Review of online measurement techniques for chemical composition of atmospheric clusters and sub-20 nm particles
- 1Zhejiang Provincial Key Laboratory of Organic Pollution Process and Control, College of Environmental and Resource Sciences, Zhejiang University, Hangzhou, China
- 2ZJU-Hangzhou Global Scientific and Technological Innovation Center, Hangzhou, China
- 3Key Laboratory of Environment Remediation and Ecological Health, Ministry of Education, Zhejiang University, Hangzhou, China
The chemical composition of aerosol particles is crucial to understanding their formation and evolution in the atmosphere. However, very limited information is available for the chemical composition of ultrafine particles, particularly for nanoclusters and newly formed particles, due to the lack of valid analytical methods. This work reviews the online measurement techniques for characterizing the chemical composition of atmospheric clusters and sub-20 nm particles. The commonly used instrumentations are divided into two categories: direct techniques based on mass spectrometry and indirect measurement techniques mainly relying on the physical properties (e.g., hygroscopicity and volatility). The advantages of these techniques are compared and their limitations in the lab and field application are summarized. The combination of direct and indirect techniques is discussed, and this may provide more comprehensive understanding of chemical information of atmospheric clusters and particles. We propose that the newly developing instrumentations are needed to improve the collection efficiency for direct techniques, or the chemical resolution for indirect techniques. Future development should focus on obtaining simultaneous measurements of particle physical and chemical properties, which can be helpful in improving the accuracy of modeling and the understanding of particle formation and evolution.
1 Introduction
Atmospheric aerosols play a significant role in altering the atmospheric radiative balance by directly scattering the sunlight and indirectly participating in the cloud formation process (IPCC, 2013). New particle formation (NPF) is a major source of particle number concentration and contributes to around half of the cloud condensation nuclei (CCN) population (Merikanto et al., 2009; Merikanto et al., 2010; Wang et al., 2013; Gordon et al., 2017). Atmospheric clusters (molecular aggregates from nucleation with typical diameter around 1.5 nm) and nucleation mode particles are important for the understanding of atmospheric NPF events (Table 1; Kulmala et al., 2014; Kulmala et al., 2017; Wang et al., 2017; Lee et al., 2019). The sub-20 nm particles (nucleation mode) dominate the particle number concentration in most atmospheric conditions (Seinfeld and Pandis, 2016; Wang D. et al., 2020; Kontkanen et al., 2020). Besides, the primary emission sources, such as combustion processes, e.g., vehicular exhaust (Rönkkö et al., 2017; Rönkkö and Timonen, 2019; Cai et al., 2020; Guo et al., 2020), residential solid fuel combustion (Wang D. et al., 2020), also produce clusters and sub-20 nm particles. Chemical composition of atmospheric clusters and newly formed particles determine the physicochemical properties and behaviors of larger particles (Dusek et al., 2010). Therefore, it is crucial to understand the chemical features of aerosol particles, especially for the atmospheric clusters and sub-20 nm particles.
A number of techniques have been developed to analyze the chemical composition of clusters and nanoparticles in the lab and field measurements. Chen et al. (2020) and Peng et al. (2022) reviewed the analytical techniques which are mainly used to investigate new particle formation and growth mechanisms. Traditional techniques collect size-resolved samples by cascade impactors (e.g., NanoMOUDI with a lower size cutoff of 10 nm), which require an integration time of 3 h or more before the chemical identification by off-line analytical techniques (Makela et al., 2001; Geller et al., 2002; Tao et al., 2016). To obtain high time/detection resolution and avoid potential sample contamination, online methods based on the mass spectrometer (MS) were developed to detect gas precursors, molecular clusters, and nanoparticles. Although the direct online techniques could obtain chemical information in real-time, the measurements would be challenging in the diameter range of 3–10 nm. It is difficult to maintain sufficient ion concentration for the detection in MS. On one hand, particles in this diameter range have lower ionization efficiency compared with sub-3 nm particles/clusters. On the other hand, 3–10 nm particles are hard to separate through pretreatment part (e.g., aerodynamic lens) and the diameter classification would decrease the mass concentration. As summarized by Smith et al. (2021), significant challenges still exist for direct techniques in gapping the chemical composition measurement of clusters and nanoparticles.
The aerosol composition can influence some physical characteristics; hence it is reasonable to derive chemical information indirectly by measuring the physical properties (e.g., hygroscopicity and volatility). For the 3–10 nm particles, the number concentration is easily retrieved by the highly developed instruments, e.g., Condensation Particle Counter (CPC). It is usually combined with Differential Mobility Analyzer (DMA) to measure the particle number size distribution. The fundamental principle of CPC enables the linkage to physical properties, based on which we can infer the chemical composition of atmospheric aerosol particles. Compared with the direct measurement techniques, the indirect techniques might not be suitable for species identification and quantification, but could still establish clear constraints on the chemical categories that may or may not be present (O'Dowd et al., 2002; Sakurai et al., 2005; Kim et al., 2016). Furthermore, the indirect techniques can cover the diameter range of 3–10 nm and provide size-resolved information because of the high number concentration. Based on the connection between physical properties and chemical composition, the bulk information obtained by indirect techniques are the dominating properties of the measured aerosol. It would be helpful to obtain both detailed chemical compositions and comparable physical properties by the combination of direct and indirect techniques.
Several recent reviews (Chen et al., 2020; Li et al., 2021a; Smith et al., 2021; Peng et al., 2022) have included the direct techniques as well as modelling method in detail for nucleation research. In this review, online measurement techniques for detecting the chemical composition of atmospheric clusters and sub-20 nm particles were divided into two categories: direct techniques based on MS and indirect techniques based on CPC and DMA. The basic principles, application, advantages and limitations of individual technique were discussed. We focused more on the indirect techniques which have not been discussed in detail in current reviews and compared the advantages and disadvantages of direct and indirect techniques. Finally, we proposed the outlook on the development of measurement techniques for the chemical composition of atmospheric clusters and sub-20 nm particles. Figure 1 summarized the characteristics of the techniques being discussed in the following sections.
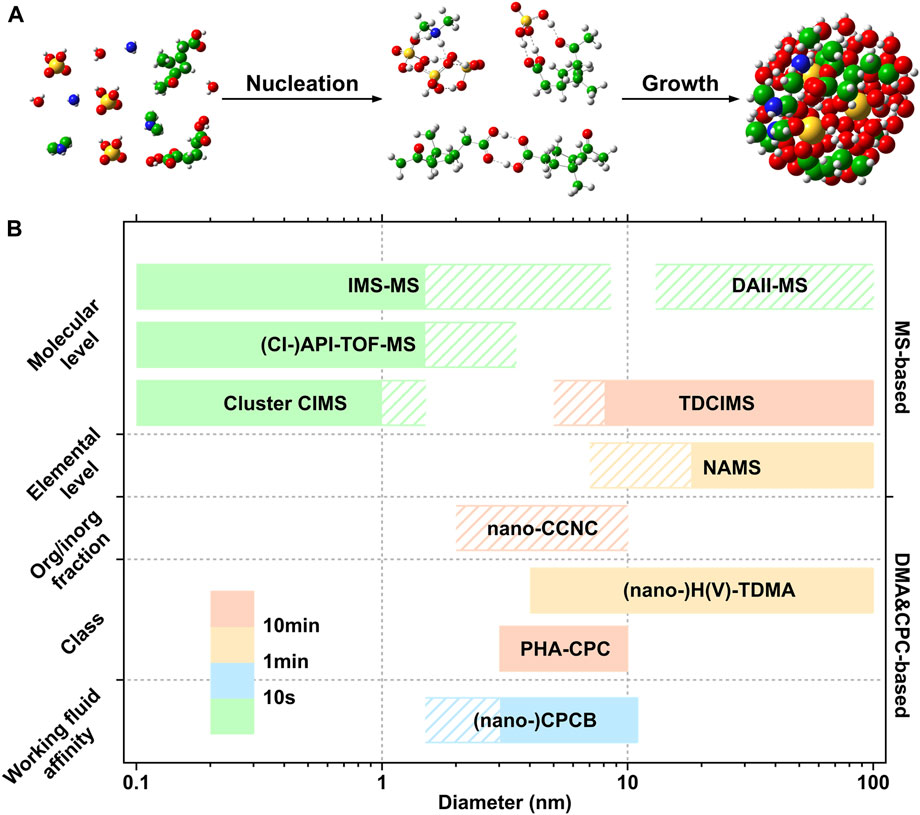
FIGURE 1. (A) Schematic of nanoparticle formation and growth. (B) Overview of the techniques that can retrieve chemical information of nanoclusters and ultrafine particles (The techniques that have not been applied for particles in the sub-20 nm diameter range are not included). The fill colors for each technique are used to show the time resolution. The shaded area by gridlines indicates the diameter range in which the techniques have not been applied in field measurement. Technique abbreviations are listed in Appendix 1.
2 Direct techniques based on mass spectrometry
Direct techniques for chemical composition measurement of nano cluster/particles mainly referred to MS-based approaches. As shown in Figure 1, current direct techniques cannot cover the whole size range from molecular cluster to sub-20 nm particles. One class of direct approaches could characterize molecular clusters/particles up to ∼3 nm (e.g., cluster-CIMS, (CI-)API-TOF-MS, IMS-MS), while another (e.g., TDCIMS, NAMS, DAII-MS), could only detect particles down to ∼10 nm which was limited by the difficulties in providing sufficient mass concentration for chemical analysis. In the following, the main direct techniques were reviewed based on their detectable size ranges.
2.1 Clusters
2.1.1 API-TOF
API-TOF consisted of a time-of-flight mass spectrometer (TOF) and an atmospheric pressure interface (API) (Junninen et al., 2010). It was capable of measuring the naturally charged ion clusters directly without extra ionization, which could protect the clusters from fragmentation during ionization process (Ortega et al., 2014). Compared with CI-API-TOF, larger clusters were measured by API-TOF in the urban atmosphere due to less fragmentation (Yin et al., 2021).
API-TOF was applied to measure the molecular composition of cluster ions involving ammonia (NH3) (Kirkby et al., 2011), amine (Almeida et al., 2013), and biogenic oxidation organics (Riccobono et al., 2014) in sulfuric acid-water (H2SO4-H2O) system. The results suggested that involving the third species in ternary nucleation, such as amine and biogenic oxidation organics, could explain the discrepancy between the theoretical and observed nucleation rates in the atmosphere. Highly oxygenated molecules (HOMs) cluster ions generated from α-pinene ozonolysis in the absence of H2SO4 were also investigated by API-TOF, which supplemented that ion-induced nucleation of pure organic particles constitutes a potentially widespread source of nanoparticles in terrestrial environments with low H2SO4 concentration (Kirkby et al., 2016). API-TOF had also been applied for field measuring of naturally charged ions. In forestry areas, HSO4-containing cluster ions were dominant in the negative spectrum during daytime, whereas highly-oxidized organic ions were more important in the negative spectrum during night (Ehn et al., 2010; Ehn et al., 2012; Bianchi et al., 2017). In megacities, H2SO4-amine was able to explain the observed nucleation (Yao et al., 2018), but the H2SO4-base cluster ions suggested that there were insufficient amines to fully stabilize the grown H2SO4 clusters and other bases such as NH3 would play an important role (Yin et al., 2021). The maximal mass for ambient clusters detected by API-TOF was 1,400 Da (Bianchi et al., 2017) and this range could be extended to 20 kDa in laboratory studies (Renzler et al., 2017), corresponding to the spherical equivalent diameters of ∼1.5 and 3.5 nm respectively. Currently, the field applications of API-TOF mainly focus on forest sites, and the measurements in urban area need to be further conducted.
2.1.2 Cluster-CIMS/CI-API-TOF
Cluster Chemical Ionization Mass Spectrometer (Cluster-CIMS) was developed for detecting neutral molecular clusters in the atmosphere (Zhao et al., 2010). Neutral clusters were charged by the nitrate ions through proton transfer or adduct reactions. By changing the chemical ionization reaction time, cluster ions formed from direct ionization and successive ion-molecule clustering processes could be distinguished.
Cluster-CIMS was firstly applied to measure H2SO4-containing clusters. Field measurements showed it was capable of detecting clusters containing up to four H2SO4, with a size up to ∼1 nm (Jiang et al., 2011; Zhao et al., 2011). The detected size could be extended to ∼1.5 nm during laboratory calibrations (Zhao et al., 2010). Cluster-CIMS was also deployed to investigate α-pinene ozonolysis products, and the results indicated these oxidation products could be classified into two categories, responsible for particle nucleation and initial growth, respectively (Zhao et al., 2013). However, the reported Cluster-CIMS adapted a quadrupole mass analyzer, which limited Cluster-CIMS at the unit mass resolution, hindering it for high resolution identification.
In order to detect neutral compounds, a designed CI source was integrated with API-TOF, transforming API-TOF to CI-API-TOF (Jokinen et al., 2012). In the CI source, reagent ions were generated by exposing to either a radiation source (Jokinen et al., 2012), an X-ray source (Massoli et al., 2018) or a corona discharge (Kürten et al., 2011), and then the neutral compounds were charged via proton transfer or addition reactions with resulted reagent ions in the reactor with different pressure.
In the laboratory experiments, CI-API-TOF was deployed to illustrate the molecular composition of neutral clusters such as (H2SO4)n (Jokinen et al., 2012), H2SO4-amine (Kürten et al., 2014; Lehtipalo et al., 2016), HNO3-NH3 (Wang M. et al., 2020) and iodine oxoacids (He et al., 2021), revealing the fundamental processes involved in nucleation and growth under atmospherically relevant conditions. CI-API-TOF has also been deployed in field measurements to detect neutral clusters involving H2SO4 and amine (Yao et al., 2018), dicarboxylic acids (Fang et al., 2020) and iodine oxoacids (Sipilä et al., 2016), which has been proved to be crucial for nucleation at megacities, rural and coastal area, respectively. The maximal mass for ambient clusters detected by CI-API-TOF was 2,200 Da (Sipilä et al., 2016), with spherical equivalent diameter being ∼1.2 nm. However, the conformation of neutral clusters would be changed during the chemical ionization, which further increases the difficulty for molecular identification of clusters.
2.1.3 IMS-MS
Ion Mobility Spectrometer coupled to a Mass Spectrometer (IMS-MS) could provide the simultaneous measurement of mobility and mass to charge ratio of cluster ions (May and McLean, 2015). Because the mobility was associated with the cluster ions structures, IMS-MS was thus applied to structural characterization and conformational dynamics for cluster ions (Lanucara et al., 2014). Drift time ion mobility spectrometry (DTIMS) (McDaniel et al., 1962) and field-asymmetric ion mobility spectrometry (FAIMS) (Purves and Guevremont, 1999) were the mainstream techniques applied in atmospheric field.
DTIMS adopted a drift tube to separate cluster ions. It was applied to demonstrate the chemical and structural characteristics of organic species in the gas and particulate phases and separate isomers produced from different chemical pathways (Krechmer et al., 2016; Zhang et al., 2017; Zhang et al., 2019). The ambient results of IMS-MS showed good consistency with laboratory spectra obtained from single-component carboxylic acids and multicomponent mixtures of isoprene and monoterpene oxidation products with the maximal mobility diameter of these ambient compounds reaching at ∼1.5 nm (Krechmer et al., 2016).
The ion mobility measurement device adopted by FAIMS was constructed of two electrodes, between which an electric field and drift gas were perpendicularly established to separate the ions (Purves and Guevremont, 1999). DMA coupled to a mass spectrometer (DMA-MS) is a typical prototype of FAIMS (Rus et al., 2010). Charged clusters can be produced by electrospray (Hogan and Fernandez de la Mora, 2009), secondary electrospray ionization (Amo-Gonzalez and Fernandez de la Mora, 2017) or exposing to a radiation source (Viitanen et al., 2010). The resulted cluster ions are drawn electrostatically into a DMA with high resolution, then cluster ions with a specific mobility are selected and detected by the coupling MS.
Currently, DMA-MS was applied to mobility and mass analysis of nanoparticles (Hogan and Fernandez de la Mora, 2009, 2010; Hogan et al., 2011; Criado-Hidalgo et al., 2013) and established their mobility-volume relationship based on measurements (Larriba et al., 2011). Atmospheric relevant clusters such as iodide salt (Oberreit et al., 2014; Oberreit et al., 2015), dimethylamine-sulfuric acid (Ouyang et al., 2015; Thomas et al., 2016) and hybrid iodine pentoxide-iodic acid (Ahonen et al., 2019) were investigated by DMA-MS and their physicochemical properties such as density, thermostability, extent of hydration and organic vapors uptake ability were illustrated. Compared with previous techniques such as API-TOF/CI-API-TOF, DMA-MS could obtain the mobility and chemical composition at the same time. Mobility can be further converted to collision cross sections (CCS) via the Mason-Schamp equation (Mason and Schamp, 1958). CCS is closely related to ion size and structure. Combined with quantum chemical calculations and ion mobility modeling, the exact structure of identified cluster ions could be determined (Bianco et al., 2022). Such methods could be utilized to bridge the gap between cluster size, chemical composition and structure. DMA-MS is a typical newly developed instrument that can perform simultaneous measurement of physical and chemical properties. It is promising to study the physicochemical properties of atmospheric clusters and the formation mechanism of particle nucleation and initial growth. However, in order to obtain the high resolution, a supercritical laminar sheath flow is required which complexes the sheath flow circulation system. Therefore, the application of DMA-MS still focused on laboratory experiments with the maximal mobility diameter of detected cluster ions reaching at ∼8.5 nm (Smith et al., 2021). A more robust system is necessary for the field application.
For the aforementioned cluster measurement techniques, the maximal size of clusters detected in field is smaller than that in laboratory experiments. This is primarily resulted from the insufficient number of clusters. On one hand, the number of ambient clusters would decrease as the size increasing. On the other hand, the diffusion loss and fragmentation in sampling and detection system would further make ambient clusters undetectable. Reducing the sampling loss and tuning MS properly may improve size upper limit. The direct techniques that currently have been used in nucleation studies are summarized in Table 2.
2.2 Sub-20 nm particles
MS-based techniques, such as Aerosol Mass Spectrometry (AMS) (Jayne et al., 2000), have also been widely used for atmospheric nanoparticles. However, AMS was not able to measure aerosol particles smaller than 50 nm due to the low transmission efficiency in the aerodynamic lens. For the sub-20 nm particles, the concentration enrichment system was thought to be necessary for the pretreatment of aerosol samples required for MS analyses. In the following, the techniques for measuring the chemical composition of sub-20 nm particles were reviewed.
2.2.1 TDCIMS
Thermal Desorption Chemical Ionization Mass Spectrometer (TDCIMS) can measure the size-resolved chemical composition of particles (Voisin et al., 2003; Smith et al., 2004; Perraud et al., 2020). The particles were pre-charged by unipolar particle charger and the electrical mobility-based size separation was achieved by DMA. Normally, several DMAs were used in parallel to multiply the sampling flow rates and thus to increase the sample amount. Additionally, the high voltage biased filament was used to accumulate charged particles by electrostatic deposition. It usually takes 5–10 min for sample collection in lab experiments, while a longer time (10–30 min) was needed for ambient measurements. After sufficient particles (usually 1–10 pg) were accumulated, the sample was transferred into a desorption region and a CIMS was used to conduct the chemical analysis. Up to now, the smallest detectable diameter of TDCIMS was extended down to 5 nm with a novel design of inlet (Perraud et al., 2020), and this diameter was limited by the small mass (0.16 ng) and the rapid decreasing charging efficiency with decreased diameter, e.g., smaller than 10% for 5 nm particles (Perraud et al., 2020; Li et al., 2021b).
Field measurements conducted in Boulder, United States demonstrated that sub-20 nm particles were composed primarily of ammonium sulfate (Smith et al., 2004). Smith et al. (2005) firstly applied TDCIMS to study the size-resolved atmospheric nanoparticles, then this technique was deployed in various atmospheric environments (e.g., forest site, urban site) to obtain size-resolved chemical information (Smith et al., 2010). These field measurements showed that ammonium salts contribute significantly to nanoparticle growth and must be accounted for in models to accurately predict the impact of new particle formation on climate. The results in the boreal forest indicated that alkylamines were likely important drivers of NPF (Lawler et al., 2018), whereas the sea-salt component was observed in a remote coastal site (Lawler et al., 2014). The results in urban Beijing indicated the ultrafine particles (Li et al., 2021b) and the newly formed 8–40 nm particles (Li et al., 2022) are mainly composed of organics, with more nitrogen-containing and sulfur-containing compounds than that at the forest and marine sites.
The ion drift (ID) tube was attempted to couple with TD-CIMS, aiming at controlling ion-molecule reaction time (Zhang et al., 2009). The results suggested that the initial growth most likely occurs by condensation of sulfuric acid and water. But this method has not been reported to measure size-resolved chemical composition. Thermal Desorption Differential Mobility Analyzer (TD-DMA) is a similar technique as TDCIMS. Particles are selected by DMA and collected on a filament by electrostatic precipitation (Wagner et al., 2018). It was used to analyze the chemical composition of size-resolved particles in the diameter range of 10–30 nm. Kreisberg et al. (2018) developed a NanoCharger consisting of a bipolar ion source and water-based condensation-evaporation system, which provided ∼40-fold enhancement for the total charged particles with a possibility of additional ion peaks.
2.2.2 NAMS
Wang et al. (2006) described a nano aerosol mass spectrometer (NAMS) for real-time characterization of individual airborne nanoparticles. The device was composed of an aerodynamic inlet, quadrupole ion guide, quadrupole ion trap, and time-of-flight mass analyzer. Relative to classical AMS, NAMS employed new design of aerodynamic inlet (lens aperture, capillary and critical orifice), which focus more on particles below 50 nm. The nanoparticles could be transported to the ionization region more efficiently and captured more stably. Then they could be ionized by the laser pulse completely and more ions could be generated for a certain amount of particulate matter (Johnston et al., 2006). Thus, NAMS can be used to measure the chemical composition of particles in smaller diameter range compared to AMS. However, the size limitation was restricted to 7 nm in the lab and 18 nm in the field due to the insufficient mass concentration be sampled, which is related with low transmission efficiency in the aerodynamic lens (Wang and Johnston, 2006; Bzdek et al., 2012; Bzdek et al., 2014). The biggest drawback of NAMS is that it can only obtain elemental data by complete fragmentation. The molecular composition, especially organic species, is difficult to determine.
NAMS was deployed to measure the composition of nanoparticles during NPF events in both coastal and urban sites (Bzdek et al., 2012). The results showed that sulfate constituted a substantial fraction of total particle mass. An optimized field adjusting lens (FAL) design had been incorporated into the NAMS and increased the particle analysis rate by over an order of magnitude (Ross Pennington and Johnston, 2012). Except the exploration and application of single NAMS, the combination with other meaningful instruments may provide more complete information of chemical composition. Bzdek et al. (2014) provided the collocated molecular composition measurement in rural/coastal environments by deriving molecular species from TDCIMS measurements and E-AIM model. The combination of elemental and molecular measurements permits determination of the key molecular components contributing to nanoparticle growth, the quantitative contribution of each molecular component to growth, and more precise determination of the elemental ratios necessary to describe carbonaceous matter (Bzdek et al., 2014).
2.2.3 DAII-MS
TD-CIMS and NAMS both have the defect that the smallest detectable size depends on the sample mass. Furthermore, the long collection time of sub-20 nm particles (7–20 nm in field measurements) hinders the high time resolution measurement. Droplet Assisted Inlet Ionization Mass Spectrometry (DAII-MS) is a potential online method for monitoring sub-20 nm aerosols without sample pretreatment. The mechanism of DAII is to first make the nano-aerosol hygroscopically grow to form droplets (3–5 μm) in the condensation growth tube (CGC). These droplets break down and the solvent evaporates to form ions through rapid heating after entering DAII. The production ions are then detected by the coupling MS (Apsokardu et al., 2020).
DAII-MS can be combined with aerosol dynamics concentrator to improve the number concentration of collected samples, which increased the outlet number concentration by more than a factor of 7 and the corresponding signal intensity of MS (Saarikoski et al., 2019). An increase in DAII-MS temperature can enhance the ionization efficiency and further lower the detection limit (Horan et al., 2017). In the laboratory, Polypropylene glycol (PPG) aerosols down to 13 nm and cortisone aerosols (29–76 nm) were successfully measured (Horan et al., 2017; Kerecman et al., 2021). Studies on secondary organic aerosols (SOAs) produced from α-pinene ozonolysis indicated that its chemical composition was affected by particle sizes (Kerecman et al., 2021). Currently, DAII-MS has only been tested in the laboratory. The experimental results indicated that the signal intensity ratio of DAII-MS scales more closely with the number concentration compared to mass concentration (Kerecman et al., 2021), which was in favor of small particle detection. This technology has shown potential for the component analysis of particles down to ∼10 nm.
3 Indirect techniques based on physical properties
Although mass spectrometry is a useful tool to detect the chemical composition, it remains a challenge to perform direct measurement for sub-10 nm particles due to insufficient sample amount for subsequent chemical analysis. On the other hand, physical properties are closely associated with chemistry, which provides an alternative way to retrieve the aerosol chemical composition. As shown in Table 3, the relationship between the physical properties and the chemical composition are summarized.
CPC is a highly developed instrument to measure the particle number concentration above a certain diameter. The detailed construction of CPC and the underlying thermodynamic principles can be found elsewhere (Kangasluoma and Attoui, 2019, and references therein). Figure 2A illustrates the evolution of target nanoparticles inside the CPC. The key parameter representing the performance of a given CPC is the counting efficiency curve, or just simply referred as cut-off diameter (D50), which is defined as the particle diameter at which the counting efficiency is 50%. The ideal counting efficiency curve should be a step function, while the particle diffusion loss and the non-uniform S distribution result in a size-dependent activation fraction. It should be noted that the counting efficiency curve is expected to be insensitive to chemical composition for accurate particle number counting in nucleation research, whereas the discrepancy between different compounds is a useful indicator for inferring chemical features. For particles of different chemical compositions, they may perform differently in the same CPC as shown in Figure 2B. Furthermore, the hygroscopic particles have smaller D50 than hydrophobic particles in water-based CPC, and the opposite phenomenon may present in butanol-based CPC.
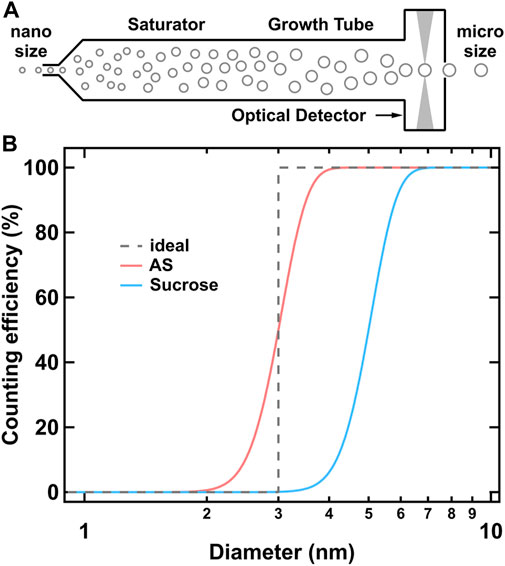
FIGURE 2. (A) Schematic of condensation growth of nanoparticles inside CPC, (B) Schematic of counting efficiency curves of different chemicals for water-based CPC.
In theory, the major features of CPC, such as final droplet size, cut-off diameter, and counting efficiency curve et al., are all associated with the aerosol chemical properties. Therefore, it is reasonable to infer the chemical information based on the fundamentals behind CPC. In addition, the DMA is commonly utilized to get monodisperse particles for the size-resolved analysis. While used in tandem, the diameter difference between two DMAs (before and after the aerosol process module) could be related to the chemical composition of particles. The relevant techniques are illustrated in Figure 3.
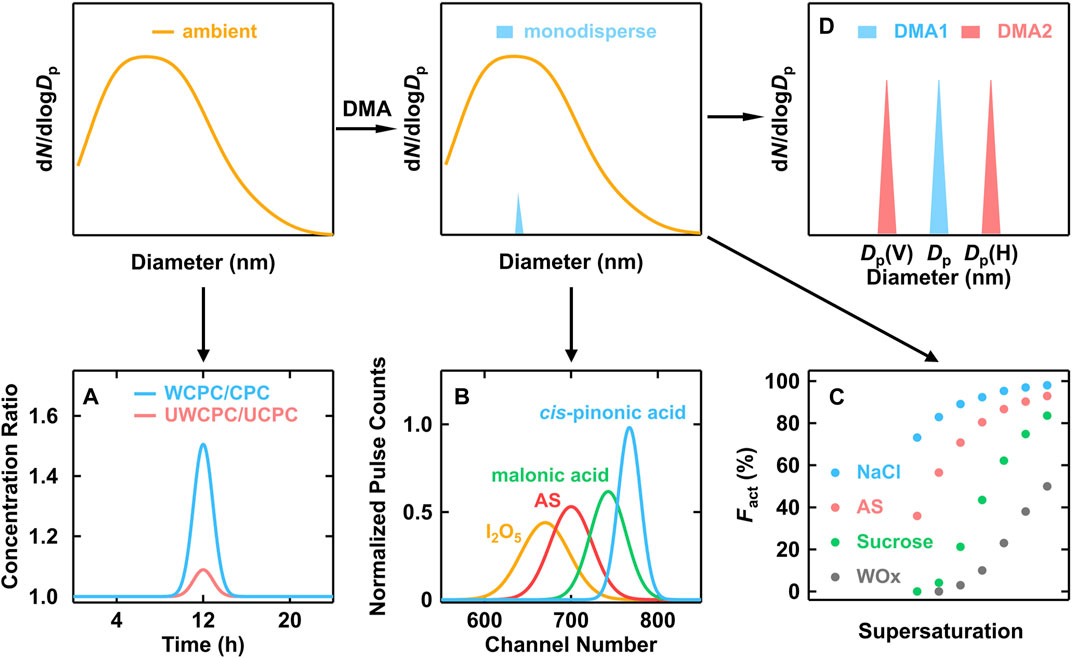
FIGURE 3. Schematic of indirect methods to infer particle chemical composition. (A) CPCB, (B) PHA-CPC, (C) Nano-CCNC, (D) Nano-H(V)-TDMA. The presented data were adopted from literatures (O'Dowd et al., 2004; Kulmala et al., 2007; Wang et al., 2015).
3.1 PHA-CPC
Nanometer particles could be activated to droplets in a near-uniform size by CPC. However, the final droplet size would vary with the initial size and chemical composition of aerosol particles in sub-10 nm diameter range. When the particles of the same size have a good affinity with the working fluid vapor, they grow already at the saturator part by absorbing the working fluid vapor, resulting in a larger final droplet. This phenomenon provides the possibility of inferring chemical composition via the observed final diameter.
Saros et al. (1996) build a Pulse-Height Analyzer CPC (PHA-CPC) to obtain final size information which was detected by the optical counter and transferred from the pulse height signals. In an attempt to yield a symmetric and smoothly varying pulse height response curve, the 90° scattering laser light system in the commercial CPCs had to be replaced with a forward scattering white light source optical system to overcome the Mie multiple scattering response effects, and the signal-processing electronics were modified to increase pulse amplification gain (Marti et al., 1996; Dick et al., 2000; O'Dowd et al., 2004). A systemic laboratory calibration was needed to establish the relationship between pulse height and chemical composition. As shown in Figure 3B, the chemical composition of 5 nm particles was a function of the channel number (PHA response). Since the organic particles (such as cis-pinonic acid and malonic acid) were more soluble in butanol, the final droplet sizes were significantly larger than that of inorganic particles like ammonium sulfate (O'Dowd et al., 2004).
A butanol-based PHA-CPC was deployed at the Hyytiälä site (O'Dowd et al., 2002; O'Dowd et al., 2004). The results agreed well with the PHA-spectra derived from laboratory generated pinic acid and cis-pinonic acid, indicating that the NPF event over forests is mainly driven by the biogenic condensable organic vapors. Similarly, the measurements conducted in the marine area suggested iodine oxides was the dominating species of newly-formed particles in the diameter range of 3–4 nm, while the oxidation production of isoprene, seemed to be responsible for the subsequent growth (Vaattovaara et al., 2006). CI-API-TOF also detect iodine and provide molecular-scale evidence of the aerosol particle formation via sequential addition of HIO3 (Sipilä et al., 2016). The results from TDCIMS did not detect I−, but showed that the chemical composition of particles down to 15 nm was dominated by sea-salt components in the marine area (Lawler et al., 2014). Combination of PHA-CPC and the direct techniques (e.g., CI-API-TOF, TDCIMS) may deepen the understanding of nucleation and subsequent growth.
Compared to direct techniques for particle analysis, PHA-CPC has no dependence on mass concentration of samples. The most important data obtained by PHA-CPC is pulse height, which is related to particle number concentration. Its work principle and the particle number size distribution in atmosphere make it suitable for the 3–10 nm particle measurement. However, it should be noticed that the PHA-CPC could only provide information of aerosol chemical species, which is significantly influenced by the laboratory calibration kernels. In other word, PHA-CPC cannot distinguish the chemical species that have similar pulse height spectra.
3.2 CPC battery
For a CPC with a certain operation condition, the inside supersaturation distribution is identical. But the activation probability would vary according to the different affinities between the working fluid and particle composition (Hermann et al., 2007). In other words, the detected particle number concentration might be different, even using CPCs with the same D50 but different working fluids (Hering et al., 2005; Wlasits et al., 2020). This discrepancy could reflect the affinity of particles to the working fluid. Kulmala et al. (2007) proposed the concept of Condensation Particle Counter Battery (CPCB), composed of two pairs of CPCs (a pair of normal CPCs and a pair of ultrafine CPCs) with different working fluids (water and butanol). By altering the temperature difference of different CPCs, the two pairs of CPCs were adjusted to the same D50 (3 and 11 nm respectively) corresponding to Ag particles, which showed affinity neither to water nor butanol. The chemical species could be recognized to be hydrophilic or hydrophobic depending on the concentration ratio between water-based CPC and butanol-based CPC (Figure 3A).
CPCB has been successfully applied in the field measurements. And the existence of water-soluble organics in the initial and further growth steps could explain the hygroscopic performance of near 3 nm aerosols. The observations in the forest site found that the newly formed particles of 3 and 11 nm were soluble which could be pointed to the involvement of sulfuric acid during the early particle formation and growth processes (Kulmala et al., 2007; Riipinen et al., 2009). In addition, to extend the size down to sub-3 nm, a nano-CPCB consisting of four ultrafine CPCs was assembled and characterized by different types of aerosols (Kangasluoma et al., 2014). But it has not been used in nucleation research yet.
Unlike the afore introduced direct techniques, which are usually hampered by the minuscule amounts of mass, CPCB makes use of the number concentration (Kulmala et al., 2007). For particles in unknown composition, CPCB may provide additional information such as the affinity to one or the other working liquid. Furthermore, CPCB takes advantage of D50 with no need of DMA classification. However, the D50 is easily shifted and frequent calibration is thus required during the long-term measurements. For example, cut-off diameters of the water CPCs in CPCB shifted approximately 0.5 and 1.5 nm for UWCPC and WCPC in around a month, respectively.
3.3 Nano-CCNC
Petters and Kreidenweis (2007) proposed a single hygroscopicity parameter κ to describe the relationship between particle dry diameter and CCN activity. A lot of measurements showed the linear relationship between κ and chemical composition, which was simply parameterized as a function of organic mass fraction (forg) (Dusek et al., 2010; Gunthe et al., 2011; Vogel et al., 2016). This established the fundamental for estimating forg with κ measured by CCN counter. But the CCN measurements were mostly focused on the particle activation sizes above ∼30 nm due to the low supersaturation (S ∼1%). Water-based CPCs have a similar principle to CCNC but much higher S, which promises its capacity to measure the hygroscopicity κ and infer the chemical composition of sub-10 nm particles.
Wang et al. (2015) extended the use of counting efficiency spectra from a nano water-based CPC and link it to the analysis of CCN activation spectra, which provided a theoretical basis for the application of a scanning supersaturation CPC (SS-CPC) as a nano-CCNC. The scanning of supersaturation was done by the variation of temperature difference inside CPC. Particles in different chemical compositions had distinguishable differences in counting efficiency (Figure 3C). The validation of nano-CCNC was done by measuring κ values of ammonium sulfate (AS), sucrose, and their mixtures. A near-linear relationship between hygroscopicity parameter κ and mass fraction of organics was established for nanoparticles down to 2.5 nm.
Besides, nano-CCNC utilized the whole counting efficiency curve compared to CCNC, which only relied on the D50. In summary, the nano-CCNC could give semi-quantitative results compared to PHA-CPC and CPCB, by resolving the mass fraction of organics and inorganics. Based on the linear equation established from lab experiments, it could be used to retrieve the organic fraction during the subsequent growth of newly formed particles. This would be helpful for gapping the understanding of clusters and larger particles. However, it has not been applied to field measurement due to the time cost for the change/scan of supersaturation by altering the temperature difference. The faster response of S variation could be achieved by scanning sample flow of nano-CCNC, which might be more suitable for the field measurements to study hygroscopicity and chemical composition of 3–10 nm atmospheric particles.
3.4 Tandem DMA
TDMA is another widely used technique to measure physical properties. In general, a humidifier or a heater was set between two DMAs, which were known as H-TDMA or V-TDMA, respectively. The diameter difference between the two DMAs reflected the hygroscopic or volatile property of aerosol particles (Figure 3D). For H-TDMA, growth factor (gf), the ratio of wet diameter after humidifier and dry diameter, is used to describe the hygroscopicity. When the measured hygroscopicity is compared to typical organic or inorganic species, this value could be used to infer the composition of particles.
An ultrafine H-TDMA was applied to measure 10 nm, 15 and 20 nm nucleation mode particles in the Hyytiälä station (Hameri et al., 2001), and the results indicated that the soluble fraction of these particles seemed to qualitatively correlate with the presence of sulfuric acid and monoterpene oxidation products. Ambient measurement with nano H(V)-TDMA in urban Atlanta (Sakurai et al., 2005) showed that the newly formed 4–10 nm nanoparticles had similar hygroscopicity and volatility to laboratory-generated ammonium sulfate particles, which was consistent with the TDCIMS measurement. The hygroscopicity parameter (κ) of nucleated nanoparticles in CLOUD chamber was investigated by a nano-HTDMA (Kim et al., 2016). The values of κ for sulfuric acid-dimethylamine and sulfuric acid were 0.61 ± 0.02 and 0.64 ± 0.02 respectively. The obtained κ values of particles in the presence of organics were much smaller, suggesting that the ozonolysis products of α-pinene play a significant role in particle growth (Kim et al., 2016). Besides water humidification, organic vapor, such as ethanol, had also been used to study the affinity between particles and organics. Keskinen et al. (2013) deployed H-TDMA and O-TDMA (organic TDMA) to investigate the hygroscopicity and ethanol affinity of particles. The organic volume fraction derived from H-TDMA is 0.8–0.9 for 63 nm particles, which showed a consistence with that of 65 nm measured by AMS. So, the (nano-)TDMA could be a meaningful supplement for the measurement of chemical composition in a wide range (larger than 4 nm).
The TDMA measurements could provide size-resolved information naturally. The performance was not dependent on the sample mass, just like the aforementioned indirect techniques. However, the number concentration of selected nanoparticles decreased significantly with decreasing particle size, due to the low charge probability and the low transmission efficiency of tandem DMA classification. And this restricted the application of nano-TDMA in field measurements.
4 Discussion
We reviewed the online measurement methods and their field applications for the chemical composition of atmospheric clusters and sub-20 nm particles. The MS-based techniques can obtain chemical composition at a molecular level for clusters and larger particles. For atmospheric clusters, there are only direct techniques for the measurement of chemical composition and these techniques are able to detect particles up to ∼3 nm currently. For sub-20 nm particles, although the MS-based techniques could directly give quantitative chemical information, they are not suitable for the diameter range of sub-10 nm particles, because the pretreatment processes would result in insufficient mass concentration for MS analysis. The highly developed CPC and DMA could measure particle physical properties and infer chemical features afterward. Compared to the direct techniques, the performance of indirect techniques is not depending on sample mass and could make use of the high number concentration of sub-10 nm particles. These indirect techniques have been applied in the size range of 3–10 nm and strengthen our understanding of the nucleation and growth processes of aerosol particles. However, they could only give qualitative information about aerosol composition, e.g. mass/volume fraction of organics.
Although both the direct and indirect techniques could give import information of chemical composition, there is no perfect tool/instrument to cover the whole range of atmosphere clusters and sub-20 nm particles. The techniques for the investigation of chemical composition need further studies. We propose the following outlooks of measurement techniques that can help improving our understanding of nucleation and subsequent growth processes:
1. Although the aforeintroduced techniques are mainly applied in different size range, the combination is still necessary to provide more details of the chemical composition. For example, the parallel measurements of nano H(V)-TDMA and TDCIMS suggested the sub-20 nm particles were mostly composed of ammoniated sulfates in urban Atlanta (Sakurai et al., 2005; Smith et al., 2005). Therefore, more comparisons between different techniques, especially direct and indirect approaches in the overlap diameter range, would be encouraged to give a more comprehensive picture of aerosol chemistry.
2. Currently, several techniques have only been tested in the lab due to the insufficient sample mass or slow time response (e.g., Nano CCNC). We propose that new assistant modules (e.g., pretreatment devices like NanoCharger) or upgraded techniques (such as fast scanning supersaturation counter), may be helpful to expand the application of these techniques to the field measurements.
3. More promising instruments or methodologies are needed to broaden the scope of future nucleation study. For example, DMA-MS could simultaneously measure the mobility and chemical composition. These measurement results could be combined with computational modeling, such as quantum chemical calculations, ion mobility modeling and atmospheric cluster dynamics code, providing reliable structures and candidate growth processes of clusters. A more comprehensive understanding of the nanoparticle revolutions in the atmosphere can be obtained with the methodologies combining measurement and simulation.
Author contributions
ZW, ZnX, and KZ contributed to conception and design of the study. KZ wrote the first draft of the manuscript. KZ, JG, and ZhX wrote sections of the manuscript. All authors contributed to manuscript revision, read, and approved the submitted version.
Funding
This work was supported by the National Natural Science Foundation of China (41805100, 91844301, 42005086), the Fundamental Research Funds for Central Universities of China (2018QNA6008).
Conflict of interest
The authors declare that the research was conducted in the absence of any commercial or financial relationships that could be construed as a potential conflict of interest.
Publisher’s note
All claims expressed in this article are solely those of the authors and do not necessarily represent those of their affiliated organizations, or those of the publisher, the editors and the reviewers. Any product that may be evaluated in this article, or claim that may be made by its manufacturer, is not guaranteed or endorsed by the publisher.
References
Ahonen, L., Li, C., Kubecka, J., Iyer, S., Vehkamaki, H., Petaja, T., et al. (2019). Ion mobility-mass spectrometry of iodine pentoxide-iodic acid hybrid cluster Anions in dry and humidified atmospheres. J. Phys. Chem. Lett. 10 (8), 1935–1941. doi:10.1021/acs.jpclett.9b00453
Almeida, J., Schobesberger, S., Kurten, A., Ortega, I. K., Kupiainen-Maatta, O., Praplan, A. P., et al. (2013). Molecular understanding of sulphuric acid-amine particle nucleation in the atmosphere. Nature 502 (7471), 359–363. doi:10.1038/nature12663
Amo-Gonzalez, M., and Fernandez de la Mora, J. (2017). Mobility peak tailing reduction in a differential mobility analyzer (DMA) coupled with a mass spectrometer and several ionization sources. J. Am. Soc. Mass Spectrom. 28 (8), 1506–1517. doi:10.1007/s13361-017-1630-2
Apsokardu, M. J., Krasnomowitz, J. M., Jiang, S., and Johnston, M. V. (2020). Ion formation from rapidly heated aqueous droplets by droplet-assisted ionization. J. Phys. Chem. A 124 (36), 7313–7321. doi:10.1021/acs.jpca.0c07101
Bianchi, F., Garmash, O., He, X., Yan, C., Iyer, S., Rosendahl, I., et al. (2017). The role of highly oxygenated molecules (HOMs) in determining the composition of ambient ions in the boreal forest. Atmos. Chem. Phys. 17 (22), 13819–13831. doi:10.5194/acp-17-13819-2017
Bianchi, F., Trostl, J., Junninen, H., Frege, C., Henne, S., Hoyle, C. R., et al. (2016). New particle formation in the free troposphere: A question of chemistry and timing. Science 352 (6289), 1109–1112. doi:10.1126/science.aad5456
Bianco, A., Neefjes, I., Alfaouri, D., Vehkamaki, H., Kurten, T., Ahonen, L., et al. (2022). Separation of isomers using a differential mobility analyser (DMA): Comparison of experimental vs modelled ion mobility. Talanta 243, 123339. doi:10.1016/j.talanta.2022.123339
Bzdek, B. R., Lawler, M. J., Horan, A. J., Pennington, M. R., DePalma, J. W., Zhao, J., et al. (2014). Molecular constraints on particle growth during new particle formation. Geophys. Res. Lett. 41 (16), 6045–6054. doi:10.1002/2014gl060160
Bzdek, B. R., Zordan, C. A., Pennington, M. R., Luther, G. W., and Johnston, M. V. (2012). Quantitative assessment of the sulfuric acid contribution to new particle growth. Environ. Sci. Technol. 46 (8), 4365–4373. doi:10.1021/es204556c
Cai, J., Chu, B. W., Yao, L., Yan, C., Heikkinen, L. M., Zheng, F. X., et al. (2020). Size-segregated particle number and mass concentrations from different emission sources in urban Beijing. Atmos. Chem. Phys. 20 (21), 12721–12740. doi:10.5194/acp-20-12721-2020
Chen, Y., Wang, W., Liu, M., and Ge, M. (2020). Measurement technologies of nanoparticle chemical composition and their application. J. Atmos. Environ. Opt. 15 (6), 402–412. doi:10.3969/j.issn.1673-6141.2020.06.001
Criado-Hidalgo, E., Fernandez-Garcia, J., and Fernandez de la Mora, J. (2013). Mass and charge distribution analysis in negative electrosprays of large polyethylene glycol chains by ion mobility mass spectrometry. Anal. Chem. 85 (5), 2710–2716. doi:10.1021/ac303054x
Dick, W. D., McMurry, P. H., Weber, R. J., and Quant, F. R. (2000). White-light detection for nanoparticle sizing with the TSI ultrafine condensation particle counter. J. Nanopart. Res. 2 (1), 85–90. doi:10.1023/A:1010042604201
Dusek, U., Frank, G. P., Curtius, J., Drewnick, F., Schneider, J., Kurten, A., et al. (2010). Enhanced organic mass fraction and decreased hygroscopicity of cloud condensation nuclei (CCN) during new particle formation events. Geophys. Res. Lett. 37 (3), 119. doi:10.1029/2009gl040930
Ehn, M., Junninen, H., Petaja, T., Kurten, T., Kerminen, V. M., Schobesberger, S., et al. (2010). Composition and temporal behavior of ambient ions in the boreal forest. Atmos. Chem. Phys. 10 (17), 8513–8530. doi:10.5194/acp-10-8513-2010
Ehn, M., Kleist, E., Junninen, H., Petaja, T., Lonn, G., Schobesberger, S., et al. (2012). Gas phase formation of extremely oxidized pinene reaction products in chamber and ambient air. Atmos. Chem. Phys. 12 (11), 5113–5127. doi:10.5194/acp-12-5113-2012
Fang, X., Hu, M., Shang, D. J., Tang, R. Z., Shi, L. L., Olenius, T., et al. (2020). Observational evidence for the involvement of dicarboxylic acids in particle nucleation. Environ. Sci. Technol. Lett. 7 (6), 388–394. doi:10.1021/acs.estlett.0c00270
Geller, M. D., Kim, S., Misra, C., Sioutas, C., Olson, B. A., and Marple, V. A. (2002). A methodology for measuring size-dependent chemical composition of ultrafine particles. Aerosol Sci. Technol. 36 (6), 748–762. doi:10.1080/02786820290038447
Gordon, H., Kirkby, J., Baltensperger, U., Bianchi, F., Breitenlechner, M., Curtius, J., et al. (2017). Causes and importance of new particle formation in the present-day and preindustrial atmospheres. J. Geophys. Res. Atmos. 122 (16), 8739–8760. doi:10.1002/2017jd026844
Gunthe, S. S., Rose, D., Su, H., Garland, R. M., Achtert, P., Nowak, A., et al. (2011). Cloud condensation nuclei (CCN) from fresh and aged air pollution in the megacity region of Beijing. Atmos. Chem. Phys. 11 (21), 11023–11039. doi:10.5194/acp-11-11023-2011
Guo, S., Hu, M., Peng, J., Wu, Z., Zamora, M. L., Shang, D., et al. (2020). Remarkable nucleation and growth of ultrafine particles from vehicular exhaust. Proc. Natl. Acad. Sci. U. S. A. 117 (7), 3427–3432. doi:10.1073/pnas.1916366117
Hameri, K., Vakeva, M., Aalto, P. P., Kulmala, M., Swietlicki, E., Zhou, J., et al. (2001). Hygroscopic and CCN properties of aerosol particles in boreal forests. Tellus B 53 (4), 359–379. doi:10.1034/j.1600-0889.2001.530404.x
He, X. C., Tham, Y. J., Dada, L., Wang, M., Finkenzeller, H., Stolzenburg, D., et al. (2021). Role of iodine oxoacids in atmospheric aerosol nucleation. Science 371 (6529), 589–595. doi:10.1126/science.abe0298
Hering, S. V., Stolzenburg, M. R., Quant, F. R., Oberreit, D. R., and Keady, P. B. (2005). A laminar-flow, water-based condensation particle counter (WCPC). Aerosol Sci. Technol. 39 (7), 659–672. doi:10.1080/02786820500182123
Hermann, M., Wehner, B., Bischof, O., Han, H. S., Krinke, T., Liu, W., et al. (2007). Particle counting efficiencies of new TSI condensation particle counters. J. Aerosol Sci. 38 (6), 674–682. doi:10.1016/j.jaerosci.2007.05.001
Hogan, C. J., and Fernandez de la Mora, J. (2010). Ion-pair evaporation from ionic liquid clusters. J. Am. Soc. Mass Spectrom. 21 (8), 1382–1386. doi:10.1016/j.jasms.2010.03.044
Hogan, C. J., and Fernandez de la Mora, J. (2009). Tandem ion mobility-mass spectrometry (IMS-MS) study of ion evaporation from ionic liquid-acetonitrile nanodrops. Phys. Chem. Chem. Phys. 11 (36), 8079–8090. doi:10.1039/b904022f
Hogan, C. J., Ruotolo, B. T., Robinson, C. V., and Fernandez de la Mora, J. (2011). Tandem differential mobility analysis-mass spectrometry reveals partial gas-phase collapse of the GroEL complex. J. Phys. Chem. B 115 (13), 3614–3621. doi:10.1021/jp109172k
Horan, A. J., Apsokardu, M. J., and Johnston, M. V. (2017). Droplet assisted inlet ionization for online analysis of airborne nanoparticles. Anal. Chem. 89 (2), 1059–1062. doi:10.1021/acs.analchem.6b04718
IPCC (2013). “Climate change: the physical science basis,” in contribution of working group I to the fifth assessment report of the intergovernmental panel on climate change. cambridge: cambridge university press.
Jayne, J. T., Leard, D. C., Zhang, X. F., Davidovits, P., Smith, K. A., Kolb, C. E., et al. (2000). Development of an aerosol mass spectrometer for size and composition analysis of submicron particles. Aerosol Sci. Technol. 33 (1-2), 49–70. doi:10.1080/027868200410840
Jen, C. N., Bachman, R., Zhao, J., McMurry, P. H., and Hanson, D. R. (2016). Diamine-sulfuric acid reactions are a potent source of new particle formation. Geophys. Res. Lett. 43 (2), 867–873. doi:10.1002/2015gl066958
Jiang, J., Zhao, J., Chen, M., Eisele, F. L., Scheckman, J., Williams, B. J., et al. (2011). First measurements of neutral atmospheric cluster and 1–2 nm particle number size distributions during nucleation events. Aerosol Sci. Technol. 45 (4), ii–v. doi:10.1080/02786826.2010.546817)
Johnston, M. V., Wang, S., and Reinard, M. S. (2006). Nanoparticle mass spectrometry: Pushing the limit of single particle analysis. Appl. Spectrosc. 60 (10), 264–272. doi:10.1366/000370206778664671
Jokinen, T., Sipila, M., Junninen, H., Ehn, M., Lonn, G., Hakala, J., et al. (2012). Atmospheric sulphuric acid and neutral cluster measurements using CI-APi-TOF. Atmos. Chem. Phys. 12 (9), 4117–4125. doi:10.5194/acp-12-4117-2012
Jokinen, T., Sipila, M., Kontkanen, J., Vakkari, V., Tisler, P., Duplissy, E. M., et al. (2018). Ion-induced sulfuric acid-ammonia nucleation drives particle formation in coastal Antarctica. Sci. Adv. 4 (11), eaat9744. doi:10.1126/sciadv.aat9744
Junninen, H., Ehn, M., Petaja, T., Luosujarvi, L., Kotiaho, T., Kostiainen, R., et al. (2010). A high-resolution mass spectrometer to measure atmospheric ion composition. Atmos. Meas. Tech. 3 (4), 1039–1053. doi:10.5194/amt-3-1039-2010
Kangasluoma, J., and Attoui, M. (2019). Review of sub-3 nm condensation particle counters, calibrations, and cluster generation methods. Aerosol Sci. Technol. 53 (11), 1277–1310. doi:10.1080/02786826.2019.1654084
Kangasluoma, J., Kuang, C., Wimmer, D., Rissanen, M. P., Lehtipalo, K., Ehn, M., et al. (2014). Sub-3 nm particle size and composition dependent response of a nano-CPC battery. Atmos. Meas. Tech. 7 (3), 689–700. doi:10.5194/amt-7-689-2014
Kerecman, D. E., Apsokardu, M. J., Talledo, S. L., Taylor, M. S., Haugh, D. N., Zhang, Y., et al. (2021). Online characterization of organic aerosol by condensational growth into aqueous droplets coupled with droplet-assisted ionization. Anal. Chem. 93 (5), 2793–2801. doi:10.1021/acs.analchem.0c03697
Keskinen, H., Virtanen, A., Joutsensaari, J., Tsagkogeorgas, G., Duplissy, J., Schobesberger, S., et al. (2013). Evolution of particle composition in CLOUD nucleation experiments. Atmos. Chem. Phys. 13 (11), 5587–5600. doi:10.5194/acp-13-5587-2013
Kim, J., Ahlm, L., Yli-Juuti, T., Lawler, M., Keskinen, H., Trostl, J., et al. (2016). Hygroscopicity of nanoparticles produced from homogeneous nucleation in the CLOUD experiments. Atmos. Chem. Phys. 16 (1), 293–304. doi:10.5194/acp-16-293-2016
Kirkby, J., Curtius, J., Almeida, J., Dunne, E., Duplissy, J., Ehrhart, S., et al. (2011). Role of sulphuric acid, ammonia and galactic cosmic rays in atmospheric aerosol nucleation. Nature 476 (7361), 429–433. doi:10.1038/nature10343
Kirkby, J., Duplissy, J., Sengupta, K., Frege, C., Gordon, H., Williamson, C., et al. (2016). Ion-induced nucleation of pure biogenic particles. Nature 533 (7604), 521–526. doi:10.1038/nature17953
Kontkanen, J., Deng, C. J., Fu, Y. Y., Dada, L., Zhou, Y., Cai, J., et al. (2020). Size-resolved particle number emissions in Beijing determined from measured particle size distributions. Atmos. Chem. Phys. 20 (19), 11329–11348. doi:10.5194/acp-20-11329-2020
Krechmer, J. E., Groessl, M., Zhang, X., Junninen, H., Massoli, P., Lambe, A. T., et al. (2016). Ion mobility spectrometry–mass spectrometry (IMS–MS) for on- and offline of atmospheric gas and aerosol species. Atmos. Meas. Tech. 9 (7), 3245–3262. doi:10.5194/amt-9-3245-2016
Kreisberg, N. M., Spielman, S. R., Eiguren-Fernandez, A., Hering, S. V., Lawler, M. J., Draper, D. C., et al. (2018). Water condensation-based nanoparticle charging system: Physical and chemical characterization. Aerosol Sci. Technol. 52 (10), 1167–1177. doi:10.1080/02786826.2018.1503640
Kulmala, M., Kerminen, V. M., Petaja, T., Ding, A. J., and Wang, L. (2017). Atmospheric gas-to-particle conversion: Why NPF events are observed in megacities? Faraday Discuss. 200, 271–288. doi:10.1039/c6fd00257a
Kulmala, M., Mordas, G., Petaja, T., Gronholm, T., Aalto, P. P., Vehkamaki, H., et al. (2007). The condensation particle counter battery (CPCB): A new tool to investigate the activation properties of nanoparticles. J. Aerosol Sci. 38 (3), 289–304. doi:10.1016/j.jaerosci.2006.11.008
Kulmala, M., Petaja, T., Ehn, M., Thornton, J., Sipila, M., Worsnop, D. R., et al. (2014). Chemistry of atmospheric nucleation: On the recent advances on precursor characterization and atmospheric cluster composition in connection with atmospheric new particle formation. Annu. Rev. Phys. Chem. 65, 21–37. doi:10.1146/annurev-physchem-040412-110014
Kürten, A., Jokinen, T., Simon, M., Sipila, M., Sarnela, N., Junninen, H., et al. (2014). Neutral molecular cluster formation of sulfuric acid-dimethylamine observed in real time under atmospheric conditions. Proc. Natl. Acad. Sci. U. S. A. 111 (42), 15019–15024. doi:10.1073/pnas.1404853111
Kürten, A., Rondo, L., Ehrhart, S., and Curtius, J. (2011). Performance of a corona ion source for measurement of sulfuric acid by chemical ionization mass spectrometry. Atmos. Meas. Tech. 4 (3), 437–443. doi:10.5194/amt-4-437-2011
Lanucara, F., Holman, S. W., Gray, C. J., and Eyers, C. E. (2014). The power of ion mobility-mass spectrometry for structural characterization and the study of conformational dynamics. Nat. Chem. 6 (4), 281–294. doi:10.1038/nchem.1889
Larriba, C., Hogan, C. J., Attoui, M., Borrajo, R., Garcia, J. F., and de la Mora, J. F. (2011). The mobility–volume relationship below 3.0 nm examined by tandem mobility–mass measurement. Aerosol Sci. Technol. 45 (4), 453–467. doi:10.1080/02786826.2010.546820
Lawler, M. J., Rissanen, M. P., Ehn, M., Mauldin, R. L., Sarnela, N., Sipila, M., et al. (2018). Evidence for diverse biogeochemical drivers of boreal forest new particle formation. Geophys. Res. Lett. 45 (4), 2038–2046. doi:10.1002/2017gl076394
Lawler, M. J., Whitehead, J., O'Dowd, C., Monahan, C., McFiggans, G., and Smith, J. N. (2014). Composition of 15–85 nm particles in marine air. Atmos. Chem. Phys. 14 (21), 11557–11569. doi:10.5194/acp-14-11557-2014
Lee, S. H., Gordon, H., Yu, H., Lehtipalo, K., Haley, R., Li, Y. X., et al. (2019). New particle formation in the atmosphere: From molecular clusters to global climate. J. Geophys. Res. Atmos. 124 (13), 7098–7146. doi:10.1029/2018jd029356
Lehtipalo, K., Rondo, L., Kontkanen, J., Schobesberger, S., Jokinen, T., Sarnela, N., et al. (2016). The effect of acid-base clustering and ions on the growth of atmospheric nano-particles. Nat. Commun. 7, 11594. doi:10.1038/ncomms11594
Li, C. X., and Hogan, C. J. (2017). Vapor specific extents of uptake by nanometer scale charged particles. Aerosol Sci. Technol. 51 (5), 653–664. doi:10.1080/02786826.2017.1288285
Li, X., Jiang, J., Wang, D., Ge, M., and Hao, J. (2021a). Research progress on the sources and the chemical composition of ambient ultrafine particles. Environ. Chem. 40 (10), 2947–2959. doi:10.7524/j.issn.0254-6108.2021032701
Li, X., Li, Y., Cai, R., Yan, C., Qiao, X., Guo, Y., et al. (2022). Insufficient condensable organic vapors lead to slow growth of new particles in an urban environment. Environ. Sci. Technol. 14, 9936. doi:10.1021/acs.est.2c01566
Li, X., Li, Y., Lawler, M. J., Hao, J., Smith, J. N., and Jiang, J. (2021b). Composition of ultrafine particles in urban beijing: Measurement using a thermal desorption chemical ionization mass spectrometer. Environ. Sci. Technol. 55 (5), 2859–2868. doi:10.1021/acs.est.0c06053
Makela, J. M., Yli-Koivisto, S., Hiltunen, V., Seidl, W., Swietlicki, E., Teinila, K., et al. (2001). Chemical composition of aerosol during particle formation events in boreal forest. Tellus B 53 (4), 380–393. doi:10.1034/j.1600-0889.2001.d01-27.x
Marti, J. J., Weber, R. J., Saros, M. T., Vasiliou, J. G., and McMurry, P. H. (1996). Modification of the TSI 3025 condensation particle counter for pulse height analysis. Aerosol Sci. Technol. 25 (2), 214–218. doi:10.1080/02786829608965392
Mason, E. A., and Schamp, H. W. (1958). Mobility of gaseous lons in weak electric fields. Ann. Phys. 4 (3), 233–270. doi:10.1016/0003-4916(58)90049-6
Massoli, P., Stark, H., Canagaratna, M. R., Krechmer, J. E., Xu, L., Ng, N. L., et al. (2018). Ambient measurements of highly oxidized gas-phase molecules during the southern oxidant and aerosol study (SOAS) 2013. ACS Earth Space Chem. 2 (7), 653–672. doi:10.1021/acsearthspacechem.8b00028
May, J. C., and McLean, J. A. (2015). Ion mobility-mass spectrometry: Time-dispersive instrumentation. Anal. Chem. 87 (3), 1422–1436. doi:10.1021/ac504720m
McDaniel, E. W., Martin, D. W., and Barnes, W. S. (1962). Drift tube-mass spectrometer for studies of low‐energy ion‐molecule reactions. Rev. Sci. Instrum. 33 (1), 2–7. doi:10.1063/1.1717656
Merikanto, J., Spracklen, D. V., Mann, G. W., Pickering, S. J., and Carslaw, K. S. (2009). Impact of nucleation on global CCN. Atmos. Chem. Phys. 9 (21), 8601–8616. doi:10.5194/acp-9-8601-2009
Merikanto, J., Spracklen, D. V., Pringle, K. J., and Carslaw, K. S. (2010). Effects of boundary layer particle formation on cloud droplet number and changes in cloud albedo from 1850 to 2000. Atmos. Chem. Phys. 10 (2), 695–705. doi:10.5194/acp-10-695-2010
O'Dowd, C. D., Aalto, P., Hmeri, K., Kulmala, M., and Hoffmann, T. (2002). Aerosol formation: Atmospheric particles from organic vapours. Nature 416 (6880), 497–498. doi:10.1038/416497a
O'Dowd, C. D., Aalto, P. P., Yoon, Y. J., and Hameri, K. (2004). The use of the pulse height analyser ultrafine condensation particle counter (PHA-UCPC) technique applied to sizing of nucleation mode particles of differing chemical composition. J. Aerosol Sci. 35 (2), 205–216. doi:10.1016/j.jaerosci.2003.08.003
Oberreit, D., Rawat, V. K., Larriba-Andaluz, C., Ouyang, H., McMurry, P. H., and Hogan, C. J. (2015). Analysis of heterogeneous water vapor uptake by metal iodide cluster ions via differential mobility analysis-mass spectrometry. J. Chem. Phys. 143 (10), 104204. doi:10.1063/1.4930278
Oberreit, D. R., McMurry, P. H., and Hogan, C. J. (2014). Analysis of heterogeneous uptake by nanoparticles via differential mobility analysis-drift tube ion mobility spectrometry. Phys. Chem. Chem. Phys. 16 (15), 6968–6979. doi:10.1039/c3cp54842b
Ortega, I. K., Olenius, T., Kupiainen-Määttä, O., Loukonen, V., Kurtén, T., and Vehkamäki, H. (2014). Electrical charging changes the composition of sulfuric acid–ammonia/dimethylamine clusters. Atmos. Chem. Phys. 14 (15), 7995–8007. doi:10.5194/acp-14-7995-2014
Ouyang, H., He, S., Larriba-Andaluz, C., and Hogan, C. J. (2015). IMS-MS and IMS-IMS investigation of the structure and stability of dimethylamine-sulfuric acid nanoclusters. J. Phys. Chem. A 119 (10), 2026–2036. doi:10.1021/jp512645g
Peng, C., Deng, C., Lei, T., Zheng, J., Zhao, J., Wang, D., et al. (2022). Measurement of atmospheric nanoparticles: Bridging the gap between gas-phase molecules and larger particles. J. Environ. Sci. doi:10.1016/j.jes.2022.03.006
Perraud, V., Li, X. X., Jiang, J. K., Finlayson-Pitts, B. J., and Smith, J. N. (2020). Size-resolved chemical composition of sub-20 nm particles from methanesulfonic acid reactions with methylamine and ammonia. ACS Earth Space Chem. 4 (7), 1182–1194. doi:10.1021/acsearthspacechem.0c00120
Petters, M. D., and Kreidenweis, S. M. (2007). A single parameter representation of hygroscopic growth and cloud condensation nucleus activity. Atmos. Chem. Phys. 7 (8), 1961–1971. doi:10.5194/acp-7-1961-2007
Purves, R. W., and Guevremont, R. (1999). Electrospray ionization high-field asymmetric waveform ion mobility spectrometry-mass spectrometry. Anal. Chem. 71 (13), 2346–2357. doi:10.1021/ac981380y
Renzler, M., Kranabetter, L., Goulart, M., Scheier, P., and Echt, O. (2017). Positively and negatively charged cesium and (C60)mCsn cluster ions. J. Phys. Chem. C 121 (20), 10817–10823. doi:10.1021/acs.jpcc.6b11928
Riccobono, F., Schobesberger, S., Scott, C. E., Dommen, J., Ortega, I. K., Rondo, L., et al. (2014). Oxidation products of biogenic emissions contribute to nucleation of atmospheric particles. Science 344 (6185), 717–721. doi:10.1126/science.1243527
Riipinen, I., Manninen, H. E., Yli-Juuti, T., Boy, M., Sipilä, M., Ehn, M., et al. (2009). Applying the Condensation Particle Counter Battery (CPCB) to study the water-affinity of freshly-formed 2–9 nm particles in boreal forest. Atmos. Chem. Phys. 9 (10), 3317–3330. doi:10.5194/acp-9-3317-2009
Rönkkö, T., Kuuluvainen, H., Karjalainen, P., Keskinen, J., Hillamo, R., Niemi, J. V., et al. (2017). Traffic is a major source of atmospheric nanocluster aerosol. Proc. Natl. Acad. Sci. U. S. A. 114 (29), 7549–7554. doi:10.1073/pnas.1700830114
Rönkkö, T., and Timonen, H. (2019). Overview of sources and characteristics of nanoparticles in urban traffic-influenced areas. J. Alzheimers Dis. 72 (1), 15–28. doi:10.3233/JAD-190170
Rose, C., Zha, Q., Dada, L., Yan, C., Lehtipalo, K., Junninen, H., et al. (2018). Observations of biogenic ion-induced cluster formation in the atmosphere. Sci. Adv. 4 (4), eaar5218. doi:10.1126/sciadv.aar5218
Ross Pennington, M., and Johnston, M. V. (2012). Trapping charged nanoparticles in the nano aerosol mass spectrometer (NAMS). Int. J. Mass Spectrom. 311, 64–71. doi:10.1016/j.ijms.2011.12.011
Rus, J., Moro, D., Sillero, J. A., Royuela, J., Casado, A., Estevez-Molinero, F., et al. (2010). IMS–MS studies based on coupling a differential mobility analyzer (DMA) to commercial API–MS systems. Int. J. Mass Spectrom. 298 (1), 30–40. doi:10.1016/j.ijms.2010.05.008
Saarikoski, S., Williams, L. R., Spielman, S. R., Lewis, G. S., Eiguren-Fernandez, A., Aurela, M., et al. (2019). Laboratory and field evaluation of the Aerosol Dynamics Inc. concentrator (ADIc) for aerosol mass spectrometry. Atmos. Meas. Tech. 12 (7), 3907–3920. doi:10.5194/amt-12-3907-2019
Sakurai, H., Fink, M. A., McMurry, P. H., Mauldin, L., Moore, K. F., Smith, J. N., et al. (2005). Hygroscopicity and volatility of 4–10 nm particles during summertime atmospheric nucleation events in urban Atlanta. J. Geophys. Res. 110 (22), D22S04. doi:10.1029/2005jd005918
Saros, M. T., Weber, R. J., Marti, J. J., and McMurry, P. H. (1996). Ultrafine aerosol measurement using a condensation nucleus counter with pulse height analysis. Aerosol Sci. Technol. 25 (2), 200–213. doi:10.1080/02786829608965391
Seinfeld, J. H., and Pandis, S. N. (2016). Atmospheric chemistry and physics: From air pollution to climate change Hoboken, NJ: John Wiley & Sons.
Sipilä, M., Sarnela, N., Jokinen, T., Henschel, H., Junninen, H., Kontkanen, J., et al. (2016). Molecular-scale evidence of aerosol particle formation via sequential addition of HIO3. Nature 537 (7621), 532–534. doi:10.1038/nature19314
Smith, J. N., Barsanti, K. C., Friedli, H. R., Ehn, M., Kulmala, M., Collins, D. R., et al. (2010). Observations of aminium salts in atmospheric nanoparticles and possible climatic implications. Proc. Natl. Acad. Sci. U. S. A. 107 (15), 6634–6639. doi:10.1073/pnas.0912127107
Smith, J. N., Draper, D. C., Chee, S., Dam, M., Glicker, H., Myers, D., et al. (2021). Atmospheric clusters to nanoparticles: Recent progress and challenges in closing the gap in chemical composition. J. Aerosol Sci. 153, 105733. doi:10.1016/j.jaerosci.2020.105733
Smith, J. N., Moore, K. F., Eisele, F. L., Voisin, D., Ghimire, A. K., Sakurai, H., et al. (2005). Chemical composition of atmospheric nanoparticles during nucleation events in Atlanta. J. Geophys. Res. 110 (D22), D22S03. doi:10.1029/2005jd005912
Smith, J. N., Moore, K. F., McMurry, P. H., and Eisele, F. L. (2004). Atmospheric measurements of sub-20 nm diameter particle chemical composition by thermal desorption chemical ionization mass spectrometry. Aerosol Sci. Technol. 38 (2), 100–110. doi:10.1080/02786820490249036
Tao, Y., Ye, X. N., Jiang, S. Q., Yang, X., Chen, J. M., Xie, Y. Y., et al. (2016). Effects of amines on particle growth observed in new particle formation events. J. Geophys. Res. Atmos. 121 (1), 324–335. doi:10.1002/2015jd024245
Thomas, J. M., He, S., Larriba-Andaluz, C., DePalma, J. W., Johnston, M. V., and Hogan, C. J. (2016). Ion mobility spectrometry-mass spectrometry examination of the structures, stabilities, and extents of hydration of dimethylamine-sulfuric acid clusters. Phys. Chem. Chem. Phys. 18 (33), 22962–22972. doi:10.1039/c6cp03432b
Vaattovaara, P., Huttunen, P. E., Yoon, Y. J., Joutsensaari, J., Lehtinen, K. E. J., O'Dowd, C. D., et al. (2006). The composition of nucleation and aitken modes particles during coastal nucleation events: Evidence for marine secondary organic contribution. Atmos. Chem. Phys. 6 (12), 4601–4616. doi:10.5194/acp-6-4601-2006
Vaattovaara, P., Rasanen, M., Kuhn, T., Joutsensaari, J., and Laaksonen, A. (2005). A method for detecting the presence of organic fraction in nucleation mode sized particles. Atmos. Chem. Phys. 5 (12), 3277–3287. doi:10.5194/acp-5-3277-2005
Viitanen, A. K., Saukko, E., Virtanen, A., Yli-Pirilaa, P., Smith, J. N., Joutsensaari, J., et al. (2010). Ion mobility distributions during the initial stages of new particle formation by the ozonolysis of alpha-pinene. Environ. Sci. Technol. 44 (23), 8917–8923. doi:10.1021/es101572u
Vogel, A. L., Schneider, J., Muller-Tautges, C., Phillips, G. J., Pohlker, M. L., Rose, D., et al. (2016). Aerosol chemistry resolved by mass spectrometry: Linking field measurements of cloud condensation nuclei activity to organic aerosol composition. Environ. Sci. Technol. 50 (20), 10823–10832. doi:10.1021/acs.est.6b01675
Voisin, D., Smith, J. N., Sakurai, H., McMurry, P. H., and Eisele, F. L. (2003). Thermal desorption chemical ionization mass spectrometer for ultrafine particle chemical composition. Aerosol Sci. Technol. 37 (6), 471–475. doi:10.1080/02786820300959
Wagner, A. C., Bergen, A., Brilke, S., Fuchs, C., Ernst, M., Hoker, J., et al. (2018). Size-resolved online chemical analysis of nanoaerosol particles: A thermal desorption differential mobility analyzer coupled to a chemical ionization time-of-flight mass spectrometer. Atmos. Meas. Tech. 11 (10), 5489–5506. doi:10.5194/amt-11-5489-2018
Wang, D., Li, Q., Shen, G., Deng, J., Zhou, W., Hao, J., et al. (2020a). Significant ultrafine particle emissions from residential solid fuel combustion. Sci. Total Environ. 715, 136992. doi:10.1016/j.scitotenv.2020.136992
Wang, M., Kong, W., Marten, R., He, X. C., Chen, D., Pfeifer, J., et al. (2020b). Rapid growth of new atmospheric particles by nitric acid and ammonia condensation. Nature 581 (7807), 184–189. doi:10.1038/s41586-020-2270-4
Wang, S., and Johnston, M. V. (2006). Airborne nanoparticle characterization with a digital ion trap–reflectron time of flight mass spectrometer. Int. J. Mass Spectrom. 258 (1-3), 50–57. doi:10.1016/j.ijms.2006.07.001
Wang, S., Zordan, C. A., and Johnston, M. V. (2006). Chemical characterization of individual, airborne sub-10-nm particles and molecules. Anal. Chem. 78 (6), 1750–1754. doi:10.1021/ac052243l
Wang, Z. B., Hu, M., Sun, J. Y., Wu, Z. J., Yue, D. L., Shen, X. J., et al. (2013). Characteristics of regional new particle formation in urban and regional background environments in the North China Plain. Atmos. Chem. Phys. 13 (24), 12495–12506. doi:10.5194/acp-13-12495-2013
Wang, Z., Su, H., Wang, X., Ma, N., Wiedensohler, A., Poschl, U., et al. (2015). Scanning supersaturation condensation particle counter applied as a nano-CCN counter for size-resolved analysis of the hygroscopicity and chemical composition of nanoparticles. Atmos. Meas. Tech. 8 (5), 2161–2172. doi:10.5194/amt-8-2161-2015
Wang, Z., Wu, Z., Yue, D., Shang, D., Guo, S., Sun, J., et al. (2017). New particle formation in China: Current knowledge and further directions. Sci. Total Environ. 577, 258–266. doi:10.1016/j.scitotenv.2016.10.177
Weber, R. J., McMurry, P. H., Mauldin, R. L., Tanner, D. J., Eisele, F. L., Clarke, A. D., et al. (1999). New particle formation in the remote troposphere: A comparison of observations at various sites. Geophys. Res. Lett. 26 (3), 307–310. doi:10.1029/1998gl900308
Wlasits, P. J., Stolzenburg, D., Tauber, C., Brilke, S., Schmitt, S. H., Winkler, P. M., et al. (2020). Counting on chemistry: Laboratory evaluation of seed-material-dependent detection efficiencies of ultrafine condensation particle counters. Atmos. Meas. Tech. 13 (7), 3787–3798. doi:10.5194/amt-13-3787-2020
Yao, L., Garmash, O., Bianchi, F., Zheng, J., Yan, C., Kontkanen, J., et al. (2018). Atmospheric new particle formation from sulfuric acid and amines in a Chinese megacity. Science 361 (6399), 278–281. doi:10.1126/science.aao4839
Yin, R., Yan, C., Cai, R., Li, X., Shen, J., Lu, Y., et al. (2021). Acid-base clusters during atmospheric new particle formation in urban beijing. Beijing: Environmental Science & Technology. doi:10.1021/acs.est.1c02701
Zhang, R., Wang, L., Khalizov, A. F., Zhao, J., Zheng, J., McGraw, R. L., et al. (2009). Formation of nanoparticles of blue haze enhanced by anthropogenic pollution. Proc. Natl. Acad. Sci. U. S. A. 106 (42), 17650–17654. doi:10.1073/pnas.0910125106
Zhang, X., Lambe, A. T., Upshur, M. A., Brooks, W. A., Gray Be, A., Thomson, R. J., et al. (2017). Highly oxygenated multifunctional compounds in alpha-pinene secondary organic aerosol. Environ. Sci. Technol. 51 (11), 5932–5940. doi:10.1021/acs.est.6b06588
Zhang, X., Zhang, H. F., Xu, W., Wu, X. K., Tyndall, G. S., Orlando, J. J., et al. (2019). Molecular characterization of alkyl nitrates in atmospheric aerosols by ion mobility mass spectrometry. Atmos. Meas. Tech. 12 (10), 5535–5545. doi:10.5194/amt-12-5535-2019
Zhao, J., Eisele, F. L., Titcombe, M., Kuang, C. G., and McMurry, P. H. (2010). Chemical ionization mass spectrometric measurements of atmospheric neutral clusters using the cluster-CIMS. J. Geophys. Res. 115 (D8), D08205. doi:10.1029/2009jd012606
Zhao, J., Ortega, J., Chen, M., McMurry, P. H., and Smith, J. N. (2013). Dependence of particle nucleation and growth on high-molecular-weight gas-phase products during ozonolysis of α-pinene. Atmos. Chem. Phys. 13 (15), 7631–7644. doi:10.5194/acp-13-7631-2013
Zhao, J., Smith, J. N., Eisele, F. L., Chen, M., Kuang, C., and McMurry, P. H. (2011). Observation of neutral sulfuric acid-amine containing clusters in laboratory and ambient measurements. Atmos. Chem. Phys. 11 (21), 10823–10836. doi:10.5194/acp-11-10823-2011
Appendix 1: List of abbreviations
Keywords: chemical composition, cluster, ultrafine particle, online measurement, new particle formation
Citation: Zhang K, Xu Z, Gao J, Xu Z and Wang Z (2022) Review of online measurement techniques for chemical composition of atmospheric clusters and sub-20 nm particles. Front. Environ. Sci. 10:937006. doi: 10.3389/fenvs.2022.937006
Received: 05 May 2022; Accepted: 20 July 2022;
Published: 09 September 2022.
Edited by:
Yue Zhao, Shanghai Jiao Tong University, ChinaReviewed by:
Xiaoxiao Li, Tsinghua University, ChinaJunfeng Wang, Nanjing University of Information Science and Technology, China
Copyright © 2022 Zhang, Xu, Gao, Xu and Wang. This is an open-access article distributed under the terms of the Creative Commons Attribution License (CC BY). The use, distribution or reproduction in other forums is permitted, provided the original author(s) and the copyright owner(s) are credited and that the original publication in this journal is cited, in accordance with accepted academic practice. No use, distribution or reproduction is permitted which does not comply with these terms.
*Correspondence: Zhibin Wang, wangzhibin@zju.edu.cn