- 1Departamento de Procesos Industriales, Facultad de Ingeniería, Universidad Católica de Temuco, Temuco, Chile
- 2Departamento de Ingeniería Química, Universidad de La Frontera, Temuco, Chile
- 3Scientific and Technological Bioresource Nucleus (BIOREN), Universidad de La Frontera, Temuco, Chile
- 4Institute of Water Research, University of Granada, Granada, Spain
- 5Department of Microbiology, Pharmacy Faculty, University of Granada, Granada, Spain
- 6IMAM, UNaM, CONICET, FCEQYN, Programa de Celulosa y Papel (PROCYP), Posadas, Argentina
- 7Instituto de Ciencias Químicas Aplicadas, Universidad Autónoma de Chile, Temuco, Chile
- 8Instituto del Medio Ambiente (IMA), Universidad de La Frontera, Temuco, Chile
Biological synthesis of high added-value compounds like adipic acid (AA), levulinic acid (LA), or polyhydroxybutyrate (PHB) using pure culture has been separately reported. However, pure culture requires sterile conditions and the use of specific carbon sources resulting in high operating costs. Different alternatives based on the use of mixed microbial cultures (MMC) have been explored to resolve this problem. MMC have been widely reported for the production of PHB, but scarcely reported for LA production and never for AA synthesis. This work presents a novel strategy for the co-production of AA LA, and PHB using MMC. The strategy consists in selecting an MMC producer of AA, LA and PHB from an inoculum obtained from a wastewater treatment plant, which is then subjected to the feast and famine culture strategy in a sequential batch reactor, coupled with a batch reactor step to enhance the accumulation of AA and LA. The results showed that the MMC could produce a 16 ± 2, 23 ± 1 and 5 ± %1 (g compound/g volatile solids) of AA, LA and PHB, respectively, using a non-fermented residual biomass rich in pentose, namely synthetic hemicellulose hydrolysate (SHH) as the carbon source. These results contribute to generating future research to better understand and optimise the biosynthesis of these compounds by MMC.
1. Introduction
The biological process to produce high value-added compounds has been gaining interest, as these processes form part of the efforts to move from a fossil fuel economy to one based on renewable resources (Jeong et al., 2018). Some of these high value-added compounds that can be produced using biological processes are adipic acid (AA), levulinic acid (LA), and polyhydroxybutyrate (PHB) (Rodriguez-Perez et al., 2018; Pinto-Ibieta et al., 2020, 2021). LA is a platform molecule that allows the production of a wide range of compounds applied in the chemical, food, and agrochemical industries, being considered among the 12 molecules with the highest added value in the world (Lappalainen and Dong, 2019; Morakile et al., 2022; Ukawa-Sato et al., 2023). AA is use to produce of nylon 6.6, a polymer in very high demand in the textile and automotive industries (Riveiro et al., 2020; Wu et al., 2023). It can also be used as an intermediate to produce different compounds like cyclopentanone and 1.6-hexanediol, which are important in the fragrance and resin production industries, respectively (Corona et al., 2018; Kruyer et al., 2020). Finally, PHB are biodegradable polyesters that can be produced in bioprocesses from renewable resources in contrast to fossil-based bio-recalcitrant polymers (Cassuriaga et al., 2018; Correa-Galeote et al., 2022a). Biological synthesis of AA, LA, and PHB using pure culture has been reported separately. For instance, engineered bacterial strains like Saccharomyces cerevisiae and Pseudomonas putida are able to synthesise AA from different substrates, even hexoses and lignin (Raj et al., 2018; Zhao et al., 2018; Wu et al., 2023). LA synthesis can be carried out by fermentation of pentoses using engineered Saccharomyces cerevisiae and Pichia stipis (Zhangelinni, 2017). PHB production from pure culture using pentoses and hexoses has been widely recognised and exploited (Li and Wilkins, 2020). In contrast with pure culture, the use of mixed microbial cultures (MMC) is gaining attention. MMC involve lower operating costs and can be adapted to the use of agro-industrial waste as a carbon source, minimising both the operational cost and the ecological footprint of these processes (Huang et al., 2018; Mohamad Fauzi et al., 2019; Yin et al., 2019a; Correa-Galeote et al., 2022b). The use of MMC subjected to a feast and famine strategy is mainly focused on PHB production using volatile fatty acids as the carbon source (Rodriguez-Perez et al., 2018). Less reported, however, has been the use of non-fermented waste as a carbon source for PHB production (Pinto-Ibieta et al., 2020, 2021). Indeed, the ability of the MMC to produce PHB when the carbon source used was reduction sugars obtained from lignocellulosic hydrolysates has been described (Yin et al., 2019b, 2020). However, in addition to PHB production, it seems that non-fermented waste as feed for MMC could favour the accumulation of other added-value compounds by controlling operational parameters such as the dissolved oxygen, the organic loading rate or the feast/famine cycle length (Pinto-Ibieta et al., 2021). For example, Pinto-Ibieta et al. (2020) reached a LA production of up to 32% (g compound/g VS) (VS, volatile solids) with a MMC fed with pentose-rich hemicellulose hydrolysate. Meanwhile, Argiz et al. (2021) managed to accumulate 18% (g compound/g VS) of triglycerides and 26% (g compound/g VS) of PHA when the MMC were fed with residual fish-canning oil. The co-production of some compounds could be possible because of a close relationship between metabolic routes. For example, the intracellular production of both AA and LA involve the pentose phosphate pathway by the transformation of the pentoses to pyruvic acid and its subsequent oxidation to acetyl-CoA (Lee et al., 2019; Miscevic et al., 2019). The synthesis of LA or AA is then carried out from succinyl CoA after the acetyl-CoA enters the Krebs cycle (Lee et al., 2019). Although the metabolic route for AA accumulation is known, to the best of our knowledge, the ability to produce AA by MMC has not been previously reported.
Therefore, this study is focused mainly on (1) proving, if possible, the use of MMC to produce AA through the use of non-fermented feedstock rich in xylose as the carbon source, and (2) if possible, co-producing AA, with LA or PHB using the MMC. Thus, the main novelty of this work lies in opening up a new strategy for the biological synthesis of AA using MMC in co-production with LA and PHB.
2. Materials and method
2.1. Operation of SBRs to adapt the MMC
Six 2-L sequential batch reactors (SBR) were simultaneously operated to evaluate the adaptation of an MMC to synthesise AA in co-production with LA under a feast and famine regime. The first condition (SBR1), assayed in triplicate, was fed with synthetic hemicellulose hydrolysate, where the composition in percentage (% g compound/g VS) was a residual stream of lignocellulosic process fractionation (Vallejos et al., 2017): 78% xylose, 9.0% acetic acid, 5.06% furfural, 4.7% arabinose, 2.3% glucose, 0.8% cellobiose, and 0.14% hydroxymethylfurfural. A second condition (SBR2), assayed in triplicate, was operated using acetic acid as the only carbon source. Both substrates were selected as models for comparing the use of non-fermented (SHH) versus acetate substrate as the carbon source to produce high added-value compounds (AA and LA) instead of PHB when the MMC are subjected to a feast and famine regimen. SBR1 and SBR2 were supplemented with the nutrients and minerals necessary for bacterial growth, including: 132 mg of peptone/L, 68 mg of beef extract/L, 112 mg of (NH4)2SO4/L, 49 mg of KH2PO4, 66 MgSO4/L, 170 mg of NH4Cl/L, 92 mg of K2HPO4/L, 45 mg KH2PO4/L, 600 mg MgSO4/L, 100 mg EDTA/L, 70 mg CaCl2/L, and 2 mL of a trace element solution (Huang et al., 2017). 10 mg Thiourea/L was also added to prevent nitrification. The trace elements solution was composed of 1,000 mg FeSO4·6H2O/L, 150 mg H3BO3/L, 150 mg CoCl2·6H2O/L, 120 mg MnCl2·4H2O/L, 120 mg ZnSO4·7H2O/L, 60 mg Na2MoO4·2H2O/L, 30 mg KI/L, and 30 mg CuSO4·5H2O/L.
SBR1 and SBR2 were inoculated with activated sludge from the municipal wastewater treatment plant in Temuco, Chile. Both reactors were operated under the same conditions: 4 L/min airflow, 30°C, 60 rpm, and a C/N/P ratio of 30/0.9/0.1 (in mmol/L). The strategy applied was the alternation of F/F periods through SBR operation to obtain MMC enriched with microorganisms capable of producing high added-value compounds. Two cycles per day were carried out in each SBR until a stable operation was reached. One cycle consisted of: a feed period (6 min) followed by an aerobic reaction (704 min) and 10 min of withdrawal (Huang et al., 2017; Wang et al., 2017). No settling phase was performed, and all excess biomass was withdrawn with the mixed liquor (Dionisi et al., 2005; Cabrera et al., 2019). Thus, the biomass retention time was equal to the hydraulic retention time. 0.25 L of substrate was fed into each reactor in each cycle using organic loading rate (OLR) of 60 Cmmol/L*day. At the end of each cycle, 0.25 L of the liquid was withdrawn by digital peristaltic pumps using a Compact DAQ system (cDAQ-9,178 chassis, National Instruments, Austin, TX, United States), with a routine programmed using the LabView software (National Instruments). SBR1 and SBR2 were operated for 100 consecutive cycles (50 days) since stable operation was reached from cycle 80 in a previous study (Pinto-Ibieta et al., 2020). To monitor the evolution of AA, LA, PHB, and biomass growth in both SBR, samples were taken at the end of a cycle once a week. Once a stable operation was determined, intermediate samples were taken during feeding cycles 80, 84, 88, and 90 from both reactors. The samples were taken in triplicate every hour to monitor the evolution of AA, AL, PHB, biomass, and carbon source uptake (pentoses and acetate) as a function of the time in the cycle. The mean and standard deviation of these cycles were calculated to corroborate the stability of the operation.
2.2. Batch assays to maximise the accumulation of target compounds
The MMC obtained from the operation of SBR1 and SBR2 were cultivated separately in batch reactors to evaluate their adaptability to the use of SHH and maximise their ability to produce LA, AA and/or PHB in a batch stage, regardless of the substrate used during the selection phase. SHH (described in point 2.1) was the only carbon source used in these batch assays. The maximum accumulation capacity of compounds was assayed without the addition of nitrogen, since it has been reported that restricting this nutrient in the culture could lead to the accumulation of storage compounds by the cell (Guerfali et al., 2018; Chong et al., 2019). Six assays, in triplicate, were carried out to evaluate the effect of increasing SHH doses (30, 75, and 120 Cmmol/L) on the production of the compounds of interest (Table 1). 80 mL of adapted MMC (with a concentration of 3 mg VS/L), obtained from each SBR reactor described in Section 2.1 were used as inoculum in Erlenmeyer flasks containing 120 mL of mineral medium without a nitrogen source (see Section 2.1), incubated for 24 h at 150 rpm and 30°C. ANOVA tests were performed to evaluate the significance of the differences observed for AA and LA accumulations at the different experimental conditions.
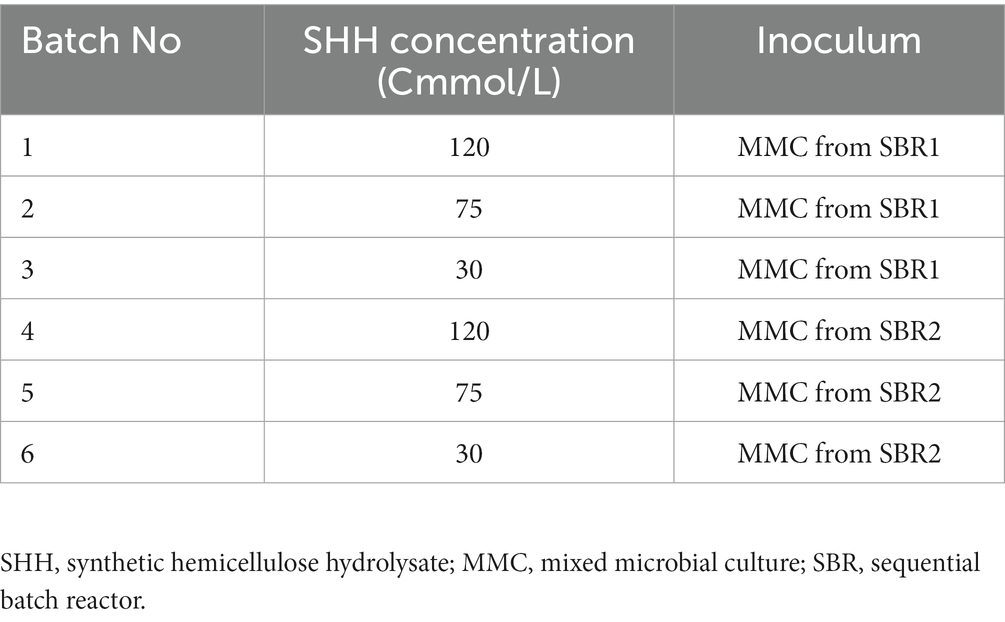
Table 1. Assays in batch reactors for the improvement of levulinic acid (LA), adipic acid (AA), and polyhydroxybutyrate (PHB) accumulation.
2.3. Analytical methods
LA, AA, and PHB were quantified and identified according to Lappalainen and Dong (2019) and Serafim et al. (2004). A GC/MS Clarus 600 (Perkin Elmer) with an ELITE 1701 column 30 m × 0.25 mm × 0.25 um was used for identification, and a GC/FID Clarus 600 (Perkin Elmer) with CAPILAR NUKOLTM SUPELCO 30 m × 0.25 mm × 0.25 um for quantification. The calibration curve was obtained by injecting a series of standards at different concentrations of AA, LA, and PHB (Sigma Aldrich). The substrate consumption was measured by determining the concentration of reducing sugars and acetate in filtered samples (0.22 μm pore size PVDF membrane, Merck). To quantify the total reducing sugars, the dinitrosalicylic acid (DNS) reagent method was used (Zhang et al., 2012; Prasertsung et al., 2017). Acetate was determined by gas chromatography in a flame ionisation detector (Clarus 400, PerkinElmer), using a NukolTM capillary column (Sigma-Aldrich, Darmstadt, Germany). VS and soluble chemical oxygen demand (SCOD) were quantified using a standard technique (AWWA, 2005).
3. Results
3.1. Mixed microbial culture selection capable of producing LA, AA and PHB
3.1.1. LA, AA and PHB concentration evolution over operation time of selection reactors using SHH and acetate
The evolution of LA, AA, PHB, and VS during the operation of SBR1 is shown in Figure 1A. An adaptation stage was observed during the first 30 days of operation followed by stable operation, reflected by constant concentrations of LA, AA, PHB and VS in successive cycle ends. At the same time, cell wash-out was observed since there was a gradual reduction in VS from 5.7 ± 0.3 g/L to 3 ± 0.1 g/L from days 0 to 35. According to Argiz et al. (2021), the decrease in the biomass concentration can be explained by the culture selection, with only the microbial populations capable of surviving at the F/F culture strategy remaining and synthesising SHH as a carbon source. The results showed that there was no AA and LA production at the beginning of the culture (Figure 1A). These compounds were produced after 20 days of operation, proving that the use of the F/F culture strategy was able to select a MMC that favours LA and AA production from SHH. Specifically, LA and AA reached mean concentration values of 5.9 ± 0.7% (g compound/g VS) and 5.2 ± 0.2% (g compound/g VS), respectively, from days 20 to 50 of operation (Figure 1A). On the other hand, the MMC selection for PHB production was not observed using SHH, since the PHB concentration stays constant throughout the operation time, i.e., 2.4 ± 0.5% (g compound/g VS).
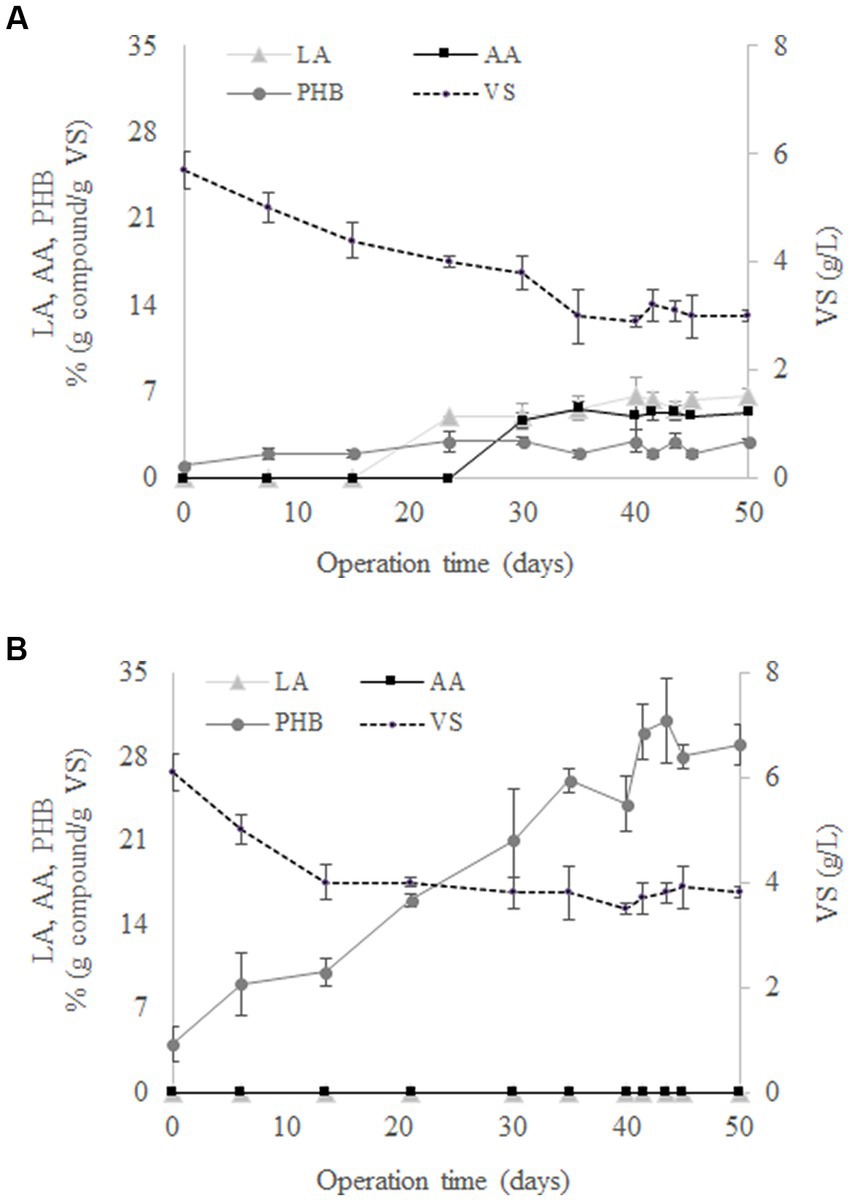
Figure 1. Evolution of levulinic acid (LA), adipic acid (AA), polyhydroxybutyrate (PHB), and volatile solids (VS) in the 50 days performed by SBR1 (A) and SBR2 (B).
Figure 1B shows that, contrary to the findings with SBR1, AA and LA were not synthesised when acetate was used as the carbon source in SBR2. Under these conditions, only PHB was determined in the reactor throughout the experimental time. In particular, PHB reached a mean value of 29.5 ± 1.3% (g compound/g VS) once the steady state was reached during days 41–50 (Figure 1B). This behaviour was expected, since it is widely reported that MMC can transform volatile fatty acids into PHB (Lee et al., 2015; Pinto-Ibieta et al., 2021; Argiz et al., 2022). Therefore, the production of AA and LA was only possible when the conditions were against the PHB through the feeding of a complex carbon source like SHH.
3.1.2. Analysis of the behaviour of LA, AA, PHB, acetate and pentoses inside the culture cycles of the selection reactors
Figure 2A shows the variation of the mean concentrations of LA, AA, PHB, acetate and pentoses determined for four culture cycles performed by SBR1 during operation days 40, 42, 44, and 50. According to the variation in the concentration of the pentoses, SHH was consumed in around 2.5 h after the start of the cycle. At the same time, after a rapid drop from 8 to 2.8 mg/L, the dissolved oxygen increased again to a value of 8 mg/L (Figure 2A). This indicates the end of the feast phase and the beginning of the famine phase. The intracellular AA content increased from 3 to 9% (g compound/g VS) in the feast phase and decreased from 9% to 5% (g compound/g VS) in the famine phase. LA increased from 4% to 13% in the feast phase and decreased from 13 to 4% in the famine phase. Meanwhile, PHB remained around 3% (g compound/g VS) during the whole cycle (Figure 2A). The results showed that during the famine phase, the MMC were able to consume the AA and LA accumulated during the feast phase, probably due to external substrate limitation. Thus, AA and LA could be considered another carbon reserve like PHB. Figure 2B shows the variation of the mean concentrations of PHB, DO and acetate measured in four operational cycles performed in SBR2, at operation days 40, 42, 44, and 50. DO concentration increased from 1 g/L to 8 g/L at the end of the feast phase (after around 3 h of operation), when PHB production reached a concentration up to 25% (g compound/g VS). However, the PHB concentration decreased during the famine phase to values around 11% (g compound/g VS). AA and LA production were negligible throughout the cycle duration (Figure 2B). These results confirmed that the AA and LA production benefitted from the feeding of a complex substrate such as SHH to the MMC.
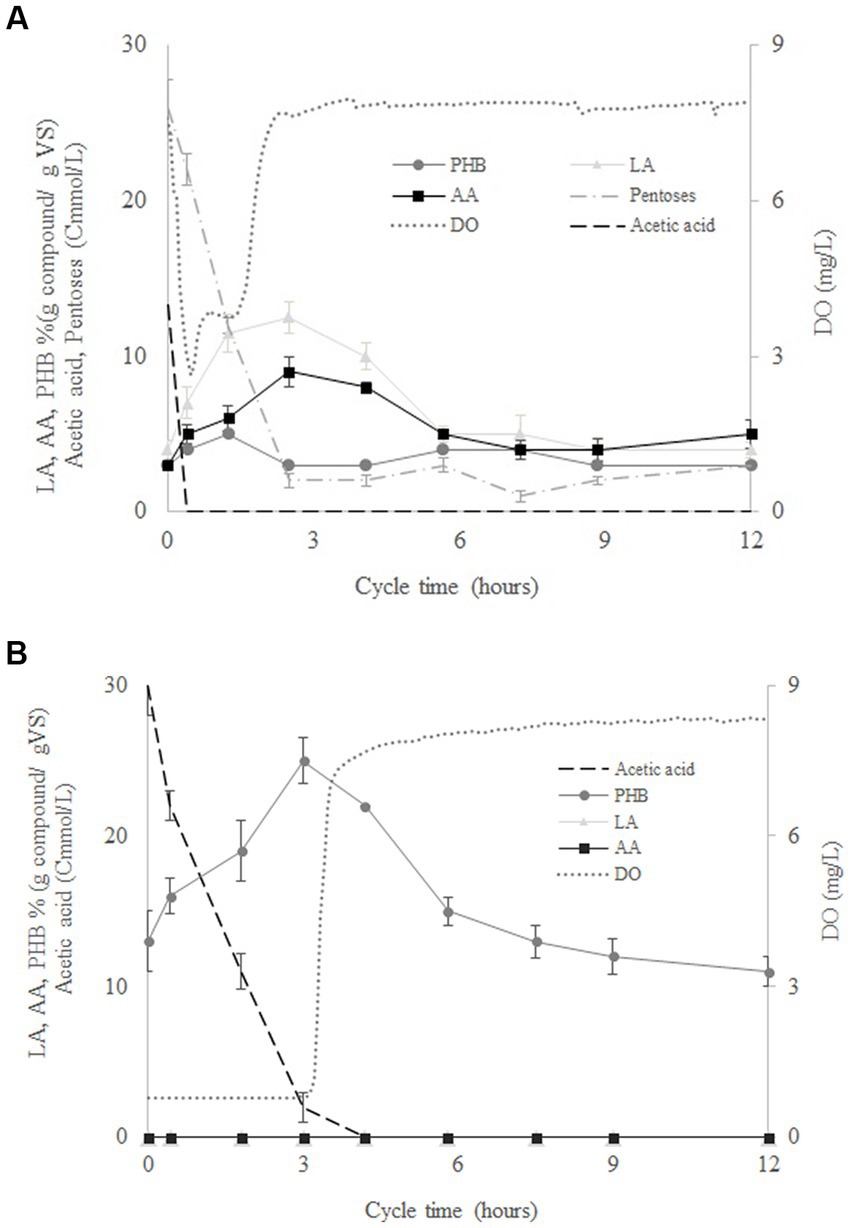
Figure 2. Variation of the concentration of levulinic acid (LA), adipic acid (AA), polyhydroxybutyrate (PHB), pentoses, acetate, volatile solids (VS), and dissolved oxygen (DO) throughout the cycle duration for SBR1 (A) and SBR2 (B).
To date, to the best of our knowledge, the biological synthesis of AA has been only described from glucose and for metabolically engineered cultures of Saccharomyces cerevisiae (Zhang et al., 2020) or Escherichia coli (Zhou et al., 2020). AA biosynthesis using MMC feed with SHH has not been reported previously. LA biosynthesis using MMC feed with SHH has recently been reported by Pinto-Ibieta et al. (2020), where the LA concentration reached a value of up to 32% (g compound/g VS). The present research demonstrated that not only genetically modified microorganisms can metabolise pentoses into AA or LA. On the other hand, some studies have achieved an accumulation of PHB of up to 20% (g compound/g VS) using synthetic pentoses or lignocellulose hydrolysate to feed MMC under a F/F strategy (Zhang et al., 2016; Yin et al., 2019b). The differences in the profile of the accumulated compounds could be explained by the microbial composition of the selected MMC, as the microbial compositions in those studies differed from that used in the present research. In this sense, Oliveira et al. (2017) reported that the selected MMC will be different as a consequence of changes in the feed strategy. The different operational conditions established for the F/F culture strategy in this work versus those established by Zhang et al., 2016 will probably lead to a MMC enriched in LA-and AA-producing microorganisms.
Production of LA, but not of AA, production was achieved in our previous studies using SHH (Pinto-Ibieta et al., 2020). Table 2 shows a comparative summary of the results and operational conditions reported by Pinto-Ibieta et al. (2020) and those for SBR1 in the present work. In both cases, SHH was the carbon source, but the operational conditions of the F/F culture strategy were different, i.e., different DO, organic loading rate and nutrient ratio were fixed (Table 2). Therefore, the type of compound (LA and AA) produced could be a response of microorganisms to these differences in the established operational conditions. For instance, Table 2 shows that when DO concentration during the feast phase was higher than 3 mg/L, the selected MMC showed a tendency to synthesize AA in co-production with LA. By contrast, when the DO concentration was lower than 3 mg/L, the microorganisms synthesised only LA. Bart and Cavallaro (2015) reported that depending on the metabolic pathway type through which AA is synthesised using pure cultures, anaerobic systems could be more efficient than aerobic, or vice-versa. On the other hand, metabolic pathways to synthesise succinyl-CoA (intermediary in AA synthesis) were reported as being more efficient in the microaerobic system than aerobic or anaerobic. Therefore, DO concentration would be a crucial operational parameter to be controlled to favour the metabolic pathways related to AA production. This is also consistent with Pinto-Ibieta et al. (2021), where different operational conditions (DO, F/F ratio, SBR cycle length, organic loading rate (OLR), pH, C/N, and temperature) established for the F/F culture strategy were compiled and analysed, showing how the variation of these operational conditions favoured the accumulation of some compounds over others. These authors found that DO concentration was the variable that most clearly influenced the accumulation of targeted compounds. At DO concentrations higher than 3 mg/L, MMC tended to synthesise PG and TAG, while DO concentrations lower than 3 mg/L favoured the production of PHB and LA. Finally, the greater accumulation of LA observed in Pinto-Ibieta et al. (2020) than in the present study may be due to the lower OLR used in the present work. According to de Oliveira et al. (2019) the amount of PHB produced will increase as the organic loading rate increases, as long as the organic loading rate does not result in substrate inhibition.
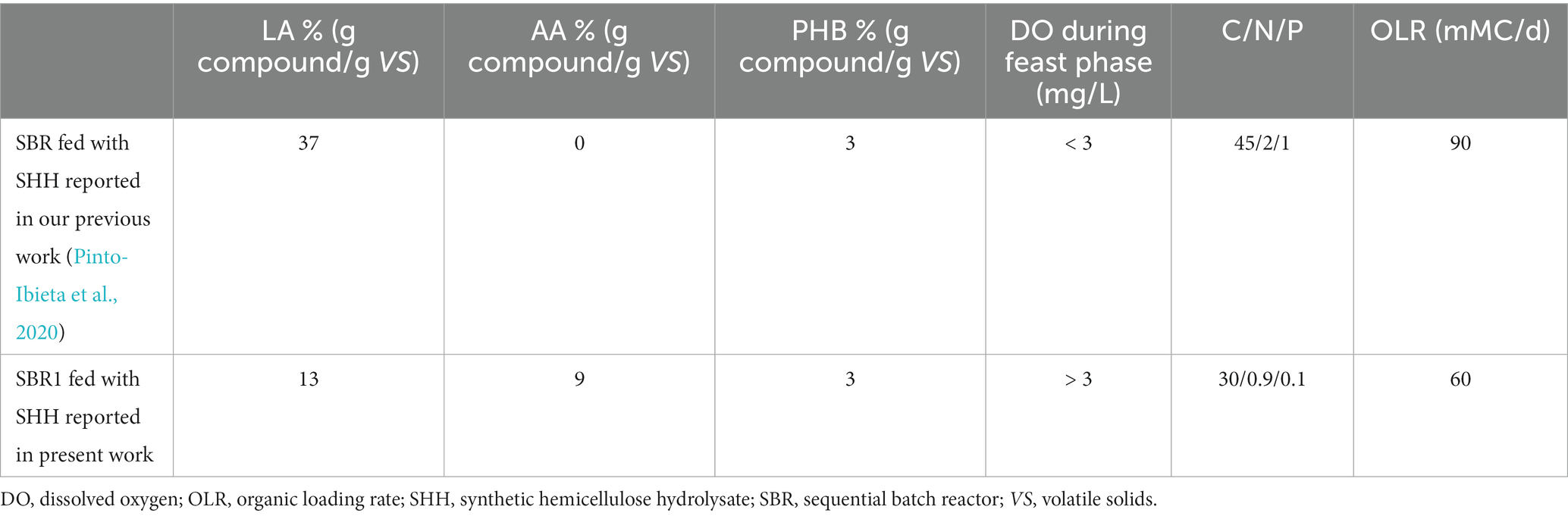
Table 2. Levulinic acid (LA), adipic acid (AA), and polyhydroxybutyrate (PHB) production obtained per operation cycle by F/F culture strategy under different operational conditions, using SHH as carbon source per one operation cycle.
Although the LA and AA co-production was possible in the present work, further studies with MMC are required to determine which of these variables could be the principal factor that favours synthesis and control in the selective accumulation of both AA and LA. In particular, the effect of DO concentration on the F/F culture strategy should be studied, as this seems to be the main variable that affects the synthesis of AA over LA. Nevertheless, the results indicate that under the operational conditions evaluated during the F/F culture strategy, the adapted MMC contain microorganisms with the required enzymatic pool for bioconversion of pentoses into AA and LA.
3.2. Batch assays for improvement of LA, AA, and PHB accumulation by previously selected microorganisms in SBR reactors
Figure 3A shows the concentrations of LA, AA, and PHB reached for the different SHH concentrations evaluated in the batch experiments conducted with the MMC previously selected in SBR1. For the three SHH concentrations evaluated, it was possible to accumulate the three added-value compounds identified in SBR1: LA, AA, and PHB. The highest concentration of SHH (120 Cmmol/L) produced the highest accumulation of each compound, obtaining yields of 23 ± 1, 16 ± 2, and 6 ± 2% g compound/g VS of LA, AA, and PHB, respectively (Figure 3A). When using 75 Cmmol/L, the LA, AA, and PHB accumulation concentrations were 20 ± 2, 14 ± 1, and 4 ± 1% (g compound/g VS), respectively; no significative difference from the latter was found in the values obtained at 120 Cmmol/L (Supplementary Table S1). The higher concentrations observed at 120 Cmmol/L could indicate that SHH was not inhibitory at this concentration (de Oliveira et al., 2019). The lowest accumulations were obtained at 30 Cmmol/L, with yields of only 15 ± 3, 9 ± 2, and 5 ± 2% (g compound/g VS) for LA, AA and PHB, respectively (Figure 3A), values which showed a significative reduction compared to those obtained at 120 Cmmol/L (Supplementary Table S1). This decrease could be caused by a limitation in the substrate, which could leading to a microbial growth limitation (Alburqueque et al., 2010; Pinto-Ibieta et al., 2021).
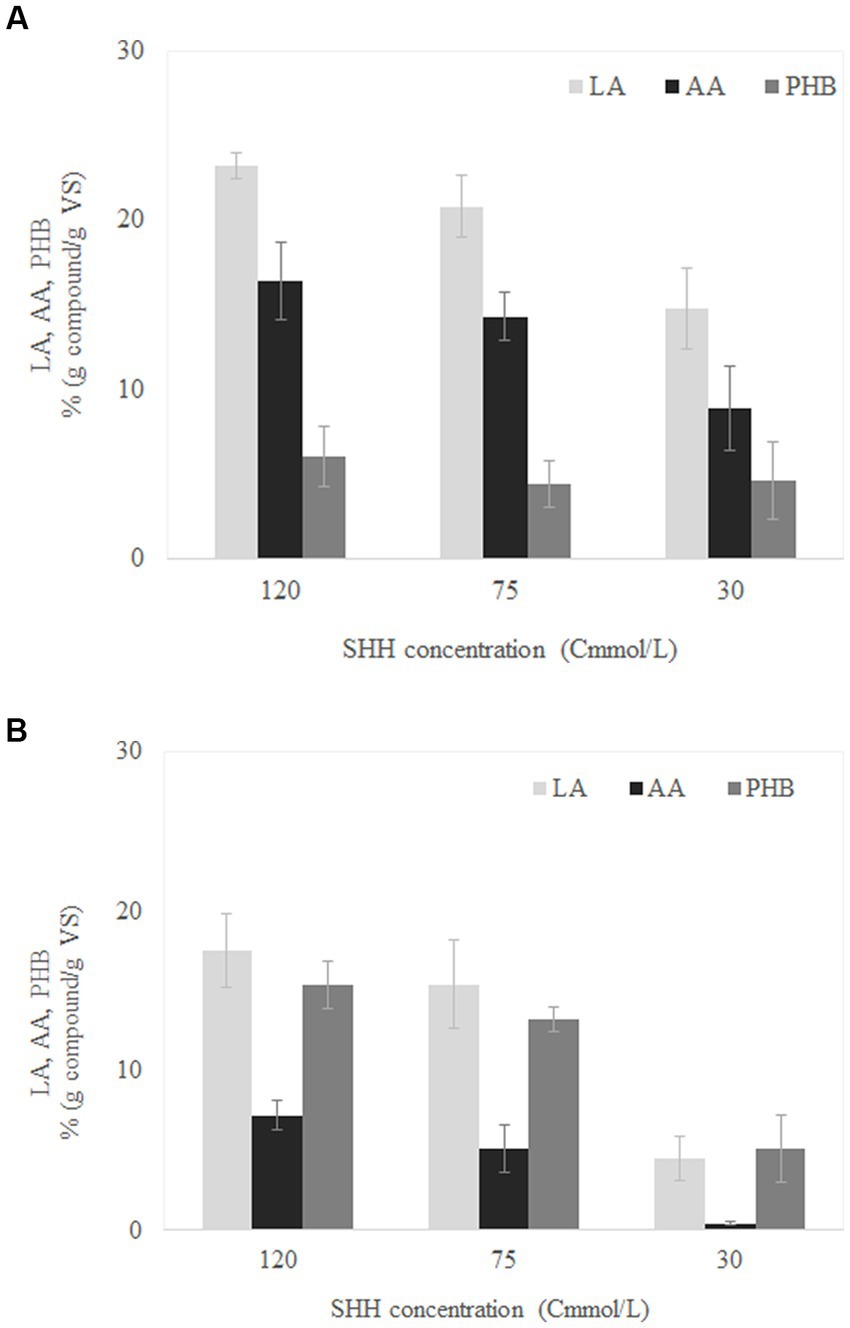
Figure 3. Maximum levulinic acid (LA), adipic acid (AA), polyhydroxybutyrate (PHB) accumulation capacity for mixed microbial cultures adapted in SBR1 (A) and SBR2 (B) at different synthetic hemicellulose hydrolysate (SHH) concentrations.
The use of MMC from SBR2 instead of SBR1 in the batch experiments resulted in general in a higher concentration of PHB and lower concentration of AA and LA compared to the results obtained using SBR1 (Figure 3). Specifically, by feeding with SHH at 120 Cmmol/L, it was possible to accumulate LA, AA, and PHB at concentrations of 18 ± 2, 7 ± 1, and 15 ± 2% (g compound/g VS), respectively (Figure 3B). On the other hand, at 30 Cmmol/L, the production of both LA and PHB was 5% (g compound/g VS), while no production of AA was observed. As in the reactors inoculated with the MMC obtained from SBR1, the statistical analysis (ANOVA) for the reactors inoculated with the MMC obtained from SBR2 only showed a significant difference when comparing 120 versus 30 Cmmol/L (Supplementary Table S2).
These results indicate that the simultaneous production of LA, AA, and PHB was possible using both adaptations of MMC under batch conditions (from SBR1 and SBR2), although the long-term adaptation of the MMC to the SHH in SBR1 favoured the accumulation of AA and LA. It may be noted that the origin and adaptation of the MMC could be a key point for the accumulation of high added-value compounds, as it is indicated by the statistical differences observed in the accumulation extents obtained from the MMC from SBR1 and SBR2 (Supplementary Table S3).
Figure 4 shows the evolution of pentoses, biomass, LA, AA, and PHB during the culture time for the assays at 120 Cmmol/L using MMC from SBR1 (Figure 4B) and from SBR2 (Figure 4B) as the inoculum. Both reactors resulted in similar production and composition of the accumulated compounds despite of the different MMC used (Figure 4). Pentoses and acetic acid were rapidly consumed while AA, LA, and PHB were produced at the same time. The maximum accumulation of AA and LA was reached at the time when the pentoses were exhausted (around 3 h). Likewise, the maximum PHB was reached once the acetic acid was exhausted in the reactors (Figure 4). Similar behaviours were observed for AA, LA, and PHB, with concentrations decreasing after maximum generation. These results could indicate AA and LA storage in some kind of carbon source similar to PHB. Therefore, MMC adapted from SBR2 were not able to synthesise LA and AA using acetate, but the same microorganisms fed with SHH were capable of synthesising LA and AA, confirming that the production of AA and LA depends on the use of SHH as carbon source. This was expected since the metabolic pathways described for both AA and LA production begin with hexoses and/or pentoses as substrate (Lee et al., 2019).
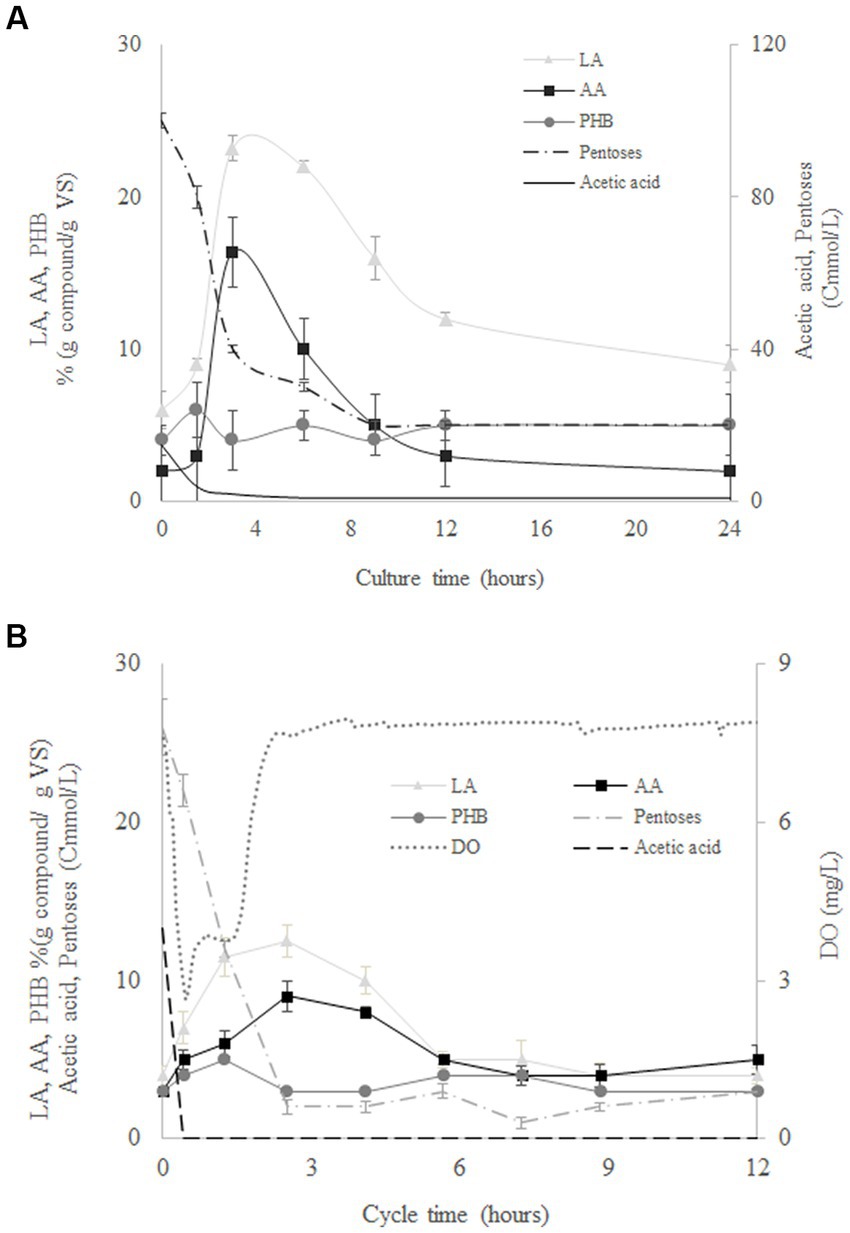
Figure 4. Evolution of levulinic acid (LA), adipic acid (AA), polyhydroxybutyrate (PHB), pentoses, acetate, and volatile solids (VS) during batch culture at 120 Cmmol/L for mixed microbial cultures from SBR1 (A) and SBR2 (B).
Although both MMC types were able to synthesise LA, AA, and PHB when cultivated in batch conditions, the culture yields were different. As expected, MMC adapted in SBR1 with SHH were able to produce higher yields of AA and LA than those from SBR2. On the other hand, the co-production of LA and PHB was favoured in the batch assays conducted with MMC selected in SBR2. Therefore, the kind of substrate used in the selection stage could stimulate the production of specific compounds in a subsequent batch stage. The possible benefits of this co-production should be evaluated considering the yields of each compound, their final properties, the difficulties, and costs of separation, etc. In addition, it is important to identify the microbial communities and their metabolic pathway involved in AA and LA synthesis.
4. Conclusion
This research demonstrate that it is possible to accumulate AA from MMC, in co-production with LA, using SHH as a non-fermented carbon source and selecting the right operational conditions of the F/F culture strategy. Depending on the carbon source used during the adaptation stage (SHH or acetic acid), different proportions of AA, LA, and PHB were obtained during the accumulation stage in the batch assays. Our study indicates that MMC have the versatility to produce different added-value compounds from SHH, demonstrating that the production of one compound or another can be favoured depending on the operational conditions established, especially DO concentration. Nevertheless, further research is needed into the optimising the operational conditions of the F/F culture strategy to improve the AA and LA production yields. Furthermore, the adapted microbial community must be identified to understand the intracellular mechanisms for AA and LA accumulation.
Data availability statement
The original contributions presented in the study are included in the article/Supplementary material, further inquiries can be directed to the corresponding author.
Author contributions
FP-I, MC, GC, and FC contributed to conception and design of the study. FP-I organized the database and wrote the first draft of the manuscript. MC, GC, FC, and AS provided guidance and suggestions for the experimental design and discussed the results. FP-I, MC, FC, GC, FF, MA, and AS wrote and edited the manuscript. All authors contributed to the article and approved the submitted version.
Funding
This research was funded by Fondecyt Postdoctorado No. 3210626, Agencia Nacional de Investigación y Desarrollo de Chile, ANID.
Acknowledgments
The authors wish to acknowledge the financial support provided by Postdoctoral Fondecyt project N°3210626. Antonio Serrano is grateful to the Economic Transformation, Industry, Knowledge, and Universities Department of the Andalucia Autonomous Government for his Emergia fellowship (EMERGIA20_00114).
Conflict of interest
The authors declare that the research was conducted in the absence of any commercial or financial relationships that could be construed as a potential conflict of interest.
Publisher’s note
All claims expressed in this article are solely those of the authors and do not necessarily represent those of their affiliated organizations, or those of the publisher, the editors and the reviewers. Any product that may be evaluated in this article, or claim that may be made by its manufacturer, is not guaranteed or endorsed by the publisher.
Supplementary material
The Supplementary material for this article can be found online at: https://www.frontiersin.org/articles/10.3389/fmicb.2023.1224543/full#supplementary-material
References
Alburqueque, M. G. E., Torres, C. A. V., and Reis, M. A. M. (2010). Polyhydroxyalkanoate (PHA) production by a mixed microbial culture using sugar molasses: effect of the influent substrate concentration on culture selection. Water Res. 44, 3419–3433. doi: 10.1016/j.watres.2010.03.021
Argiz, L., González-Cabaleiro, R., Val del Río, Á., González-López, J., and Mosquera-Corral, A. (2021). A novel strategy for triacylglycerides and polyhydroxyalkanoates production using waste lipids. Sci. Total Environ. 763:142944. doi: 10.1016/j.scitotenv.2020.142944
Argiz, L., Correa-Galeote, D., Val del Río, Á., Mosquera-Corral, A., and González-Cabaleiro, R. (2022). Valorization of lipid-rich wastewaters: A theoretical analysis to tackle the competition between polyhydroxyalkanoate and triacylglyceride-storing populations. Sci. Total Environ. 807:150761. doi: 10.1016/j.scitotenv.2021.150761
AWWA. (2005). Standard methods for the examination of water and wastewater. 20th ed., Washington, DC, USA: AWWA.
Bart, J. C. J., and Cavallaro, S. (2015). Transiting from adipic acid to bioadipic acid. Part II. Biosynthetic pathways. Ind. Eng. Chem. Res. 54, 567–576. doi: 10.1021/ie502074d
Cabrera, F., Torres, Á., Campos, J. L., and Jeison, D. (2019). Effect of operational conditions on the behaviour and associated costs of mixed microbial cultures for PHA production. Polymers (Basel). 11:191. doi: 10.3390/polym11020191
Cassuriaga, A. P. A., Freitas, B. C. B., Morais, M. G., and Costa, J. A. V. (2018). Innovative polyhydroxybutyrate production by Chlorella fusca grown with pentoses. Bioresour. Technol. 265, 456–463. doi: 10.1016/j.biortech.2018.06.026
Chong, J. F., Fadhullah, W., Lim, V., and Lee, C. K. (2019). Two-stage cultivation of the marine microalga Chlorella salina for starch and carbohydrate production. Aquac. Int. 27, 1269–1288. doi: 10.1007/s10499-019-00385-3
Corona, A., Biddy, M., Vardon, D., Birkved, M., Hauschild, M., and Beckam, G. (2018). Life cycle assessment of adipic acid production from lignin. Green Chem. 20, 3857–3866. doi: 10.1039/C8GC00868J
Correa-Galeote, D., Argiz, L., Mosquera-Corral, A., Del Rio, A. V., Juarez-Jimenez, B., Gonzalez-Lopez, J., et al. (2022a). Structure of fungal communities in sequencing batch reactors operated at different salinities for the selection of triacylglyceride-producers from a fish-canning lipid-rich waste stream. New Biotechnol. 71, 47–55. doi: 10.1016/j.nbt.2022.08.001
Correa-Galeote, D., Argiz, L., Val del Rio, A., Mosquera-Corral, A., Juarez-Jimenez, B., Gonzalez-Lopez, J., et al. (2022b). Dynamics of PHA-accumulating bacterial communities fed with lipid-rich liquid effluents from fish-canning industries. Polymers (Basel). 14:1396. doi: 10.3390/polym14071396
de Oliveira, G. H. D., Niz, M. Y. K., Zaiat, M., and Rodrigues, J. A. D. (2019). Effects of the organic loading rate on Polyhydroxyalkanoate production from sugarcane stillage by mixed microbial cultures. Appl. Biochem. Biotechnol. 189, 1039–1055. doi: 10.1007/s12010-019-03051-9
Dionisi, D., Carucci, G., Papini, M. P., Riccardi, C., Majone, M., and Carrasco, F. (2005). Olive oil mill effluents as a feedstock for production of biodegradable polymers. Water Res. 39, 2076–2084. doi: 10.1016/j.watres.2005.03.011
Guerfali, M., Ayadi, I., Mohamed, N., and Ayadi, W. (2018). Triacylglycerols accumulation and glycolipids secretion by the oleaginous yeast Rhodotorula babjevae Y-SL7: structural identification and biotechnological applications. Bioresour. Technol. 273, 326–334. doi: 10.1016/j.biortech.2018.11.036
Huang, L., Chen, Z., Wen, Q., and Lee, D. (2017). Enhanced polyhydroxyalkanoate production by mixed microbial culture with extended cultivation strategy. Bioresour. Technol. 241, 802–811. doi: 10.1016/j.biortech.2017.05.192
Huang, L., Chen, Z., Wen, Q., Zhao, L., Lee, D. J., Yang, L., et al. (2018). Insights into feast-famine polyhydroxyalkanoate (PHA)-producer selection: microbial community succession, relationships with system function and underlying driving forces. Water Res. 131, 167–176. doi: 10.1016/j.watres.2017.12.033
Jeong, H., Park, S. Y., Ryu, G. H., Choi, J. H., Kim, J. H., Choi, W. S., et al. (2018). Catalytic conversion of hemicellulosic sugars derived from biomass to levulinic acid. Catal. Commun. 117, 19–25. doi: 10.1016/j.catcom.2018.04.016
Kruyer, N. S., Wauldron, N., Bommarius, A. S., and Peralta-Yahya, P. (2020). Fully biological production of adipic acid analogs from branched catechols. Sci. Rep. 10:13367. doi: 10.1038/s41598-020-70158-z
Lappalainen, K., and Dong, Y. (2019). Simultaneous production of furfural and levulinic acid from pine sawdust via acid-catalysed mechanical depolymerization and microwave irradiation. Biomass Bioenergy 123, 159–165. doi: 10.1016/j.biombioe.2019.02.017
Lee, W. S., Chua, A. S. M., Yeoh, H. K., Nittami, T., and Ngoh, G. C. (2015). Strategy for the biotransformation of fermented palm oil mill effluent into biodegradable polyhydroxyalkanoates by activated sludge. Chem. Eng. J. 269, 288–297. doi: 10.1016/j.cej.2015.01.103
Lee, S. Y., Kim, H. U., Chae, T. U., Cho, J. S., Kim, J. W., Shin, J. H., et al. (2019). A comprehensive metabolic map for production of bio-based chemicals. Nat. Catal. 2, 18–33. doi: 10.1038/s41929-018-0212-4
Li, M., and Wilkins, M. R. (2020). Recent advances in polyhydroxyalkanoate production: Feedstocks, strains and process developments. Int. J. Biol. Macromol. 156, 691–703. doi: 10.1016/j.ijbiomac.2020.04.082
Miscevic, D., Srirangan, K., Kefale, T., Abedi, D., Moo-Young, M., and Chou, C. P. (2019). Production of cellulosic butyrate and 3-hydroxybutyrate in engineered Escherichia coli. Appl. Microbiol. Biotechnol. 103, 5215–5230. doi: 10.1007/s00253-019-09815-x
Mohamad Fauzi, A. H., Chua, A. S. M., Yoon, L. W., Nittami, T., and Yeoh, H. K. (2019). Enrichment of PHA-accumulators for sustainable PHA production from crude glycerol. Process. Saf. Environ. Prot. 122, 200–208. doi: 10.1016/j.psep.2018.12.002
Morakile, T., Mandegari, M., Farzad, S., and Görgens, J. F. (2022). Comparative techno-economic assessment of sugarcane biorefineries producing glutamic acid, levulinic acid and xylitol from sugarcane. Ind. Crop. Prod. 184:115053. doi: 10.1016/j.indcrop.2022.115053
Oliveira, C. S. S., Silva, C. E., Carvalho, G., and Reis, M. A. (2017). Strategies for efficiently selecting PHA producing mixed microbial cultures using complex feedstocks: feast and famine regime and uncoupled carbon and nitrogen availabilities. New Biotechnol. 37, 69–79. doi: 10.1016/j.nbt.2016.10.008
Pinto-Ibieta, F., Cea, M., Cabrera, F., Abanto, M., Felissia, F. E., Area, M. C., et al. (2020). Strategy for biological co-production of levulinic acid and polyhydroxyalkanoates by using mixed microbial cultures fed with synthetic hemicellulose hydrolysate. Bioresour. Technol. 309:123323. doi: 10.1016/j.biortech.2020.123323
Pinto-Ibieta, F., Serrano, A., Cea, M., Ciudad, G., and Fermoso, F. G. (2021). Beyond PHA: stimulating intracellular accumulation of added-value compounds in mixed microbial cultures. Bioresour. Technol. 337:125381. doi: 10.1016/j.biortech.2021.125381
Prasertsung, I., Chutinate, P., Watthanaphanit, A., Saito, N., and Damrongsakkul, S. (2017). Conversion of cellulose into reducing sugar by solution plasma process (SPP). Carbohydr. Polym. 172, 230–236. doi: 10.1016/j.carbpol.2017.05.025
Raj, K., Partow, S., Correia, K., Khusnutdinova, A. N., Yakunin, A. F., and Mahadevan, R. (2018). Biocatalytic production of adipic acid from glucose using engineered Saccharomyces cerevisiae. Metab. Eng. Commun. 6, 28–32. doi: 10.1016/j.meteno.2018.02.001
Riveiro, E., González, B., and Domínguez, Á. (2020). Extraction of adipic, levulinic and succinic acids from water using TOPO-based deep eutectic solvents. Sep. Purif. Technol. 241:116692. doi: 10.1016/j.seppur.2020.116692
Rodriguez-Perez, S., Serrano, A., Pantión, A. A., and Alonso-Fariñas, B. (2018). Challenges of scaling-up PHA production from waste streams. A review. J. Environ. Manage. 205, 215–230. doi: 10.1016/j.jenvman.2017.09.083
Serafim, S., Lemos, P. C., Oliveira, R., and Reis, M. A. M. (2004). Optimization of Polyhydroxybutyrate production by mixed cultures submitted to aerobic dynamic feeding conditions. Biotechnol. Bioeng. 87, 145–160. doi: 10.1002/bit.20085
Ukawa-Sato, R., Hirano, N., and Fushimi, C. (2023). Design and techno–economic analysis of levulinic acid production process from biomass by using co-product formic acid as a catalyst with minimal waste generation. Chem. Eng. Res. Des. 192, 389–401. doi: 10.1016/j.cherd.2023.02.046
Vallejos, M. E., Felissia, F. E., and Area, M. C. (2017). Hydrothermal treatments applied to agro-and forest-industrial waste to produce high added-value compounds. BioRes. 12, 2058–2080. doi: 10.15376/biores.12.1.Vallejos
Wang, X., Oehmen, A., Freitas, E. B., Carvalho, G., and Reis, M. A. M. (2017). The link of feast-phase dissolved oxygen (DO) with substrate competition and microbial selection in PHA production. Water Res. 112, 269–278. doi: 10.1016/j.watres.2017.01.064
Wu, M., Di, J., Gong, L., He, Y.-C., Ma, C., and Deng, Y. (2023). Enhanced adipic acid production from sugarcane bagasse by a rapid room temperature pretreatment. Chem. Eng. J. 452:139320. doi: 10.1016/j.cej.2022.139320
Yin, F., Li, D., Ma, X., Li, J., and Qiu, Y. (2020). Poly (3-hydroxybutyrate-3-hydroxyvalerate) production from pretreated waste lignocellulosic hydrolysates and acetate co-substrate. Bioresour. Technol. 316:123911. doi: 10.1016/j.biortech.2020.123911
Yin, F., Li, D., Ma, X., and Zhang, C. (2019a). Bioresource technology pretreatment of lignocellulosic feedstock to produce fermentable sugars for poly (3-hydroxybutyrate-co-3-hydroxyvalerate) production using activated sludge. Bioresour. Technol. 290:121773. doi: 10.1016/j.biortech.2019.121773
Yin, F., Li, D., Ma, X., and Zhang, C. (2019b). Pretreatment of lignocellulosic feedstock to produce fermentable sugars for poly(3-hydroxybutyrate-co-3-hydroxyvalerate) production using activated sludge. Bioresour. Technol. 290:121773. doi: 10.1016/j.biortech.2019.121773
Zhang, L., Zhu, M., Shi, T., Gui, C., Huang, Y., Chen, Y., et al. (2016). Recovery of dietary fiber and polyphenols from grape juice pomace and evaluation of their functional properties and polyphenol composition. Food Funct. 7. doi: 10.1039/C6FO01423B
Zhang, X., Liu, Y., Wang, J., Zhao, Y., and Deng, Y. (2020). Biosynthesis of adipic acid in metabolically engineered Saccharomyces cerevisiae. J. Microbiol. 58, 1065–1075. doi: 10.1007/s12275-020-0261-7
Zhang, Z., Liu, B., and Zhao, Z. (2012). Efficient acid-catalyzed hydrolysis of cellulose in organic electrolyte solutions. Polym. Degrad. Stab. 97, 573–577. doi: 10.1016/j.polymdegradstab.2012.01.010
Zhangelinni, A. (2017). Fermentation route for the production of levulinic acid, levulinate esters and valerolantone and derivatives thereof. United States patent US 9,523,105
Zhao, M., Huang, D., Zhang, X., Koffas, M. A. G., Zhou, J., and Deng, Y. (2018). Metabolic engineering of Escherichia coli for producing adipic acid through the reverse adipate-degradation pathway. Metab. 47, 254–262. doi: 10.1016/j.ymben.2018.04.002
Keywords: adipic acid, levulinic acid, mixed microbial cultures (MMC), hemicellulose hydrolysate, feast and famine
Citation: Pinto-Ibieta F, Cea M, Serrano A, Felissia FE, Area MC, Cabrera F and Ciudad G (2023) Toward the use of mixed microbial cultures for the biological production of adipic and levulinic acid. Front. Microbiol. 14:1224543. doi: 10.3389/fmicb.2023.1224543
Edited by:
Annamalai Neelamegam, Dartmouth College, United StatesReviewed by:
Sathish Kumar Ramamoorthy, Sathyabama Institute of Science and Technology, IndiaBishal Sharma, Dartmouth College, United States
Copyright © 2023 Pinto-Ibieta, Cea, Serrano, Felissia, Area, Cabrera and Ciudad. This is an open-access article distributed under the terms of the Creative Commons Attribution License (CC BY). The use, distribution or reproduction in other forums is permitted, provided the original author(s) and the copyright owner(s) are credited and that the original publication in this journal is cited, in accordance with accepted academic practice. No use, distribution or reproduction is permitted which does not comply with these terms.
*Correspondence: Fernanda Pinto-Ibieta, fpinto@uct.cl