- 1Facultad de Ciencias Ambientales y Bioquímica, Área de Fisiología Vegetal, Universidad de Castilla-La Mancha, Toledo, Spain
- 2Institute for Multidisciplinary Research in Applied Biology (IMAB), Universidad Pública de Navarra, Pamplona, Spain
- 3International Research Organization for Advanced Science and Technology (IROAST), Kumamoto University, Kumamoto, Japan
The total global food demand is expected to increase up to 50% between 2010 and 2050; hence, there is a clear need to increase plant productivity with little or no damage to the environment. In this respect, biochar is a carbon-rich material derived from the pyrolysis of organic matter at high temperatures with a limited oxygen supply, with different physicochemical characteristics that depend on the feedstock and pyrolysis conditions. When used as a soil amendment, it has shown many positive environmental effects such as carbon sequestration, reduction of greenhouse gas emissions, and soil improvement. Biochar application has also shown huge benefits when applied to agri-systems, among them, the improvement of plant growth either in optimal conditions or under abiotic or biotic stress. Several mechanisms, such as enhancing the soil microbial diversity and thus increasing soil nutrient-cycling functions, improving soil physicochemical properties, stimulating the microbial colonization, or increasing soil P, K, or N content, have been described to exert these positive effects on plant growth, either alone or in combination with other resources. In addition, it can also improve the plant antioxidant defenses, an evident advantage for plant growth under stress conditions. Although agricultural residues are generated from a wide variety of crops, cereals account for more than half of the world’s harvested area. Yet, in this review, we will focus on biochar obtained from residues of the most common and relevant cereal crops in terms of global production (rice, wheat, maize, and barley) and in their use as recycled residues to stimulate plant growth. The harvesting and processing of these crops generate a vast number and variety of residues that could be locally recycled into valuable products such as biochar, reducing the waste management problem and accomplishing the circular economy premise. However, very scarce literature focused on the use of biochar from a crop to improve its own growth is available. Herein, we present an overview of the literature focused on this topic, compiling most of the studies and discussing the urgent need to deepen into the molecular mechanisms and pathways involved in the beneficial effects of biochar on plant productivity.
Introduction
The world’s population increases day by day and is expected to reach between 9.4 and 10.1 billion by 2050 (United Nations, Department of Economic and Social Affairs, Population Division, 2019). Similarly, the total global food demand is expected to increase by 35%–56% between 2010 and 2050 (van Dijk et al., 2021), requiring new means of improving agricultural production to ensure the food supply.
Biochar is a carbon-rich material derived from the pyrolysis of organic matter at high temperature with a limited oxygen supply (Lehmann and Joseph, 2009). This process transforms the organic material into variable fractions of solid (biochar), liquid (bio-oil), and gas (syngas) products. The beneficial effect of biochar or charcoal amendments on soil fertility was first described on the black-earth-like anthropogenic amazon soils known as Terra Preta (reviewed in Glaser, 2014) derived from soils enriched with black carbon (C) from incompletely burned residues. These soils had enhanced fertility attributed to higher water and nutrient holding capacity, higher pH values, and higher levels of organic matter and nutrients such as potassium (K), calcium (Ca), phosphorous (P), or nitrogen (N; Glaser et al., 2001).
Biochar production depends on the feedstock, heating rate, final temperature, and residence time used, among other parameters. Different thermal treatments can be carried out, including slow and fast pyrolysis, gasification, or torrefaction. However, high-yield and high-quality biochar can be generally obtained with a residence time of a few hours at temperatures around 400°C (Wang D. et al., 2020). Their environmental and health implications need to be analyzed in-depth in order to extend the use of a given biochar, as it can remain in the soil for hundreds of years (Kamali et al., 2022). Some of the problems associated with the use of biochar include the crystallization of organic matter due to high temperatures or the release of heavy metals and aromatic organic compounds, issues that are currently being addressed (Kamali et al., 2022). In this respect, the European Biochar Certificate (EBC) is an international secure control and assessment system that provides guidelines [European Biochar Certificate (EBC), 2012–2022] for sustainable biochar production, processing, and sale, providing customers and producers with a reliable quality standard. It includes limit values for heavy metals and other potential contaminants such as polychlorinated dibenzo-p-dioxins, furans, or polycyclic aromatic hydrocarbons, and it is updated on a regular basis to align with the ongoing development of relevant European legislation and scientific advances. Moreover, according to EBC, the feedstock used for biochar production must be free of non-organic residues, such as plastic or scrap metal, and must be free of organic pollutants, such as paints or solvents (European Biochar Certificate (EBC), (2012–2022)). In addition, and according to the International Biochar Initiative (IBI), the feedstock used should be thoroughly described, specifying for example the composition of the material or whether it was processed or not [International Biochar Initiative (IBI), 2015].
The physicochemical characteristics of biochar are mainly dependent on the feedstock and the pyrolysis conditions used (Yakout, 2017; Chandra and Bhattacharya, 2019). Fixed carbon, recalcitrance, hydrophobicity, aromaticity, pH level, and specific surface area tend to increase with increasing pyrolysis temperature (Enders et al., 2012; Kloss et al., 2012; Angin, 2013; Gai et al., 2014; Jassal et al., 2015; Cheng et al., 2017), while biochar yield, electrical conductivity, cation exchange capacity, and N, hydrogen (H) and oxygen (O) content, decrease (Gai et al., 2014; Cheng et al., 2017).
The application of biochar can benefit the agricultural sector in many ways, as reviewed in Allohverdi et al. (2021). Biochar can improve the stability and water holding capacity of soils (Basso et al., 2013), modify and control microbial soil populations (Bai et al., 2019; Kamau et al., 2019; Chew et al., 2020), and reduce the need for fertilizer as well as fertilizer leaching (Zhang et al., 2021; Zhou et al., 2021). It can also help to ameliorate drought (Yoo et al., 2020), salinity (Liang et al., 2021), and heat stress (Fahad et al., 2016) effects. In addition, it can also protect plants against pathogens (reviewed in Poveda et al., 2021). All these factors should contribute to increased crop production. Aside from agriculture, the incorporation of biochar as a soil amendment has other beneficial environmental effects, e.g., removing inorganic contaminants from soils and water, such as pharmaceuticals (Jung et al., 2015; Du et al., 2021), heavy metals, or pesticides (Cao et al., 2011; Ali et al., 2019); carbon sequestration and reduction of greenhouse gas emissions (Bi et al., 2019; Ginebra et al., 2022) or energy production (reviewed in Bhatia et al., 2021), among others.
Biochar from many different sources has been shown to be effective in improving plant growth or productivity, either from agriculture and forestry residues or from animal/human/farming wastes. Some of the non-agricultural residues used are sewage sludge (Chu et al., 2020), municipal solid waste (Bonanomi et al., 2017), poultry litter (Masud et al., 2020), manure pellet (Pokharel and Chang, 2019), or residues from the brewery industry (Manolikaki and Diamadopoulos, 2020). The forestry residues used are mainly wood (Tartaglia et al., 2020), sawdust (Bonanomi et al., 2020), and paper fiber (Prasad et al., 2018). Although agricultural residues are generated from a wide variety of crops, in this review, we will focus on biochar obtained from residues of the most common and relevant cereal crops in terms of global production (rice, wheat, maize, and barley) and their use as recycled wastes to stimulate plant growth. However, it is important to mention that biochar prepared from other crops, such as peanut (Wu et al., 2019), walnut (Khorram et al., 2018), bamboo (Wang et al., 2019b), cotton (Zhang Z. et al., 2020), sugarcane (Kumar et al., 2021), tomato (Monterumici et al., 2015), coconut (Zhao et al., 2019), soybean (Zheng et al., 2019), etc., are also reported in the literature.
Diverse mechanisms have been described to promote plant growth, including the increase in nutrient availability and uptake (Zhao et al., 2019; Xi et al., 2020; Cui et al., 2021; You et al., 2021), improved soil physicochemical properties (Huang et al., 2019; Liu et al., 2019), changes in the soil microbial populations (Hansen et al., 2017; Zheng et al., 2018), or changes in gene expression patterns which may influence plant growth (Jaiswal et al., 2020; Mehmood et al., 2020; Figure 1). All these mechanisms will be further explored in the next sections.
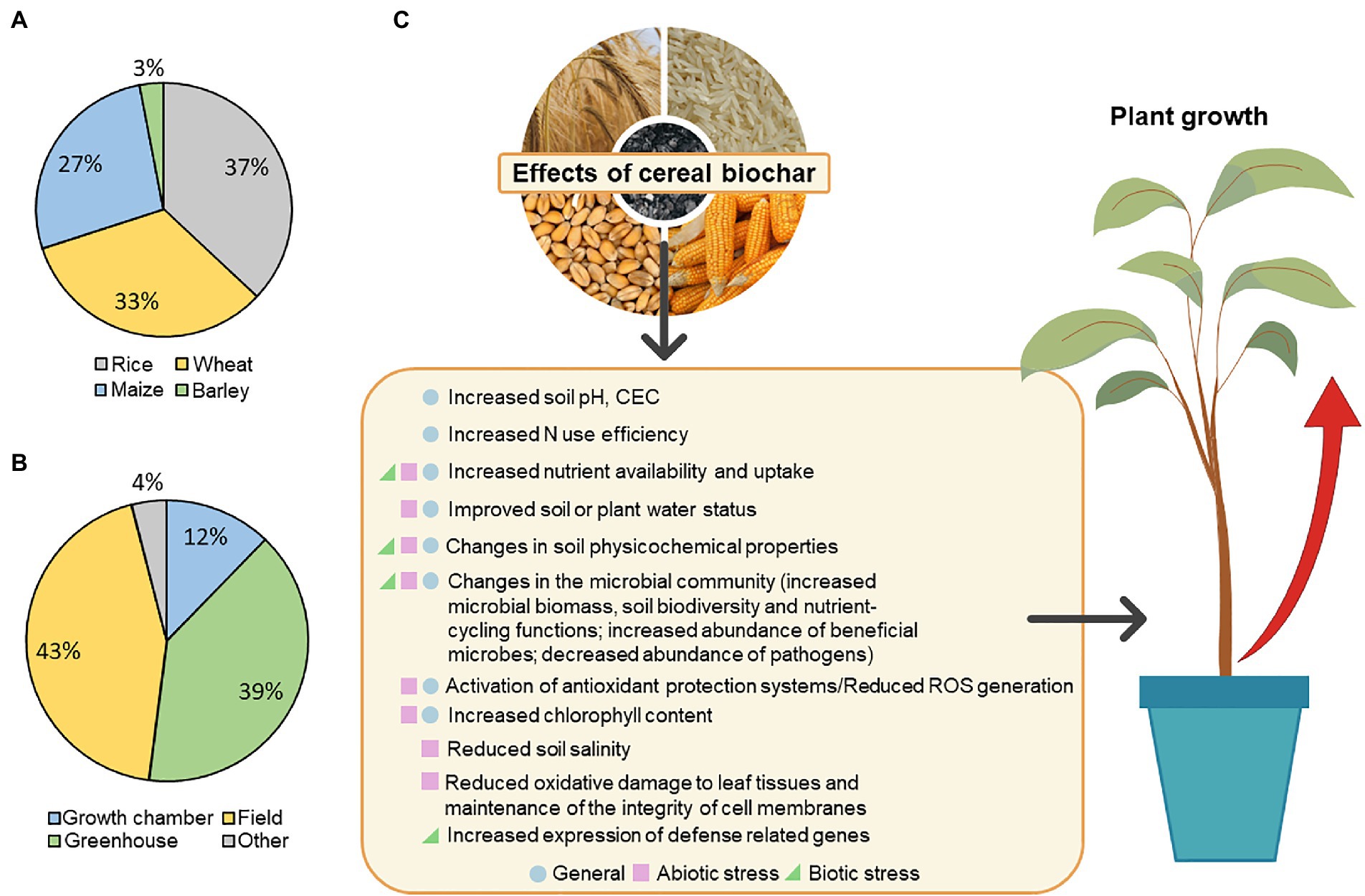
Figure 1. Meta-analyses of publications included in this review and general graphical abstract. (A) Percentage of publications on the use of biochar from each cereal (rice, wheat, maize, and barley) to stimulate plant growth. (B) Classification of the same publications according to the type of experiments performed with the different biochars. (C) Diagram representing the described effects that could influence plant growth after rice, wheat, maize, or barley biochar application.
Hence, biochar production represents a potential way of transforming residues into valuable products, reducing their waste management problem, and meeting the premise of the circular economy. As mentioned above, in this article, we will focus on the available literature on biochar produced from residues of the main cereal crops (maize, rice, wheat, and barley), and its effect on plant growth promotion, as cereals are the most produced and traded commodity in the world and account for more than half of the world’s harvested area [Food and Agriculture Organization of the United Nations (FAO), 2021a]. This type of feedstock (i.e., residues) is not in competition with land for food or feed production, which could be a relevant factor if the deployment of biochar strategies increases.
Effects of biochar from major cereal crops on plant growth
The use of biochar to stimulate or improve crop production has a great potential for the development of minimal residue circular economies in which the biochar produced is applied on the same crop that was used as feedstock. For this reason, we reviewed the current available studies of biochar made from residues of cereal crops with a high impact in terms of production within the agri-food system. The main residues used to produce biochar from cereals are husk and straw, which have been reported to stimulate plant growth either in optimal conditions or under abiotic/biotic stress. Herein, we made an overview of the available literature on this topic, compiling all the studies in Table 1 and on a meta-analysis represented in Figures 1A and 1B. It is important to mention that the impact of biochar application on abiotic/biotic stress is not considered as the focus of this review, although the cases where positive effects on plant growth were described are mentioned.

Table 1. Compilation of the most relevant studies regarding biochar production from four main cereal crops (rice, wheat, maize, and barley) and their effects on plant growth.
Biochar from rice
Rice (Oryza spp.) is one of the most important crops in the world and the primary food source for more than half of the world’s population. With 0.8 billion tons, rice accounts for 8% of global production of the primary crops, being the third after sugarcane and maize. Rice production is led by Asia, with 90% of the total global production [Food and Agriculture Organization of the United Nations (FAO), 2021a]. It is widely cultivated in South Asia, China, Thailand, Japan, and Korea and provides up to 76% of the caloric intake of people in Southeast Asia (Fitzgerald et al., 2009).
Rice processing generates different types of solid waste, the main ones being straw, husk, ash, bran, and broken rice. Rice straw accounts for 50% of the dry weight of rice; it is separated from the grain during harvest and is usually left or burned in the open field, wasting what could be a valuable resource for energy generation, ethanol production, animal feed, or stable litter. Rice husk is a protective layer on the grain (around 20% of the grain’s weight). Unlike straw, rice husk is not appropriate for animal feeding, but it can be used for power or ethanol production and as poultry litter (Moraes et al., 2014). Both residues have shown great potential for biochar production, being the two most used rice by-products. The pyrolysis temperature and residency time for biochar production in most studies usually vary from 30 min to 8 h at 400°C–800°C for rice straw and from 15 min to 3 h at 350°C–1100°C for rice husk (see Table 1). The product yield is highly dependent on the type of reaction and the temperature used, among other factors. As one example, for rice residues with reaction temperatures between 300°C and 800°C, biochar yield is around 36%–65%, while syngas and bio-oil yields are 12%–45% and 22%–45%, respectively (Dunnigan et al., 2018; Fermanelli et al., 2020; Su et al., 2020; Bhatnagar et al., 2022).
The application of rice-derived biochar has shown promising results improving plant growth and plant productivity (Table 1; Figure 1). Rice straw pyrolyzed at 400°C and applied at 2.5% (w/w) increased P and K availability and showed a positive correlation between ryegrass biomass increase and available K (Zhang et al., 2017). Similar results were obtained by Xi et al. (2020), where 2% (w/w) rice biochar application increased soil available N and K, resulting in taller lettuce plants, with longer roots, stronger leaves and stems, as well as greater leaf area. Tomato height, weight, number of flowers, and fruit yield were also improved after 7.5 t ha−1 rice husk application (Adebajo et al., 2022), and similar results were obtained in faba bean varieties with higher grain yield, fruit protein content, and plant height (Essa et al., 2021). In both cases, the growth improvement may be related to the impact of biochar on the physicochemical characteristics of the soils. Similarly, Huang et al. (2019) proposed that rice straw biochar contributed to the increase of total soil N content, making it more available to Phragmites communis and promoting its growth. But rice biochar can also stimulate C and N cycling by changing the microbial community. For example, increased rape shoot biomass (from 2.31 to 4.23 g) after rice biochar application was the result of an improvement of soil chemical conditions (soil pH and cation exchange capacity) and nutrient availability (total C and N), together with changes in the associated microbiota (Xu et al., 2014).
Rice straw or rice husk biochar has also been used in combination with a variety of other resources such as bacteria, inorganic fertilizers, vermicompost, or dredged sediments (Table 1; Figure 1). For example, Zheng et al. (2018) used biochar as an inoculum carrier for inorganic-phosphate-solubilizing bacteria (iPSB), providing a protective environment for the survival and growth of the iPSB community within the biochar pores, which consequently resulted in increased N availability, P uptake, and rape growth promotion. To study the mechanisms behind the increase in soil P availability due to biochar, Baquy et al. (2020) tested rice straw biochar combined with a P fertilizer (100 mg P kg−1) in two different soils, resulting in a significant increase of weight, P uptake and P recovery of maize plants in 2% biochar-amended soils. This increase in dry weight was due to an increased P availability, soil pH, and decreased soil exchangeable Al3+. The proposed mechanism responsible for these effects was that functional groups on the biochar surface can compete with soil PO43− to form complexes with Fe and Al, thereby increasing soil P availability and decreasing exchangeable Al3+ (and its associated toxicity). Farid et al. (2022) also described the use of co-composted biochar (70% rice straw, 15% farmyard manure, 10% rice straw biochar, and 5% mineral fertilizer) to improve zucchini growth. The co-composted biochar improved plant height, chlorophyll content, and dry weight, which could be the result of changes in soil characteristics and increased nutrient availability. Moreover, mixed application of biochar and fertilizers could be used to accelerate the restoration of ecosystems. In this respect, Huang et al. (2019) used rice straw biochar alone and with dredged sediments, where a combined application of 50% dredged sediments and 5% straw biochar led to a higher P. communis growth rate than the control with only biochar or sediments separately. In general, the addition of dredged sediments increased soil organic C (SOC) while biochar preferentially increased soil P. However, the combination of both improved the physicochemical properties of the soil and altered the rhizosphere microbial community abundance; hence, this method was proposed for the improvement of urban river revetment ecosystems.
Rice straw or rice husk biochar has been proved to be effective in fostering plant tolerance to abiotic stress (Table 1; Figure 1). In this respect, Nehela et al. (2021) used a combination of plant growth-promoting rhizobacteria (PGPR; Azotobacter chroococcum SARS 10 and Pseudomonas koreensis MG209738) and biochar to alleviate the negative effect of salt stress. This combined application led to higher values of several physicochemical parameters such as chlorophyll, carotenoids, soluble sugars, and relative water content, as well as higher nutrient content (K, N, and P) resulting in improved grain and stover yield of maize plants. A significant increase in the plant water status and tomato fruit yield was also observed by Akhtar et al. (2014) under different irrigation regimes after the application of 5% (w/w) biochar made from rice husk and shell of cotton seeds. In line with this, El Nahhas et al. (2021) tested the combined use of rice straw biochar (15 t ha−1) and exogenous jasmonic acid to alleviate the effects of salt stress on faba bean plants. The combined treatment enhanced the growth, the number of flowers, and productivity of salt-stressed plants, as well as their water status and photosynthetic pigments. These results were associated with the maintenance of the integrity of cell membranes and the reduction of the oxidative damage of leaf tissues by enhancing catalase (CAT), peroxidase (POX), superoxide dismutase (SOD), and glutathione reductase (GR) activities. In agreement with this, Kim et al. (2016) applied rice husk biochar to reclaimed tidal land soil, which often contains high levels of soluble salts and exchangeable Na. It increased the soil nutrient content and promoted plant growth since maize dry weight was 101% higher under 5% biochar than the control. The decrease in maize salt stress was attributed to a high K content in the biochar that hindered maize sodium (Na) uptake through competition. Interestingly, K content in the maize tissue was 14% higher than the control, and the decrease in Na tissue content influenced the activity of ascorbate peroxidase (APX) and GR, both genes associated with the amelioration of oxidative stress. A similar upregulation of genes involved in mitigating oxidative stress was found after the application of a mixture of rice biochar and vermicompost to minimize the effects of soil salinity and water stress on wheat plants. This treatment increased chlorophyll, proline and carotenoid content, N, P and K uptake, and relative water content of wheat plants grown under water stress (50%–75% field capacity) in a saline sodic soil. Vermicompost + biochar also increased the expression levels of CAT and APX genes, decreasing oxidative stress (Hafez et al., 2021b). Chitosan-modified biochar (CMB) was also effective relieving soybean from salt stress (NaCl 40 mM and 80 mM), as the root length of soybean plants was significantly increased (29% and 31%, respectively) after the application of unmodified biochar (UM), and it was further increased when using CMB (56% and 80%, respectively; Mehmood et al., 2020). Different mechanisms were proposed to explain the stimulation of plant growth by CMB, such as increased nutrient uptake and increased chlorophyll, soluble proteins and sugar contents, which are normally reduced under salt stress. In addition, it promoted the upregulation of antioxidant (APX, CAT, SOD, and POX) and salt-tolerance genes (CHS and GmSALT3). Therefore, CMB minimized the effects of salinity enabling plant protection (Mehmood et al., 2020).
Regarding biotic stress, the application of rice hull or rice husk biochar has reported interesting results (Table 1; Figure 1). A combination of rice husk biochar and biocontrol agents (B. subtilis and Trichoderma harzianum) was able to enhance the biomass of tomato plants and reduce Meloidogyne incognita infection by triggering defense-related genes (PR-1b and JERF3; Arshad et al., 2021). Rice biochar has also been helpful in alleviating the effects of the replanting disease (mainly caused by the accumulation of soil-borne pathogens; Wu et al., 2022). In this respect, an application of 80 g k−1 of rice husk biochar resulted in higher root length, surface area, and volume of apple tree seedlings, reducing the negative effect of the apple replant disease, and actively suppressing Fusarium solani infection (Wang et al., 2019a). In a similar way, the combination of rice hull biochar and plant growth-promoting rhizobacteria led to increased leaf area and biomass of Radix pseudostellariae, stimulated soil beneficial organisms, and suppressed pathogens through the increased production of soil metabolites, thus alleviating the effects of the replanting disease (Wu et al., 2022).
The use of biochar from rice residues to promote its own growth
On-site rice biochar application to grow rice (Table 1; in bold; Figure 1) is a returning strategy that contributes in some way to the circular economy, and several studies have indicated its positive effect on rice growth and productivity.
Influence of rice biochar on soil biological and chemical properties, nutrient availability, and nutrient uptake for rice growth
The continuous application of 20 t ha−1 of rice biochar to a rice field resulted in plant growth promotion and an increase of 14%–26% in soil N uptake, 7%–11% in internal N use efficiency, and a 6% in grain yield (Huang et al., 2018). Bi et al. (2019) showed an enhanced yield (up to 35%) in different rice-wheat rotated soils, probably due to the release of plant macronutrients and micronutrients contained in the rice biochar. Interestingly, biochar-extracted liquor [1%–5% (w/w) in water] also promoted plant height and root growth in rice seedlings. The mechanism of action proposed was based on the overexpression of the ABP1 gene and the accumulation of its protein product. Accordingly, molecular modeling showed a molecule on the biochar surface that was able to interact with the ABP1 protein, although it was not experimentally proven (Yang et al., 2019). ABP1 was formerly believed as an auxin binding protein with main roles in embryogenesis and postembryonic shoot and root development. However, more recent discoveries showed that abp1-null mutants were not compromised for auxin signaling or development, questioning the physiological significance of biochar auxin-binding capacity (Gelová et al., 2021). As previously described, rice biochar can also exert its effects on plant growth by modifying the microbial community of the soil (Table 1). In this respect, Nan et al. (2020) reported a clear improvement in soil bacterial cooperative relationships after treatment with rice biochar in a four-year field trial. The complexity of the rhizosphere bacterial community was enhanced, most probably due to an increase in total soil C content, alongside with an increased total N content and soil available K and magnesium (Mg), which increased rice yield up to 14.5%. Rice biochar has also been used in combination with other resources to promote rice growth. For example, Win et al. (2019) combined rice husk biochar with a biofertilizer (Bacillus pumilus strain TUAT-1) to study its influence in two rice genotypes. The biochar-only treatment increased soil total C, C/N ratio, exchangeable K+, chlorophyll content, and grain yield, and a positive combined effect of biochar and biofertilizer was observed for plant nutrient uptake, although it was genotype-dependent. Zheng et al. (2020) combined 50% bare urea with 50% controlled-release urea and 7.5 t ha−1 of rice straw biochar, resulting in an increase of the yield and N uptake of rice by 71.5% and 91.1%, respectively, when compared to the biochar only treatment, and 10.2% and 7.4% when compared to the same treatment with no biochar. In a similar way, Yin et al. (2021) observed an increased rice yield (38%–41%) after the application of N-enriched rice straw and waste wood biochar, due to increased levels of soil C and N contents, as well as iron (Fe) availability. Liu Z. et al. (2021) combined 1.0% (w/w) rice straw biochar with a N-fertilizer, which enhanced the N use efficiency of rice plants and resulted in increased shoot and root biomass (26%–29%) and grain yield (34%), as well as an enhanced soil microbial biomass.
Rice biochar to improve rice tolerance to abiotic stresses
Rice biochar has also been used to ameliorate abiotic stress in rice. In one of the very few studies available, 2.5% (w/w) rice husk biochar was combined with a P fertilizer. This combination was more effective to alleviate the effects of high temperature (32°C–35°C vs. a control temperature of 28°C) than biochar or the fertilizer alone. The main reason for this increase was an improvement in the plant water status (Fahad et al., 2016). Moreover, the dual application of rice husk + maize stalk biochar and exogenous glycine betaine significantly enhanced the growth, physiology, productivity, grain quality, and osmotic stress tolerance of rice plants, as well as nutrient uptake and soil properties, probably due to the activation of the enzymatic antioxidant machinery, i.e., improved activity of antioxidant enzymes including CAT, APX, and POX (Hafez et al., 2021a).
Biochar from wheat
Wheat (Triticum spp.) is one of the first domesticated food crops, being for 8,000 years the main food supply for the major civilizations of Europe, West Asia and North Africa. Nowadays, it is still one of the most widely grown cereals worldwide (more than 219 million ha), being a primary source of nutrients for around 40% of the world’s population. Moreover, as a consequence of its agronomic adaptability, simple storage, and easy conversion of grain into flour, its world trade is greater than for all other crops combined (Giraldo et al., 2019; Food and Agriculture Organization of the United Nations (FAO), 2021b).
Wheat cultivation worldwide produces different types of harvest wastes, mainly husks, and straw (Duque-Acevedo et al., 2020). Throughout the 20th century, the main use of these wastes was as animal feed or stable litter, but other alternatives emerged, such as their incorporation into the field as an amendment by burial (Duque-Acevedo et al., 2020). In the 21st century, new lines of research were developed to obtain products derived from their cellulosic biomass, like bioethanol (Duque-Acevedo et al., 2020).
Wheat harvest residues have been used as raw material to obtain biochar, showing interesting benefits after its application in agricultural systems. The main raw material used for biochar production is wheat straw, although its pyrolysis processing varies from 30 min at 350°C to 8 h at 750°C for most studies (see Table 1). For this type of feedstock and reaction temperatures of 300°C–700°C, biochar production is generally around 16%–47%, while syngas and bio-oil yields are 10%–46% and 4%–52%, respectively (Sanna et al., 2011; Fermanelli et al., 2020; Bhatnagar et al., 2022). The application of biochar from wheat straw to crops other than wheat has reported promising results in terms of plant growth and productivity (Table 1; Figure 1). For example, the application of up to 10% (v/v) of wheat straw biochar increased P uptake in barley plants in controlled conditions (Shepherd et al., 2017), and in maize plants grown in rhizoboxes (application rate of 15 g kg−1), with an increased shoot biomass and N use efficiency due to a fine root proliferation and an increase in the amount of N and P in soil (Song et al., 2020). Moreover, the application of this type of biochar in greenhouse pots provided a higher grain yield for rice plants by increasing the soil N content (Lu et al., 2020). In the field, the application of wheat straw biochar (5–40 t ha−1) promoted the growth of Lens culinaris (lentil) by increasing the organic C content and improving other physicochemical properties of the soil (Khorram et al., 2018), and it was also able to increase maize yield by 23.7% by promoting N uptake (Zhang et al., 2016). In addition, the application of biochar from wheat straw (20 t ha−1) in rice fields increased yield by 17%, as a consequence of a higher N and P supply, together with an improvement of more than 10% in the N use efficiency (Liu Y. et al., 2021; Liu M. et al., 2022). The application of 2% (w/w) wheat straw biochar to tobacco plants grown in greenhouses enhanced the rhizosphere C and N stocks and P and K availability, but caused a negative effect on the aboveground N-pool (Liu X. et al., 2022). Interestingly, recent reports point to a relevant mechanism that contributes to the increase in rice biomass and N and P uptake, after treatment with wheat straw biochar (micron-size biochar particles). Yet, a more negative electrical potential at the root epidermal cell layer than at the root surface is created, and this difference may have been the driving force for mineral nutrient absorption (Chew et al., 2020, 2022). Nevertheless, the use of biochar in promoting plant growth can also have other side beneficial effects for the environment. For example, the application of biochar from wheat straw and peanut shell (1:1, v/v) allowed the restoration of degraded soils such as landfill soils, by favoring soil biodiversity and nutrient-cycling functions (Chen et al., 2018), therefore improving plant productivity, species richness, and diversity.
Wheat straw biochar can also be used in combination with other resources (Table 1). In maize plants, wheat straw biochar together with compost and biogas slurry, significantly increased plant height, chlorophyll content, water use efficiency, and grain weight, due to an increase in P, K, N and microbial biomass in the soil (Abbas et al., 2020). When applied together with ammonium nitrate, this biochar increased N content and enzyme activities in the soil, improving the height, chlorophyll content, photosynthetic rate, and N, P, Fe, and K accumulation in Carya illinoinensis (pecan; Hou et al., 2020).
Under abiotic stress situations, wheat straw biochar promotes tolerance of different crops (Table 1; Figure 1). In this respect, an increased nutrient supply to plants can improve their tolerance against abiotic stresses, e.g., in tomato plants, wheat biochar amendment increased vegetative growth, yield, and quality parameters under saline irrigation, due to the adsorption of Na+ ions and the release of K+, Ca+2 and Mg+2 (She et al., 2018). Similarly, the application of wheat straw biochar in soybean plants subjected to salinity and drought increased the N content in the soil, favoring plant growth (Zhang Y. et al., 2020). Another mechanism through which wheat straw biochar can increase plant tolerance to drought is the improvement of soil hydrophysical properties (soil water content, bulk density, and water-holding capacity) reported in tobacco plants (Liu X. et al., 2021).
Regarding biotic stress (Table 1; Figure 1), the use of wheat biochar has also reported interesting results. In tomato, the use of wheat straw biochar reduced the disease incidence of bacterial wilt caused by Ralstonia solanacearum by up to 75%. This was due to an increase in the diversity and activity of rhizosphere microorganisms, together with alterations of the rhizosphere organic acid and amino acid composition. In addition, this increased microbial rhizosphere activity led to an increased supply of N and P to the plants, resulting in an increased plant biomass and length (Tian et al., 2021).
The use of biochar from wheat residues to promote its own growth
The use of wheat straw biochar in wheat crops (Table 1; in bold) is overall an interesting strategy in the context of a minimal-waste circular economy. In this respect, reusing wheat residues in form of biochar can stimulate wheat growth, likely as a result of favoring the microbial diversity of the soil (Meng et al., 2019; Hu et al., 2021). It can also produce a significant increase in shoot and root biomass, probably as a consequence of a higher P uptake due to a stimulation of mycorrhizal root colonization (Solaiman et al., 2019). In the field, improvements have also been reported in the fungal community structure of soils where wheat straw biochar has been applied, due to a better soil aggregation (Bai et al., 2019). Similarly, improvements in bacteria and protist populations, leading to an increase in K available for wheat plants, were also described following wheat biochar application (Hansen et al., 2017). Moreover, biochar can ameliorate the toxic effects of some persistent herbicides such as fomesafen. It is used to control pre- and post-emergent weeds in crop fields such as soybean and peanut. However, it is toxic to some cereals as wheat, usually used as a rotational crop. The use of biochar from wheat reduced the uptake of this herbicide in wheat, therefore decreasing its toxicity (Meng et al., 2019). However, specific studies should be carried out to adjust the herbicide doses in those complex agri-systems.
Interestingly, the agricultural benefits obtained from the use of wheat biochar as an amendment has led to the development of new products compatible with a sustainable agriculture. One of the recent examples is the formulation of nano-biochar particles with wheat straw biochar and different salts of N, P, K, Ca, Fe, Na, chlorine (Cl) and zinc (Zn), obtaining a nano-fertilizer with a high water-retention capacity and prolonged release of nutrients (Khan et al., 2021).
Biochar from maize
Maize (Zea mays L.) is an important source of carbohydrates for human diets in developing countries and for animal feed in the developed world (Ngoune-Tandzi and Mutengwa, 2020). The annual productivity of maize at present is 1.1 billion tons, being 12% of the total world annual crop production (Food and Agriculture Organization of the United Nations (FAO), 2021a). Maize cultivation worldwide produces mainly husks and straw as harvest wastes, which have been historically used as animal feed, stable litter, soil amendment, or cellulosic biomass for ethanol production (Duque-Acevedo et al., 2020).
Maize harvest residues have been used as raw material to obtain biochar with interesting agricultural benefits (Table 1; Figure 1). In this sense, the main raw material used to obtain biochar from maize residues is straw, varying their processing from pyrolysis for 1 h at 350° (Ndiate et al., 2021) to 30 min at 600° (Egamberdieva et al., 2021; see Table 1). The amount of biochar obtained can change depending on the pyrolysis temperature and the type of thermal treatment used. However, for temperatures between 300°C and 684°C, biochar yield is generally 23%–42%, while syngas and bio-oil yields are 39%–50% and 25%–36%, respectively (Zhu et al., 2015; Chen et al., 2016). As a representative example of the actual production of biochar from cereals, in the case of maize, the production of plant biomass residues is around 8 tons per hectare (Bahri et al., 2018). Considering the use of 50% of those residues for biochar production, as the return of some unprocessed residues is important to maintain the soil organic carbon level in the original soil, and also considering that the pyrolysis process would reduce about one-third the original biomass weight, we would obtain between 1 and 1.5 tons of biochar per hectare of maize. However, as mentioned before, exact biochar yield calculations are almost impossible as it depends on many parameters, and they should therefore be locally assessed considering all the different variables involved.
Interestingly, plant growth promotion could be a consequence of bacterial growth in the rhizosphere following the application of maize stover biochar. This is due to an increased nutrient availability due to the nutritional contribution of the biochar and the microhabitats created within its particles (Liu et al., 2017). In this sense, maize straw biochar increased the biomass of rice plants in the field due to an enhanced number of Fe-reducing bacteria and phosphate-solubilizing bacteria in the soil (Xu et al., 2019). In addition, biochar from maize cob or straw was also capable of promoting plant growth in ryegrass fields by increasing P and K uptake in plants, without the identified action of rhizosphere bacteria (Zhang et al., 2017), or in fields of soybean due to increased SOC content (Li et al., 2020). Furthermore, water extracts from maize biochar could act as a bio-stimulator at a low dosage under hydroponic conditions, as low molecular weight organic acids and nanoparticles contained in the biochar can promote root growth (Liu M. et al., 2021).
The combined use of maize biochar with microbial inoculants can also result in growth benefits for different crops. For example, 18% increase in lettuce dry biomass was achieved through the combined application of biochar from maize seeds and the microorganisms Klebsiella sp. (bacteria), Talaromyces calidicanius and T. purpureogenus (fungi), due to a direct supply of N, P and indole-3-acetic acid (IAA) to the plant by the microorganisms (Ma et al., 2022). Under abiotic stresses, such as drought and salinity, the application of biochar from maize has reported significant increases in plant tolerance (Table 1). In quinoa plants, maize cob biochar increased the plant antioxidant machinery, reducing the accumulation of reactive oxygen species (ROS) and increasing nutrient uptake under drought and salinity stress (Ramzani et al., 2017). However, in licorice plants grown with maize biochar in growth chambers, the increase of plant tolerance under salt stress was a consequence of an increased soil microbial enzymatic activity and nutrient supply to the plant (Egamberdieva et al., 2021).
Under biotic stress, the use of maize biochar can also improve crop responses (Table 1; Figure 1). In pepper, the application of biochar from maize stalk reduced the incidence of Phytophthora blight (caused by Phytophthora capsica) by up to 50%, due to an increase in the abundance and diversity of biocontrol fungi within the genus Aspergillus, Chaetomium and Trichoderma. In addition, this biochar also improved soil qualities related to plant growth and development by increasing soil organic matter and N, P, and K content (Wang G. et al., 2020).
The use of biochar from maize residues to promote its own growth
As mentioned for other crops, the use of biochar from maize harvest residues in maize crops (Table 1; in bold) has a great potential for the development of circular economies. In this way, the application of biochar from maize straw in the field caused a significant increase in the productivity of maize for several years, due to increased SOC and improved soil aggregation (Jin et al., 2020). This enhanced maize productivity could be directly related to a promotion of the photosynthetic rate and an increase in the N utilization rate and water holding capacity of the soil (Feng et al., 2021; Xia et al., 2022). The use of biochar from maize can also be a good strategy to increase the tolerance of maize crops under abiotic stress. One of the most recent studies showed that the application of maize straw biochar under salinity and drought conditions increased the biomass of maize plants by more than 60%, as a consequence of an increase in plant antioxidant activity and nutrient supply (Ndiate et al., 2021).
Biochar from barley
Barley (Hordeum vulgare) is one of the most important cereal crops in the world. In 2020, the global barley production was 157 million tons, with 5 million ha cultivated [Food and Agriculture Organization of the United Nations (FAO), 2021a]. Europe accounts for 61.7% of global barley production [Food and Agriculture Organization of the United Nations (FAO), 2021c], being the third most cultivated cereal in the continent. Barley is primarily used for animal feeding and the brewing industry, with a very low percentage used for human food. Barley processing generates different by-products derived from processes such as pearling, milling, or malting. These by-products (straw, pearling by-products, barley middling’s, hulls, fiber, malt sprouts, etc.) are mainly used for animal feed or stable litter, ethanol production, incorporation in pasta, bread formulations, etc. (Papageorgiou and Skendi, 2018).
Barley straw and spent grain are the main by-products from which biochar is produced. The amount of biochar obtained from these feedstocks depends on the type of thermal reaction and parameters used. However, at pyrolysis temperatures of 460°C–540°C, biochar yield is around 15%–22%, while syngas and bio-oil yields are 37%–44% and 39%–48%, respectively (Sanna et al., 2011). Different studies have shown the potential of barley biochar to improve plant growth (Table 1; Figure 1). In this way, application of 10 t ha−1 of barley straw biochar produced by pyrolysis at 400°C had significant positive effects on soil physicochemical properties and increased Chinese cabbage yield in a field experiment (Kang et al., 2018). In addition, after a combined application of biochar + fertilizer, the fresh weight of Chinese cabbage was significantly increased by 112% and 28.5% when compared to the control and the biochar-only treatments, respectively. Similarly, several biometric parameters, such as the leaf number, mean width and length of cabbage, as well as the N, P and K uptake were also promoted under biochar + fertilizer treatments compared to the non-treated control and the biochar-only treatment (Kang et al., 2018). In a similar study, Kang et al. (2019) studied the optimal conditions for biochar application, which were identified as an optimal rate of 20 t ha−1, 14 days before rice planting. The results of the biochar application, alone or combined with the inorganic fertilizer under those optimal conditions, significantly increased the culm lengths of rice, the number of grains per panicle, the 1,000 grain weight, and rice yield. Yet, the combined application of biochar + fertilizer had greater effects on all measured parameters than the single application of biochar or a fertilizer alone. There was no literature available on the use of biochar from barley residues for its own growth (Table 1).
Considering all the data presented in this review, the application of biochar shows a general common tendency to stimulate plant growth through several mechanisms, such as enhancing the soil microbial diversity (thus increasing the nutrient-cycling functions in the soil), improving the soil physicochemical properties, stimulating mycorrhizal colonization, increasing the P, K or N content of the soil, or improving the antioxidant defenses of the plant (an advantage under salinity, drought or heat stress; Figure 1). However, a deep knowledge of the molecular mechanisms or pathways involved in plant growth promotion stimulated by biochar is still lacking for many crops as rice, wheat, or maize, particularly when recycled for their own growth. In this respect, it is important to mention that recent data of transcriptomic profiles from tomato plants after the application of biochar from greenhouse-grown pepper, primarily indicated the upregulation of genes and pathways associated with defenses and growth, such as jasmonic acid, brassinosteroids, cytokinins, auxins, and flavonoid synthesis (Jaiswal et al., 2020). Similarly, pepper plants in soils amended with bamboo biochar showed an improvement in plant photosynthesis, energy production, enhanced stress signaling pathways, as well as plant defenses, among others. These effects were tightly coordinated with the differential expression of genes and accumulation of metabolites involved in plant–pathogen interactions, photosynthesis, phenylpropanoid biosynthesis, and protein processing in the endoplasmic reticulum (Zhu et al., 2021). Future research should contribute to increasing this mentioned knowledge in cereal crops.
Conclusion
In conclusion, a total of 67 publications centered on the use of biochar from main cereals to stimulate plant growth were analyzed, of which 37% were from rice, 33% from wheat, 27% from maize and 3% from barley (Figure 1A). The main raw material used to produce biochar was straw (66% of the total number of studies), i.e., 15 publications on rice, 21 on wheat, 6 on maize, and 2 on barley. Biochar from these main cereals has been primarily used in these studies to stimulate the growth of crops other than the ones used for biochar production (70% of all studies). Furthermore, rice accumulates the largest number of studies focused on the use of its residues as biochar to stimulate its own growth (10 out of a total of 25 publications), followed by maize and wheat (Figure 1). However, generally, biochar has been randomly applied to crops other than those used as feedstock for biochar production. There is, therefore, a general lack of information about the effects of using biochar produced from the same crop. Regarding the type of experiments carried out (Figure 1B), the majority of them were conducted in the field (43%) or greenhouses (39%), a relevant sign of the potential extrapolation of these results for their future application in established agricultural systems.
Hence, biochar is a precious product that can be used as soil amendment with many positive environmental effects such as carbon sequestration, reduction of greenhouse gas emissions, soil improvement, or plant growth promotion. However, in-depth scientific research is still needed in order to be able to apply agricultural cereal residues transformed in biochar locally, which would be compatible with a circular economy. Furthermore, research on how biochar produced from cereal crops can be used to improve the growth of that same crop is still very scarce (in bold, Table 1).
Author contributions
ÁM-G and JP gathered most of the literature regarding the topic and participated in the writing of the manuscript and the classification of the selected papers in an informative table and a figure (Table 1; Figure 1). ÁM-G made a detailed selection of the papers to focus on the topic. CE participated in the writing, supervised the manuscript structure, selected the papers, and added the critical view. All authors contributed to the article and approved the submitted version.
Funding
This work was supported by the Spanish Government (PID2019-105924RB-I00 MCIN/AEI/10.13039/501100011033 and RED2018-102407-T) and the Castilla-La Mancha Government (SBPLY/17/180501/000287 and SBPLY/21/ 180501/000033) to CE. The laboratory received support from UCLM intramural funds, and ÁM-G was recipient of a PhD grant from Fundación Tatiana Pérez de Guzmán el Bueno. EU FEDER funds complemented all the grants.
Acknowledgments
The authors thank the support received from UCLM intramural funds and EU FEDER for new equipment and maintenance that complemented all the grants. The authors also thank Fundación Tatiana Pérez de Guzmán el Bueno, as ÁM-G was recipient of a PhD grant.
Conflict of interest
The authors declare that the research was conducted in the absence of any commercial or financial relationships that could be construed as a potential conflict of interest.
Publisher’s note
All claims expressed in this article are solely those of the authors and do not necessarily represent those of their affiliated organizations, or those of the publisher, the editors and the reviewers. Any product that may be evaluated in this article, or claim that may be made by its manufacturer, is not guaranteed or endorsed by the publisher.
References
Abbas, A., Naveed, M., Azeem, M., Yaseen, M., Ullah, R., Alamri, S., et al. (2020). Efficiency of wheat straw biochar in combination with compost and biogas slurry for enhancing nutritional status and productivity of soil and plant. Plan. Theory 9, 1516. doi: 10.3390/plants9111516
Adebajo, S. O., Oluwatobi, F., Akintokun, P. O., Ojo, A. E., Akintokun, A. K., and Gbodope, I. S. (2022). Impacts of rice-husk biochar on soil microbial biomass and agronomic performances of tomato (Solanum lycopersicum L.). Sci. Rep. 12, 1787. doi: 10.1038/s41598-022-05757-z
Akhtar, S. S., Li, G., Andersen, M. N., and Liu, F. (2014). Biochar enhances yield and quality of tomato under reduced irrigation. Agr. Water Manage. 138, 37–44. doi: 10.1016/j.agwat.2014.02.016
Ali, N., Khan, S., Yao, H., and Wang, J. (2019). Biochars reduced the bioaccessibility and (bio)uptake of organochlorine pesticides and changed the microbial community dynamics in agricultural soils. Chemosphere 224, 805–815. doi: 10.1016/j.chemosphere.2019.02.163
Allohverdi, T., Mohanty, A. K., Roy, P., and Misra, M. (2021). A review on current status of biochar uses in agriculture. Molecules 26:5584 doi: 10.3390/molecules26185584
Angin, D. (2013). Effect of pyrolysis temperature and heating rate on biochar obtained from pyrolysis of safflower seed press cake. Bioresour. Technol. 128, 593–597. doi: 10.1016/j.biortech.2012.10.150
Arshad, U., Azeem, F., Mustafa, G., Bakhsh, A., Toktay, H., McGiffen, M., et al. (2021). Combined application of biochar and biocontrol agents enhances plant growth and activates resistance against Meloidogyne incognita in tomato. Gesunde Pflanz. 73, 591–601. doi: 10.1007/s10343-021-00580-4
Bahri, S., Natsir, A., Sirajuddin, S. N., and Hasan, S. (2018). Integrated agricultural system study on crops: intercropping of corn–peanut and beef cattle fattening. Int. J. Curr. Res. Biosci. Plant Biol. 5, 24–29. doi: 10.20546/ijcrbp.2018.504.004
Bai, N., Zhang, H., Li, S., Zheng, X., Zhang, J., Zhang, H., et al. (2019). Long-term effects of straw and straw-derived biochar on soil aggregation and fungal community in a rice-wheat rotation system. PeerJ 6:e6171. doi: 10.7717/peerj.6171
Baquy, M. A., Jiang, J., and Xu, R. (2020). Biochars derived from crop straws increased the availability of applied phosphorus fertilizer for maize in Ultisol and Oxisol. Environ. Sci. Pollut. R. Int. 27, 5511–5522. doi: 10.1007/s11356-019-06695-6
Basso, A. S., Miguez, F. E., Laird, D. A., Horton, R., and Westgate, M. (2013). Assessing potential of biochar for increasing water-holding capacity of sandy soils. GCB Bioenergy 5, 132–143. doi: 10.1111/gcbb.12026
Bhatia, S. K., Palai, A. K., Kumar, A., Bhatia, R. K., Patel, A. K., Thakur, V. K., et al. (2021). Trends in renewable energy production employing biomass-based biochar. Bioresour. Technol. 340:125644. doi: 10.1016/j.biortech.2021.125644
Bhatnagar, A., Singhal, A., Tolvanen, H., Valtonen, K., Joronen, T., and Konttinen, J. (2022). Effect of pretreatment and biomass blending on bio-oil and biochar quality from two-step slow pyrolysis of rice straw. Waste Manag. 138, 298–307. doi: 10.1016/j.wasman.2021.12.013
Bi, Y., Cai, S., Wang, Y., Xia, Y., Zhao, X., Wang, S., et al. (2019). Assessing the viability of soil successive straw biochar amendment based on a five-year column trial with six different soils: views from crop production, carbon sequestration and net ecosystem economic benefits. J. Environ. Manage. 245, 173–186. doi: 10.1016/j.jenvman.2019.03.051
Bonanomi, G., Ippolito, F., Cesarano, G., Nanni, B., Lombardi, N., Rita, A., et al. (2017). Biochar as plant growth promoter: better off alone or mixed with organic amendments? Front. Plant Sci. 8:1570. doi: 10.3389/fpls.2017.01570
Bonanomi, G., Zotti, M., Idbella, M., Di Silverio, N., Carrino, L., Cesarano, G., et al. (2020). Decomposition and organic amendments chemistry explain contrasting effects on plant growth promotion and suppression of Rhizoctonia solani damping off. PLoS One 15:e0230925. doi: 10.1371/journal.pone.0230925
Cao, X., Ma, L., Liang, Y., Gao, B., and Harris, W. (2011). Simultaneous immobilization of lead and atrazine in contaminated soils using dairy-manure biochar. Environ. Sci. Technol. 45, 4884–4889. doi: 10.1021/es103752u
Chandra, S., and Bhattacharya, J. (2019). Influence of temperature and duration of pyrolysis on the property heterogeneity of rice straw biochar and optimization of pyrolysis conditions for its application in soils. J. Clean. Prod. 215, 1123–1139. doi: 10.1016/j.jclepro.2019.01.079
Chen, T., Liu, R., and Scott, N. R. (2016). Characterization of energy carriers obtained from the pyrolysis of white ash, switchgrass and corn stover—Biochar, syngas and bio-oil. Fuel Process. Technol. 142, 124–134. doi: 10.1016/j.fuproc.2015.09.034
Chen, X. W., Wong, J. T. F., Chen, Z. T., Tang, T. W. L., Guo, H. W., Leung, A. O. W., et al. (2018). Effects of biochar on the ecological performance of a subtropical landfill. Sci. Total Environ. 644, 963–975. doi: 10.1016/j.scitotenv.2018.06.379
Cheng, H., Jones, D. L., Hill, P., Bastami, M. S., and Tu, C. I. (2017). Influence of biochar produced from different pyrolysis temperature on nutrient retention and leaching. Arch. Agr. Soil Sci. 64, 850–859. doi: 10.1080/03650340.2017.1384545
Chew, J., Joseph, S., Chen, G., Zhang, Y., Zhu, L., Liu, M., et al. (2022). Biochar-based fertilizer enhances nutrient uptake and transport in rice seedlings. Sci. Total Environ. 826:154174. doi: 10.1016/j.scitotenv.2022.154174
Chew, J., Zhu, L., Nielsen, S., Graber, E., Mitchell, D. R., Horvat, J., et al. (2020). Biochar-based fertilizer: supercharging root membrane potential and biomass yield of rice. Sci. Total Environ. 713:136431. doi: 10.1016/j.scitotenv.2019.136431
Chu, Q., Xue, L., Singh, B. P., Yu, S., Müller, K., Wang, H., et al. (2020). Sewage sludge-derived hydrochar that inhibits ammonia volatilization, improves soil nitrogen retention and rice nitrogen utilization. Chemosphere 245:125558. doi: 10.1016/j.chemosphere.2019.125558
Cui, Q., Xia, J., Yang, H., Liu, J., and Shao, P. (2021). Biochar and effective microorganisms promote Sesbania cannabina growth and soil quality in the coastal saline-alkali soil of the Yellow River Delta, China. Sci. Total Environ. 756:143801. doi: 10.1016/j.scitotenv.2020.143801
Du, Y., Zhang, X., Shu, L., Feng, Y., Lv, C., Liu, H., et al. (2021). Safety evaluation and ibuprofen removal via an Alternanthera philoxeroides-based biochar. Environ. Sci. Pollut. Res. Int. 28, 40568–40586. doi: 10.1007/s11356-020-09714-z
Dunnigan, L., Ashman, P. J., Zhang, X., and Kwong, C. W. (2018). Production of biochar from rice husk: particulate emissions from the combustion of raw pyrolysis volatiles. J. Clean. Prod. 172, 1639–1645. doi: 10.1016/j.jclepro.2016.11.107
Duque-Acevedo, M., Belmonte-Urena, L. J., Cortés-García, F. J., and Camacho-Ferre, F. (2020). Agricultural waste: review of the evolution, approaches and perspectives on alternative uses. Glob. Ecol. Conserv. 22:e00902. doi: 10.1016/j.gecco.2020.e00902
Egamberdieva, D., Ma, H., Alaylar, B., Zoghi, Z., Kistaubayeva, A., Wirth, S., et al. (2021). Biochar amendments improve licorice (Glycyrrhiza uralensis Fisch.) growth and nutrient uptake under salt stress. Plan. Theory 10, 2135. doi: 10.3390/plants10102135
El Nahhas, N., AlKahtani, M. D., Abdelaal, K. A., Al Husnain, L., AlGwaiz, H. I., Hafez, Y. M., et al. (2021). Biochar and jasmonic acid application attenuates antioxidative systems and improves growth, physiology, nutrient uptake and productivity of faba bean (Vicia faba L.) irrigated with saline water. Plant Physiol. Biochem. 166, 807–817. doi: 10.1016/j.plaphy.2021.06.033
Enders, A., Hanley, K., Whitman, T., Joseph, S., and Lehmann, J. (2012). Characterization of biochars to evaluate recalcitrance and agronomic performance. Bioresour. Technol. 114, 644–653. doi: 10.1016/j.biortech.2012.03.022
Essa, R. E., Afifi, A. A., El-Ashry, S. M., and Ahmed, M. A. (2021). Rice straw biochar application and its impact on yield of some faba bean varieties in sandy soil. Pak. J. Biol. Sci. 24, 1236–1245. doi: 10.3923/pjbs.2021.1236.1245
European Biochar Certificate (EBC) (2012–2022). Guidelines for a Sustainable Production of Biochar. European Biochar Foundation, Arbaz, Switzerland. Available at: https://www.european-biochar.org/en/ct/2-EBC-guidelines-documents (Version 10.1 from 10th Jan 2022, Accessed 20 May 2022).
Fahad, S., Hussain, S., Saud, S., Hassan, S., Tanveer, M., Ihsan, M. Z., et al. (2016). A combined application of biochar and phosphorus alleviates heat-induced adversities on physiological, agronomical and quality attributes of rice. Plant Physiol. Biochem. 103, 191–198. doi: 10.1016/j.plaphy.2016.03.001
Farid, I. M., Siam, H. S., Abbas, M. H., Mohamed, I., Mahmoud, S. A., Tolba, M., et al. (2022). Co-composted biochar derived from rice straw and sugarcane bagasse improved soil properties, carbon balance, and zucchini growth in a sandy soil: a trial for enhancing the health of low fertile arid soils. Chemosphere 292:133389. doi: 10.1016/j.chemosphere.2021.133389
Feng, W., Yang, F., Cen, R., Liu, J., Qu, Z., Miao, Q., et al. (2021). Effects of straw biochar application on soil temperature, available nitrogen and growth of corn. J. Environ. Manage. 277:111331. doi: 10.1016/j.jenvman.2020.111331
Fermanelli, C. S., Córdoba, A., Pierella, L. B., and Saux, C. (2020). Pyrolysis and copyrolysis of three lignocellulosic biomass residues from the agro-food industry: A comparative study. Waste Manag. 102, 362–370. doi: 10.1016/j.wasman.2019.10.057
Fitzgerald, M. A., McCouch, S. R., and Hall, R. D. (2009). Not just a grain of rice: the quest for quality. Trends Plant Sci. 14, 133–139. doi: 10.1016/j.tplants.2008.12.004
Food and Agriculture Organization of the United Nations (FAO) (2021a). World Food and Agriculture—Statistical Yearbook 2021. Rome, Italy. doi: 10.4060/cb4477en
Food and Agriculture Organization of the United Nations (FAO) (2021b). FAOSTAT Database. Harvested area of Wheat in World, year 2020. Available at: https://www.fao.org/faostat/en/#data/QCL (Accessed 23 March 2022).
Food and Agriculture Organization of the United Nations (FAO) (2021c). FAOSTAT Database. Production share of Barley by region, year 2020. Available at: https://www.fao.org/faostat/en/#data/QCL (Accessed 16 March 2022).
Gai, X., Wang, H., Liu, J., Zhai, L., Liu, S., Ren, T., et al. (2014). Effects of feedstock and pyrolysis temperature on biochar adsorption of ammonium and nitrate. PLoS One 9, e113888. doi: 10.1371/journal.pone.0113888
Gelová, Z., Gallei, M., Pernisová, M., Brunoud, G., Zhang, X., Glanc, M., et al. (2021). Developmental roles of Auxin binding protein 1 in Arabidopsis thaliana. Plant Sci. 303:110750. doi: 10.1016/j.plantsci.2020.110750
Ginebra, M., Muñoz, C., Calvelo-Pereira, R., Doussoulin, M., and Zagal, E. (2022). Biochar impacts on soil chemical properties, greenhouse gas emissions and forage productivity: a field experiment. Sci. Total Environ. 806, 150465. doi: 10.1016/j.scitotenv.2021.150465
Giraldo, P., Benavente, E., Manzano-Agugliaro, F., and Gimenez, E. (2019). Worldwide research trends on wheat and barley: A bibliometric comparative analysis. Agronomy 9, 352. doi: 10.3390/agronomy9070352
Glaser, B. (2014). “Soil biogeochemistry. “From molecular to ecosystem level using terra preta and biochar as examples”” in Agroecology, Ecosystems, and Sustainability. ed. N. Benkeblia (Boca Raton, FL: CRC Press), 1–40.
Glaser, B., Haumaier, L., Guggenberger, G., and Zech, W. (2001). The “Terra Preta” phenomenon: a model for sustainable agriculture in the humid tropics. Naturwissenschaften 88, 37–41. doi: 10.1007/s001140000193
Hafez, E. M., Gowayed, S. M., Nehela, Y., Sakran, R. M., Rady, A., Awadalla, A., et al. (2021a). Incorporated biochar-based soil amendment and exogenous glycine betaine foliar application ameliorate rice (Oryza sativa L.) tolerance and resilience to osmotic stress. Plants 10, 1930. doi: 10.3390/plants10091930
Hafez, E. M., Omara, A. E. D., Alhumaydhi, F. A., and El-Esawi, M. A. (2021b). Minimizing hazard impacts of soil salinity and water stress on wheat plants by soil application of vermicompost and biochar. Physiol. Plant. 172, 587–602. doi: 10.1111/ppl.13261
Hansen, V., Müller-Stöver, D., Imparato, V., Krogh, P. H., Jensen, L. S., Dolmer, A., et al. (2017). The effects of straw or straw-derived gasification biochar applications on soil quality and crop productivity: a farm case study. J. Environ. Manage. 186, 88–95. doi: 10.1016/j.jenvman.2016.10.041
Hou, Z., Tang, Y., Li, C., Lim, K. J., and Wang, Z. (2020). The additive effect of biochar amendment and simulated nitrogen deposition stimulates the plant height, photosynthesis and accumulation of NPK in pecan (Carya illinoinensis) seedlings. AoB Plants 12, plaa035. doi: 10.1093/aobpla/plaa035
Hu, Y., Sun, B., Wu, S., Feng, H., Gao, M., Zhang, B., et al. (2021). After-effects of straw and straw-derived biochar application on crop growth, yield, and soil properties in wheat (Triticum aestivum L.)-maize (Zea mays L.) rotations: a four-year field experiment. Sci. Total Environ. 780:146560. doi: 10.1016/j.scitotenv.2021.146560
Huang, M., Fan, L., Chen, J., Jiang, L., and Zou, Y. (2018). Continuous applications of biochar to rice: effects on nitrogen uptake and utilization. Sci. Rep. 8, 11461–11469. doi: 10.1038/s41598-018-29877-7
Huang, X., Li, S., Li, S., Ye, G., Lu, L., Zhang, L., et al. (2019). The effects of biochar and dredged sediments on soil structure and fertility promote the growth, photosynthetic and rhizosphere microbial diversity of Phragmites communis (Cav.) Trin. ex Steud. Sci. Total Environ. 697, 134073. doi: 10.1016/j.scitotenv.2019.134073
International Biochar Initiative (IBI) (2015). Standardized product definition and product testing guidelines for biochar that is used in soil. Available at: https://biochar-international.org/characterizationstandard/ (Accessed 20 May 2022).
Jaiswal, A. K., Alkan, N., Elad, Y., Sela, N., Philosoph, A. M., Graber, E. R., et al. (2020). Molecular insights into biochar-mediated plant growth promotion and systemic resistance in tomato against Fusarium crown and root rot disease. Sci. Rep. 10, 13934. doi: 10.1038/s41598-020-70882-6
Jassal, R. S., Johnson, M. S., Molodovskaya, M., Black, T. A., Jollymore, A., and Sveinson, K. (2015). Nitrogen enrichment potential of biochar in relation to pyrolysis temperature and feedstock quality. J. Environ. Manage. 152, 140–144. doi: 10.1016/j.jenvman.2015.01.021
Jin, L., Wei, D., Yin, D., Zhou, B., Ding, J., Wang, W., et al. (2020). Investigations of the effect of the amount of biochar on soil porosity and aggregation and crop yields on fertilised black soil in northern China. PLoS One 15:e0238883. doi: 10.1371/journal.pone.0238883
Jung, C., Boateng, L. K., Flora, J. R. V., Oh, J., Braswell, M. C., Son, A., et al. (2015). Competitive adsorption of selected non-steroidal anti-inflammatory drugs on activated biochars: experimental and molecular modeling study. Chem. Eng. J. 264, 1–9. doi: 10.1016/j.cej.2014.11.076
Kamali, M., Sweygers, N., Al-Salem, S., Appels, L., Aminabhavi, T. M., and Dewil, R. (2022). Biochar for soil applications-sustainability aspects, challenges and future prospects. Chem. Eng. J. 428:131189. doi: 10.1016/j.cej.2021.131189
Kamau, S., Karanja, N. K., Ayuke, F. O., and Lehmann, J. (2019). Short-term influence of biochar and fertilizer-biochar blends on soil nutrients, fauna and maize growth. Biol. Fertil. Soils 55, 661–673. doi: 10.1007/s00374-019-01381-8
Kang, S., Kim, S. H., Park, J. H., Seo, D. C., Ok, Y. S., and Cho, J. S. (2018). Effect of biochar derived from barley straw on soil physicochemical properties, crop growth, and nitrous oxide emission in an upland field in South Korea. Environ. Sci. Pollut. R. 25, 25813–25821. doi: 10.1007/s11356-018-1888-3
Kang, S. W., Park, J. H., Kim, S. H., Seo, D. C., Ok, Y. S., and Cho, J. S. (2019). Establishment of optimal barley straw biochar application conditions for rice cultivation in a paddy field. Environ. Geochem. Health 41, 1793–1803. doi: 10.1007/s10653-017-0009-4
Khan, H. A., Naqvi, S. R., Mehran, M. T., Khoja, A. H., Niazi, M. B. K., Juchelková, D., et al. (2021). A performance evaluation study of nano-biochar as a potential slow-release nano-fertilizer from wheat straw residue for sustainable agriculture. Chemosphere 285:131382. doi: 10.1016/j.chemosphere.2021.131382
Khorram, M., Fatemi, A., Khan, M. A., Kiefer, R., and Jafarnia, S. (2018). Potential risk of weed outbreak by increasing biochar's application rates in slow-growth legume, lentil (Lens culinaris Medik.). J. Sci. Food Agr. 98, 2080–2088. doi: 10.1002/jsfa.8689
Kim, H. S., Kim, K. R., Yang, J. E., Ok, Y. S., Owens, G., Nehls, T., et al. (2016). Effect of biochar on reclaimed tidal land soil properties and maize (Zea mays L.) response. Chemosphere 142, 153–159. doi: 10.1016/j.chemosphere.2015.06.041
Kloss, S., Zehetner, F., Dellantonio, A., Hamid, R., Ottner, F., Liedtke, V., et al. (2012). Characterization of slow pyrolysis biochars: effects of feedstocks and pyrolysis temperature on biochar properties. J. Environ. Qual. 41, 990–1000. doi: 10.2134/jeq2011.0070
Kumar, A., Singh, E., Singh, L., Kumar, S., and Kumar, R. (2021). Carbon material as a sustainable alternative towards boosting properties of urban soil and foster plant growth. Sci. Total Environ. 751:141659. doi: 10.1016/j.scitotenv.2020.141659
Latini, A., Fiorani, F., Galeffi, P., Cantale, C., Bevivino, A., and Jablonowski, N. D. (2021). Phenotyping of different italian durum wheat varieties in early growth stage with the addition of pure or digestate-activated biochars. Front. Plant Sci. 12:782072. doi: 10.3389/fpls.2021.782072
Lehmann, J., and Joseph, S. (2009). “Biochar for environmental management: an introduction,” in Biochar for Environmental Management: Science and Technology. eds. J. Lehmann and S. Joseph (London, U.K: Earthscan), 1–12.
Li, Q., Wang, M., Fu, Q., Li, T., Liu, D., Hou, R., et al. (2020). Short-term influence of biochar on soil temperature, liquid moisture content and soybean growth in a seasonal frozen soil area. J. Environ. Manage. 266:110609. doi: 10.1016/j.jenvman.2020.110609
Liang, J. F., Li, Q. W., Gao, J. Q., Feng, J. G., Zhang, X. Y., Wu, Y. Q., et al. (2021). Biochar rhizosphere addition promoted Phragmites australis growth and changed soil properties in the Yellow River Delta. Sci. Total Environ. 761:143291. doi: 10.1016/j.scitotenv.2020.143291
Liu, B., Cai, Z., Zhang, Y., Liu, G., Luo, X., and Zheng, H. (2019). Comparison of efficacies of peanut shell biochar and biochar-based compost on two leafy vegetable productivity in an infertile land. Chemosphere 224, 151–161. doi: 10.1016/j.chemosphere.2019.02.100
Liu, M., Ke, X., Liu, X., Fan, X., Xu, Y., Li, L., et al. (2022). The effects of biochar soil amendment on rice growth may vary greatly with rice genotypes. Sci. Total Environ. 810:152223. doi: 10.1016/j.scitotenv.2021.152223
Liu, Y., Li, J., Jiao, X., Li, H., Hu, T., Jiang, H., et al. (2021). Effects of biochar on water quality and rice productivity under straw returning condition in a rice-wheat rotation region. Sci. Total Environ. 819, 152063. doi: 10.1016/j.scitotenv.2021.152063
Liu, M., Lin, Z., Ke, X., Fan, X., Joseph, S., Taherymoosavi, S., et al. (2021). Rice seedling growth promotion by biochar varies with genotypes and application dosages. Front. Plant Sci. 12:1200. doi: 10.3389/fpls.2021.580462
Liu, X., Ma, Y., Manevski, K., Andersen, M. N., Li, Y., Wei, Z., et al. (2022). Biochar and alternate wetting-drying cycles improving rhizosphere soil nutrients availability and tobacco growth by altering root growth strategy in Ferralsol and Anthrosol. Sci. Total Environ. 806:150513. doi: 10.1016/j.scitotenv.2021.150513
Liu, S., Tang, W., Yang, F., Meng, J., Chen, W., and Li, X. (2017). Influence of biochar application on potassium-solubilizing Bacillus mucilaginosus as potential biofertilizer. Prep. Biochem. Biotechnol. 47, 32–37. doi: 10.1080/10826068.2016.1155062
Liu, X., Wei, Z., Ma, Y., Liu, J., and Liu, F. (2021). Effects of biochar amendment and reduced irrigation on growth, physiology, water-use efficiency and nutrients uptake of tobacco (Nicotiana tabacum L.) on two different soil types. Sci. Total Environ. 770:144769. doi: 10.1016/j.scitotenv.2020.144769
Liu, Z., Wu, X., Li, S., Liu, W., Bian, R., Zhang, X., et al. (2021). Quantitative assessment of the effects of biochar amendment on photosynthetic carbon assimilation and dynamics in a rice–soil system. New Phytol. 232, 1250–1258. doi: 10.1111/nph.17651
Lu, H., Feng, Y., Gao, Q., Xing, J., Chen, Y., Yang, L., et al. (2020). Surface soil mixing is more beneficial than the plough layer mixing mode of biochar application for nitrogen retention in a paddy system. Sci. Total Environ. 718:137399. doi: 10.1016/j.scitotenv.2020.137399
Ma, H., Shurigin, V., Jabborova, D., Cruz, J. A. D., Wirth, S., Bellingrath-Kimura, S. D., et al. (2022). The integrated effect of microbial inoculants and biochar types on soil biological properties, and plant growth of lettuce (Lactuca sativa L.). Plan. Theory 11, 423. doi: 10.3390/plants11030423
Manolikaki, I., and Diamadopoulos, E. (2020). Agronomic potential of biochar prepared from brewery byproducts. J. Environ. Manage. 255, 109856. doi: 10.1016/j.jenvman.2019.109856
Masud, M. M., Baquy, M. A., Akhter, S., Sen, R., Barman, A., and Khatun, M. R. (2020). Liming effects of poultry litter derived biochar on soil acidity amelioration and maize growth. Ecotox. Environ. Safe. 202:110865. doi: 10.1016/j.ecoenv.2020.110865
Mehmood, S., Ahmed, W., Ikram, M., Imtiaz, M., Mahmood, S., Tu, S., et al. (2020). Chitosan modified biochar increases soybean (Glycine max l.) resistance to salt-stress by augmenting root morphology, antioxidant defense mechanisms and the expression of stress-responsive genes. Plan. Theory 9, 1–25. doi: 10.3390/plants9091173
Meng, L., Sun, T., Li, M., Saleem, M., Zhang, Q., and Wang, C. (2019). Soil-applied biochar increases microbial diversity and wheat plant performance under herbicide fomesafen stress. Ecotox. Environ. Safe. 171, 75–83. doi: 10.1016/j.ecoenv.2018.12.065
Monterumici, C. M., Rosso, D., Montoneri, E., Ginepro, M., Baglieri, A., Novotny, E. H., et al. (2015). Processed vs. non-processed biowastes for agriculture: effects of post-harvest tomato plants and biochar on radish growth, chlorophyll content and protein production. Int. J. Mol. Sci. 16, 8826–8843. doi: 10.3390/ijms16048826
Moraes, C. A. M., Fernandes, I. J., Calheiro, D., Kieling, A. G., Brehm, F. A., Rigon, M. R., et al. (2014). Review of the rice production cycle: by-products and the main applications focusing on rice husk combustion and ash recycling. Waste Manag. Res. 32, 1034–1048. doi: 10.1177/0734242x14557379
Nan, Q., Wang, C., Wang, H., Yi, Q., Liang, B., Xu, J., et al. (2020). Biochar drives microbially-mediated rice production by increasing soil carbon. J. Hazard. Mater. 387, 121680. doi: 10.1016/j.jhazmat.2019.121680
Ndiate, N. I., Saeed, Q., Haider, F. U., Liqun, C., Nkoh, J. N., and Mustafa, A. (2021). Co-application of biochar and arbuscular mycorrhizal fungi improves salinity tolerance, growth and lipid metabolism of maize (Zea mays L.) in an alkaline soil. Plan. Theory 10, 2490. doi: 10.3390/plants10112490
Nehela, Y., Mazrou, Y. S., Alshaal, T., Rady, A., El-Sherif, A., Omara, A. E. D., et al. (2021). The integrated amendment of sodic-saline soils using biochar and plant growth-promoting rhizobacteria enhances maize (Zea mays L.) resilience to water salinity. Plants 10, 1960. doi: 10.3390/plants10091960
Ngoune-Tandzi, L., and Mutengwa, C. S. (2020). Estimation of maize (Zea mays L.) yield per harvest area: appropriate methods. Agronomy 10, 29. doi: 10.3390/agronomy10010029
Papageorgiou, M., and Skendi, A. (2018). “Introduction to cereal processing and by-products,” in Sustainable Recovery and Reutilization of Cereal Processing By-Products. ed. C. M. Galanakis (Sawston, Cambridge: Woodhead Publishing, Elsevier), 1–25.
Pokharel, P., and Chang, S. X. (2019). Manure pellet, woodchip and their biochars differently affect wheat yield and carbon dioxide emission from bulk and rhizosphere soils. Sci. Total Environ. 659, 463–472. doi: 10.1016/j.scitotenv.2018.12.380
Poveda, J., Martínez-Gómez, Á., Fenoll, C., and Escobar, C. (2021). The use of biochar for plant pathogen control. Phytopathology 111, 1490–1499. doi: 10.1094/phyto-06-20-0248-rvw
Prasad, M., Tzortzakis, N., and McDaniel, N. (2018). Chemical characterization of biochar and assessment of the nutrient dynamics by means of preliminary plant growth tests. J. Environ. Manage. 216, 89–95. doi: 10.1016/j.jenvman.2017.04.020
Ramzani, P. M. A., Shan, L., Anjum, S., Ronggui, H., Iqbal, M., Virk, Z. A., et al. (2017). Improved quinoa growth, physiological response, and seed nutritional quality in three soils having different stresses by the application of acidified biochar and compost. Plant Physiol. Biochem. 116, 127–138. doi: 10.1016/j.plaphy.2017.05.003
Sanna, A., Li, S., Linforth, R., Smart, K. A., and Andrésen, J. M. (2011). Bio-oil and bio-char from low temperature pyrolysis of spent grains using activated alumina. Bioresour. Technol. 102, 10695–10703. doi: 10.1016/j.biortech.2011.08.092
She, D., Sun, X., Gamareldawla, A. H., Nazar, E. A., Hu, W., and Edith, K. (2018). Benefits of soil biochar amendments to tomato growth under saline water irrigation. Sci. Rep. 8, 14743–14710. doi: 10.1038/s41598-018-33040-7
Shepherd, J. G., Joseph, S., Sohi, S. P., and Heal, K. V. (2017). Biochar and enhanced phosphate capture: mapping mechanisms to functional properties. Chemosphere 179, 57–74. doi: 10.1016/j.chemosphere.2017.02.123
Solaiman, Z. M., Abbott, L. K., and Murphy, D. V. (2019). Biochar phosphorus concentration dictates mycorrhizal colonisation, plant growth and soil phosphorus cycling. Sci. Rep. 9, 5062–5011. doi: 10.1038/s41598-019-41671-7
Song, X., Razavi, B. S., Ludwig, B., Zamanian, K., Zang, H., Kuzyakov, Y., et al. (2020). Combined biochar and nitrogen application stimulates enzyme activity and root plasticity. Sci. Total Environ. 735:139393. doi: 10.1016/j.scitotenv.2020.139393
Su, Y., Liu, L., Zhang, S., Xu, D., Du, H., Cheng, Y., et al. (2020). A green route for pyrolysis poly-generation of typical high ash biomass, rice husk: effects on simultaneous production of carbonic oxide-rich syngas, phenol-abundant bio-oil, high-adsorption porous carbon and amorphous silicon dioxide. Bioresour. Technol. 295:122243. doi: 10.1016/j.biortech.2019.122243
Tartaglia, M., Arena, S., Scaloni, A., Marra, M., and Rocco, M. (2020). Biochar administration to san Marzano tomato plants cultivated under low-input farming increases growth, fruit yield, and affects gene expression. Front. Plant Sci. 11, 1281. doi: 10.3389/fpls.2020.01281
Tian, J. H., Shuang, R. A. O., Yang, G. A. O., Yang, L. U., and Cai, K. Z. (2021). Wheat straw biochar amendment suppresses tomato bacterial wilt caused by Ralstonia solanacearum: potential effects of rhizosphere organic acids and amino acids. J. Integr. Agric. 20, 2450–2462. doi: 10.1016/S2095-3119(20)63455-4
United Nations, Department of Economic and Social Affairs, Population Division (2019). World Population Prospects 2019: Data Booklet (ST/ESA/SER.A/424).
van Dijk, M., Morley, T., Rau, M. L., and Saghai, Y. (2021). A meta-analysis of projected global food demand and population at risk of hunger for the period 2010–2050. Nat. Food 2, 494–501. doi: 10.1038/s43016-021-00322-9
Wang, D., Jiang, P., Zhang, H., and Yuan, W. (2020). Biochar production and applications in agro and forestry systems: a review. Sci. Total Environ. 723:137775. doi: 10.1016/j.scitotenv.2020.137775
Wang, G., Ma, Y., Chenia, H. Y., Govinden, R., Luo, J., and Ren, G. (2020). Biochar-mediated control of phytophthora blight of pepper is closely related to the improvement of the rhizosphere fungal community. Front. Microbiol. 11:1427. doi: 10.3389/fmicb.2020.01427
Wang, Y., Ma, Z., Wang, X., Sun, Q., Dong, H., Wang, G., et al. (2019a). Effects of biochar on the growth of apple seedlings, soil enzyme activities and fungal communities in replant disease soil. Sci. Hortic. 256:108641. doi: 10.1016/j.scienta.2019.108641
Wang, Y., Zhong, B., Shafi, M., Ma, J., Guo, J., Wu, J., et al. (2019b). Effects of biochar on growth, and heavy metals accumulation of moso bamboo (Phyllostachy pubescens), soil physical properties, and heavy metals solubility in soil. Chemosphere 219, 510–516. doi: 10.1016/j.chemosphere.2018.11.159
Win, T., Okazaki, K., Ookawa, T., Yokoyama, T., and Ohwaki, Y. (2019). Influence of rice-husk biochar and Bacillus pumilus strain TUAT-1 on yield, biomass production, and nutrient uptake in two forage rice genotypes. PLoS One 14, e0220236. doi: 10.1371/journal.pone.0220236
Wu, X., Wang, D., Riaz, M., Zhang, L., and Jiang, C. (2019). Investigating the effect of biochar on the potential of increasing cotton yield, potassium efficiency and soil environment. Ecotox. Environ. Safe. 182:109451. doi: 10.1016/j.ecoenv.2019.109451
Wu, H., Wu, H., Jiao, Y., Zhang, Z., Rensing, C., and Lin, W. (2022). The combination of biochar and PGPBs stimulates the differentiation in rhizosphere soil microbiome and metabolites to suppress soil-borne pathogens under consecutive monoculture regimes. GCB Bioenergy 14, 84–103. doi: 10.1111/gcbb.12906
Xi, J., Li, H., Xi, J., Tan, S., Zheng, J., and Tan, Z. (2020). Effect of returning biochar from different pyrolysis temperatures and atmospheres on the growth of leaf-used lettuce. Environ. Sci. Pollut. R. 27, 35802–35813. doi: 10.1007/s11356-020-09840-8
Xia, H., Riaz, M., Zhang, M., Liu, B., Li, Y., El-Desouki, Z., et al. (2022). Biochar-N fertilizer interaction increases N utilization efficiency by modifying soil C/N component under N fertilizer deep placement modes. Chemosphere 286:131594. doi: 10.1016/j.chemosphere.2021.131594
Xu, M., Gao, P., Yang, Z., Su, L., Wu, J., Yang, G., et al. (2019). Biochar impacts on phosphorus cycling in rice ecosystem. Chemosphere 225, 311–319. doi: 10.1016/j.chemosphere.2019.03.069
Xu, H. J., Wang, X. H., Li, H., Yao, H. Y., Su, J. Q., and Zhu, Y. G. (2014). Biochar impacts soil microbial community composition and nitrogen cycling in an acidic soil planted with rape. Environ. Sci. Technol. 48, 9391–9399. doi: 10.1021/es5021058
Yakout, S. M. (2017). Physicochemical characteristics of biochar produced from rice straw at different pyrolysis temperature for soil amendment and removal of organics. P. Natl. A. Sci. India A. 87, 207–214. doi: 10.1007/s40010-017-0343-z
Yang, E., Meng, J., Hu, H., Cheng, D., Zhu, C., and Chen, W. (2019). Effects of organic molecules from biochar-extracted liquor on the growth of rice seedlings. Ecotox. Environ. Safe. 170, 338–345. doi: 10.1016/j.ecoenv.2018.11.108
Yin, X., Peñuelas, J., Sardans, J., Xu, X., Chen, Y., Fang, Y., et al. (2021). Effects of nitrogen-enriched biochar on rice growth and yield, iron dynamics, and soil carbon storage and emissions: a tool to improve sustainable rice cultivation. Environ. Pollut. 287:117565. doi: 10.1016/j.envpol.2021.117565
Yoo, S. Y., Kim, Y. J., and Yoo, G. (2020). Understanding the role of biochar in mitigating soil water stress in simulated urban roadside soil. Sci. Total Environ. 738:139798. doi: 10.1016/j.scitotenv.2020.139798
You, X., Yin, S., Suo, F., Xu, Z., Chu, D., Kong, Q., et al. (2021). Biochar and fertilizer improved the growth and quality of the ice plant (Mesembryanthemum crystallinum L.) shoots in a coastal soil of Yellow River Delta. China. Sci. Total Environ. 775:144893. doi: 10.1016/j.scitotenv.2020.144893
Zhang, Y., Ding, J., Wang, H., Su, L., and Zhao, C. (2020). Biochar addition alleviate the negative effects of drought and salinity stress on soybean productivity and water use efficiency. BMC Plant Biol. 20, 288–211. doi: 10.1186/s12870-020-02493-2
Zhang, Z., Dong, X., Wang, S., and Pu, X. (2020). Benefits of organic manure combined with biochar amendments to cotton root growth and yield under continuous cropping systems in Xinjiang, China. Sci. Rep. 10, 1–10. doi: 10.1038/s41598-020-61118-8
Zhang, G., Guo, X., Zhu, Y., Han, Z., He, Q., and Zhang, F. (2017). Effect of biochar on the presence of nutrients and ryegrass growth in the soil from an abandoned indigenous coking site: the potential role of biochar in the revegetation of contaminated site. Sci. Total Environ. 601–602, 469–477. doi: 10.1016/j.scitotenv.2017.05.218
Zhang, M., Liu, Y., Wei, Q., and Gou, J. (2021). Biochar enhances the retention capacity of nitrogen fertilizer and affects the diversity of nitrifying functional microbial communities in karst soil of Southwest China. Ecotox. Environ. Safe. 226, 112819. doi: 10.1016/j.ecoenv.2021.112819
Zhang, D., Pan, G., Wu, G., Kibue, G. W., Li, L., Zhang, X., et al. (2016). Biochar helps enhance maize productivity and reduce greenhouse gas emissions under balanced fertilization in a rainfed low fertility inceptisol. Chemosphere 142, 106–113. doi: 10.1016/j.chemosphere.2015.04.088
Zhao, F., Zou, G., Shan, Y., Ding, Z., Dai, M., and He, Z. (2019). Coconut shell derived biochar to enhance water spinach (Ipomoea aquatica Forsk) growth and decrease nitrogen loss under tropical conditions. Sci. Rep. 9, 20291. doi: 10.1038/s41598-019-56663-w
Zheng, B.-X., Ding, K., Yang, X.-R., Wadaan, M. A. M., Hozzein, W. N., Peñuelas, J., et al. (2019). Straw biochar increases the abundance of inorganic phosphate solubilizing bacterial community for better rape (Brassica napus) growth and phosphate uptake. Sci. Total Environ. 647, 1113–1120. doi: 10.1016/j.scitotenv.2018.07.454
Zheng, Y., Han, X., Li, Y., Liu, S., Ji, J., and Tong, Y. (2020). Effects of mixed controlled release nitrogen fertilizer with rice straw biochar on rice yield and nitrogen balance in Northeast China. Sci. Rep. 10, 1–10. doi: 10.1038/s41598-020-66300-6
Zheng, H., Wang, X., Chen, L., Wang, Z., Xia, Y., Zhang, Y., et al. (2018). Enhanced growth of halophyte plants in biochar-amended coastal soil: roles of nutrient availability and rhizosphere microbial modulation. Plant Cell Environ. 41, 517–532. doi: 10.1111/pce.12944
Zhou, M., Ying, S., Chen, J., Jiang, P., and Teng, Y. (2021). Effects of biochar-based fertilizer on nitrogen use efficiency and nitrogen losses via leaching and ammonia volatilization from an open vegetable field. Environ. Sci. Pollut. Res. Int. 28, 65188–65199. doi: 10.1007/s11356-021-15210-9
Zhu, Q., Chen, L., Chen, T., Xu, Q., He, T., Wang, Y., et al. (2021). Integrated transcriptome and metabolome analyses of biochar-induced pathways in response to Fusarium wilt infestation in pepper. Genomics 113, 2085–2095. doi: 10.1016/j.ygeno.2021.04.031
Keywords: biochar, circular economy, main cereal crops, plant growth, recycling
Citation: Martínez-Gómez Á, Poveda J and Escobar C (2022) Overview of the use of biochar from main cereals to stimulate plant growth. Front. Plant Sci. 13:912264. doi: 10.3389/fpls.2022.912264
Edited by:
Hans-Peter Kaul, University of Natural Resources and Life Sciences Vienna, AustriaReviewed by:
Gerhard Soja, Austrian Institute of Technology (AIT), AustriaMazhar Rafique, The University of Haripur, Pakistan
Dilfuza Egamberdieva, Leibniz Center for Agricultural Landscape Research (ZALF), Germany
Copyright © 2022 Martínez-Gómez, Poveda and Escobar. This is an open-access article distributed under the terms of the Creative Commons Attribution License (CC BY). The use, distribution or reproduction in other forums is permitted, provided the original author(s) and the copyright owner(s) are credited and that the original publication in this journal is cited, in accordance with accepted academic practice. No use, distribution or reproduction is permitted which does not comply with these terms.
*Correspondence: Carolina Escobar, carolina.escobar@uclm.es
†These authors have contributed equally to this work