Research on a new power system development planning model based on two-tier planning
- 1State Grid Sichuan Economic Research Institute, Chengdu, Sichuan, China
- 2State Grid Sichuan New Power System Research Institute, Chengdu, Sichuan, China
With the rapid development of the power industry, the safe and stable operation of the power system has become an important basis for safeguarding economic and social development. In order to adapt to the trend of rapid growth of new energy sources and the demand for transformation and upgrading of the power industry, the capacity allocation scheme of the power system needs to be constantly optimized and adjusted. To address the development planning of new power systems, a new power system development planning model based on two-layer planning is constructed, taking into account the operational output results of various types of units and the interaction between the configuration schemes. Through the analysis of the evolution of the power system in a region in the next 15 years, the development pattern of the new power system in different stages under the high, medium, and low scenarios of new energy development is optimally calculated to guide the development of the power system. The results show that the model can provide a quantitative reference for decision-making on energy policies and power strategies in the medium and long term.
1 Introduction
In September 2020, China put forward the “carbon peak and carbon neutrality” goal of “striving to reach peak CO2 emissions by 2030 and achieving carbon neutrality by 2060″ at the United Nations General Assembly. Under the guidance of the “carbon peak and carbon neutrality” target, it has become a major trend to establish an energy supply system with renewable energy as the mainstay and achieve clean and low-carbon energy transformation and development (Zhou et al., 2023). Under the existing technical conditions, new energy sources are significantly affected by natural conditions, and their reliability is low under extreme scenarios. Their large-scale access poses a great challenge to the balance and adjustability of the power system. For this reason, it is important to plan the capacity allocation of the power system rationally, strengthen the coordination between new energy and grid-related planning and construction, and ensure the safe and stable operation of the power system.
Power system planning and configuration is a complex task involving multiple factors and interdependencies. For this reason, it is crucial to study power system development paths and planning models. With regard to the power system development path, the current literature is relatively limited in the scope of research on the power system development path. Although it involves research on carbon neutrality targets, renewable energy, etc., it does not address, for example, the impacts of national policies and energy market changes on the power system development path. In terms of power system development paths, Zhang et al. (2021) analyzed multiple challenges faced in building a new power system with new energy as the mainstay, such as safety and stability, optimal dispatching, transmission, and consumption, and proposed the development paths for building a new power system with new energy as the mainstay from the power supply side, grid side, and load side, respectively, taking into account the current situation of new energy development in China. Based on the future development trend of power grid evolution, Lu et al. (2021) proposed a global sensitivity method considering flexibility balance, took into account multiple uncertainties affecting the evolution path, sampled a large number of samples for power system evolution simulation, and analyzed the evolution path of China’s high proportion of renewable energy development using the northwest Chinese power grid as an example. Li et al. (2021a) studied the development of the power system in the 14th Five-Year Plan in terms of power demand growth, power supply structure adjustment, grid pattern optimization, and application of new technologies. A calculation model for energy and power development based on the goal of carbon compliance and carbon neutrality is proposed, and an outlook on the medium- and long-term energy and power evolution path is given. Zhang and Kang (2022) analyzed the connotation and characteristics of the new power system from four aspects, namely, structure, form, technology, and mechanism, and the evolution path of the new power system is analyzed and judged. Based on the characteristics of China’s power supply and load distribution and the future changes in power supply composition and taking into account the increasing intermittent power supply in China and the development trend of the energy internet, Yang et al. (2018) foresaw the structural forms required for China’s ultra-high-voltage power grid in the transitional and long-term periods before and after 2030 and 2050. Dallmann et al. (2022) addressed the issue of potential pathways for the Egyptian power system, considering regional deployment and its associated impact on the transmission network, and evaluated the renewable energy potential of 320 sites in eight different sub-regions in Egypt, finding that a pathway for power system development that emphasizes wind and PV generation is cost-competitive. Kabeyi and Olanrewaju (2022) proposed a sustainable energy transition roadmap for sustainable power generation and supply based on energy efficiency on the demand side, generation efficiency at the production level, and various energy transition strategies such as renewable energy sources and low-carbon nuclear energy alternatives to fossil fuels, in conjunction with the Paris Agreement commitments. Chen et al. (2021a) combined hourly power system simulations for all provinces throughout the year to build a cross-sectoral, high-resolution assessment model to evaluate renewable energy balancing costs and explored cost-competitive pathways to decarbonize the power system. Wang et al. (2021a) addressed the issue of carbon attainment and carbon neutrality in China’s thermal power industry, considered the current status and future development trend of thermal power generation technology and carbon capture technology, and found that in terms of thermal power low-carbon applications and technologies, the focus should be on high-efficiency thermal power technology and peaking technology, which points the way to low-carbon development in the thermal power industry. Cai et al. (2022), taking into account the driving factors for China to achieve the “carbon peak and carbon neutrality” target and the long-term research and development of CAS in the energy field, proposed the path of technology development to achieve the “carbon peak and carbon neutrality” target, including clean and efficient utilization and coupled substitution of fossil energy and non-fossil energy. Shu et al. (2021) proposed a technology development pathway to achieve the “carbon peak and carbon neutrality” goal, including clean and efficient utilization and coupled substitution of fossil energy, multi-energy complementarity, and large-scale application of non-fossil energy. Based on the evaluation of power carbon balance, Shu et al. (2021) constructed three power transition scenarios, namely, deep low-carbon, zero-carbon, and negative-carbon, studied key boundary conditions such as electricity demand, and determined the low-carbon transition paths for power structure, power carbon emission, and power supply cost under different scenarios. Feng and Li (2022) proposed a “three-step” carbon reduction strategy for coal-fired power units on the basis of the existing relevant technology systems, i.e., low carbon through technical carbon reduction (coal reduction and deep peaking), zero carbon through fuel decarbonization, and negative carbon through flue gas decarbonization. Current research studies are often limited to a specific aspect of research, such as power system planning, energy transition, and power market, and lack a comprehensive and systematic study of the entire power system. The development of the power system involves the interaction of several key areas, and the above studies have not yet considered the interaction between equipment capacity allocation and the optimal operation of the power system.
In terms of power system planning models, Chen et al. (2021b) analyzed the main influencing factors of carbon emissions in the process of power supply structure transformation and their related relationships, constructed system dynamic models for four different development scenarios, and simulated and analyzed the evolution trend of power generation structure and power carbon emissions under four development paths. It is found that the development of market consumption mechanism and consumption technology promotes the transformation of power generation structure of the power system, which is important for the realization of carbon peak and carbon neutrality target. Lu et al. (2017) addressed the issue of the power system structure morphology of high-proportional renewable energy and established a model for the evolution of power system morphology driven by high-proportional renewable energy, taking into account the development trend of high-proportional renewable energy. XIE et al. (2018) addressed the problem of future power system evolution and development form, introduced power, electricity, peaking, and environmental constraints into the power system evolution model with the minimum construction cost as the optimization objective, established a simplified model of several frontier technologies from the perspective of power system operation, and finally proposed a solution method for the evolution model by combining heuristic rules and simulated plant growth algorithms. Wei et al. (2022) constructed the source network expansion planning model with the total planning cost as the optimization objective, and the “carbon-peak–carbon-neutral” transition path of China’s power system from 2020 to 2060 is planned and optimized, taking into account the natural resource endowment of renewable energy areas and the operational safety constraints of the power system in order to address the morphological structure of the power system in the process of low-carbon transition. Wyrwa et al. (2022) developed a long-term power system development planning model combining a generation expansion planning model (i.e., TIMES-PL) with a unit commitment and economic dispatch model (MEDUSA) to address the issue of a carbon-neutral pathway for the power system, and three scenarios for the development of the power system were formulated based on the goal of achieving a 95% reduction in CO2 emissions by 2050 compared to 1990. Li et al. (2021b) considered uncertainties in wind output and contingency probabilities and proposed a robust transmission expansion planning model that reduces different types of uncertainties in the power system planning phase and improves the reliability and cost effectiveness of the power system. Hamidpour et al. (2022) minimized the sum of power system expansion planning, operation, and reliability costs, while considering a network model based on AC optimal tide constraints as well as reliability and flexibility considerations, to develop a flexible coordinated power system expansion planning model. He et al. (2020) addressed the pathway to decarbonize China’s power system by approximately 2030, considered the latest trends in renewable energy and energy storage costs, modeled capacity expansion for solar, wind, hydro, and conventional technology investments, and analyzed the impact of rapidly declining renewable energy costs. Quiroga et al. (2019) considered optimal expansion plans for large power plant installations and renewable energy-based distributed generation and developed an optimization model based on mixed integer linear programming to analyze the impact of implementing CO2 and local pollutant emission taxes on power system expansion planning in five different policy-relevant scenarios. Wang et al. (2021b) proposed a stochastic investment planning model considering demand response for the power system planning problem with high variable renewable energy penetration levels, which effectively addresses the lack of operational flexibility of the power system and enhances the disaster tolerance potential of the power system to avoid energy storage investments. Wei et al. (2023) proposed an optimization model for the heating system in Northeast China based on the energy policy stage objectives, considering carbon taxes and subsidies, found that carbon taxes and subsidies have an impact on the changes in regional heating sources, and numerically optimized the carbon taxes and subsidies in regional heating. Li et al. (2021c) comprehensively considered the construction and operation costs of power plants and constructed an optimal expansion planning model for combined thermal-landscape voltaic power generation systems under the boundary conditions of ensuring the safe operation of the power grid, and the proposed expansion scheme can achieve the synergistic development of the three types of power sources under multiple boundary conditions. In order to effectively deal with the uncertainty and operational flexibility of renewable energy sources, Moradi-Sepahvand and Amraee (2021) established an integrated multi-cycle model for the long-term expansion planning of the transmission grid, generation technologies, and energy storage devices, giving the types, sizes, and locations of generation, transmission, and storage devices to meet the electricity load demand within the planning horizon. Many of the power system planning models in the above literature only focus on a single objective, such as economics or environmental sustainability, and have not yet examined power system development in a comprehensive and integrated manner. Most of the models used in the above literature are single-layer planning models, while the two-layer planning model is a more complex optimization model that can better consider the continuous optimization and adjustment of the capacity allocation scheme of the power system.
In summary, power supply restructuring, grid pattern optimization, new technology application, and carbon emission constraints have been analyzed in existing studies, but the studies have not considered the interaction between equipment capacity allocation and optimal power system operation and have not fully considered the organic combination of system capacity allocation and system construction costs.
In order to optimize the power system operation and design a reasonable power system capacity allocation scheme, this paper proposes a new power system development planning model based on two-tier planning. First, the framework of the new power system development planning model based on two-tier planning is proposed. Then, a new two-tier power system development planning model is established, in which the upper model takes the shortest payback period as the objective function to determine the installed capacity of new hydropower generation, wind power generation, photovoltaic power generation, energy storage, and other types of units and the lower model considers the results of the installed capacity of hydropower generation, wind power generation, photovoltaic power generation, thermal power generation, and energy storage and is responsible for optimizing the operation of the power system. Finally, the simulation results show that the capacity optimization scheme of the proposed model can significantly improve the economy and stability of the power system.
2 Description of the problem
With the rapid development of the electric power industry, the safe and stable operation of the electric power system has established an important foundation for safeguarding economic and social development. In order to adapt to the trend of rapid growth of new energy sources and the demand for transformation and upgrading of the power industry, the development planning of new power systems faces the following problems:
(1) Flexibility and dispatchability of power systems: While traditional power systems are dominated by large-scale power plants, newer power systems will utilize more distributed energy sources and renewable energy sources, such as photovoltaic and wind power. This decentralized energy structure increases the flexibility and dispatchability requirements of the power system, requiring the establishment of more flexible and intelligent power system planning and operation mechanisms to adapt to the rapid changes in different energy sources and the dynamic adjustment of demand.
(2) Environmental sustainability and carbon emission constraints: The development of the new power system should be consistent with the goals of environmental sustainability and carbon emission reduction. Therefore, environmental issues need to be considered in power system development planning.
(3) Policy support and market issues: With the rapid development of new energy sources, the energy market and policies are constantly changing, and the development planning of new power systems needs to pay attention to policy and market changes.
3 Introduction to the methodology
In order to reduce system planning costs and maximize system operation benefits, this paper proposes to adopt a new power system development planning model with two-layer planning. The model is usually used to solve complex problems with two levels in planning the development of new power systems. The two-layer planning model can take into account the sustainability of the environment and, at the same time, consider the interests of the upper and lower levels and coordinate and solve the conflicts between different levels. Here, the power system as a whole is considered for the overall optimization of the power system not only to maintain the efficient operation of the power system but also to analyze the changing policy environment, help the upper and lower levels of the main body to make timely and corresponding changes, and facilitate flexible planning.
The two-layer planning model applied to the development of new power system planning considers the upper and lower levels of the main body: the upper level, on behalf of the overall planning of the power system, is responsible for the comprehensive consideration of the upper and lower levels of goals and constraints and coordination of decision-making; the lower level, on behalf of the power system of the participants, such as power generation and distributed energy, is responsible for corresponding to the upper level of the planning program and the specific decision-making to achieve the desired benefits of the operation of the system. The two-layer planning model coordinates the upper and lower layers and influences each other to help realize the sustainable development of the power system.
4 Two-layer new power system development planning model
The new power system development planning model based on two-layer planning includes two models: power system planning and power system operation. The model takes the premise of reducing system planning costs and maximizing system operation benefits as the starting point and iteratively calculates through two-layer decision-making to obtain a power system capacity allocation scheme that meets the demand for collaborative and optimized operation of the power system. The upper model is a capacity allocation model for the power system with the lowest total cost as the objective, and it generates capacity allocation schemes for hydropower generation, wind power generation, photovoltaic power generation, energy storage, and other types of units to the lower layer; the lower layer generates system operation and maintenance costs to the upper model with the objective of maximizing the operating benefits of hydropower generation, wind power generation, photovoltaic power generation, thermal power generation, and energy storage and other types of units of the system. The upper- and lower-layer objectives are subjected to upper-layer constraints (new hydropower capacity, new energy storage capacity, new capacity of scenic units, etc.) and lower-layer constraints (system power balance, system inter-provincial channel, physical characteristics of units, etc.), respectively. Figure 1 shows the model framework. In the specific decision, the upper-layer objectives make the decision first, while the lower layer reacts according to the upper-layer decision, thus obtaining the optimal planning solution by balancing the interests of the two layers.
4.1 Upper-layer model
4.1.1 Objective function
The upper layer is built into the system with the shortest payback period as the objective function:
where
(1) Total system investment cost
To meet load demand, new energy development, and maximum self-sufficiency in the system, the province needs to add various types of machine assembly machines, such as hydropower, wind power, photovoltaic power generation, and energy storage. In the formula,
(2) Total system operation and maintenance costs
where
4.1.2 Constraints
The upper-tier model is constrained by the capacity/number of units in each category.
(1) Hydropower new capacity constraints:
where
(2) New capacity constraints for energy storage:
where
(3) Additional capacity of wind turbine NW constraints:
where
(4) PV panel capacity addition NP constraints
where
4.2 Lower-level model
4.2.1 Objective function
The lower objective function is the maximum expected total benefit to the system:
where
where a, b, and c are the generation cost factors for thermal power units.
where
4.2.2 Constraints
(1) System power balance constraint
where,
(2) System inter-provincial channel constraints
where
(3) Thermal power unit constraints
where
(4) Hydropower unit constraints
where
(5) Energy storage constraints
where
(6) Total carbon emission constraint
This indicator can reflect the low carbon level of the new power system, which is bounded by.
where
(7) The proportion of renewable energy generation
This index can reflect the process of low-carbon transformation of the new power system and meet the requirements of the new energy quota system in the energy policy. Its constraints are as follows:
where
4.3 Solving algorithm
The new power system development planning model established in this paper is a two-layer programming model. The framework design of the two-layer programming model enables it to consider the two aspects of power system planning and operation comprehensively so as to obtain the optimal power system capacity allocation scheme. In the upper model, the installed capacity of all types of generating units is optimized by considering constraints such as the new capacity of all types of units so as to obtain the capacity configuration scheme of the power system with the lowest total cost. The optimization variables include the installed capacity of hydropower, thermal power, wind power, photovoltaic, and other types of units. The lower model optimizes the output results of all types of generating units with the goal of maximizing the operation benefit of the system so as to obtain the system operation and maintenance costs and other feedback to the upper model. The optimization variables include the output of hydropower, thermal power, wind power, photovoltaic, provincial input power, and other power sources, and the installed capacity of energy storage is solved according to the demand for energy storage in the optimization operation.
At the same time, the two-tier planning model also considers environmental protection, safety, and reliability factors as well as external factors such as national policies and energy market changes. In the upper-level planning model, we adopt the two-carbon target constraint and the renewable energy generation proportion constraint to limit the lower limit of installed thermal power capacity and installed solar power capacity so as to promote the utilization of renewable energy and reduce carbon emissions. In the lower operation model, we adopted the piecewise linearization method of the time-sharing operation curve and carried out detailed modeling and optimization on the power balance of the system, the charge and discharge control of the energy storage system, and the start and stop of the unit.
The optimization objective of this two-layer model is to obtain the lowest total cost by the sum of the construction cost and operation and maintenance cost of all types of units and the maximum total income from the operation of all types of units such as hydraulic power generation, wind power generation, photovoltaic power generation, thermal power generation, and energy storage. For the upper programming model, it is transformed into a linear programming problem, and constraints are added. For the lower programming model, the piecewise linearization method is used to transform it into a linear programming problem, and constraints are added.
The solving process of this model can adopt the large-scale linear programming method and use mature solvers (such as CPLEX and Gurobi) so as to improve the solving efficiency.
5 Example analysis
5.1 Considering the basic case
On the basis of considering the development planning model of a two-tier new power system, this paper takes the data of a provincial power grid in Southwest China as an example to solve the installed capacity of the new power system in this region at a 5-year interval from 2025 to 2035. In the current situation, the power generation in this region is mainly hydropower, with some thermal power, and the province is vigorously developing new energy power generation, mainly wind power and photovoltaic power. In 2022, the region will be affected by continuous high temperature and drought, which will bring great challenges to the power grid. Therefore, the province is also vigorously building foreign power input channels to enhance the power system’s ability to cope with the challenge of extreme scenarios. At the same time, as this region is an important sending end of “power transmission from west to east,” it needs to bear a large responsibility of power transmission. Therefore, the construction of the transmission channel is also carried out synchronously in this region.
The installed capacity, investment cost, and operation cost data of each type of unit in this region in 2021 are summarized in Table 1. At the same time, in order to comprehensively consider the influence of randomness and intermittency of solar power on system capacity configuration, wind speed distribution parameters and solar radiation intensity distribution parameters in the recent 3 years in the region were calculated as samples, and then the monthly unit value of predicted wind power and photovoltaic power generation power within a year was calculated through probability distribution, as shown in Figure 2.
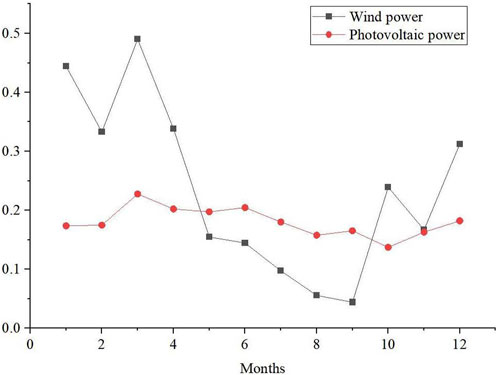
FIGURE 2. From January to December, the standard value of wind power and photovoltaic power generation is predicted in the region.
5.2 Result analysis
Hydropower is the main source of power generation in this region, and its cost is advantageous. Therefore, in this example, hydropower is planned first while ensuring the balance of the system. Meanwhile, in order to verify the influence of carbon emission and renewable energy constraints on the planning of new power systems, three scenarios are set up in this section for comparative analysis:
Low scenario: continue the current development policy focusing on hydropower and vigorously develop hydropower. In this case, carbon target and carbon emission are not considered; that is, Eqs 12–21 are taken as constraint conditions to optimize the capacity allocation scheme of the power system, and there is no restriction on the development of thermal power. Wind power and photovoltaic have shown moderate growth with policy support, with the permeability constraints of wind power and photovoltaic increasing linearly from 4% and 1% to 15% and 18%, respectively, during 2021–2035. Due to the orderly growth of wind power and photovoltaic, their investment costs gradually decreased, with a decline rate of 3% and 2% in 5 years, respectively.
Middle scenario: orderly development of hydropower and active development of scenery and other new energy power generation. In this case, carbon target and carbon emission constraints are added; that is, Eqs 12–23 are used as constraint conditions to solve the operation condition of landscape hydrothermal power generation in this region. Thermal power will be gradually reduced from 2021 to 2035, and new energy generation such as wind and scenery will be actively developed when hydropower is saturated. Other parameters are the same in the lower scenario.
High scene: vigorously develop scenery and other new energy power generation. In this scenario, thermal power is gradually reduced, hydropower is saturated, and wind power and photovoltaic are vigorously developed to meet the load demand. The permeability of wind power and photovoltaic will increase from 9% and 10%– to 33% and 34%, respectively, during 2021–2035. Due to the intermittency and volatility of wind power and photovoltaic, energy storage (including pumping and storage) should be developed as a regulation measure in addition to the system regulation capacity. In this case, the pumped storage energy storage cost remains unchanged, and the investment cost of battery energy storage decreases by 3% in 5 years. In addition, due to the vigorous development of wind power and photovoltaic, their investment costs in 5 years will be 5% and 4%, respectively.
Through MATLAB programming, the YALMIP toolkit and Gurobi solver are used to solve the large-scale linear programming problem. The planning and operation results of the new power system are obtained through the optimization solution, as shown in Figures 3, 4.
(1) Low scenario
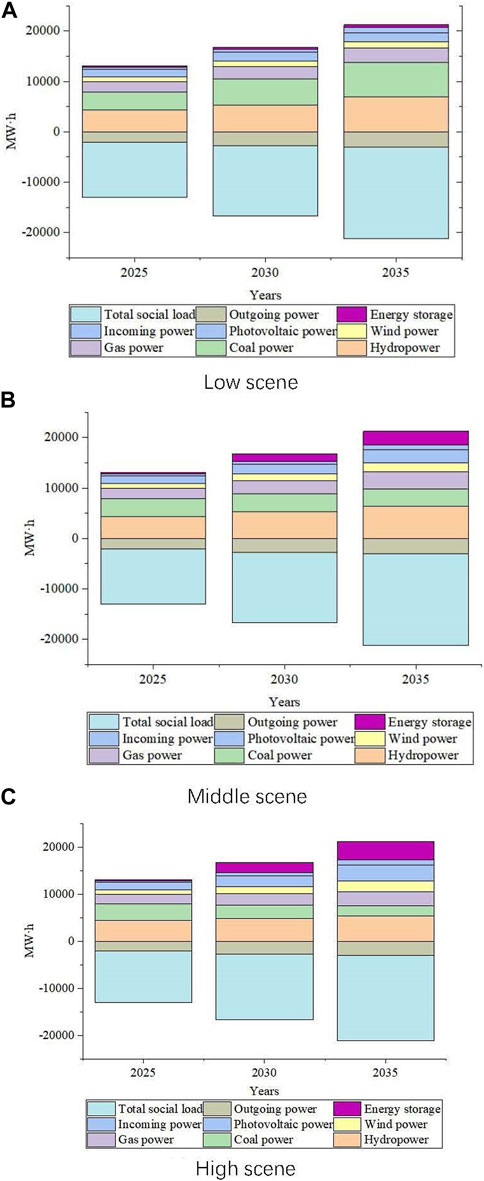
FIGURE 4. Planning and operation results of new power system in different scenarios at different stages.
From the low scenario, it can be seen that in the continuation of the current development plan dominated by hydropower, hydropower will further strengthen its dominant position by 2035, which is estimated to account for 77.72% of the installed capacity in 2021. In the current installed capacity, without the need to consider the case because of carbon constraints, thermal power has a great advantage and will develop rapidly. In this case, wind power generation and photovoltaic power generation have no cost advantage and only grow gradually according to the lower limit of installed capacity. In this case, both wind and light abandonment rates are 0. It shows that in the process of energy transformation, the region also takes into account the goal of safe, reliable, and sustainable development of the power system. While promoting clean energy power generation such as wind power and photovoltaic power generation, it also continues to develop coal power and gas power in an orderly manner to ensure the stable supply of the power system. In addition to the development of traditional energy sources, the region also makes full use of regional resource advantages to develop energy storage, mainly pumped storage, to ensure the full consumption of clean energy. At the same time, the region is vigorously building outbound and inbound channels to enhance the trans-regional transmission capacity of the power grid and improve the economic benefits of electricity.
In order to better protect the safety of users, the district has taken measures in several aspects. First, the power system’s monitoring and warning capabilities have been significantly improved. Through the construction of an efficient and intelligent power grid monitoring system, it can monitor the running status and changes in the power grid in real time, warn of risks, deal with faults in time, and ensure the smooth operation of the power system. Second, the maintenance and upgrading of the power system have been strengthened. For the weak links in the power system, such as line aging, cable joints, and substation equipment, regular inspection and maintenance and timely updating and upgradation of equipment are required to ensure the safety and stability of equipment operation. In addition, a series of technical means such as distribution automation technology and peak-regulating energy storage technology have been adopted to improve the operation efficiency and safety of the power system.
The participation and cooperation of users is also crucial in ensuring the safety of their use of electricity. In the popularization of clean energy, it is also necessary to educate and publicize users, improve their knowledge and understanding of the power system, and promote their active participation in energy conservation, consumption reduction, low-carbon environmental protection, and other aspects. In addition, it is also necessary to establish a sound user service mechanism, improve user service level, timely respond to user demand and feedback, and ensure the quality and safety of user electricity.
In short, ensuring the safety of users is the top priority of power system operation in low scenarios. The region has adopted a variety of measures to enhance the safety and reliability of power systems while also requiring the active participation and cooperation of users. We believe that with the joint efforts of all parties, we will be able to achieve the goal of transforming from high carbon emissions to low carbon emissions and make due contributions to environmental protection and carbon emission reduction.
(2) Middle situation
As can be seen from the scene, due to the abundant hydropower resources in this region, hydropower will always occupy the dominant position during 2025–2035, but its proportion will gradually decrease. The installed capacity of wind power and photovoltaic in the region will steadily increase. It is expected that by 2025, 2030, and 2035, the total installed capacity of scenery will reach 32.49 million kW, 62 million kW, and 80 million kW, respectively, accounting for 18.84%, 28.28%, and 31.63% of the total installed capacity, respectively. This means that clean energy will continue to grow in the coming years and gradually become one of the major energy sources in the region.
At the same time, the installed capacity of coal power is increasing gradually, while the installed capacity of gas power is increasing rapidly. The slow growth of coal power and the fast growth of gas power reflect the trend of energy structure adjustment in this region. With the development and application of clean energy, the proportion of coal power in the power system will gradually decrease, while clean energy such as natural gas will gradually occupy a larger market share. At the same time, the stock of coal electricity will continue to play its supporting role in the power system to ensure the stable operation of the power system.
In view of the instability of wind power and photovoltaic power generation, energy storage technology will be widely used in the power system. Energy storage technology can convert electricity into other forms of energy storage so that it can be released when needed. With the development of energy storage technology and the reduction in cost, battery energy storage, super capacitor, compressed air energy storage, and other technologies have been widely used in the power system. It is expected that the installed capacity of energy storage will increase rapidly to support the stable operation of the power system.
In addition to energy storage technology, the construction of provincial access channel is also one of the important directions of power system optimization in this region. As an important energy dispatching channel in the power system, the provincial channel is a high-voltage power transmission line that crosses the regional boundary. The construction of provincial access can expand the scope of power supply, optimize the structure of the power system, and improve the power supply capacity and reliability of the power system. It is expected that in the next few years, the capacity of the provincial access channel will grow rapidly to cope with the consumption demand of clean energy.
In general, in the medium scenario, the development of the power system in this region in the next few years will be dominated by clean energy, supported by energy storage technology and the construction of the provincial access channel, and assisted by the optimization and adjustment of coal and gas electricity to ensure the supply capacity and reliability of the power system so as to achieve sustainable and efficient energy utilization.
(3) High scene
As can be seen from the high scenario, with the saturation of hydropower and the gradual withdrawal of thermal power, in order to meet the load demand, we choose to vigorously develop the power system dominated by wind power and photovoltaic power. From 2025 to 2035, the installed capacity of wind power and photovoltaic power will grow rapidly, and the installed proportion of new energy will gradually increase, which is one of the important measures in the transformation and upgrading process of the regional power system. As the installed capacity of renewable energy continues to expand, the construction of new power systems is progressing steadily, and the installed hydropower is also developing in an orderly manner, forming a renewable energy system dominated by hydropower, which will provide a more sustainable and clean electricity supply for the region and promote the achievement of global emission reduction targets.
While the installed capacity of new energy is growing rapidly, the installed capacity of coal power is steadily decreasing, gas power is growing rapidly, and total carbon dioxide emissions are under strict control. This not only promoted the technical upgrading of coal power units, reduced coal consumption of thermal power units, promoted the scientific and safe transformation of coal power units after their service term into emergency backup and peak-regulating power sources but also optimized the thermal power structure and supported the safe operation of the power grid. In addition, the rapid growth of energy storage technology, the continuous improvement of the capacity of provincial access channels, the enhancement of clean power transmission capacity, and the increasingly perfect interconnection and mutual economy of the power grid have improved the capacity of the new power system in the region to accept and absorb clean energy.
Although the rapid connection of new energy has promoted the large-scale development of clean energy, it has also brought new challenges to the power balance and safe and stable operation of the local power grid. To address these challenges, the region’s power system has implemented a series of measures, including the construction of standby peak-regulating units, optimization of grid planning and management, and construction of smart grids and smart energy storage systems. These measures effectively promote the stable operation of the power system and the security of users.
In conclusion, the region’s power system has made remarkable progress in the construction of new power systems, the rapid growth of new energy installed capacity, and the consumption of clean energy while also facing a series of new challenges. Through continuous technological upgrading, scientific and rational planning and policies, and enhanced intelligent management, the power system in the region will further improve its safety and reliability, ensure the safety of users, and make positive contributions to sustainable development.
6 Discussion and analysis
The use of a two-layer planning model can have a powerful impact on the decision-making of the development planning of new power systems.
The model can simulate the long-term evolution of the future power system planning and operation in practical application and calculate the quantitative data of the development pattern of the new power system at various stages. Taking a region in Southwest China as an example, the two-layer planning model is used to obtain the capacity allocation planning decisions for the region’s power system in the next 15 years: the low scenario emphasizes on guaranteeing the safety of electricity consumption by users; the medium scenario emphasizes on the development of the power system in the next few years with the dominance of clean energy; and the high scenario emphasizes on the further enhancement of safety and reliability, which will contribute to the sustainable development, and the obtained results provide the guidance for the region’s next step of development. The model used in this paper is applicable to all countries and regions around the world that have a similar power system structure to the one in this paper and is universal; however, for countries or regions that have not yet reached the stage of power system development in this paper or are unable to do so because of resource distribution, they need to make improvements and modifications based on this model.
7 Conclusion
In this paper, a new power system development planning model based on double-layer programming is proposed, and it is applied to a case in Southwest China, and the capacity allocation scheme of the power system in the next 15 years is obtained, and the corresponding analysis is carried out. The results show that the proposed model can simulate the long-term evolution of the future power system planning and operation and provide the quantitative data of the development pattern of the new power system at each stage. The results explain the capacity allocation scheme of the new power system in different situations, enable the comparison of evolution results between different scenarios, reflect the value of various technologies under different development priorities, and provide a certain reference for the follow-up technology research and development and long-term energy policy.
Data availability statement
The original contributions presented in the study are included in the article/Supplementary Material; further inquiries can be directed to the corresponding author.
Author contributions
LF: experiment and writing–original draft. XK: experiment and methodology. LY: experiment and methodology. XW and MR: supervision and writing–review and editing. SY, LC, and CW: supervision and writing–review and editing. All authors contributed to the article and approved the submitted version.
Funding
This work was financially supported by State Grid Sichuan Electric Power Company (Research on the theoretical method of constructing the new power system in Sichuan under the “carbon peaking and carbon neutrality” goal, SGSCJY00NYJS2200058).
Conflict of interest
The authors declare that the research was conducted in the absence of any commercial or financial relationships that could be construed as a potential conflict of interest.
The authors declare that this study received funding from State Grid Sichuan Electric Power Company. The funder had the following involvement in the study: data collection and analysis.
Publisher’s note
All claims expressed in this article are solely those of the authors and do not necessarily represent those of their affiliated organizations, or those of the publisher, the editors, and the reviewers. Any product that may be evaluated in this article, or claim that may be made by its manufacturer, is not guaranteed or endorsed by the publisher.
Supplementary material
The Supplementary Material for this article can be found online at: https://www.frontiersin.org/articles/10.3389/fenrg.2023.1221555/full#supplementary-material
References
Cai, R., Zhu, H., Li, W., Xiao, Y., and Liu, Z. (2022). Development path of energy science and technology under “dual carbon” goals: perspective of multi-energy system integration. Bull. Chin. Acad. Sci. Chin. Version) 37 (4), 502–510.
Chen, W., Xiang, Y., Peng, G., Liu, Y., and Liu, J. (2021b). Dynamic modeling and analysis of power system supply-side morphological development under "two-carbon" target. J. Shanghai Jiaot. Univ. 55 (12), 1567–1576. doi:10.16183/j.cnki.jsjtu.2021.294
Chen, X., Liu, Y., Wang, Q., Lv, J., Wen, J., et al. (2021a). Pathway toward carbon-neutral electrical systems in China by mid-century with negative CO2 abatement costs informed by high-resolution modeling. Joule 5 (10), 2715–2741. doi:10.1016/j.joule.2021.10.006
Dallmann, C., Schmidt, M., and Möst, D. (2022). Between path dependencies and renewable energy potentials: a case study of the Egyptian power system. Energy Strategy Rev. 41, 100848. doi:10.1016/j.esr.2022.100848
Feng, W. Z., and Li, L. (2022). Research and practice on development path of low-carbon, zero-carbon and negative carbon transformation of coal-fired power units under “double carbon” targets. Power Gener. Technol. 43 (3), 452.
Hamidpour, H., Aghaei, J., Pirouzi, S., Niknam, T., Nikoobakht, A., Lehtonen, M., et al. (2022). Coordinated expansion planning problem considering wind farms, energy storage systems and demand response. Energy 239, 122321. doi:10.1016/j.energy.2021.122321
He, G., Lin, J., Sifuentes, F., Liu, X., Abhyankar, N., and Phadke, A. (2020). Rapid cost decrease of renewables and storage accelerates the decarbonization of China’s power system. Nat. Commun. 11 (1), 2486. doi:10.1038/s41467-020-16184-x
Kabeyi, M. J. B., and Olanrewaju, O. A. (2022). Sustainable energy transition for renewable and low carbon grid electricity generation and supply. Front. Energy Res. 9. doi:10.3389/fenrg.2021.743114
Li, H., Liu, D., and Yao, D. Y. (2021a). Analysis and reflection on the development of power system towards the goal of carbon emission peak and carbon neutrality. Proc. CSEE 41 (18), 6245–6259. doi:10.13334/j.0258-8013.pcsee.210050
Li, W., Zhao, L., Bo, Y., Wang, W., Wang, M., Liu, S., et al. (2021b). Robust transmission expansion planning model considering multiple uncertainties and active load. Glob. Energy Interconnect. 4 (5), 476–484. doi:10.1016/j.gloei.2021.11.009
Li, Y., Dai, M., Hao, S., Qiu, G., Li, G., Xiao, G., et al. (2021c). Optimal generation expansion planning model of a combined thermal–wind–PV power system considering multiple boundary conditions: a case study in Xinjiang, China. Energy Rep. 7, 515–522. doi:10.1016/j.egyr.2021.01.020
Lu, Z. X., Hao, L., and Qiao, Y. (2021). Morphological evolution of power systems with high share of renewable energy generations from the perspective of flexibility balance. J. Glob. Energy Interconnect. 4 (01), 12–18. doi:10.19705/j.cnki.issn2096-5125.2021.01.003
Lu, Z. X., Huang, H., and Shan, B. G. (2017). Structure and form evolution of power system with high proportion of renewable energy and power forecast prospect. Automation Electr. Power Syst. 41 (09), 12–18.
Moradi-Sepahvand, M., and Amraee, T. (2021). Integrated expansion planning of electric energy generation, transmission, and storage for handling high shares of wind and solar power generation. Appl. Energy 298, 117137. doi:10.1016/j.apenergy.2021.117137
Quiroga, D., Sauma, E., and Pozo, D. (2019). Power system expansion planning under global and local emission mitigation policies. Appl. Energy 239, 1250–1264. doi:10.1016/j.apenergy.2019.02.001
Shu, Y., Zhang, L., Zhang, Y., Wang, Y., Lu, G., Yuan, B., et al. (2021). Carbon peak and carbon neutrality path for China’s power industry. Strategic Study Chin. Acad. Eng. 23 (6), 1–14. doi:10.15302/j-sscae-2021.06.001
Wang, P., Du, E., Zhang, N., Xu, X., and Gao, Y. (2021b). Power system planning with high renewable energy penetration considering demand response. Glob. Energy Interconnect. 4 (1), 69–80. doi:10.1016/j.gloei.2021.03.007
Wang, Y., Zhang, X., Qiao, Y., Wu, J., Yang, Y., Bai, W., et al. (2021a). “Development status of advanced thermal power technology and low-carbon path of China's thermal power industry,” in 2021 IEEE sustainable power and energy conference (iSPEC) (IEEE), 2049–2066.
Wei, C., Cong, M., Wang, R., Zhao, Y., Zhou, Z., Yang, D., et al. (2023). Optimization of district heating system considering carbon taxes and subsidies based on energy policy stage goals. Appl. Therm. Eng. 226, 120295. doi:10.1016/j.applthermaleng.2023.120295
Wei, H. Y., Zhuo, Z. Y., and Zhang, N. (2022). Carbon peaking · Carbon neutral transition path optimization and influencing factors analysis in China's power system. Automation Electr. Power Syst. 46 (19), 12.
Wyrwa, A., Suwała, W., Pluta, M., Raczyński, M., Zyśk, J., and Tokarski, S. (2022). A new approach for coupling the short-and long-term planning models to design a pathway to carbon neutrality in a coal-based power system. Energy 239, 122438. doi:10.1016/j.energy.2021.122438
Xie, Y. X., Zhang, X. M., and Luo, J. S. (2018). Evolution model for future power system under massive penetration of renewable energy. Proc. CSEE 38 (02), 421–430+673. doi:10.13334/j.0258-8013.pcsee.172257
Yang, H. T., Ji, P., and Miao, M. (2018). An analysis of the relationship between the structure form and power supply composition of China's UHV power grid in the future. Automation Electr. Power Syst. 42 (6), 9.
Zhang, J. P., Zhou, Q., and Wang, D. M. (2021). Research on the development path of new power system to achieve carbon peaking and carbon neutrality. Integr. Intell. Energy 43 (12), 46–51.
Zhang, Z. G., and Kang, C. Q. (2022). Challenges and prospects for constructing the new-type power system towards a carbon neutrality future. Proc. CSEE 42 (08), 2806–2819. doi:10.13334/j.0258-8013.pcsee.220467
Zhou, J. P., Du, X. H., and Zhou, X. B. (2023). Study on the role and development planning of hydropower in new power system [J/OL]. J. Hydroelectr. Eng., 1-12. Available at: http://kns.cnki.net/kcms/detail/11.2241.TV.20220530.1446.002.html.
Keywords: new power systems, two-tier planning, new energy sources, capacity allocation, power system planning
Citation: Fang L, Ke X, Yang L, Weiding X, Ruiguang M, Tiannan M, Yunche S, Chang L and Wei C (2024) Research on a new power system development planning model based on two-tier planning. Front. Energy Res. 11:1221555. doi: 10.3389/fenrg.2023.1221555
Received: 12 May 2023; Accepted: 04 December 2023;
Published: 03 January 2024.
Edited by:
Kunjie Yu, Zhengzhou University, ChinaCopyright © 2024 Fang, Ke, Yang, Weiding, Ruiguang, Tiannan, Yunche, Chang and Wei. This is an open-access article distributed under the terms of the Creative Commons Attribution License (CC BY). The use, distribution or reproduction in other forums is permitted, provided the original author(s) and the copyright owner(s) are credited and that the original publication in this journal is cited, in accordance with accepted academic practice. No use, distribution or reproduction is permitted which does not comply with these terms.
*Correspondence: Liu Fang, email@uni.edu