Effects of microalgae as dietary supplement on palatability, digestibility, fecal metabolites, and microbiota in healthy dogs
- 1REQUIMTE, LAQV, ICBAS, School of Medicine and Biomedical Sciences, University of Porto, Porto, Portugal
- 2HoLMiR – Hohenheim Center for Livestock Microbiome Research, University of Hohenheim, Stuttgart, Germany
- 3Institute of Animal Science, University of Hohenheim, Stuttgart, Germany
The current trend of dog owners increasingly favoring the functional value of food to assure preventive health and wellbeing of their pets has been raising the interest in microalgae as natural additives with bioactive properties. However, scientific studies addressing the effects of microalgae supplementation in diets for dogs are scarce. This study aimed to evaluate the effects of dietary supplementation with three microalgae species (Chlorella vulgaris, Nannochloropsis oceanica, and Tetradesmus obliquus) on diet palatability, total tract digestibility, metabolizable energy content, fecal metabolites and microbiota of dogs. Twelve adult Beagle dogs were used in three two-bowl tests to compare the palatability of a commercial complete diet for adult dogs without (reference diet) and with 1.5% supplementation of each microalgae. From the results obtained, three digestibility trials were performed according to a replicated Latin square 3 × 3, with six adult Beagle dogs, three experimental periods of 10 days each, and three dietary supplementation levels of microalgae (0.5, 1.0, and 1.5%). In each trial, effects of microalgae supplementation levels on total tract digestibility, metabolizable energy content, fecal metabolites and microbiota of dogs were evaluated. First diet approached or tasted was not significantly affected by microalgae inclusion, but dogs showed a preference for the reference diet over the diets with 1.5% inclusion of C. vulgaris and N. oceanica, no difference being observed with 1.5% T. obliquus. In all digestibility trials, dietary supplementation with microalgae up to 1.5% did not greatly affected the dietary chemical composition and kept unaffected food intake, fecal output and metabolites, and digestibility of nutrients and energy. Compared with the reference diet, supplementation with C. vulgaris increased protein digestibility. Fecal characteristics and metabolites were affected by microalgae supplementation, being the effects dependent on the species. Fecal microbiota composition of dogs fed with microalgae-supplemented diets was modified by promoting the beneficial Turicibacter and Peptococcus genera associated with gut health and activation of the immune system. Overall, the results support C. vulgaris, N. oceanica, and T. obliquus as sustainable functional supplements that potentially enhance gastrointestinal health of dogs through the selective stimulation of microbiota without detrimental effects on food intake and digestibility.
1. Introduction
Microalgae are unicellular photosynthetic microorganisms rich in macro- and micronutrients and in bioactive compounds such as proteins, peptides, lipids, polyunsaturated fatty acids, pigments, minerals, and polysaccharides (1). Microalgae have been suggested to be a viable strategy for a more sustainable food sector due to their ability to convert inorganic and organic carbon sources into nutrient-rich biomass more efficiently than terrestrial plants and require less land and water resources (2). However, to take advantage of microalgae’s potential in pet food, their market availability at reasonable prices is crucial. The large-scale cultivation of some microalgae species developed in recent years have promoted their use in animal feeding, comprising 19% of the European production (3). In the pet food sector, the demand for microalgae is expected to continue growing (4), being microalgae currently used mainly as additives (generally with declared levels lower than 0.5%) in balanced food and in supplements or treats to benefit from their functional value as immunomodulatory (5), antioxidant, antimicrobial, and anti-inflammatory effects (6). The current tendency of dog owners increasingly choose the food that provides increased nutrition, health and wellness to their pets and the growing numbers of senior animals contributes to the raised interest in microalgae in the pet food market in recent years (7).
Despite the recently unveiled potential of microalgae as alternative and more sustainable food for dogs (8) and the commercial availability of microalgae-based pet food products, scientific studies addressing the effects of microalgae supplementation of diets for dogs are scarce. Positive effects of 0.4% Schizochytrium sp. dietary inclusion has been reported on palatability, protein digestibility and oxidative stability of diets, and phagocytic cell numbers of dogs (9), and to contribute to healthy brain function in a canine model of senescence (10). In dogs with the hematopoietic system damaged by irradiation, a polysaccharide of Arthrospira platensis (formerly Spirulina platensis) included at 0.08% (corresponding to 10.7% whole, dried spirulina) increased white blood cell number (11). A dietary inclusion of 0.2% spray-dried A. platensis has been shown to have an immune-stimulation effect as a higher vaccine response and higher levels of fecal IgA were observed in supplemented dogs compared to the control group (5). Although palatability of nutraceuticals can greatly impact convenience of administration and owner compliance (12), only scarce studies have assessed the palatability of microalgae supplemented diets.
Despite the known effects of some microalgae species on gut microbiota (13), to the best of our knowledge, there are no studies evaluating effects on dog gut microbiota, with the only exception of the work performed by Delsante et al. (14) using an in vitro canine gut model. In this study, microalgae species (A. platensis, Haematococcus pluvialis, Phaeodactylum tricornutum, and Chlorella vulgaris) have been shown to affect microbial saccharolytic activities and fecal bacterial composition, though in a less extent than anticipated from other species.
To deeper the knowledge on the potential of microalgae as supplements for dog feeding, this study aimed to evaluate the effects of different supplementation levels of C. vulgaris, Nannochloropsis oceanica, and Tetradesmus obliquus, among the top produced species in Europe (3), on palatability, apparent total tract digestibility of nutrients and energy (ATTD), metabolizable energy (ME) content, fecal metabolites and microbiota of dogs.
2. Materials and methods
Trials were approved by the Animal Ethics Committee of School of Medicine and Biomedical Sciences, University of Porto (Permit No. 344). Animal handling and procedures were performed in accordance with good animal welfare practices (European Union Directive 2010/63/EU) by trained scientists in laboratory animal science (FELASA, category C). All dogs were subjected to physical and clinical examinations to check their suitability to participate in the trial. Dogs were healthy throughout the length of the study, with no clinical signs of disease.
2.1. Animals and housing
Twelve healthy Beagle dogs (mean age: 2.2 ± 0.03 years; mean body weight (BW): 12.6 ± 1.55 kg), six males and six females, housed at the kennel of the School of Medicine and Biomedical Sciences, University of Porto, were used in the experimental protocols. Sample size was defined in accordance with the minimum number of animals recommended by the FEDIAF (15) for digestibility experiments. Animals were housed in pairs in environmentally enriched and communicating boxes with sliding doors to allow their individual feeding. Each box comprises an interior and an exterior area of 1.8 and 3.5 m2, respectively. Animals were leash walked once a day for at least 30 min and had free access to an outdoor park area between daily meals to exercise and socialize. During the feces collection period of the digestibility trials, animals were housed individually, having supervised access to an outdoor park between daily meals to ensure the collection of individual feces. Temperature and relative humidity of the kennel were monitored daily.
2.2. Palatability trials
A high economy commercial extruded complete diet for adult dogs (SilverDog, Sorgal Pet Food, Ovar, Portugal) widely available in retail stores as supermarkets and hypermarkets and including (label information) cereals, animal meals, wheat bran and beet pulp, and without the inclusion of microalgae was used as a reference diet. Three two-bowl tests (16) were conducted to determine palatability by the pairwise comparison of the reference diet with the reference diet supplemented with 1.5% of each microalgae species in substitution of the reference diet. The three studied commercially available microalgae species were produced locally in photobioreactors (Allmicroalgae – Natural Products, S.A.; Pataias, Portugal) and were provided as a spray dried powder in airtight bags protected from light. Microalgae were added to the reference diet immediately before offering the mixture to each dog, thus not being included in the reference diet kibble. After overnight fasting and for two consecutive days, the animals (n = 12) were given the choice between the two diets in two different bowls (45 cm apart). The position of the bowls was switched between days to control side bias. Daily feed allowance was calculated to supply the energy requirements of dogs (15). The bowl that was first approached and the diet that was first tasted were recorded. Trials ended after 30 min or until animals consumed all the food available in one bowl. Food offered and food residues were weighed to calculate the intake ratio of the two diets.
2.3. Digestibility trials
All digestibility trials were conducted using the method of total fecal collection. The in vivo digestibility of the reference diet was firstly determined using 12 animals for 10 days following the guidelines of FEDIAF (15). Then, three digestibility trials were conducted to evaluate the effects of increasing levels of dietary supplementation (0.5, 1.0, and 1.5% in substitution of the reference diet) of C. vulgaris, N. oceanica, and T. obliquus. The levels of microalgae supplementation were defined after evaluating the palatability of diets with 1.5% inclusion of each microalgae. Microalgae were added to the reference diet immediately before offering the mixture to each dog, thus not being included in the kibble.
The three trials were designed according to a replicated Latin square 3 × 3, with six animals (three males and three females, selected from the 12 animals used for the evaluation of the in vivo digestibility of the reference diet), three experimental periods, and three dietary inclusion levels (0.5, 1.0, and 1.5% in substitution of the reference diet). Each period lasted 10 days, with 5 days for diet adaptation, and 5 days for total feces collection. In all trials, daily food allowance was calculated to meet the ME requirements according to the ideal BW of individuals [ME (kcal/day) = 110 × BW0.75; (15)], and adjusted to body condition score assessed through a scale from 1 to 9 (17). Animals were individually fed twice a day the daily ration in two equal meals, at 8:30 h and 17:00 h, and had free access to fresh water at all times.
During total feces collection period, the number of defecations was recorded every day and individual fecal samples collected from the concrete floor were scored using a 5-point scale [from 1, corresponding to watery diarrhoea, to 5, corresponding to powdery hard mass pellets; (18)], weighed, mixed, subsampled at different locations and immeditaley frozen at −20°C until the end of the trials to perform analysis of chemical composition, fecal pH, ammonia-N and volatile fatty acids concentrations, and fecal microbiota. Analysis were carried out in feces composited by period and dog.
2.4. Analytical procedures
2.4.1. Proximate analysis
The proximate composition of the reference diet (dried at 65°C and 1 mm milled), of the microalgae species and of the feces (dried at 65°C and 1 mm milled) was analyzed in duplicate according to official methods (19), as described by Cabrita et al. (8). Briefly, samples were analyzed for dry matter (DM; ID 934.01), ash (ID 942.05), total lipids, and Kjeldahl N (ID 990.03). Crude protein (CP) was calculated as Kjeldahl N × 6.25. Neutral detergent fiber (with α-amylase and without sodium sulfite, NDF) was analyzed in all samples and expressed exclusive of residual ash (20). Acid detergent fiber (ADF) of the reference diet and microalgae species were also analyzed and expressed exclusive of residual ash (21). For microalgae, hydrolyzed samples were filtered through a glass microfiber filter (Whatman GF/A, 1.6 μm porosity, Merck KGaA, Darmstadt, Germany). For the reference diet and microalgae, starch content was determined according to Salomonsson et al. (22) and gross energy (GE) using an adiabatic bomb calorimeter (Werke C2000, IKA, Staufen, Germany). The chemical composition of the reference and experimental diets supplemented with increasing levels of each microalga (0.5, 1.0, and 1.5%) in substitution of the reference diet is presented in Tables 1, 2; chemical composition of the studied microalgae species and a more detailed characterization of the reference and the experimental diets being presented in Supplementary Tables S1, S2.
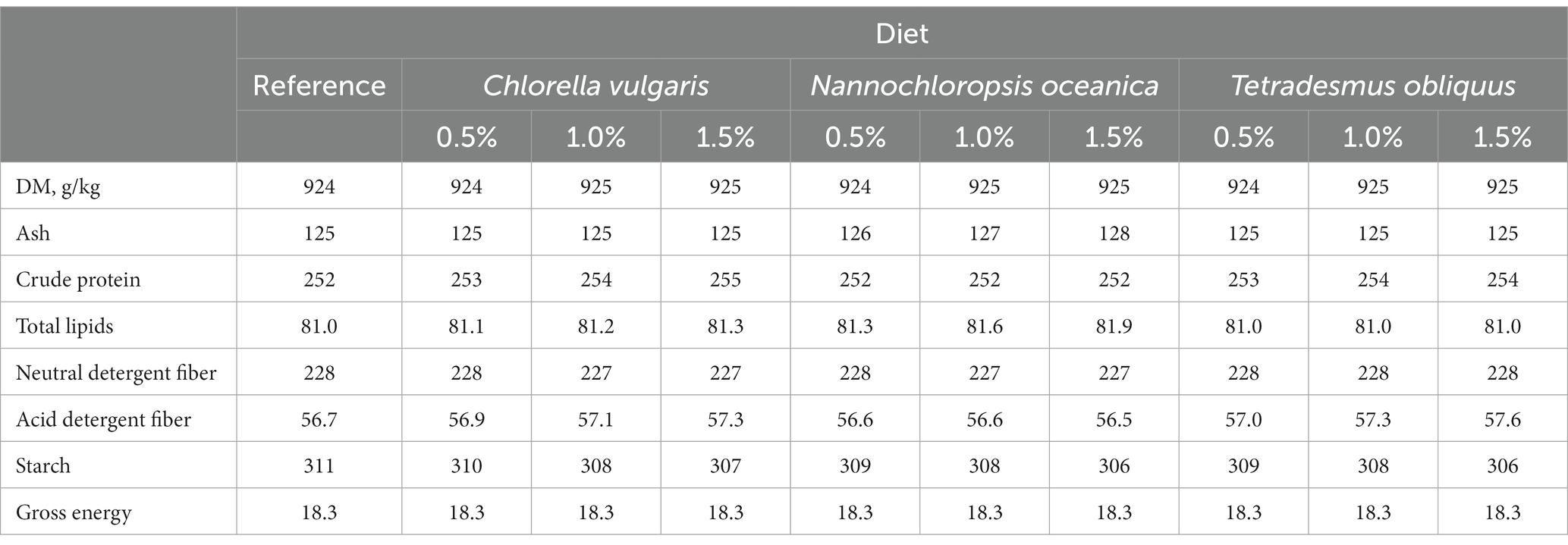
Table 1. Proximate composition (g kg−1 dry matter, DM) and gross energy (MJ kg−1 DM) of the reference and experimental diets with inclusion of microalgae in substitution of the reference diet.
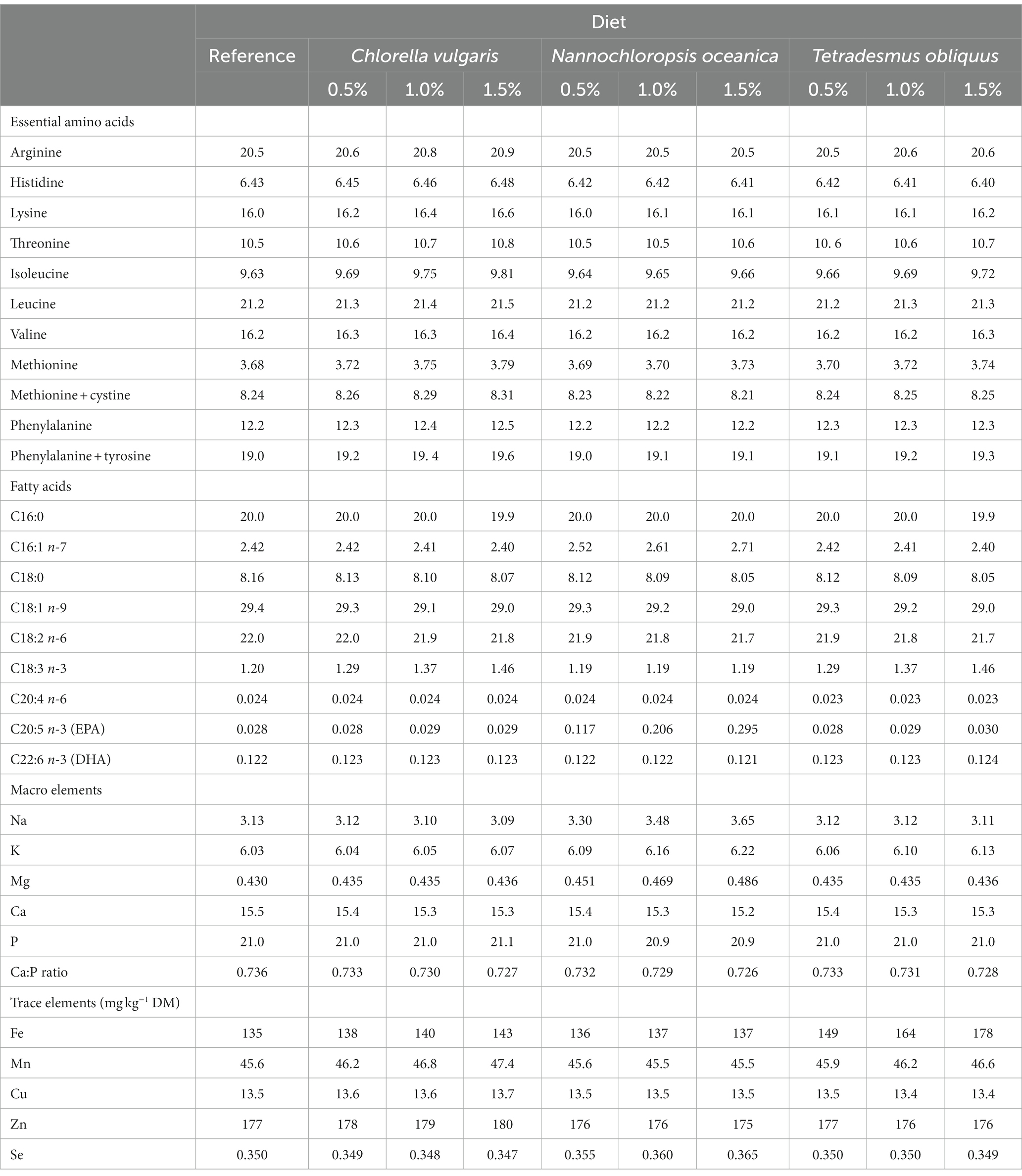
Table 2. Essential amino acids, macro- and trace elements, and selected fatty acids content (g kg−1 dry matter, DM) of the reference and experimental diets with inclusion of microalgae in substitution of the reference diet.
2.4.2. Amino acid analysis
Amino acid analysis were performed in duplicate. Samples of the reference diet and microalgae species were hydrolyzed with 6 M HCl solution at 116°C for 48 h and precolumn derivatized with Waters AccQ Fluor Reagent (6-aminoquinolyl-N-hydroxysuccinimidyl carbamate) according to the AccQ Tag method (Waters, Milford, MA). Analyses were carried out by ultra-high-performance liquid chromatography on a Waters reversed-phase amino acid analysis system with norvaline as internal standard. The resulting peaks were analysed with EMPOWER software [Waters; (23)].
2.4.3. Fatty acid analysis
Fatty acids of the reference diet and microalgae samples were converted to fatty acid methyl esters by acid-catalyzed transesterification with methanolic HCl (24) and analyzed by gas chromatography as reported by Maia et al. (25). Nonadecanoic acid (Matreya LLC, Pleasant Gap, PA) was used as internal standard. Fatty acids were identified by comparing retention times to commercially available standards and quantified with the internal standard.
2.4.4. Mineral analysis
Minerals and trace elements of microalgae and reference diet samples were determined in triplicate as described by Cabrita et al. (26). Briefly, reference diet and microalgae samples were mineralized (MLS 1200 Mega high-performance microwave digestion unit, Milestone, Sorisole, Italy) and sample solutions analyzed by inductively coupled plasma-mass spectrometry (ICP-MS; iCAP Q ICP-MS instrument, Thermo Fisher Scientific, Waltham, MA) and flame atomic absorption spectrometry (FAAS; AAnalyst 200 FAAS instrument, PerkinElmer, Shelton, CT). Calibration standards from 1,000 mg/L single-element standard stock solutions (Fluka, Buchs, Switzerland) were diluted with HNO3 0.2% (v/v) for FAAS analysis. For ICP-MS determinations, internal standards and tuning solutions from diluted commercial solutions were prepared (Periodic table mix 3 for ICP-MS, TraceCERT®, Sigma-Aldrich, Buchs, Switzerland; custom solution, SCP Science, Baie D’Urfé, QC, Canada).
2.4.5. Fecal pH, ammonia-N, and volatile fatty acids concentrations
Analysis was run in duplicate. Thawed feces were diluted to 1:10 (w/v) in 20 mL of water, sonicated, and incubated for 10 min at room temperature. The pH was determined using a potentiometer (pH and Ion-Meter GLP 22, Crison, Barcelona, Spain). The concentration of ammonia-N was determined using the method of Smith et al. (27) adapted to dog feces. Briefly, 1 g of feces were solubilized in 10 mL of KCl 2 M, centrifuged for 60 min at 5200 × g at 4°C, and the supernatant filtered using a 0.45 μm pore size polyethersulfone syringe filter (FILTER-LAB, Barcelona, Spain). Forty μL of supernatant were mixed with 40 μL of water, 2.5 mL of phenol solution and 2 mL of alkaline hypochlorite solution. After incubation for 10 min at 37°C and 40 min in the dark at 22°C, the absorbance of samples was read at 550 nm in a SynergyTM HT Multimode plate reader (BioTek® Instruments Inc., Winooski, VT). An ammonia solution (32 mg/dL) was used as standard. For volatile fatty acids (VFA) analysis, feces were acidified with ortho-phosphoric acid solution, centrifuged for 60 min at 2360 × g at 4°C, and the supernatant analyzed by gas chromatography as described by Pereira et al. (28).
2.4.6. Fecal microbiota
For microbiota analysis, fecal DNA in thawed samples was extracted by FastDNA™ Spin Kit for soil (MP Biomedicals, Irvine, CA) and used for 16S library preparation, targeting bacterial V1–V2 hypervariable regions (29). Unique barcodes (6-nt) were attached to forward primers, and index adapters were linked to reverse. Amplicons were obtained by two-step polymerase chain reaction (PCR). Briefly, 1 μL of DNA was added for the first PCR, in a 20 μL reaction with 0.2 μL of PrimeSTAR HS DNA polymerase (TaKaRa, Beijing, China) and 0.5 μL of each primer. The second PCR, which used 1 μL of the first PCR as a template, ran in a total volume of 50 μL. An initial denaturation at 95°C for 3 min was followed by 15 cycles (first PCR) or 20 cycles (second PCR) of denaturation at 98°C for 10 s, subsequent annealing at 55°C for 10 s, extension step at 72°C for 45 s and a final extension for 2 min at 72°C. Amplicon normalization was performed by the SequalPrep Normalization Kit (Invitrogen Inc., Carlsbad, CA) and sequenced with the 250 bp paired-end Illumina NovaSeq 6,000 platform.
Sequences were demultiplexed with Sabre1 and analyzed using Qiime2 (30). Primers were trimmed with q2-cutadapt plugin (31). Denoising and merging were accomplished by the q2-dada2 (32). Taxonomic classification of amplicon sequence variants (ASVs) was carried out with VSEARCH-based consensus (33) and pre-fitted sklearn-based classifiers (34) against the Silva database [v138.1, 16S 99%; (35)], for which reference reads and corresponding taxonomies were prepared by RESCRIPt (36). A phylogenetic tree was constructed by the q2-phylogeny, utilizing MAFFT [v7.3; (37)] and FastTree [v2.1; (38)]. For calculation of diversity metrics, the dataset was rarefied to 15,000 reads. Alpha diversity was assessed by Shannon’s entropy (39) and beta diversity by Bray–Curtis (40) distances. Beta diversity ordination was carried out by principal-coordinate analysis [PCoA; (41)]. Alpha diversity metrics were compared by Wilcoxon test (42), and beta diversity distances by the adonis test [999 permutations; (43)]. Differentially abundant genera (only for counts of genera with relative abundance ≥1% and prevalence ≥10%) were detected by ALDEx2 (44). All p-values obtained from multiple comparisons were adjusted using the Benjamini-Hochberg procedure (45).
Raw sequences are available at the European Nucleotide Archive (ENA) under accession number PRJEB61064.
2.5. Calculations and statistical analysis
Diet first-approach and first taste results were submitted to the Chi-square test and the intake ratio (intake of reference diet or diet with 1.5% microalgae inclusion / total intake of both diets) to the Student’s t-test, both at 5% probability level (n = 24).
Fecal production (%) was calculated as:
Apparent total tract digestibility (%) of the reference diet and diets with microalgae inclusion was calculated using the equation as follows:
The following equation calculated ME content (MJ/kg DM) of diets (46):
For each digestibility trial, data on food and nutrient intake, fecal production and characteristics, ATTD, fecal pH, and ammonia-N and VFA concentrations were analyzed according to a replicated 3 × 3 Latin square. The model included the fixed effects of the square, dog within the square, period, level of microalgae inclusion and the residual error (SAS 2021, release 3.1.0., SAS Institute, Cary, NC, United States). When differences were significant (p < 0.05), the least significant difference test was used to compare means.
As experimental period had a minor effect on the parameters measured in the digestibility trials, and regarding ATTD, only ATTD of DM, organic matter and NDF were affected by period in the trial with C. vulgaris supplementation, a t-test was performed to compare the reference diet with diets with inclusion of microalgae (SAS 2021, release 3.1.0.) to mimic the at-home scenario of dog owners changing the diet offered to their animals, thus understand the perceived effects. For each microalgae under study, data from the six dogs collected during the digestibility trial on the reference diet were used for comparison.
3. Results
3.1. Palatability trials
The results of the two-bowl tests are shown in Table 3. First diet approached and first diet tasted were not affected by microalgae inclusion (p > 0.05). Dogs showed a preference for the reference diet in comparison with diets with 1.5% inclusion of C. vulgaris (p = 0.003) and N. oceanica (p < 0.001), no difference being observed for intake ratio with the inclusion of 1.5% of T. obliquus (p = 0.121).

Table 3. First approach and taste, and intake ratio of reference diet and experimental diets supplemented with 1.5% of microalgae in substitution of the reference diet.
3.2. Chemical composition of reference and experimental diets
The chemical composition of the commercial complete diet for adult dogs used as the reference diet presented 252 g/kg CP, 81.0 g/kg total lipids, 16.0 g/kg Lys, 22.0 g/kg C18:2 n-6, and 0.736 Ca:P ratio. Dietary supplementation with microalgae up to 1.5% did not greatly affect the chemical composition of diets, with observed minor changes reflecting the chemical composition of microalgae species (Tables 1, 2).
3.3. Digestibility trials
All dogs remained healthy throughout the studies. No weight loss, vomiting, or diarrhea were observed. As food allowance was adjusted according to ideal BW and body condition score, BW of dogs remained unchanged during all the trials.
3.3.1. Experiment 1. Chlorella vulgaris
Level of C. vulgaris supplementation did not affect food and nutrient intake and fecal output (p > 0.05), but number of defecations was higher when dogs were fed the diet supplemented with 1.0% of C. vulgaris (p = 0.026; Table 4). Digestibility of DM, nutrients and energy and ME content was not affected by C. vulgaris supplementation level, except ATTD of NDF that tended (p = 0.058; Table 4) to be higher with 1.5% supplementation. Fecal pH, and ammonia-N and VFA concentrations were not affected by C. vulgaris supplementation (p > 0.05; Table 4).
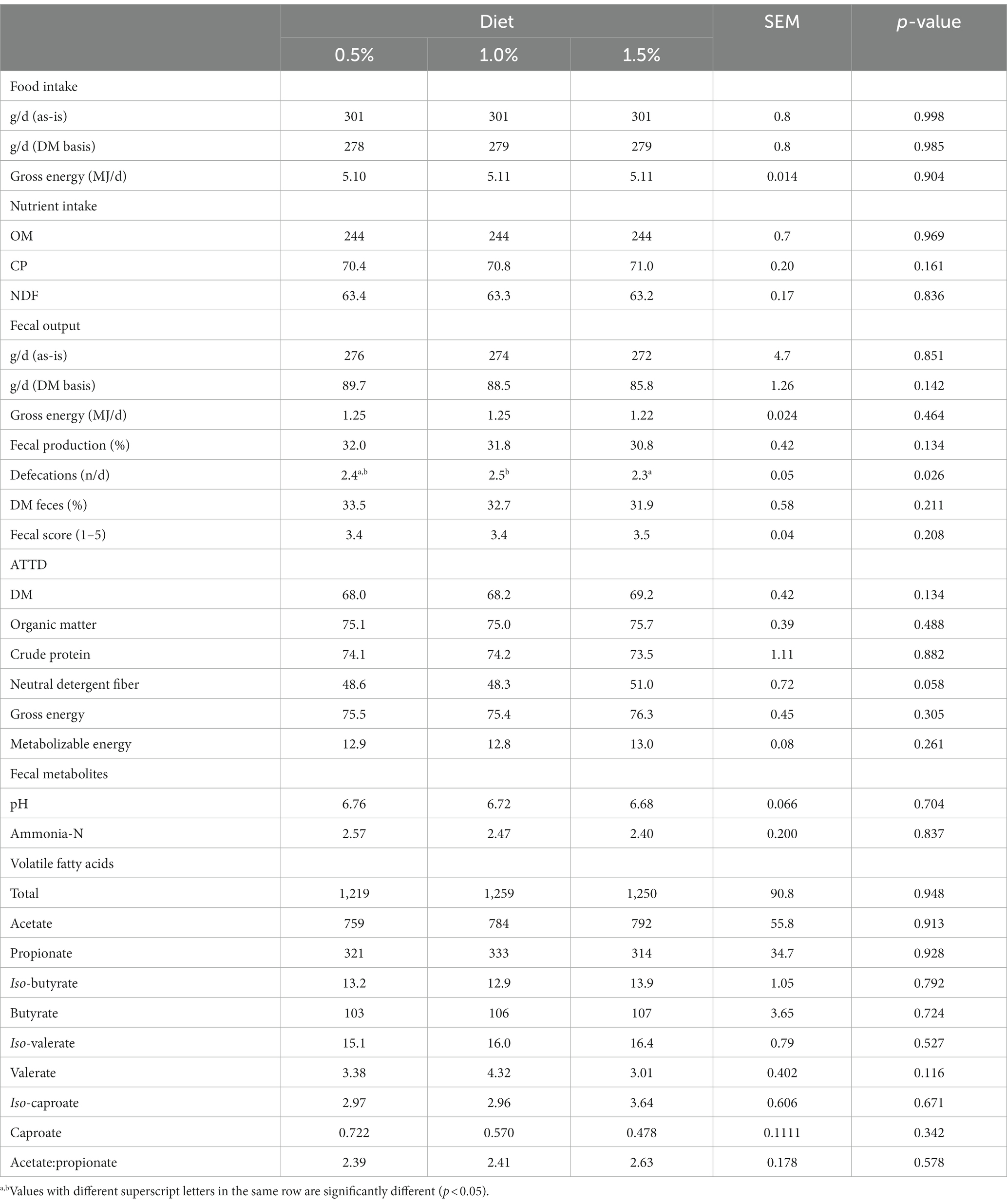
Table 4. Experiment 1. Effects of increasing supplementation levels of Chlorella vulgaris on food, gross energy and nutrient intake (dry matter, DM, basis), fecal output and characteristics, apparent total tract digestibility (ATTD, %), metabolizable energy content (MJ kg−1 DM), and fecal pH, concentration of ammonia-N (g kg−1 DM), and concentration of volatile fatty acids (μmol g−1 DM).
3.3.2. Experiment 2. Nannochloropsis oceanica
Supplementation level of N. oceanica did not affect food and nutrient intake and fecal output (p > 0.05; Table 5). Despite the significant effect of N. oceanica inclusion level on fecal score (p = 0.036; Table 5), differences observed (3.3 vs. 3.4) lack biological meaning. Digestibility of DM, nutrients and energy, and ME content and fecal pH, and ammonia-N and VFA concentrations were not affected (p > 0.05) by level of supplementation with N. oceanica, except for iso-valerate (p = 0.051) and iso-caproate (p = 0.079) concentrations where a tendency was observed (Table 5).
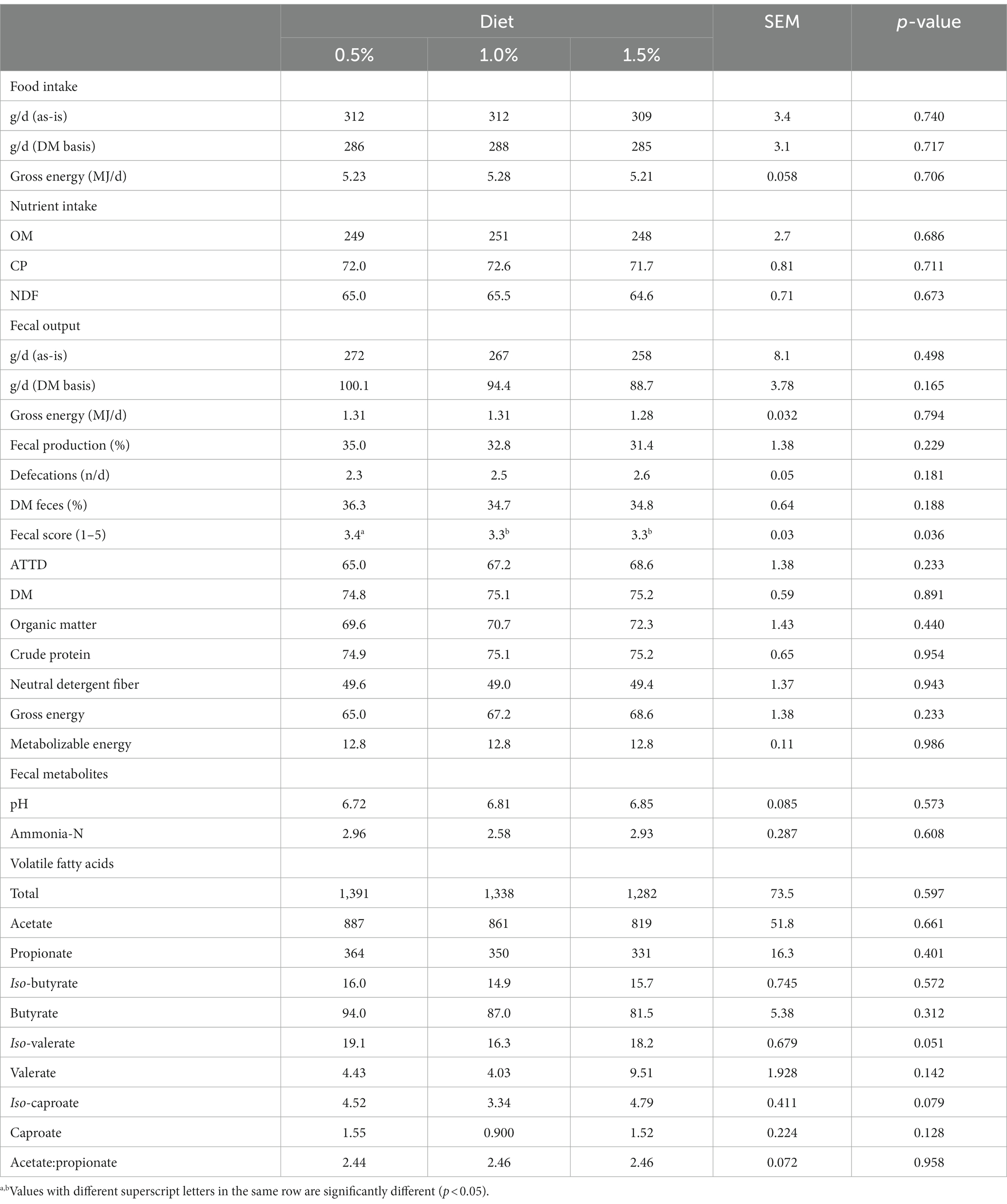
Table 5. Experiment 2. Effects of increasing supplementation levels of Nannochloropsis oceanica on food, gross energy and nutrient intake (dry matter, DM, basis), fecal output and characteristics, apparent total tract digestibility (ATTD, %), metabolizable energy content (MJ kg−1 DM), and fecal pH, concentration of ammonia-N (g kg−1 DM), and concentration of volatile fatty acids (μmol g−1 DM).
3.3.3. Experiment 3. Tetradesmus obliquus
Table 6 presents the effects of level of T. obliquus supplementation on food and nutrient intake, fecal output and characteristics, ATTD of DM, nutrients and energy and ME content, and fecal metabolites. Level of microalgae supplementation did not affect any of the parameters measured (p > 0.05) with the only exception of number of defecations that tended to be higher (p = 0.06) with 1.5% of supplementation.
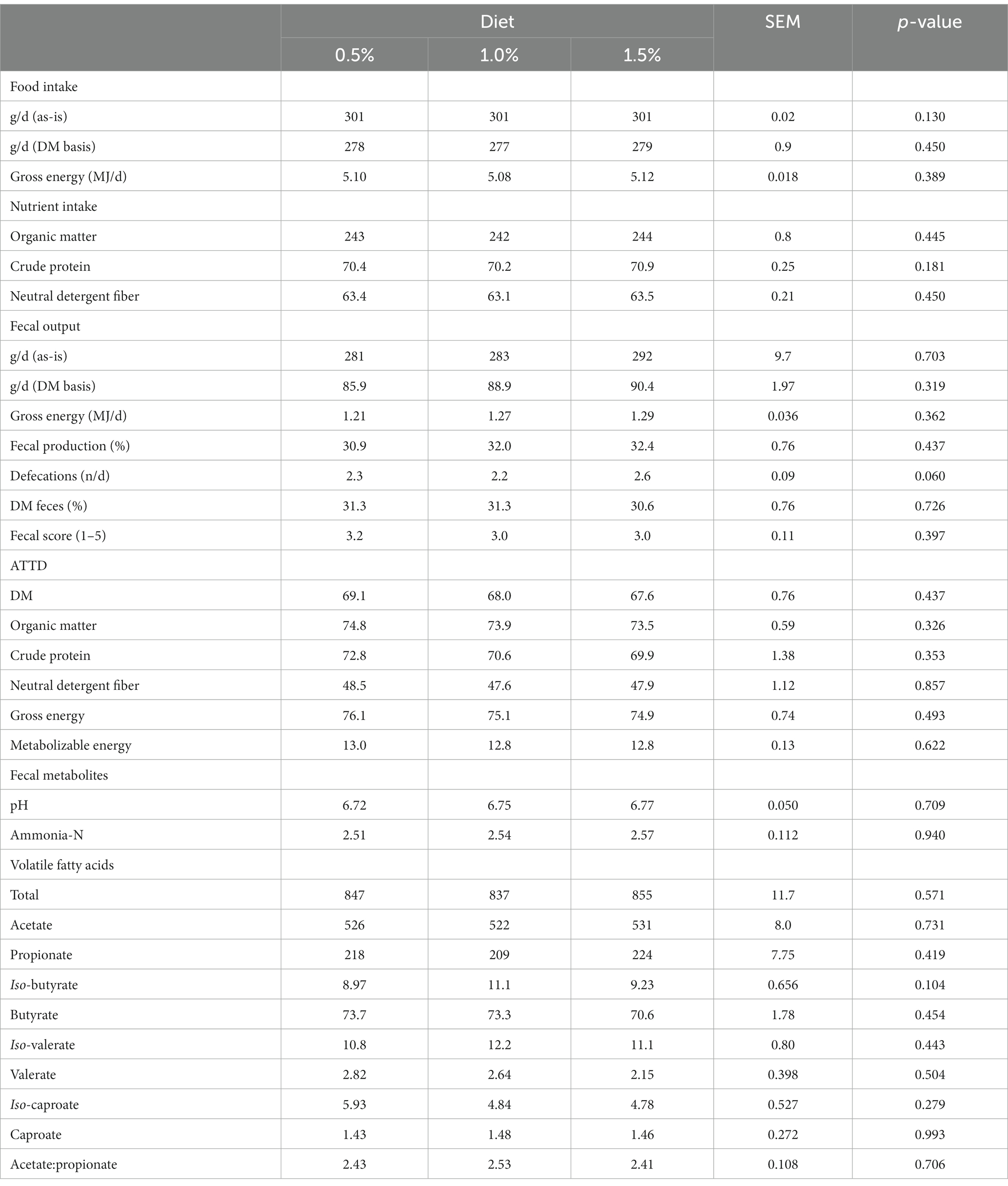
Table 6. Experiment 3. Effects of increasing supplementation levels of Tetradesmus obliquus on food, gross energy and nutrient intake (dry matter, DM, basis), fecal output and characteristics, apparent total tract digestibility (ATTD, %), metabolizable energy content (MJ kg−1 DM), and fecal pH, concentration of ammonia-N (g kg−1 DM), and concentration of volatile fatty acids (μmol g−1 DM).
3.4. Comparison between reference and experimental diets
Food and nutrient intake and fecal output from dogs fed diets supplemented with microalgae were not significantly different from the reference diet (p > 0.05; Table 7). Comparing to the reference diet, the dietary supplementation with C. vulgaris (p = 0.044) and T. obliquus (p = 0.035) decreased the number of defecations (Table 7). Consistency of feces of dogs fed the reference diet or diets with microalgae was classified as soft, shaped, and moist stools leaving spots on the floor (3.0) to approximately firm, shaped, and dry stools (3.5). Dry matter content of feces was higher with N. oceanica supplementation over the reference diet (35.5% vs. 32.0%; p = 0.010), no effect being observed with T. obliquus and C. vulgaris dietary inclusion (Table 7).
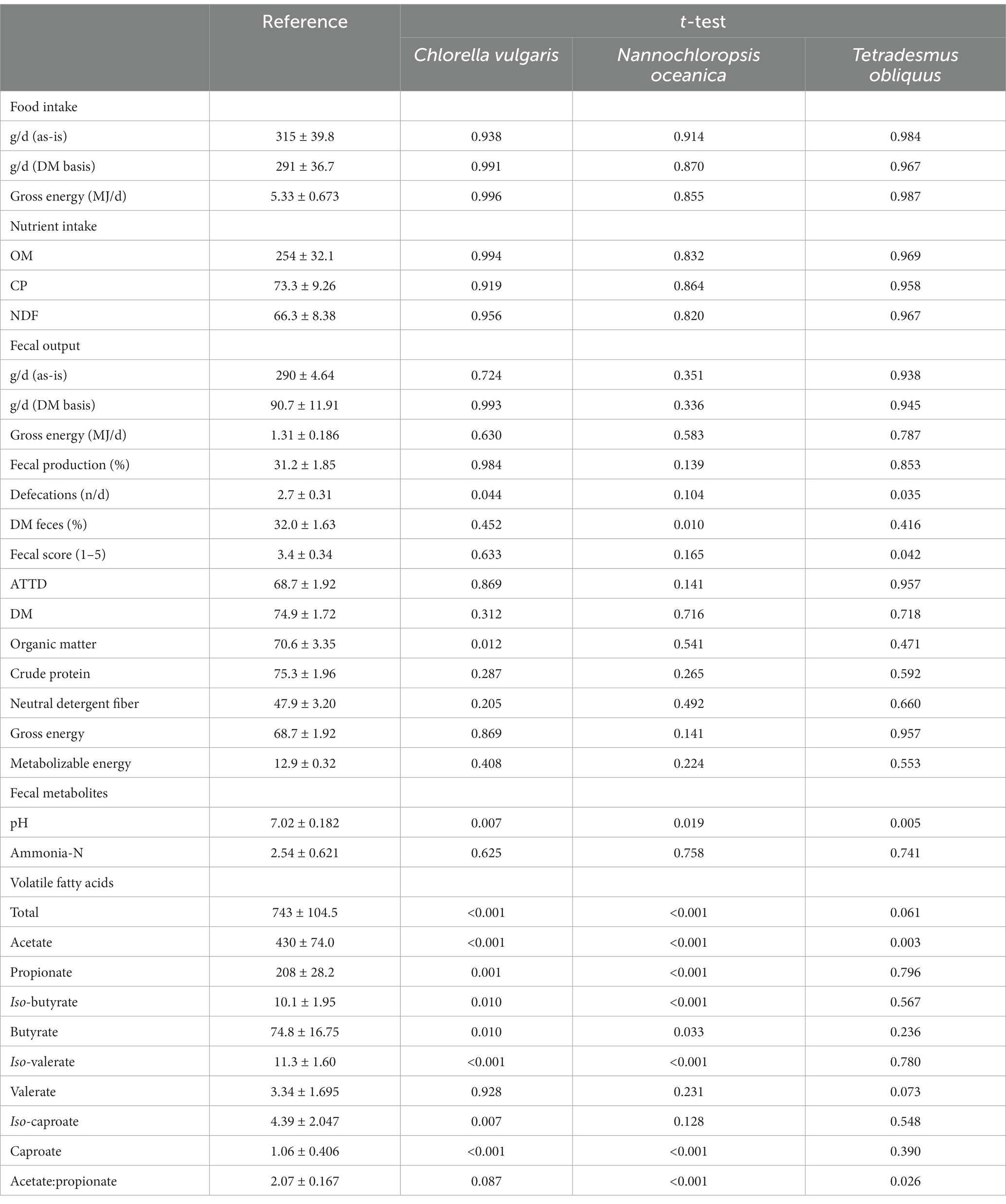
Table 7. Comparison between effects of reference diet and experimental diets supplemented with microalgae inclusion on food, gross energy and nutrient intake (dry matter, DM, basis), fecal output and characteristics, apparent total tract digestibility (ATTD, %), metabolizable energy content (MJ kg−1 DM), and fecal pH, concentration of ammonia-N (g kg−1 DM), and concentration of volatile fatty acids (μmol g−1 DM).
Compared to the reference diet, the dietary supplementation with T. obliquus decreased the fecal score (3.1 vs. 3.4; p = 0.042), no changes being observed with C. vulgaris and N. oceanica (p > 0.05; Table 7). The reference diet showed an ATTD of DM and CP, respectively, of 68.7 and 70.6%, and a ME content of 12.9 MJ kg−1 DM (Table 7). Compared with the reference diet, the dietary inclusion of microalgae did not affect ATTD of DM, nutrients and energy, and ME content (p > 0.05), except for the inclusion of C. vulgaris that increased ATTD of CP (73.9% vs. 70.6%; p = 0.012; Table 7). Microalgae supplementation decreased fecal pH and increased acetate concentration, regardless of the microalgae species (Table 7). Supplementation with C. vulgaris and N. oceanica increased total VFA production and concentrations of propionate, iso-butyrate, butyrate and iso-valerate over the reference diet (p < 0.05; Table 7). Dietary supplementation with T. obliquus tended to increase (p = 0.061) total VFA production and increased acetate:propionate ratio (p = 0.026; Table 7). Caproate concentration decreased and increased with the inclusion of C. vulgaris and N. oceanica, respectively (Table 7). Chlorella vulgaris decreased (p < 0.001) iso-caproate concentration and tended to increase acetate:propionate ratio (p = 0.087; Table 7). Fecal ammonia-N concentration was not affected by microalgae supplementation (Table 7).
3.5. Fecal microbiota
Bacterial composition (Figure 1) was affected in studies with the supplementation of C. vulgaris (p = 0.043) and N. oceanica (p = 0.026), while in the study with T. obliquus only borderline significance was observed (p = 0.072). Differences between the reference diet and microalgae supplementation levels in the pairwise mode were significant for N. oceanica study (all p = 0.030) and borderline significant in study with C. vulgaris (all p = 0.050–0.051).
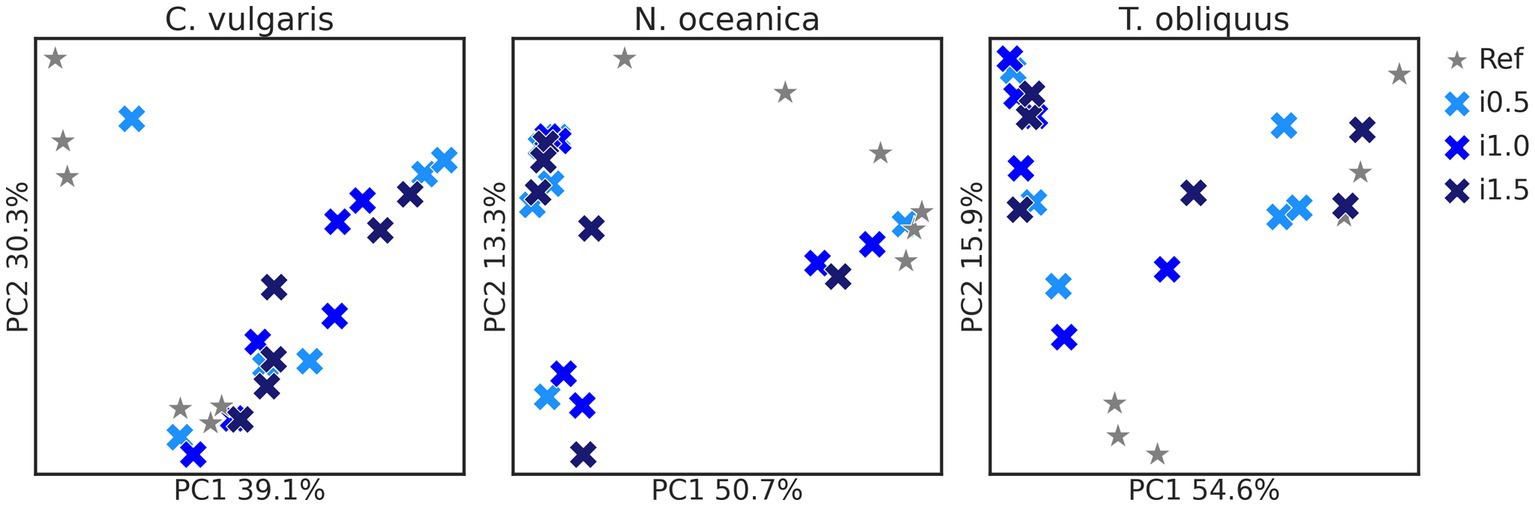
Figure 1. Principal-coordinate analysis plots of Chlorella vulgaris, Nannochloropsis oceanica, and Tetradesmus obliquus Bray–Curtis distances. Reference and supplemented diets differentiated by shapes and color and inclusion levels by color gradient.
Regarding alpha diversity, some decrease in Shannon entropy (Figure 2) index was observed in boxplots of supplemented diets for all three studies, however, when tested with Wilcoxon test for dependent samples none of those differences turned out to be significant.
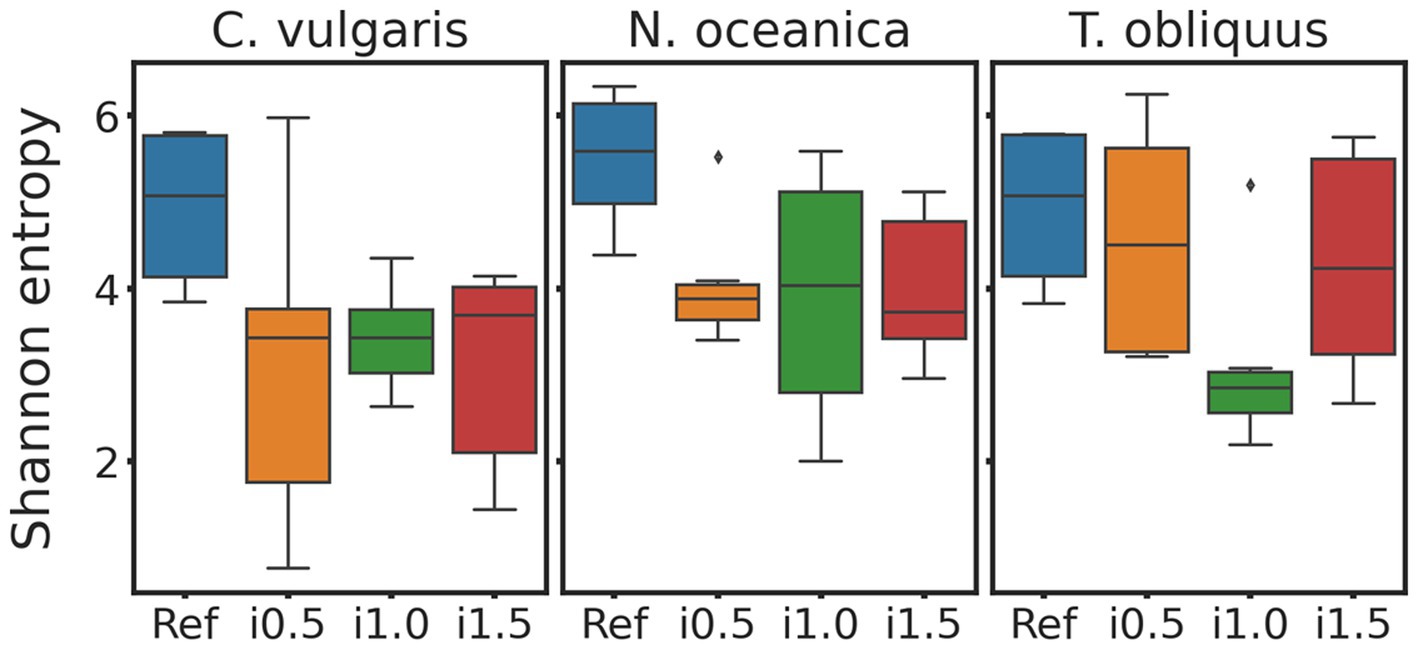
Figure 2. Boxplots of Chlorella vulgaris, Nannochloropsis oceanica, and Tetradesmus obliquus Shannon entropy. Reference and supplemented diets differentiated by color.
Among all studies, Turicibacter genus was consistently the most abundant, followed by unclassified Peptostreptococcaceae. Blautia was the third most abundant genus in N. oceanica and T. obliquus studies, but in the study with C. vulgaris supplementation, its abundance was outnumbered by Clostridium (sensu stricto 1) (Figure 3).
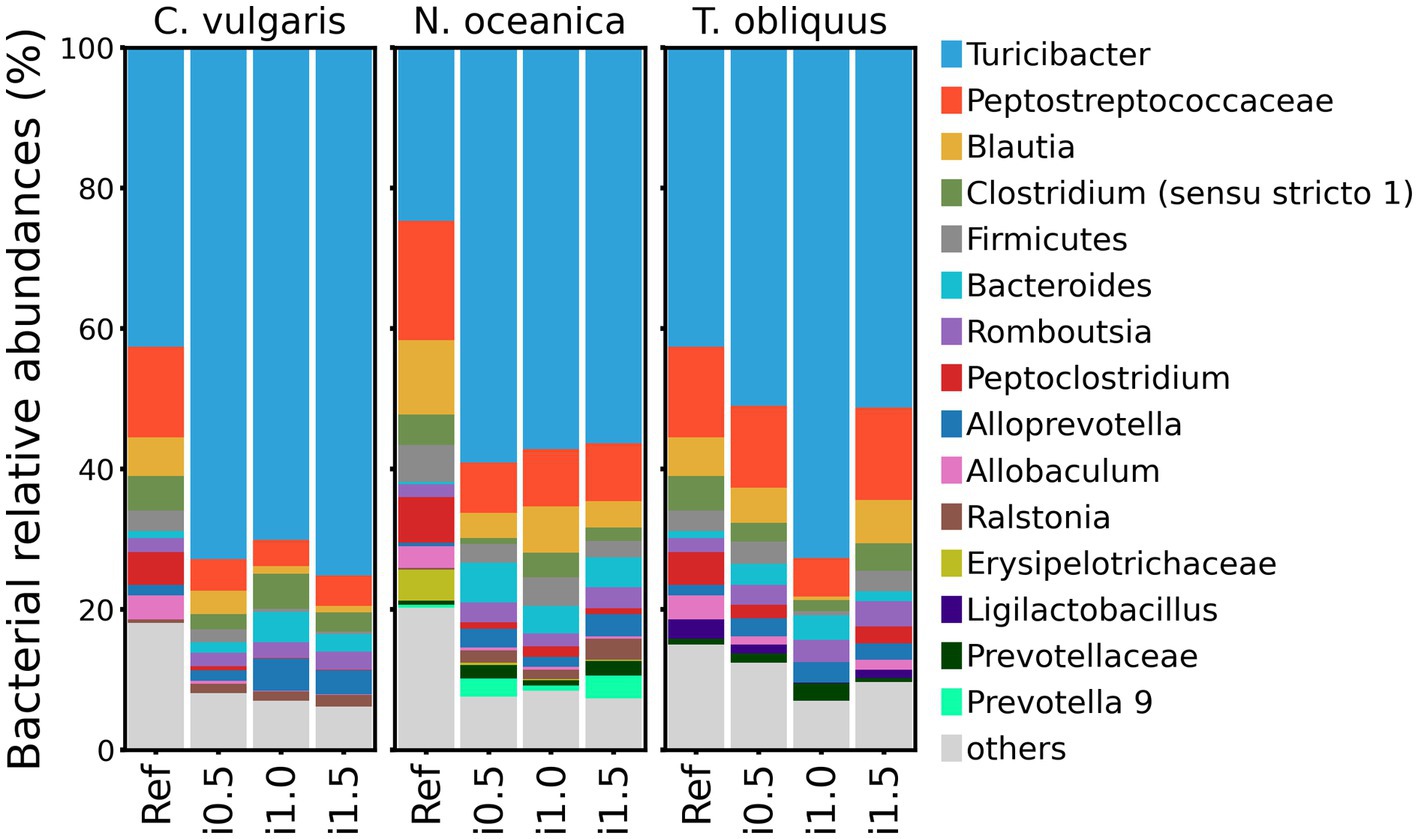
Figure 3. Taxonomy barplots of reference diet and diets with inclusion of Chlorella vulgaris, Nannochloropsis oceanica, and Tetradesmus obliquus at genus level or last available rank (if genus level was not assigned).
Supplementation of C. vulgaris resulted in increased abundances (for inclusion levels 1 and 1.5%) of Arthromitus, Bacteroides, Ralstonia, Romboutsia, and Turicibacter, while abundances of Ligilactobacillus and Dubosiella decreased (Figure 4). In a study with N. oceanica its addition mostly affected bacterial abundances at inclusion levels 0.5 and 1.5%. So, counts of Bacteroides, Ralstonia, Prevotella 9, Romboutsia, Turicibacter, Alloprevotella, Prevotellaceae, and Peptococcus were increased and such of Ruminococcus, unclassified Erysipelotrichaceae, Allobaculum, Ligilactobacillus, Bifidobacterium, Eubacterium, and Lactobacillus decreased. The effect of the T. obliquus on bacterial genera was detected only at inclusion level 1%. Abundances of Arthromitus, Bacteroides, Ralstonia, unclassified Peptostreptococcaceae, Clostridida, Romboutsia, Turicibacter, and Alloprevotella increased, while representation of Blautia, Allobaculum, Ligilactobacillus, Dubosiella, and Lactobacillus went down. However, it should be noted that the significance of those differences between reference and supplemented diets were not dependent on the inclusion level itself. No differentially abundant genera were discovered between inclusion levels in all three studies.
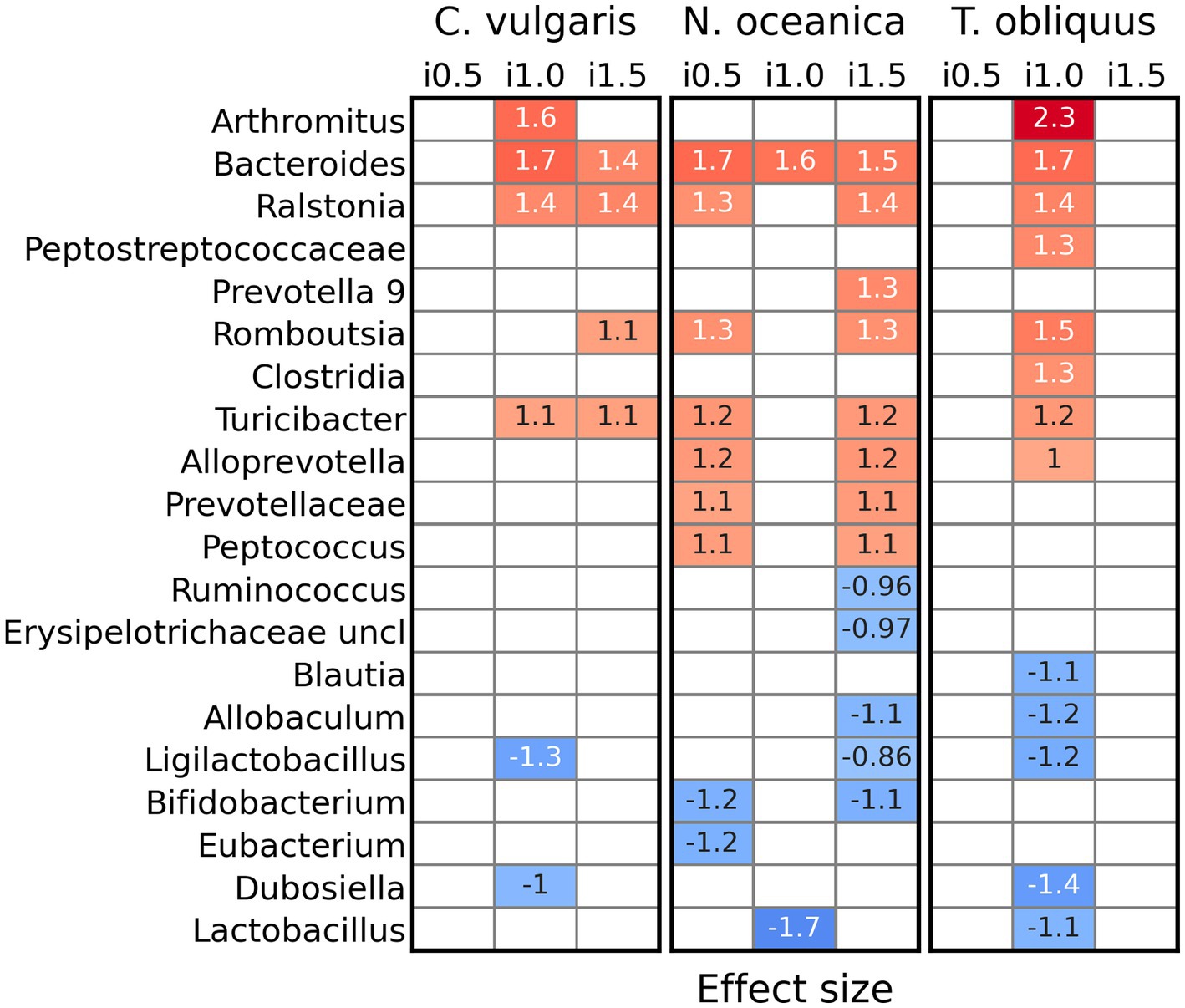
Figure 4. Differentially abundant genera (ALDEx2) between reference diet and diets with inclusion of Chlorella vulgaris, Nannochloropsis oceanica, and Tetradesmus obliquus.
4. Discussion
4.1. Palatability
Palatability is affected by several factors, such as flavor, food texture, size and shape of kibble, diet chemical composition [e.g., protein and fat contents; (47)], and intrinsic variables of the animals (48). Although first approach and taste were not significantly affected by 1.5% of dietary inclusion of the three microalgae species studied, the intake ratio was reduced with C. vulgaris and N. oceanica, with dogs preferring the reference diet. To the best of our knowledge, no studies evaluated the palatability of these microalgae species in dogs. However, Souza et al. (9) reported a positive effect of the dietary inclusion of 0.4% of microalgae Schizochytrium sp. in intake ratio and first choice, suggesting that the characteristic flavor (fishy smell) of the microalgae was responsible for the results obtained. Similarly, a recent study showed that dietary supplementation of A. platensis (0.05–0.19 g kg−1 BW) was well tolerated and accepted by dogs (7). Conversely, low palatability of microalgae of genera Chlorella and Nannochloropsis has been reported in fish and livestock (49, 50), and this lower acceptability has been associated with microalgae taste, odor, and physical structure of the dry powdery form (51). As palatability can be improved by changing the texture of the feed and adding palatants (52), the acceptability of dog diets with microalgae may be promoted if microalgae are included in the kibble, and not offered as-is as occurred in the present study.
4.2. Chemical composition
Dietary inclusion of the microalgae species studied up to 1.5% had minor effects on chemical composition of the experimental diets, that greatly reflected the composition of the reference diet and ensured the nutrient requirements established by FEDIAF (15) for adult dogs. Protein-rich microalgae species, like C. vulgaris, were proposed as an alternative protein ingredient to replace traditional human and animal feeding sources. Their high production cost and low cell wall digestibility have been overcome through the use of high yielding strains, optimization of culturing conditions, and pre-treatments of algal cells (53). In the present study, the dietary inclusion of C. vulgaris and T. obliquus suggested the potential to increase diet CP content and essential amino acids, with the only exceptions of His with T. obliquus. These results indicate that if included in higher levels in complete feeds, the microalgae studied can constitute useful protein sources to balance amino acids supply, namely lysine that is commonly the first limiting amino acid in diets including cereals and soybean (54). Additionally, the microalgae studied, particularly C. vulgaris, presented a higher content of individual essential amino acids, except histidine, than pet-food grade poultry by-product meal, a popular protein source in pet food (55).
Inclusion of N. oceanica can promote dietary lipids and eicosapentaenoic acid (EPA; C20:5 n-3) contents, decreasing the n-6/n-3 ratio. In commercial dog foods, the levels of individual fatty acids are quite variable and reflect different strategies of food producers in choosing lipid sources to ensure essential polyunsaturated fatty acids (PUFA) requirements (56). Higher levels of n-3 PUFA have been considered beneficial for animal health, namely for dogs with pruritus, renal insufficiency, and other inflammatory disorders, due to their anti-inflammatory properties (57, 58), particularly of the long-chain n-3 PUFA EPA and docosahexaenoic acid [DHA; C22:6 n-3; (59)]. Fish oil is the most common source of long-chain n-3 PUFA in commercial pet foods. However, the sustainability of this strategy is questionable as the high demand for fish oil for human and animal feeding is endangering fish stocks (60). In this context, N. oceanica can contribute to a more sustainable source of long-chain n-3 PUFA for dog feeding.
Microalgae are considered good sources of minerals. The species studied in the present study stands out for the high level of Na in N. oceanica and Fe in T. obliquus and C. vulgaris (8). Healthy dogs adjust to different dietary levels of Na through the rennin-angiotensin-aldosterone mechanisms, and no strong evidence is available on the risk of hypertension promoted by a high Na intake and on the ideal dietary Na level for dogs with cardiac deficiency (61). Moreover, increased dietary Na has been used as a dietary strategy to reduce the risk of urolithiasis (62). Storage of Fe in the organism is tightly regulated to ensure cellular needs without the development of toxicity and Fe homeostasis is controlled by the regulation of Fe efflux in the enterocytes through the hormone hepcidin (63). An excessive accumulation of Fe in hepatocytes can cause hemochromatosis, fibrosis and cirrhosis, whereas Fe deficiency can lead to anemia and other metabolic dysfunctions (64).
4.3. Food intake, fecal output and characteristics, in vivo digestibility, and metabolizable energy
Despite the commercial availability of some microalgae species, their use as ingredients in complete diets for dogs is limited, mainly by their still high production cost, being microalgae most commonly used as additives (in declared amounts lower than 0.5%) to take advantage of their functional value. From the results obtained in the palatability trials, the levels of microalgae supplementation to be studied in the digestibility trials were set at 0.5% (representing the most commonly used level in commercial foods and in scientific studies), 1.0, and 1.5%. Studies with other animal species found that a very low, economical acceptable level of dietary inclusion of microalgae biomass (0.1 to 1%) can benefit animal performance. Indeed, positive effects on mortality rate in mice (65), number of piglets alive, total weight of each litter and mortality rate prior and after weaning (66), and improved nutrient digestibility, feed intake and feed conversion in growing pigs (67) have been reported.
Microalgae supplementation up to 1.5% did not affect food intake, fecal output, digestibility of nutrients and energy and ME content, regardless of the species. Although information on digestibility and ME content of individual ingredients is valuable for diet formulation, studies on ingredient digestibility in dogs are scarce, and ME content is often estimated by chemical composition (15). To evaluate ingredient digestibility, the difference and the regression methods have been largely used in livestock (68, 69), but also applied to dogs (70, 71). The regression method requires the use of several experimental diets, to get information about the effects of different levels of inclusion, but it is more expensive. The generated equation is valid only for the range of the levels of ingredient inclusion used, whereas the difference method assumes no associative effects between the studied ingredient and the basal diet and requires a greater ingredient inclusion level to reduce estimative errors. These two methods can give conflicting results, as previous reported (72). In the present study, it was not possible to estimate digestibility of the three microalgae species studied as no linear regression was obtained between microalgae inclusion and diet digestibility, and the extrapolation to 100% microalgae inclusion provided digestibility values higher than 100%. Similarly, Kawauchi et al. (72) could not determine all nutrients digestibility by the regression and difference methods. No studies evaluating the digestibility of the three microalgae species studied were found in the literature.
Digestibility and consequent fecal output indicate diet quality and assume relevant importance to pet owners from a waste disposal perspective. The commercial complete food used as the reference diet includes cereals and animal meals as main ingredients (label information) and presented modest ATTD of OM, CP, and energy. Digestibility is known to be affected by several factors such as chemical composition, processing methods, and the physiological state of the animal, and depending on the water holding capacity, higher nutrient digestibility usually results in lower fecal output. The digestibility of microalgae, particularly in monogastric animals, is mainly constrained by their rigid cellulosic cell wall that limits the access to the cell contents. Therefore, processing methods of microalgae to disrupt the cell wall have been proposed (53), with reported benefits on growth, feed conversion (73), and digestibility (74). In the present study, dogs were fed whole microalgae without previous processing, thus, higher digestibility might be expected if disrupted microalgae are used.
Number of defecations decreased with C. vulgaris and T. obliquus inclusion over the reference diet but were not significantly affected by microalgae level. Dietary contents of soluble and insoluble fiber and ash (72, 75), have been suggested to affect frequency of defecation, but results are contradictory. Indeed, El-Wahab et al. (76) reported no differences in the frequency of defecation (1.86–2.29) between vegetarian diets with an ash content of 41.3 to 53.8 g/kg DM. Similarly, Ingenpaß et al. (77) observed no effect on frequency of defecation (2.30–2.57) between meat and vegetarian-based diets with an ash content of 62.4 and 55.1 g/kg DM, respectively. In the present study, soluble and insoluble dietary fiber was not analyzed, and dietary ash content was very similar among diets. However, the high ash content of N. oceanica might affect the frequency of defecation if higher inclusion levels are used.
Along with frequency of defecation, dog owners judge the quality of a commercial feed based on fecal quality. Compared to the reference diet, inclusion of N. oceanica increased feces DM content, and T. obliquus decreased fecal scores. Fecal DM content and fecal consistency scores are used to determine fecal consistency (78), but an association between these two parameters is not always observed (77). Increased proteolytic fermentation in the hindgut and diet supplementation with non-digestible oligosaccharides are known to decrease fecal DM content (79). Indeed, indigestible protein provide a substrate for fermentation by proteolytic bacteria, promoting the osmotic pressure, thus greater water to the intestinal lumen and reducing fecal quality (46). Similarly, end-products of fiber fermentation in the large intestine increase the osmotic pressure in the intestinal lumen. The high-water holding capacity of soluble fibers decreases fecal DM content (80). However, microalgae inclusion effects on dietary fiber digestibility and colonic fermentation products do not support differences between experimental diets on extent of protein and fiber fermentation in the large intestine.
4.3.1. Fecal pH, ammonia-N, and volatile fatty acids concentrations
Comparing to the reference diet, the dietary inclusion of the three microalgae species studied decreased fecal pH, suggesting a reduced colonic fermentation of nitrogen compounds, as it is known that the fermentation of non-digested protein produces nitrogen compounds, such as ammonia-N and branched-chain VFA that increases intestinal pH and might harm intestinal health and worse fecal odor (81). However, no effects of microalgae inclusion have been observed on fecal ammonia-N concentration and C. vulgaris and N. oceanica increased branched-chain VFA concentrations. These results suggest that the decrease in feces pH was not mainly driven by effects on the amount of indigestible protein in the colon, but rather due to total VFA production.
Dietary inclusion of all microalgae species studied increased total VFA (p = 0.061 for T. obliquus). Several factors are known to affect VFA production, such as substrate source for colonic fermentation, gastrointestinal transit time and microbiota composition. VFA comprise a source of energy for bacterial metabolism, growth of epithelial cells, and for the host animal providing up to 7% of the maintenance energy requirements of an adult dog (82). Additionally, VFA regulates luminal pH and mucus secretion and provides some health-promoting effects, namely anti-inflammatory, immunomodulatory, and anticarcinogenic (83).
Acetate, propionate and butyrate are the most abundant VFA in dog feces, comprising approximately 60, 25, and 10% of the total VFA (84). Fecal acetate concentration increased with the dietary inclusion of all microalgae studied and C. vulgaris and N. oceanica inclusion also increased the concentrations of the other measured VFA except for valerate and iso-caproate (for N. oceanica). Acetate is the most abundant VFA in the colon and most bacteria produce acetate from carbohydrate fermentation. It is mainly metabolized by peripheral tissues, thus, high concentrations reach the systemic circulation, and depending on the tissues is involved in the citric acid cycle or fatty acid synthesis (85). Similarly to acetate, the majority of the propionate produced enters the portal circulation and is metabolized in the liver. Propionate is used for glucose synthesis, contributing to a reduction of blood sugar and serum cholesterol, and exerts anti-inflammatory effects in the intestine, decreasing the production of pro-inflammatory cytokines such as IL-6, IL-8, and TNFα (86). As fecal concentration of propionate is decreased in dogs with gastrointestinal diseases, it comprises a biomarker of gut functionality in dogs (87). Only small amounts (<10%) of butyrate reaches the portal circulation, being butyrate the main energy source for colonocytes, also plays a role in maintaining cell growth and differentiation in the gut, preventing colonic cancer and reducing inflammation (85).
Branched-chain VFA represent minor components of the VFA and result from the bacterial degradation of the branched-chain amino acids valine, leucine and isoleucine (88). The increase of branched-chain VFA observed in the present study with the dietary inclusion of C. vulgaris and N. oceanica suggests an increased proteolytic activity of some bacterial populations.
4.4. Fecal microbiota
It is well known that diet can exert a strong influence on gastrointestinal health, fecal microbiota, and fecal metabolite concentrations (89, 90). Most studies comprise a dietary period of 2 to 4 weeks before sampling feces to allow the stabilization of the microbial community and activity (91). However, the longitudinal changes promoted by a dietary change in gut microbial phylogeny and function, and metabolite profiles have not been well studied in dogs. Recently, Lin et al. (92) studied the kinetics required by a dietary change from a control diet to a fiber supplemented diet or a protein-rich canned diet to modify the fecal microbiome and metabolites in healthy adult Beagle dogs and found that fecal microbial diversity, composition and function as well as metabolite profiles (pH, VFA, ammonia-N) dramatically changed and stabilized within a few days (2 d for metabolites and 6 d for microbiota) after dietary changes. In the present study, dogs were fed each diet for 10 days, and unlike previous research (93), microalgae supplementation affected the microbial composition of canine feces. Thus, abundances of Turicibacter and Peptococcus genera, which were shown to be correlated with butyrate (94, 95), increased in the feces of dogs on microalgae-supplemented diets. A similar enhancement of Turicibacter abundances in response to algae supplementation was reported in rats (96). Our study observed that dogs fed on diets with microalgae supplementation decreased the number of defecations. In a comparative study of healthy dogs and dogs with chronic diarrhea, Turicibacter abundances were shown to be correlated with the healthy group, suggesting its protective role in gut health (97, 98). Other genera, which abundances increased in dogs fed microalgae-supplemented diets were Bacteroides, Peptococcus, Romboutsia, Prevotella 9, Alloprevotella, Arthromitus, and some unassigned to genus level Prevotellaceae, Clostridia, and Peptostreptococcaceae. Members of those taxa are often associated with elevated VFA content (99–102), which agrees with the observed increased amount of total VFA production in feces of dogs fed with microalgae-supplemented diets. Moreover, Bacteroides and Prevotella spp. are involved in the degradation of complex plant polysaccharides (103–105) and their increased content may be a direct consequence of algae supplementation. Bacteroides ability to utilize urea as a nitrogen source (106) also corresponds with lower feces pH under supplemented diets. Arthromitus spp. are not only associated with VFA production, but also related to the activation of the immune system (107). Peptococcus abundances were reported to be positively correlated with fecal butyrate and likewise associated with dogs’ gut health (95). More genera whose growth was stimulated by algae supplementation, Romboutsia and Alloprevotella, were shown to play an important role in dogs’s health via higher carbohydrate utilization (90, 108) and improvements in body-weight regulation (109), respectively. Interestingly, some bacterial taxa that were in lower abundance with algae supplementation, such as Blautia, Allobaculum, and Ruminococcus were previously reported to be essential players in dogs weight regulation (110, 111). In our study, Turicibacter genus average abundances prevailed in all diets, including the reference. However, as is shown in the Supplementary Figure S1, feces microbiome varied between different dogs, and despite the average dominance, the Turicibacter genus was not the most abundant one in some dogs, supporting interindividual variability (112) between even adult dogs. Relatively high abundances of the Turicibacter genus in the reference diet compared to the other studies (113, 114) suggest that in our study either housing conditions or targeted 16S rRNA region (V1–V2) were responsible for the Turicibacter dominance.
5. Conclusion
The present study shows that dietary inclusion of C. vulgaris, N. oceanica, and T. obliquus up to 1.5% had no negative effect on chemical composition, food intake and digestibility of diets, but the acceptability of diets for dogs with microalgae can be favored if the microalgae are included in the kibbles. In addition, feeding dogs with microalgae-supplemented diets affected fecal characteristics and altered microbiota composition towards the promotion of Turicibacter and Peptococcus genera associated with gut health and activation of the immune system. Overall, the results support the potential of T. obliquus, C. vulgaris, and N. oceanica as sustainable functional additives for dog feeding.
Data availability statement
The datasets presented in this study can be found in online repositories. The names of the repository/repositories and accession number(s) can be found below: [https://www.ebi.ac.uk/ena AND PRJEB61064].
Ethics statement
The animal study was approved by Animal Ethics Committee of School of Medicine and Biomedical Sciences, University of Porto. The study was conducted in accordance with the local legislation and institutional requirements.
Author contributions
AC designed the experiment, analyzed the data, obtained funding, drafted the original manuscript, and elaborated the final version of the manuscript. JG-F performed the experimental protocols. MS performed the experimental protocols. MM designed the experiment, participated in the data analysis, and critically revised the manuscript. TY performed the microbiota analysis and drafted the original manuscript. AC-S performed the microbiota analysis and critically revised the manuscript. AF designed the experiment, obtained funding, critically revised the manuscript, and elaborated the final version of the manuscript. All authors contributed to the article and approved the submitted version.
Funding
This research was funded by the Portuguese Foundation for Science and Technology (FCT/MCTES) through projects UIDB/50006/2020 and UIDP/50006/2020, and from projects NovInDog (POCI-01-0247-FEDER-047003) and AlgaValor (POCI-01-0247-FEDER-035234; Lisboa-01-0247-FEDER-035234; ALG-01-0247-FEDER-035234) supported by Portugal 2020 program through the European Regional Development Fund. JG-F was funded by FCT and Soja de Portugal (PD/BDE/150527/2019), and MRGM by FCT through program DL 57/2016 – Norma transitória (SFRH/BPD/70176/2010).
Acknowledgments
The authors thank Sílvia Azevedo and Cátia Mota from the School of Medicine and Biomedical Sciences, University of Porto, and Agostinho Almeida from the Faculty of Pharmacy, University of Porto, and Tanja Sims from the University of Hohenheim for the valuable technical assistance in analytical determinations. Authors also acknowledge the staff from the kennel of the School of Medicine and Biomedical Sciences, University of Porto, for their assistance on the daily management of the dogs. We also acknowledge the support of the High Performance and Cloud Computing Group at the Zentrum für Datenverarbeitung of the University of Tübingen, the state of Baden-Württemberg through bwHPC, and the German Research Foundation (DFG) through grant no. INST 37/935-1FUGG.
Conflict of interest
The authors declare that the research was conducted in the absence of any commercial or financial relationships that could be construed as a potential conflict of interest.
Publisher’s note
All claims expressed in this article are solely those of the authors and do not necessarily represent those of their affiliated organizations, or those of the publisher, the editors and the reviewers. Any product that may be evaluated in this article, or claim that may be made by its manufacturer, is not guaranteed or endorsed by the publisher.
Supplementary material
The Supplementary material for this article can be found online at: https://www.frontiersin.org/articles/10.3389/fvets.2023.1245790/full#supplementary-material
Footnotes
References
1. Valente, LMP, Cabrita, ARJ, Maia, MRG, Valente, IM, Engrola, S, Fonseca, AJM, et al. Microalgae as feed ingredients for livestock production and aquaculture. In: Galanakis CM, editor. Microalgae. Cambridge, MA: Academic Press. (2021). p. 239–312
2. Chang, H, Zou, Y, Hu, R, Feng, H, Wu, H, Zhong, N, et al. Membrane applications for microbial energy conversion: a review. Environ Chem Lett. (2020) 18:1581–92. doi: 10.1007/s10311-020-01032-7
3. Araújo, R, Calderón, F, López, J, Azevedo, I, Bruhn, A, Fluch, S, et al. Current status of the algae production industry in Europe: an emerging sector of the blue bioeconomy. Front Mar Sci. (2021) 7:626389. doi: 10.3389/fmars.2020.626389
4. FMI, Microalgae market size, share & forecast report by 2033, Food and beverages. (2023), Newark, DE: Future Market Insights. 342.
5. Satyaraj, E, Reynolds, A, Engler, R, Labuda, J, and Sun, P. Supplementation of diets with Spirulina influences immune and gut function in dogs. Front Nutr. (2021) 8:667072. doi: 10.3389/fnut.2021.667072
6. Lauritano, C, Andersen, JH, Hansen, E, Albrigtsen, M, Escalera, L, Esposito, F, et al. Bioactivity screening of microalgae for antioxidant, anti-inflammatory, anticancer, anti-diabetes, and antibacterial activities. Front Mar Sci. (2016) 3:3. doi: 10.3389/fmars.2016.00068
7. Stefanutti, D, Tonin, G, Morelli, G, Zampieri, RM, La Rocca, N, and Ricci, R. Oral palatability and owners’ perception of the effect of increasing amounts of Spirulina (Arthrospira platensis) in the diet of a cohort of healthy dogs and cats. Animals. (2023) 13:1275. doi: 10.3390/ani13081275
8. Cabrita, ARJ, Guilherme-Fernandes, J, Valente, IM, Almeida, A, Lima, SAC, Fonseca, AJM, et al. Nutritional composition and untargeted metabolomics reveal the potential of Tetradesmus obliquus, Chlorella vulgaris and Nannochloropsis oceanica as valuable nutrient sources for dogs. Animals. (2022) 12:2643. doi: 10.3390/ani12192643
9. Souza, CMM, de Lima, DC, Bastos, TS, de Oliveira, SG, Beirão, BCB, and Félix, AP. Microalgae Schizochytrium sp. as a source of docosahexaenoic acid (DHA): effects on diet digestibility, oxidation and palatability and on immunity and inflammatory indices in dogs. Anim Sci J. (2019) 90:1567–74. doi: 10.1111/asj.13294
10. Hadley, KB, Bauer, J, and Milgram, NW. The oil-rich alga Schizochytrium sp. as a dietary source of docosahexaenoic acid improves shape discrimination learning associated with visual processing in a canine model of senescence. Prostaglandins Leukot Essent Fat Acids. (2017) 118:10–8. doi: 10.1016/j.plefa.2017.01.011
11. Zhang, HQ, Lin, AP, Sun, Y, and Deng, YM. Chemo- and radio-protective effects of polysaccharide of Spirulina platensis on hemopoietic system of mice and dogs. Acta Pharmacol Sin. (2001) 22:1121–4.
12. Berk, BA, Packer, RM-A, Fritz, J, and Volk, HA. Oral palatability testing of a medium-chain triglyceride oil supplement (MCT) in a cohort of healthy dogs in a non-clinical setting. Animals. (2022) 12:1639. doi: 10.3390/ani12131639
13. Patel, AK, Singhania, RR, Awasthi, MK, Varjani, S, Bhatia, SK, Tsai, M-L, et al. Emerging prospects of macro- and microalgae as prebiotic. Microb Cell Factories. (2021) 20:112. doi: 10.1186/s12934-021-01601-7
14. Delsante, C, Pinna, C, Sportelli, F, Dalmonte, T, Stefanelli, C, Vecchiato, CG, et al. Assessment of the effects of edible microalgae in a canine gut model. Animals. (2022) 12:2100. doi: 10.3390/ani12162100
15. FEDIAF. Nutritional guidelines for complete and complementary pet food for cats and dogs. Belgium: Bruxelles (2021).
16. Aldrich, GC, and Koppel, K. Pet food palatability evaluation: a review of standard assay techniques and interpretation of results with a primary focus on limitations. Animals Open Access J MDPI. (2015) 5:43–55. doi: 10.3390/ani5010043
17. Laflamme, D. Development and validation of a body condition score system for dogs. Canine Pract. (1997) 22:10–5.
18. Carciofi, AC, De-Oliveira, LD, Valério, AG, Borges, LL, de Carvalho, FM, Brunetto, MA, et al. Comparison of micronized whole soybeans to common protein sources in dry dog and cat diets. Anim Feed Sci Technol. (2009) 151:251–60. doi: 10.1016/j.anifeedsci.2009.01.002
19. AOAC In: W Horwitz, editor. Official methods of analysis of AOAC international. Gaithersburg, MD: AOAC International (2000)
20. Van Soest, PJ, Robertson, JB, and Lewis, BA. Methods for dietary fiber, neutral detergent fiber, and nonstarch polysaccharides in relation to animal nutrition. J Dairy Sci. (1991) 74:3583–97. doi: 10.3168/jds.S0022-0302(91)78551-2
21. Robertson, J., and Van Soest, P., The detergent system of analysis, The analysis of dietary fiber in food, W. James and O. Teander, (Eds.) (1981), Marcel Dekker Inc.: New York. p. 123–158.
22. Salomonsson, AC, Theander, O, and Westerlund, E. Chemical characterization of some Swedish cereal whole meal and bran fractions. Swed J Agric Res. (1984) 14:111–7.
23. Aragão, C, Cabano, M, Colen, R, Fuentes, J, and Dias, J. Alternative formulations for gilthead seabream diets: towards a more sustainable production. Aquac Nutr. (2020) 26:444–55. doi: 10.1111/anu.13007
24. Lepage, G, and Roy, CC. Improved recovery of fatty acid through direct transesterification without prior extraction or purification. J Lipid Res. (1984) 25:1391–6. doi: 10.1016/S0022-2275(20)34457-6
25. Maia, MRG, Fonseca, AJM, Cortez, PP, and Cabrita, ARJ. In vitro evaluation of macroalgae as unconventional ingredients in ruminant animal feeds. Algal Res. (2019) 40:101481. doi: 10.1016/j.algal.2019.101481
26. Cabrita, ARJ, Maia, MRG, Oliveira, HM, Sousa-Pinto, I, Almeida, AA, Pinto, E, et al. Tracing seaweeds as mineral sources for farm-animals. J Appl Phycol. (2016) 28:3135–50. doi: 10.1007/s10811-016-0839-y
27. Smith, F, and Murphy, T. Analysis of Rumen Ammonia & Blood Urea Nitrogen. Nebraska, NE: L. Univ (2013).
28. Pereira, AM, Maia, MRG, Pinna, C, Biagi, G, Matos, E, Segundo, MA, et al. Effects of zinc source and enzyme addition on the fecal microbiota of dogs. Front Microbiol. (2021) 12:688392. doi: 10.3389/fmicb.2021.688392
29. Kaewtapee, C, Burbach, K, Tomforde, G, Hartinger, T, Camarinha-Silva, A, Heinritz, S, et al. Effect of Bacillus subtilis and Bacillus licheniformis supplementation in diets with low- and high-protein content on ileal crude protein and amino acid digestibility and intestinal microbiota composition of growing pigs. J Anim Sci Biotechnol. (2017) 8:37. doi: 10.1186/s40104-017-0168-2
30. Bolyen, E, Rideout, JR, Dillon, MR, Bokulich, NA, Abnet, CC, Al-Ghalith, GA, et al. Reproducible, interactive, scalable and extensible microbiome data science using QIIME 2. Nat Biotechnol. (2019) 37:852–7. doi: 10.1038/s41587-019-0209-9
31. Martin, M. Cutadapt removes adapter sequences from high-throughput sequencing reads. EMBnet J. (2011) 17:3. doi: 10.14806/ej.17.1.200,
32. Callahan, BJ, McMurdie, PJ, Rosen, MJ, Han, AW, Johnson, AJA, et al. DADA2: high-resolution sample inference from Illumina amplicon data. Nat Methods. (2016) 13:581–3. doi: 10.1038/nmeth.3869
33. Rognes, T, Flouri, T, Nichols, B, Quince, C, and Mahé, F. VSEARCH: a versatile open source tool for metagenomics. PeerJ. (2016) 4:e2584. doi: 10.7717/peerj.2584
34. Pedregosa, F, Varoquaux, G, Gramfort, A, Michel, V, Thirion, B, Grisel, O, et al. Scikit-learn: machine learning in Python. J Mach Learn Res. (2011) 12:2825–30. doi: 10.48550/arXiv.1201.0490
35. Quast, C, Pruesse, E, Yilmaz, P, Gerken, J, Schweer, T, Yarza, P, et al. The SILVA ribosomal RNA gene database project: improved data processing and web-based tools. Nucleic Acids Res. (2013) 41:D590–6. doi: 10.1093/nar/gks1219
36. Robeson, MS, O’Rourke, DR, Kaehler, BD, Ziemski, M, Dillon, MR, Foster, JT, et al. RESCRIPt: Reproducible sequence taxonomy reference database management. PLoS Comput Biol. (2021) 17:e1009581. doi: 10.1371/journal.pcbi.1009581
37. Katoh, K, and Standley, DM. MAFFT multiple sequence alignment software version 7: improvements in performance and usability. Mol Biol Evol. (2013) 30:772–80. doi: 10.1093/molbev/mst010
38. Price, MN, Dehal, PS, and Arkin, AP. FastTree 2 – approximately maximum-likelihood trees for large alignments. PLoS One. (2010) 5:e9490. doi: 10.1371/journal.pone.0009490
39. Shannon, CE. A mathematical theory of communication. Bell Syst Tech J. (1948) 27:379–423. doi: 10.1002/j.1538-7305.1948.tb01338.x
40. Bray, JR, and Curtis, JT. An ordination of the upland forest communities of southern Wisconsin. Ecol Monogr. (1957) 27:325–49. doi: 10.2307/1942268
41. Halko, N, Martinsson, P-G, Shkolnisky, Y, and Tygert, M. An algorithm for the principal component analysis of large data sets. SIAM J Sci Comput. (2011) 33:2580–94. doi: 10.1137/100804139
42. Wilcoxon, F. Individual comparisons by ranking methods. Biom Bull. (1945) 1:80. doi: 10.2307/3001968
43. Anderson, MJ. A new method for non-parametric multivariate analysis of variance. Austral Ecol. (2001) 26:32–46. doi: 10.1111/j.1442-9993.2001.01070.pp.x
44. Fernandes, AD, Macklaim, JM, Linn, TG, Reid, G, and Gloor, GB. ANOVA-like differential expression (ALDEx) analysis for mixed population RNA-Seq. PLoS One. (2013) 8:e67019. doi: 10.1371/journal.pone.0067019
45. Benjamini, Y, and Hochberg, Y. Controlling the false discovery rate: a practical and powerful approach to multiple testing. J R Stat Soc B. (1995) 57:289–300. doi: 10.1111/j.2517-6161.1995.tb02031.x
46. Hall, JA, Melendez, LD, and Jewell, DE. Using gross energy improves metabolizable energy predictive equations for pet foods whereas undigested protein and fiber content predict stool quality. PLoS One. (2013) 8:e54405. doi: 10.1371/journal.pone.0054405
47. Case, LP, Daristotle, L, Hayek, MG, and Raasch, M. Canine and feline nutrition: A resource for companion animal professionals. Philadelphia, PA: Elsevier Health Sciences (2010).
48. Hall, NJ, Péron, F, Cambou, S, Callejon, L, and Wynne, CD. Food and food-odor preferences in dogs: a pilot study. Chem Senses. (2017) 42:361–70. doi: 10.1093/chemse/bjx016
49. Lamminen, M, Halmemies-Beauchet-Filleau, A, Kokkonen, T, Jaakkola, S, and Vanhatalo, A. Different microalgae species as a substitutive protein feed for soya bean meal in grass silage based dairy cow diets. Anim Feed Sci Technol. (2019) 247:112–26. doi: 10.1016/j.anifeedsci.2018.11.005
50. Walker, AB, and Berlinsky, DL. Effects of partial replacement of fish meal protein by microalgae on growth, feed intake, and body composition of Atlantic cod. N Am J Aquac. (2011) 73:76–83. doi: 10.1080/15222055.2010.549030
51. Kholif, AE, and Olafadehan, OA. Chlorella vulgaris microalgae in ruminant nutrition: a review of the chemical composition and nutritive value. Ann Anim Sci. (2021) 21:789–806. doi: 10.2478/aoas-2020-0117
52. Nagappan, S, Das, P, AbdulQuadir, M, Thaher, M, Khan, S, Mahata, C, et al. Potential of microalgae as a sustainable feed ingredient for aquaculture. J Biotechnol. (2021) 341:1–20. doi: 10.1016/j.jbiotec.2021.09.003
53. Machado, L, Carvalho, G, and Pereira, RN. Effects of innovative processing methods on microalgae cell wall: prospects towards digestibility of protein-rich biomass. Biomass. (2022) 2:80–102. doi: 10.3390/biomass2020006
54. Donadelli, RA, Aldrich, CG, Jones, CK, and Beyer, RS. The amino acid composition and protein quality of various egg, poultry meal by-products, and vegetable proteins used in the production of dog and cat diets. Poult Sci. (2019) 98:1371–8. doi: 10.3382/ps/pey462
55. Dozier, WA, Dale, NM, and Dove, CR. Nutrient compostion of feed-grade and pet-food-grade poultry by-product meal. J Appl Poult Res. (2003) 12:526–30. doi: 10.1093/japr/12.4.526
56. Ahlstrøm, Ø, Krogdahl, AS, Vhile, SG, and Skrede, A. Fatty acid composition in commercial dog foods. J Nutr. (2004) 134:2145S–7S. doi: 10.1093/jn/134.8.2145S
57. Brown, SA, Brown, CA, Crowell, WA, Barsanti, JA, Allen, T, Cowell, C, et al. Beneficial effects of chronic administration of dietary ω-3 polyunsaturated fatty acids in dogs with renal insufficiency. J Lab Clin Med. (1998) 131:447–55. doi: 10.1016/S0022-2143(98)90146-9
58. Watson, TDG. Diet and skin disease in dogs and cats. J Nutr. (1998) 128:S2783–9. doi: 10.1093/jn/128.12.2783S
59. Stoeckel, K, Nielsen, LH, Fuhrmann, H, and Bachmann, L. Fatty acid patterns of dog erythrocyte membranes after feeding of a fish-oil based DHA-rich supplement with a base diet low in n-3 fatty acids versus a diet containing added n-3 fatty acids. Acta Vet Scand. (2011) 53:57. doi: 10.1186/1751-0147-53-57
60. Sargent, JR, and Tacon, AG. Development of farmed fish: a nutritionally necessary alternative to meat. Proc Nutr Soc. (1999) 58:377–83. doi: 10.1017/S0029665199001366
61. Chandler, ML. Pet food safety: sodium in pet foods. Top Companion Anim Med. (2008) 23:148–53. doi: 10.1053/j.tcam.2008.04.008
62. Queau, Y, Bijsmans, ES, Feugier, A, and Biourge, VC. Increasing dietary sodium chloride promotes urine dilution and decreases struvite and calcium oxalate relative supersaturation in healthy dogs and cats. J Anim Physiol Anim Nutr. (2020) 104:1524–30. doi: 10.1111/jpn.13329
64. Grindem, CB. Schalm’s veterinary hematology, 6th edition. Editors: Douglas J. Weiss, K. Jane Wardrop. Vet Clin Pathol. (2011) 40:270–18. doi: 10.1111/j.1939-165X.2011.00324.x
65. Janczyk, P, Langhammer, M, Renne, U, Guiard, V, and Souffrant, W. Effect of feed supplementation with Chlorella vulgaris powder on mice reproduction. Arch Zootech. (2006) 9:122–34.
66. Köhler, P, Storandt, R, and Pulz, O. Study on influence of algal supplementation on both reproduction performance of sows and parameters of piglet breeding. Acta Agronom Óváriensis. (2008) 50:53–66.
67. Yan, L, Lim, S, and Kim, I. Effect of fermented chlorella supplementation on growth performance, nutrient digestibility, blood characteristics, fecal microbial and fecal noxious gas content in growing pigs. Asian Australas J Anim Sci. (2012) 25:1742–7. doi: 10.5713/ajas.2012.12352
68. Fang, REA, Yin, Y, Wang, K, He, J, Chen, Q, Li, T, et al. Comparison of the regression analysis technique and the substitution method for the determination of true phosphorus digestibility and faecal endogenous phosphorus losses associated with feed ingredients for growing pigs. Livest Sci. (2007) 109:251–4. doi: 10.1016/j.livsci.2007.01.108
69. Fan, M, and Sauer, W. Determination of apparent ileal amino acid digestibility in barley and canola meal for pigs with the direct, difference, and regression methods. J Anim Sci. (1995) 73:2364–74. doi: 10.2527/1995.7382364x
70. Carciofi, A, Sakomura, N, Kawauchi, I, and Vasconcellos, R. Digestibility and metabolizable energy of some carbohydrate sources for dogs. Anim Feed Sci Technol. (2010) 156:121–5. doi: 10.1016/j.anifeedsci.2010.01.009
71. Félix, AP, Menezes Souza, CM, Bastos, TS, Kaelle, GCB, Oliveira, SG, and Maiorka, A. Digestibility of raw soybeans in extruded diets for dogs determined by different methods. Ital J Anim Sci. (2020) 19:95–102. doi: 10.1080/1828051X.2019.1698324
72. Kawauchi, IM, Sakomura, NK, Vasconcellos, RS, De-Oliveira, LD, Gomes, MOS, et al. Digestibility and metabolizable energy of maize gluten feed for dogs as measured by two different techniques. Anim Feed Sci Technol. (2011) 169:96–103. doi: 10.1016/j.anifeedsci.2011.05.005
73. Agboola, JO, Teuling, E, Wierenga, PA, Gruppen, H, and Schrama, JW. Cell wall disruption: an effective strategy to improve the nutritive quality of microalgae in African catfish (Clarias gariepinus). Aquac Nutr. (2019) 25:783–97. doi: 10.1111/anu.12896
74. Teuling, E, Wierenga, PA, Agboola, JO, Gruppen, H, and Schrama, JW. Cell wall disruption increases bioavailability of Nannochloropsis gaditana nutrients for juvenile Nile tilapia (Oreochromis niloticus). Aquaculture. (2019) 499:269–82. doi: 10.1016/j.aquaculture.2018.09.047
75. Fahey, G Jr, Merchen, N, Corbin, J, Hamilton, A, Serbe, K, Lewis, S, et al. Dietary fiber for dogs: I. effects of graded levels of dietary beet pulp on nutrient intake, digestibility, metabolizable energy and digesta mean retention time. J Anim Sci. (1990) 68:4221–8. doi: 10.2527/1990.68124221x
76. El-Wahab, AA, Wilke, V, Grone, R, and Visscher, C. Nutrient digestibility of a vegetarian diet with or without the supplementation of feather meal and either corn meal, fermented Rye or Rye and its effect on fecal quality in dogs. Animals (Basel). (2021) 11:20496. doi: 10.3390/ani11020496
77. Ingenpaß, L, Abd El-Wahab, A, Ullrich, C, Kölln, M, Ahmed, MFE, Visscher, C, et al. Nitrogen output in the urban environment using a vegetarian canine diet. PLoS One. (2021) 16:e0257364. doi: 10.1371/journal.pone.0257364
78. Sunvold, GD, Fahey, GC, Merchen, NR, Titgemeyer, EC, Bourquin, LD, et al. Dietary fiber for dogs: IV. In vitro fermentation of selected fiber sources by dog fecal inoculum and in vivo digestion and metabolism of fiber-supplemented diets. J Anim Sci. (1995) 73:1099–109. doi: 10.2527/1995.7341099x
79. Propst, EL, Flickinger, E, Bauer, L, Merchen, N, and Fahey, G Jr. A dose-response experiment evaluating the effects of oligofructose and inulin on nutrient digestibility, stool quality, and fecal protein catabolites in healthy adult dogs. J Anim Sci. (2003) 81:3057–66. doi: 10.2527/2003.81123057x
80. Silvio, J, Harmon, DL, Gross, KL, and McLeod, KR. Influence of fiber fermentability on nutrient digestion in the dog. Nutrition. (2000) 16:289–95. doi: 10.1016/S0899-9007(99)00298-1
81. Windey, K, De Preter, V, and Verbeke, K. Relevance of protein fermentation to gut health. Mol Nutr Food Res. (2012) 56:184–96. doi: 10.1002/mnfr.201100542
82. Herschel, DA, Argenzio, RA, Southworth, M, and Stevens, CE. Absorption of volatile fatty acid, Na, and H2O by the colon of the dog. Am J Vet Res. (1981) 42:1118–24.
83. Fattahi, Y, Heidari, HR, and Khosroushahi, AY. Review of short-chain fatty acids effects on the immune system and cancer. Food Biosci. (2020) 38:100793. doi: 10.1016/j.fbio.2020.100793
84. Sunvold, G, Hussein, H, Fahey, G, Merchen, N, and Reinhart, G. In vitro fermentation of cellulose, beet pulp, citrus pulp, and citrus pectin using fecal inoculum from cats, dogs, horses, humans, and pigs and ruminal fluid from cattle. J Anim Sci. (1995) 73:3639–48. doi: 10.2527/1995.73123639x
85. Binder, HJ. Role of colonic short-chain fatty acid transport in diarrhea. Annu Rev Physiol. (2010) 72:297–313. doi: 10.1146/annurev-physiol-021909-135817
86. Vinolo, MA, Rodrigues, HG, Hatanaka, E, Sato, FT, Sampaio, SC, and Curi, R. Suppressive effect of short-chain fatty acids on production of proinflammatory mediators by neutrophils. J Nutr Biochem. (2011) 22:849–55. doi: 10.1016/j.jnutbio.2010.07.009
87. Felix, AP, Souza, CMM, and de Oliveira, SG. Biomarkers of gastrointestinal functionality in dogs: a systematic review and meta-analysis. Anim Feed Sci Technol. (2022) 283:115183. doi: 10.1016/j.anifeedsci.2021.115183
88. Nery, J, Goudez, R, Biourge, V, Tournier, C, Leray, V, Martin, L, et al. Influence of dietary protein content and source on colonic fermentative activity in dogs differing in body size and digestive tolerance1. J Anim Sci. (2012) 90:2570–80. doi: 10.2527/jas.2011-4112
89. Pereira, AM, Pinna, C, Biagi, G, Stefanelli, C, Maia, MRG, Matos, E, et al. Supplemental selenium source on gut health: insights on fecal microbiome and fermentation products of growing puppies. FEMS Microbiol Ecol. (2020) 96:FIAA212. doi: 10.1093/femsec/fiaa212
90. Xu, J, Becker, A, Luo, Y, Zhang, W, Ge, B, Leng, C, et al. The fecal microbiota of dogs switching to a raw diet only partially converges to that of wolves. Front Microbiol. (2021) 12:701439. doi: 10.3389/fmicb.2021.701439
91. Jarett, JK, Kingsbury, DD, Dahlhausen, KE, and Ganz, HH. Best practices for microbiome study design in companion animal research. Front Vet Sci. (2021) 8:644836. doi: 10.3389/fvets.2021.644836
92. Lin, CY, Jha, AR, Oba, PM, Yotis, SM, Shmalberg, J, Honaker, RW, et al. Longitudinal fecal microbiome and metabolite data demonstrate rapid shifts and subsequent stabilization after an abrupt dietary change in healthy adult dogs. Anim Microbiome. (2022) 4:46. doi: 10.1186/s42523-022-00194-9
93. Pinna, C, Vecchiato, CG, Grandi, M, Stefanelli, C, Zannoni, A, and Biagi, G. Seaweed supplementation failed to affect fecal microbiota and metabolome as well as fecal Iga and apparent nutrient digestibility in adult dogs. Animals Open Access J MDPI. (2021) 11:2234. doi: 10.3390/ani11082234
94. Zhong, Y, Nyman, M, and Fåk, F. Modulation of gut microbiota in rats fed high-fat diets by processing whole-grain barley to barley malt. Mol Nutr Food Res. (2015) 59:2066–76. doi: 10.1002/mnfr.201500187
95. Sandri, M, Dal Monego, S, Conte, G, Sgorlon, S, and Stefanon, B. Raw meat based diet influences faecal microbiome and end products of fermentation in healthy dogs. BMC Vet Res. (2017) 13:65. doi: 10.1186/s12917-017-0981-z
96. Wan, X-Z, Ai, C, Chen, Y-H, Gao, X-X, Zhong, R-T, Liu, B, et al. Physicochemical characterization of a polysaccharide from green microalga Chlorella pyrenoidosa and its hypolipidemic activity via gut microbiota regulation in rats. J Agric Food Chem. (2020) 68:1186–97. doi: 10.1021/acs.jafc.9b06282
97. Suchodolski, JS, Markel, ME, Garcia-Mazcorro, JF, Unterer, S, Heilmann, RM, Dowd, SE, et al. The fecal microbiome in dogs with acute diarrhea and idiopathic inflammatory bowel disease. PLoS One. (2012) 7:e51907. doi: 10.1371/journal.pone.0051907
98. Pilla, R, and Suchodolski, JS. The role of the canine gut microbiome and metabolome in health and gastrointestinal disease. Front Vet Sci. (2019) 6:498. doi: 10.3389/fvets.2019.00498
99. Rajilić-Stojanović, M, and de Vos, WM. The first 1000 cultured species of the human gastrointestinal microbiota. FEMS Microbiol Rev. (2014) 38:996–1047. doi: 10.1111/1574-6976.12075
100. Gerritsen, J, Hornung, B, Renckens, B, van Hijum, SAFT, Martins Dos Santos, VAP, Rijkers, GT, et al. Genomic and functional analysis of Romboutsia ilealis CRIBT reveals adaptation to the small intestine. PeerJ. (2017) 5:e3698. doi: 10.7717/peerj.3698
101. Weimer, PJ, Nerdahl, M, and Brandl, DJ. Production of medium-chain volatile fatty acids by mixed ruminal microorganisms is enhanced by ethanol in co-culture with Clostridium kluyveri. Bioresour Technol. (2015) 175:97–101. doi: 10.1016/j.biortech.2014.10.054
102. Ephraim, E, Cochrane, C-Y, and Jewell, DE. Varying protein levels influence metabolomics and the gut microbiome in healthy adult dogs. Toxins. (2020) 12:517. doi: 10.3390/toxins12080517
103. Zafar, H, and Saier, MH. Gut Bacteroides species in health and disease. Gut Microbes. (2021) 13:1–20. doi: 10.1080/19490976.2020.1848158
104. Morotomi, M, Nagai, F, Sakon, H, and Tanaka, R. Paraprevotella clara gen. Nov., sp. nov. and Paraprevotella xylaniphila sp. nov., members of the family 'Prevotellaceae' isolated from human faeces. Int J Syst Evol Microbiol. (2009) 59:1895–900. doi: 10.1099/ijs.0.008169-0
105. Wu, CC, Johnson, JL, Moore, WE, and Moore, LV. Emended descriptions of Prevotella denticola, Prevotella loescheii, Prevotella veroralis, and Prevotella melaninogenica. Int J Syst Bacteriol. (1992) 42:536–41. doi: 10.1099/00207713-42-4-536
106. Yatsunenko, T, Rey, FE, Manary, MJ, Trehan, I, Dominguez-Bello, MG, Contreras, M, et al. Human gut microbiome viewed across age and geography. Nature. (2012) 486:222–7. doi: 10.1038/nature11053
107. Oemcke, LA, Anderson, RC, Altermann, E, Roy, NC, and McNabb, WC. The role of segmented filamentous bacteria in immune barrier maturation of the small intestine at weaning. Front Nutr. (2021) 8:759137. doi: 10.3389/fnut.2021.759137
108. Gerritsen, J, Hornung, B, Ritari, J, Paulin, L, Rijkers, GT, Schaap, PJ, et al. A comparative and functional genomics analysis of the genus Romboutsia provides insight into adaptation to an intestinal lifestyle. bioRxiv. (2019):845511. doi: 10.1101/845511
109. Morelli, G, Patuzzi, I, Losasso, C, Ricci, A, Contiero, B, Andrighetto, I, et al. Characterization of intestinal microbiota in normal weight and overweight border collie and Labrador retriever dogs. Sci Rep. (2022) 12:9199. doi: 10.1038/s41598-022-13270-6
110. Thomson, P, Santibáñez, R, Rodríguez-Salas, C, Flores-Yañez, C, and Garrido, D. Differences in the composition and predicted functions of the intestinal microbiome of obese and normal weight adult dogs. PeerJ. (2022) 10:e12695. doi: 10.7717/peerj.12695
111. Oba, PM, Kelly, J, Kostiuk, D, and Swanson, KS. Effects of weight loss and feeding specially formulated diets on the body composition, blood metabolite profiles, voluntary physical activity, and fecal metabolites and microbiota of obese dogs. J Anim Sci. (2023) 101:73. doi: 10.1093/jas/skad073
112. Garrigues, Q, Apper, E, Chastant, S, and Mila, H. Gut microbiota development in the growing dog: a dynamic process influenced by maternal, environmental and host factors. Front Vet Sci. (2022) 9:964649. doi: 10.3389/fvets.2022.964649
113. Reddy, KE, Kim, HR, Jeong, JY, So, KM, Lee, S, Ji, SY, et al. Impact of breed on the fecal microbiome of dogs under the same dietary condition. J Microbiol Biotechnol. (2019) 29:1947–56. doi: 10.4014/jmb.1906.06048
Keywords: digestibility, dog, fecal metabolites, microalgae, microbiota, palatability
Citation: Cabrita ARJ, Guilherme-Fernandes J, Spínola M, Maia MRG, Yergaliyev T, Camarinha-Silva A and Fonseca AJM (2023) Effects of microalgae as dietary supplement on palatability, digestibility, fecal metabolites, and microbiota in healthy dogs. Front. Vet. Sci. 10:1245790. doi: 10.3389/fvets.2023.1245790
Edited by:
Giovanna Martelli, University of Bologna, ItalyReviewed by:
Ilias Giannenas, Aristotle University of Thessaloniki, GreeceJulia Hankel, University of Veterinary Medicine Hannover, Germany
Copyright © 2023 Cabrita, Guilherme-Fernandes, Spínola, Maia, Yergaliyev, Camarinha-Silva and Fonseca. This is an open-access article distributed under the terms of the Creative Commons Attribution License (CC BY). The use, distribution or reproduction in other forums is permitted, provided the original author(s) and the copyright owner(s) are credited and that the original publication in this journal is cited, in accordance with accepted academic practice. No use, distribution or reproduction is permitted which does not comply with these terms.
*Correspondence: Ana R. J. Cabrita, arcabrita@icbas.up.pt
†Present address: Maria Spínola, Centre for Interdisciplinary Research in Animal Health (CIISA), Associate Laboratory for Animal and Veterinary Sciences (AL4AnimalS), Faculty of Veterinary Medicine, University of Lisbon, Lisbon, Portugal