold increase in fenvalerate resistance in H. armigera (Joußen et al., 2012). If a population of H. armigera had recently undergone a bottleneck, for example, pest control methods targeting insecticide resistance could be more effective than on a population undergoing purifying selection to get rid of deleterious alleles. Alternatively, it could increase the prevalence of favorable genes in a new habitat.
Population genetics of wild H. zea x H. armigera hybrids
In 2017, expanding on the study by Anderson and colleagues, Leite and colleagues used microsatellite markers to determine population structure and gene flow between Brazilian H. armigera, Brazilian H. zea, and US H. zea (Leite et al., 2017). Putative hybrids were highest during the years 2012–2013, with H. armigera populations showing the highest proportions of hybrids (up to 40%) compared to H. zea (up to 18%) (Leite et al., 2017). In both Brazil and the US, both species seemed to have random breeding patterns, but also showed greater genetic variation in Brazil. Although the genetic identities of H. armigera and H. zea are generally preserved in South America, most likely due to prezygotic isolation based on differences in genital morphology (Pogue, 2004), hybridization still occurs at low rates and hybrids could be better adapted to certain climates, pathogens, or pest control methods. Given that both species are widely distributed, have a high number of shared traits (including high fecundity, polyphagy, and high migration and dispersion), and with evidence of H. armigera being detected in Central America and Florida, the authors suggest further monitoring of species ranges and implementation of robust integrated pest management strategies (Leite et al., 2017).
Potential resistance to certain insecticides and transgenic crops is also concerning considering the polyphagous nature of both species (Leite et al., 2017). Pearce and colleagues published an investigation of the evolutionary trajectory of various gene families in the H. zea/H. armigera lineage (Pearce et al., 2017). They found that prior to the divergence of this lineage from the common ancestor around 1.5 million years ago, hundreds of gene families involved in detoxification of compounds (particularly pesticides or host plants), digestion, and gustatory receptors for CO2 detection were accumulated compared to lepidopterans with more narrow host ranges. However, differences have emerged, with H. armigera showing a higher number of pesticide and Bt crop resistance genes and wider host range, while H. zea has lost some genes for GRs, detoxification, and pesticide resistance (Pearce et al., 2017). In the last decade, H. armigera has invaded South America, is showing signs of invading Central and North America, and is largely displacing H. zea in Brazil (Pearce et al., 2017). The authors suggest that evidence of hybridization and introgression of H. armigera genes into H. zea could be occurring in South America, and the high level of similarity between species suggests that hybrids would not break down over time (Pearce et al., 2017). The additional GRs, genes for tolerance to plant defenses, and resistance to pyrethroids and Bt could be passed from H. armigera to H. zea and cause further crop damage (Pearce et al., 2017).
In 2018, Anderson and colleagues identified nine hybrid individuals from Brazil, eight being largely H. armigera (91–98%) with some introgression of H. zea genes, and the ninth individual showing greater similarity to H. zea (Anderson et al., 2018). In the ninth individual, the H. armigera-like regions contained genes for a gustatory receptor and esterase genes responsible for host range, and a cytochrome p450 enzyme gene (CYP337B3) involved with resistance to pyrethroids (Anderson et al., 2018). There is a potential that a combination of the traits that allowed H. zea to successfully adapt to the New World will mix with the traits that give H. armigera its greater ability to resist pesticides and transgenic crops and its wider host range to create a much more problematic pest (Anderson et al., 2018). Although this study suggests a low level of backcrossing of H. armigera with H. zea and thus a lower likelihood of H. zea inheriting these traits, high selection pressures still exist (Valencia-Montoya et al., 2020).
To determine the potential effects of secondary contact between these species, Valencia-Montoya and colleagues performed whole-genome sequencing on populations of H. armigera and H. zea from shortly after the H. armigera invasion (2012–2013) and more recently (2016–2017). The researchers found that species boundaries were generally well-maintained, introgression is not specific to any regions of the H. armigera genome, and that admixture has decreased in Brazilian H. armigera (Valencia-Montoya et al., 2020). For H. zea, there was strong introgression of a region containing the insecticide resistance gene CYP337B3, which had previously only been found in invasive Brazilian H. armigera (Valencia-Montoya et al., 2020). In a principal component analysis (PCA), two individuals fell between the clusters of H. zea and H. armigera, and thus these individuals were suspected to be early generation hybrids. Out of 132 moths analyzed, 66 individuals had a closer relationship to H. armigera with 0–9.2% of their alleles derived from H. zea, while 27 individuals genetically closer to H. zea had 0–4.1% H. armigera ancestry, suggesting that hybridization was rare in more recent Brazilian populations (Valencia-Montoya et al., 2020). Using fixation index (FST), the researchers found only one peak of differentiation between H. zea from the US and Brazil corresponding to a region on chromosome 15 containing a locus for insecticide resistance, suggesting localized introgression (Valencia-Montoya et al., 2020). Phylogenetic comparisons between the genomes of Brazilian H. zea and H. armigera showed large-scale introgression in recent populations and discordant topology around the CYP337B3 locus on chromosome 15 (Valencia-Montoya et al., 2020). With introgression only occurring around this region, the researchers concluded that introgression was not widespread in H. zea. However, there was also strong evidence of gene flow between Brazilian H. zea and H. armigera between 2013–2017, with introgression decreasing over time in H. armigera but increasing in H. zea, particularly of the CYP337B3 gene, with the gene being present in 14/18 (77.8%) of H. zea in 2017 (Valencia-Montoya et al., 2020). In H. armigera, nearly entire chromosomes showed introgression in the earlier samples, particularly on chromosome 18, but shorter introgressed regions and lower effective migration rates in more recent samples, with indications of purifying selection against introgression of H. zea genes in H. armigera (Valencia-Montoya et al., 2020). There is evidence for strong positive selection of the CYP337B3 locus in both H. zea and H. armigera (Valencia-Montoya et al., 2020). Overall, the evidence gathered by Valencia-Montoya and colleagues demonstrated that H. zea and H. armigera remain two distinct species and hybridization levels remain low, likely due to the incompatibility of genitalia and differences in sex pheromone composition. While both blends contain different proportions of Z11-16:Ald, Z-9-hexadecenal (Z9-16:Ald), and Z-7-hexadecenal (Z7-16:Ald), the H. armigera blend contains Z9-14:Ald, which is antagonistic to male H. zea (Table 2; Vickers et al., 1991). While the pheromone blends of the parental species are known, no studies have been published on pheromone blend composition produced by these hybrids. Despite differences in pheromone blends, there is still strong introgression of the CYP337B3 locus from H. armigera into H. zea. This increase in introgression may have been driven by increased use of pesticides in 2012–2013 following the H. armigera invasion, selecting for early hybrids (Valencia-Montoya et al., 2020). The researchers warn that with global trade, invasive pests are becoming more common, and excessive pesticide use may drive selection for introgression and hybridization (Valencia-Montoya et al., 2020).
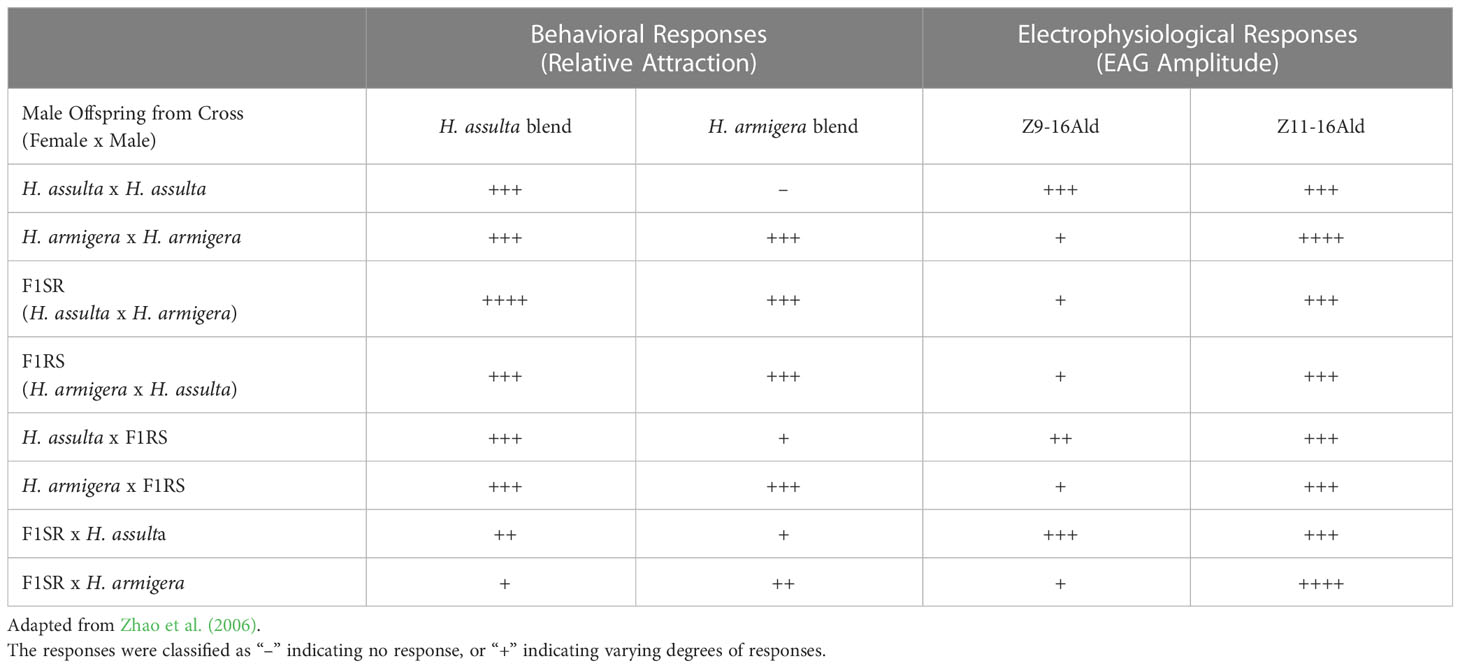
Table 2 Summary of behavioral and electrophysiological responses of H. assulta, H. armigera, their hybrids, and backcross males from insect lines tested by Zhao et al. (2006).
In 2020, Cordeiro and colleagues also used single nucleotide polymorphisms (SNPs) to detect H. zea x H. armigera hybrids in various crop fields in Brazil. The researchers found hybrids at 5 different sites, with 26 insects (15%) showing mixed ancestry and an average of 10% introgression (Cordeiro et al., 2020). The main gene flow between species was found to be from H. zea to H. armigera (Cordeiro et al., 2020). Variations in climate and landscape affected the rate of introgression into H. armigera, with the presence of maize, tree plantations, and soybeans being associated with higher introgression, H. zea being associated with maize, and H. armigera with soybean (Cordeiro et al., 2020). Although few hybridization events occur, large sections of the genome can be introgressed, and multiple hybridizations may create an adaptive bridge between the species and increase the fitness of hybrids (Cordeiro et al., 2020). The researchers point to the CYP337B3v2 resistance gene, found in both H. zea and H. armigera, as a salient example, along with genes for detoxification and gustatory receptors that could be passed from H. armigera to H. zea (Pearce et al., 2017). They also suggest that rotation of crops may increase hybridization, and since H. armigera is resistant to pyrethroids and H. zea to the Cry1Ac Bt-protein in some soybean crops, resistance to each of these due to introgressions is likely to occur in areas where maize and soybeans are cultivated together (Cordeiro et al., 2020).
Helicoverpa armigera and Helicoverpa assulta
First laboratory studies of H. armigera x H. assulta hybrids
In addition to H. zea, H. armigera is also known to interbreed with Helicoverpa assulta, a major polyphagous pest of Africa, Asia, Australia, and Oceania (Figure 3). Although this hybrid has only been documented under laboratory conditions, the two do have overlapping habitats on these continents (Hillier and Baker, 2016). The first attempt to study H. armigera x H. assulta hybrids occurred in 1984, with the authors concluding at the time that the two species could not interbreed (Wang and Li, 1984; Wang and Dong, 2001). However, the original study had a small sample size, and thus Wang and Dong decided to re-attempt the experiment in 2001 with more robust methods to evaluate the potential for producing sterile hybrids that could be used for pest management (Figure 3; Wang and Dong, 2001). The researchers found that the hybrids of H. armigera females and H. assulta males were all males, although there was no evidence of hybrid sterility in either the offspring or the backcross of the hybrid offspring with H. armigera (Figure 3; Wang and Dong, 2001). However, the backcross offspring did show a distorted sex ratio of 4 males to 1 female, and the original H. armigera female x H. assulta male hybrid males exhibited greater signs of fitness than their parents, showing a possibility of using these crosses as a control mechanism (Wang and Dong, 2001). The researchers suggested further studies showing how the hybrids could compete against wild H. armigera.
Pheromone biosynthesis pathways in H. armigera x H. assulta hybrids
In 2005, Wang and colleagues further investigated the biosynthesis and composition of sex pheromones in H. armigera x H. assulta crosses. Both H. armigera and H. assulta use Z11-16:Ald and Z9-16:Ald in their blends, however, while Z9-16:Ald is the major component in H. assulta and Z11-16:Ald the minor component, Z11-16:Ald is the major component in H. armigera, with the minor components being Z9-16:Ald and Z9-14:Ald (Table 1; Figure 1; Hillier and Baker, 2016). In this study, H. armigera was found to have a 2.1:100 ratio of Z9-16:Ald to Z11-16:Ald, and H. assulta a 1739:100 ratio (Table 1; Wang et al., 2005). These ratios were close to the ratios of the precursor compounds for Z9-16:Ald and Z11-16:Ald, Z-9-hexadecanol (Z9-16:OH) and Z-11-hexadecanol (Z11-16:OH), respectively (Wang et al., 2005). The hybrids had a ratio of 4:100, which was closer to the H. armigera ratio but still significantly different (Table 1; Wang et al., 2005). Quantities of Z9-16:Ald, Z11-16:Ald, Z9-16:OH, Z11-16:OH, Z11-16:OAc, and Z-9-hexadecanoate (Z9-16:OAc) were also found in the hybrids (Table 1; Wang et al., 2005). Additionally, the relative amounts of Z9-16:OH and Z11-16:OH to Z11-16:Ald in the hybrid were much higher than in H. armigera, but their ratio to each other followed a similar pattern (Table 1; Wang et al., 2005). Small quantities of Z9-16:OAc and Z11-16:OAc were found in the hybrid gland extracts, with their ratios being similar to that of their corresponding alcohols in H. armigera but different from their corresponding acetates in H. assulta (Table 1; Wang et al., 2005). Based on their analysis of the chemical composition of the pheromone blends and their precursors, the researchers deduced the biosynthetic pathways of Z11-16:Ald and Z9-16:Ald in both species and their hybrid, with the hybrids having a combination of the two pathways (Wang et al., 2005). The ratio of Z9-16:Ald to Z11-16:Ald in the hybrid is regulated by both the Δ11 and Δ9 desaturases, which act on both palmitic acid and stearic acid, respectively, leading to a greater ratio of Z9-16:Acid to Z11-16:Acid (Table 1; Wang et al., 2005). The researchers concluded that the sex pheromone biosynthetic pathways are under polygenic control, with desaturase genes being most primitive in H. assulta, followed by H. zea, and becoming most complex in H. armigera (Wang et al., 2005). The pheromone biosynthesis pathway of hybrids was generally found to be similar to the pathway of H. zea, with the hybrid being able to produce Z9-16:Ald as a secondary component much like in parental H. armigera (Table 1; Choi et al., 2002; Wang et al., 2005). While this study revealed the composition of the hybrid pheromone blend, no further research has been done to compare the responses of hybrids or parental species to the hybrid blends.
Morphology and sex ratios of H. armigera x H. assulta crosses
In 2005, Zhao and colleagues documented the development and morphological characteristics of H. armigera x H. assulta crosses. Out of 492 hybrid larvae resulting from the H. armigera female x H. assulta male crosses, 374 (76%) completed larval development and pupated, 171 of these (~46%) showing abnormal development (Figure 3; Zhao et al., 2005). While the normal hybrids were all males capable of backcrossing, the abnormal hybrids were sterile with undeveloped or malformed reproductive structures (Figure 3; Zhao et al., 2005). Normal male hybrids showed greater flight activity as adults compared to the parental species (Zhao et al., 2005). Larvae and pupae that grew into abnormal adults took longer to develop (Zhao et al., 2005). Crosses between H. assulta females and H. armigera males yielded normal offspring with a 1:1 sex ratio which could then be self-mated to form mostly normal offspring with a 1:1 sex ratio (Figure 3; Zhao et al., 2005). When female hybrid offspring from the female H. assulta x male H. armigera cross were mated with male H. armigera, the sex ratio of the resulting offspring was 0.58:1, with males outnumbering females (Figure 3; Zhao et al., 2005). The backcross of female H. armigera and the male offspring of female H. armigera x male H. assulta crosses resulted in a male-biased sex ratio and 43% sterility (Figure 3; Zhao et al., 2005). The sterility of F1 hybrids from crosses between H. armigera females and H. assulta males, as well as the sterility of two backcross hybrids (H. armigera females x F1 males from both reciprocal crosses) may have been due to interactions between the Z chromosome from H. assulta and autosomes from H. armigera (Zhao et al., 2005). This interaction may also explain the sex biases observed in the backcrosses stated (Zhao et al., 2005). The ability to create a higher ratio of sterile hybrid males through H. armigera x H. assulta crosses could be beneficial for pest control, but the authors note several limitations (Zhao et al., 2005). First, the presence of normal hybrid males with increased flight capacity could result in introduction of pests to new areas. Second, although the species could mate under laboratory conditions, hybrids have never been found in the wild and various prezygotic isolation barriers exist, including differences in host plant range, pheromone composition, and calling periods (Zhao et al., 2005).
Electrophysiological responses and brain morphology of H. armigera x H. assulta hybrids
In 2006, Zhao, Yan, and Wang further studied the behavior and electrophysiological responses of H. assulta x H. armigera crosses to sex pheromone blends. In the field, a 95:5 blend of Z9-16:Ald to Z11-16:Ald was found to be most attractive to H. assulta males, while a 97:3 blend was found to be most attractive to H. armigera males, with some variation of these ratios occurring in different regions (Table 2; Zhao et al., 2006). In response to the H. armigera blend (97:3 Z11-16:Ald to Z9-16:Ald), H. assulta males remained inactive, while males of both H. armigera x H. assulta crosses showed attraction to the blend similar to H. armigera (Table 2; Zhao et al., 2006). Very few of the crosses between H. assulta and the hybrid offspring of H. assulta females x H. armigera males responded to the H. armigera blend and they behaved similarly to male H. assulta (Table 2; Zhao et al., 2006). However, male offspring of crosses between H. armigera females x F1RS males (the offspring of H. armigera females x H. assulta males) and H. armigera males x F1SR females (the offspring of H. assulta female x H. armigera males) showed attraction to the sex pheromones, with the number of moths responding in the former group being very close to H. armigera (Table 2; Zhao et al., 2006). When electroantennograms (EAGs) were recorded in response to the H. assulta and H. armigera blends, the only significant differences were found to be between F1RS males, who showed lower responses than H. assulta and H. armigera at 104 and 105 ng/μl concentrations (Table 2; Zhao et al., 2006). EAGs were also recorded in response to individual components of pheromone blends (Table 2; Zhao et al., 2006). In H. assulta males, both Z9-16:Ald and Z11-16:Ald evoked dose-dependent responses but were especially high in the 103–105 ng/μl range (Table 2; Zhao et al., 2006). In H. armigera males, only Z11-16:Ald evoked dose-dependent responses, while Z9-16:Ald evoked weak responses (Table 2; Zhao et al., 2006). The normal F1 hybrids with both sex combinations showed similar dose-dependent responses to both Z11-16:Ald and Z9-16:Ald, with Z11-16:Ald evoking higher responses in each (Table 2; Zhao et al., 2006). Abnormal offspring from the female H. armigera x male H. assulta cross showed no significant responses to either compound (Table 2; Zhao et al., 2006). Comparisons between groups at the 105 ng/μl dose showed that H. assulta males showed significantly higher responses to Z9-16:Ald than H. armigera, while the response of male hybrids was similar to H. armigera males (Table 2; Zhao et al., 2006). Male offspring from the backcross of H. assulta females x F1RS males and H. assulta males x F1SR females responded more strongly than H. armigera males, with the former cross showing a lower response than H. assulta but the latter exhibiting a similar response to H. assulta (Table 2; Zhao et al., 2006). Males of the F1SR female x H. armigera male and F1RS male x H. armigera female cross showed responses to Z9-16:Ald similar to male H. armigera (Table 2; Zhao et al., 2006). Males of the F1 hybrids with both sex/species combinations exhibited an intermediate response to Z11-16:Ald between that of the parent species (Table 2; Zhao et al., 2006). Males from the backcrosses of female H. assulta x F1RS males and male H. assulta x F1SR females had similar Z11-16:Ald responses to male H. assulta, while the response of males from the back crosses H. armigera males x F1SR females and H. armigera females x F1RS males were similar to those of male H. armigera (Table 2; Zhao et al., 2006). Flight experiments indicated that H. armigera genes tended to be dominant in the inheritance of sex pheromone responses, particularly to Z9-16:Ald (Table 2; Zhao et al., 2006).
Since H. assulta females from different geographic areas have different sex pheromone blend ratios and males different responses, differences in blend specificity between regions may cause diversification of species. Alternatively, lower specificity in H. armigera could lead to widespread random mating, thereby reducing diversification. Males of H. assulta and H. armigera showed no significant differences in EAG responses, but H. assulta males still showed strong dose-dependent responses to Z9-16:Ald, and H. armigera a much weaker response (Table 2; Zhao et al., 2006). OSNs of H. assulta were tuned to Z9-16:Ald and co-occurred in the sensilla with neurons tuned to Z9-14:Ald, the secondary pheromone component in the blend of H. virescens females (Figure 1; Zhao et al., 2006). Few or none of these Z9-16:Ald-tuned neurons existed in H. armigera (Figure 1; Zhao et al., 2006). In the case of Z11-16:Ald, males of both species displayed strong dose-dependent EAG responses, while males of both F1 hybrids showed intermediate responses compared to the parental generation (Table 2; Zhao et al., 2006). Overall, H. armigera males are mostly sensitive to Z11-16:Ald, while male H. assulta are sensitive to both Z11-16:Ald and Z9-16:Ald (Table 2; Figure 1; Zhao et al., 2006). The researchers concluded that genes on autosomal chromosomes, not sex chromosomes or cytoplasmic factors, are likely responsible for sex pheromone responses in hybrid offspring, but that further research was needed to conclusively determine the pattern of inheritance (Zhao et al., 2006).
In 2017, Xu and colleagues did further work on inheritance of the pheromone sensory system in H. armigera and H. assulta. The researchers compared olfactory responses to pheromone components in the periphery and antennal lobes of males and found that pheromone responses to two sex pheromones were controlled by a major gene, with the allele from H. armigera being dominant as suggested by Zhao, Yan, and Wang in 2006 (Zhao et al., 2006; Xu et al., 2017). The male specific sensilla found in both species were also found in the male hybrids and backcrosses, in addition to two new subtypes of sensilla with broader responses (Xu et al., 2017). They hypothesized that when female H. armigera and male H. assulta hybridize, male hybrids can successfully backcross with female H. armigera, leading to introgression from H. assulta into H. armigera (Figure 3; Xu et al., 2017). All H. armigera individuals had more A type sensilla than C type, while the opposite was found in H. assulta (Table 3; Xu et al., 2017). The F1 (female H. armigera x male H. assulta) and BC2 (F1 x female H. assulta) exhibited the same sensilla patterns as H. armigera, but the BC1 (F1 x female H. armigera) backcrosses were comprised of approximately half H. armigera-like individuals and half H. assulta-like individuals (Table 3; Xu et al., 2017). While the Type A sensilla in H. armigera and H. assulta only respond to Z11-16:Ald, 8% of the Type A sensilla in F1 (named expanded A), responded to Z11-16:OH and Z9-14:Ald (Table 3; Xu et al., 2017). The Type C sensilla were divided into two subgroups in H. armigera (Harm C1, Harm C2) and three in H. assulta (Hass C1, Hass C2, Hass C3), and all were found in F1 and all backcrosses (Table 3; Xu et al., 2017). An “expanded C” subtype of sensilla was discovered F1 and BC2, which were responsive to a wider array of compounds than Type C sensilla (Table 3; Xu et al., 2017). In F1, 35.6% of Type C sensilla had H. armigera-like responses, 46.7% H. assulta-like responses, while 17.8% were the extended C type (Table 3; Xu et al., 2017). In BC1 and BC2, the majority of Type C sensilla responded as H. assulta and H. armigera, respectively (Table 3; Xu et al., 2017). Calcium-imaging studies of male brains showed that the α glomerulus (responsive to Z11-16:Ald) was the largest in H. armigera, while the β glomerulus (responsive to Z9-16:Ald) was largest in H. assulta (Xu et al., 2017). In F1 and BC2, male brains had similar topography and α:β glomerulus ratio to H. armigera (Xu et al., 2017). In BC1 males, half were more similar to H. assulta and the other half to H. armigera regarding the same criteria (Xu et al., 2017). The macroglomerular complexes (MGC) of parents and hybrids were classified into α, β, and γ glomeruli and 3D imaging showed that F1 had a similar topography to H. armigera, although the β glomerulus was larger in F1 (Xu et al., 2017). Overall, the F1 and BC2 sex pheromone responses were similar to H. armigera, albeit with some broader responses (Xu et al., 2017). The researchers suggest that the expanded Type C sensilla, which responded to five compounds in comparison to three or four in the parental species, could be a product of genetic recombination (Xu et al., 2017). Two different pheromone receptors co-expressed or co-localized in the Type C olfactory sensory neurons (OSNs) could change the compounds to which they are tuned (Xu et al., 2017).
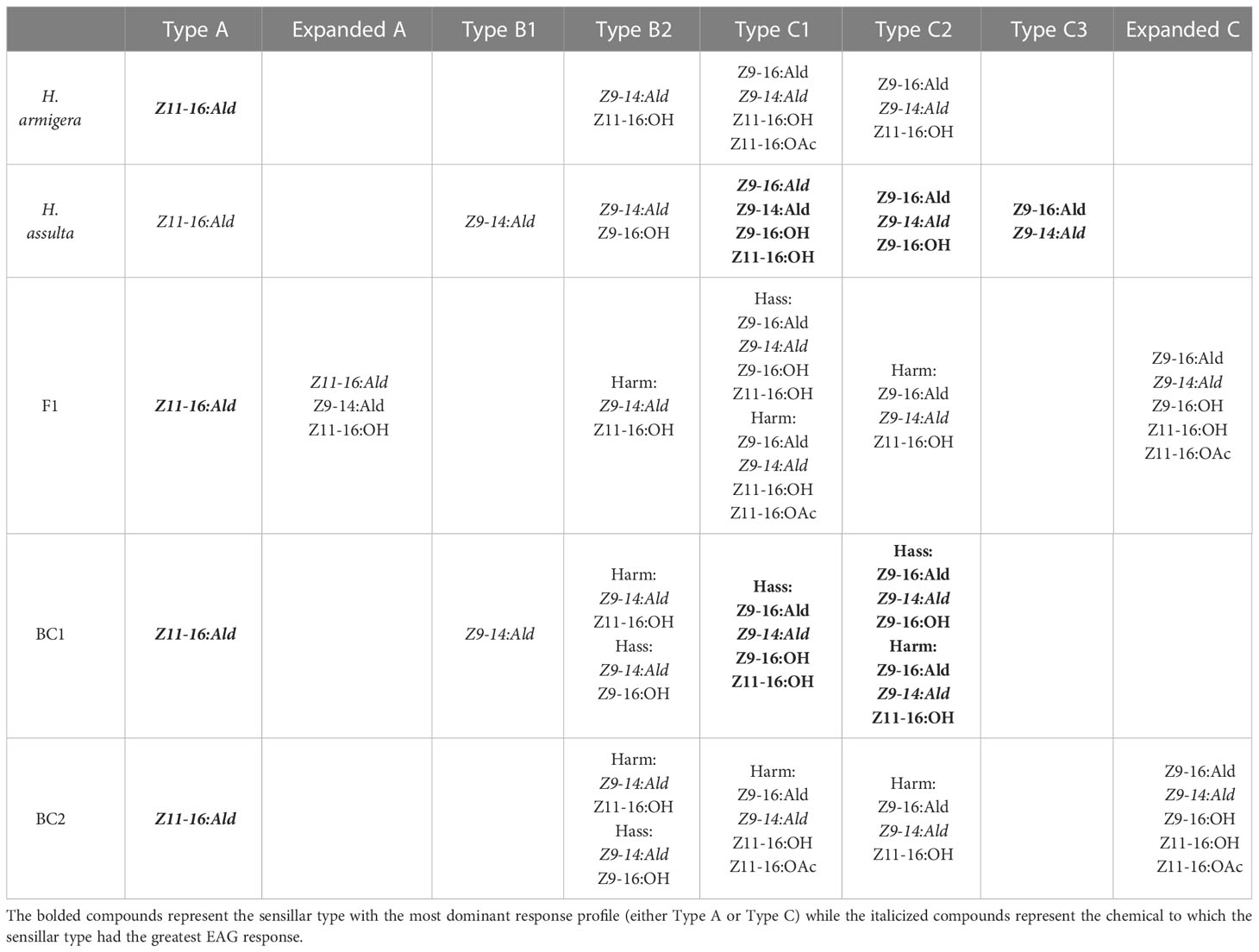
Table 3 Sensillar types and the compounds to which they are responsive in H. armigera, H. assulta, and their F1 hybrids and BC1 (F1 x female H. armigera) or BC2 (F1 x female H. assulta) backcrosses as studied by Xu et al. (2017).
The work of Xu et al. (2017) provides a link between changes in sensillar profiles and antennal lobe morphology, proving that hybridization can alter olfactory pathways at both levels of the nervous system (peripheral and central). The emergence of a new subtype of sensilla could provide hybrids with an adaptational advantage; since the pheromone blends of females are under less selective pressure and therefore more susceptible to variation, the ability of males to detect these new variations could improve their fitness (Xu et al., 2017). Female H. armigera x male H. assulta hybrids are easier to produce than the opposite hybrid, and these hybrids are more likely to be attracted to H. armigera females based on their responses to their blend (Xu et al., 2017). These factors, in combination with the high success of the BC2 backcross, mean BC2 would likely be the dominant cross in the wild (Xu et al., 2017). Since BC2 individuals have an H. armigera-like male olfactory system and female sex pheromone synthesis, they could potentially backcross with H. armigera, allowing H. assulta genes to introgress, new types of sensilla with broader tuning to emerge, and H. armigera fitness to increase (Xu et al., 2017). The success of H. armigera as an Old World pest may be attributed to similar mechanisms, and their increased fitness under these circumstances could lead to evasion of existing pest management strategies reliant on pheromones (Xu et al., 2017). Furthermore, the potential introgression of olfactory receptor genes and the presence of more broadly-tuned sensillae could lead to altered sensitivity to novel pheromone blends, resulting in changes in male preference and eventual speciation within hybrid zones (Xu et al., 2017).
Genetics of H. armigera x H. assulta crosses
To date, the only genetic study done on H. armigera x H. assulta crosses was by Guo and colleagues in 2022, analyzing the connection between gene expression and the divergence of pheromone sensing in the two species (Guo et al., 2022). Allele-specific expression of cis-regulatory genes (segments of non-coding DNA which regulate transcription of neighboring genes, such as enhancers, promoters, or silencers) and trans-regulatory genes (segments of coding DNA which regulate transcription of distant genes) was monitored in F1 hybrids (Wang et al., 2019; Guo et al., 2022). The crosses of H. armigera females and H. assulta males produced some fertile males, some abnormal males, and all abnormal females, while the reciprocal cross produced fertile males and females (Figure 3; Guo et al., 2022). Parental males and normal hybrid males from both crosses had strong electrophysiological responses to Z11-16:Ald, but abnormal males had a very low response (Guo et al., 2022). For Z9-16:Ald and Z9-14:Ald, H. assulta males had the strongest responses, abnormal male hybrids almost no response, and all other hybrid males intermediate responses (Guo et al., 2022). Transcriptome reads identified greater expression of H. assulta genes in all F1 hybrids, suggesting that more cis-regulatory gene changes are fixed in this species (Guo et al., 2022). Principal component analysis revealed large variances between sterile and normal hybrids, and H. armigera genes were more variable in their expression in sterile F1 hybrids and H. armigera female x H.assulta male hybrid males (Guo et al., 2022). The most variable genes in the abnormal hybrids had functions relating to sex pheromone detection, reproduction, and development, which are likely the cause of differences in pheromone perception, sterility, and abnormal morphology in these hybrids (Guo et al., 2022). Abnormal hybrids had more mis-expressed genes in their antennae, particularly genes with pheromone reception, explaining their low electrophysiological responses (Guo et al., 2022). Even normal hybrids of the H. assulta female x H. armigera male crosses had abnormal gene expression in antennae (Guo et al., 2022). Incompatibility of genes on the chromosomes of F1 hybrids was the likely cause of mis-expressed genes, leading to developmental defects and divergences in regulatory patterns in different hybrids (Guo et al., 2022). Antennal gene expression was sexually dimorphic, with many all cis-regulatory genes being more expressed in males and more trans-regulatory genes being expressed in females, potentially contributing to sexual dimorphism in pheromone detection (Guo et al., 2022). Trans-regulatory genes are more pleiotropic, usually affecting multiple phenotypic traits and leading to increased variation (Guo et al., 2022). Female antennae must be adapted to a wide range of host plant volatiles, making trans-regulatory genes more appropriate for this function, while the more tightly-regulated cis-regulatory genes in male antennae are more conducive to being finely-tuned to species-specific female sex pheromone blends (Guo et al., 2022). Cis-regulatory elements generally have larger and faster effects on gene expression, which could be advantageous for males if changes in female sex pheromone blends require rapid adaptation via changing the expression of ORs (Guo et al., 2022). Hybrid males were the most accurate predictors of regulatory patterns, particularly the H. armigera female x H. assulta male hybrids, so these hybrids were used to study regulatory patterns of olfactory receptor genes (i.e., ORs, odorant binding proteins, sensory neuron membrane proteins) (Guo et al., 2022). The results suggested that cis-regulation of genes is an important factor in differences in olfactory sensation between H. armigera and H. assulta males, particularly HarmOR13 (responsible for detecting Z11-16:Ald the major component of the H. armigera blend) and HassOR14 (responsible for detecting Z9-16:Ald, the major component of the H. assulta blend), which were both cis-regulated and more highly expressed in the hybrid antennae than their counterparts in the other parental species (Table 1; Guo et al., 2022). However, it is important to consider that other non-OR genes or transcription factors may be important in male adaptation and could take effect even before changes in female pheromone blends occur (Unbehend et al., 2021). As such, many yet-to-be identified genes and gene functions may influence olfactory sensitivity and pheromone preference. For example in Ostrinia nubilalis, the cis-regulatory element bric à brac, encoded by the bab1 gene expressed early in antennal development, is the key locus for variation in male preference (Unbehend et al., 2021).
Heliothis virescens and Heliothis subflexa
First laboratory studies of H. virescens x H. subflexa hybrids
Arguably the most-studied heliothine hybrid is the Heliothis virescens x Heliothis subflexa cross. The hybrid was first investigated by Laster (1972) as a potential method for controlling crop damages via genetic suppression, with the two species being chosen due to their close relation and overlapping ranges in Mississippi, USA, although they do overlap in other parts of the Americas (Laster, 1972; Hillier and Baker, 2016). While H. virescens females and H. subflexa males mated but did not produce offspring in Laster’s experiments, H. subflexa females and H. virescens males readily mated and produced offspring (F1) (Figure 4; Laster, 1972). Male offspring were sterile, and backcrossing hybrid females (F1) with H. virescens males passed this sterility onto their male offspring (BC1) (Figure 4; Laster, 1972). Using H. virescens males in the crosses always produced offspring, while all other combinations of H. virescens, H. subflexa, F1, BC1, and BC2 individuals did not result in offspring (Figure 1; Laster, 1972). This data provided evidence that control of H. virescens could be achieved using sterile F1 males from the H. subflexa female x H. virescens male cross (Figure 4; Laster, 1972). However, since F1 females could mate with H. virescens males, the authors suggested that characteristics of their offspring (such as pesticide resistance and feeding habits) needed to be monitored in case F1 females were accidentally released during sterile-male programs (Figure 4; Laster, 1972).
Following the discovery of this hybrid by Laster (1972); Proshold and Lachance (1974) investigated the fertility of hybrids, as well as the genetic and physiological mechanisms underlying their sterility. Both parental crosses from the Laster (1972) experiment were studied, and the hybrids resulting from these crosses were backcrossed to males or females of the parental species, although only offspring resulting from the hybrids crossed with H. virescens were used for further backcrosses (Figure 4; Proshold and Lachance, 1974). The progeny of hybrids x H. virescens were BC1 while the progeny of BC1 x H. virescens were BC2 (Figure 4; Proshold and Lachance, 1974). From replications of the parental crosses, all produced offspring, but the H. subflexa females x H. virescens males were the most successful hybrid cross, with 75.4% mating and 56% of females producing progeny (Figure 4; Proshold and Lachance, 1974). Both types of hybrid larvae had similar survival rates as H. virescens larvae, with 87% pupating (Proshold and Lachance, 1974). Male F1 hybrids from both parental crosses mated readily with females of either species (89.6–97.2% of the time), but hybrid males backcrossed with H. virescens females tended to mate more frequently (~3 times) than those backcrossed with H. subflexa females (~2 times) (Figure 4; Proshold and Lachance, 1974). Although hybrid males from the H. virescens male x H. subflexa female cross were more fertile (2–6.2% egg hatch) than the H. virescens female x H. subflexa male cross (<1%), none of these males could induce a normal egg-laying response in the females they mated with (Proshold and Lachance, 1974). Most hybrid males from both crosses were sterile since they could not transfer eupyrene (nucleated) sperm to females to fertilize their eggs (Proshold and Lachance, 1974). Regarding female F1 hybrids, those from the H. subflexa female x H. virescens male cross had low mating success and egg-laying, but high percentages of eggs hatching (Figure 4; Proshold and Lachance, 1974). Female F1 hybrids from the H. subflexa male x H. virescens female cross were more successful at mating and egg-laying but had only moderate egg-hatching rates (Figure 4; Proshold and Lachance, 1974). The BC1 male offspring from crosses of hybrid females (H. virescens males x H. subflexa females) and male H. virescens were sterile or semi-sterile, delivering no or irregular sperm in most instances, but BC1 females were fertile (Figure 4; Proshold and Lachance, 1974). Crosses between BC1 females x H. virescens males produced sterile males and fertile females (BC2), while crosses between BC1 males x H. virescens females produced fertile males and females (Figure 4; Proshold and Lachance, 1974). The cross between H. virescens females x H. subflexa males became more fertile with each generation, so BC2 individuals were partially or fully fertile (Figure 4; Proshold and Lachance, 1974). When hybrid females and their female offspring were crossed with H. virescens males, male offspring showed increased fertility (Figure 4; Proshold and Lachance, 1974). Both H. virescens and H. subflexa males had 2n = 62 chromosomes, and thus each gamete should contain 31 chromosomes (Proshold and Lachance, 1974). However, only 20–28 chromosomes were found in all types of hybrid males, while the other chromosomes were unpaired (Proshold and Lachance, 1974). The lack of pairing was attributed to desynapsis, a failure in the fusion of chromosome pairs due to homologous chromosomes separating after meiosis (Proshold and Lachance, 1974). Overall, the researchers found that reproductive capacity in both male and female hybrids was reduced, with males being sterile or semi-sterile and females having reduced fecundity or fertility (Proshold and Lachance, 1974). Male sterility was attributed to the absence of eupyrene sperm in females who mated with hybrid males, possibly due to lower production or abnormal activity of the sperm (Proshold and Lachance, 1974). These deficiencies were attributed to desynapsis, as continued backcrossing of fertile BC1 hybrid females reintroduced chromosomal homology, allowing some BC2 males to be fertile (Figure 4; Proshold and Lachance, 1974). Desynapsis was also the explanation for reduced egg hatches in hybrid females (Proshold and Lachance, 1974).
Mating experiments further indicated that the female mating urge was not adequately served by hybrid males, particularly in the case of female H. subflexa which tended to only mate with one normal male but had to mate several times with hybrid males (Proshold and Lachance, 1974). The authors suggested that hybrid females could be used to suppress H. virescens males in the field since they produced sterile male offspring when crossed (Proshold and Lachance, 1974). However, key differences in the mating behaviors and fertility of each hybrid pose challenges. While the female H. subflexa x male H. virescens hybrid females tended to enter diapause more often than regular females and mated poorly with normal males, they did produce sterile BC1 males and fertile BC1 females, who could then be crossed with male H. virescens to produce more sterile BC2 male hybrids (Figure 4; Proshold and Lachance, 1974). Alternatively, while the female H. virescens x male H. subflexa hybrids did not diapause, mated readily, and had reduced fertility, BC1 and BC2 offspring could have fertility restored, making them less suitable for population control (Figure 4; Proshold and Lachance, 1974).
In a 1975 follow-up study, Proshold and LaChance studied sperm production and transfer by the H. subflexa x H. virescens hybrid males compared to their parent species (Proshold et al., 1975). While both parent and hybrid males produced the same number of spermatophores, when the hybrids mated with females, only apyrene (non-nucleated) sperm were delivered to the female spermatheca (Proshold et al., 1975). Although it would seem that hybrid males incapable of delivering viable sperm to females would have no influence on female oviposition and calling, and thus no benefit in population suppression, hybrid males could make up for this insufficiency with hybrid vigor, outcompeting irradiated males traditionally used for population control (Proshold et al., 1975). Further research the same year concluded that abnormal morphology of hybrid male sperm (such as the presence of two-tailed sperm) and lower counts of eupyrene sperm were likely the cause of their sterility (Richard et al., 1975). This would later be corroborated by a 1977 study by Karpenko and Proshold, which showed that females crossed with BC1 males (the offspring of crosses between hybrid females and H. subflexa males) did not often receive eupyrene sperm, although females mated with BC2 males were twice as likely to receive eupyrene sperm than from BC1 males (Figure 4; Karpenko and Proshold, 1977). Laster and colleagues were able to maintain sterile hybrid males and fertile hybrid females over 40 generations by mating backcross hybrid females with H. virescens males (Figure 4; Laster et al., 1976). Their research led to the development of a potential population suppression strategy: fertile hybrid females released in sufficient numbers could outcompete native H. virescens females, mating with normal H. virescens males to create more sterile male hybrids (Figure 5; Laster et al., 1976; Parvin et al., 1976). The principle was that over many generations, more normal H. virescens females would die unmated and sterile males would dominate, leading to death of the native H. virescens population (Figure 5; Laster et al., 1976; Parvin et al., 1976). Their model was further supported by the discovery that offspring of hybrid crosses had mating behaviors similar to those of normal H. virescens and that pheromone blends of both hybrid females and H. virescens females were equally attractive to H. virescens males in trapping experiments (Laster et al., 1977; Pair et al., 1977; Laster et al., 1978). Furthermore, female progeny of backcrosses between H. virescens males and hybrid females (H. subflexa female x H. virescens male) were found to attract H. virescens males equally as well as normal H. virescens females (Figures 4, 5; Tingle et al., 1978). Male progeny of these backcrosses were attracted to both H. virescens females and their pheromone extracts, while F1 hybrid males did not respond to the pheromone (Tingle et al., 1978). However, in 1979, Makela and Huettel made amendments to the Laster-Parvin model, as Laster and colleagues had incorrectly assumed that H. virescens populations would be driven to extinction regardless of the ratio of hybrid to native moths upon release (Figures 5, 6; Laster et al., 1976; Parvin et al., 1976; Makela and Huettel, 1979). Makela and Huettel proposed that in the Laster-Parvin model, the ratio of hybrid females to native H. virescens would remain constant, assuming no immigration occurs (Figures 5, 6; Makela and Huettel, 1979). With this assumption in mind, the success of the model would be dependent on the insemination rate of H. virescens males and the growth rate of the population (Figures 5, 6; Makela and Huettel, 1979). In application, migration would likely decrease the ratio of hybrid females and backcross offspring to native H. virescens, leading to diminishing hybrid populations and thus a diluted effect (Figures 5, 6; Makela and Huettel, 1979). Furthermore, the effects of selection against hybrid females and assortative mating in the wild would need to be considered (Figures 5, 6; Makela and Huettel, 1979). To maintain the population suppression effects of the hybrids, several releases of fertile hybrid females would likely need to be done with careful monitoring of the ratio of the hybrid and native populations throughout the program (Figures 5, 6; Makela and Huettel, 1979).
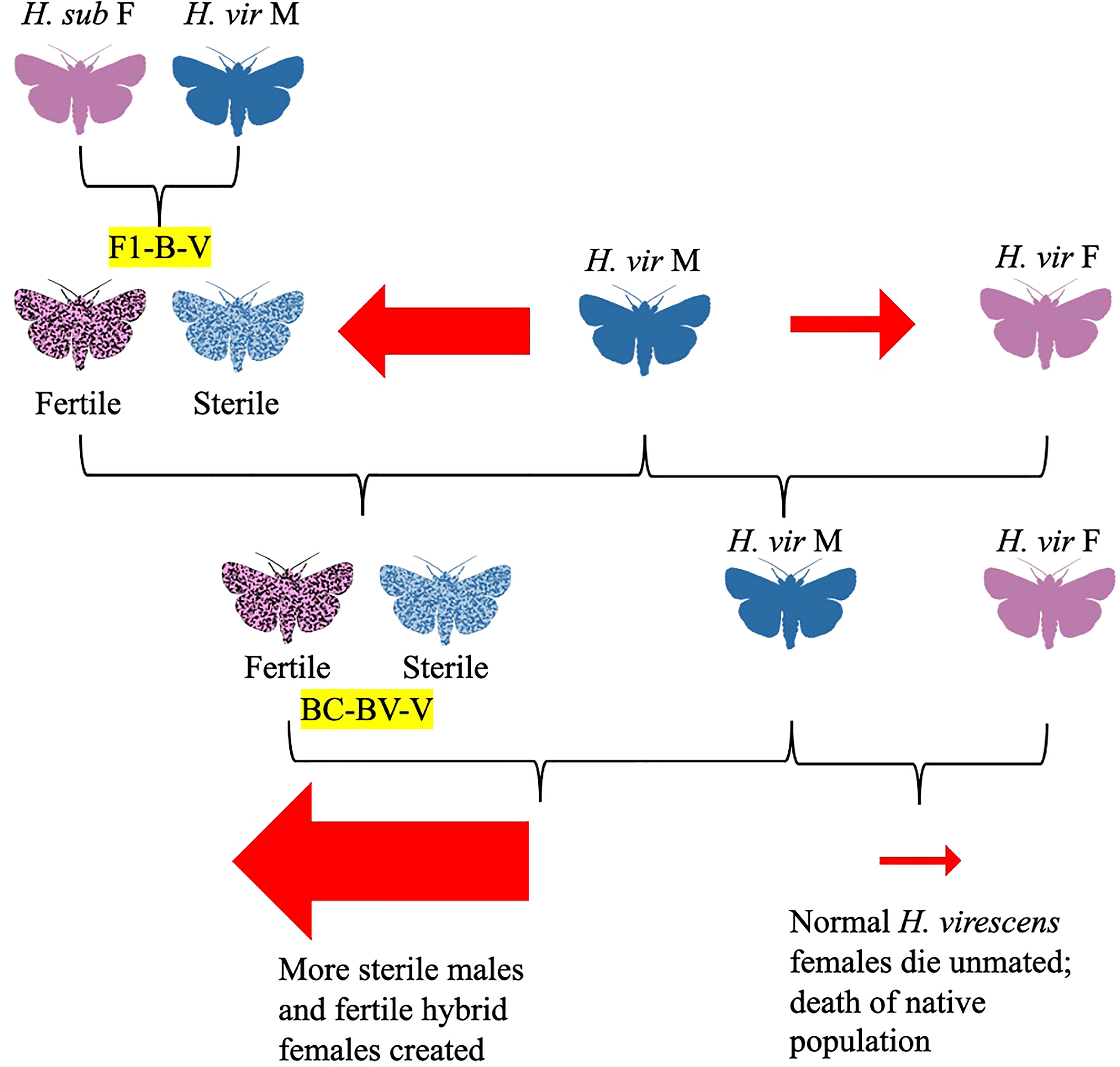
Figure 5 Diagram depicting the Laster-Parvin Model (Laster et al., 1976; Parvin et al., 1976). According to this model, the hybrid female to native female ratio would increase over generations.
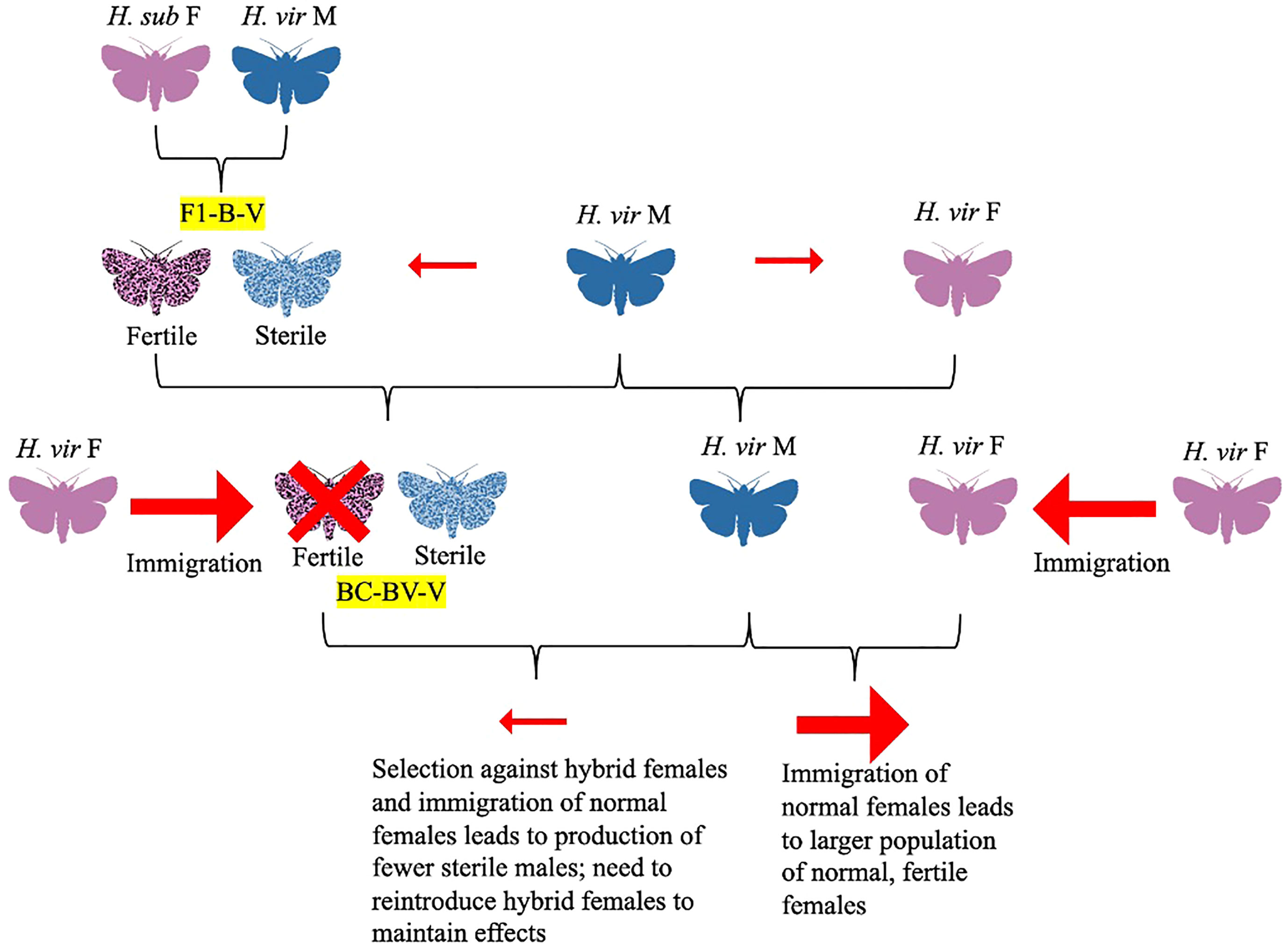
Figure 6 Diagram depicting the Makela-Huettel Model (Makela and Huettel, 1979). In the Laster-Parvin model, the hybrid female to native female would be constant over generations, assuming no immigration of females (Laster et al., 1976; Parvin et al., 1976). However, the Makela-Huettel model proposes that the success of the hybrid sterility program would depend on the insemination rate of H. virescens males and growth rate of the population (must be hybrid>native). Immigration of native females would likely decrease the hybrid female to native female ratio. Selection against hybrid females and assortative mating also need to be assessed. To maintain the effects of the hybrid sterility program, several releases of hybrid females (ideally from BC7 or greater) should be accompanied by monitoring of hybrid to native female ratio.
The viability of the Laster-Parvin model (and the further corrections by Makela and Huettel) were investigated by Cibrian-Tovar and Mitchell, specifically by comparing the courtship behaviors of parental H. subflexa and H. virescens to their hybrids and backcrosses (Figures 5, 6; Cibrian-Tovar and Mitchell, 1991). Mating behaviors of male and female H. subflexa, H. virescens, and backcross hybrids (H. virescens males mated with hybrid females derived from H. virescens males x H. subflexa females) were observed in a wind tunnel (Figure 4; Cibrian-Tovar & Mitchell, 1991). When observing pre-copulatory calling behavior, backcross females exhibited similar behaviors to normal H. virescens females, and their calling period overlapped with that of H. subflexa females, making the calling periods of the backcrosses and parental species indistinguishable (Cibrian-Tovar and Mitchell, 1991). Backcross males were much less active than H. virescens males, and in mating experiments, 20–25% were not responsive to calling virgin H. subflexa or backcross females (Cibrian-Tovar and Mitchell, 1991). Similar to H. virescens males but unlike H. subflexa males, backcross males did not touch the ovipositors of calling females with their antenna, and likely only attracted females with displays from hair-pencil glands, structures which secrete sex pheromones (Cibrian-Tovar and Mitchell, 1991). Only one-third of backcross males mated successfully, their poor performance attributed to their relative flight inactivity, inability to move parallel to the calling females, and improper copulation (Cibrian-Tovar and Mitchell, 1991). Many unsuccessful males spent more time copulating or sitting on the walls of the wind tunnel than successful males (Cibrian-Tovar and Mitchell, 1991). When comparing between H. virescens, H. subflexa, and backcross insects, their flight orientation, landing, and copulatory behaviors were very similar, but they differed mostly in closer-range behaviors (Cibrian-Tovar and Mitchell, 1991). Because backcross males from earlier generations (1st to 6th) were found to be much more inactive than their parental species, while later generations (7th and beyond) were comparable to normal H. virescens males, the authors suggested the use of 7th generation or greater backcross hybrids for field suppression of H. virescens, as well as further research into post-mating behaviors (Figure 4; Cibrian-Tovar and Mitchell, 1991).
Abnormalities in sperm and consequences for sterility of H. virescens x H. subflexa hybrids
Throughout the 1980s, further evidence was gathered indicating abnormal sperm as a contributor to hybrid sterility (Goodpasture et al., 1980; Lachance and Karpenko, 1983; Miller et al., 1986; Lachance and Olstad, 1988; Degrugillier, 1989; Degrugillier and Newman, 1993). In 1980, Goodpasture and colleagues found that chromosome pairing remained normal in later generations of H. virescens x H. subflexa backcrosses and the presence of multiple-tailed sperm diminished (Goodpasture et al., 1980). However, sperm production remained abnormal to the point where parental hybrid males (H. subflexa females x H. virescens males) could be distinguished from backcross males (F1 hybrid females x H. virescens males) by sperm morphology (Figure 4; Goodpasture et al., 1980). The authors suggested that these abnormalities could be used to screen for normal and hybrid males in field experiments and hybrid sterility programs (Goodpasture et al., 1980). In 1983, LaChance and Karpenko subjected backcross males to antiviral treatments and extreme heat and still detected hybrid sterility, suggesting that a microorganism was not responsible for hybrid sterility (Lachance and Karpenko, 1983). In 1986, Miller and colleagues presented evidence that abnormalities in mitochondrial function, particularly RNA metabolism, played a factor in hybrid male sterility (Miller et al., 1986). This was corroborated by a 1988 study by LaChance and Olstad which found abnormal mitochondrial derivatives in many backcross males (Lachance and Olstad, 1988). Studies by Degrugillier (1989) and Degrugillier and Newman (1993) pointed to the presence of virus-like particles (VLPs) in the spermatocyst and follicle cells of H. virescens, H. subflexa, F1 (H. virescens males x H. subflexa females and the reciprocal cross), and backcross males (F1 females x H. virescens males) (Figure 4; Degrugillier, 1989; Degrugillier and Newman, 1993). In the parental species, infection was dependent on age, ranging from 4–8% of young males to 90% in older males, while prevalence in backcross males was 100% (Degrugillier, 1989). There was a high correlation between the presence of VLPs in certain males and abnormal sperm, and many F1 and BC1 males presented with multiple-tailed eupyrene sperm (Figure 4; Degrugillier and Newman, 1993). The VLPs were hypothesized to be fragments of a hereditary virus that has become integrated into the genomes of various Heliothis and Helicoverpa species via retrotransposons (Degrugillier and Newman, 1993). Despite this, the authors also suggest that VLPs could appear in backcross spermatocysts secondarily due to stressors, such as the presence of organisms resembling rickettsia bacteria in the testes and sperm, although this would contradict the findings of Lachance and Karpenko (1983).
Genetics, electrophysiology, and behavior in relation to sex pheromones and hair-pencil compounds in H. virescens x H. subflexa hybrids
In addition to reproductive isolating mechanisms such as gametic isolation, hybrid sterility, and hybrid viability, the effects of behavioral isolation (specifically sex pheromone blend composition) have been investigated in relation to the hybridization of H. subflexa and H. virescens. In 1993, Teal and Oostendorp studied the inheritance of hair-pencil glands in H. subflexa x H. virescens hybrids (Teal and Oostendorp, 1993). Hair-pencil glands are structures found on some male lepidopterans which are responsible for secreting sex pheromones that attract conspecific females and repel conspecific males (Hillier and Vickers, 2004; Hillier and Baker, 2016). Male H. virescens have hair-pencil glands, while male H. subflexa do not (Brazzel et al., 1953). Their hybrids and some backcrosses were found to exhibit hair-pencil glands similar to those of H. virescens, suggesting that the presence of hair-pencils is a dominant sex-linked trait of males controlled by the Z chromosome (Teal and Oostendorp, 1993). Despite this, a 1995 follow-up study by the pair found that production of hair-pencil pheromones was under autosomal control and that pheromone blends of backcross males were actually more similar to H. subflexa in some cases (Teal and Oostendorp, 1995). The hair-pencil glands of parental H. virescens males had 16:OAc and 16:OH in a 4:1 ratio as their primary components, while H. subflexa males showed minute amounts of 16:OH and traces of other compounds in their abdomens, although none were characteristic of the species (Teal and Oostendorp, 1995). The average ratio of 16:OAc and 16:OH in H. subflexa female x H. virescens male hybrids, their reciprocal cross, and backcross males was approximately 1:4, showing greater similarity to H. subflexa males in all cases (Figure 4; Teal and Oostendorp, 1995). Therefore, the production of these compounds was deduced to be under the control of dominant autosomal genes from the H. subflexa genome. This work in male hybrids was compared to previous work by Klun and colleagues (1982) in hybrid females, in which females produced from crosses between H. subflexa females x H. virescens males and backcrosses of these hybrid females to H. virescens males were shown to produce sex pheromone blends similar to H. virescens females (Figure 4; Klun et al., 1982; Teal and Oostendorp, 1995). Thus, the authors concluded that unlike males, the regulation of sex pheromone production in hybrid females is under the control of dominant autosomal genes from the H. virescens genome (Klun et al., 1982; Teal and Oostendorp, 1995). Later research would confirm that such dominant genes include those which control the ratios of 14-carbon aldehydes in the female pheromone blend and the activity of several biosynthetic enzymes in the female pheromone glands (acetyl transferase, desaturases, and a fatty acyl reductase or alcohol oxidase) (Teal and Tumlinson, 1997; Groot et al., 2009b). Further research into pheromone production by Teal and Oostendorp found that while F1 females from the H. subflexa females x H. virescens males cross had similar pheromone blends to H. virescens females, they have no periodicity in their calling period, which could explain the poor mating efficacy of this hybrid as previously documented, although not all hybrid females are affected (Figure 4; Proshold and Lachance, 1974; Karpenko and Proshold, 1977; Teal and Oostendorp, 1995). However, in the reciprocal cross (H. subflexa male x H. virescens female), periodicity of pheromone calling matched the parental species, which the authors hypothesized was the likely cause of their mating success with both (Figure 4; Proshold and Lachance, 1974; Teal and Oostendorp, 1995). Only when the hybrid females were injected with pheromonotropic substances could they adequately produce blends in amounts similar to or greater than the parental species, suggesting the presence of hybrid vigor (Teal and Oostendorp, 1995). The inadequate production and mature eggs observed in these hybrids lead the authors to conclude that hybridization could lead to reproductive arrest, perhaps due to a lack of juvenile hormone (Proshold and Lachance, 1974; Teal and Oostendorp, 1995).
Although previous work had proven that the offspring of hybrid females backcrossed with H. virescens males could produce a pheromone blend identical to normal H. virescens females, and thus compete with these normal females in hybrid sterility-based control programs (Klun et al., 1982; Cibrian-Tovar and Mitchell, 1991; Teal and Tumlinson, 1997), and that hybrid males could produce hair-pencil compounds (male courtship pheromones) similar to those of H. subflexa, comprehensive behavioral studies were lacking. In 2006, Vickers investigated the effects of female blend composition on the responses of hybrid males. In the wild, reproductive isolation between H. virescens and H. subflexa is maintained by H. subflexa females producing specific levels of acetates (including Z11-16:OAc) to attract H. subflexa males and repel male H. virescens, and H. virescens females failing to produce adequate levels of Z9-16:Ald and Z11-16:OH, which are needed to attract H. subflexa males (Table 1; Figure 1; Groot et al., 2006; Vickers, 2006a). Hybrids (both H. virescens male x H. subflexa female and H. virescens female x H. subflexa male, but particularly the former) were found to be highly attracted to blends of Z11-16:Ald, Z9-16:Ald, and Z11-16:OH in a 1:0.5:0.1 ratio with or without the addition of 0.1 Z11-16:OAc, which was also found to be attractive to H. subflexa males (Figure 1; Figure 4; Vickers, 2002; Vickers, 2006a). Replacing Z9-16:Ald with 0.1 Z9-14:Ald (an important compound in the H. virescens blend) made the blend significantly less attractive to H. virescens male x H. subflexa female hybrids, but not the reciprocal cross, although there was some variability between individuals (Figure 1; Figure 4; Vickers, 2006a). These results indicated that the inheritance of sex pheromone olfactory reception genes was co-dominant or exhibited incomplete dominance (Vickers, 2006a). This characteristic was also evident in electrophysiological data, which indicated that olfactory pathways in hybrids were equally tuned to Z9-16:Ald and Z9-14:Ald (Figure 1; Vickers, 2006a). The hybrids were also much more attracted to blends containing Z11-16:OH, suggesting a dominant effect of H. subflexa genes on sex pheromone perception (Figure 1; Vickers, 2006a). However, not all hybrid males needed Z11-16:OH to show a response, indicating variability in male behavior and OSN composition on the antennae (Vickers, 2006a). The B-type and C-type sensillae of hybrid males showed a range of similarities to each parental species, and males with sensillae more similar to those of H. virescens could be able to respond to blends lacking Z11-16:OH (Figure 1; Vickers, 2006a). The responses of hybrids to Z11-16:OAc also indicated variability in C-type sensillae, as it repelled certain hybrid males but had no effect on most others (Figure 1; Vickers, 2006a). Z11-16:OAc may function behaviorally as an attractant in blends (i.e. produced by female H. subflexa to attract conspecific males) or more frequently to antagonize heterospecific males (i.e. it is inhibitory to male H. zea and male H. virescens when presented in otherwise attractive blends) (Vickers, 2002; Groot et al., 2006; Hillier and Baker, 2016). Electrophysiological recordings from OSNs in trichoid sensillae showed that hybrid males produced from crosses between female H. subflexa and male H. virescens had spike amplitudes and OSN co-compartmentalization more similar to H. subflexa, while dose-response profiles were mostly intermediate between the two parental species (Figure 1; Figure 4; Baker et al., 2006). In A-type hybrid sensillae, there was a response to Z11-16:OH not present in either parental species (Figure 1; Baker et al., 2006). Hybrid males showed higher sensitivity to Z9-14:Ald (a compound in the H. virescens blend) in B-type sensillae OSNs normally responsive to Z9-16:Ald and seemed to be able to substitute between Z9-14:Ald and Z9-16:Ald sensitivity unlike either of their parental species (Table 1; Figure 1; Baker et al., 2006). This shift between the two compounds was hypothesized to be a result of two different types of pheromone receptors being co-expressed on the same OSNs, with variation in the hybrid population being attributed to different co-expression of parental alleles or receptors being coded for by different genes (Baker et al., 2006). The C-type sensillae of hybrid males also contained OSNs which showed decreased cross-sensitivity to Z11-16:OAc and Z9-14:Ald compared to parental H. subflexa males, although they still retained more similarity to this species than to H. virescens (Figure 1; Baker et al., 2006). To further the understanding of H. subflexa x H. virescens hybrid olfactory processing, the projections of pheromone-receptive neurons to specific areas of the brain was investigated (Vickers, 2006b). The hybrid A-type sensillae OSNs detecting Z11-16:Ald/Z11-16:OH projected to the cumulus, just like the A-type sensillae of the parental species, although the parental species only detect Z11-16:Ald (Figure 1; Vickers, 2006b). The hybrid B-type sensillae OSNs detecting Z9-16:Ald/Z9-14:Ald projected to the dorsomedial glomeruli (DM), just like the parental species, although the detection of Z9-16:Ald is absent in parental H. virescens and detection to Z9-14:Ald weak in parental H. subflexa (Figure 1; Vickers, 2006b). The hybrid C-type sensillae detecting Z11-16:OAc/Z9-14:Ald projected to the ventromedial glomeruli (VM), while the hybrid C-type sensillae detecting Z11-16:OH projected to the anteromedial glomeruli (AM) (Figure 1; Vickers, 2006b). Once again, these projections were the same as the parental species, but parental H. virescens detect Z11-16:OH/Z9-14:Ald and Z11-16:OAc, while parental H. subflexa detect Z11-16:OAc/Z9-14:Ald and Z11-16:OH, making the hybrid C-type sensillae identical to H. subflexa (Figure 1; Vickers, 2006b). Based on the evidence gathered cross the three studies, the researchers concluded that H. subflexa genetic factors were dominant in determining pheromone blend preference in males (Baker et al., 2006; Vickers, 2006a; Vickers, 2006b).
Further research on the genetic mechanisms underlying sex pheromone production in the H. virescens x H. subflexa hybrid was conducted in 2009 by Groot and colleagues. In this study, H. virescens females were crossed with H. subflexa males, and the resulting F1 hybrid females were crossed with either H. subflexa males (S-backcrosses) or H. virescens males (V-backcrosses) (Figure 4; Groot et al., 2009b). Normally, male H. virescens respond to Z11-16:Ald and Z9-14:Ald, the primary and secondary components of the conspecific female blend, respectively (Table 1; Figure 1). Male H. subflexa primarily respond to Z9-16:Ald and Z11-16:OH and show an enhanced response to Z11-16:OAc, a compound which repels H. virescens (Table 1; Figure 1). In the V-backcrosses, Hs chromosome 24 was associated with higher production of Z9-16:Ald (from the H. subflexa blend), while Hs chromosome 7 was associated with lower production of Z9-14:Ald (from the H. virescens blend) (Groot et al., 2009b). In the S-backcrosses, the presence of Hv chromosomes 14, 15, 19, and 22 was associated with higher production of Z9-14:Ald (from the H. virescens blend) and its precursor tetradecenal (14:Ald), while Hv chromosomes 19 and 24 were associated with lower production of Z9-16:Ald (from the H. subflexa blend) (Table 1; Groot et al., 2009b). Decreased production of acetates from the H. subflexa blend was associated with Hv chromosomes 4 and 22 (Groot et al., 2009b). Based on this analysis, it was confirmed that female sex pheromone production is under the control of several quantitative trait loci (QTL), regions of DNA consisting of several genes that can be expressed in different combinations to produce a phenotypic trait with varying degrees (Members of the Complex Trait Consortium, 2003; Groot et al., 2009b). Many of these QTL were responsible for the activity of several biosynthetic enzymes, such as acetyl transferase, desaturases, and a fatty acyl reductase or alcohol oxidase, and corroborated earlier evidence that dominant genes from the H. virescens genome influence female sex pheromone production (Teal & Oostendorp, 1995; Teal and Tumlinson, 1997; Groot et al., 2009b).
In 2010, another analysis of QTL was performed by Gould and colleagues, this time focusing on sex pheromone perception rather than production (Groot et al., 2009b; Gould et al., 2010). As in the study by Groot and colleagues in 2009, H. virescens females were crossed with H. subflexa male hybrids, and females resulting from this cross were mated with either H. virescens males (creating Hv-BC offspring) or H. subflexa males (creating Hs-BC offspring) (Figure 4; Groot et al., 2009b; Gould et al., 2010). This study found that the responses of males to key components from female pheromone blends were controlled by a single QTL (Gould et al., 2010). Hv-BC and Hs-BC males flew towards a source of Z9-16:Ald at rates of 54% and 40%, respectively (Gould et al., 2010).The researchers found that responses to these compounds were under the control of at least four OR genes on chromosome 27, based on an analysis of backcrosses (Gould et al., 2010). Females with Hs-c27 were repeatedly backcrossed to H. virescens males, and 5th and 15th generation males with Hs-c27 had significantly higher attraction to blends containing Z9-16:Ald (Gould et al., 2010). Backcross individuals heterozygous for Hs-c27/Hv-c27 tended to respond less to blends with Z11-16:OAc, while 67% responded to blends without Z11-16:OH (Gould et al., 2010). In backcross individuals homozygous for Hv-c27, 25% responded less to Z11-16:OAc, while 77% of backcross individuals homozygous for Hs-c27 responded to blends without Z11-16:OH, much like heterozygous individuals (Gould et al., 2010). Pure H. virescens B-type OSNs were activated by Z9-14:Ald, not Z9-16:Ald, pure H. subflexa B-type OSNs were more sensitive to Z9-16:Ald, but backcross individuals with all H. virescens genes but having Hs-c27 had their B-type OSNs respond like pure H. subflexa individuals (Figure 1; Gould et al., 2010). Pure H. virescens C-type OSNs responded to Z11-16:OH and Z11-16:OAc, and pure H. subflexa C-type OSNs only responded strongly to Z11-16:OH, while backcrosses with all H. virescens genes but having Hs-c27 had C-type OSNs respond similarly to pure H. subflexa individuals (Figure 1; Gould et al., 2010). The gene HR14 which binds Z11-16:OAc was shown to have a significant effect on differences in response profiles between species, while the genes HR16 (which binds Z11-16:OH), HR15 and HR6 were also suspected to be responsible for differences in sensitivity to Z9-16:Ald and Z9-14:Ald (Gould et al., 2010). Different combinations of these genes in hybrids could result in variability of response profiles in males, thereby affecting speciation and subsequent isolation, as could the introduction of novel mutations (Gould et al., 2010). In 2021, Cao and colleagues showed that a single point mutation in the OR6 gene, which is primarily responsible for detecting Z9-16:Ald in H. subflexa and Z9-14:Ald in H. virescens, could alter the response profile of H. virescens to become more like that of H. subflexa, but not the other way around (Table 1; Figure 1; Cao et al., 2021). Thus, the H. subflexa OR6 must have evolved from the H. virescens OR6 (Cao et al., 2021). Evidence from Cao, Gould, and colleagues provides a genetic mechanism for the emergence of new sex pheromone tuning profiles and responses in males (Gould et al., 2010; Cao et al., 2021). This plasticity would allow males to adapt and optimize detection of novel female blends, providing a selective advantage not only to these males, but to females producing these blends.
Conclusion
The study of hybridization in heliothines is significant not only for the development of pest management programs, but in discerning evolutionary relationships between species and how they may have emerged due to the plasticity of pheromone blend production and detection (Yang and Wang, 2020). The ability to create sterile hybrids, such as with H. virescens and H. subflexa, presents a mechanism to suppress H. virescens populations. Normal H. virescens females will “waste” their reproductive capacity on mating with normal H. subflexa males, producing sterile males and reproductive females who can pass their sterility onto further generations by mating with normal H. virescens males (Figure 4; Laster, 1972). Autosomal genes from the H. subflexa genome for the production of hair-pencil compounds were dominant over those of H. virescens in their hybrids (Teal and Oostendorp, 1995a). In contrast, sex pheromone production in females of this cross is under the control of dominant autosomal genes from the H. virescens genome, meaning they could compete with normal H. virescens females in the wild (Klun et al., 1982; Teal & Oostendorp, 1995b). Hybrid males could outcompete normal males due to such hybrid vigor, particularly 7th generation or greater backcross hybrids which were found to be on par with normal H. virescens males (Figures 4–6; Proshold et al., 1975; Cibrian-Tovar & Mitchell, 1991). However, several measures need to be taken in such sterile hybrid programs. The amendments of Makela and Huettel to the Laster-Parvin model must be observed by coordinating several releases of hybrid females (ideally 7th generation or greater) while monitoring growth rates of the native and hybrid population, immigration of native females, potential selection against hybrid females, and potential assortative mating (Figures 5, 6; Laster et al., 1976; Parvin et al., 1976; Makela and Huettel, 1979). Additionally, reproductive F1 hybrid females can mate with normal H. virescens males to create sterile offspring, so these offspring must be monitored for pesticide resistance and feeding habits, lest they become a more destructive pest than the parental species (Figures 5, 6; Laster, 1972). Finally, not all hybrids are equally suited to sterile male programs, with reciprocal crosses having different advantages and disadvantages (Proshold and Lachance, 1974). The mechanism of sterility in these hybrids remains uncertain, with the question of sperm abnormalities being caused by live organisms (such as bacteria or viruses) or genomic integration of VLPs left open. If studied further, the cause could be understood, although the use of sperm abnormalities to screen for hybrids in the wild could currently be adopted even without this knowledge (Goodpasture et al., 1980; Lachance and Karpenko, 1983).
While hybrids may be beneficial for pest management in the case of H. virescens x H. subflexa, the presence of H. zea x H. armigera hybrids in the wild poses an issue, as the latter may be able to pass beneficial genes on to its hybrid offspring (Leite et al., 2017; Pearce et al., 2017; Cordeiro et al., 2020; Valencia-Montoya et al., 2020). Studies have shown that H. armigera has accumulated genes for Bt crop resistance, insecticide resistance (i.e., CYP337B3), and wider host range, while H. zea has lost genes for GRs, and detoxification of insecticides and host plant compounds (Pearce et al., 2017). Given that both species have high reproductive and dispersive abilities and are both polyphagous, a combination of their most advantageous traits in a hybrid could create a problematic pest (Leite et al., 2017). Additionally, with H. zea potentially preventing bottlenecking in H. armigera populations, insecticidal control could be less effective, leading to excessive pesticide use which could further drive introgression and hybridization (Anderson et al., 2016; Valencia-Montoya et al., 2020). This effect could be strengthened in areas where mixed cropping and frequent rotation of maize and soybean occurs (Cordeiro et al., 2020). Interestingly, not much work has been done on production and detection of sex pheromones or hair-pencil compounds in H. armigera x H. zea hybrids, leaving a potential area for future research with additional urgency added by the emergence of hybrids in the wild. In addition to existing genetic studies, electrophysiological and behavioral data would be beneficial.
Much like H. virescens x H. subflexa, hybrids of H. armigera and H. assulta have not been found in the wild although the biased sex ratios of F1 hybrids (which produce all males) and backcrosses (which have a 4:1 ratio of males to females) could aid in controlling the parental species in a release program (Figure 3; Wang & Dong, 2001). However, prezygotic isolation barriers could make mating difficult in the wild after initial release (Zhao et al., 2005). Even if release was successful, with pheromone responses in H. armigera x H. assulta crosses being controlled by a dominant gene from the H. armigera genome, the hybrids would likely be more attracted to their blend, potentially introducing some H. assulta genes into H. armigera populations leading to increased fitness in wild H. armigera and potential evasion of pest management (Zhao et al., 2006; Xu et al., 2017). Hybrid males could also reach new geographic areas due to their increased flight capacity, destroying more crops (Zhao et al., 2005). Since H. assulta females from different areas can have different blend variations, these hybrid males with compatible variations in blend detection could mate with them, leading to diversification (Zhao et al., 2006). Alternatively, greater perception diversity in hybrid males could lead to random mating, thereby lowering diversification (Zhao et al., 2006). Much like H. zea x H. armigera hybrids, data is lacking on the behavioral responses to sex pheromone or hairpencil gland compounds in H. assulta x H. armigera hybrids, and genetic data is scarce (Guo et al., 2022). Genetic studies will be important, as introgression of H. assulta genes could change blend production and preference, as evidenced by the emergence of new subtypes of trichoid sensilla in H. armigera x H. assulta hybrids, and males able to detect new blends could have increased fitness (Zhao et al., 2006; Xu et al., 2017). In addition to potentially aiding with sterility-based control programs, these traits of hybrids could lead to changes in blend preference and production (Groot et al., 2010).
Hybridization studies have provided important insights into mechanisms of speciation in heliothines (as summarized in Table 4), particularly changes in sex pheromone responses driven by genetic inheritance. However, a limitation of these studies is that they do not explore changes that can occur within the lifetime of an organism, i.e., phenotypic plasticity. Significant gaps in our knowledge of how epigenetic changes or post-translational modifications could affect sex pheromone detection remain. Performing studies focusing on such plasticity could help determine why heliothine sex pheromone communication systems are not under stabilizing selection as expected (Löfstedt et al., 1989; Phelan, 1992; Löfstedt, 1993; Jurenka et al., 1994; Haynes, 1997; Phelan, 1997a; Phelan, 1997b; Baker, 2002; Roelofs et al., 2002).
Author contributions
Concept and draft of manuscript written by VI. VI collected and analyzed all relevant literature, and developed graphics and tables for data presentation. NH assisted in discussions for concept development and in editing of the manuscript. Both authors contributed to the article and approved the submitted version.
Funding
We thank the Atlantic Canada Opportunities Agency – Atlantic Innovation Fund (197853), Canada Foundation for Innovation (22087), Natural Sciences and Engineering Research Council of Canada (RGPIN-2017-04319) for funding support to NH, and an NSERC Post Graduate Award-Masters (NSERC-PGSM -(553339-2020) for support to VI.
Conflict of interest
The authors declare that the research was conducted in the absence of any commercial or financial relationships that could be construed as a potential conflict of interest.
Publisher’s note
All claims expressed in this article are solely those of the authors and do not necessarily represent those of their affiliated organizations, or those of the publisher, the editors and the reviewers. Any product that may be evaluated in this article, or claim that may be made by its manufacturer, is not guaranteed or endorsed by the publisher.
References
Anderson P. (2003). Pre-exposure modulates attraction to sex pheromone in a moth. Chem. Sens. 28 (4), 285–291. doi: 10.1093/chemse/28.4.285
Anderson C. J., Oakeshott J. G., Tay W. T., Gordon K. H. J., Zwick A., Walsh T. K. (2018). Hybridization and gene flow in the mega-pest lineage of moth, Helicoverpa. Proc. Natl. Acad. Sci. 115 (19), 5034–5039. doi: 10.1073/pnas.1718831115
Anderson C. J., Tay W. T., McGaughran A., Gordon K., Walsh T. K. (2016). Population structure and gene flow in the global pest, Helicoverpa armigera. Mol. Ecol. 25 (21), 5296–5311. doi: 10.1111/mec.13841
Andersson J., Borg-Karlson A.-K., Vongvanich N., Wiklund C. (2007). Male Sex pheromone release and female mate choice in a butterfly. J. Exp. Biol. 210 (6), 964–970. doi: 10.1242/jeb.02726
Bailey N. W., Zuk M. (2008). Acoustic experience shapes female mate choice in field crickets. Proc. R. Soc. B: Biol. Sci. 275 (1651), 2645–2650. doi: 10.1098/rspb.2008.0859
Baker T. C. (1989). Sex pheromone communication in the Lepidoptera: new research progress. Experientia 45 (3), 248–262. doi: 10.1007/BF01951811
Baker T. C. (2002). Mechanism for saltational shifts in pheromone communication systems. Proc. Natl. Acad. Sci. 99 (21), 13368–13370. doi: 10.1073/pnas.222539799
Baker T. C. (2008). Balanced olfactory antagonism as a concept for understanding evolutionary shifts in moth sex pheromone blends. J. Chem. Ecol. 34, 971–981. doi: 10.1007/s10886-008-9468-5
Baker T. C., Quero C., Ochieng’ S. A., Vickers N. J. (2006). Inheritance of olfactory preferences II. olfactory receptor neuron responses from Heliothis subflexa × heliothis virescens hybrid male moths. Brain Behav. Evol. 68 (2), 75–89. doi: 10.1159/000093375
Bashir T., Hodges R. J., Birkinshaw L. A., Hall D. R., Farman D. I. (2003). Phenotypic plasticity of Rhyzopertha dominica pheromone signaling: the effects of different hosts and presence of conspecific females on male produced aggregation pheromone. J. Chem. Ecol. 29 (4), 945–959. doi: 10.1023/A:1022940018168
Brazzel J. R., Lincoln C., Newsom L. D., Williams F. J., Roussel J. S., Barnes. G. (1953). Bollworm and tobacco budworm as cotton pests in Louisiana and Arkansas. Louisiana Tech. Bull., 482.
Behere G. T., Tay W., Russell D. A., Heckel D. G., Appleton B. R., Kranthi K. R., et al. (2007). Mitochondrial DNA analysis of field populations of Helicoverpa armigera (Lepidoptera: noctuidae) and of its relationship to H. zea. BMC Evolution. Biol. 7 (1), 117. doi: 10.1186/1471-2148-7-117
Cao S., Liu Y., Wang B., Wang G. (2021). A single point mutation causes one-way alteration of pheromone receptor function in two Heliothis species. iScience 24 (9), 102981. doi: 10.1016/j.isci.2021.102981
Cardé R. T., Baker T. C. (1984). “Sexual communication with pheromones,” in Chemical ecology of insects. Eds. Bell W. J., Cardé R. T. (US: Springer), 355–383. doi: 10.1007/978-1-4899-3368-3_13
Cho S., Mitchell A., Mitter C., Regier J., Matthews M., Robertson R. (2008). Molecular phylogenetics of heliothine moths (Lepidoptera: noctuidae: heliothinae), with comments on the evolution of host range and pest status. Systemat. Entomol. 33 (4), 581–594. doi: 10.1111/j.1365-3113.2008.00427.x
Choi M.-Y., Han K. S., Boo K. S., Jurenka R. A. (2002). Pheromone biosynthetic pathways in the moths Helicoverpa zea and Helicoverpa assulta. Insect Biochem. Mol. Biol. 32 (11), 1353–1359. doi: 10.1016/S0965-1748(02)00055-3
Cibrian-Tovar J., Mitchell E. R. (1991). Courtship behavior of Heliothis subflexa (Gn.) (Lepidoptera: noctuidae) and associated backcross insects obtained from hybridization with H. virescens (F.). Environ. Entomol. 20 (2), 419–426. doi: 10.1093/ee/20.2.419
Cordeiro E. M. G., Pantoja-Gomez L. M., de Paiva J. B., Nascimento A. R. B., Omoto C., Michel A. P., et al. (2020). Hybridization and introgression between Helicoverpa armigera and H. zea: an adaptational bridge. BMC Evolution. Biol. 20 (1), 61. doi: 10.1186/s12862-020-01621-8
Crispo E. (2008). Modifying effects of phenotypic plasticity on interactions among natural selection, adaptation and gene flow. J. Evolution. Biol. 21 (6), 1460–1469. doi: 10.1111/j.1420-9101.2008.01592.x
Degrugillier M. E. (1989). Virus-like particles in testes of Heliothis virescens, h. subflexa, and backcross males derived from the hybridization of H. virescens males × h. subflexa females. J. Invertebrate Pathol. 54 (3), 281–299. doi: 10.1016/0022-2011(89)90112-2
Degrugillier M. E., Newman S. M. (1993). Hereditary viruses of Heliothis? chromatin-associated virus-like particles in testes of six species of Heliothis and Helicoverpa, F1, and backcross males. J. Invertebrate Pathol. 61 (2), 147–155. doi: 10.1006/jipa.1993.1027
Goodpasture C., LaChance L. E., Richard R. D. (1980). Persistence of abnormal spermiogenesis in the backcross generations of interspecific hybrids between Heliothis virescens x H. subflexa. Ann. Entomol. Soc. America 73 (4), 397–403. doi: 10.1093/aesa/73.4.397
Gould F., Estock M., Hillier N. K., Powell B., Groot A. T., Ward C. M., et al. (2010). Sexual isolation of male moths explained by a single pheromone response QTL containing four receptor genes. Proc. Natl. Acad. Sci. 107 (19), 8660–8665. doi: 10.1073/pnas.0910945107
Groot A. T., Classen A., Staudacher H., Schal C., Heckel D. G. (2010). Phenotypic plasticity in sexual communication signal of a noctuid moth: phenotypic plasticity in a moth pheromone signal. J. Evolution. Biol. 23 (12), 2731–2738. doi: 10.1111/j.1420-9101.2010.02124.x
Groot A. T., Estock M. L., Horovitz J. L., Hamilton J., Santangelo R. G., Schal C., et al. (2009b). QTL analysis of sex pheromone blend differences between two closely related moths: insights into divergence in biosynthetic pathways. Insect Biochem. Mol. Biol. 39, 568–577. doi: 10.1016/j.ibmb.2009.05.002
Groot A. T., Horovitz J. L., Hamilton J., Santangelo R. G., Schal C., Gould F. (2006). Experimental evidence for interspecific directional selection on moth pheromone communication. Proc. Natl. Acad. Sci. 103 (15), 5858–5863. doi: 10.1073/pnas.0508609103
Groot A. T., Inglis O., Bowdridge S., Santangelo R. G., Blanco C., López J. D. Jr., et al. (2009a). Geographic and temporal variation in moth chemical communication. Evolution 63 (8), 1987–2003. doi: 10.1111/j.1558-5646.2009.00702.x
Guo P.-P., Li G.-C., Dong J.-F., Gong X.-L., Wang L., Yang K., et al. (2022). The genetic basis of gene expression divergence in antennae of two closely related moth species, Helicoverpa armigera and Helicoverpa assulta. Int. J. Mol. Sci. 23 (17), 10050. doi: 10.3390/ijms231710050
Hardwick D. F. (1965). The corn earworm complex. Memoirs Entomol. Soc. Canada 97 (40), 1–247. doi: 10.4039/entm9740fv
Haynes K. F. (1997). Genetics of pheromone communication in the cabbage looper moth, Trichoplusia ni. Insect Pheromone Res. (Boston, MA). doi: 10.1007/978-1-4615-6371-6_45
Hillier N. K., Vickers N. J. (2004). The role of heliothine hairpencil compounds in female Heliothis virescens (Lepidoptera: noctuidae) behavior and mate acceptance. Chem. Sens. 29 (6), 499–511. doi: 10.1093/chemse/bjh052
Hillier N. K., Baker T. C. (2016). “Pheromones of heliothine moths“. Pheromone Communication Moths: Evolution Behavior Application. (US), 301–333.
Joußen N., Agnolet S., Lorenz S., Schöne S. E., Ellinger R., Schneider B., et al. (2012). Resistance of Australian Helicoverpa armigera to fenvalerate is due to the chimeric P450 enzyme CYP337B3. Proc. Natl. Acad. Sci. 109 (38), 15206–15211. doi: 10.1073/pnas.1202047109
Jurenka R. A., Haynes K. F., Adlof R. O., Bengtsson M., Roelofs W. L. (1994). Sex pheromone component ratio in the cabbage looper moth altered by a mutation affecting the fatty acid chain-shortening reactions in the pheromone biosynthetic pathway. Insect Biochem. Mol. Biol. 24 (4), 373–381. doi: 10.1016/0965-1748(94)90030-2
Karpenko C. P., Proshold F. I. (1977). Fertility and mating performance of interspecific crosses between Heliothis virescens and H. subflexa backcrossed for three generations to H. subflexa. Ann. Entomol. Soc. America 70 (5), 737–740. doi: 10.1093/aesa/70.5.737
Kent C., Azanchi R., Smith B., Formosa A., Levine J. D. (2008). Social context influences chemical communication in D. melanogaster males. Curr. Biol. 18 (18), 1384–1389. doi: 10.1016/j.cub.2008.07.088
Klun J. A., Leonhardt B. A., Lopez J. D., Lachance L. E. (1982). Female Heliothis subflexa (Lepidoptera: noctuidae) sex pheromone: chemistry and congeneric comparisons. Environ. Entomol. 11 (5), 1084–1090. doi: 10.1093/ee/11.5.1084
Krupp J. J., Kent C., Billeter J.-C., Azanchi R., So A. K.-C., Schonfeld J. A., et al. (2008). Social experience modifies pheromone expression and mating behavior in male Drosophila melanogaster. Curr. Biol. 18 (18), 1373–1383. doi: 10.1016/j.cub.2008.07.089
Lachance L. E., Karpenko C. P. (1983). Hybrid sterility in Heliothis subflexa × h. virescens (Lepidoptera: noctuidae) crosses: expression after injection with antiviral agents, heat shocks, and rearing at extreme temperatures. Ann. Entomol. Soc. America 76 (1), 104–109. doi: 10.1093/aesa/76.1.104
Lachance L. E., Olstad G. (1988). Spermiogenesis of Heliothis virescens (Lepidoptera: noctuidae): an ultrastructure study of eupyrene sperm in sterile backcross males. Ann. Entomol. Soc. America 81 (2), 301–307. doi: 10.1093/aesa/81.2.301
Laster M. L. (1972). Interspecific hybridization of Heliothis virescens and H. subflexa. Environ. Entomol. 1 (6), 682–687. doi: 10.1093/ee/1.6.682
Laster M. L., Hardee D. D. (1995). Intermating compatibility between north American Helicoverpa zea and Heliothis armigera (Lepidoptera: noctuidae) from Russia. J. Economic Entomol. 88 (1), 77–80. doi: 10.1093/jee/88.1.77
Laster M. L., Goodpasture C. E., King E. G., Twine P. (1985). Results from crossing the bollworms Helicoverpa armigera × H. zea in search of backcross sterility. Proc. Beltwide Cotton Production Res. Conferences (Memphis, TN, USA), 146–149.
Laster M. L., Martin D. F., Parvin D. W. Jr. (1976). Potential for suppressing tobacco budworm (Lepidoptera: noctuidae) by genetic sterilization. Tech. Bull. Mississippi State Univ. Agric. Forestry Exp. Station 82.
Laster M. L., Martin D. F., Pair S. D. (1977). Mating incidence of male H. virescens, hybrid and backcross males from H. subflexa x H. virescens crosses. Ann. Entomol. Soc Am. 70, 293–295. doi: 10.1093/aesa/70.3.293
Laster M. L., Martin D. F., Pair S. D. (1978). The attraction of wild Heliothis virescens males to sex pheromone traps baited with H. virescens and backcross females. Environ. Entomol. 7 (1), 19–20. doi: 10.1093/ee/7.1.19
Laster M. L., Sheng C. F. (1995). Search for hybrid sterility for Helicoverpa zea in crosses between the north American H. zea and H. armigera (Lepidoptera: noctuidae) from China. J. Economic Entomol. 88 (5), 1288–1291. doi: 10.1093/jee/88.5.1288
Leite N. A., Correa A. S., Michel A. P., Alves-Pereira A., Pavinato V. A. C., Zucchi M. I., et al. (2017). Pan-American similarities in genetic structures of Helicoverpa armigera and Helicoverpa zea (Lepidoptera: noctuidae) with implications for hybridization. Environ. Entomol. 46 (4), 1024–1034. doi: 10.1093/ee/nvx088
Löfstedt C. (1993). Moth pheromone genetics and evolution. philosophical transactions of the royal society of London. Ser. B: Biol. Sci. 340 (1292), 167–177. doi: 10.1098/rstb.1993.0055
Löfstedt C., Hansson B. S., Roelofs W., Bengtsson B. O. (1989). No linkage between genes controlling female pheromone production and male pheromone response in the European corn borer, Ostrinia nubilalis hübner (Lepidoptera; pyralidae). Genetics 123 (3), 553–556. doi: 10.1093/genetics/123.3.553
Makela M. E., Huettel M. D. (1979). Model for genetic control of Heliothis virescens. Theor. Appl. Genet. 54 (5), 225–233. doi: 10.1007/BF00267712
Members of the Complex Trait Consortium (2003). The nature and identification of quantitative trait loci: a community’s view. Nat. Rev. Genet. 4 (11), 911–916. doi: 10.1038/nrg1206
Miller S. G., Huettel M. D., Davis M.-T. B., Weber E. H., Weber L. A. (1986). Male Sterility in Heliothis virescens x H. subflexa backcross hybrids: evidence for abnormal mitochondrial transcripts in testes. Mol. Gen. Genet. MGG 203 (3), 451–461. doi: 10.1007/BF00422070
Pair S. D., Laster M. L., Martin D. F. (1977). Hybrid sterility of the tobacco budworm: effects of alternate sterile and normal matings on fecundity and fertility. Ann. Entomol. Soc. Am 70 (6), 952–954. doi: 10.1093/aesa/70.6.952
Parvin D. W. Jr., Laster M. L., Martin D. F. (1976). A computer program for simulating the theoretical suppression of the tobacco budworm by genetic sterilization. AgEcon Tech. Publications 26.
Pearce S. L., Clarke D. F., East P. D., Elfekih S., Gordon K. H. J., Jermiin L. S., et al. (2017). Genomic innovations, transcriptional plasticity and gene loss underlying the evolution and divergence of two highly polyphagous and invasive Helicoverpa pest species. BMC Biol. 15 (1), 63. doi: 10.1186/s12915-017-0402-6
Petfield D., Chenoweth S. F., Rundle H. D., Blows M. W. (2005). Genetic variance in female condition predicts indirect genetic variance in male sexual display traits. Proc. Natl. Acad. Sci. 102 (17), 6045–6050. doi: 10.1073/pnas.0409378102
Phelan P. L. (1992). “Evolution of sex pheromones and the role of asymmetric tracking,” in Insect chemical ecology: an evolutionary approach. Eds. Roitberg B. D., Isman M. B. (New York, NY: Chapman and Hall), 265–314.
Phelan P. L. (1997a). “Genetics and phylogenetics in the evolution of sex pheromones,” in Insect pheromone research. Eds. Cardé R. T., Minks A. K. (US: Springer), 563–579. doi: 10.1007/978-1-4615-6371-6_48
Phelan P. L. (1997b). “Evolution of mate–signaling in moths: phylogenetic considerations and predictions from the asymmetric tracking hypothesis,” in The evolution of mating systems in insects and arachnids, 1st ed. Eds. Choe J. C., Crespi B. J. (UK: Cambridge University Press), 240–256. doi: 10.1017/CBO9780511721946.015
Pogue M. G. (2004). A new synonym of Helicoverpa zea (Boddie) and differentiation of adult males of H. zea and H. armigera (Hübner) (Lepidoptera: noctuidae: heliothinae). Ann. Entomol. Soc. America 97 (6), 1222–1226. doi: 10.1603/0013-8746(2004)097[1222:ANSOHZ]2.0.CO;2
Proshold F. I., Lachance L. E. (1974). Analysis of sterility in hybrids from interspecific crosses between Heliothis virescens and H. subflexa. Ann. Entomol. Soc. America 67 (3), 445–449. doi: 10.1093/aesa/67.3.445
Proshold F. I., Lachance L. E., Richard R. D. (1975). Sperm production and transfer by Heliothis virescens, H. subflexa, and the sterile hybrid males. Ann. Entomol. Soc. America 68 (1), 31–34. doi: 10.1093/aesa/68.1.31
Richard R. D., Lachance L. E., Proshold F. I. (1975). An ultrastructural study of sperm in sterile hybrids from crosses of Heliothis virescens and Heliothis subflexa. Ann. Entomol. Soc. America 68 (1), 35–39. doi: 10.1093/aesa/68.1.35
Rios D. A., Specht A., Roque-Specht V. F., Sosa-Gómez D. R., Fochezato J., Malaquias J. V., et al. (2021). Helicoverpa armigera and Helicoverpa zea hybridization: constraints, heterosis, and implications for pest management. Pest Manage. Sci. 78 (3), 955–964. doi: 10.1002/ps.6705
Roelofs W. L., Liu W., Hao G., Jiao H., Rooney A. P., Linn C. E. (2002). Evolution of moth sex pheromones via ancestral genes. Proc. Natl. Acad. Sci. 99 (21), 13621–13626. doi: 10.1073/pnas.152445399
Teal P. E. A., Oostendorp A. (1993). Interspecific hybridization between Heliothis virescens and H. subflexa (Lepidoptera: noctuidae) affects the presence and structure of hair-pencil glands of males. Ann. Entomol. Soc. America 86 (3), 322–326. doi: 10.1093/aesa/86.3.322
Teal P. E. A., Oostendorp A. (1995). Production of pheromone by hairpencil glands of males obtained from interspecific hybridization between Heliothis virescens and H. subflexa (Lepidoptera: noctuidae). J. Chem. Ecol. 21 (1), 59–67. doi: 10.1007/BF02033662
Teal P. E. A., Tumlinson J. H. (1997). “Effects of interspecific hybridization between heliothis virescens and heliothis subflexa on the sex pheromone communication system,” in Insect pheromone research. Eds. Cardé R. T., Minks A. K. (US: Springer), 535–547. doi: 10.1007/978-1-4615-6371-6_46
Tingle F. C., Mitchell E. R., Baumhover A. H. (1978). Sex pheromone specificity in Heliothis. J. Chem. Ecol. 4 (4), 471–479. doi: 10.1007/BF00989503
Unbehend M., Kozak G. M., Koutroumpa F., Coates B. S., Dekker T., Groot A. T., et al. (2021). bric à brac controls sex pheromone choice by male European corn borer moths. Nat. Commun. 12, 2818. doi: 10.1038/s41467-021-23026-x
Valencia-Montoya W. A., Elfekih S., North H. L., Meier J. I., Warren I. A., Tay W. T., et al. (2020). Adaptive introgression across semipermeable species boundaries between local Helicoverpa zea and invasive Helicoverpa armigera moths. Mol. Biol. Evol. 37 (9), 2568–2583. doi: 10.1093/molbev/msaa108
Vickers N. J., Christensen T. A., Mustaparta H., Baker T. C. (1991). Chemical communication in heliothine moths III. flight behavior of male helicoverpa zea and Heliothis virescens in response to varying ratios of intra- and interspecific sex pheromone components. J. Comp. Physiol. A 169, 275–280. doi: 10.1007/BF00206991
Vickers N. J. (2002). Defining a synthetic pheromone blend attractive to male Heliothis subflexa under wind tunnel conditions. J. Chem. Ecol. 28 (6), 1255–1267. doi: 10.1023/A:1016242019571
Vickers N. J. (2006a). Inheritance of olfactory preferences i. pheromone-mediated behavioral responses of Heliothis subflexa × Heliothis virescens hybrid male moths. Brain Behav. Evol. 68 (2), 63–74. doi: 10.1159/000093374
Vickers N. J. (2006b). Inheritance of olfactory preferences III. processing of pheromonal signals in the antennal lobe of Heliothis subflexa × heliothis virescens hybrid male moths. Brain Behav. Evol. 68 (2), 90–108. doi: 10.1159/000093376
Wang N. C., Li Z. H. (1984). Studies on the biology of cotton bollworm (Heliothis armigera Hübner) and tobacco budworm (H. assulta Quenee). J. Shandong Agric. Univ. Z1, 13–24.
Wang C., Dong J. (2001). Interspecific hybridization of Helicoverpa armigera and H. assulta (Lepidoptera: noctuidae). Chin. Sci. Bull. 46 (6), 489–491. doi: 10.1007/BF03187264
Wang Q., Jia Y., Wang Y., Jiang Z., Xiang Z., Zhang Z., et al. (2019). Evolution of cis- and trans-regulatory divergence in the chicken genome between two contrasting breeds analyzed using three tissue types at one-day-old. BMC Genomics 20, 933. doi: 10.1186/s12864-019-6342-5
Wang H.-L., Zhao C.-H., Wang C.-Z. (2005). Comparative study of sex pheromone composition and biosynthesis in Helicoverpa armigera, h. assulta and their hybrid. Insect Biochem. Mol. Biol. 35 (6), 575–583. doi: 10.1016/j.ibmb.2005.01.018
Xu M., Dong J.-F., Wu H., Zhao X.-C., Huang L.-Q., Wang C.-Z. (2017). The inheritance of the pheromone sensory system in two Helicoverpa species: dominance of H. armigera and possible introgression from H. assulta. Front. Cell. Neurosci. 10. doi: 10.3389/fncel.2016.00302
Yang K., Wang C. (2020). Review of pheromone receptors in heliothine species: expression, function, and evolution. Entomol. Experimentalis Applicata 169 (2), 156–171. doi: 10.1111/eea.12982
Zhao X.-C., Dong J.-F., Tang Q.-B., Yan Y.-H., Gelbic I., Van Loon J. J. A., et al. (2005). Hybridization between Helicoverpa armigera and Helicoverpa assulta (Lepidoptera: noctuidae): development and morphological characterization of F1 hybrids. Bull. Entomol. Res. 95 (5), 409–416. doi: 10.1079/BER2005372
Keywords: heliothine moths, Heliothinae, species hybridization, hybrid sterility, pheromone communication, prezygotic barriers, hybrid vigor, backcross hybrids
Citation: Ivey V and Hillier NK (2023) Hybridization in heliothine moths: impacts on reproduction, pheromone communication, and pest management. Front. Ecol. Evol. 11:1208079. doi: 10.3389/fevo.2023.1208079
Received: 18 April 2023; Accepted: 13 June 2023;
Published: 06 July 2023.
Edited by:
Juergen Gross, Julius Kühn-Institut, GermanyReviewed by:
Xin-Cheng Zhao, Henan Agricultural University, ChinaAstrid T Groot, University of Amsterdam, Netherlands
Copyright © 2023 Ivey and Hillier. This is an open-access article distributed under the terms of the Creative Commons Attribution License (CC BY). The use, distribution or reproduction in other forums is permitted, provided the original author(s) and the copyright owner(s) are credited and that the original publication in this journal is cited, in accordance with accepted academic practice. No use, distribution or reproduction is permitted which does not comply with these terms.
*Correspondence: Victoria Ivey, victoriaivey@acadiau.ca; Neil Kirk Hillier, kirk.hillier@acadiau.ca