A Review of Gassy Sediments: Mechanical Property, Disaster Simulation and In-Situ Test
- 1College of Environmental Science and Engineering, Ocean University of China, Qingdao, China
- 2Shandong Provincial Key Laboratory of Marine Environment and Geological Engineering, Ocean University of China, Qingdao, China
- 3Laboratory for Marine Geology, Pilot National Laboratory for Marine Science and Technology, Qingdao, China
Gassy sediments are an important cause of engineering disasters such as large-area coastal submarine landslides, excessive tilting of marine foundations, and excessive deformation of tunnels. Under different stress paths, the gassy soil exhibits different microstructure changes and mechanical responses. This paper introduces the current research status regarding the mechanical responses, numerical simulation and the in-situ test methods of gassy sediment. In terms of mechanical responses, it summarized the strength and deformation characteristics of gassy soil under different stress paths, tracking the study on constitutive model. The disaster simulation work using constitutive model of gassy sediment is introduced. It also analyzes the advantages and limitations of various methods in the in-situ test. It can provide theoretical support for further study on disaster prevention and geological risk assessment of gassy sediments.
Introduction
Gassy sediments are widely distributed in the coastal areas of all the world’s continents (Figure 1) (P et al., 2001). The gases are usually formed by microbial degradation of organic matter under anaerobic conditions, thermogenic methanogenesis, or volcanic eruptions. Their main components include methane, carbon dioxide, and nitrogen (Figure 2). The gases mostly exist in the pores as dissolved or discrete bubbles (Wheeler, 1986; Grozic et al., 2000; Sánchez et al., 2017).
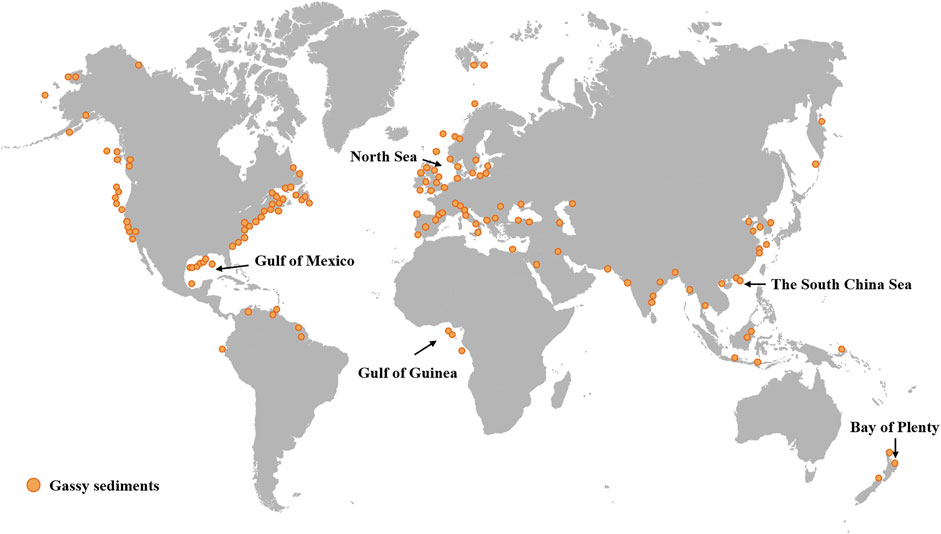
FIGURE 1. Distribution of marine gassy sediments in the five continents (Fleischer et al., 2001).
Unlike unsaturated soil, the saturation of gassy soil is usually in the range of 85–90%, and the gas phase usually exists in the form of discrete bubbles while the water phase is in a connected state. The size of the soil particles determines the microstructure of gassy soils, and gassy soils are mainly divided into two categories according to the size of the air bubbles (Wheeler, 1988b; Sills and Wheeler, 1992). In one category, the air bubbles are smaller than the soil particles and pore size. The air bubbles are discrete in the soil pores, which only changes the compressibility of the pore fluid and does not affect the soil structure (Wang et al., 2018; Hong et al., 2021b; Xu et al., 2022). In the other category, the air bubbles are much larger than the soil particles and pore size. The bubbles rearrange the soil particles and thus affect the soil structure (Hong et al., 2020a; Gao et al., 2020). This microstructure often exists in fine-grained air-bearing soils, as shown in Figure 3. Hong et al. (2017) and Guo et al. (2021) observed the microstructure of typical fine-grained gassy soil by scanning electron microscopy and computed tomography. The results are shown in Figure 4. The undissolved gas exists as large discrete bubbles in the saturated matrix, and the bubbles are much larger than the soil particles and pores.
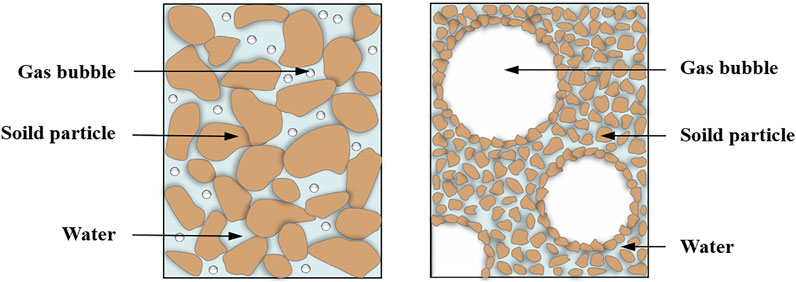
FIGURE 3. Types of soil containing air bubbles (Wheeler, 1988b).
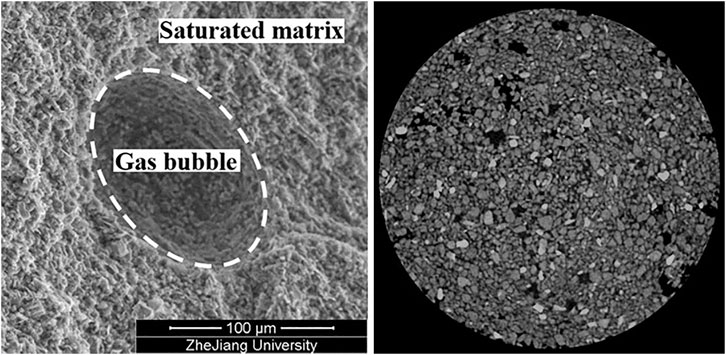
FIGURE 4. Microscopic scan of a typical gassy soil (Hong et al., 2017; Guo et al., 2021).
Due to the structural characteristics of gassy soil, the mechanical properties are considerably different from those of saturated soils and unsaturated soils. Kaminski et al. (2020) summarized the undrained shear strength of gassy soils under different conditions. The mechanical properties of gassy soils under different conditions change the engineering characteristics of the corresponding soil layers. During construction engineering, geological disasters have often been induced by the presence of shallow gas, and some major engineering accidents have occurred, causing serious economic losses (Sobkowicz and Morgenstern, 1984; Rad et al., 1994; Rowe et al., 2002; Kortekaas and Peuchen, 2008; Sultan et al., 2012; Xu et al., 2017; Rowe and Mabrouk, 2018; Jommi et al., 2019).
Experimental Testing
In the gas-bearing soil test, due to the release of pressure caused by deep-water sampling, the gas in the soil is dissolved and expanded, which causes changes in the soil structure and even cracks (Zhang and Lunne, 2003; Sultan et al., 2012). It is therefore difficult to obtain undisturbed gassy soil samples under in-situ conditions. Previous experiments have mainly used remolded gassy soil samples. There are three main preparation methods: the zeolite molecular sieve technique (Sills et al., 1991; Hong et al., 2017; Hong et al., 2020b) the axis-translation technique (Sultan et al., 2012; Wang et al., 2018; Blouin et al., 2019), and the biological anaerobic fermentation technique (Sills and Gonzalez, 2001; Hu, 2010).
The zeolite molecular sieve technique replaces the adsorbed gas by adding the zeolite-absorbing gas into the slurry. This method is simple and effective while the stress history is known. In this method, the samples can be prepared in batches with similar physical properties under in-situ conditions. The main principle is shown in Figure 5A. The gas content of the prepared samples cannot be accurately controlled, but the bubble distribution is relatively uniform.
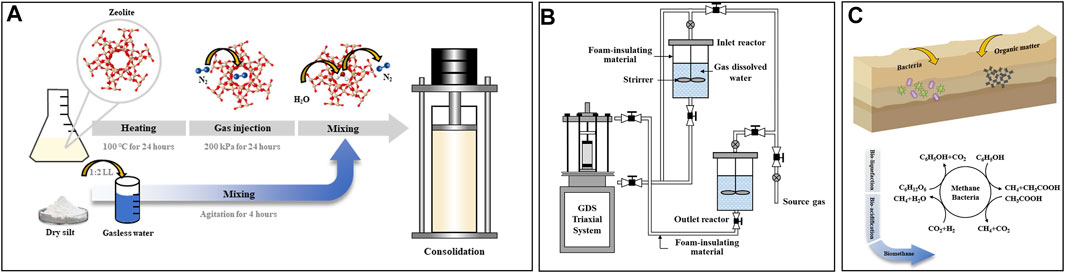
FIGURE 5. Gassy specimen preparation methods: (A) the zeolite molecular sieve technique; (B) the axis-translation technique; (C) the biological anaerobic fermentation method.
The axis-translation technique involves replacing the internal pore water by circulating the dissolved gas water through the saturated sample and releasing the gas by unloading to form the gassy soil sample. The test system is shown in Figure 5B. This method is mainly applicable to coarse-grained soil, and the gas-charging effect of fine-grained soil is poor.
The principle of the biological anaerobic fermentation technique is that organic matter is decomposed by anaerobic bacteria to release methane and carbon dioxide, as shown in Figure 5C. Its mechanism is closest to the formation process of bubbles in the marine environment. The preparation process, however, requires certain values for temperature, pH, redox potential, and other parameters. Only by satisfying these biological requirements will the fermentation cycle be short and the gas production suitable.
Both zeolite molecular sieve technique and axis-translation technique can prepare uniform samples for triaxial test. zeolite molecular sieve technique is used for fine-grained gassy soil, which can mimic the natural formation process of free bubbles in soil. Axis-translation technique is used to prepare coarse-grained gassy soil, which can mimic the process of gas exsolution due to stress release. Although the bubble formation process of the biological anaerobic fermentation technique is the closest in the marine environment, its sample preparation method is complex and immature, so it cannot be used for triaxial test to determine the mechanical properties. It can be used for model box test, and it needs further research. The zeolite molecular sieve technique and axis-translation technique can produce specimens containing a uniform distribution of gas bubbles, but the gas saturation of gassy soil cannot be measured specifically. Therefore, the double-cell system can be installed in triaxial apparatus to measure the change of gas saturation. The accuracy of the volumetric system can reach 31.4 mm3 (equivalent to 0.04% volumetric strain of the soil sample with a diameter of 38 mm and a height of 76 mm) (Ng et al., 2002). Figure 6 shows the structural diagram.
Mechanical Behavior of Gassy Soil
On the basis of the soil microstructure, many researchers have analyzed the mechanical properties and discussed the relationship between those properties and the microscopic mechanism (Nageswaran, 1983; Thomas, 1987; Wheeler, 1988a; Gardner and Goringe, 1988; Sills et al., 1991; Sham, 1992; Hong et al., 2017; Bai, 2018). The bubble-water-soil skeleton microstructure interaction in fine-grained gassy soil includes two mechanisms: bubble flooding and gas intrusion into the saturated matrix (Wheeler, 1988b). The existence of bubbles make the effective stress and void ratio in the saturated matrix unevenly distributed (Sham, 1992). The bubble-water-soil skeleton interaction is affected by the initial pore water pressure (Hong et al., 2017). When the initial pore water pressure is low, the bubbles in the soil are large and the radius of the meniscus at the water–gas interface is large, which means that the water inflow value of the bubble cavity is relatively small. During the undrained shear process, with the increase of the pore water pressure, bubble flooding may occur (the water in the saturated matrix enters the bubble cavity, and partial drainage occurs), resulting in a reduction of the excess pore pressure and an increase in strength, as shown in Figure 7A. When the initial pore water pressure is high, the gas pressure in the soil is also relatively high, which produces micro-cracks in the surrounding saturated matrix. In the process of undrained shear, the micro-cracks may collapse, resulting in reduced strength and increased excess pore pressure, as shown in Figure 7B.
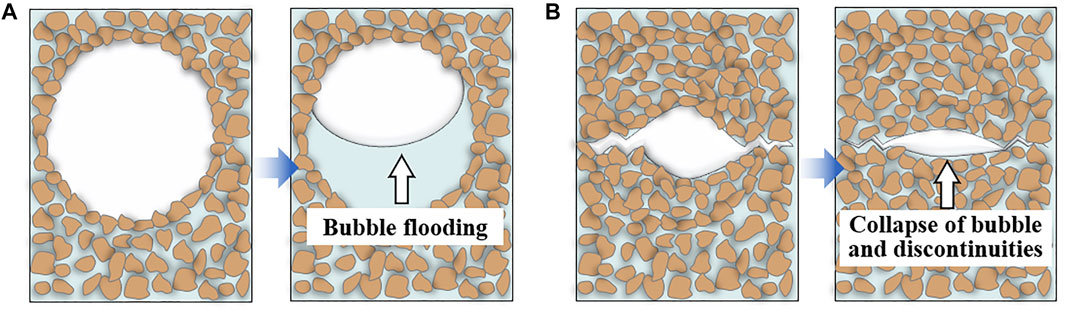
FIGURE 7. Schematic diagrams showing fine-grained gassy soil under different back pressures (Hong et al., 2017): (A) Relatively low initial back pressure; (B) Relatively high initial back pressure.
Bubbles in gassy soil change the compression characteristics of the pore fluid and the structure of the soil, which affects the mechanical properties, such as compression, and the static and dynamic characteristics. A large number of compression, monotonic, and cyclic shear tests of gassy fine-grained soil and gassy sand have been carried out, and the key influencing factors of the modulus, monotonic, and cyclic shear strength of gassy soil have been determined. The results show a clear influence of air content on the elastic shear modulus and shear strength of gassy soil (Duffy et al., 1994; Pietruszczak and Pande, 1996; Mathiroban, 2004; Vega-Posada et al., 2014; Hong et al., 2017). The drainage shear test of sand shows strain hardening, and the strain law of undrained shear is related to the initial state of gassy sand (Amaratunga and Grozic, 2009; Vega-Posada et al., 2014). Under dynamic load, the cyclic stress of gassy sand is linearly related to liquefied vibration times and the increase of the gas content delays the liquefaction of gassy sand (Guan, 2017). The greater the gas content, the slower the pore pressure dissipation and the smaller the amplitude of excess pore water pressure (Han, 2020). The change in the cyclic stiffness of gassy fine-grained soil is related to the initial pore pressure. The high pressure enhances show the stiffness enhancement and the low pressure weakens the stiffness (Hong et al., 2021a). Research into various stress-path problems in actual projects has shown that the deformation of the soil can be reduced by gradual deflation (Wang, 2009). The change direction of the confining pressure is closely related to the stress–strain characteristics of the sample. The increase of the confining pressure induces strain hardening of the sample, and the decrease induces strain softening (Zhong, 2007). The gas evolution and bubble expansion caused by pressure unloading enhances the compressibility of gassy soil, reduces the pre-consolidation pressure and the undrained shear strength. The structural change caused by gas evolution influences the effective stress path (Sultan et al., 2012). The rapid accumulation of gas leads to a rapid increase of the pore water pressure and a reduction of the effective stress of the soil, triggering the liquefaction of gassy soil (Liu, 2018; Kong et al., 2019). The change of temperature and pressure of gassy soil also affects the compressibility and permeability. After the dissipation of excess pore pressure, the consolidation time is prolonged, resulting in long-term settlement and showing a complex consolidation creep process. The lower the saturation is, the more obvious the phenomenon is (Wang, 2021; Zhu et al., 2021).
Researchers have proposed mechanical models for engineering calculations. The fine-grained gassy soil mechanical model is an improvement on the Cambridge model, while the coarse-grained gassy soil mechanical model is used to construct the mechanical analysis model by describing the compressibility of the “bubble-water” mixture. Wheeler and Gardner (1989) regarded soil containing large bubbles as a composite material containing spherical filler. They derived the calculation formulas of the shear modulus and bulk modulus of soil containing large bubbles under drainage and undrained conditions. Grozic J. L. H. et al. (2005) quantitatively simulated the enhancement effect of bubbles on the undrained shear strength of fine-grained gassy soil, but their model cannot describe the strength attenuation caused by bubbles. Hong et al. (2021b) proposed a critical-state model that takes into account both hardening and softening by defining the ratio of the deviatoric stress increment to the plastic shear strain increment. Pietruszczak and Pande (1996) introduced “gas–water interfacial tension” into the pore pressure expression and established the rotational hardening model of gassy sand. The change of pressure in gassy sand causes gas dissolution, and so Grozic J. L. et al. (2005) considered the compressibility and solubility of gas at the same time using Henry’s law and Boyle’s law. By introducing a volume dissolution coefficient h, they deduced the expression of pore pressure:
This model can accurately predict the strain softening but not the strain hardening.
Gao et al. (2020) established a critical-state model that takes into account the influence of the initial pore water pressure and initial air content on the yield and dilatancy characteristics of gassy soil. However, it is unable to predict the undrained unloading response. Sultan et al. (2012) explored the influence of gas evolution on soil in undrained unloading stress paths through triaxial tests. They also introduced the gas-phase damage parameter d (related to gas content) and proposed the following relationship between gassing volume and soil pre-consolidation pressure:
Smith et al. (2022) proposed a model that takes into account the bubble damage effect. The anisotropic yield surface function is defined as
Using the non-associated flow rule, this model can simulate the strength reduction of gassy soil caused by gas evolution.
At present, however, the mechanical models of gassy soil mainly focus on the description of static loading characteristics; the gas-phase damage under static unloading conditions has only been partially considered.
The theory of granular solid hydrodynamics are combined with the temperature motion equation of soil particles (Yang and Bai, 2020):
Yang and Bai (2020) established a thermodynamic model describing the mechanical properties and temperature effect of fine-grained soil containing gas. The results show that the increase of temperature under drainage conditions increases the compression coefficient and the thermal shrinkage coefficient, and the pore water pressure under undrained conditions also increases. The influence of temperature on the undrained shear characteristics depends on the initial conditions.
Vanoudheusden et al. (2003) established a numerical model to describe the mechanical properties of unsaturated expansive soil based on the elastic-plastic model. Under undrained conditions, its characteristics are controlled by the drainage curve of gassy soil (the relationship between saturation and capillary pressure) and the solubility of the gas.
Dilatancy is an important property of the mechanical responses of gassy soil. For coarse-grained gassy soil, bubbles only change the compressibility of the pore fluid, and so the effective stress principle is still applicable to this type of soil (Pietruszczak and Pande, 1996; Grozic J. L. et al., 2005). The study of dilatancy function, plastic modulus, and related material parameters of saturated sand is still relevant for gassy sand (Yin and Chang, 2013; Xiao et al., 2015; Kong et al., 2016; Xiao et al., 2019).
However, there are few studies on the dilatancy of fine-grained gassy soil. The dilatancy function used in the existing constitutive model of gassy soil is mostly the same as that of saturated soil (Pietruszczak and Pande, 1996) and they cannot consider the potential effect of gas bubbles on dilatancy. While the dilatancy function proposed by Hong et al. (2019) introduces the initial pore water pressure and gas volume fraction on the basis of Grozic J. L. et al. (2005). To characterize the influence of gas content on the dilatancy, the relevant material parameters need to be calibrated. The Stress−dilatancy relations assumed in the existing models for fine-grained gassy soil is shown in Table 1.
Numerical Simulation
The existence of gas in the soil layer affects the mechanical properties of the stratum. Thus, in the design of offshore foundations, offshore drilling, and slope stability analysis, a constitutive model that can uniformly describe the response of the gassy soil under different conditions is needed. Current research on fine-grained gassy soil mainly focuses on the unit response in the triaxial test. Few studies have been conducted on practical engineering issues and the constitutive model needs further development (Grozic J. L. H. et al., 2005; Sultan et al., 2012; Hong et al., 2017; Sánchez et al., 2017; Goao and Hong, 2019; Hong et al., 2019; Hong et al., 2020a; Gao et al., 2020). The constitutive model of coarse-grained soil is relatively mature, but the analysis is mostly base on liquefaction and landslide (Grozic, 2003; Atigh and Byrne, 2004; Mabrouk and Rowe, 2011; Hong and Xu, 2020; Thomas, 2021a; Hong et al., 2021b; Thomas, 2021b).
Grozic (2003) introduced the concept of flow potential and proposed a method to evaluate the liquefaction potential of loose sand, which can judge the potential liquefaction area according to the flow potential. The zone of potential liquefaction is shown in Figure 8.
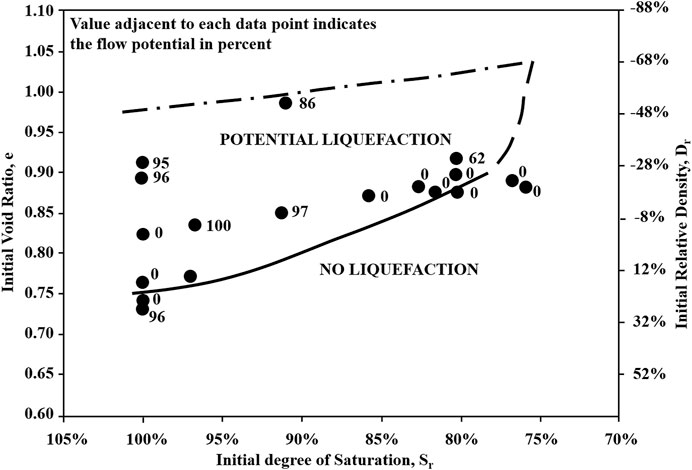
FIGURE 8. The zone of potential liquefaction (Grozic, 2003).
Atigh and Byrne (2004) proposed a liquefied sand flow model to analyze the liquefaction-sliding flow of loose gassy sand caused by tidal changes. Dense sand slopes are more common in real environments, however. Hong et al. (2021b) established a model of coarse-grained gassy soil, quantified the influence of such soil under different initial conditions, and used the model to analyze the stability of submarine slopes under undrained conditions. For loose gassy sand, gas has an enhancing effect on the stability of submarine slopes; for dense gassy sand, it has a weakening effect, as shown in Figure 9.
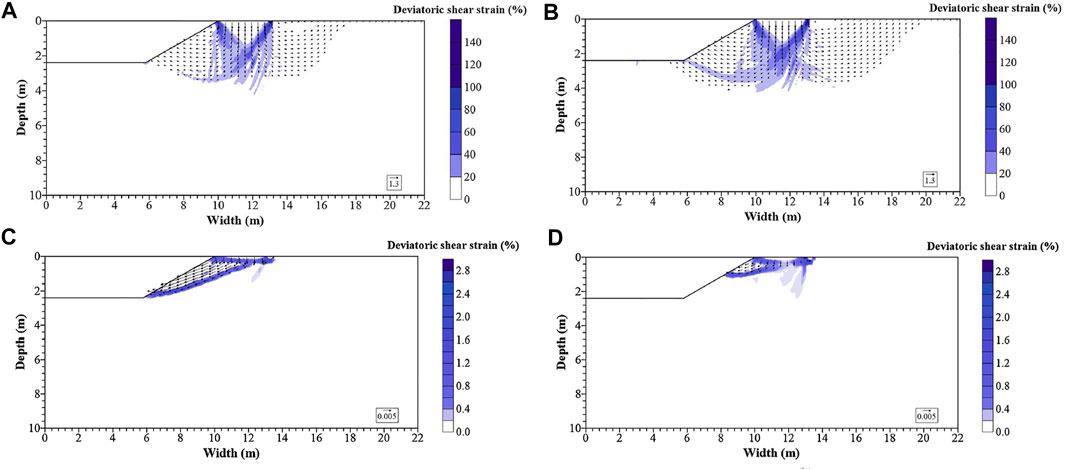
FIGURE 9. Contours of the shear strain and displacement vectors for gassy sand (Hong et al., 2021b): (A) Saturated dense sand; (B) Gassy dense sand; (C) Saturated loose sand; (D) Gassy loose sand.
Hong and Xu (2020) simulated the undrained shear of gassy sand using the discrete element method. They analyzed the influence of different gas solubilities, and this method can be used to effectively simulate the gas dissolution in the process of undrained unloading (Figure 10).
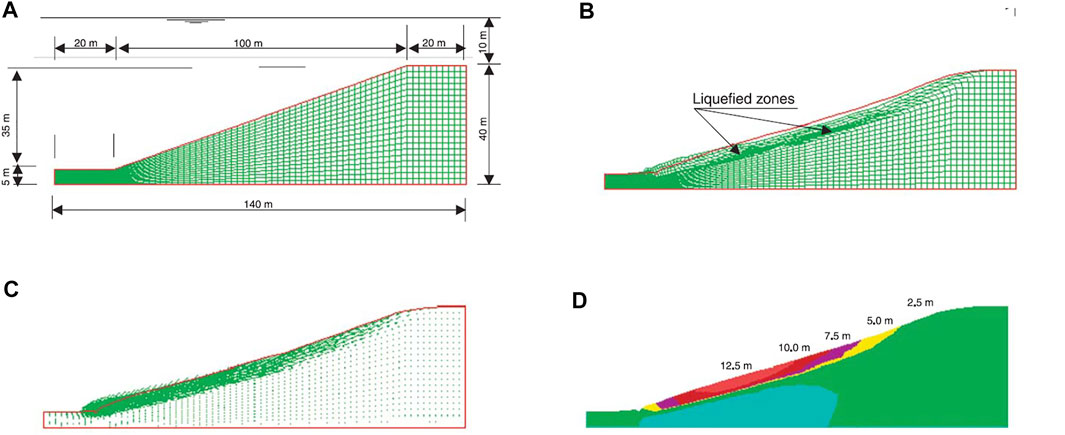
FIGURE 10. Submarine slope of gassy soil (Hong and Xu, 2020): (A) grid used in analysis; (B) deformed mesh during low tide; (C) displacement vectors; (D) horizontal displacement contours (in m).
For fine-grained gassy soil, Thomas (2021a, 2021b) proposed the governing equation of porous elastic gas-bearing soil based on the modified Biot theory to simulate the changes of stress, displacement, and pore water pressure of a gassy seabed with buried pipelines under wave pressure loading. In the buried pipelines, the stress and pore pressure are concentrated, which may lead to local liquefaction.
In-Situ Investigation
In-situ sampling of gassy sediments inevitably causes disturbances. Although temperature-preserving and pressure-holding sampling technology can be used to obtain in-situ gassy sediment samples, the sampling cost is high (Bai and Li, 2010; Zhu et al., 2011; Wu et al., 2022; Zhu et al., 2022). Experiments have therefore mainly been carried out using laboratory gassy soil samples. Moreover, the current sample preparation method for gassy soil cannot effectively reproduce the gas-production process of microbial bacteria in the coastal soft soil layer under in-situ conditions. It is also difficult to realize the batch of gassy soil sample preparation and the quantitative introduction of gas. It is therefore essential to study the in-situ test equipment that can quantitatively detect the gas content in the soil. Accidents involving uncontrolled gas leaks caused by drilling in marine engineering can be avoided by researching the in-situ testing technology of gassy soil. The simulation parameters can be accurately established for numerical calculation.
Rad and Lunne (1994) developed a new type of offshore in-situ testing device, the BAT probe, which is pushed to the required depth to obtain water-air samples in the gassy soil layer. Its structure is shown in Figure 11. After the recovery of the device, the samples are analyzed using a gas chromatograph for gas composition and gas saturation in pore water to assess the possibility of a shallow air bladder in the soil. Hong et al. (2018) also developed a new type of device. After penetration, the device takes horizontal samples, performs in-situ sonic testing, and determines the gas content by in-situ compression to minimize the influence of sampling disturbance.
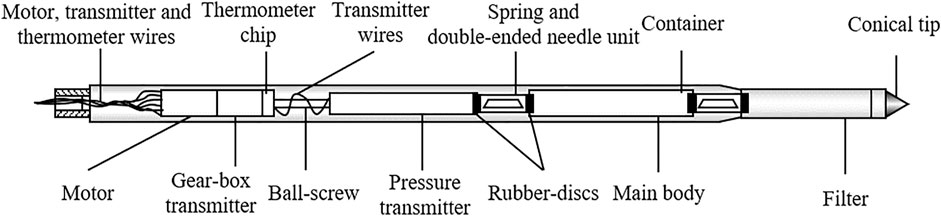
FIGURE 11. BAT probe (Rad and Lunne, 1994).
Since the BAT probe can only be used for in-situ water-gas sample testing and has a single function, recent research has mainly used the geophysical prospecting measurements to detect the source layer, reservoir and burial depth of gassy soil (Figure 12A). The gassy soil can be identified as shown in Figure 12B. The shear wave seismic section can delineate the boundary of the gassy sand layer (Wood et al., 2000; Pruessmann et al., 2004; Reeves, 2005; Lin et al., 2006; Zheng et al., 2006; Li et al., 2009). In addition, the change of resistivity can be used to judge the gassy layer by the electromagnetic exploration method, allowing the distribution range to be delineated (Lee and Collett, 2006; Li et al., 2007; Pezard et al., 2015). The irregular and robust reflection interface of the gassy formation can be formed by the shallow stratum cross-section method using sound-wave propagation and scattering (Mustafa et al., 2002; Wang et al., 2013; Tóth et al., 2014; Janiewicz et al., 2019).
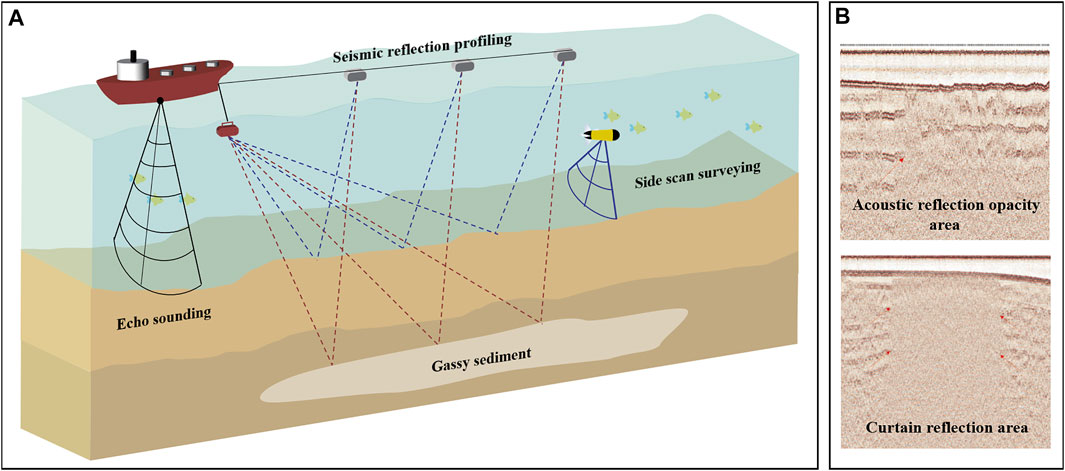
FIGURE 12. Geophysical prospecting: (A) methods; (B) Sub-bottom profile of gassy sediment (Xing et al., 2017).
The cone penetration test (CPT) can be used to carry out a variety of test methods, and as the scope of the investigation is extensive, CPT can be used to identify the gassy layer (Wang et al., 2019). Guo et al. (2007) found that CPT can also preliminarily determine whether biogas is present in sand. When the tip resistance increases, the friction–resistance ratio decreases, the fluctuation amplitude of the pore water pressure remains small, and there is no negative excess pore water pressure during the penetration process. It can thus be determined preliminarily that there may be shallow biogas in the sand layer.
Li et al. (2009) improved the CPT and separated the probe and probe rod to realize gas sampling and pressure measurement. Lai et al. (2016) invented a Membrane Interface Probe and Cone Penetration Technology that decomposes and passes organic matter by adding a MIP film. It uses gas chromatography to determine the organic matter’s phase state and content.
CPT with different probes can be used to detect different physical properties of sediments, such as gas occurrence, temperature gradient, acoustic characteristics, and chemical anomalies. Detecting these characteristics can improve the current conventional in-situ measurements in terms of the identification accuracy of gassy soils. Thus, CPT has a wide application prospect in gassy soil exploration.
Research Prospects
Due to its mechanical properties, gassy sedments are an important cause of engineering disasters. The researches mainly focus on the mechanical response and development of constitutive model. From this summary of the current research, the author believes that future research on gassy soils should explore the following aspects:
1) Mechanical responses of gassy soils with different stress paths. Combined with the actual engineering or disaster, based on the soil stress path under the actual conditions of gassy soil shield, waves, landslides etc., research could be conducted into mechanics and deformation mechanism to achieve accurate prediction;
2) The constitutive model of fine-grained gassy soil. The numerical simulation of coarse-grained gassy soil is relatively mature, whereas the simulation of fine-grained gassy soil has mainly focused on the unit scale, and there is no mature constitutive model for practical engineering problems;
3) The bubble-migration mechanism in gassy soil. Under real conditions, due to soil faults and cracks, discrete bubbles may migrate, accumulate, and be released. The relationship between the triggering conditions of bubble migration and gassy soil mechanical behaviour needs to be furthur research.
4) Effect of microstructure of bubbles in gassy soil. The change of bubbles in the mechanical test can be observed by means of microscopic scanning device to clarify microcosmic mechanism.
5) Risk assessment of geological disasters such as liquefaction, landslides, and the settlement of gassy soil. Based on the research into the deformation mechanism of gassy soil under different conditions, engineers should carry out site risk assessments and implement disaster-prevention and mitigation measures;
6) In-situ measurements of gassy soil. At present, the in-situ measurements for gassy soils are mainly the gassy soil distribution range detection. It is necessary to improve the accuracy of the in-situ parameters of gassy soils to improve the accuracy of research into constitutive models.
Author Contributions
TL, XY, and YZ contributed to conception and design of the study. TL wrote the first draft of the manuscript. XY and YZ wrote sections of the manuscript. All authors contributed to manuscript revision, read, and approved the submitted version”.
Funding
The study is supported by the National Natural Science Foundation of China (No. U2006213) and the Fundamental Research Funds for the Central Universities (201962011).
Conflict of Interest
The authors declare that the research was conducted in the absence of any commercial or financial relationships that could be construed as a potential conflict of interest.
Publisher’s Note
All claims expressed in this article are solely those of the authors and do not necessarily represent those of their affiliated organizations, or those of the publisher, the editors and the reviewers. Any product that may be evaluated in this article, or claim that may be made by its manufacturer, is not guaranteed or endorsed by the publisher.
References
Amaratunga, A., and Grozic, J. L. H. (2009). On the Undrained Unloading Behaviour of Gassy Sands. Can. Geotech. J. 46, 1267–1276. doi:10.1139/t09-056
Atigh, E., and Byrne, P. M. (2004). Liquefaction Flow of Submarine Slopes under Partially Undrained Conditions: an Effective Stress Approach. Can. Geotech. J. 41, 154–165. doi:10.1139/t03-079
Bai, C. (2018). Evaluation of Uniformity and Preliminary Mechanical Properties of Simulated Samples of Soft Soil Containing Bubbles. Tianjiaan, Huainan, Anhui, China: AnHui University of Science and Technology.
Bai, Y., and Li, Q. (2010). Progress on Natural Gas Hydrate Sampling Techniques and Tools. Pet. Drill. Tech. 038, 116. doi:10.3969/j.issn.1001-0890.2010.06.026
Blouin, A., Sultan, N., Callot, J-P., and Imbertb, P. (2019). Sediment Damage Caused by Gas Exsolution: A Key Mechanism for Mud Volcano Formation. Eng. Geol. 263, 105313. doi:10.1016/j.enggeo.2019.105313
Duffy, S. M., Wheeler, S. J., and Bennell, J. D. (1994). Shear Modulus of Kaolin Containing Methane Bubbles. J. Geotech. Engrg. 120, 781–796. doi:10.1061/(asce)0733-9410(1994)120:5(781)
Fleischer, P., Orsi, T., Richardson, M., and Anderson, A. (2001). Distribution of Free Gas in Marine Sediments: a Global Overview. Geo-Marine Lett. 21, 103–122. doi:10.1007/s003670100072
Gao, Z., and Hong, Y. (2019). Constitutive Modelling of Gassy Clay. E3S Web Conf. 92, 15005. doi:10.1051/e3sconf/20199215005
Gao, Z., Hong, Y., and Wang, L. (2020). Constitutive Modelling of Fine‐grained Gassy Soil: A Composite Approach. Int. J. Numer. Anal. Methods Geomech. 44, 1350–1368. doi:10.1002/nag.3065
Gardner, T. N., and Goringe, M. J. (1988). The Measurement of Gas Bubble Size Distributions in a Three Phase Laboratory Gassy Soil. Geotechnical Test. J. 11, 49–55.
Grozic, J. L. H. (2003). “Liquefaction Potential of Gassy Marine Sands,” in Submarine Mass Movements and Their Consequences (Dordrecht, Netherlands: Springer), 37–45. doi:10.1007/978-94-010-0093-2_5
Grozic, J. L. H., Nadim, F., and Kvalstad, T. J. (2005b). On the Undrained Shear Strength of Gassy Clays. Comput. Geotechnics 32, 483–490. doi:10.1016/j.compgeo.2005.10.002
Grozic, J. L., Imam, S. M., Robertson, P. K., and Morgenstern, N. R. (2005a). Constitutive Modeling of Gassy Sand Behaviour. Can. Geotech. J. 42, 812–829. doi:10.1139/t05-015
Grozic, J. L., Robertson, P. K., and Morgenstern, N. R. (2000). Cyclic Liquefaction of Loose Gassy Sand. Can. Geotech. J. 37, 843–856. doi:10.1139/t00-008
Guan, X. (2017). Experimental Research on Liquefaction Characteristics of Seabed Gassy Sand under Cyclic Loading. Huainan, Anhui, China: AnHui University of Science and Technology.
Guo, A., Kong, L., Chen, J., and Zhong, F-J. (2007). On Engineering Characteristics of Sallow Biogenetic Gassy Sand by Piezocone Test. Rock Soil Mech. 28, 1539. doi:10.3969/j.issn.1000-7598.2007.08.003
Guo, Z., Liu, T., Wu, C., Su, X., and Li, S. (2021). Characterization and Reconstruction of Meso-Structure of Gas-Bearing Soils at Different Storage Pressures. Haiyang Xuebao 43, 96–104. doi:10.12284/hyxb2021154
Han, Z. (2020). Study on the Dynamic Response of Gas-Bearing Sandy Seabed Induced by Wave Loadings [M]. Guilin, Guangxi, China: Guilin University of Technology.
Hong, J., and Xu, M. (2020). DEM Study on the Undrained Mechanical Behavior of Gassy Sand. Acta Geotech. 15, 2179–2193. doi:10.1007/s11440-019-00910-x
Hong, Y., Chen, X. Y., Wang, L. Z., and Bin, Y. (2021a). Distinct Undrained Cyclic Behavior of Fine-Grained Gassy Soil at Various Initial Pore Water Pressures. J. geotechnical geoenvironmental Eng. 147, 06020027. doi:10.1061/(asce)gt.1943-5606.0002419
Hong, Y., Wang, L., and Yang, B. (2018). A Device for In-Situ Measurement of Gas Content in Submarine Gassy Soil and Measurement Method.
Hong, Y., Wang, L., Yang, B., and Zhang, J. (2019). Stress-dilatancy Behaviour of Bubbled Fine-Grained Sediments. Eng. Geol.(C) 260, 105196. doi:10.1016/j.enggeo.2019.105196
Hong, Y., Wang, L., Zhang, J., and Gao, Z. (2020a). 3D Elastoplastic Model for Fine-Grained Gassy Soil Considering the Gas-Dependent Yield Surface Shape and Stress-Dilatancy. J. Eng. Mech. [J] 146, 04020037. doi:10.1061/(ASCE)EM.1943-7889.0001760
Hong, Y., Wang, X., Wang, L., and Gao, Z. (2021b). A State-dependent Constitutive Model for Coarse-Grained Gassy Soil and its Application in Slope Instability Modelling. Comput. Geotechnics 129, 103847. doi:10.1016/j.compgeo.2020.103847
Hong, Y., Wang, L. Z., Ng, C. W. W., and Yang, B. (2017). Effect of Initial Pore Pressure on Undrained Shear Behaviour of Fine-Grained Gassy Soil. Can. Geotech. J. 54, 1592–1600. doi:10.1139/cgj-2017-0015
Hong, Y., Zhang, J. F., Wang, L. Z., and Liu, T. (2020b). On Evolving Size and Shape of Gas Bubble in Marine Clay under Multi-Stage Loadings: Microcomputed Tomography (μCT) Characterization and Cavity Contraction Analysis. Can. Geotech. J. 57, 1072–1091. doi:10.1139/cgj-2019-0076
Hu, G. (2010). Identification of Submarine Landslides along the Continental Slope of the East China Sea and Analysisi of Factors Causing Submarine Landslides. Qingdao: Ocean University of China.
Janiewicz, D., Klusek, Z., Brodecka-Goluch, A., and Jerzy, B. (2019). Acoustic Investigations of Shallow Gas in the Southern Baltic Sea (Polish Exclusive Economic Zone): a Review. Geo-Marine Lett. 39, 1–17. doi:10.1007/s00367-018-0555-5
Jommi, C., Muraro, S., Trivellato, E., and Zwanenburg, C. (2019). Experimental Results on the Influence of Gas on the Mechanical Response of Peats. Géotechnique 69, 753–766. doi:10.1680/jgeot.17.p.148
Kaminski, P., Urlaub, M., Grabe, J., and Berndt, C. (2020). Geomechanical Behaviour of Gassy Soils and Implications for Submarine Slope Stability: a Literature Analysis. Geol. Soc. Lond. Spec. Publ. 500, 277–288. doi:10.1144/sp500-2019-149
Kong, L., Liu, W., Yuan, Q., and Dong, T. (2019). Triaxial Test on Gassy Sandy Soil under Constant Shear Stress Paths. Rock Soil Mech. 40, 3319–3326. doi:10.16285/j.rsm.2018.1863
Kong, X., Liu, J., Zou, D., and Liu, H. (2016). Stress-dilatancy Relationship of Zipingpu Gravel under Cyclic Loading in Triaxial Stress States. Int. J. Geomech. 16, 04016001. doi:10.1061/(asce)gm.1943-5622.0000584
Kortekaas, S., and Peuchen, J. (2008). “Measured Swabbing Pressures and Implications for Shallow Gas Blow-Out,” in Offshore Technology Conference, Houston, Texas. OnePetro.
Lai, X., Chen, Z., and Gou, Z. (2016). Seabed Shallow-Layer Gas Detection Method Based on MIP-CPT Technology.
Lee, M. W., and Collett, T. S. (2006). A Method of Shaly Sand Correction for Estimating Gas Hydrate Saturations Using Downhole Electrical Resistivity Log Data. Scientific Investigations Report2006-5121. Available at: https://pubs.er.usgs.gov/publication/sir20065121.
Li, L., Zhao, Y., and Yu, L. (2009). Exploration for Quaternary Shallow Biogenic Gas by Sealed Core Drilling and Modified CPT. Coal. Geol. Explor. 37, 72–76.
Li, Y., Lin, C., and Yu, J. (2007). Application of Stratagem EH4 Electromagnetic Imaging System in the Late Quaternary Strata in the Hangzhou Bay Area. Geol. Rev. 53, 413–420. doi:10.16509/j.georeview.2007.03.016
Lin, C., Li, G., and Li, Y. (2006). The Exploration Method of Late Quaternary Shallow Biogenic Gas Reservoirs in Hangzhou Bay Area. Geophys. Prospect. Petroleum. 45 (2), 202.
Liu, W. (2018). Triaxial Testing Study on Gassy Sand Soil under Constant Shear Stress Paths. Qingdao, China: Qingdao University of Technology.
Mabrouk, A., and Rowe, R. K. (2011). Effect of Gassy Sand Lenses on a Deep Excavation in a Clayey Soil. Eng. Geol. 122, 292–302. doi:10.1016/j.enggeo.2011.06.009
Mathiroban, S. (2004).A Model to Predict the Undrained Response of Loose Gassy Sand. (Unpublished master's thesis).Calgary, AB: University of Calgary.
Mustafa, E., Derman, D., and Günay, Ç. (2002). Acoustic Evidence for Shallow Gas Accumulations in the Sediments of the Eastern Black Sea. Terra nova. 14, 313–320. doi:10.1046/j.1365-3121.2002.00434.x
Nageswaran, S. (1983).Effect of Gas Bubbles on the Sea Bed Behaviour. Thesis (Ph.D.), United Kingdom: University of Oxford.
Ng, C. W. W., Zhan, L. T., and Cui, Y. J. (2002). A New Simple System for Measuring Volume Changes in Unsaturated Soils. Can. Geotech. J. 39, 757–764. doi:10.1139/t02-015
Pezard, P. A., Abdoulghafour, H., Denchik, N., Perroud, H., Lofi, J., Brondolo, F., et al. (2015). On Baseline Determination and Gas Saturation Derivation from Downhole Electrical Monitoring of Shallow Biogenic Gas Production. Energy Procedia 76, 555–564. doi:10.1016/j.egypro.2015.07.910
Pietruszczak, S., and Pande, G. N. (1996). Constitutive Relations for Partially Saturated Soils Containing Gas Inclusions. J. geotechnical Eng. 122, 50–59. doi:10.1061/(asce)0733-9410(1996)122:1(50)
Pruessmann, J., Coman, R., Endres, H., and Trappe, H. (2004). Improved Imaging and AVO Analysis of a Shallow Gas Reservoir by CRS. Lead. Edge 23, 915–918. doi:10.1190/1.1803503
Rad, N. S., and Lunne, T. (1994). Gas in Soil. I: Detection and η‐Profiling. J. Geotech. Engrg. 120, 697–715. doi:10.1061/(asce)0733-9410(1994)120:4(697)
Rad, N. S., Vianna, A. J. D., and Berre, T. (1994). Gas in Soils. II: Effect of Gas on Undrained Static and Cyclic Strength of Sand. J. Geotech. Engrg. 120, 716–736. doi:10.1061/(asce)0733-9410(1994)120:4(716)
Reeves, J. J. (2005). “An Integrated 3D-Seismic Exploration Method for Fractured Reservoirs in Tight Gas Sands,” in SPE Latin American and Caribbean Petroleum Engineering Conference. OnePetro.
Rowe, R., Goveas, L., and Dittrich, J. (2002). Briefing: Excavations in Gassy Soils. United Kingdom: Thomas Telford Ltd.
Rowe, R. K., and Mabrouk, A. (2018). Three-dimensional Analysis of Unanticipated Behavior of a Deep Excavation. Can. Geotechnical J. 55, 1647–1656. doi:10.1139/cgj-2017-0511
Sánchez, M., Gai, X., and Santamarina, J. C. (2017). A Constitutive Mechanical Model for Gas Hydrate Bearing Sediments Incorporating Inelastic Mechanisms. Comput. Geotechnics 84, 28–46. doi:10.1016/j.compgeo.2016.11.012
Sham, W. K. (1992). The Undrained Shear Strength of Soils Containing Large Gas Bubbles. Géotechnique 38, 399
Sills, G. C., and Gonzalez, R. (2001). Consolidation of Naturally Gassy Soft Soil. Géotechnique 51, 629–639. doi:10.1680/geot.2001.51.7.629
Sills, G. C., and Wheeler, S. J. (1992). The Significance of Gas for Offshore Operations. Cont. Shelf Res. 12, 1239–1250. doi:10.1016/0278-4343(92)90083-v
Sills, G. C., Wheeler, S. J., Thomas, S. D., and Gardner, T. N. (1991). Behaviour of Offshore Soils Containing Gas Bubbles. Géotechnique 41, 227–241. doi:10.1680/geot.1991.41.2.227
Sobkowicz, J. C., and Morgenstern, N. R. (1984). The Undrained Equilibrium Behaviour of Gassy Sediments. Can. Geotech. J. 21, 439–448. doi:10.1139/t84-048
Sultan, N., De Gennaro, V., and Puech, A. (2012). Mechanical Behaviour of Gas-Charged Marine Plastic Sediments. Géotechnique 62, 751–766. doi:10.1680/geot.12.og.002
Sultan, N., and Garziglia, S. (2014). Mechanical Behaviour of Gas-Charged Fine Sediments: Model Formulation and Calibration. Geotechnique 64, 851–864. doi:10.1680/geot.13.P.125
Thomas, S. D. (1987). The Consolidation Behaviour of Gassy Soil. United Kingdom: University of Oxford.
Thomas, S. D. (2021a). “Finite Element Model of Wave Loading on a Soil Seabed Part I: Multi-Layered Anisotropic Gassy Soil Conditions,” in Vietnam Symposium on Advances in Offshore Engineering. Editors D. V. K Huynh, A. M. Tang, D. H. Doan, and P. Watson (Singapore: Springer), 105
Thomas, S. D. (2021b). “Finite Element Model of Wave Loading on a Soil Seabed Part II: Heterogeneous Gassy Soil Conditions,” in Vietnam Symposium on Advances in Offshore Engineering. Editors D. V. K. Huynh, A. M. Tang, D. H. Doan, and P. Watson (Singapore: Springer), 114
Tóth, Z., Spieß, V., and Jensen, J. (2014). Seismo-acoustic Signatures of Shallow Free Gas in the Bornholm Basin, Baltic Sea. Cont. Shelf Res. 88, 228–239. doi:10.1016/j.csr.2014.08.007
Vanoudheusden, E., Sultan, N., and Cochonat, P. (2003). “Hydro-mechanical Behaviour of Gassy Soils,” in Submarine Mass Movements and Their Consequences. Editors J. Locat, J. Mienert, and L. Boisvert (Dordrecht: Springer), 145–153. doi:10.1007/978-94-010-0093-2_17
Vega-Posada, C. A., Finno, R. J., and Zapata-Medina, D. G. (2014). Effect of Gas on the Mechanical Behavior of Medium-Dense Sands. J. Geotech. Geoenviron. Eng. 140, 04014063. doi:10.1061/(asce)gt.1943-5606.0001163
Wang, J., Qin, C., Luo, X., Wang, S., and Yang, N. (2019). Application Prospect of in Gas Hydrate Exploration. Mar. Geol. Front. 35, 52–59. doi:10.16028/j.1009-2722.2019.11008
Wang, L. (2021). On the Consolidation and Creep Behaviour of Layered Viscoelastic Gassy Sediments. Eng. Geol. 293, 106298. doi:10.1016/j.enggeo.2021.106298
Wang, Y. (2009). Study on Evolution Characteristics of Engineering Effects and the Disaster Mechanism for Shallow Gassy Sand. Beijing, China: Institute of Rock & Soil Mechanics, Chinese Accdemy of Sciences.
Wang, Y., Wang, Z., and Liu, W. (2013). Measurement of Shallow Stratigraphic Sections for Seabed Gas Exploration in Northern Hangzhou Bay. Shanghai Land & Resour. 34, 59
Wang, Y., Kong, L., Wang, Y., Wang, M., and Wang, M. (2018). Liquefaction Response of Loose Gassy Marine Sand Sediments under Cyclic Loading. Bull. Eng. Geol. Environ. 77, 963–976. doi:10.1007/s10064-017-1164-7
Wheeler, S. J. (1986).The Stress-Strain Behaviour of Soils Containing Gas Bubbles. MPLSthesis.United Kingdom: University of Oxford.
Wheeler, S. J. (1988a). A Conceptual Model for Soils Containing Large Gas Bubbles. Géotechnique 38, 389–397. doi:10.1680/geot.1988.38.3.389
Wheeler, S. J., and Gardner, T. N. (1989). Elastic Moduli of Soils Containing Large Gas Bubbles. Géotechnique 39, 333–342. doi:10.1680/geot.1989.39.2.333
Wheeler, S. J. (1988b). The Undrained Shear Strength of Soils Containing Large Gas Bubbles. Géotechnique 38, 399–413. doi:10.1680/geot.1988.38.3.399
Wood, W. T., Holbrook, W. S., and Hoskins, H. (2000).27 Situ Measurements of P-Wave Attenuation in the Methane Hydrate–and Gas-Bearing Sediments of the Blake Ridge1,” in Proceedings of the Ocean Drilling Program. Scientific Results, Ocean Drilling Program, 265
Wu, S-J., Wang, X., wang, S., Zhang, B., Yang, C-J., and Zhi, H. (2022). Active Temperature-Preserving Deep-Sea Water Sampler Configured with a Pressure-Adaptive Thermoelectric Cooler Module. Oceanogr. Res. Pap. 181, 103701. doi:10.1016/j.dsr.2022.103701
Xiao, Y., Liu, H., Sun, Y., Liu, H., and Chen, Y. (2015). Stress-dilatancy Behaviors of Coarse Granular Soils in Three-Dimensional Stress Space. Eng. Geol. 195, 104–110. doi:10.1016/j.enggeo.2015.05.029
Xiao, Y., Long, L., Matthew Evans, T., Zhou, H., Liu, H., and Stuedlein, A. W. (2019). Effect of Particle Shape on Stress-Dilatancy Responses of Medium-Dense Sands. J. Geotech. Geoenviron. Eng. 145, 04018105. doi:10.1061/(asce)gt.1943-5606.0001994
Xing, L., Jiao, J., and Liu, X. (2017). Distribution and Seismic Reflection Characteristics of Shallow Gas in Bohai Sea. Periodical Ocean Univ. China 47, 9. doi:10.16441/j.cnki.hdxb.20160354
Xu, D.-S., Su, Z.-Q., Lalit, B., and Qin, Y. (2022). A Hybrid FBG-Based Load and Vibration Transducer with a 3D Fused Deposition Modelling Approach. Meas. Sci. Technol. 33, 065106. doi:10.1088/1361-6501/ac5a9b
Xu, Y.-S., Wu, H.-N., Shen, J. S., and Zhang, N. (2017). Risk and Impacts on the Environment of Free-phase Biogas in Quaternary Deposits along the Coastal Region of Shanghai. Ocean. Eng. 137, 129–137. doi:10.1016/j.oceaneng.2017.03.051
Yang, G., and Bai, B. (2020). A Thermodynamic Model to Simulate the Thermo-Mechanical Behavior of Fine-Grained Gassy Soil. Bull. Eng. Geol. Environ. 79, 2325–2339. doi:10.1007/s10064-019-01694-w
Yin, Z.-Y., and Chang, C. S. (2013). Stress-dilatancy Behavior for Sand under Loading and Unloading Conditions. Int. J. Numer. Anal. Meth. Geomech. 37, 855–870. doi:10.1002/nag.1125
Zhang, R., and Lunne, T. (2003). Deepwater Sample Disturbance Due to Stress Relief. Chin. Jounal Geotechnical Eng. 25, 356. doi:10.3321/j.issn:1000-4548.2003.03.023
Zheng, H., Jiang, W., and Zhang, T. (2006). Using AVO Technique to Detect Quaternary Shallow Biogas in Hangzhou Bay Area. Oil Geophys. Prospect. 21-24, 142
Zhong, F. (2007). Research on Pressure Characteristics of Gas Reservoir and Mechanical Effect of Stress Path for Shallow Gassy Sand. Beijing, China: Institute of Rock & Soil Mechanics, Chinese Academy of Sciences.
Zhu, B., Huang, J., Wang, L., and Ye, Z. (2021). Precise Numerical Study on the Behaviour of Gassy Marine Soils Subjected to Thermal and Mechanical Loadings. Comput. Geotechnics 137, 104269. doi:10.1016/j.compgeo.2021.104269
Zhu, H., Chen, J.-w., Ren, Z.-q., Zhang, P.-h., Gao, Q.-l., Le, X.-l., et al. (2022). A New Technique for High-Fidelity Cutting Technology for Hydrate Samples. J. Zhejiang Univ. Sci. A 23, 40–54. doi:10.1631/jzus.a2100188
Keywords: gassy sediments, mechanical responses, constitutive model, in-situ methods, disaster simulation
Citation: Liu T, Yang X and Zhang Y (2022) A Review of Gassy Sediments: Mechanical Property, Disaster Simulation and In-Situ Test. Front. Earth Sci. 10:915735. doi: 10.3389/feart.2022.915735
Received: 08 April 2022; Accepted: 23 May 2022;
Published: 13 June 2022.
Edited by:
Ya Ping Wang, East China Normal University, ChinaReviewed by:
Lizhong Wang, Zhejiang University, ChinaZhongnian Yang, Qingdao University of Technology, China
Copyright © 2022 Liu, Yang and Zhang. This is an open-access article distributed under the terms of the Creative Commons Attribution License (CC BY). The use, distribution or reproduction in other forums is permitted, provided the original author(s) and the copyright owner(s) are credited and that the original publication in this journal is cited, in accordance with accepted academic practice. No use, distribution or reproduction is permitted which does not comply with these terms.
*Correspondence: Yan Zhang, zhangyan4850@ouc.edu.cn