Physiological oxygen measurements in vitro-Schrödinger’s cat in 3D cell biology
- 1Institute of Functional Interfaces, Karlsruhe Institute of Technology, Karlsruhe, Germany
- 2PreSens Precision Sensing GmbH, Regensburg, Germany
After the development of 3D cell culture methods in the middle of the last century and the plethora of data generated with this culture configuration up to date, it could be shown that a three-dimensional arrangement of cells in most of the cases leads to a more physiological behavior of the generated tissue. However, a major determinant for an organotypic function, namely, the dissolved oxygen concentration in the used in vitro-system, has been neglected in most of the studies. This is due to the fact that the oxygen measurement in the beginning was simply not feasible and, if so, disturbed the measurement and/or the in vitro-system itself. This is especially true for the meanwhile more widespread use of 3D culture systems. Therefore, the tissues analyzed by these techniques can be considered as the Schrödinger’s cat in 3D cell biology. In this perspective paper we will outline how the measurement and, moreover, the regulation of the dissolved oxygen concentration in vitro-3D culture systems could be established at all and how it may be possible to determine the oxygen concentration in organoid cultures and the respiratory capacity via mito stress tests, especially in spheroids in the size range of a few hundred micrometers, under physiological culture conditions, without disturbances or stress induction in the system and in a high-throughput fashion. By this, such systems will help to more efficiently translate tissue engineering approaches into new in vitro-platforms for fundamental and applied research as well as preclinical safety testing and clinical applications.
1 Introduction
Since the introduction of 3D cell culture techniques by (Holtfreter et al., 1944; Moscona et al., 1957) in the middle of the last century, it has been shown that three-dimensional in vitro-approaches are superior to their two-dimensional counterparts in many respects. This superiority is characterized by changes in key cellular parameters that influence the organotypic behavior such as morphology (Puschmann et al., 2013; Li et al., 2017), ability to establish cell-cell and cell-matrix contacts (Duval et al., 2017; Kapałczyńska et al., 2018; Yamada et al., 2022), proliferation behavior (Kapałczyńska et al., 2018; Souza et al., 2018), metabolism (Tidwell et al., 2022; Rybkowska et al., 2023), differentiation capacity and differentiation status (Altmann et al., 2008; Li et al., 2017; Gaut et al., 2020; Mora-Roldan et al., 2021), mechanotransduction (Chhetri et al., 2021; Man et al., 2022), and drug sensitivity (Stock et al., 2016; Bell et al., 2018). Consequently, this knowledge has led to the development of tissue engineering approaches that better recapitulate the in vivo-situation and therefore deliver much more reliable data with regard to transferability to the human situation. One aspect that considerably contributed to that was the development of organoid technology. Organoids can be generated from primary cells as well as embryonal or induced pluripotent stem cells. Once the latter have been appropriately differentiated, they display an unprecedented level of physiomimetic function. Meanwhile, organoid technology is able to generate tissues from all three germ layers with skin (Lee et al., 2020) and brain organoids as derivatives of the ectoderm (Bagley et al., 2017), with kidney (Morizane et al., 2015), blood vessels (Wimmer et al., 2019) and heart (Drakhlis et al., 2021) as mesodermal derivatives, and liver (Hu et al., 2018), small intestine (Sato et al., 2009), and colon (Sugimoto et al., 2018) as examples of endodermal derivatives.
But as obvious from the above-mentioned list of parameters and tissue engineering approaches that have extensively been characterized, only a few studies dealt with the oxygen concentration and its regulation in the used culture system although it has been shown as early as 1972 that neoplastic and non-neoplastic fetal tissues from mouse and rat have an equal or better plating efficiency in 1%–3% oxygen compared to 20% (Richter et al., 1972). Lewis lung tumor and B16 melanoma cells taken directly from the mouse showed an in vivo-like behavior with regard to survival after irradiation when cultured under 5% oxygen in agar gels for 8–15 days (Courtenay, 1976). It was also shown that colony formation of hematopoietic cells resulted in increased colony numbers as well as larger colonies when cultivated under 5% oxygen in contrast to 18% (Bradley et al., 1978). Typically, animal cells are reported to have been cultured at 37°C and under “ambient atmosphere” which refers to an oxygen concentration of 20.9% in air. First of all, this concentration is typically not reached in conventional incubator atmospheres equipped with a CO2 supplementation and its higher humidity, leading to a typical concentration of only 18.5% oxygen in the incubation chamber (Keeley and Mann, 2019) (Figure 1A). Secondly, even the 18.5% oxygen concentration is not reached, apart from the trachea, in any human or animal tissue but still, cell culture is routinely performed at this concentration (Figures 1B, C).
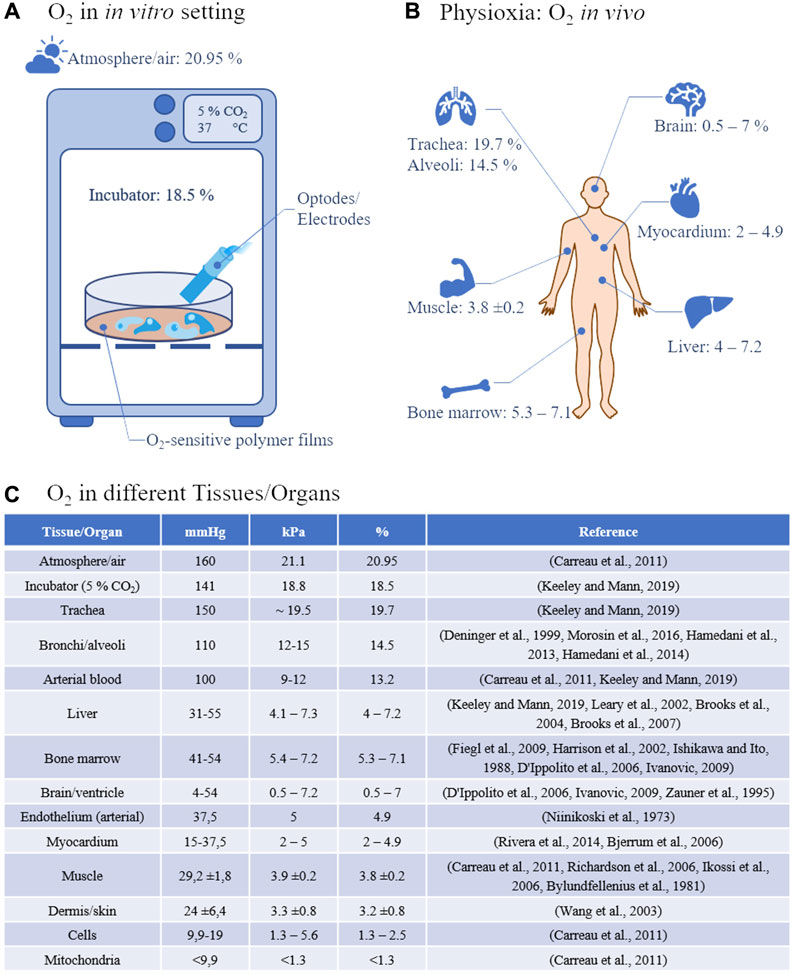
FIGURE 1. (A) Oxygen concentration and measurement in vitro: Under standard incubator conditions, 21% oxygen translates into 18.5% oxygen at 37°C, 5% CO2, and increased humidity. Measurements in 2D cell cultures are currently carried out either classically via optodes or electrodes or via oxygen-sensitive polymer films, e.g., on the bottom of the cell culture vessels. (B) Physioxia describes tissue-specific oxygen concentrations in vivo. The variations between the single tissues are enormous from, e.g., almost 20% in the trachea to less than 1% in the brain. (C) Typical air and tissue oxygen partial pressures in the most common measurement units.
Moreover, this is oftentimes termed “normoxia” which, from the cell’s/tissue’s perspective, is a hyperoxia. This hyperoxia leads to oxygen stress on a cellular level with the generation of reactive oxygen species (ROS) and their influence on, e.g., the NrF2-, NF-κB- and MAPK-signaling cascade (Alva et al., 2022). Although small concentrations of reactive oxygen species are used in cellular signaling (Droge, 2002) and in the short term, can be compensated for due to a number of defense mechanisms that have evolved over time, in the long run, too high ROS concentrations are implicated in being involved in the generation of several diseases such as cancer (Jelic et al., 2021), male infertility (Bisht et al., 2017), type 2 diabetes mellitus (Bigagli and Lodovici, 2019), heart failure (van der Pol et al., 2019), and aging (Liguori et al., 2018), among others. On the other hand, a hypoxia is believed to play a major role in many diseases as well (Semenza, 2014). This leads us to the necessity of defining a tissue specific normoxia which might be more appropriately be termed as physioxia (Carreau et al., 2011). When looking into the literature, this physioxia, as data are available at all, is measured either as a concentration in µM, or as a physiologically more relevant partial pressure, reported in kPa or mmHg whereas oxygen in in vitro-systems is often determined as the dissolved oxygen concentration given in % of the respective medium with 1% oxygen corresponding to 1.02 kPa or 7.65 mmHg. To adjust oxygen to physiological levels in vitro it is imperative to measure physioxia in body fluids and living tissues. For this, several measurement techniques have been developed of which the Clark-type electrodes (Clark et al., 1953) and the Whalen-type electrodes (Whalen et al., 1973) were used to determine the pO2 in body liquids and tissues, respectively. However, due to their measurement principle of reducing O2 on a polarized electrode that consumes oxygen and which is becoming more difficult the smaller the sample volume is, renders this technique less accurate when measuring low pO2 levels. Moreover, the piercing of the tissue with one or more electrodes, to be able to measure more representative O2 levels, may damage the tissue leading to altered O2 values (Jamieson and van den Brenk, 1965). This and the fact of being hardly able to control the post-diffusion into the penetration channel is making the tissue with this kind of measurement technique to a kind of Schrödinger’s cat in cell biology. Alternative techniques such as hemoglobin/myoglobin spectroscopy which measures changes in the absorption characteristics upon binding O2 can be converted into an approximate pO2 although this conversion is subject to changes in local pH/pCO2 and temperature and hence should be used with caution (Keeley and Mann, 2019). Moreover, hemoglobin/myoglobin is rarely used in in vitro-systems. An interesting clinical measuring technique is the so-called dynamic contrast-enhanced near-infrared spectroscopy (NIRS) (Davies et al., 2015) using indocyanine green (ICG) (Forcione et al., 2020). With the help of this technique it could be shown that there is a mismatch between the tissue partial oxygen pressure and tissue respiration (Forcione et al., 2021). This is an extremely important finding since it shows that even in case of a sufficient oxygen partial pressure, the parenchymal tissue respiration might be impaired. Therefore, it is important to look at both aspects in in vitro-systems as well. More sophisticated techniques to measure tissue oxygen like, e.g., magnetic, paramagnetic, and electron spin resonance techniques require expensive instrumentation, dedicated and trained personnel, as well as considerably more space which is why these techniques are not very common. In addition, the spatial resolution here is more useful for mapping, e.g., rodents rather than very small organoids, which is of course counterproductive if one wants to reduce animal experiments. With the development of phosphorescent dyes which react with the so-called dynamic quenching effect upon contact with O2, a new method for the detection of tissue oxygen concentrations was possible (Rumsey et al., 1988). The effect is based on the collision of an O2 molecule with a luminophore in its lowest excited state, resulting in a radiationless deactivation and return to the ground state both of which can be measured. As a result, luminescence intensity (I) and lifetime (τ) are reduced in the presence of O2. These luminophore reporter dyes, among others, comprise Pt (II)- and Pd(II)-porphyrine complexes that are suitable for oxygen measurements in the range of 0–200 µM due to their quenching effects (Dmitriev and Papkovsky, 2012). Moreover, the reporter dyes can be formulated into different formats by immobilizing them in special polymers that allow oxygen diffusion but prevent the dyes from bleeding into the measurement medium so that extra- (Dunphy et al., 2002) and intracellular (Neugebauer et al., 2008) measurements can be performed. By this, very high spatial resolutions are possible (Blancke Soares et al., 2021). Meanwhile, there is a wide variety of fluorescence optical oxygen sensors available (Wang and Wolfbeis, 2014).
In general, the fluorescence optical sensor technique has led into the development of sophisticated instruments with which mitochondrial respiration and glycolytic activity of monolayers can be measured in real-time metabolic assays (Agilent Technologies, Incyton) that have led to so-called mito stress test and mito complex assays. Although this type of assay is a very powerful technique to test the cellular reactions upon, e.g., drug administration, the system is neither able to perform these measurements in 3D cultures nor can they be performed under physiological conditions. Due to the used measurement technique that requires the lowering of the measuring device into the well and thereby blocking atmospheric exchange, a severe hypoxia is generated leading to experiment durations of only 30–60 min. However, one of the greatest advantages of the luminescence approach is the fact that the required reporter molecules can be incorporated into polymer matrices like polystyrene, with or without covalent linkage to the polymer backbone (Babilas et al., 2005). Anyway, up to now this was only possible in two-dimensional in vitro-cultures.
What significantly adds up to the non-physiological in vitro-culture conditions is the lack of a flow. Most of the experimental (oxygen) data available have been generated in static culture systems which do not provide a physiological environment. This changed with the introduction of microbioreactors that not only miniaturized the systems, thereby being able to parallelize experiments, but moreover were able to incorporate an active flow. Beginning with rather simple setups, like the PDMS (polydimethylsiloxane) perfusion microbioreactor for the 3D culture of chondrocytes (Wu et al., 2006), this area of research developed into the major driver for the advancement of organotypic culture systems, that is, currently represented in many types of single- or multi-organ-, or human/body-on-a-chip (OOAC) systems. But although capabilities for, e.g., mechanical or electrical stimulation can be incorporated, only a few systems are capable of on-chip analysis of the metabolic and physiological behavior of the tissue and are analyzed by classical off-chip technologies such as PCR, enzyme-linked immunosorbent assays (ELISA), and mass spectrometry (MS) (Li and Tian, 2018). Again, a major driver for the incorporation of sensors that are capable of characterizing online and in real-time the status of the culture in OOAC systems, was miniaturization which was used in lab-on-a-chip systems for the analysis of smaller volumes in shorter times. Basic sensing principles comprise electrical, electrochemical, and optical techniques. Within the electrical sensor family, trans-endothelial/endothelial resistance (TEER) electrodes (Odijk et al., 2015) have become relatively common to characterize the barrier function of cells. For the characterization of electrically active cells, the extracellular field potential measurement technique, realized in multielectrode arrays (MEA), can be employed (Koutsouras et al., 2017). Among electrochemical sensors, potentiometric and amperometric sensors can be distinguished. Both are making use of the transformation of an electrochemical interaction between an analyte and an electrode (Fuchs et al., 2021) leading to a read-out, that is, either a voltage difference or a current flow between two electrodes. Common analytes for this measurement technique are oxygen and pH with the disadvantages in oxygen measurements as discussed earlier. Optical sensors are based on detecting changes in an optical property, such as luminescence, absorption, refractive index, or scattering (Fuchs et al., 2021). Of these, photoluminescence is a very attractive approach for measuring oxygen since it does not interfere with the analyte and the tissue because the required fluorophores (indicator and reference dyes) can be incorporated into a polymer matrix thereby passivating the sensor and separating the indicator dyes from the tissue. With this technique, a sensitivity of 0.067 Pa for oxygen can be achieved (Wolfbeis et al., 1986).
2 Paving the road for oxygen measurements in 3D cultures
So far, only very limited studies showing oxygen measurements in 3D culture systems are available. One of the approaches used a needle-type sensor equipped with an optical sensor foil which was mounted on computer-aided micromanipulator for z-axis oxygen profiling in collagen gels (Wolff et al., 2019). A similar system, capable of increasing the throughput by automation and therefore, to perform microphysiological measurements in real-time over extended cultivation periods, was introduced by Eggert et al. (2021). A phosphorescence lifetime-based system is able to measure the dissolved oxygen concentration even within 3D cellular constructs by incorporating oxygen-sensitive microbeads (Weyand et al., 2015; Wesseler et al., 2022). However, as discussed above, these measurement techniques only deliver spot-like measurement data, may destroy the tissue architecture within the piercing channel with the associated adverse effects on the cells, and are not able to characterize the cells’ metabolism further. Multiplexing is also limited by, e.g., the fiber optic cable management or the system setup. It would therefore be of prime importance to be able to measure oxygen in a multiplex-fashion in 3D culture systems in a real-time, label-free, oxygen consumption-free method in the direct microenvironment of the tissue under physiological conditions. Such a system has recently been introduced by Grün et al. (2023) and was realized by using an oxygen-sensitive polymer film that was subjected to a microthermoforming process resulting in an oxygen-sensitive microcavity array, so-called sensor arrays. The sensor arrays cannot only be used as the system for the generation and culture of the spheroids/organoids themselves (self-assembly of 3D aggregates which show very similar sizes and shapes by simply pipetting the cells into form-exact microcavities) but moreover deliver oxygen data from the direct environment of the aggregates. The system also delivers oxygen gradient profiles due to the integrated bevel on the upper side of the microcavities (Figure 2). This would deliver the necessary information of the tissue supply with oxygen via diffusion which would compare to perfusion of tissues with indocyanine green to characterize tissue integrity (Forcione et al., 2020).
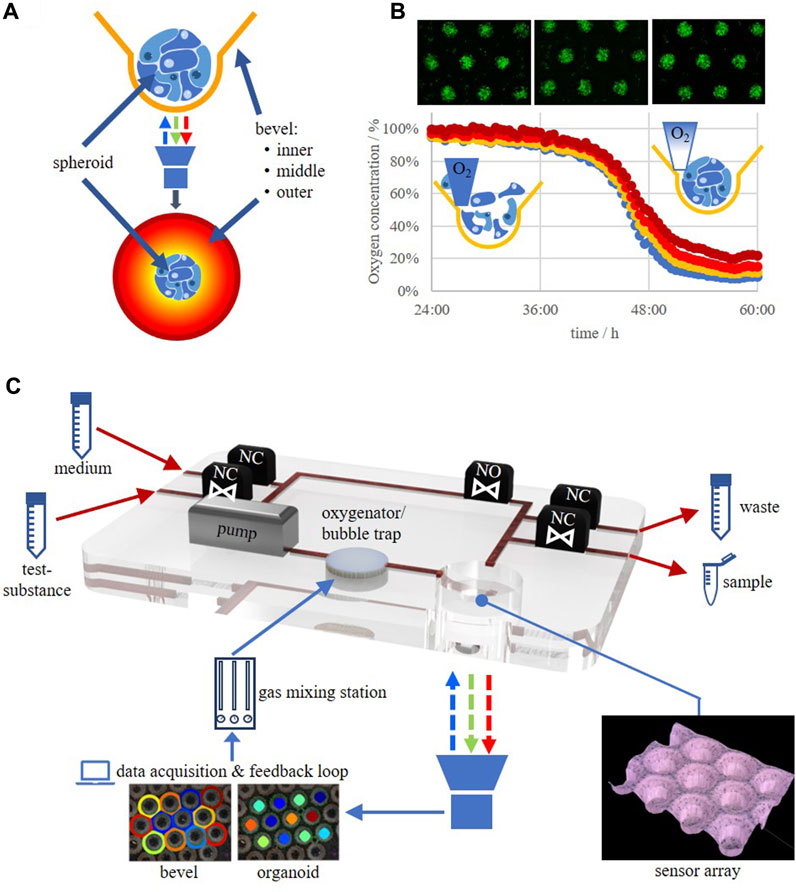
FIGURE 2. (A) Spheroid/organoid in an oxygen-sensitive microcavity. An oxygen gradient is established by realizing an oxygen post-diffusion from the culture medium to the cells mimicking the situation in post-capillary tissues. The dissolved oxygen concentration in the direct microenvironment of the spheroid/organoid as well as oxygen gradients at the upper side of the microcavity can be measured. (B) HepG2 cells seeded as a single cell suspension into a sensor array and the corresponding change in oxygen levels in the time frame from 24 to 60 h after seeding. (C) Schematic drawing of an oxygen-feedback regulated microbioreactor circulation. NO, valve, normally open; NC, valve, normally closed.
By the help of the sensor array technology it would now be feasible to run, e.g., mito stress tests under physiological conditions, unlike those that are currently performed in 2D cultures which are, furthermore, restricted in time due to the fact that the measurement principle generates a hypoxic atmosphere itself. Now, 3D mito stress tests, for the characterization of the respiratory capacity of 3D cultures/organoids can be performed which was recently demonstrated (Grün et al., 2023). By such techniques it will now be possible to determine the oxygen concentration for individual cell types under physiologically relevant concentrations and/or best physiomimetic function. This could be achieved by, e.g., determining the expression of typical tissue markers on a genetic as well as a protein level and/or enzymatic functions. By this, a database will evolve in which optimal in vitro oxygen concentrations are collected, leading to an oxygen footprint for each individual cell type, be it a cell line or a primary cell. Therefore, such systems will help to more efficiently translate tissue engineering approaches into new in vitro-platforms for fundamental and applied research as well as preclinical safety testing and clinical applications.
3 Discussion
We are just at the beginning of a new era in in vitro cell biology in which we will be able to not only culture cells in 3D but moreover in an organotypic way that will resemble the tissue of origin in an unprecedented manner. This will largely be achieved by incorporating oxygen measurement techniques into the culture systems that do not interfere with the culture itself and do not change the culture inherent oxygen concentrations. This being said, it is only a small step to 3D culture systems that use the oxygen measurement data as an input for a feedback-regulated measurement environment in which the cells regulate the optimal oxygen concentration themselves (Figure 2). The application of a regulated oxygen tension via the culture medium into the 3D cell construct mimics the natural supply process in vascularized tissue, or more precisely, the process taking place in the post-capillary tissue zone. This will finally overcome the problems with the so far called normoxia in current in vitro cultures which at the same time will generate the need to establish new standards for the cultivation of the different cells currently in use. Associated with this is a critical evaluation of older data because the physioxic data may differ from what has been measured under hyperoxic conditions that formerly have been designated as “normoxic conditions” (18.5% oxygen).
As usual, the technological development will set the pace for an even further advancement of physiomimetic in vitro culture systems. Currently, artificial intelligence is finding its way into image analysis to judge the status of the culture, organoid intelligence is used as a means of a genuine biological computing that harnesses brain organoids using scientific and bioengineering advances in an ethically responsible manner (Smirnova et al., 2023), exosomes and liquid crystal biosensors can be used for the early diagnosis of cancer and pathogens (Pani et al., 2023; Shin et al., 2023), short-wave infrared quantum dots have been shown to act as molecular probes for the labeling and detection of single antibodies, growth factors, and nucleic acid molecules in fluorescence microscopy applications (Sarkar et al., 2020), to name just a few. In any case will the new systems be smaller and smarter, will decrease the resource needs thus making the systems economically more attractive, will deliver more reliable results and by this, will dramatically increase the transferability to the in vivo situation.
Data availability statement
The original contributions presented in the study are included in the article/Supplementary Material, further inquiries can be directed to the corresponding author.
Author contributions
EG wrote the draft of the manuscript. CG generated the figures and proofread the manuscript. CN investigated the literature for the chapter of in vivo normoxia data and proofread the manuscript, and GL wrote the luminescent oxygen sensor part of the manuscript and critically reviewed the rest of the manuscript. All authors contributed to the article and approved the submitted version.
Conflict of interest
Author GL is employed by the company PreSens Precision Sensing GmbH.
The remaining authors declare that the research was conducted in the absence of any commercial or financial relationships that could be construed as a potential conflict of interest.
Publisher’s note
All claims expressed in this article are solely those of the authors and do not necessarily represent those of their affiliated organizations, or those of the publisher, the editors and the reviewers. Any product that may be evaluated in this article, or claim that may be made by its manufacturer, is not guaranteed or endorsed by the publisher.
References
Altmann, B., Giselbrecht, S., Weibezahn, K. F., Welle, A., and Gottwald, E. (2008). The three-dimensional cultivation of the carcinoma cell line HepG2 in a perfused chip system leads to a more differentiated phenotype of the cells compared to monolayer culture. Biomed. Mat. 3, 034120. doi:10.1088/1748-6041/3/3/034120
Alva, R., Mirza, M., Baiton, A., Lazuran, L., Samokysh, L., Bobinski, A., et al. (2022). Oxygen toxicity: cellular mechanisms in normobaric hyperoxia. Cell Biol. Toxicol. 39, 111–143. doi:10.1007/s10565-022-09773-7
Babilas, P., Liebsch, G., Schacht, V., Klimant, I., Wolfbeis, O. S., Szeimies, R. M., et al. (2005). In Vivo Phosphorescence Imaging of pO<b>2</b>Using Planar Oxygen Sensors. Microcirculation 12, 477–487. doi:10.1080/10739680591003314
Bagley, J. A., Reumann, D., Bian, S., Levi-Strauss, J., and Knoblich, J. A. (2017). Fused cerebral organoids model interactions between brain regions. Nat. Methods 14, 743–751. doi:10.1038/nmeth.4304
Bell, C. C., Dankers, A. C. A., Lauschke, V. M., Sison-Young, R., Jenkins, R., Rowe, C., et al. (2018). Comparison of Hepatic 2D Sandwich Cultures and 3D Spheroids for Long-term Toxicity Applications: A Multicenter Study. Toxicol. Sci. 162, 655–666. doi:10.1093/toxsci/kfx289
Bigagli, E., and Lodovici, M. (2019). Circulating Oxidative Stress Biomarkers in Clinical Studies on Type 2 Diabetes and Its Complications. Oxid. Med. Cell. Longev. 5953685, 1–17. doi:10.1155/2019/5953685
Bisht, S., Faiq, M., Tolahunase, M., and Dada, R. (2017). Oxidative stress and male infertility. Nat. Rev. Urol. 14, 470–485. doi:10.1038/nrurol.2017.69
Blancke Soares, A., Meier, R., Liebsch, G., Schwenk-Zieger, S., Kirmaier, M. E., Theurich, S., et al. (2021). High-resolution spatiotemporal pHe and pO2 imaging in head and neck and oesophageal carcinoma cells. Cancer Metab. 9, 21. doi:10.1186/s40170-021-00257-6
Bradley, T. R., Hodgson, G. S., and Rosendaal, M. (1978). The effect of oxygen tension on haemopoietic and fibroblast cell proliferation in vitro. J. Cell. Physiol. 97, 517–522. doi:10.1002/jcp.1040970327
Carreau, A., El Hafny-Rahbi, B., Matejuk, A., Grillon, C., and Kieda, C. (2011). Why is the partial oxygen pressure of human tissues a crucial parameter? Small molecules and hypoxia. J. Cell. Mol. Med. 15, 1239–1253. doi:10.1111/j.1582-4934.2011.01258.x
Chhetri, A., Risploi, J. V., and Lelièvre, S. A. (2021). 3D Cell Culture for the Study of Microenvironment-Mediated Mechanostimuli to the Cell Nucleus: an Important Step for Cancer Research. Front. Mol. Biosci. 8, 628386. doi:10.3389/fmolb.2021.628386
Clark, L. C., Wolf, R., Granger, D., and Taylor, Z. (1953). Continuous recording of blood oxygen tensions by polarography. J. Appl. Physiol. 6, 189–193. doi:10.1152/jappl.1953.6.3.189
Courtenay, V. D. (1976). A soft agar colony assay for Lewis lung tumour and B16 melanoma taken directly from the mouse. Br. J. Cancer 34, 39–45. doi:10.1038/bjc.1976.119
Davies, D. J., Su, Z., Clancy, M. T., Lucas, S. J., Deghani, H., Logan, A., et al. (2015). Near-Infrared Spectroscopy in the Monitoring of Adult Traumatic Brain Injury: A Review. J. Neurotrauma 32, 933–941. doi:10.1089/neu.2014.3748
Dmitriev, R. I., and Papkovsky, D. B. (2012). Optical probes and techniques for O2 measurement in live cells and tissue. Cell. Mol. Life Sci. 69, 2025–2039. doi:10.1007/s00018-011-0914-0
Drakhlis, L., Devadas, S. B., and Zweigerdt, R. (2021). Generation of heart-forming organoids from human pluripotent stem cells. Nat. Protoc. 16, 5652–5672. doi:10.1038/s41596-021-00629-8
Droge, W. (2002). Free radicals in the physiological control of cell function. Physiol. Rev. 82, 47–95. doi:10.1152/physrev.00018.2001
Dunphy, I., Vinogradov, S. A., and Wison, D. F. (2002). Oxyphor R2 and G2: phosphors for measuring oxygen by oxygen-dependent quenching of phosphorescence. Anal. Biochem. 310, 191–198. doi:10.1016/s0003-2697(02)00384-6
Duval, K., Grover, H., Han, L. H., Mou, Y., Pegoraro, A. F., Fredberg, J., et al. (2017). Modeling Physiological Events in 2D vs. 3D Cell Culture. Physiol. (Bethesda) 32, 266–277. doi:10.1152/physiol.00036.2016
Eggert, S., Gutbrod, M. S., Liebsch, G., Meier, R., Meinert, C., and Hutmacher, D. W. (2021). Automated 3D Microphysiometry Facilitates High-Content and Highly Reproducible Oxygen Measurements within 3D Cell Culture Models. ACS Sens. 6, 1248–1260. doi:10.1021/acssensors.0c02551
Forcione, M., Ganau, M., Prisco, L., Chiarelli, A. M., Bellelli, A., Belli, A., et al. (2021). Mismatch between Tissue Partial Oxygen Pressure and Near-Infrared Spectroscopy Neuromonitoring of Tissue Respiration in Acute Brain Trauma: the Rationale for Implementing a Multimodal Monitoring Strategy. Int. J. Mol. Sci. 22, 1122. doi:10.3390/ijms22031122
Forcione, M., Yakoub, K. M., Chiarelli, A. M., Perpetuini, D., Merla, A., Sun, R., et al. (2020). Dynamic contrast-enhanced near-infrared spectroscopy using indocyanine green on moderate and severe traumatic brain injury: a prospective observational study. Quant. Imaging Med. Surg. 10, 2085–2097. doi:10.21037/qims-20-742
Fuchs, S., Johansson, S., Tjell, A. Ø., Werr, G., Mayr, T., and Tenje, M. (2021). In-Line Analysis of Organ-on-Chip Systems with Sensors: integration, Fabrication, Challenges, and Potential. ACS Biomater. Sci. Eng. 7, 2926–2948. doi:10.1021/acsbiomaterials.0c01110
Gaut, L., Bonnin, M. A., Blavet, C., Cacciapuoti, I., Orpel, M., Mericskay, M., et al. (2020). Mechanical and molecular parameters that influence the tendon differentiation potential of C3H10T1/2 cells in 2D- and 3D-culture systems. Biol. Open 9, bio047928. doi:10.1242/bio.047928
Grün, C., Pfeifer, J., Liebsch, G., and Gottwald, E. (2023). O2-sensitive microcavity arrays: A new platform for oxygen measurements in 3D cell cultures. Front. Bioeng. Biotechnol. 11, 1111316. doi:10.3389/fbioe.2023.1111316
Holtfreter, J. (1944). A study of the mechanics of gastrulation. J. Exp. Zool. 95, 171–212. doi:10.1002/jez.1400950203
Hu, H., Gehart, H., Artegiani, B., C, L. O.-I., Dekkers, F., Basak, O., et al. (2018). Long-Term Expansion of Functional Mouse and Human Hepatocytes as 3D Organoids. Cell 175, 1591–1606.e19. doi:10.1016/j.cell.2018.11.013
Jamieson, D., and Van Den Brenk, H. A. (1965). Electrode size and tissue pO2 measurement in rats exposed to air or high pressure oxygen. J. Appl. Physiol. 20, 514–518. doi:10.1152/jappl.1965.20.3.514
Jelic, M. D., Mandic, A. D., Maricic, S. M., and Srdjenovic, B. U. (2021). Oxidative stress and its role in cancer. J. Cancer Res. Ther. 17, 22–28. doi:10.4103/jcrt.JCRT_862_16
Kapałczyńska, M., Kolenda, T., Przybyła, W., Zajączkowksa, M., Teresiak, A., Filas, V., et al. (2018). 2D and 3D cell cultures - a comparison of different types of cancer cell cultures. Arch. Med. Sci. 14, 910–919. doi:10.5114/aoms.2016.63743
Keeley, T. P., and Mann, G. E. (2019). Defining Physiological Normoxia for Improved Translation of Cell Physiology to Animal Models and Humans. Physiol. Rev. 99, 161–234. doi:10.1152/physrev.00041.2017
Koutsouras, D. A., Perrier, R., Villarroel Marquez, A., Pirog, A., Pedraza, E., Cloutet, E., et al. (2017). Simultaneous monitoring of single cell and of micro-organ activity by PEDOT:PSS covered multi-electrode arrays. Mat. Sci. Eng. C Mat. Biol. Appl. 81, 84–89. doi:10.1016/j.msec.2017.07.028
Lee, J., Rabbani, C. C., Gao, H., Steinhart, M. R., Woodruff, B. M., Pflum, Z. E., et al. (2020). Hair-bearing human skin generated entirely from pluripotent stem cells. Nature 582, 399–404. doi:10.1038/s41586-020-2352-3
Li, D. W., He, F. L., He, J., Deng, X., Liu, Y. L., Liu, Y. Y., et al. (2017). From 2D to 3D: the morphology, proliferation and differentiation of MC3T3-E1 on silk fibroin/chitosan matrices. Carbohydr. Polym. 178, 69–77. doi:10.1016/j.carbpol.2017.09.035
Li, X., and Tian, T. (2018). Recent advances in an organ-on-a-chip: biomarker analysis and applications. Anal. Methods 10, 3122–3130. doi:10.1039/C8AY00970H
Liguori, I., Russo, G., Curcio, F., Bulli, G., Aran, L., Della-Morte, D., et al. (2018). Oxidative stress, aging, and diseases. Clin. Interv. Aging 13, 757–772. doi:10.2147/cia.S158513
Man, K., Liu, J., Phan, K. M., Wang, K., Lee, J. Y., Sun, X., et al. (2022). Dimensionality-Dependent Mechanical Stretch Regulation of Cell Behavior. ACS Appl. Mat. Interfaces 14, 17081–17092. doi:10.1021/acsami.2c01266
Mora-Roldan, G. A., Ramirez-Ramirez, D., Pelayo, R., and Gazarian, K. (2021). Assessment of the Hematopoietic Differentiation Potential of Human Pluripotent Stem Cells in 2D and 3D Culture Systems. Cells 10, 2858. doi:10.3390/cells10112858
Morizane, R., Lam, A. Q., Freedman, B. S., Kishi, S., Valerius, M. T., and Bonventre, J. V. (2015). Nephron organoids derived from human pluripotent stem cells model kidney development and injury. Nat. Biotechnol. 33, 1193–1200. doi:10.1038/nbt.3392
Moscona, A. (1957). The Development in Vitro of Chimeric Aggregates of Dissociated Embryonic Chick and Mouse Cells. Proc. Natl. Acad. Sci. U. S. A. 43, 184–194. doi:10.1073/pnas.43.1.184
Neugebauer, U., Pellegrin, Y., Devocelle, M., Forster, R. J., Signac, W., Moran, N., et al. (2008). Ruthenium polypyridyl peptide conjugates: membrane permeable probes for cellular imaging. Chem. Commun. (Camb), 5307–5309. doi:10.1039/b810403d
Odijk, M., Van Der Meer, A. D., Levner, D., Kim, H. J., Van Der Helm, M. W., Segerink, L. I., et al. (2015). Measuring direct current trans-epithelial electrical resistance in organ-on-a-chip microsystems. Lab. Chip 15, 745–752. doi:10.1039/C4LC01219D
Pani, I., Sil, S., and Pal, S. K. (2023). Liquid Crystal Biosensors: A New Therapeutic Window to Point-of-Care Diagnostics. Langmuir 39, 909–917. doi:10.1021/acs.langmuir.2c02959
Puschmann, T. B., Zandén, C., De Pablo, Y., Kirchhoff, F., Pekna, M., Liu, J., et al. (2013). Bioactive 3D cell culture system minimizes cellular stress and maintains the in vivo-like morphological complexity of astroglial cells. Glia 61, 432–440. doi:10.1002/glia.22446
Richter, A., Sanford, K. K., and Evans, V. J. (1972). Influence of oxygen and culture media on plating efficiency of some mammalian tissue cells. J. Natl. Cancer Inst. 49, 1705–1712. doi:10.1093/jnci/49.6.1705
Rumsey, W. L., Vanderkooi, J. M., and Wilson, D. F. (1988). Imaging of phosphorescence: a novel method for measuring oxygen distribution in perfused tissue. Science 241, 1649–1651. doi:10.1126/science.241.4873.1649
Rybkowksa, P., Radoszkiewicz, K., Kawalec, M., Dymkowska, D., Zabłocka, B., Zabłocki, K., et al. (2023). The Metabolic Changes between Monolayer (2D) and Three-Dimensional (3D) Culture Conditions in Human Mesenchymal Stem/Stromal Cells Derived from Adipose Tissue. Cells 12, 178. doi:10.3390/cells12010178
Sarkar, S., Le, P., Geng, J., Liu, Y., Han, Z., Zahid, M. U., et al. (2020). Short-Wave Infrared Quantum Dots with Compact Sizes as Molecular Probes for Fluorescence Microscopy. J. Amer. Chem. Soc. 142, 3449–3462. doi:10.1021/jacs.9b11567
Sato, T., Vries, R. G., Snippert, H. J., Van De Wetering, M., Barker, N., Stange, D. E., et al. (2009). Single Lgr5 stem cells build crypt-villus structures in vitro without a mesenchymal niche. Nature 459, 262–265. doi:10.1038/nature07935
Semenza, G. L. (2014). Oxygen Sensing, Hypoxia-Inducible Factors, and Disease Pathophysiology. Ann. Rev. Pathol. 9, 47–71. doi:10.1146/annurev-pathol-012513-104720
Shin, H., Choi, B. H., Shim, O., Kim, J., Park, Y., Cho, S. K., et al. (2023). Single test-based diagnosis of multiple cancer types using Exosome-SERS-AI for early stage cancers. Nat. Commun. 14, 1644. doi:10.1038/s41467-023-37403-1
Smirnova, L., Caffo, B. S., Gracias, D. H., Huang, Q., Morales Pantoja, I. E., Tang, B., et al. (2023). Organoid intelligence (OI): the new frontier in biocomputing and intelligence-in-a-dish. Front. Sci. 1. doi:10.3389/fsci.2023.1017235
Souza, A. G., Silva, I. B. B., Campos-Fernandez, E., Barcelos, L. S., Souza, J. B., Marangoni, K., et al. (2018). Comparative Assay of 2D and 3D Cell Culture Models: proliferation, Gene Expression and Anticancer Drug Response. Curr. Pharm. Des. 24, 1689–1694. doi:10.2174/1381612824666180404152304
Stock, K., Estrada, M. F., Vidic, S., Gjerde, K., Rudisch, A., Santo, V. E., et al. (2016). Capturing tumor complexity in vitro: comparative analysis of 2D and 3D tumor models for drug discovery. Sci. Rep. 6, 28951. doi:10.1038/srep28951
Sugimoto, S., Ohta, Y., Fujii, M., Matano, M., Shimokawa, M., Nanki, K., et al. (2018). Reconstruction of the Human Colon Epithelium In Vivo. Cell Stem Cell 22, 171–176 e5. doi:10.1016/j.stem.2017.11.012
Tidwell, T. R., Røswell, G. V., Tronstad, K. J., Søreide, K., and Hagland, H. R. (2022). Metabolic flux analysis of 3D spheroids reveals significant differences in glucose metabolism from matched 2D cultures of colorectal cancer and pancreatic ductal adenocarcinoma cell lines. Cancer Metab. 10, 9. doi:10.1186/s40170-022-00285-w
Van Der Pol, A., Van Gilst, W. H., Voors, A. A., and Van Der Meer, P. (2019). Treating oxidative stress in heart failure: past, present and future. Eur. J. Heart Fail. 21, 425–435. doi:10.1002/ejhf.1320
Wang, X. D., and Wolfbeis, O. S. (2014). Optical methods for sensing and imaging oxygen: materials, spectroscopies and applications. Chem. Soc. Rev. 43, 3666–3761. doi:10.1039/c4cs00039k
Wesseler, M. F., Johansen, M. N., Kisiltay, A., Mortensen, K. I., and Larsen, N. B. (2022). Optical 4D oxygen mapping of microperfused tissue models with tunable in vivo-like 3D oxygen microenvironments. Lab. Chip 22, 4167–4179. doi:10.1039/D2LC00063F
Weyand, B., Nohre, M., Schmalzlin, E., Stolz, M., Israelowitz, M., Gille, C., et al. (2015). Noninvasive Oxygen Monitoring in Three-Dimensional Tissue Cultures Under Static and Dynamic Culture Conditions. Biores. Open Access 4, 266–277. doi:10.1089/biores.2015.0004
Whalen, W. J., Nair, P., and Ganfield, R. A. (1973). Measurements of oxygen tension in tissues with a micro oxygen electrode. Microvasc. Res. 5, 254–262. doi:10.1016/0026-2862(73)90035-6
Wimmer, R. A., Leopoldi, A., Aichinger, M., Wick, N., Hantusch, B., Novatchkova, M., et al. (2019). Human blood vessel organoids as a model of diabetic vasculopathy. Nature 565, 505–510. doi:10.1038/s41586-018-0858-8
Woflbeis, O. S., Leiner, M. J. P., and Posch, H. E. (1986). A new sensing material for optical oxygen measurement, with the indicator embedded in an aqueous phase. Microchim. Acta 90, 359–366. doi:10.1007/BF01199278
Wolff, P., Heimann, L., Liebsch, G., Meier, R. J., Gutbrod, M., Van Griensven, M., et al. (2019). Oxygen-distribution within 3-D collagen I hydrogels for bone tissue engineering. Mat. Sci. Eng. C Mat. Biol. Appl. 95, 422–427. doi:10.1016/j.msec.2018.02.015
Wu, M. H., Urban, J. P. G., Cui, Z., and Cui, Z. F. (2006). Development of PDMS microbioreactor with well-defined and homogenous culture environment for chondrocyte 3-D culture. Biomed. Microdev. 8, 331–340. doi:10.1007/s10544-006-9597-y
Keywords: physiological oxygen concentration, in vitro, 3D cell culture, organoid, microcavity sensor arrays, optical O2 measurement
Citation: Gottwald E, Grün C, Nies C and Liebsch G (2023) Physiological oxygen measurements in vitro-Schrödinger’s cat in 3D cell biology. Front. Bioeng. Biotechnol. 11:1218957. doi: 10.3389/fbioe.2023.1218957
Received: 08 May 2023; Accepted: 29 September 2023;
Published: 11 October 2023.
Edited by:
Francesco Urciuolo, University of Naples Federico II, ItalyReviewed by:
Mario Ganau, Oxford University Hospitals NHS Trust, United KingdomTanya Amanda Camacho-Villegas, CONACYT Centro de Investigación y Asistencia en Tecnología y Diseño del Estado de Jalisco (CIATEJ), Mexico
Reiner Class, UCB Biopharma SPRL, Belgium
Copyright © 2023 Gottwald, Grün, Nies and Liebsch. This is an open-access article distributed under the terms of the Creative Commons Attribution License (CC BY). The use, distribution or reproduction in other forums is permitted, provided the original author(s) and the copyright owner(s) are credited and that the original publication in this journal is cited, in accordance with accepted academic practice. No use, distribution or reproduction is permitted which does not comply with these terms.
*Correspondence: Eric Gottwald, eric.gottwald@kit.edu