Neuroendocrine Regulation of Reproductive Dormancy in the Fruit Fly Drosophila melanogaster: A Review of Juvenile Hormone-Dependent Regulation
- 1Degree Programs in Life and Earth Sciences, Graduate School of Science and Technology, University of Tsukuba, Tsukuba, Japan
- 2Life Science Center for Survival Dynamics, Tsukuba Advanced Research Alliance, University of Tsukuba, Tsukuba, Japan
Animals can adjust their physiology, helping them survive and reproduce under a wide range of environmental conditions. One of the strategies to endure unfavorable environmental conditions such as low temperature and limited food supplies is dormancy. In some insect species, this may manifest as reproductive dormancy, which causes their reproductive organs to be severely depleted under conditions unsuitable for reproduction. Reproductive dormancy in insects is induced by a reduction in juvenile hormones synthesized in the corpus allatum (pl. corpora allata; CA) in response to winter-specific environmental cues, such as low temperatures and short-day length. In recent years, significant progress has been made in the study of dormancy-inducing conditions dependent on CA control mechanisms in Drosophila melanogaster. This review summarizes dormancy control mechanisms in D. melanogaster and discusses the implications for future studies of insect dormancy, particularly focusing on juvenile hormone-dependent regulation.
Introduction
In animals, neuroendocrine systems play a crucial role in facilitating adaptation to a wide variety of environments (Schmidt-Nielsen, 1997). In the temperate zone, winter is a challenging season for many animals because of the freezing temperatures and food shortages, which are sustained over several months. Hence, in winter, animals often suspend or slow down their normal physical functions, a process known as dormancy (Hand et al., 2016). To date, dormancy is recognized as an adaptive phenomenon in a wide variety of animal species (Hand et al., 2016).
Insects are one of the main animal groups that have been intensively used in dormancy studies. This is because control of insect dormancy could benefit many aspects of industry and agriculture. For example, since the silkworm Bombyx mori possesses an egg dormancy stage, a technology to flexibly regulate the induction and end of egg dormancy is useful to enable sericulture throughout the year (Yamashita and Yaginuma, 1991). In addition, pest dormancy studies are thought to be important because understanding the seasonal distribution of pests is critical for generating predictive models to accurately determine when pests are destructive (Denlinger, 2008). Moreover, there have been attempts to select specific dormancy phenotypes in biocontrol agents, which cannot become dormant in a season when crop pest populations continue to grow (Lirakis and Magalhães, 2019). In this regard, it is noteworthy that a branch of the United Nations promoted a coordinated research project on dormancy management to enable mass-rearing and increase the efficacy of sterile insects and natural enemies from 2014 to 2019 (Food and Agriculture Organization of the United Nations, 2013).
Previous studies have revealed that the developmental stages of dormancy differ between insect species (Tauber et al., 1986; Danks, 1987, 2006; Schiesari and O’Connor, 2013). For example, as described above, B. mori enters dormancy only in the egg stage (Yamashita and Yaginuma, 1991). However, in other species, dormancy is not restricted to the egg but can occur at other stages, including the adult life. Adult dormancy results in many metabolic and behavioral changes, such as the slow-down of reproduction, decreased food ingestion, suppressed metabolism, increased stress resistance, and extended adult lifespan (Danks, 1987; Tatar and Yin, 2001; Hahn and Denlinger, 2011; Kubrak et al., 2014). Among the multiple changes, reproductive slow-down is thought to be a key event of adult dormancy, known as reproductive dormancy. Since adult insects allocate a considerable amount of energy to gametogenesis, reproductive dormancy allows insects to reduce their energy consumption and produce offspring again in the spring when winter is over. Reproductive dormancy is found in both male and female adults; In particular, females exhibit a drastic suppression of gametogenesis (oogenesis) by inhibiting vitellogenesis, accessory gland activity, and mating behavior (Saunders et al., 1989; Pener, 1992; Kubrak et al., 2016).
Previous studies have shown that reproductive dormancy and other types of dormancy are regulated by a complex interplay of multiple hormones and neurotransmitters in insects (Denlinger, 2002; Emerson et al., 2009a; Denlinger et al., 2012). In particular, several major hormones influence reproductive dormancy, including juvenile hormones (JHs), insulin-like peptides, and ecdysteroids (Denlinger, 2002; Emerson et al., 2009a; Denlinger et al., 2012). All of three classes of hormones are known to have a stimulatory effect on ovarian development, and a decrease in these signals in dormant individuals suppresses ovarian development (Denlinger, 2002; Emerson et al., 2009a; Denlinger et al., 2012; Uryu et al., 2015; Lenaerts et al., 2019; Santos et al., 2019; Swevers, 2019; Semaniuk et al., 2021). Among these three, JHs, the arthropod-specific sesquiterpenoid hormones, have been the most intensively studied in the long history of entomology to unravel their vital role in regulating reproductive dormancy in female adults. Since JHs are essential for promoting vitellogenesis in normal (non-dormancy-inducing) conditions, the reduction in JH levels is conversely required for suppressing vitellogenesis, leading to reproductive dormancy in females (Denlinger et al., 2012; Santos et al., 2019). This mechanism was well demonstrated in a study using the Colorado potato beetle Leptinotarsa decemlineata (de Wilde and de Boer, 1961, 1969; Schooneveld et al., 1977; Kort, 1990), which has frequently been used for studies on reproductive dormancy. After hatching under short-day condition, the adults burrow into the soil and enter dormancy. When the endocrine organ known as the corpus allatum (pl. corpora allata; CA), responsible for biosynthesizing JHs, is dissected out from a non-dormant beetle and then transplanted to a dormant beetle, the dormant status is released from the beetle receiving the transplant. Such release of dormancy is also observed when JH is applied to a dormant beetle. In contrast, when the CA of a non-dormant beetle is surgically ablated, the ovarian development is inhibited even under long-day (non-dormancy-inducing) conditions. These results indicate that the reduced concentration of JH, which is biosynthesized in the CA, causes reproductive dormancy in L. decemlineata females. After this discovery, the importance of JH reduction to induce female reproductive dormancy has been confirmed in many insect species (Denlinger et al., 2012; Hand et al., 2016). Therefore, there must be essential, common mechanisms by which information about dormancy-inducing environmental cues, such as low temperature and short-day condition, are transmitted to the CA to control JH biosynthesis or release. However, the mechanisms at the molecular, cellular, and neuroendocrine levels have not yet been fully elucidated.
Among several insects used in laboratories, the fruit fly Drosophila melanogaster has contributed substantially to the discovery of various biological phenomena based on powerful genetic tools such as the GAL4-UAS system (Brand and Perrimon, 1993; Neckameyer and Argue, 2013). In the last decade, significant progress has been made in the study of reproductive dormancy in females of D. melanogaster. Specifically, there have been several recent studies to address how dormancy-inducing stimuli are transmitted to the CA to regulate reproductive dormancy through the reduction of JH titer. Importantly, a part of D. melanogaster research is beginning to contribute to understanding the molecular mechanisms of reproductive dormancy in other insects, as we describe below. This review summarizes current knowledge about the mechanisms of reproductive dormancy in D. melanogaster females and discusses the remaining questions of the D. melanogaster dormancy, particularly focusing on juvenile hormone-dependent regulation.
An Overview of Drosophila Melanogaster Dormancy
First, we would like to define our usage of the term “dormancy.” In general, dormancy can be broadly classified into two types, diapause and quiescence. Diapause is induced by seasonal cues that do not directly prevent development but foreshow the arrival of unfavorable conditions. On the other hand, quiescence is characterized by slowed metabolism and directly results from unfavorable environmental conditions such as low temperature (Koštál, 2006; Schiesari et al., 2011; Schiesari and O’Connor, 2013). There is still a debate about whether reproductive dormancy in D. melanogaster should be classified as diapause or quiescence (Tatar et al., 2001; Koštál, 2006; Lirakis et al., 2018). However, it seems obvious that reproductive dormancy in D. melanogaster is influenced by both short-day conditions and low temperature (Schiesari et al., 2016; Zonato et al., 2017; Figure 1). This fact implies that reproductive dormancy in D. melanogaster has both quiescence and diapause properties. Therefore, most papers published in recent years simply use “dormancy” to describe the short-day and low temperature-induced changes in D. melanogaster adults (Kubrak et al., 2016; Liu et al., 2016; Andreatta et al., 2018; Lirakis et al., 2018; Ojima et al., 2018; Nagy et al., 2019; Abrieux et al., 2020). In this paper, as is customary, we will refer to the D. melanogaster phenotypes in terms of dormancy.
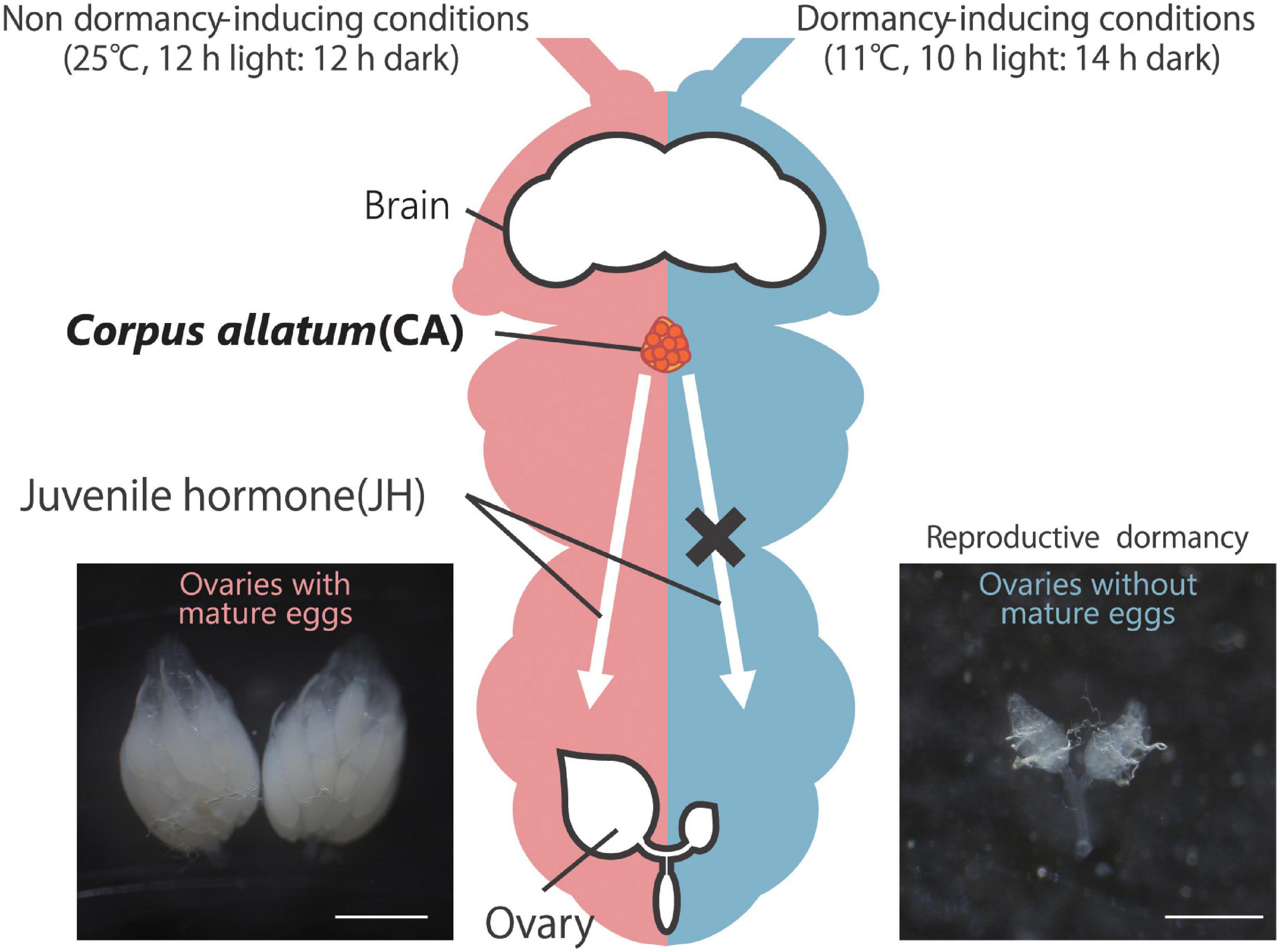
Figure 1. Reproductive dormancy in Drosophila melanogaster adult females. Under non-dormancy-inducing conditions (25°C, 12 h light:12 h dark; left), approximately 20–60 mature eggs, in which yolk proteins are well accumulated, are observed in the female abdomen. On the other hand, under dormancy-inducing conditions (11°C, 10 h light:14 h dark; right), oogenesis is severely suppressed, and mature eggs with yolk protein are rarely observed. In non-dormant flies, yolk protein accumulation is positively controlled by juvenile hormone (JH) produced in and secreted from the corpus allatum (CA, orange). Conversely, in dormant flies, JH biosynthesis in the CA is suppressed, leading to the slow-down of oogenesis. Scale bars, 0.5 mm.
In laboratories, D. melanogaster dormancy is commonly induced by transferring virgin females into dormancy-inducing conditions shortly after (usually 2–6 h) eclosion under non-dormancy-inducing conditions. Such newly eclosed female flies are supposed to have previtellogenic or even few early vitellogenic egg chambers but not mature eggs. When the newly eclosed females are maintained in dormancy-inducing conditions, the flies continuously keep the immature ovaries even 2 or 3 weeks after eclosion (Zonato et al., 2017; Lirakis et al., 2018; Figure 1). However, since reproductive dormancy is a “slow-down” and not a complete arrest, in ovarian development, the percentage of non-dormant flies increases about 4 weeks after hatching (Schiesari et al., 2016; Zonato et al., 2017; Lirakis et al., 2018). Meanwhile, rearing flies under conditions of temperature and day length that mimic winter can prolong the slow-down of oogenesis and maintain a higher reproductive dormancy rate than simple dormancy-inducing conditions even 3 months after eclosion (Zonato et al., 2017). It is still unclear why such environmental fluctuation is important to extend reproductive dormancy.
Under dormancy-inducing conditions in laboratories, D. melanogaster adults display some physiological changes, such as reduced metabolism (Saunders et al., 1989; Saunders and Gilbert, 1990; Tatar and Yin, 2001; Tatar et al., 2001; Kubrak et al., 2014; Anduaga et al., 2018). Previous studies have shown that absolute amounts of trehalose and glucose positively correlate with the dormancy rate (Kubrak et al., 2014; Anduaga et al., 2018). In addition, trehalose/glycogen and trehalose/glucose ratios also positively correlate with the dormancy rate (Watanabe et al., 2002; Kubrak et al., 2014; Anduaga et al., 2018). These observations raise a hypothesis that the biosynthesis of trehalose, an energy source and an anti-freezing material, is activated in dormant flies (Crowe, 2007; MacRae, 2010; Hahn and Denlinger, 2011). On the other hand, protein amount does not correlates with dormancy rate (Kubrak et al., 2014; Anduaga et al., 2018). Dormancy-associated physiological changes also include an increase in stress tolerance, an extended lifespan, and the activation of the innate immune system in both male and female fly adults (Saunders et al., 1989; Saunders and Gilbert, 1990; Tatar et al., 2001; Tatar and Yin, 2001; Kubrak et al., 2014; Kuèerová et al., 2016; Anduaga et al., 2018). In addition, in D. melanogaster dormant females, the intestine is markedly shortened (Kubrak et al., 2014). Some of these physiological changes are also observed in other insects when they enter dormancy (Tatar and Yin, 2001; Denlinger, 2002; Hahn and Denlinger, 2007, 2011; Sim and Denlinger, 2013).
Along with the changes in metabolic state, suppression of vitellogenesis in oogenesis is another major aspect of dormancy (Figure 2); hence this process is called reproductive dormancy. Among the 14 distinct stages of D. melanogaster oogenesis (King, 1970; Bastock and St Johnston, 2008), vitellogenesis occurs from stage 8 (Bownes, 1994; Soller et al., 1997). Therefore, oocytes before and at stage 7 are defined as previtellogenic oocytes, while those after and at stage 8 are defined as vitellogenic oocytes. Most studies classify flies as dormant if their ovaries have only previtellogenic egg chambers, while flies with ovaries containing vitellogenic egg chambers are characterized as non-dormant (Saunders et al., 1989, 1990; Saunders, 1990; Saunders and Gilbert, 1990; Williams, 1993; Richard et al., 1998; Tatar et al., 2001; Schmidt and Conde, 2006; Williams et al., 2006; Schmidt and Paaby, 2008; Schmidt et al., 2008; Emerson et al., 2009b; Lee et al., 2011; Fabian et al., 2015; Schiesari et al., 2016; Zhao et al., 2016; Zonato et al., 2017; Andreatta et al., 2018; Ojima et al., 2018; Nagy et al., 2019; Figure 1). However, there is still an ongoing debate about whether this commonly used classification is truly valid, as discussed in several papers (Tatar et al., 2001; Lee et al., 2011; Lirakis et al., 2018; Erickson et al., 2020). These papers claim that oogenesis in dormancy-inducing conditions is not arrested at provitellogenic stages but rather at stage 9 corresponding to early vitellogenesis. In fact, stage 9 oocytes are largely different from stage 10 and later oocytes from the following perspectives. First, stage 10 oocytes massively grow and are drastically enlarged compared to stage 9 oocytes (He et al., 2011). Second, stage 9 oocytes possess a very small amount of yolk, but stage 10 oocytes accumulate it well (He et al., 2011). Third, stage 8 oocytes self-synthesize yolk proteins, while stage 10 oocytes incorporate extracellular yolk proteins synthesized in the fat body cells and released into the hemolymph (Brennan et al., 1982). Importantly, several studies have shown that dormant oocytes incorporate hemolymph yolk proteins in lesser amounts (Saunders, 1990; Saunders et al., 1990; Richard et al., 2001b). This issue is still being debated.
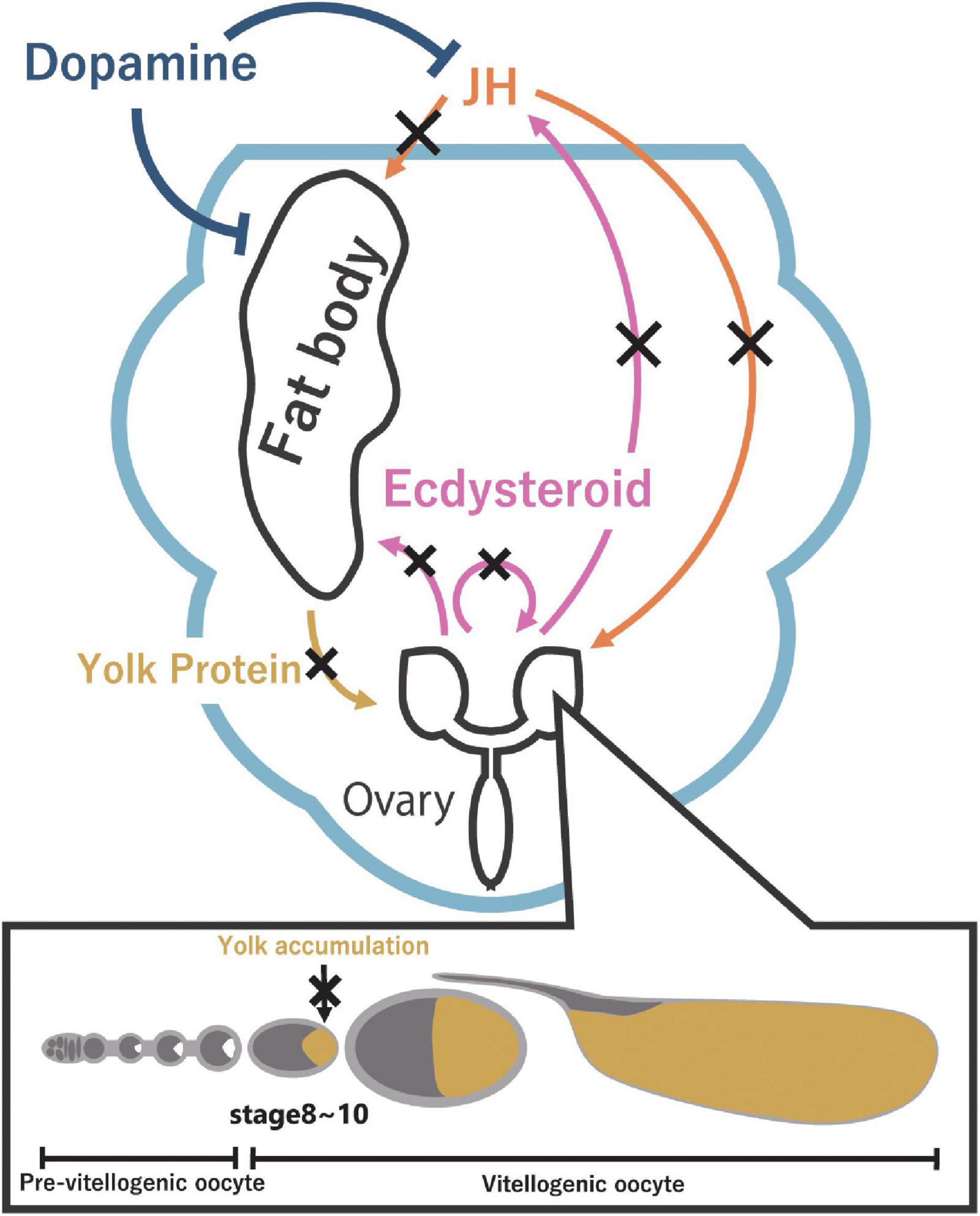
Figure 2. Reproductive dormancy is regulated by JH through the production of yolk protein in the fat body. JH (orange) promotes the production of yolk protein in the fat body and the uptake of yolk protein in the ovary. On the other hand, ecdysteroids (pink) promote yolk protein production in the fat body, affect JH biosynthesis in the CA, and regulate oogenesis. Under dormancy-inducing conditions, a decrease in JH level and ecdysteroid level suppresses the yolk protein accumulation (dark yellow) in oocytes, leading to reproductive dormancy. Furthermore, dopamine (dark blue) might be involved in inducing reproductive dormancy via yolk protein production in the fat body.
Previous laboratory studies have reported that, like other insects, low temperature and short-day condition influence the induction of dormancy in D. melanogaster adults (Saunders et al., 1989; Saunders and Gilbert, 1990; Allen, 2007; Figure 1). However, in contrast to the importance of short-day condition to induce dormancy in many other insect species, it is known that low temperature, but not short-day condition, is the crucial dormancy stimulus in D. melanogaster (Saunders and Gilbert, 1990; Zonato et al., 2017; Anduaga et al., 2018). In other words, D. melanogaster adults enter dormancy at temperatures less than 13∘C even in long-day condition, but they never enter dormancy in short-day condition if the temperature is higher than 13°C. In D. melanogaster, the photoperiod appears to modulate reproductive dormancy within a 10–13°C range of permissiveness temperature (Saunders et al., 1989; Saunders and Gilbert, 1990; Schiesari et al., 2011). Curiously, a recent study has suggested that a very subtle difference in temperature, such as a 0.3°C difference, possibly has a significant impact on the induction of dormancy (Anduaga et al., 2018).
A concern about most laboratory experiments is that these previous data were obtained under artificial light on/off conditions, which is the unnatural constant light-constant dark photoperiodic cycle. However, a recent study has claimed that such artificial light on/off conditions does not reflect natural light/dark change and, thus, underestimate the effect of photoperiod on D. melanogaster reproductive dormancy (Nagy et al., 2018). This study revealed that a photoperiod-dependent reproductive dormancy is more clearly observed in D. melanogaster European strains when the animals are reared under gradual elevation and reduction of light that mimic the natural light/dark change (Nagy et al., 2018). In addition to low temperature and short-day condition, starvation is an additional factor that enhances dormancy rate (Lirakis et al., 2018; Ojima et al., 2018).
In the wild, it has been well described that there are geographic variations in reproductive dormancy in D. melanogaster (Williams et al., 2006; Tauber et al., 2007; Sandrelli et al., 2007; Schmidt and Paaby, 2008; Schmidt et al., 2008; Emerson et al., 2009b; Kolaczkowski et al., 2011; Lee et al., 2011; Fabian et al., 2012; Bergland et al., 2014; Kapun et al., 2016; Zhao et al., 2016; Zonato et al., 2017; Lirakis et al., 2018; Betancourt et al., 2021; Machado et al., 2021). D. melanogaster originated in sub-Saharan Africa and subsequently expanded its habitat, possibly in association with humans and agriculture (Flatt, 2020). The flies have colonized temperate habitats in Eurasia and, more recently, North America and Australia. Genotypes from some populations enter reproductive dormancy readily in response to low temperature and short-day conditions, whereas others have low or zero dormancy propensity (Williams, 1993; Schmidt and Paaby, 2008; Emerson et al., 2009b; Fabian et al., 2015). Seasonal high-latitude populations show much greater dormancy inducibility than flies from subtropical/tropical low-latitude populations. In addition, geographic variations in reproductive dormancy in wild D. melanogaster are associated with single nucleotide polymorphisms in several loci, as we discuss later, suggesting that the traits of reproductive dormancy are genetically constrained.
The Role of Juvenile Hormones in Drosophila Melanogaster Reproductive Dormancy
As in other insect species, the induction of D. melanogaster reproductive dormancy is associated with reduced JH levels (Figure 1). A previous study using an in vitro assay system has shown that the dissected CA from animals under reproductive dormancy-inducing conditions exhibits reduced JH biosynthesis (Saunders et al., 1990). In contrast, administration of the JH analog methoprene can break reproductive dormancy (Tatar and Yin, 2001). Phenotypes of reproductive dormancy are also observed in female flies in which JH biosynthetic enzymes are enhanced or suppressed. For example, overexpression of a gene encoding juvenile hormone acid O-methyltransferase (Jhamt) significantly reduces the percentage of dormant flies (Shinoda and Itoyama, 2003; Niwa et al., 2008; Noriega, 2014; Ojima et al., 2018). Conversely, the CA-specific transgenic double-strand RNA interference (RNAi) of Jhamt increases the percentage of dormant flies (Ojima et al., 2018). Taken together, these observations are consistent with the fact that JH accelerates oogenesis in most insects, including D. melanogaster, under non-dormancy-inducing conditions.
The role of JH in the regulation of D. melanogaster reproductive dormancy has also been investigated at the level of oogenesis. It is well known that JH is essential for facilitating vitellogenesis from the oocyte stage 8 (Bownes, 1994; Soller et al., 1997). More precisely, JH promotes yolk protein production, which occurs mainly in the fat body, and also acts on the ovarian follicle cells to promote vitellogenesis by enhancing yolk protein uptake to oocytes (Postlethwait and Weiser, 1973; Bownes, 1989; Soller et al., 1997, 1999; Richard et al., 2001a; Santos et al., 2019). The suppression of yolk protein production in the dormancy-inducing condition correlates well with reproductive dormancy (Saunders et al., 1990; Figure 2). Experimentally, application of the JH analog to dormant females increased yolk protein production and the number of vitellogenic oocytes as compared to an experimental control group (Saunders et al., 1990; Tatar et al., 2001). These data indicate that, in D. melanogaster and many other insects, reproductive dormancy is due to the suppression of vitellogenesis, at least in part, by JH titer reduction.
Neuroendocrine Mechanisms That Directly or Indirectly Control Juvenile Hormone Biosynthesis in the Corpus Allatum in Drosophila Melanogaster
To understand how JH biosynthesis in the CA is suppressed when the animals are placed in dormancy-inducing conditions, it is important to unravel the mechanisms transmitting environmental cues to the CA to influence the activity of JH biosynthesis. Although the mechanisms have not yet been fully elucidated, recent studies on D. melanogaster have collectively argued that several neuroendocrine factors are crucial for inducing JH-mediated reproductive dormancy. Hereafter we are describing the recent knowledge of neuroendocrine regulation of reproductive dormancy in D. melanogaster. As we described earlier, insect reproductive dormancy is regulated not only by JHs but also many other hormones, including insulin-like peptides and ecdysteroids. Although it might be plausible to consider that insulin-like peptides and ecdysteroids have roles in reproductive dormancy independent of JHs, recent D. melanogaster studies have indicated that insulin-like peptides and ecdysteroids influence JH biosynthesis in the CA in multiple way. Therefore, in this review, instead of deliberately focusing on the whole picture of hormone interactions, we dare to focus on JHs and discuss the involvement of other hormones and neurotransmitters.
Mechanisms of Insulin Signaling-Mediated Reproductive Dormancy
Insulin signaling controls development, growth, and physiology by regulating nutrient metabolism and energy expenditure in animals. In D. melanogaster, insulin signaling has versatile functions, including reproductive dormancy (Sim and Denlinger, 2013; Kubrak et al., 2014; Liu et al., 2016; Schiesari et al., 2016; Ojima et al., 2018; Semaniuk et al., 2021). Drosophila insulin-like peptides (Dilps), orthologs of vertebrate insulin and insulin growth factors, are the endocrine factors activating insulin signaling in D. melanogaster (Semaniuk et al., 2021; Figures 3A,B). Their secretion is regulated by the activity of insulin-producing cells (IPCs), which are neurons located in the pars intercerebralis (PI) of the brain. Dilps are then released from IPCs into the hemolymph (Semaniuk et al., 2021; Figure 3A). A previous study has demonstrated that, in flies reared at 11°C, short-day condition results in lower neuronal activity of IPCs than long-day condition (Ojima et al., 2018). Furthermore, when IPCs are artificially activated by overexpression of a transient receptor potential cation channel under dormancy-inducing conditions, the percentage of dormant flies decreases. Conversely, an artificial inhibition of IPCs by overexpressing an inward rectifier potassium channel increases the percentage of dormant flies (Venken et al., 2011; Schiesari et al., 2016; Ojima et al., 2018). These results suggest that IPCs negatively regulate reproductive dormancy. Phenotypes of reproductive dormancy are also observed in loss- and gain-of-function of Dilps and insulin receptor (InR). For example, overexpression of Dilp2 and Dilp5 in the IPCs suppresses the induction of dormancy (Schiesari et al., 2016). On the other hand, IPC-specific RNAi of Dilp2, Dilp5 upregulates the induction of dormancy (Schiesari et al., 2016). Moreover, molecules downstream of Dilps and InR are also involved in reproductive dormancy. For example, Phosphatidylinositol-3 kinase (PI3K) influences of the rate of reproductive dormancy in the North American population of D. melanogaster (Williams et al., 2006). In addition, FoxO, a well-known transcription factor downstream of Dilp signaling, also plays a crucial role in inducing reproductive dormancy, as revealed by an experiment using a FoxO-mediated gene expression reporter (Schiesari et al., 2016). Taken together, the production and secretion of Dilps are greatly suppressed in dormancy-inducing conditions, resulting in reduced insulin signaling, followed by the suppression of oogenesis (Figure 3B).
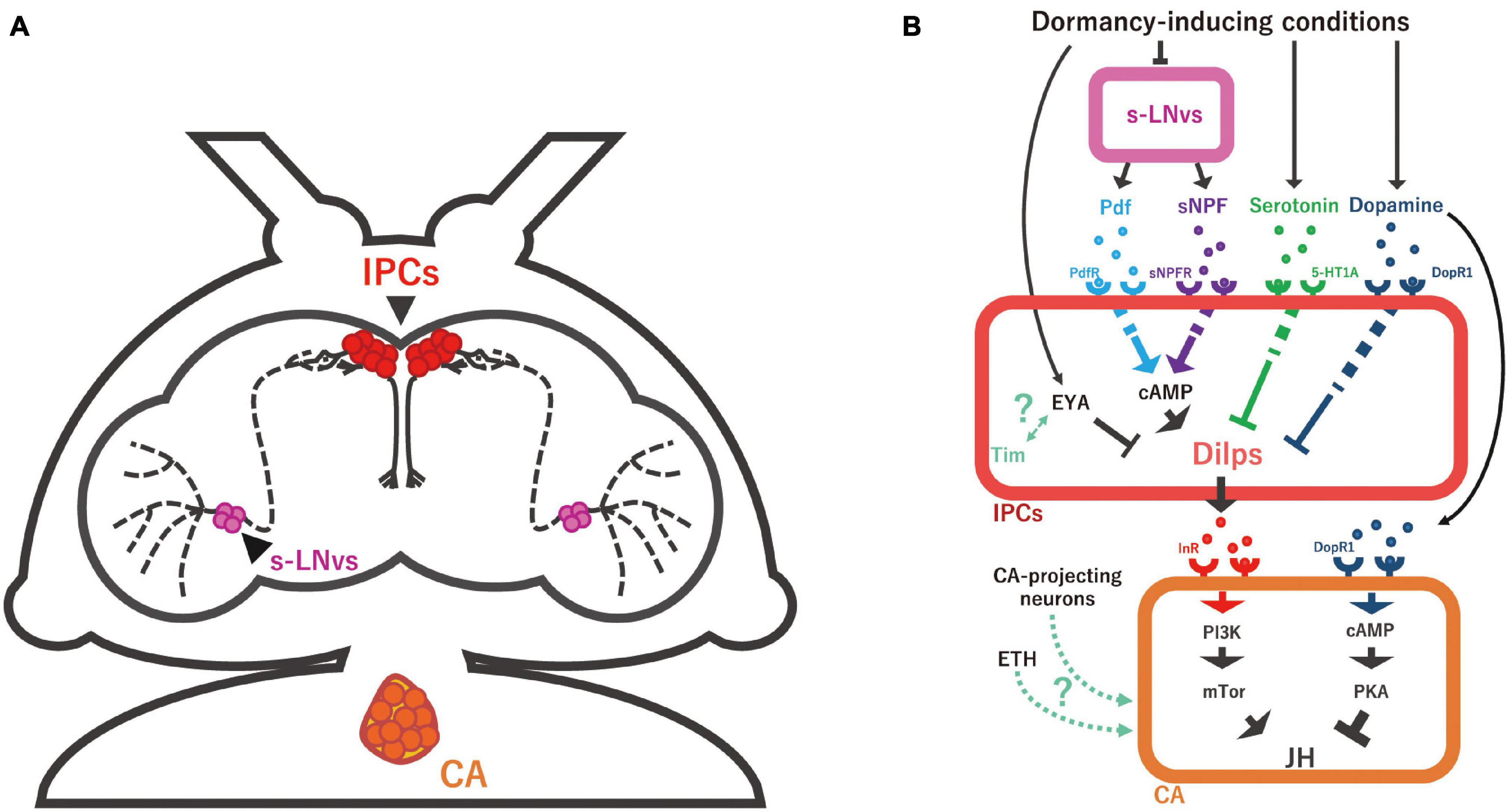
Figure 3. Schematic representation of the neuroendocrine mechanisms regulating JH biosynthesis. (A) Anatomy of small ventral lateral neurons (s-LNv; magenta), insulin-producing cells (IPCs; red), and the corpus allatum (CA) in D. melanogaster adult female. s-LNvs are the circadian clock pacemaker neurons whose axons seem to have direct synaptic connections with IPCs. (B) The s-LNv-IPC-CA axis and multiple humoral factors to regulate JH biosynthesis in the CA. Under dormant conditions, the secretion of pigment-dispersing factor (Pdf) and short neuropeptide F (sNPF) from s-LNv is reduced, followed by the suppression of IPC activity and consequently that of Dilp production. Serotonin and dopamine are also involved in inducing reproductive dormancy by repressing Dilp expression in IPCs. It is noteworthy that, whereas it is generally considered that serotonin receptor (5-HT1A) and dopamine receptor (DopR1) positively evoke intracellular cAMP level, it is unclear how serotonin-5-HT1A and dopamine-DopR1 signaling negatively regulate Dilp expression, differently from Pdf-Pdf receptor (PdfR) and sNPF-sNPF receptor (sNPFR) signaling. In addition to these hormones and neurotransmitters, Dilp expression is intracellularly regulated by the transcriptional regulator Eye absent (Eya) and possibly the core circadian clock gene timeless (Tim). Dilps and Ecdysis-triggering hormone (ETH) have a function to promote JH biosynthesis in the CA. On the other hand, dopamine has a function to suppress JH production in the CA under dormancy-inducing conditions. In many insects, direct projection nerves of the CA are essential for the regulation of reproductive dormancy, while it is still unclear whether this is also the case in D. melanogaster.
How does the reduction of Dilp signaling suppress oogenesis? One mechanism is that reduced Dilp signaling in the CA downregulates JH biosynthesis (Figure 3B). For example, overexpression of constitutively active InR or PI3K in the CA significantly decreases in flies with ovarian arrest in dormancy-inducing conditions (Ojima et al., 2018). Conversely, InR RNAi or dominant-negative PI3K or mTOR, another downstream element of insulin signaling, increases the proportion of flies with ovarian arrest in both normal and dormancy-inducing conditions (Ojima et al., 2018). On the other hand, besides the JH-mediated action of Dilps, there are other mechanisms by which Dilps directly act on ovarian cells to regulate oogenesis or indirectly influence oogenesis through the regulation of ecdysteroid biosynthesis (Tu et al., 2002, 2005; LaFever and Drummond-Barbosa, 2005).
The role of insulin signaling in controlling developmental arrest has also been suggested in species other than insects, such as the nematode Caenorhabditis elegans and the annual killifish Austrofundulus limnaeus. In C. elegans, low food levels or other unfavorable environmental conditions lead to larval developmental arrest, known as dauer (Hu, 2007). Previous studies have identified and characterized genes mutated in various mutants exhibiting dysregulation of dauer arrest, called daf mutants. Some of the identified genes encode insulin-like peptides, insulin receptor, and signal transduction molecules downstream of insulin receptor (Golden and Riddle, 1982, 1984; Cornils et al., 2011; Sim and Denlinger, 2013). In the annual killifish, downregulation of insulin signaling appears essential for regulating embryonic diapause (Woll and Podrabsky, 2017). Considering that JHs are arthropod-specific and neither nematodes nor vertebrates biosynthesize JHs, it would be intriguing to examine whether and how insulin signaling interacts with other hormones and other bioactive molecules to regulate developmental arrest in these animals, such as dafachronic acids in C. elegans (Hu, 2007; Niwa and Niwa, 2014) and vitamin D3 in the annual killifish (Romney et al., 2018).
Mechanisms of Aminergic Signaling-Mediated Reproductive Dormancy
Previous studies on pupal dormancy in butterflies and moths have shown that hemolymph levels of aminergic neurotransmitters, such as dopamine and serotonin, are elevated during dormancy (Noguchi and Hayakawa, 1997; Isabel et al., 2001; Hsu et al., 2020; Figure 3B). Recent studies have revealed that serotonin and dopamine are also involved in regulating reproductive dormancy in D. melanogaster (Andreatta et al., 2018).
In D. melanogaster, the dopamine level in the whole adult body significantly increases under dormancy-inducing conditions (Andreatta et al., 2018). Furthermore, the percentage of flies with reproductive dormancy is reduced in mutants of DOPA decarboxylase (Ddc), which is involved in the biosynthesis of dopamine and serotonin (Sherald and Wright, 1974; Wright et al., 1976). On the other hand, activation of the dopaminergic neurons results in an increased proportion of flies with reproductive dormancy. These results indicate that dopamine, in contrast to Dilps, positively regulates reproductive dormancy. However, among the approximately 130 bona fide dopaminergic neurons in the D. melanogaster brain (Kasture et al., 2018; Karam et al., 2020), the dopaminergic neuron(s) important for inducing reproductive dormancy have not yet been identified.
A previous study has revealed that Dop1R1, the gene encoding one of the dopamine receptors, is expressed at the CA and the fat body (Gruntenko et al., 2012; Figure 3B). Knockdown of Dop1R1 either in the CA or the fat body significantly reduces the percentage of dormant flies (Andreatta et al., 2018), suggesting that dopamine induces reproductive dormancy via both the CA and the fat body. It should be remembered that the fat body is the main organ producing yolk protein, which is essential for vitellogenesis (Bownes, 1994). Therefore, these data raise the possibility that dopamine might inhibit yolk protein production in the fat body, which indirectly suppresses vitellogenesis in dormant flies (Figure 2). Besides the CA and the fat body, dopamine seems to act on IPCs as well. While it is unclear whether any of the dopamine receptors are expressed in IPCs, Dop1R1 knockdown in IPCs also reduces the percentage of dormant flies (Andreatta et al., 2018). Therefore, Dilps production may be reduced when the IPCs receive dopamine (Figure 3B). Taken together, these data indicate that dopamine simultaneously acts on multiple tissues to regulate reproductive dormancy.
It is known that Dop1R1 is coupled with the stimulatory trimeric G-protein, known as Gs, and utilizes intracellular cyclic AMP (cAMP) as a second messenger (Gotzes et al., 1994; Sugamori et al., 1995). A previous study has shown that the CA-specific forced expression of a dominant-negative form of protein kinase A (PKA), a cAMP-activating kinase (Francis and Corbin, 1994), represses reproductive dormancy in the dormancy-inducing condition (Andreatta et al., 2018). Moreover, the animals in this study exhibited expression changes in JH-responsive genes, which seemed to reflect the increased JH titer. These results are also consistent with the involvement of Dop1R1 signaling in the CA in regulating reproductive dormancy (Figure 3B).
In addition to dopamine, serotonin also regulates reproductive dormancy in D. melanogaster. Artificial neuronal activation of serotonergic neurons by overexpressing a voltage-gated ion channel causes a significant increase in the percentage of dormant flies (Venken et al., 2011; Andreatta et al., 2018). Furthermore, one of the serotonin receptors, 5-HT1A, is expressed in IPCs and negatively regulates IPCs (Luo et al., 2012, 2014). Knockdown of 5-HT1A in IPCs greatly reduces the percentage of dormant flies. Consistent with this observation, the IPC-specific knockdown of 5-HT1A enhances the expression levels of Dilp2 and Dilp5. These results indicate that serotonin acts on IPCs to promote dormancy via regulation of insulin signaling (Figure 3B).
Other bioactive monoamines include octopamine and γ-aminobutyric acid (GABA) (Nässel, 2018). It has been demonstrated that the activation of octopaminergic neurons suppresses reproductive dormancy, while GABAergic signaling does not consistently affect dormancy (Andreatta et al., 2018). Since the octopaminergic signal is known to influence the production and release of Dilps in non-dormancy-inducing conditions (Enell et al., 2010; Luo et al., 2014), a previous study has examined whether octopamine regulates reproductive dormancy by acting on IPCs. However, the silencing of the octopamine receptor in mushroom bodies (OAMB) in the IPCs does not affect the percentage of dormant flies (Andreatta et al., 2018). On the other hand, a previous study has suggested that octopamine suppresses JH degradation, leading to a rise in JH titer under heat stress (Gruntenko et al., 2000; Rauschenbach et al., 2001). Although this study does not focus on reproductive dormancy, this observation implies that activation of octopaminergic neurons may facilitate reproductive dormancy. However, it is still unclear which tissues receive octopamine and whether octopamine-dependent control of reproductive dormancy requires JH signaling.
Mechanisms of Ecdysteroid Signaling-Mediated Reproductive Dormancy
The classical scheme of insect endocrinology describes how insect developmental transitions are regulated by three primary circulating factors: prothoracicotropic hormone, JHs, and ecdysteroids (Jindra et al., 2013; Truman, 2019). In D. melanogaster, besides JHs, ecdysteroids are also known to play an important role in regulating reproductive dormancy (Richard et al., 1998, 2001b). Ovaries of reproductively dormant flies exhibit lower ecdysteroid biosynthesis activity in vitro compared to those of non-dormant flies (Richard et al., 1998). On the other hand, the ovarian ecdysteroid level is elevated during the dormancy-breaking process (Richard et al., 1998). The elevation of the ovarian ecdysteroids occurs before mature eggs start to be produced in the dormancy-broken ovaries. Moreover, microinjection of 20-hydroxyecdysone (20E), the most active form of ecdysteroid, to reproductively dormant females evokes vitellogenesis (Richard et al., 1998, 2001b). Notably, vitellogenesis is severely suppressed in the ecdysone receptor (EcR) loss-of-function ovaries in non-dormant flies (Carney and Bender, 2000). Taken together, these results indicate that ecdysteroid biosynthesis in the ovary needs to be suppressed in the dormancy-inducing condition, while the elevation of the ovarian ecdysteroid level and the activation of 20E signaling are required for breaking reproductive dormancy (Figure 2).
Currently, however, the role of ecdysteroids in the regulation of reproductive dormancy in D. melanogaster has not been as well documented as that of the JHs. It is also unclear how the ovarian ecdysteroid levels are suppressed in the dormancy-inducing condition. Nevertheless, it has been hypothesized that three major pathways might be involved in the 20E-mediated regulation of reproductive dormancy. First, the downregulation of 20E signaling in the fat body seems to suppress yolk protein production in the dormancy-inducing condition, as 20E stimulates the fat body in non-dormant females, leading to the promotion of yolk protein production (Postlethwait and Handler, 1979; Jowett and Postlethwait, 1980; Bownes, 1982; Handler and Maroy, 1989; Bownes et al., 1996; Richard et al., 2001a). Second, the downregulation of 20E signaling in the ovary might suppress many aspects of oogenesis in the dormancy-inducing condition. Previous studies have indicated that 20E has versatile roles in D. melanogaster oogenesis, including niche formation, germline stem cell proliferation and maintenance, cystocyte differentiation, and the developmental checkpoint at the stage 8–10 egg chambers (Belles and Piulachs, 2015; Uryu et al., 2015; Ameku and Niwa, 2016; Ameku et al., 2017; Swevers, 2019). Third, the downregulation of 20E signaling might suppress JH biosynthesis in the CA in the dormancy-inducing condition. In fact, very recent studies have just revealed the relationship between 20E signaling and JH biosynthesis, which is at least in part mediated by the peptide hormone ecdysis-triggering hormone (ETH). We provide more details in the next section.
Mechanisms of Ecdysis-Triggering Hormone-Mediated Reproductive Dormancy
ETH has been identified and characterized as the peptide hormone playing an essential role in regulating innate ecdysis behavior in the tobacco horn moth Manduca sexta and D. melanogaster (Žitòan et al., 1996; Kingan et al., 1997; Park et al., 1999). ETH is produced by Inka cells located close to tracheal pits in the thorax and abdomen and then secreted into the hemolymph. While early studies have shown that the released ETH is received in the brain, after which there is activation of a neuroendocrine mechanism to control ecdysis behavior, the recent studies using non-dormant D. melanogaster have also revealed that ETH has a function to promote JH biosynthesis in the CA, leading to ovarian development in females and mating behavior in males (Lee et al., 2017; Meiselman et al., 2017). In females, knockdown of ETH results in the reduction of JH levels and slow-down of ovarian development (Lee et al., 2017; Meiselman et al., 2017). In addition, live imaging experiments have shown that ETH is directly received by the ETH receptor on the CA to elevate intracellular Ca2+ level (Lee et al., 2017; Meiselman et al., 2017). Curiously, it is known that ETH production is promoted by 20E (Park et al., 1999). Therefore, it is plausible to expect that, in dormancy-inducing conditions, the downregulations of ecdysteroid biosynthesis and 20E signaling indirectly suppress JH biosynthesis via ETH, yet this fascinating possibility has not been experimentally tested in D. melanogaster so far (Figure 3B).
Interestingly, a recent study has shown that ecdysteroids regulate dormancy via ETH in the cabbage beetle Colaphellus bowringi (Guo et al., 2021). In this insect, reduction of ecdysteroid biosynthesis is required for suppressed oogenesis and accumulated lipid storage in the fat body in dormancy-inducing conditions. The reduction of ecdysteroid biosynthesis causes a decrease in ETH expression level, leading to a decrease in JH biosynthesis, which is essential for entering reproductive dormancy. The study on C. bowringi beetle nicely demonstrated that the ecdysteroid-ETH-JH relay is important to regulate reproductive dormancy. This study is an excellent example of how findings on the regulation of JH biosynthesis from analyses with D. melanogaster led to an understanding of the mechanisms of reproductive dormancy in other insects. Conversely, it would be intriguing to examine whether the ecdysteroid-ETH-JH-mediated mechanism found in C. bowringi also exists in D. melanogaster.
Is There a Direct Neuronal Regulation of Juvenile Hormone Biosynthesis in the Corpus Allatum in Drosophila melanogaster?
In some insects other than D. melanogaster, JH-mediated reproductive dormancy is regulated by not only humoral factors remotely acting on the CA but also by direct inputs from neurons projecting to the CA (de Wilde and de Boer, 1969; Shiga and Numata, 2000; Shimokawa et al., 2008). For example, in the northern blowfly P. terraenovae and the bean bug R. pedestris, the neurons projecting to the CA (hereafter referred to as the CA-projecting neurons) are essential for inducing reproductive dormancy in dormancy-inducing conditions (Shiga et al., 2003; Shimokawa et al., 2008). In adults of the brown-winged green bug Plautia stali, the CA-projecting neurons co-produce several neuropeptides, such as Diuretic hormone 44, Insulin-like peptide 1, and Plautia stali myoinhibitory protein (Plast-MIP). In particular, Plast-MIP is considered to suppress JH biosynthesis in the CA directly (Matsumoto et al., 2017; Hasegawa et al., 2020; Hasebe and Shiga, 2021). In these insects, reproductive dormancy can be broken by surgically removing the CA-projecting neurons, confirming the importance of the CA-projecting neurons in the regulation of reproductive dormancy. However, in almost all cases, except P. stali, the neurotransmitter and/or neurohormone produced in the CA-projecting neurons has not been clarified. For a better understanding of the neuroendocrine mechanisms of JH biosynthesis in the CA, clarification and characterization of such neurotransmitters and/or neurohormones are crucial.
In D. melanogaster larvae, two types of neurons, designated CA-LP1 and CA-LP2 neurons, have been reported to directly innervate the CA (Siegmund and Korge, 2001). These neurons are involved in the epithelial movement of male genitalia by inhibiting JH biosynthesis during pupal development in non-dormancy-inducing condition (Ádám et al., 2003). It is possible that these neurons are also present in the adult stage and positively regulate reproductive dormancy via inhibiting JH biosynthesis. In addition, our recent study has reported new CA-projection neurons that produce the neuropeptide hugin in D. melanogaster adults, which is unexpected given that the CA-projecting hugin neurons may not have a significant impact on JH biosynthesis in the CA (Mizuno et al., 2021). Further studies would be needed to clarify the role of the CA-projecting neurons in reproductive dormancy in D. melanogaster (Figure 3B).
Mechanisms of Dormancy Control by Circadian Clocks
In general, dormancy is significantly influenced by photoperiodism (Denlinger, 2002; Saunders, 2014). In D. melanogaster, although a low temperature is the main environmental cue to induce reproductive dormancy, photoperiodism additionally influences the dormancy entry; the short-day but not the long-day condition accelerates the entry of reproductive dormancy in D. melanogaster (Saunders et al., 1989; Saunders and Gilbert, 1990; Nagy et al., 2018). In a wide range of insects, photoperiodism and photoperiodism-associated dormancy are under the control of the circadian clock system (Bloch et al., 2013; Meuti and Denlinger, 2013; Meuti et al., 2015; Saunders, 2020). Indeed, photoperiodism significantly influences JH titers in D. melanogaster (Saunders, 1990) and other insects (Kramer, 1978; Khan et al., 1982; Meuti and Denlinger, 2013; Matsumoto et al., 2017), although molecular and cellular mechanisms of this phenomenon remain largely unclear. On the other hand, recent studies have shown that circadian clock neurons and circadian clock genes play an important role in modulating the neuroendocrine system to control reproductive dormancy in D. melanogaster. Below, we mainly summarize the role of clock neurons and circadian clock genes in reproductive dormancy, and briefly mention a possible contribution of the clock machinery to JH biosynthesis in the CA.
The molecular machinery of the circadian clock has been extensively studied in D. melanogaster, where the circadian master clock comprises about 150 neurons located in the central brain (Helfrich-Förster, 2004). Among the identified circadian clock neurons, the small ventral lateral neurons (s-LNvs) act as central circadian pacemakers to regulate various daily changes in D. melanogaster physiology. Indeed, s-LNvs are involved in the dormancy-dependent regulation of neuronal activity of IPCs (Nagy et al., 2019). The axons of D. melanogaster s-LNvs project to the dorsal protocerebrum, where the cell bodies and dendrites of IPCs are located, implying that s-LNvs and IPCs might have direct synaptic contact with each other (Figure 3A).
The s-LNvs are known to produce and release two neuropeptides required for the normal functioning of the behavioral circadian rhythm, namely pigment-dispersing factor (Pdf) (Park et al., 2000) and short neuropeptide F (sNPF) (Johard et al., 2009). Overexpression of either Pdf or sNPF, specifically in s-LNvs, decreases the percentage of reproductively dormant flies in dormancy-inducing condition. Furthermore, inhibition of the sNPF receptor only in IPCs also increases the percentage of dormant flies in dormancy-inducing condition. Consistent with these results, administration of synthetic Pdf or sNPF ligand to cultured brains evokes an increase in intracellular cAMP, which acts as a second messenger for the Pdf receptor and sNPF receptor in IPCs (Nagy et al., 2019). According to these data, it is hypothesized that the change in day length during winter suppresses the production and secretion of Pdf and sNPF in s-LNvs, which might provide less stimulation for IPCs and consequently induce reproductive dormancy (Figure 3B). Therefore, the suppression of IPC neuronal activity might be due to reduced activation of the receptors for Pdf and sNPF (Ojima et al., 2018). In addition, it is very likely that Dilp-mediated regulation of JH biosynthesis in the CA would be under the control of Pdf- and sNPF-dependent regulation of the circadian rhythm; however, this hypothesis has not been closely examined.
It has been reported that Pdf-dependent regulation of reproductive dormancy has been observed in the blow fly Protophormia terraenovae (Shiga and Numata, 2009), the mosquito Culex pipiens (Meuti et al., 2015), and the bean bug Riptortus pedestris (Ikeno et al., 2014). These data imply that Pdf-producing clock neurons are involved in reproductive dormancy beyond Drosophilidae species.
Besides the regulation mediated by the central clock pacemaker neurons, the activity of IPCs is also regulated by transcription regulators expressed in IPCs. This mechanism has been revealed in a study on a gene called eyes absent (eya) (Abrieux et al., 2020). Eya encodes a highly conserved transcriptional coactivator and protein phosphatase that plays vital roles in multiple developmental processes in organisms ranging from Drosophila to humans. While the best-known function of Eya is to control eye formation, it is also known that eya is expressed in IPCs. Knockdown of eya in IPCs causes an increase in the size of the ovary, implying that Eya negatively regulates oogenesis through IPCs. Interestingly, the expression of eya has a circadian rhythm (Abrieux et al., 2020). More precisely, the peak of Eya protein levels is delayed in the short-day condition in comparison to the long-day condition. In addition, the protein levels of Eya in IPCs are higher under the short-day condition than the long-day condition. In parallel, Eya protein level is also upregulated under low-temperature conditions. These observations raise the possibility that the upregulation of Eya protein in dormancy-inducing conditions is necessary for oogenesis suppression (Figure 3B).
In the same study (Abrieux et al., 2020), the authors have demonstrated that the timeless (tim) gene also functions in IPCs to regulate reproductive dormancy. The tim gene is notable for its role in the transcriptional-translational autoregulatory feedback loops of the circadian core clock system in D. melanogaster (Dunlap, 1999; Hardin, 2005). In the tim mutant, a higher rate of reproductive dormancy is observed in any daylight length condition compared to wild type, and the impact of the short-day condition is less effective to enhance reproductive dormancy (Tauber et al., 2007; Abrieux et al., 2020). Interestingly, Tim is involved in the regulation of the Eya protein level. For example, the circadian rhythm of Eya protein is abolished in the tim mutant under non-dormancy-inducing conditions (Abrieux et al., 2020). Furthermore, the increased level of Eya protein at low temperatures is thought to be mediated by Tim. The Tim-mediated upregulation of Eya seems to be regulated by a unique splice isoform generated only at low temperature (Anduaga et al., 2019; Foley et al., 2019), which physically interacts with EYA protein (Abrieux et al., 2020). Based on these findings, it is hypothesized that the low temperature-specific Tim splice isoform binds Eya to suppress IPC activity, while the nature of the cell types in which this physical interaction takes place has not been precisely elucidated. Taken together, IPCs activity seems to be regulated by the external and internal regulatory system of the circadian clock in D. melanogaster, both of which might be required for regulating reproductive dormancy by inhibiting JH biosynthesis in the CA (Figure 3B).
Lastly, we would like to mention that a part of the circadian clock-mediated mechanisms of photoperiodism in D. melanogaster might be conserved even in mammals. For example, EYA3, one of the mammalian orthologs of eya, is required for the photoperiodism-dependent expression change of the thyroid-stimulating hormone gene in the mouse and the sheep (Dardente et al., 2010; Masumoto et al., 2010; Hut, 2011; Wood and Loudon, 2014; Wood et al., 2015).
Future Perspectives
Although the studies on D. melanogaster in the last decades have improved our understanding of JH-mediated regulation of reproductive dormancy, several issues remain to be clarified. Here, we list the five main possible research lines, in our opinion.
(1) Do hemolymph JH titers change during reproductive dormancy induction in D. melanogaster and how do they change? In fact, no previous study using D. melanogaster has made a conclusive report about the temporal fluctuation of hemolymph JH titers. This information must be a solid foothold for D. melanogaster reproductive dormancy studies and, therefore, should be obtained by the D. melanogaster research community in the near future. Liquid chromatography-mass spectrometry-based methods to measure hemolymph JH titers have already been reported by several groups (Reiff et al., 2015; Lee et al., 2017; Meiselman et al., 2017; Ramirez et al., 2020; Zhang et al., 2021).
(2) How does the information regarding low temperature eventually get transmitted to the CA? It has been revealed that the antenna is a vital organ for detecting cold sensations (Gallio et al., 2011; Li and Gong, 2017; Alpert et al., 2020). Therefore, there must be a neuronal circuit from the antennal cold-sensitive sensilla to the CA; however, the nature of this circuit is largely unclear. At least, it is likely that the neuronal circuit may contain some dopaminergic neurons, based on the observations that dopamine suppresses JH biosynthesis and is elevated in dormancy-inducing conditions (Andreatta et al., 2018). On the other hand, it should be noted that low temperature suppresses overall biological activity, including feeding. Since a lower level of feeding results in the suppression of energy expenditure, the dormancy-inducing low temperature might negatively affect IPC activity, possibly leading to reproductive dormancy. Meanwhile, curiously and paradoxically, some studies have reported that IPCs are activated by cold-sensing neurons at 18°C rather than 25°C (Li and Gong, 2015; Zhang et al., 2019), whereas the function of cold-sensing neurons in dormancy-inducing conditions has not yet been investigated. Further studies will be needed to clarify how low temperatures influence IPC activity to induce reproductive dormancy.
(3) How does the reproductive dormancy status influence the physiology and remodeling in each tissue? It has been reported that reproductive dormancy suppresses oogenesis and is also associated with several changes in various organs of the body. For example, the intestine is markedly shortened, and the innate immune system is activated during reproductive dormancy in D. melanogaster (Kubrak et al., 2014); however, the mechanisms involved are still unknown.
(4) How is male reproductive dormancy regulated? It has been reported that low temperatures and short-day condition also induce male reproductive dormancy, such as the slow-down of spermatogenesis and degeneration of male accessory glands (Kubrak et al., 2016). However, the molecular and neuroendocrine mechanisms of male reproductive dormancy have not been investigated. It has not even been elucidated whether this process depends on JHs. In the future, it would be intriguing to examine whether and how the neuroendocrine regulatory system of female reproductive dormancy resembles that of male reproductive dormancy.
(5) How does the JH-mediated regulation of reproductive dormancy contribute to the evolutionary and ecological adaptation of wild D. melanogaster? To date, most studies on D. melanogaster reproductive dormancy have been conducted in laboratories. However, since reproductive dormancy is the adaptive response of organisms to the severe winter season, it must be important to investigate the reproductive dormancy of wild animals. As described earlier, geological differences of genetic bias influence reproductive dormancy in D. melanogaster. Indeed, previous studies have shown that geographic variations in reproductive dormancy in wild D. melanogaster is associated with single nucleotide polymorphisms in the loci of tim, InR, FoxO, and couch potato (Williams et al., 2006; Sandrelli et al., 2007; Tauber et al., 2007; Schmidt et al., 2008; Paaby et al., 2010, 2014; Kolaczkowski et al., 2011; Lee et al., 2011; Fabian et al., 2012; Bergland et al., 2014; Kapun et al., 2016; Zhao et al., 2016; Betancourt et al., 2021; Machado et al., 2021). In the case of tim, it is hypothesized that the allelic frequencies of two distinct isoforms, ls-tim and s-tim (Rosato et al., 1997), may have evolved to cope with winter when D. melanogaster expanded into Europe (Tauber et al., 2007). This hypothesis is based on the observation that flies expressing ls-tim have a higher dormancy rate than those with s-tim (Sandrelli et al., 2007; Tauber et al., 2007). It would be interesting to investigate whether such genetic variations and allelic frequencies among these geographically distinct D. melanogaster populations affect neuroendocrine mechanisms for reproductive dormancy.
(6) How does the JH-mediated regulation of reproductive dormancy contribute to the evolutionary and ecological adaptation of other Drosophilidae species? Other Drosophilidae species also enter adult dormancy in the winter, and many biological events during dormancy are essentially the same as we found in D. melanogaster, although the ease of dormancy varies among species (Carson and Stalker, 1948; Lumme et al., 1974; Schmidt and Conde, 2006; Yamada and Yamamoto, 2011; Salminen and Hoikkala, 2013; Zhai et al., 2016; Lirakis et al., 2018). Therefore, wild non-melanogaster Drosophilidae species are interesting research subjects for understanding the evolutionary significance of genetic and ecological variations in reproductive dormancy, which may influence flies’ fitness in nature.
Well-developed and sophisticated genetic tools for D. melanogaster will shed light on the fundamental mechanisms of JH-mediated reproductive dormancy, not only in D. melanogaster, but also in other insect species. As mentioned in the Introduction, studies on reproductive dormancy would increase our understanding of the seasonal distribution of pests (Denlinger, 2008). Notably, in recent years, several researchers have used the spotted wing Drosophilidae fly D. suzukii for studying reproductive dormancy (Toxopeus et al., 2016; Wallingford et al., 2016; Zhai et al., 2016, 2019). Since D. suzukii is a well-known agricultural pest, the motivation for these studies is to explore new pest control strategies to disrupt the mechanisms of reproductive dormancy. Therefore, future studies on reproductive dormancy in Drosophilidae species, including D. melanogaster, have the potential to contribute to the fields of basic animal physiology and applied agricultural biology in the future.
Author Contributions
YK and RN wrote the first draft of the manuscript. All authors contributed to manuscript revision, read, and approved the submitted version.
Funding
This work was supported by grant from the JSPS KAKENHI 21J20365 to YK.
Conflict of Interest
The authors declare that the research was conducted in the absence of any commercial or financial relationships that could be construed as a potential conflict of interest.
Publisher’s Note
All claims expressed in this article are solely those of the authors and do not necessarily represent those of their affiliated organizations, or those of the publisher, the editors and the reviewers. Any product that may be evaluated in this article, or claim that may be made by its manufacturer, is not guaranteed or endorsed by the publisher.
Acknowledgments
YK and EI were recipients of a fellowship from the Japan Society for the Promotion of Science. We would like to thank Louis John Irving and Editage (www.editage.com) for English language editing.
References
Abrieux, A., Xue, Y., Cai, Y., Lewald, K. M., Nguyen, H. N., Zhang, Y., et al. (2020). EYES ABSENT and TIMELESS integrate photoperiodic and temperature cues to regulate seasonal physiology in Drosophila. Proc. Natl. Acad. Sci. U.S.A. 117, 15293–15304. doi: 10.1073/pnas.2004262117
Ádám, G., Perrimon, N., and Noselli, S. (2003). The retinoic-like juvenile hormone controls the looping of left-right asymmetric organs in Drosophila. Development 130, 2397–2406. doi: 10.1242/dev.00460
Alpert, M. H., Frank, D. D., Kaspi, E., Flourakis, M., Zaharieva, E. E., Allada, R., et al. (2020). A circuit encoding absolute cold temperature in Drosophila. Curr. Biol. 30, 2275–2288.e5. doi: 10.1016/j.cub.2020.04.038
Ameku, T., and Niwa, R. (2016). Mating-induced increase in germline stem cells via the neuroendocrine system in female Drosophila. PLoS Genet. 12:e1006123. doi: 10.1371/journal.pgen.1006123
Ameku, T., Yoshinari, Y., Fukuda, R., and Niwa, R. (2017). Ovarian ecdysteroid biosynthesis and female germline stem cells. Fly 11, 185–193. doi: 10.1080/19336934.2017.1291472
Andreatta, G., Kyriacou, C. P., Flatt, T., and Costa, R. (2018). Aminergic signaling controls ovarian dormancy in Drosophila. Sci. Rep. 8:2030. doi: 10.1038/s41598-018-20407-z
Anduaga, A. M., Evanta, N., Patop, I. L., Bartok, O., Weiss, R., and Kadener, S. (2019). Thermosensitive alternative splicing senses and mediates 2 temperature adaptation in Drosophila. eLife 8:e44642. doi: 10.7554/eLife.44642
Anduaga, A. M., Nagy, D., Costa, R., and Kyriacou, C. P. (2018). Diapause in Drosophila melanogaster – Photoperiodicity, cold tolerance and metabolites. J. Insect Physiol. 105, 46–53. doi: 10.1016/j.jinsphys.2018.01.003
Bastock, R., and St Johnston, D. (2008). Drosophila oogenesis. Curr. Biol. 18, R1082–R1087. doi: 10.1016/j.cub.2008.09.011
Belles, X., and Piulachs, M. D. (2015). Ecdysone signalling and ovarian development in insects: from stem cells to ovarian follicle formation. Biochim. Biophys. Acta Gene Regul. Mech. 1849, 181–186. doi: 10.1016/j.bbagrm.2014.05.025
Bergland, A. O., Behrman, E. L., O’Brien, K. R., Schmidt, P. S., and Petrov, D. A. (2014). Genomic evidence of rapid and stable adaptive oscillations over seasonal time scales in Drosophila. PLoS Genet. 10:e1004775. doi: 10.1371/JOURNAL.PGEN.1004775
Betancourt, N. J., Rajpurohit, S., Durmaz, E., Fabian, D. K., Kapun, M., Flatt, T., et al. (2021). Allelic polymorphism at foxo contributes to local adaptation in Drosophila melanogaster. Mol. Ecol. 30, 2817–2830. doi: 10.1111/MEC.15939
Bloch, G., Hazan, E., and Rafaeli, A. (2013). Circadian rhythms and endocrine functions in adult insects. J. Insect Physiol. 59, 56–69. doi: 10.1016/j.jinsphys.2012.10.012
Bownes, M. (1982). The role of 20-hydroxy-ecdysone in yolk-polypeptide synthesis by male and female fat bodies of Drosophila melanogaster. J. Insect Physiol. 28, 317–328. doi: 10.1016/0022-1910(82)90043-9
Bownes, M. (1989). The roles of juvenile hormone, ecdysone and the ovary in the control of Drosophila vitellogenesis. J. Insect Physiol. 35, 409–413. doi: 10.1016/0022-1910(89)90115-7
Bownes, M. (1994). The regulation of the yolk protein genes, a family of sex differentiation genes in Drosophila melanogaster. Bioessays 16, 745–752. doi: 10.1002/bies.950161009
Bownes, M., Ronaldson, E., and Mauchline, D. (1996). 20-Hydroxyecdysone, but not juvenile hormone, regulation of yolk protein gene expression can be mapped to cis-acting DNA sequences. Dev. Biol. 173, 475–489. doi: 10.1006/dbio.1996.0041
Brand, A. H., and Perrimon, N. (1993). Targeted gene expression as a means of altering cell fates and generating dominant phenotypes. Development 118, 401–415.
Brennan, M. D., Weiner, A. J., Goralski, T. J., and Mahowald, A. P. (1982). The follicle cells are a major site of vitellogenin synthesis in Drosophila melanogaster. Dev. Biol. 89, 225–236. doi: 10.1016/0012-1606(82)90309-8
Carney, G. E., and Bender, M. (2000). The Drosophila ecdysone receptor (EcR) gene is required maternally for normal oogenesis. Genetics 154, 1203–1211. doi: 10.1093/genetics/154.3.1203
Carson, H. L., and Stalker, H. D. (1948). Reproductive diapause in Drosophila robusta. Proc. Natl. Acad. Sci. U.S.A. 34, 124–129. doi: 10.1073/pnas.34.3.124
Cornils, A., Gloeck, M., Chen, Z., Zhang, Y., and Alcedo, J. (2011). Specific insulin-like peptides encode sensory information to regulate distinct developmental processes. Development 138, 1183–1193. doi: 10.1242/DEV.060905
Crowe, J. H. (2007). Trehalose As a “Chemical Chaperone”. Adv. Exp. Med. Biol. 594, 143–158. doi: 10.1007/978-0-387-39975-1_13
Danks, H. (1987). Insect Dormancy: An Ecological Perspective. Ottawa: Entomological Society of Canada.
Danks, H. V. (2006). Key themes in the study of seasonal adaptations in insects II. Life-cycle patterns. Appl. Entomol. Zool. 41, 1–13. doi: 10.1303/aez.2006.1
Dardente, H., Wyse, C. A., Birnie, M. J., Dupré, S. M., Loudon, A. S. I., Lincoln, G. A., et al. (2010). A molecular switch for photoperiod responsiveness in mammals. Curr. Biol. 20, 2193–2198. doi: 10.1016/j.cub.2010.10.048
de Wilde, J., and de Boer, J. A. (1961). Physiology of diapause in the adult Colorado beetle-II. Diapause as a case of pseudo-allatectomy. J. Insect Physiol. 6, 152–161. doi: 10.1016/0022-1910(61)90037-3
de Wilde, J., and de Boer, J. A. (1969). Humoral and nervous pathways in photoperiodic induction of diapause in Leptinotarsa decemlineata. J. Insect Physiol. 15, 661–675. doi: 10.1016/0022-1910(69)90263-7
Denlinger, D. L. (2002). Regulation of diapause. Annu. Rev. Entomol. 47, 93–122. doi: 10.1146/annurev.ento.47.091201.145137
Denlinger, D. L. (2008). Why study diapause? Entomol. Res. 38, 1–9. doi: 10.1111/j.1748-5967.2008.00139.x
Denlinger, D. L., Yocum, G. D., and Rinehart, J. P. (2012). Hormonal Control of Diapause. Insect Endocrinol. 3, 430–463. doi: 10.1016/B978-0-12-384749-2.10010-X
Dunlap, J. C. (1999). Molecular bases for circadian clocks. Cell 96, 271–290. doi: 10.1016/S0092-8674(00)80566-8
Emerson, K. J., Bradshaw, W. E., and Holzapfel, C. M. (2009a). Complications of complexity: integrating environmental, genetic and hormonal control of insect diapause. Trends Genet. 25, 217–225. doi: 10.1016/j.tig.2009.03.009
Emerson, K. J., Uyemura, A. M., McDaniel, K. L., Schmidt, P. S., Bradshaw, W. E., and Holzapfel, C. M. (2009b). Environmental control of ovarian dormancy in natural populations of Drosophila melanogaster. J. Comp. Physiol. A Neuroethol. Sensory Neural Behav. Physiol. 195, 825–829. doi: 10.1007/s00359-009-0460-5
Enell, L. E., Kapan, N., Söderberg, J. A. E., Kahsai, L., and Nässe, D. R. (2010). Insulin signaling, lifespan and stress resistance are modulated by metabotropic GABA receptors on insulin producing cells in the brain of Drosophila. PLoS One 5:15780. doi: 10.1371/journal.pone.0015780
Erickson, P. A., Weller, C. A., Song, D. Y., Bangerter, A. S., Schmidt, P., and Bergland, A. O. (2020). Unique genetic signatures of local adaptation over space and time for diapause, an ecologically relevant complex trait, in Drosophila melanogaster. PLoS Genet. 16:e1009110. doi: 10.1371/JOURNAL.PGEN.1009110
Fabian, D. K., Kapun, M., Nolte, V., Kofler, R., Schmidt, P. S., Schlötterer, C., et al. (2012). Genome-wide patterns of latitudinal differentiation among populations of Drosophila melanogaster from North America. Mol. Ecol. 21, 4748–4769. doi: 10.1111/J.1365-294X.2012.05731.X
Fabian, D. K., Lack, J. B., Mathur, V., Schlötterer, C., Schmidt, P. S., Pool, J. E., et al. (2015). Spatially varying selection shapes life history clines among populations of Drosophila melanogaster from sub-Saharan Africa. J. Evol. Biol. 28, 826–840. doi: 10.1111/jeb.12607
Flatt, T. (2020). Life-history evolution and the genetics of fitness components in Drosophila melanogaster. Genetics 214, 3–48. doi: 10.1534/genetics.119.300160
Foley, L. E., Ling, J., Joshi, R., Evantal, N., Kadener, S., and Emery, P. (2019). Drosophila PSI controls circadian period and the phase of circadian behavior under temperature cycle via tim splicing. eLife 8:e50063. doi: 10.7554/eLife.50063
Food and Agriculture Organization of the United Nations (2013). New Coordinated Research Projects, Insect Pest Control - NAFA. Available online at: http://www-naweb.iaea.org/nafa/ipc/crp/new-crps-ipc-1.html (accessed May 1, 2021).
Francis, S. H., and Corbin, J. D. (1994). Structure and function of cyclic nucleotide-dependent protein kinases. Annu. Rev. Physiol. 56, 237–272. doi: 10.1146/annurev.ph.56.030194.001321
Gallio, M., Ofstad, T. A., Macpherson, L. J., Wang, J. W., and Zuker, C. S. (2011). The coding of temperature in the Drosophila brain. Cell 144, 614–624. doi: 10.1016/j.cell.2011.01.028
Golden, J., and Riddle, D. (1982). A pheromone influences larval development in the nematode Caenorhabditis elegans. Science 218, 578–580. doi: 10.1126/SCIENCE.6896933
Golden, J. W., and Riddle, D. L. (1984). The Caenorhabditis elegans dauer larva: developmental effects of pheromone, food, and temperature. Dev. Biol. 102, 368–378. doi: 10.1016/0012-1606(84)90201-X
Gotzes, F., Balfanz, S., and Baumann, A. (1994). Primary structure and functional characterization of a Drosophila dopamine receptor with high homology to human D(1/5) receptors. Recept. Channels 2, 131–141.
Gruntenko, N., Wilson, T., Monastirioti, M., and Rauschenbach, I. (2000). Stress-reactivity and juvenile hormone degradation in Drosophila melanogaster strains having stress-related mutations. Insect Biochem. Mol. Biol. 30, 775–783. doi: 10.1016/S0965-1748(00)00049-7
Gruntenko, N. Y., Laukhina, O. V., and Rauschenbach, I. Y. (2012). Role of D1- and D2-like receptors in age-specific regulation of juvenile hormone and 20-hydroxyecdysone levels by dopamine in Drosophila. J. Insect Physiol. 58, 1534–1540. doi: 10.1016/j.jinsphys.2012.09.005
Guo, S., Tian, Z., Wu, Q. W., King-Jones, K., Liu, W., Zhu, F., et al. (2021). Steroid hormone ecdysone deficiency stimulates preparation for photoperiodic reproductive diapause. PLoS Genet. 17:e1009352. doi: 10.1371/JOURNAL.PGEN.1009352
Hahn, D. A., and Denlinger, D. L. (2007). Meeting the energetic demands of insect diapause: nutrient storage and utilization. J. Insect Physiol. 53, 760–773. doi: 10.1016/J.JINSPHYS.2007.03.018
Hahn, D. A., and Denlinger, D. L. (2011). Energetics of insect diapause. Annu. Rev. Entomol. 56, 103–121. doi: 10.1146/annurev-ento-112408-085436
Hand, S. C., Denlinger, D. L., Podrabsky, J. E., and Roy, R. (2016). Mechanisms of animal diapause: recent developments from nematodes, crustaceans, insects, and fish. Am. J. Physiol.Regul. Integr. Comp. Physiol. 310, R1193–R1211. doi: 10.1152/ajpregu.00250.2015
Handler, A. M., and Maroy, P. (1989). Ecdysteroid receptors in Drosophila melanogaster adult females. Mol. Cell. Endocrinol. 63, 103–109. doi: 10.1016/0303-7207(89)90086-5
Hardin, P. E. (2005). The circadian timekeeping system of Drosophila. Curr. Biol. 15, R714–R722. doi: 10.1016/j.cub.2005.08.019
Hasebe, M., and Shiga, S. (2021). Photoperiodic response in the pars intercerebralis neurons, including Plast-MIP neurons, in the brown-winged green bug, Plautia stali. Zoolog. Sci. 38, 317–325. doi: 10.2108/zs210005
Hasegawa, T., Hasebe, M., and Shiga, S. (2020). Immunohistochemical and direct mass spectral analyses of Plautia stali myoinhibitory peptides in the cephalic ganglia of the brown-winged green bug Plautia stali. Zoolog. Sci. 37, 42–49. doi: 10.2108/zs190092
He, L., Wang, X., and Montell, D. J. (2011). Shining light on Drosophila oogenesis: live imaging of egg development. Curr. Opin. Genet. Dev. 21, 612–619. doi: 10.1016/J.GDE.2011.08.011
Helfrich-Förster, C. (2004). The circadian clock in the brain: a structural and functional comparison between mammals and insects. J. Comp. Physiol. A Neuroethol. Sens. Neural Behav. Physiol. 190, 601–613. doi: 10.1007/s00359-004-0527-2
Hsu, S. K., Jakšiæ, A. M., Nolte, V., Lirakis, M., Kofler, R., Barghi, N., et al. (2020). Rapid sex-specific adaptation to high temperature in Drosophila. eLife 9:e53237. doi: 10.7554/ELIFE.53237
Hu, P. (2007). “Dauer,” in WormBook, ed. W. The C. elegans Research Community. Available online at: http://www.wormbook.org (accessed August 08, 2007).
Hut, R. A. (2011). Photoperiodism: Shall EYA compare thee to a summers day? Curr. Biol. 21, R22–R25. doi: 10.1016/j.cub.2010.11.060
Ikeno, T., Numata, H., Goto, S. G., and Shiga, S. (2014). Involvement of the brain region containing pigment-dispersing factor-immunoreactive neurons in the photoperiodic response of the bean bug, Riptortus pedestris. J. Exp. Biol. 217, 453–462. doi: 10.1242/jeb.091801
Isabel, G., Gourdoux, L., and Moreau, R. (2001). Changes of biogenic amine levels in haemolymph during diapausing and non-diapausing status in Pieris brassicae L. Comp. Biochem. Physiol. A Mol. Integr. Physiol. 128, 117–127. doi: 10.1016/S1095-6433(00)00284-1
Jindra, M., Palli, S. R., and Riddiford, L. M. (2013). The juvenile hormone signaling pathway in insect development. Annu. Rev. Entomol. 58, 181–204. doi: 10.1146/annurev-ento-120811-153700
Johard, H. A. D., Yoishii, T., Dircksen, H., Cusumano, P., Rouyer, F., Helfrich-Förster, C., et al. (2009). Peptidergic clock neurons in Drosophila: ion transport peptide and short neuropeptide F in subsets of dorsal and ventral lateral neurons. J. Comp. Neurol. 516, 59–73. doi: 10.1002/cne.22099
Jowett, T., and Postlethwait, J. H. (1980). The regulation of yolk polypeptide synthesis in Drosophila ovaries and fat body by 20-hydroxyecdysone and a juvenile hormone analog. Dev. Biol. 80, 225–234. doi: 10.1016/0012-1606(80)90510-2
Kapun, M., Fabian, D. K., Goudet, J., and Flatt, T. (2016). Genomic evidence for adaptive inversion clines in Drosophila melanogaster. Mol. Biol. Evol. 33, 1317–1336. doi: 10.1093/MOLBEV/MSW016
Karam, C. S., Jones, S. K., and Javitch, J. A. (2020). Come Fly with Me: an overview of dopamine receptors in Drosophila melanogaster. Basic Clin. Pharmacol. Toxicol. 126, 56–65. doi: 10.1111/bcpt.13277
Kasture, A. S., Hummel, T., Sucic, S., and Freissmuth, M. (2018). Big lessons from tiny flies: Drosophila melanogaster as a model to explore dysfunction of dopaminergic and serotonergic neurotransmitter systems. Int. J. Mol. Sci. 19:1788. doi: 10.3390/ijms19061788
Khan, M. A., Doderer, A., Koopmanschap, A. B., and de Kort, C. A. D. (1982). Improved assay conditions for measurement of corpus allatum activity in vitro in the adult Colorado potato beetle, Leptinotarsa decemlineata. J. Insect Physiol. 28, 279–284. doi: 10.1016/0022-1910(82)90088-9
King, R. C. (1970). The meiotic behavior of the Drosophila oocyte. Int. Rev. Cytol. 28, 125–168. doi: 10.1016/S0074-7696(08)62542-5
Kingan, T. G., Gray, W., Zitnan, D., and Adams, M. E. (1997). Regulation of ecdysis-triggering hormone release by eclosion hormone. J. Exp. Biol. 200, 3245–3256. doi: 10.1242/jeb.200.24.3245
Kolaczkowski, B., Kern, A. D., Holloway, A. K., and Begun, D. J. (2011). Genomic differentiation between temperate and tropical Australian populations of Drosophila melanogaster. Genetics 187, 245–260. doi: 10.1534/GENETICS.110.123059
Kort, C. A. D. (1990). Thirty-five years of diapause research with the Colorado potato beetle. Entomol. Exp. Appl. 56, 1–13. doi: 10.1111/j.1570-7458.1990.tb01376.x
Koštál, V. (2006). Eco-physiological phases of insect diapause. J. Insect Physiol. 52, 113–127. doi: 10.1016/j.jinsphys.2005.09.008
Kramer, S. J. (1978). Age-dependent changes in corpus allatum activity in vitro in the adult Colorado potato beetle, Leptinotarsa decemlineata. J. Insect Physiol. 24, 461–464. doi: 10.1016/0022-1910(78)90046-X
Kubrak, O. I., Kuèerová, L., Theopold, U., and Nässel, D. R. (2014). The sleeping beauty: how reproductive diapause affects hormone signaling, metabolism, immune response and somatic maintenance in Drosophila melanogaster. PLoS One 9:e113051. doi: 10.1371/journal.pone.0113051
Kubrak, O. I., Kucerová, L., Theopold, U., Nylin, S., and Nässel, D. R. (2016). Characterization of reproductive dormancy in male Drosophila melanogaster. Front. Physiol. 7:572. doi: 10.3389/fphys.2016.00572
Kuèerová, L., Kubrak, O. I., Bengtsson, J. M., Strnad, H., Nylin, S., Theopold, U., et al. (2016). Slowed aging during reproductive dormancy is reflected in genome-wide transcriptome changes in Drosophila melanogaster. BMC Genomics 17:50. doi: 10.1186/s12864-016-2383-1
LaFever, L., and Drummond-Barbosa, D. (2005). Direct control of germline stem cell division and cyst growth by neural insulin in Drosophila. Science 309, 1071–1073. doi: 10.1126/SCIENCE.1111410
Lee, S. F., Sgro, C. M., Shirriffs, J., Wee, C. W., Rako, L., van Heerwaarden, B., et al. (2011). Polymorphism in the couch potato gene clines in eastern Australia but is not associated with ovarian dormancy in Drosophila melanogaster. Mol. Ecol. 20, 2973–2984. doi: 10.1111/J.1365-294X.2011.05155.X
Lee, S. S., Ding, Y., Karapetians, N., Rivera-Perez, C., Noriega, F. G., and Adams, M. E. (2017). Hormonal signaling cascade during an early-adult critical period required for courtship memory retention in Drosophila. Curr. Biol. 27, 2798–2809.e3. doi: 10.1016/j.cub.2017.08.017
Lenaerts, C., Monjon, E., Van Lommel, J., Verbakel, L., and Vanden Broeck, J. (2019). Peptides in insect oogenesis. Curr. Opin. Insect Sci. 31, 58–64. doi: 10.1016/J.COIS.2018.08.007
Li, K., and Gong, Z. (2017). Feeling hot and cold: thermal sensation in Drosophila. Neurosci. Bull. 33, 317–322. doi: 10.1007/s12264-016-0087-9
Li, Q., and Gong, Z. (2015). Cold-sensing regulates Drosophila growth through insulin-producing cells. Nat. Commun. 6:10083. doi: 10.1038/ncomms10083
Lirakis, M., Dolezal, M., and Schlötterer, C. (2018). Redefining reproductive dormancy in Drosophila as a general stress response to cold temperatures. J. Insect Physiol. 107, 175–185. doi: 10.1016/j.jinsphys.2018.04.006
Lirakis, M., and Magalhães, S. (2019). Does experimental evolution produce better biological control agents? A critical review of the evidence. Entomol. Exp. Appl. 167, 584–597. doi: 10.1111/EEA.12815
Liu, Y., Liao, S., Veenstra, J. A., and Nässel, D. R. (2016). Drosophila insulin-like peptide 1 (DILP1) is transiently expressed during non-feeding stages and reproductive dormancy. Sci. Rep. 6:26620. doi: 10.1038/srep26620
Lumme, J., Oikarinen, A., Lakovaara, S., and Alatalo, R. (1974). The environmental regulation of adult diapause in Drosophila littoralis. J. Insect Physiol. 20, 2023–2033. doi: 10.1016/0022-1910(74)90109-7
Luo, J., Becnel, J., Nichols, C. D., and Nässel, D. R. (2012). Insulin-producing cells in the brain of adult Drosophila are regulated by the serotonin 5-HT 1A receptor. Cell. Mol. Life Sci. 69, 471–484. doi: 10.1007/s00018-011-0789-0
Luo, J., Lushchak, O. V., Goergen, P., Williams, M. J., and Nässel, D. R. (2014). Drosophila insulin-producing cells are differentially modulated by serotonin and octopamine receptors and affect social behavior. PLoS One 9:e99732. doi: 10.1371/journal.pone.0099732
Machado, H. E., Bergland, A., Taylor, R. W., Tilk, S., Behrman, E., Dyer, K., et al. (2021). Broad geographic sampling reveals the shared basis and environmental correlates of seasonal adaptation in Drosophila. eLife 10:e67577. doi: 10.7554/ELIFE.67577
MacRae, T. H. (2010). Gene expression, metabolic regulation and stress tolerance during diapause. Cell. Mol. Life Sci. 67, 2405–2424. doi: 10.1007/S00018-010-0311-0
Masumoto, K. H., Ukai-Tadenuma, M., Kasukawa, T., Nagano, M., Uno, K. D., Tsujino, K., et al. (2010). Acute induction of Eya3 by late-night light stimulation triggers TSHβ expression in photoperiodism. Curr. Biol. 20, 2199–2206. doi: 10.1016/j.cub.2010.11.038
Matsumoto, K., Suetsugu, Y., Tanaka, Y., Kotaki, T., Goto, S. G., Shinoda, T., et al. (2017). Identification of allatostatins in the brown-winged green bug Plautia stali. J. Insect Physiol. 96, 21–28. doi: 10.1016/j.jinsphys.2016.10.005
Meiselman, M., Lee, S. S., Tran, R.-T., Dai, H., Ding, Y., Rivera-Perez, C., et al. (2017). Endocrine network essential for reproductive success in Drosophila melanogaster. Proc. Natl. Acad. Sci. U.S.A. 114, E3849–E3858. doi: 10.1073/pnas.1620760114
Meuti, M. E., and Denlinger, D. L. (2013). Evolutionary links between circadian clocks and photoperiodic diapause in insects. Integr. Comp. Biol. 53, 131–143. doi: 10.1093/icb/ict023
Meuti, M. E., Stone, M., Ikeno, T., and Denlinger, D. L. (2015). Functional circadian clock genes are essential for the overwintering diapause of the Northern house mosquito, Culex pipiens. J. Exp. Biol. 218, 412–422. doi: 10.1242/jeb.113233
Mizuno, Y., Imura, E., Kurogi, Y., Shimada-Niwa, Y., Kondo, S., Tanimoto, H., et al. (2021). A population of neurons that produce hugin and express the diuretic hormone 44 receptor gene projects to the corpora allata in Drosophila melanogaster. Dev. Growth Differ. 63, 249–261. doi: 10.1111/dgd.12733
Nagy, D., Andreatta, G., Bastianello, S., Anduaga, A. M., Mazzotta, G., Kyriacou, C. P., et al. (2018). A semi-natural approach for studying seasonal diapause in Drosophila melanogaster reveals robust photoperiodicity. J. Biol. Rhythms 33, 117–125. doi: 10.1177/0748730417754116
Nagy, D., Cusumano, P., Andreatta, G., Anduaga, A. M., Hermann-Luibl, C., Reinhard, N., et al. (2019). Peptidergic signaling from clock neurons regulates reproductive dormancy in Drosophila melanogaster. PLoS Genet. 15:e1008158. doi: 10.1371/journal.pgen.1008158
Nässel, D. R. (2018). Substrates for neuronal cotransmission with neuropeptides and small molecule neurotransmitters in Drosophila. Front. Cell. Neurosci. 12:83. doi: 10.3389/fncel.2018.00083
Neckameyer, W. S., and Argue, K. J. (2013). Comparative approaches to the study of physiology: Drosophila as a physiological tool. Am. J. Physiol. Regul. Integr. Comp. Physiol. 304, R177–R188. doi: 10.1152/ajpregu.00084.2012
Niwa, R., Niimi, T., Honda, N., Yoshiyama, M., Itoyama, K., Kataoka, H., et al. (2008). Juvenile hormone acid O-methyltransferase in Drosophila melanogaster. Insect Biochem. Mol. Biol. 38, 714–720. doi: 10.1016/j.ibmb.2008.04.003
Niwa, R., and Niwa, Y. (2014). Enzymes for ecdysteroid biosynthesis: their biological functions in insects and beyond. Biosci. Biotechnol. Biochem. 78, 1283–1292. doi: 10.1080/09168451.2014.942250
Noguchi, H., and Hayakawa, Y. (1997). Role of dopamine at the onset of pupal diapause in the cabbage armyworm Mamestra brassicae. FEBS Lett. 413, 157–161. doi: 10.1016/S0014-5793(97)00848-X
Noriega, F. G. (2014). Juvenile hormone biosynthesis in insects: What is new, what do we know, and what questions remain? Int. Sch. Res. Not. 2014:967361. doi: 10.1155/2014/967361
Ojima, N., Hara, Y., Ito, H., and Yamamoto, D. (2018). Genetic dissection of stress-induced reproductive arrest in Drosophila melanogaster females. PLoS Genet. 14:e1007434. doi: 10.1371/journal.pgen.1007434
Paaby, A. B., Bergland, A. O., Behrman, E. L., and Schmidt, P. S. (2014). A highly pleiotropic amino acid polymorphism in the Drosophila insulin receptor contributes to life-history adaptation. Evolution 68, 3395–3409. doi: 10.1111/EVO.12546
Paaby, A. B., Blacket, M. J., Hoffman, A. A., and Schmidt, P. S. (2010). Identification of a candidate adaptive polymorphism for Drosophila life history by parallel independent clines on two continents. Mol. Ecol. 19, 760–774. doi: 10.1111/J.1365-294X.2009.04508.X
Park, J. H., Helfrich-Förster, C., Lee, G., Liu, L., Rosbash, M., and Hall, J. C. (2000). Differential regulation of circadian pacemaker output by separate clock genes in Drosophila. Proc. Natl. Acad. Sci. U.S.A. 97, 3608–3613. doi: 10.1073/pnas.070036197
Park, Y., Zitnan, D., Gill, S. S., and Adams, M. E. (1999). Molecular cloning and biological activity of ecdysis-triggering hormones in Drosophila melanogaster. FEBS Lett. 463, 133–138. doi: 10.1016/S0014-5793(99)01622-1
Pener, M. P. (1992). Environmental cues, endocrine factors, and reproductive diapause in Male insects. Chronobiol. Int. 9, 102–113. doi: 10.3109/07420529209064521
Postlethwait, J. H., and Handler, A. M. (1979). The roles of juvenile hormone and 20-hydroxy-ecdysone during vitellogenesis in isolated abdomens of Drosophila melanogaster. J. Insect Physiol. 25, 455–460. doi: 10.1016/0022-1910(79)90014-3
Postlethwait, J. H., and Weiser, K. (1973). Vitellogenesis induced by juvenile hormone in the female sterile mutant apterous-four in Drosophila melanogaster. Nat. New Biol. 244, 284–285. doi: 10.1038/newbio244284a0
Ramirez, C. E., Nouzova, M., Michalkova, V., Fernandez-Lima, F., and Noriega, F. G. (2020). Common structural features facilitate the simultaneous identification and quantification of the five most common juvenile hormones by liquid chromatography-tandem mass spectrometry. Insect Biochem. Mol. Biol. 116, 103287. doi: 10.1016/J.IBMB.2019.103287
Rauschenbach, I. Y., Gruntenko, N. E., Chentsova, N. S., Hirashima, A., Sukhanova, M. Z., Andreenkova, E. V., et al. (2001). Regulation of reproductive function in Drosophila females due to hormonal interaction under stress is genetically determined. Russ. J. Genet. 37, 1041–1048. doi: 10.1023/A:1011961514711
Reiff, T., Jacobson, J., Cognigni, P., Antonello, Z., Ballesta, E., Tan, K. J., et al. (2015). Endocrine remodelling of the adult intestine sustains reproduction in Drosophila. eLife 4:e06930. doi: 10.7554/eLife.06930
Richard, D. S., Gilbert, M., Crum, B., Hollinshead, D. M., Schelble, S., and Scheswohl, D. (2001a). Yolk protein endocytosis by oocytes in Drosophila melanogaster: immunofluorescent localization of clathrin, adaptin and the yolk protein receptor. J. Insect Physiol. 47, 715–723. doi: 10.1016/S0022-1910(00)00165-7
Richard, D. S., Jones, J. M., Barbarito, M. R., Cerula, S., Detweiler, J. P., Fisher, S. J., et al. (2001b). Vitellogenesis in diapausing and mutant Drosophila melanogaster: further evidence for the relative roles of ecdysteroids and juvenile hormones. J. Insect Physiol. 47, 905–913. doi: 10.1016/S0022-1910(01)00063-4
Richard, D. S., Watkins, N. L., Serafin, R. B., and Gilbert, L. I. (1998). Ecdysteroids regulate yolk protein uptake by Drosophila melanogaster oocytes. J. Insect Physiol. 44, 637–644. doi: 10.1016/S0022-1910(98)00020-1
Romney, A. L. T., Davis, E. M., Corona, M. M., Wagner, J. T., and Podrabsky, J. E. (2018). Temperature-dependent vitamin D signaling regulates developmental trajectory associated with diapause in an annual killifish. Proc. Natl. Acad. Sci. U.S.A. 115, 12763–12768. doi: 10.1073/PNAS.1804590115
Rosato, E., Trevisan, A., Sandrelli, F., Zordan, M., Kyriacou, C. P., and Costa, R. (1997). Conceptual translation of timeless reveals alternative initiating methionines in Drosophila. Nucleic Acids Res. 25, 455–457. doi: 10.1093/nar/25.3.455
Salminen, T. S., and Hoikkala, A. (2013). Effect of temperature on the duration of sensitive period and on the number of photoperiodic cycles required for the induction of reproductive diapause in Drosophila montana. J. Insect Physiol. 59, 450–457. doi: 10.1016/j.jinsphys.2013.02.005
Sandrelli, F., Tauber, E., Pegoraro, M., Mazzotta, G., Cisotto, P., Landskron, J., et al. (2007). A molecular basis for natural selection at the timeless locus in Drosophila melanogaster. Science 316, 1898–1900. doi: 10.1126/science.1138426
Santos, C. G., Humann, F. C., and Hartfelder, K. (2019). Juvenile hormone signaling in insect oogenesis. Curr. Opin. Insect Sci. 31, 43–48. doi: 10.1016/j.cois.2018.07.010
Saunders, D. S. (1990). The circadian basis of ovarian diapause regulation in Drosophila melanogaster: Is the period gene causally involved in photoperiodic time measurement?: J. Biol. Rhythms 5, 315–331. doi: 10.1177/074873049000500404
Saunders, D. S. (2014). Insect photoperiodism: effects of temperature on the induction of insect diapause and diverse roles for the circadian system in the photoperiodic response. Entomol. Sci. 17, 25–40. doi: 10.1111/ens.12059
Saunders, D. S. (2020). Dormancy, diapause, and the role of the circadian system in insect photoperiodism. Annu. Rev. Entomol. 65, 373–389. doi: 10.1146/annurev-ento-011019-025116
Saunders, D. S., and Gilbert, L. I. (1990). Regulation of ovarian diapause in Drosophila melanogaster by photoperiod and moderately low temperature. J. Insect Physiol. 36, 195–200. doi: 10.1016/0022-1910(90)90122-V
Saunders, D. S., Henrich, V. C., and Gilbert, L. I. (1989). Induction of diapause in Drosophila melanogaster: photoperiodic regulation and the impact of arrhythmic clock mutations on time measurement. Proc. Natl. Acad. Sci. U.S.A. 86, 3748–3752. doi: 10.1073/pnas.86.10.3748
Saunders, D. S., Richard, D. S., Applebaum, S. W., Ma, M., and Gilbert, L. I. (1990). Photoperiodic diapause in Drosophila melanogaster involves a block to the juvenile hormone regulation of ovarian maturation. Gen. Comp. Endocrinol. 79, 174–184. doi: 10.1016/0016-6480(90)90102-R
Schiesari, L., Andreatta, G., Kyriacou, C. P., O’Connor, M. B., and Costa, R. (2016). The insulin-like proteins dILPs-2/5 determine diapause inducibility in Drosophila. PLoS One 11:e0163680. doi: 10.1371/journal.pone.0163680
Schiesari, L., Kyriacou, C. P., and Costa, R. (2011). The hormonal and circadian basis for insect photoperiodic timing. FEBS Lett. 585, 1450–1460. doi: 10.1016/j.febslet.2011.02.026
Schiesari, L., and O’Connor, M. B. (2013). Diapause. Delaying the developmental clock in response to a changing environment. Curr. Top. Dev. Biol. 105, 213–246. doi: 10.1016/B978-0-12-396968-2.00008-7
Schmidt, P. S., and Conde, D. R. (2006). Environmental heterogeneity and the maintenance of genetic variation for reproductive diapause in Drosophila melanogaster. Evolution 60, 1602–1611. doi: 10.1111/j.0014-3820.2006.tb00505.x
Schmidt, P. S., and Paaby, A. B. (2008). Reproductive diapause and life-history clines in North American populations of Drosophila melanogaster. Evolution 62, 1204–1215. doi: 10.1111/j.1558-5646.2008.00351.x
Schmidt, P. S., Zhu, C.-T., Das, J., Batavia, M., Yang, L., and Eanes, W. F. (2008). An amino acid polymorphism in the couch potato gene forms the basis for climatic adaptation in Drosophila melanogaster. Proc. Natl. Acad. Sci. U.S.A. 105, 16207–16211. doi: 10.1073/PNAS.0805485105
Schmidt-Nielsen, K. (1997). Animal Physiology: Adaptation and Environment, 5th Edn. Cambridge: Cambridge University Press.
Schooneveld, H., Otazo Sanchez, A., and de Wilde, J. (1977). Juvenile hormone-induced break and termination of diapause in the Colorado potato beetle. J. Insect Physiol. 23, 689–696. doi: 10.1016/0022-1910(77)90085-3
Semaniuk, U., Piskovatska, V., Strilbytska, O., Strutynska, T., Burdyliuk, N., Vaiserman, A., et al. (2021). Drosophila insulin-like peptides: from expression to functions – a review. Entomol. Exp. Appl. 169, 195–208. doi: 10.1111/eea.12981
Sherald, A. F., and Wright, T. R. F. (1974). The analog inhibitor, α-methyl dopa, as a screening agent for mutants elevating levels of dopa decarboxylase activity in Drosophila melanogaster. Mol. Gen. Genet. 133, 25–36. doi: 10.1007/BF00268674
Shiga, S., Hamanaka, Y., Tatsu, Y., Okuda, T., and Numata, H. (2003). Juvenile hormone biosynthesis in diapause and nondiapause females of the adult blow fly Protophormia terraenovae. Zoolog. Sci. 20, 1199–1206. doi: 10.2108/zsj.20.1199
Shiga, S., and Numata, H. (2000). The role of neurosecretory neurons in the pars intercerebralis and pars lateralis in reproductive diapause of the blowfly, Protophormia terraenovae. Naturwissenschaften 87, 125–128. doi: 10.1007/s001140050689
Shiga, S., and Numata, H. (2009). Roles of PER immunoreactive neurons in circadian rhythms and photoperiodism in the blow fly, Protophormia terraenovae. J. Exp. Biol. 212, 867–877. doi: 10.1242/jeb.027003
Shimokawa, K., Numata, H., and Shiga, S. (2008). Neurons important for the photoperiodic control of diapause in the bean bug, Riptortus pedestris. J. Comp. Physiol. A Neuroethol. Sens. Neural Behav. Physiol. 194, 751–762. doi: 10.1007/s00359-008-0346-y
Shinoda, T., and Itoyama, K. (2003). Juvenile hormone acid methyltransferase: a key regulatory enzyme for insect metamorphosis. Proc. Natl. Acad. Sci. U.S.A. 100, 11986–11991. doi: 10.1073/pnas.2134232100
Siegmund, T., and Korge, G. (2001). Innervation of the ring gland of Drosophila melanogaster. J. Comp. Neurol. 431, 481–491.
Sim, C., and Denlinger, D. L. (2013). Insulin signaling and the regulation of insect diapause. Front. Physiol. 4:189. doi: 10.3389/fphys.2013.00189
Soller, M., Bownes, M., and Kubli, E. (1997). Mating and sex peptide stimulate the accumulation of yolk in oocytes of Droosophila melanogaster. Eur. J. Biochem. 243, 732–738. doi: 10.1111/j.1432-1033.1997.00732.x
Soller, M., Bownes, M., and Kubli, E. (1999). Control of oocyte maturation in sexually mature Drosophila females. Dev. Biol. 208, 337–351. doi: 10.1006/dbio.1999.9210
Sugamori, K. S., Demchyshyn, L. L., McConkey, F., Forte, M. A., and Niznik, H. B. (1995). A primordial dopamine D1-like adenylyl cyclase-linked receptor from Drosophila melanogaster displaying poor affinity for benzazepines. FEBS Lett. 362, 131–138. doi: 10.1016/0014-5793(95)00224-W
Swevers, L. (2019). An update on ecdysone signaling during insect oogenesis. Curr. Opin. Insect Sci. 31, 8–13. doi: 10.1016/j.cois.2018.07.003
Tatar, M., Chien, S. A., and Priest, N. K. (2001). Negligible senescence during reproductive dormancy in Drosophila melanogaster. Am. Nat. 158, 248–258. doi: 10.1086/321320
Tatar, M., and Yin, C. M. (2001). Slow aging during insect reproductive diapause: Why butterflies, grasshoppers and flies are like worms. Exp. Gerontol. 36, 723–738. doi: 10.1016/S0531-5565(00)00238-2
Tauber, E., Zordan, M., Sandrelli, F., Pegoraro, M., Osterwalder, N., Breda, C., et al. (2007). Natural selection favors a newly derived timeless allele in Drosophila melanogaster. Science 316, 1895–1898. doi: 10.1126/science.1138412
Tauber, M., Tauber, C., and Masaki, S. (1986). Seasonal Adaptations of Insects. Oxford: Oxford University Press.
Toxopeus, J., Jakobs, R., Ferguson, L. V., Gariepy, T. D., and Sinclair, B. J. (2016). Reproductive arrest and stress resistance in winter-acclimated Drosophila suzukii. J. Insect Physiol. 89, 37–51. doi: 10.1016/j.jinsphys.2016.03.006
Truman, J. W. (2019). The evolution of insect metamorphosis. Curr. Biol. 29, R1252–R1268. doi: 10.1016/j.cub.2019.10.009
Tu, M.-P., Yin, C.-M., and Tatar, M. (2002). Impaired ovarian ecdysone synthesis of Drosophila melanogaster insulin receptor mutants. Aging Cell 1, 158–160. doi: 10.1046/J.1474-9728.2002.00016.X
Tu, M. P., Yin, C. M., and Tatar, M. (2005). Mutations in insulin signaling pathway alter juvenile hormone synthesis in Drosophila melanogaster. Gen. Comp. Endocrinol. 142, 347–356. doi: 10.1016/J.YGCEN.2005.02.009
Uryu, O., Ameku, T., and Niwa, R. (2015). Recent progress in understanding the role of ecdysteroids in adult insects: Germline development and circadian clock in the fruit fly Drosophila melanogaster. Zool. Lett. 1:32. doi: 10.1186/s40851-015-0031-2
Venken, K. J. T., Simpson, J. H., and Bellen, H. J. (2011). Genetic manipulation of genes and cells in the nervous system of the fruit fly. Neuron 72, 202–230. doi: 10.1016/j.neuron.2011.09.021
Wallingford, A. K., Lee, J. C., and Loeb, G. M. (2016). The influence of temperature and photoperiod on the reproductive diapause and cold tolerance of spotted-wing Drosophila, Drosophila suzukii. Entomol. Exp. Appl. 159, 327–337. doi: 10.1111/eea.12443
Watanabe, M., Kikawada, T., Minagawa, N., Yukuhiro, F., and Okuda, T. (2002). Mechanism allowing an insect to survive complete dehydration and extreme temperatures. J. Exp. Biol. 205, 2799–2802. doi: 10.1242/JEB.205.18.2799
Williams, K. D. (1993). Diapause in Drosophila melanogaster females: a genetic analysis. Heredity 71, 315–317. doi: 10.1038/hdy.1993.141
Williams, K. D., Busto, M., Suster, M. L., So, A. K.-C., Ben-Shahar, Y., Leevers, S. J., et al. (2006). Natural variation in Drosophila melanogaster diapause due to the insulin-regulated PI3-kinase. Proc. Natl. Acad. Sci. U.SA. 103, 15911–15915. doi: 10.1073/PNAS.0604592103
Woll, S. C., and Podrabsky, J. E. (2017). Insulin-like growth factor signaling regulates developmental trajectory associated with diapause in embryos of the annual killifish Austrofundulus limnaeus. J. Exp. Biol. 220, 2777–2786. doi: 10.1242/JEB.151373
Wood, S., and Loudon, A. (2014). Clocks for all seasons: unwinding the roles and mechanisms of circadian and interval timers in the hypothalamus and pituitary. J. Endocrinol. 222, R39–R59.
Wood, S. H., Christian, H. C., Miedzinska, K., Saer, B. R. C., Johnson, M., Paton, B., et al. (2015). Binary wwitching of calendar cells in the pituitary defines the phase of the circannual cycle in mammals. Curr. Biol. 25, 2651–2662. doi: 10.1016/j.cub.2015.09.014
Wright, T. R. F., Bewley, G. C., and Sherald, A. F. (1976). The genetics of dopa decarboxylase in Drosophila melanogaster. II. Isolation and characterization of dopa decarboxylase deficient mutants and their relationship to the α methyl dopa hypersensitive mutants. Genetics 84, 287–310.
Yamada, H., and Yamamoto, M. T. (2011). Association between circadian clock genes and diapause incidence in Drosophila triauraria. PLoS One 6:e27493. doi: 10.1371/journal.pone.0027493
Yamashita, O., and Yaginuma, T. (1991). “Silkworm eggs at low temperatures: implications for sericulture,” in Insects at Low Temperature, eds R. E. Lee and D. L. Denlinger (Boston, MA: Springer), 424–445. doi: 10.1007/978-1-4757-0190-6_18
Zhai, Y., Dong, X., Gao, H., Chen, H., Yang, P., Li, P., et al. (2019). Quantitative proteomic and transcriptomic analyses of metabolic regulation of adult reproductive diapause in Drosophila suzukii (Diptera: Drosophilidae) females. Front. Physiol. 10:344. doi: 10.3389/fphys.2019.00344
Zhai, Y., Lin, Q., Zhang, J., Zhang, F., Zheng, L., and Yu, Y. (2016). Adult reproductive diapause in Drosophila suzukii females. J. Pest Sci. 89, 679–688. doi: 10.1007/s10340-016-0760-9
Zhang, S. X., Glantz, E. H., Miner, L. E., Rogulja, D., and Crickmore, M. A. (2021). Hormonal control of motivational circuitry orchestrates the transition to sexuality in Drosophila. Sci. Adv. 7:eabg6926. doi: 10.1126/SCIADV.ABG6926
Zhang, X. O., Cui, X., and Zhu, D. (2019). Insulin producing cells monitor the cold and compensate the cold-induced sleep in Drosophila. bioRxiv [Preprint]. doi: 10.1101/419200
Zhao, X., Bergland, A. O., Behrman, E. L., Gregory, B. D., Petrov, D. A., and Schmidt, P. S. (2016). Global transcriptional profiling of diapause and climatic adaptation in Drosophila melanogaster. Mol. Biol. Evol. 33, 707–720. doi: 10.1093/MOLBEV/MSV263
Žitòan, D., Kingan, T. G., Hermesman, J. L., and Adams, M. E. (1996). Identification of ecdysis-triggering hormone from an epitracheal endocrine system. Science 271, 88–91. doi: 10.1126/science.271.5245.88
Keywords: reproductive dormancy, corpus allatum (corpora allata), juvenile hormone (JH), monoamine, insulin-like peptides (ILPs), ecdysone, ecdysis-triggering hormone, circadian clock
Citation: Kurogi Y, Mizuno Y, Imura E and Niwa R (2021) Neuroendocrine Regulation of Reproductive Dormancy in the Fruit Fly Drosophila melanogaster: A Review of Juvenile Hormone-Dependent Regulation. Front. Ecol. Evol. 9:715029. doi: 10.3389/fevo.2021.715029
Received: 26 May 2021; Accepted: 30 August 2021;
Published: 23 September 2021.
Edited by:
Takashi Koyama, University of Copenhagen, DenmarkReviewed by:
Gabriele Andreatta, Max F. Perutz Laboratories GmbH, AustriaManolis Lyrakis, University of Veterinary Medicine Vienna, Austria
Copyright © 2021 Kurogi, Mizuno, Imura and Niwa. This is an open-access article distributed under the terms of the Creative Commons Attribution License (CC BY). The use, distribution or reproduction in other forums is permitted, provided the original author(s) and the copyright owner(s) are credited and that the original publication in this journal is cited, in accordance with accepted academic practice. No use, distribution or reproduction is permitted which does not comply with these terms.
*Correspondence: Ryusuke Niwa, ryusuke-niwa@tara.tsukuba.ac.jp
†Present address: Eisuke Imura, Department of Entomology, Institute for Integrative Genome Biology, University of California, Riverside, Riverside, CA, United States