Antimicrobial Peptides: Bringing Solution to the Rising Threats of Antimicrobial Resistance in Livestock
- 1Faculty Veterinary Medicine, University Malaysia Kelantan, Pengkalan Chepa, Malaysia
- 2Faculty of Veterinary Science, University of Nyala, Nyala, Sudan
- 3Department of Biochemistry, University of Veterinary Medicine Hannover, Hanover, Germany
- 4Research Center for Emerging Infections and Zoonoses (RIZ), University of Veterinary Medicine Hannover, Hanover, Germany
- 5Department of Microbiology, Faculty of Veterinary Medicine, University of Khartoum, Khartoum North, Sudan
Antimicrobial therapy is the most applied method for treating and preventing bacterial infection in livestock. However, it becomes less effective due to the development of antimicrobial resistance (AMR). Therefore, there is an urgent need to find new antimicrobials to reduce the rising rate of AMR. Recently, antimicrobial peptides (AMPs) have been receiving increasing attention due to their broad-spectrum antimicrobial activity, rapid killing activities, less toxicity, and cell selectivity. These features make them potent and potential alternative antimicrobials to be used in animals. Here, we discuss and summarize the AMPs in animals, classification, structures, mechanisms of action, and their potential use as novel therapeutic alternative antimicrobials to tackle the growing AMR threat.
Introduction
Livestock infectious disease represents a big threat to animal health, welfare, public health, environment, and food security (1). It can cause huge economic impacts due to the increased mortality, cost of treatment and control, reduced productivity, loss in trade, and decreased gross domestic product (GDP) (2). The treatment and control of livestock diseases is crucial for livestock industries, safeguarding public health, and securing global food supplies. Antimicrobials are commonly used for the treatment and prevention of bacterial infection in livestock. These practices, in part, are associated with increased rates of antimicrobial resistance (AMR) among pathogenic bacteria isolated from animals (3). There is rising concern that overuse of antimicrobials has led to the emergence of resistant organisms to most or all antimicrobials and thus can lead to therapeutic failure (4). Resistant bacteria can spread from food animals to humans through different routes including direct contact with livestock, indirect contact through food consumption, and animal waste used as a fertilizer of crops and can contaminate water supplies (5, 6). Therefore, there is an increasing need to evaluate and develop alternative methods for antimicrobial treatment (7). A wide range of alternative antimicrobial approaches have been developed by researchers worldwide to find effective methods to tackle the infection caused by AMR. These methods include the application of antimicrobial peptides (AMPs) (8–12).
AMPs are gene-encoded polypeptide sequences that are considered essential elements of immune defenses in all organisms (13). The antibacterial properties of AMPs observed in in vitro conditions have long attracted the attention of scientists looking to address AMR's problem. Both natural and synthetic AMPs have proved strong and broad-spectrum antibacterial activity in vitro and efficacy in various animal infection models. At the same time, their actions are considered unaffected by canonical bacterial resistance mechanisms that reduce the effectiveness of conventional antibiotics (14). Besides the direct antimicrobial activity, AMPs has an anti-biofilm effect by suppressing biofilm formation and destabilizing the biofilm structure (13). In addition, AMPs have immunomodulatory effects by stimulating the immune response. The versatile role of AMPs is highlighted not only in eliminating pathogens but also in boosting immunity to induce better protection against infection (15). Therefore, the AMPs have potential for the development of novel alternative antimicrobials to replace the existing antibiotics. This review will summarize and discuss the recent update with AMPs in animals and their mechanisms of antimicrobial activity. Also highlighted is the therapeutic application of novel alternative antimicrobials for the treatment of bacterial infection in livestock.
Antimicrobial Peptides (AMPs)
AMPs are small-sized proteins that are crucial elements in host immune defense in most living organisms, including animals, humans, insects, fish, and plants (16). AMPs are short-chain amino acids (composed of 10–50 amino acids), amphiphilic, and positively charged (17). This feature allows them to bind and penetrate the bacterial membrane bilayer to induce pores by “toroidal-pore”, “barrel-stave,” and “carpet”, thus causing intracellular leakage (16). AMPs have received a great amount of attention due to their broad spectrum of antimicrobial activity against various microorganisms such as fungi, viruses, and bacteria in both veterinary and human pathogens (18), rapid killing activities, less toxicity, and cell selectivity (18, 19). In addition, a new study found that the combination of AMPs with conventional antibiotic increased the efficacy in killing bacteria and preventing the development of AMR. These findings highlight the potential adjunctive administration of AMPs in clinical applications (20).
Classification of AMPs
AMPs are large and diverse and can basically be divided into major groups based on structure, source, and biological activity (20) (Figure 1). Based on the sources, the peptide can be found in animals, humans, insects, microorganisms, and plants, and based on structure, the AMPs can be divided into four categories: α-helix, β-sheet, extended, and loop (9). On the other hand, based on biological activity, peptides can be divided into antibacterial peptides, antifungal peptides, antiviral peptides, antiparasitic peptides, and anticancer peptides (21).
Animal AMPs
Animals possess a wide range of AMPs that protect them against infection. Some of these peptides have been fully studied and characterized, and most of them have activity against diverse pathogens. The mammalian AMPs can be divided into two major classes: cathelicidins and defensins (21). AMPs from animals can be found in many parts of their bodies such as skin, intestine, blood, saliva, and milk. The most AMPs from animals include indolicidin, Bovine Psoriasin, Bovine Defensin 1, Protegrin, Cecropin P1, Ovine Defensins, and lactoferrecin. Table 1 summarizes the major AMPs. These peptides play an essential role in host immunity and protect them from infection (22). Dairy is a rich source of AMPs; many peptides have been identified from casein, lactoglobulin, and lactoferrin, among them is lactoferrecin, considered the most common peptide found in milk (23). Some of these AMPs such as indolicidin have been approved to be used in the treatment of bovine mastitis (24), while the majority of AMPs are still in preclinical studies.
Amphibian AMPs
AMPs from amphibians have a vital role in the protection of amphibians from the invasion by pathogenic microorganisms (22). Certain amphibians can produce AMPs, and frogs are considered a major source of amphibian peptides (35). Magainins and dermaseptins are two classes of cationic, amphipathic alpha-helical peptides that have been identified in the skin extracts of frogs Phyllomedusa sauvagei and Xenopus laevis (36). Magainins are 23-amino-acid-long peptides that belong to a large family of amphibian amphipathic α-helical AMPs. These peptides have been reported to have a wide spectrum of antimicrobial activities against Gram-negative and Gram-positive bacteria and fungi, and mechanisms of action due to the disruption of the bacterial membrane (20). On the other hand, these peptides are also reported to have a cytotoxic effect toward mammalian cells at 31.25 μg/ml (20), but they did not cause red blood cell (RBC) hemolysis up to 300 μg/ml (37). Dermaseptins are linear polycationic peptides, composed of 28–34 amino acids, which are structured in amphipathic-helices in apolar solvents (38). These peptides demonstrated broad-spectrum antimicrobial activity, including multidrug-resistant strains. In addition, dermaseptins have been found to be less cytotoxic compared to magainins (20).
Insect AMPs
Insects are one of the biggest sources of AMPs (39). Several AMPs are produced by insects, and these AMPs are mainly found in blood cells or in fat tissues of insects and help in survival mechanisms to protect the insect from diseases (22). The most common insect AMPs are defensins, cecropins, ponericins, drosomycin, drosocin, attacins, diptericins, and metchnikowin (37). Cecropins are effective against both Gram-negative and Gram-positive bacteria, whereas defensins can selectively kill Gram-positive bacteria only (38). Most insect AMPs have strong antimicrobial activities and low toxicity, making them excellent candidates to be developed as alternative antimicrobials.
Plant AMPs
Plants are known to have several AMPs, and it is reported that they have potent antimicrobial activity against pathogenic bacteria, viruses, and parasites besides having anticancer and anti-inflammatory activity (21). Most AMPs from plants share the same feature as those from animals, insects, and microorganisms. For example, defensins, thionins, and cyclotides are the common plant AMPs, and they look similar to AMPs from animals in terms of amphipathic nature, molecular form, positive charge, and Cys-rich peptides (39, 40). On the other hand, some plant peptides are different from those from animals such as hevein-like peptides that bind chitins (40). Currently, thousands of plant AMPs have been identified and none of them has been approved yet for clinical use.
Microorganism AMPs
AMPs can be obtained from microorganisms such as fungi and bacteria. Some common peptides are bacteriocins derived from lactic acid bacteria such as Lactococcus lactis, Bacillus brevis, and Bacillus subtilis (21). The most common bacteriocin includes nisin, mersacidin, lacticin 481, and lacticin 3147. Among them, nisin has been approved for commercial use for the treatment of bovine mastitis (25), while mersacidin and lacticin have promising results for the treatment of infection caused by bacteria, particularly antibiotic-resistant bacterial strains such as methicillin-resistant Staphylococcus aureus (MRSA) and vancomycin-resistant enterococci (VRE) (41). Plectasin is another APM that belongs to defensin from microorganisms isolated particularly from the fungus saprophytic ascomycete Pseudoplectania nigrella. Plectasin demonstrated strong bactericidal activity against Gram-positive bacteria such as S. aureus and particularly multidrug-resistant strains with extremely low toxicity (42).
Structural Classes of AMPs
AMPs are generally divided into four major classes based on their structure: α-helical, β-sheet, extended, and loop peptides (17) (Figure 2). Both natural and synthesis peptides shared the same structure, and the most common feature for all peptide groups is the ability to fold into amphiphilic or amphipathic conformation due to their interaction with membrane (17). The α-helical peptides are the most abundant class of natural peptides in nature. These peptides will, upon interaction with target membranes fold into an amphipathic α-helix. “Amphipathicity” is the property of having hydrophobic and hydrophilic regions separated in space” with one face of the helix predominantly containing the hydrophobic amino acids and the opposite face containing the charged amino acids (43). β-sheet peptides are indicated by the presence of an antiparallel β-sheet stabilized by two or more disulfide bonds (17) and include, among others, the defensins of vertebrates, insects, and plants (44). Extended peptides such as indolicidin contain high proportions of certain amino acids such as tryptophan, histidine, and proline (45). Most of these peptides adopt extended structures upon interaction with the membrane, and this is stabilized by hydrogen bonds and van der Waals forces with lipids rather than interresidue hydrogen bonds (46). Loop peptides are characterized by their loop structure imparted by the presence of a single bond, i.e., disulfide and amide (17).
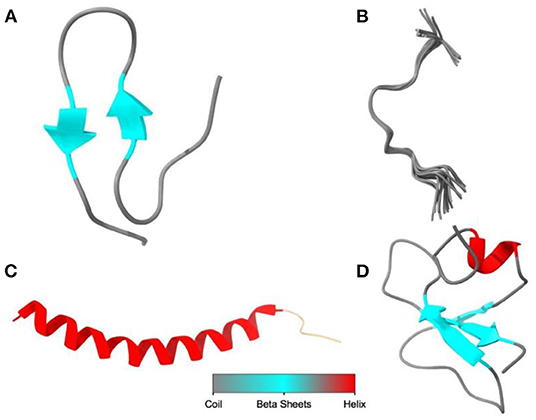
Figure 2. The diagram illustrated the main structural classes of AMPs: (A) β-sheet, defensins, and protegrins; (B) extended, indolicidin; (C) α-helical, nisin, and lactoferricin; (D) loop or combined structure, plectasin. The image was created using UCSF Chimera (http://www.cgl.ucsf.edu/chimera).
Antimicrobial Mechanisms of AMPs
The antimicrobial mode of action of AMPs is complicated, and one single peptide can target different sites in the microbe (47). The antimicrobial activity of AMPs is believed to be due to inhibition of cell wall, nucleic acid and protein synthesis, and inhibition of enzymatic activity (21, 47). The antimicrobial activity of AMPs is particularly linked to its corresponding amino acid composition and physicochemical characteristics (45). Several studies have identified that the antimicrobial mechanism of AMPs kills bacteria due to increased membrane permeability, induction of lipid asymmetry, and loss of cellular components and essential metabolites, which ultimately leads to cell death (47–49). In addition to membrane permeabilization, AMPs can kill bacterial cells by targeting not only DNA but also the biosynthesis of cell wall, LPS, and other biological pathways (50).
Membrane Interaction Mechanisms
AMPs attach to the bacteria cell wall by electrostatic interactions between the anionic component of a membrane and the positive charge of a peptide (21, 51, 52). After binding, the peptides cross the cell wall and cell membrane to contact the cellular membrane in Gram-positive bacteria. For Gram-negative bacteria, the first action of AMPs involves the competitive displacement of Mg2+ and Ca2+. In this way, peptides destabilize this supramolecular assembly and gain access to both inner and outer membranes. Following the attachment, AMPs are inserted into the membrane to form transmembrane pores and are divided into four models: (1) the barrel-stave model in which the peptides penetrate the membrane and form pores in the hydrophilic portion, (2) the carpet model in which the peptides disrupt the membrane structure by a detergent-like action, (3) the toroidal model in which the hydrophilic portion of the amphipathic conformation of peptides is associated with the lipid headgroup, and (4) the aggregate model in which the peptide penetrates the membrane and damaging it (21) (Figure 3).
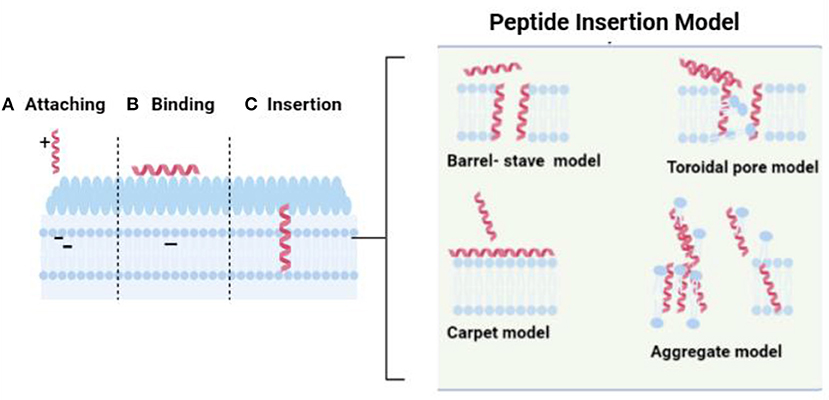
Figure 3. The interaction between peptide and bacterial cellular membrane. The image was created using BioRender illustrator (https://Biorender.com/).
Targeting Intracellular Components
Besides the membrane damage, the peptide can kill bacteria by inhibiting the biosynthesis of nucleic acid, proteins, and some essential enzymes from synthesizing cell walls and bacteria growth (21). Figure 4 summarizes the mechanisms for the intracellular AMPs. AMPs can interfere with key enzymes involved in transcription, translation, and assembly, such as chaperones, leading to inhibition of proteins. For example, pyrrhocoricin targets ribosomes to inhibit protein translation, whereas PR-39 inhibits protein synthesis in E. coli by inhibiting the transition from the initial to the extension phase (34, 53).
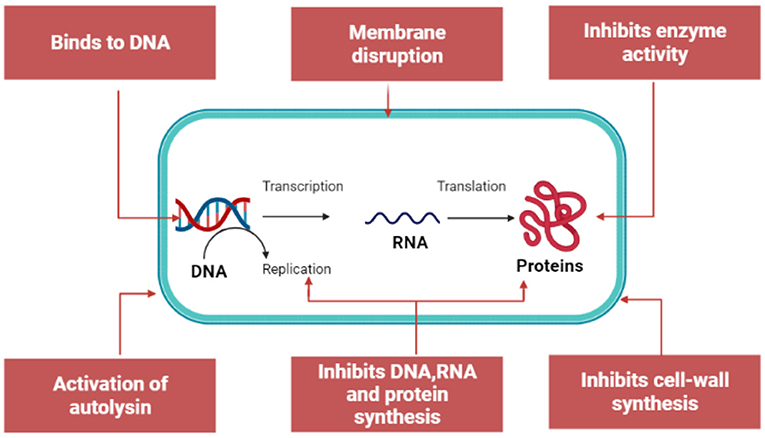
Figure 4. Mechanism for intracellular antimicrobial peptide activity. The image was created using BioRender illustrator (https://Biorender.com/).
Furthermore, AMPs can induce degradation of DNA and RNA or affect key enzymes involved in DNA synthesis. For example, indolicidin can target a basic site of DNA to crosslink single- or double-stranded DNA, and it can also inhibit DNA topoisomerase I (54, 55). Besides inhibiting nucleic acids and proteins, AMPs can inhibit the metabolic activity of cells due to the effect on protease activity (21).
Recent Advances in the Use of AMPs for the Treatment of Bacterial Infection in Animals
Peptide-based antimicrobials have shown efficacy towards pathogenic bacteria in animals. Some of them such as nisin were approved for clinical use while others are at the advanced stages of clinical trials (Table 2). Several studies have reported that AMPs have strong antibacterial activity against a wide range of pathogens. Tomasinsig et al. (24) found that the cathelicidin family of peptide from bovine such as BMAP-27, BMAP-28, Bac5, and indolicidin has a broad spectrum of activity against most bacterial isolates from bovine mastitis with MIC in the range of 0.5–32 μM. Besides the mastitis in cattle, AMPs particularly lactoferricin (lfcin) and nisin have demonstrated strong antibacterial, anti-fungal, and antiparasitic activity with potential use in both animals and humans for the treatment of infection (31, 56).
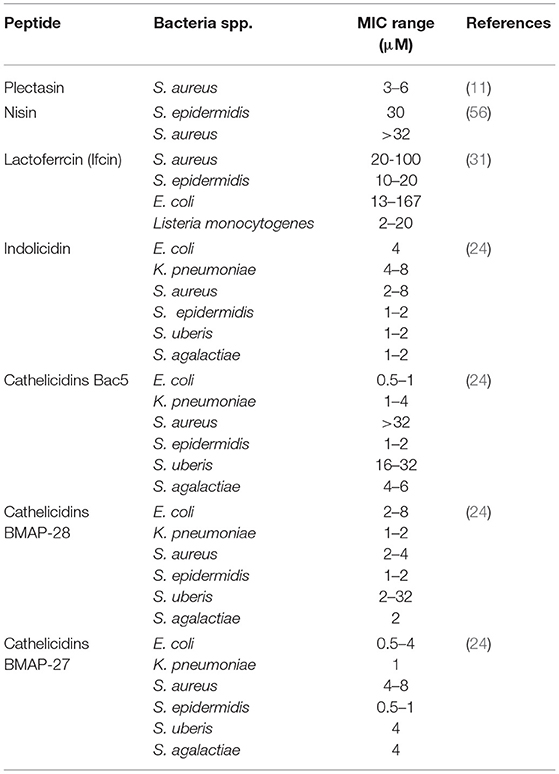
Table 2. Antimicrobial activity of the peptide-based antimicrobial compound against pathogenic bacteria isolated from livestock.
Plectasin
Plectasin is a peptide-based antimicrobial (Figure 5A) first isolated from the saprophytic ascomycete Pseudoplectania nigrella, and it was reported to have strong antimicrobial activity (11, 42). Plectasin peptide can kill bacteria through interference with cell wall biosynthesis by specifically binding to Lipid II, which is the vital element of bacterial cell wall precursor (57). Despite the strong antimicrobial activity, plectasin was reported to have low toxicity toward mammalian cells, making them a strong candidate as an alternative antimicrobial (12). In a recent study conducted by Li et al. (11), they found that plectasin-derived AMPs such as NZ2114 and MP1102 have strong bactericidal activity toward S. aureus mastitis with MIC of 1–2 μg/ml in media. In addition, the plectasin AMPs demonstrated potency against intracellular S. aureus at 100 μg/ml, reducing 100% of S. aureus inside the mammary epithelial cells.
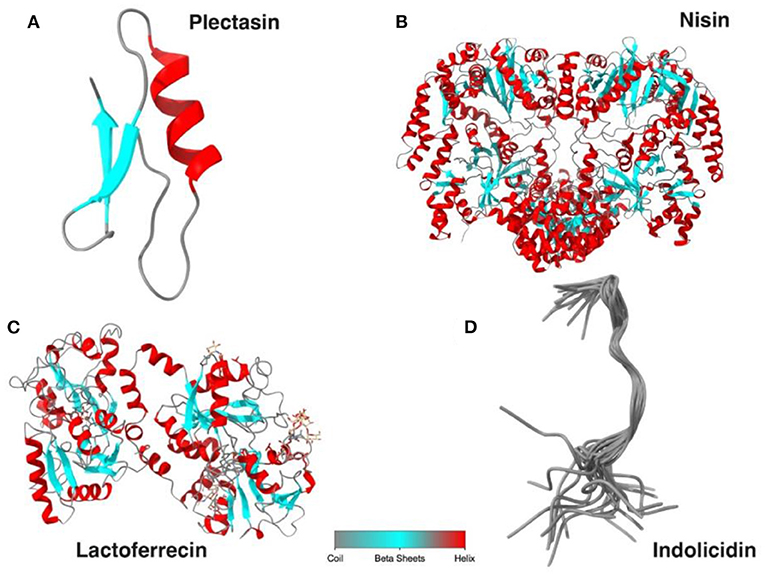
Figure 5. The diagram illustrates the peptide structure for plectasin (A), nisin (B), lactoferricin (C), and indolicin (D). The image was created using UCSF Chimera (http://www.cgl.ucsf.edu/chimera).
Nisin
Nisin is an AMP belonging to the lantibiotic family (Figure 5B) that has strong antimicrobial activities against Gram-positive bacteria (56). This peptide is commonly used as a food preservative, and it is approved by FDA and WHO. Nisin is produced by the bacteria Lactococcus lactis with 34 amino acids. It is considered as a promising alternative antimicrobial to replace existing antibiotics, particularly for the treatment of infections caused by multidrug-resistant bacteria. Nisin displays bactericidal properties due to it binding to lipid II in bacteria membrane, leading to inhibition of cell wall biosynthesis and induced form in bacterial cell membrane (56, 58). Recently, nisin is developed and formulated as the product to be used clinically to treat mastitis, and it shows promising results. For example, the study conducted by Cao et al. reported that treatment of bovine mastitis with intramammary administration of 2,500,000 IU resulted in a 90.2% clinical cure rate. In addition, all S. aureus isolated from mastitis were sensitive toward nisin, while 82.5% of them were resistant to penicillin and 35.3% were resistant to gentamicin (42).
Lactoferrecin
Lactoferrecin is an iron-binding glycoprotein peptide derived from lactoferrin F (Figure 5C). Besides its host immune defense, this molecule displays strong antimicrobial activity against bacteria, virus, fungi, and parasite and can be an excellent alternative antimicrobial to combat many diseases such as mastitis (31). The bactericidal effect of lactoferricin is believed to be due to its ability to disrupt microbe cellular permeability and inhibition of cell wall due to electrostatic interaction between the positive charge in amino acids such as arginine in peptide and the negative charge in bacteria membrane such as LPS in Gram-negative and lipoteichoic and teichoic acids in Gram-positive bacteria. On the other hand, lactoferricin also displays anticancer activity and low toxicity toward mammalian cells (59). In addition, lactoferricin is considered an excellent alternative drug for mastitis treatment due to its activity against different mastitis pathogens. In the study, Kawai et al. examined the efficacy of these compounds toward subclinical mastitis caused by Staphylococci and E. coli. The study recorded the decrease in bacteria load on the first day of treatment and a 100% cure rate from mastitis after 14 days (60).
Indolicidin
Indolicidin is an extended cationic antimicrobial peptide and is a member of the cathelicidin group with 13 amino acids, rich in tryptophan and proline, and is amidated at the carboxyl terminus in nature (32) (Figure 5D). It was initially purified from the cytoplasmic granules of neutrophils from bovine (61). It can also be synthesized in bone marrow cells as a 144-aa-long precursor (62). Indolicidin demonstrated antimicrobial activity against several microorganisms including Candida albicans, Cryptococcus neoformans, S. aureus, and E. coli, and its antimicrobial action includes disruption of the bacterial membrane by channel formation and inhibition of DNA replication (8, 63). The membrane damage exhibited by indolicidin could be due to its optimum hydrophobicity along with the pore formation in bacterial membrane resulting in membrane lipid–bilayer partition (64, 65). In the recent study conducted by Vergis (66), they found that indolicidin was effective against multidrug-resistant enteroaggregative E. coli (MDR-EAEC) with a MIC of 32 μM, and at 2 × MIC and 4 × MIC, clearance of MDR-EAEC was completed after 120 min of coincubation. This bacterial elimination by AMPs highlighted the potency of the peptide compared to conventional antibiotics.
Cathelicidins
Cathelicidins are short cationic peptides that are part of the innate immune system, and they are found in mammals, including humans (24). These peptides demonstrated broad-spectrum activity against microorganisms. In addition, they can protect against infection by modulating other components of the innate or adaptive immune response (24, 67, 68). Cathelicidins belong to the family of host defense peptides (HDPs), with each cathelicidin encoded by a single gene, consisting of four exons (69). Bovine cathelicidins BMAP-27, BMAP-28, and Bac5 produced by myeloid-derived cells are well studied for their antimicrobial activity (24). For example, a study conducted by Tomasinsig et al. (24) found that the AMPs from family cathelicidins showed a potent antibacterial activity toward bacteria isolated from bovine mastitis. The tested peptides include BMAP-27, BMAP-28, and Bac5 with a MIC value of 0.5–32 μM, and the mechanisms of antimicrobials are due to disruption of bacterial membrane integrity. In addition, the study also found that these peptides were also able to effectively trigger expression of the proinflammatory mediator TNF in bovine mammary epithelial cells and stimulate the immune response to act against invading bacteria (24).
Conclusions
Antimicrobial agents serve as an important component in the treatment and control of bacterial infection in livestock. However, the treatment becomes ineffective due to the development of AMR, leading to therapeutic failure. Therefore, there is an urgent need to find alternatives to existing antimicrobials. Recently, AMPs have been explored and introduced as a novel alternative antimicrobial due to their unique antimicrobial properties in target bacteria, fungi, viruses, and parasites; AMPs also have anticancer activity and an immunomodulatory effect. In addition, AMPs are considered less toxic to mammalian cells. However, there is limited information regarding the antimicrobial application of AMPs in animals. In this review, we have summarized the importance of antimicrobials, classification, and source and mechanism of peptides' antimicrobial activity. In addition, recent advances in AMP use for the treatment of bacterial infection in livestock are highlighted. AMPs demonstrated promising results in the treatment of bacterial infection, particularly the AMR strain. Therefore, AMPs could be an excellent alternative antimicrobial to be used as replacement for existing antibiotics.
Author Contributions
SS designed the framework of the review, provided the illustration, and wrote the first draft. AM contributed to manuscript writing. EA and NK supervised the work and editing and revised the final version of the manuscript for publication. All authors read and approved the final manuscript for publication.
Funding
This work was supported by the Fundamental Research Grant Scheme by Malaysia Ministry of Higher Education grant code FRGS/1/2017/SKK11/UMK/03/1.
Conflict of Interest
The authors declare that the research was conducted in the absence of any commercial or financial relationships that could be construed as a potential conflict of interest.
Publisher's Note
All claims expressed in this article are solely those of the authors and do not necessarily represent those of their affiliated organizations, or those of the publisher, the editors and the reviewers. Any product that may be evaluated in this article, or claim that may be made by its manufacturer, is not guaranteed or endorsed by the publisher.
Acknowledgments
We thank Pritam K. Panda for providing help to illustrate the peptide image.
References
1. Tomley FM, Shirley MW. Livestock infectious diseases and zoonoses. Philos Trans R Soc B Biol Sci. (2009) 364:2637–42. doi: 10.1098/rstb.2009.0133
2. Barratt AS, Rich KM, Eze JI, Porphyre T, Gunn GJ, Stott AW. Framework for estimating indirect costs in animal health using time series analysis. Front Vet Sci. (2019) 6:190. doi: 10.3389/fvets.2019.00190
3. Landers TF, Cohen B, Wittum TE, Larson EL. A review of antibiotic use in food animals: Perspective, policy, and potential. Public Health Rep. (2012) 127:4–22. doi: 10.1177/003335491212700103
4. Serwecińska L. Antimicrobials and antibiotic-resistant bacteria: A risk to the environment and to public health. Water (Switzerland). (2020) 12:3313. doi: 10.3390/w12123313
5. Woolhouse MEJ, Ward MJ. Sources of antimicrobial resistance. Science. (2013) 341:1460–1. doi: 10.1126/science.1243444
6. Economou V, Gousia P. Agriculture and food animals as a source of antimicrobial-resistant bacteria. Infect Drug Resist. (2015) 8:49–61. doi: 10.2147/IDR.S55778
7. Bartlett JG, Gilbert DN. Spellberg B. Seven ways to preserve the miracle of antibiotics. Clin Infect Dis. (2018) 56:1445–50. doi: 10.1093/cid/cit070
8. Toke O. Antimicrobial peptides: new candidates in the fight against bacterial infections. Biopolym Pept Sci Sect. (2005) 80:717–35. doi: 10.1002/bip.20286
9. Rima M, Rima M, Fajloun Z, Sabatier JM, Bechinger B, Naas T. Antimicrobial peptides: A potent alternative to antibiotics. Antibiotics. (2021) 10:1095. doi: 10.3390/antibiotics10091095
10. Moretta A, Scieuzo C, Petrone AM, Salvia R, Manniello MD, Franco A, et al. Antimicrobial peptides: a new hope in biomedical and pharmaceutical fields. Front Cell Infect Microbiol. (2021) 11:1–26. doi: 10.3389/fcimb.2021.668632
11. Li L, Wang L, Gao Y, Wang J, Zhao X. Effective antimicrobial activity of plectasin-derived antimicrobial peptides against Staphylococcus aureus infection in mammary glands. Front Microbiol. (2017) 8:1–8. doi: 10.3389/fmicb.2017.02386
12. Andes D, Craig W, Nielsen LA, Kristensen HH. In vivo pharmacodynamic characterization of a novel plectasin antibiotic, NZ2114, in a murine infection model. Antimicrob Agents Chemother. (2009) 53:3003–9. doi: 10.1128/AAC.01584-08
13. Fjell CD, Hiss JA, Hancock REW, Schneider G. Designing antimicrobial peptides: form follows function. Nat Rev Drug Discov. (2012) 11:37–51. doi: 10.1038/nrd3591
14. Hancock REW, Alford MA, Haney EF. Antibiofilm activity of host defence peptides: complexity provides opportunities. Nat Rev Microbiol. (2021) 19:786–97. doi: 10.1038/s41579-021-00585-w
15. Li W, Separovic F, O'Brien-Simpson NM, Wade JD. Chemically modified and conjugated antimicrobial peptides against superbugs. Chem Soc Rev. (2021) 50:4932–73. doi: 10.1039/D0CS01026J
16. Mandal SM, Roy A, Ghosh AK, Hazra TK, Basak A, Franco OL. Challenges and future prospects of antibiotic therapy: from peptides to phages utilization. Front Pharmacol. (2014) 5:1–12. doi: 10.3389/fphar.2014.00105
17. Powers JPS, Hancock REW. The relationship between peptide structure and antibacterial activity. Peptides. (2003) 24:1681–91. doi: 10.1016/j.peptides.2003.08.023
18. Datta S, Roy A. Antimicrobial peptides as potential therapeutic agents: a review. Int J Pept Res Ther. (2021) 27:555–77. doi: 10.1007/s10989-020-10110-x
19. Bengtsson-Palme J, He S. Strategies to reduce or eliminate resistant pathogens in the environment. Antiobio Drug Resist. (2019) 23:637. doi: 10.1002/9781119282549
20. Lin B, Hung A, Li R, Barlow A, Singleton W, Matthyssen T, et al. Systematic comparison of activity and mechanism of antimicrobial peptides against nosocomial pathogens. Eur J Med Chem. (2022) 231:114135. doi: 10.1016/j.ejmech.2022.114135
21. Lei J, Sun LC, Huang S, Zhu C, Li P, He J, et al. The antimicrobial peptides and their potential clinical applications. Am J Transl Res. (2019) 11:3919–31.
22. Huan Y, Kong Q, Mou H, Yi H. Antimicrobial Peptides: classification, design, application and research progress in multiple fields. Front Microbiol. (2020) 11:1–21. doi: 10.3389/fmicb.2020.582779
23. Sibel Akalin A. Dairy-derived antimicrobial peptides: Action mechanisms, pharmaceutical uses and production proposals. Trends Food Sci Technol. (20s14) 36:79–95. doi: 10.1016/j.tifs.2014.01.002
24. Tomasinsig L, De Conti G, Skerlavaj B, Piccinini R, Mazzilli M, D'Este F, et al. Broad-spectrum activity against bacterial mastitis pathogens and activation of mammary epithelial cells support a protective role of neutrophil cathelicidins in bovine mastitis. Infect Immun. (2010) 78:1781–8. doi: 10.1128/IAI.01090-09
25. Li J, Hu S, Jian W, Xie C, Yang X. Plant antimicrobial peptides: structures, functions, and applications. Bot Stud. (2021) 62:5. doi: 10.1186/s40529-021-00312-x
26. Ribeiro ARM, Felgueiras HP, Costa SPG, Pereira-Lima SMMA. Synthesis of peptaibolin, an antimicrobial peptide. Proceedings. (2020) 78:47. doi: 10.3390/IECP2020-08654
27. Guo C, Cong P, He Z, Mo D, Zhang W, Chen Y, et al. Inhibitory activity and molecular mechanism of protegrin-1 against porcine reproductive and respiratory syndrome virus in vitro. Antivir Ther. (2015) 20:573–82. doi: 10.3851/IMP2918
28. Subbalakshmi C, Sitaram N. Mechanism of antimicrobial action of indolicidin. FEMS Microbiol Lett. (1998) 160:91–6. doi: 10.1111/j.1574-6968.1998.tb12896.x
29. Linde CMA, Hoffner SE, Refai E, Andersson M. In vitro activity of PR-39, a proline-arginine-rich peptide, against susceptible and multi-drug-resistant Mycobacterium tuberculosis. J Antimicrob Chemother. (2001) 47:575–80. doi: 10.1093/jac/47.5.575
30. Ackermann MR, Gallup JM, Zabner J, Evans RB, Brockus CW, Meyerholz DK, et al. Differential expression of sheep beta-defensin-1 and−2 and interleukin 8 during acute Mannheimia haemolytica pneumonia. Microb Pathog. (2004) 37:21–7. doi: 10.1016/j.micpath.2004.04.003
31. Bruni N, Capucchio MT, Biasibetti E, Pessione E, Cirrincione S, Giraudo L, et al. Peptides and applications in human and veterinary medicine. Molecules. (2016) 21:752. doi: 10.3390/molecules21060752
32. Hsu CH, Chen C, Jou ML, Lee AYL, Lin YC Yu YP, et al. Structural and DNA-binding studies on the bovine antimicrobial peptide, indolicidin: evidence for multiple conformations involved in binding to membranes and DNA. Nucleic Acids Res. (2005) 33:4053–64. doi: 10.1093/nar/gki725
33. Reddy KVR, Yedery RD, Aranha C. Antimicrobial peptides: Premises and promises. Int J Antimicrob Agents. (2004) 24:536–47. doi: 10.1016/j.ijantimicag.2004.09.005
34. Brogden KA. Antimicrobial peptides: Pore formers or metabolic inhibitors in bacteria? Nat Rev Microbiol. (2005) 3:238–50. doi: 10.1038/nrmicro1098
35. Soltaninejad H, Zare-Zardini H, Ordooei M, Ghelmani Y, Ghadiri-Anari A, Mojahedi S, et al. Antimicrobial peptides from amphibian innate immune system as potent antidiabetic agents: a literature review and bioinformatics analysis. J Diabetes Res. (2021) 2021:2894722. doi: 10.1155/2021/2894722
36. Hani K, Zairi A, Tangy F, Bouassida K. Dermaseptins and magainins: antimicrobial peptides from frogs' skin-new sources for a promising spermicides microbicides-a mini review. J Biomed Biotechnol. (2009) 2009:452567. doi: 10.1155/2009/452567
37. Clara A, Manjramkar DD, Reddy VK. Preclinical evaluation of magainin-A as a contraceptive antimicrobial agent. Fertil Steril. (2004) 81:1357–65. doi: 10.1016/j.fertnstert.2003.09.073
38. MOR A, NICOLAS P. Isolation and structure of novel defensive peptides from frog skin. Eur J Biochem. (1994) 219:145–54. doi: 10.1111/j.1432-1033.1994.tb19924.x
39. Wu Q, Patočka J, Kuča K. Insect antimicrobial peptides, a mini review. Toxins (Basel). (2018) 10:1–17. doi: 10.3390/toxins10110461
40. Józefiak A, Engberg RM. Insect proteins as a potential source of antimicrobial peptides in livestock production. A review J Anim Feed Sci. (2017) 26:87–99. doi: 10.22358/jafs/69998/2017
41. Tam JP, Wang S, Wong KH, Tan WL. Antimicrobial peptides from plants. Pharmaceuticals. (2015) 8:711–57. doi: 10.3390/ph8040711
42. Cao LT, Wu JQ, Xie F, Hu SH, Mo Y. Efficacy of nisin in treatment of clinical mastitis in lactating dairy cows. J Dairy Sci. (2007) 90:3980–5. doi: 10.3168/jds.2007-0153
43. Sang Y, Blecha F. Antimicrobial peptides and bacteriocins : alternatives to traditional Antimicrobial peptides and bacteriocins : alternatives to traditional antibiotics. (2012) (2008) 9:227–35. doi: 10.1017/S1466252308001497
44. Mygind PH, Fischer RL, Schnorr KM, Hansen MT, Sönksen CP, Ludvigsen S, et al. Plectasin is a peptide antibiotic with therapeutic potential from a saprophytic fungus. Nature. (2005) 437:975–80. doi: 10.1038/nature04051
45. Hancock REW, Patrzykat A. Clinical development of cationic antimicrobial peptides: From natural to novel antibiotics. Curr Drug Targets Infect Disord. (2002) 2:79–83. doi: 10.2174/1568005024605855
46. McPhee J, Scott M, Hancock R. Design of host defence peptides for antimicrobial and immunity enhancing activities. Comb Chem High Throughput Screen. (2005) 8:257–72. doi: 10.2174/1386207053764558
47. Andrès E. Cationic antimicrobial peptides in clinical development, with special focus on thanatin and heliomicin. Eur J Clin Microbiol Infect Dis. (2012) 31:881–8. doi: 10.1007/s10096-011-1430-8
48. Zhang QY, Yan Z Bin, Meng YM, Hong XY, Shao G, Ma JJ, et al. Antimicrobial peptides: mechanism of action, activity and clinical potential. Mil Med Res. (2021) 8:1–25. doi: 10.1186/s40779-021-00343-2
49. Borah A, Deb B, Chakraborty S. A Crosstalk on Antimicrobial Peptides. Int J Pept Res Ther. (2021) 27:229–44. doi: 10.1007/s10989-020-10075-x
50. Li J, Koh JJ, Liu S, Lakshminarayanan R, Verma CS, Beuerman RW. Membrane active antimicrobial peptides: translating mechanistic insights to design. Front Neurosci. (2017) 11:1–18. doi: 10.3389/fnins.2017.00073
51. Mahlapuu M, Håkansson J, Ringstad L, Björn C. Antimicrobial peptides: an emerging category of therapeutic agents. Front Cell Infect Microbiol. (2016) 6:1–12. doi: 10.3389/fcimb.2016.00194
52. Bechinger B, Gorr S. Antimicrobial peptides : mechanisms of action and resistance. J Dent Res. (2017) 96:254–60. doi: 10.1177/0022034516679973
53. Kumar R, Ali SA, Singh SK, Bhushan V, Mathur M, Jamwal S, et al. Antimicrobial peptides in farm animals: an updated review on its diversity, function, modes of action and therapeutic prospects. Vet Sci. (2020) 7:1–28. doi: 10.3390/vetsci7040206
54. Lomakin IB, Gagnon MG, Steitz TA. Antimicrobial peptides targeting bacterial ribosome. Oncotarget. (2015) 6:18744–5. doi: 10.18632/oncotarget.5114
55. Polikanov YS, Aleksashin NA, Beckert B, Wilson DN. The mechanisms of action of ribosome-targeting peptide antibiotics. Front Mol Biosci. (2018) 5:1–21. doi: 10.3389/fmolb.2018.00048
56. Lewies A, Plessis LH Du, Wentzel JF. The cytotoxic, antimicrobial and anticancer properties of the antimicrobial peptide nisin Z alone and in combination with conventional treatments. Cytotoxicity. (2018) 25:21–42. doi: 10.5772/intechopen.71927
57. Schneider T, Kruse T, Wimmer R, Wiedemann I, Sass V, Pag U, et al. Plectasin, a fungal defensin, targets the bacterial cell wall precursor lipid II. Science. (2010) 328:1168–72. doi: 10.1126/science.1185723
58. Field D, Connor RO, Cotter PD, Ross RP., Hill C. In Vitro activities of nisin and nisin derivatives alone and in combination with antibiotics against Staphylococcus biofilms. Front Microbiol. (2016) 7:1–11. doi: 10.3389/fmicb.2016.00508
59. Strøm MB, Rekdal Ø, Svendsen JS. The effects of charge and lipophilicity on the antibacterial activity of undecapeptides derived from Bovine lactoferricin. J Pept Sci. (2002) 43:36–43. doi: 10.1002/psc.365
60. Kawai K, Nagahata H, Lee N, Anri A. Shimazaki K. Eject of infusing lactoferrin hydrolysate into bovine mammary glands with subclinical mastitis. Vet Res Commun. (2003) 27:539–48. doi: 10.1023/A:1026039522286
61. Selsted ME, Novotny MJ, Morris WL, Tang YQ, Smith W, Cullor JS. Indolicidin, a novel bactericidal tridecapeptide amide from neutrophils. J Biol Chem. (1992) 267:4292–5. doi: 10.1016/S0021-9258(18)42830-X
62. Kościuczuk EM, Lisowski P, Jarczak J, Strzakowska N, Jóźwik A, Horbańczuk J, et al. Cathelicidins family of antimicrobial peptides: A review. Mole Biol Rep. (2012) 39:10957–70. doi: 10.1007/s11033-012-1997-x
63. Nicolas P. Multifunctional host defense peptides: intracellular-targeting antimicrobial peptides. FEBS J. (2009) 276:6483–96. doi: 10.1111/j.1742-4658.2009.07359.x
64. Kumar P, Kizhakkedathu JN, Straus SK. Antimicrobial peptides: diversity, mechanism of action and strategies to improve the activity and biocompatibility in vivo. Biomolecules. (2018) 8:4. doi: 10.3390/biom8010004
65. Rokitskaya TI, Kolodkin NI, Kotova EA, Antonenko YN. Indolicidin action on membrane permeability: Carrier mechanism versus pore formation. Biochim Biophys Acta Biomembr. (2011) 1808:91–7. doi: 10.1016/j.bbamem.2010.09.005
66. Vergis J, Malik SS, Pathak R, Kumar M, Ramanjaneya S, Kurkure NV, et al. Antimicrobial efficacy of indolicidin against multi-drug resistant enteroaggregative Escherichia coli in a galleria mellonella model. Front Microbiol. (2019) 10:2723. doi: 10.3389/fmicb.2019.02723
67. De Souza Cândido E, E Silva Cardoso MH, Sousa DA, Viana JC, De Oliveira-Júnior NG, Miranda V, et al. The use of versatile plant antimicrobial peptides in agribusiness and human health. Peptides. (2014) 55:65–78. doi: 10.1016/j.peptides.2014.02.003
68. Barksdale SM, Hrifko EJ, van Hoek ML. Cathelicidin antimicrobial peptide from Alligator mississippiensis has antibacterial activity against multi-drug resistant Acinetobacter baumanii and Klebsiella pneumoniae. Dev Comp Immunol. (2017) 70:135–44. doi: 10.1016/j.dci.2017.01.011
Keywords: antimicrobial peptides (AMPs), livestock, antimicrobial resistance (AMR), bacterial infection, alternative antimicrobial
Citation: Saeed SI, Mergani A, Aklilu E and Kamaruzzaman NF (2022) Antimicrobial Peptides: Bringing Solution to the Rising Threats of Antimicrobial Resistance in Livestock. Front. Vet. Sci. 9:851052. doi: 10.3389/fvets.2022.851052
Received: 08 January 2022; Accepted: 28 February 2022;
Published: 08 April 2022.
Edited by:
Nora Mestorino, National University of La Plata, ArgentinaReviewed by:
Wenyi Li, The University of Melbourne, AustraliaCopyright © 2022 Saeed, Mergani, Aklilu and Kamaruzzaman. This is an open-access article distributed under the terms of the Creative Commons Attribution License (CC BY). The use, distribution or reproduction in other forums is permitted, provided the original author(s) and the copyright owner(s) are credited and that the original publication in this journal is cited, in accordance with accepted academic practice. No use, distribution or reproduction is permitted which does not comply with these terms.
*Correspondence: Shamsaldeen Ibrahim Saeed, shams88ns@gmail.com; Nor Fadhilah Kamaruzzaman, norfadhilah@umk.edu.my