- State Key Laboratory of Crop Biology, College of Horticulture Science and Engineering, Shandong Agricultural University, Tai’an, China
In order to solve the problems of nitrogen (N) losses and fruit quality degradation caused by excessive N fertilizer application, different dosages of the nitrification inhibitor, 3,4-dimethylpyrazole phosphate (DMPP) (0, 0.5, 1, 2, and 4 mg kg–1 soil), were applied during the later stage of ‘Red Fuji’ apple (Malus domestica Borkh.) fruit expansion in 2017 and 2018. The effects of DMPP on soil N transformation, carbon (C)–N nutrition of tree, and fruit quality were investigated. Results revealed that DMPP decreased the abundance of ammonia-oxidizing bacteria (AOB) amoA gene, increased the retention of NH4+-N, and decreased NO3–-N concentration and its vertical migration in soil. DMPP reduced 15N loss rates and increased 15N residual and recovery rates compared to the control. 13C and 15N double isotope labeling results revealed that DMPP reduced the capacity of 15N absorption and regulation in fruits, decreased 15N accumulation in fruits and whole plant, and increased the distribution of 13C from vegetative organs to fruits. DMPP increased fruit anthocyanin and soluble sugar contents, and had no significant effect on fruit yield. The comprehensive analysis revealed that the application of 1 mg DMPP kg–1 soil during the later stage of fruit expansion effectively reduced losses due to N and alleviated quality degradation caused by excessive N fertilizer application.
Introduction
China possesses the largest apple cultivation area and production in the world (FAOSTAT, 2018). Unilateral pursuit of high yields and large fruits by fruit farmers, the excessive application of nitrogen (N) fertilizer has become a common problem in China. At present, the amount of N fertilizer application in apple orchards has reached 600 to 800 kg ha–1, which far exceeds the demands of plants (Ge et al., 2015; Zhu et al., 2018). Farmers have applied more N fertilizer during the early stages of the growing season in order to meet the growth and development needs of apple trees, although the utilization rate of N fertilizer in apple trees is generally low (5.2–31.3%) (Liu et al., 2010; Ding et al., 2017; Wang et al., 2020b). Most N fertilizer that is not absorbed has been left in the soil profile as inorganic N or organic combination forms and is integrated into the soil N pool (Ju, 2014).
During the later stage of apple fruit expansion, high temperature and rainy weather would lead to the mineralization of organic N in soil and to produce a large amount of ammonium N. And ammonium N can be easily transformed into nitrate N through nitrification (Dessureault-Rompré et al., 2010; Guntiñas et al., 2012). Nitrate N pollutes surface water and groundwater through surface runoff and leaching losses, as well as the atmosphere through denitrification (Vinzent et al., 2018; Wen et al., 2019). Additionally, nitrate N is more easily absorbed by apple trees than ammonium N (Li et al., 2013). Large amount of absorbed nitrate N would affect the carbon (C)–N balance of trees and result in excessive N in apple fruits. The imbalance of C and N in trees is not conducive to the flow of photosynthetic to fruits, and high N contents of fruits negatively affect fruits color, soluble solids, and other quality indicators (Wang et al., 2017; Wang X. F. et al., 2018; An et al., 2018; Zhang et al., 2020). Therefore, applying exogenous substances to inhibit soil nitrification is important for simultaneously controlling agricultural N pollution and improving fruit quality.
Nitrification inhibitors are widely used to delay the bacterial oxidation of NH4+ to nitrite (NO2–) by suppressing ammonia monooxygenase (AMO) activities in soil (Chen et al., 2010; Bell et al., 2016; Gilsanz et al., 2016). Recent researches about amoA gene, the gene that encodes the first subunit of AMO enzyme, revealed that ammonia-oxidizing archaea (AOA) and ammonia-oxidizing bacteria (AOB) play a major role in soil nitrification (Chen et al., 2015, 2019). Nitrification inhibitors decrease N losses by reducing NO3– leaching through the retention of N in low mobility forms (e.g., NH4+) and by decreasing N2O emissions through the reduction of NO3– concentrations for the denitrification process (Zhu et al., 2015; Friedl et al., 2017; Ni et al., 2018; Wang et al., 2020c). The new nitrification inhibitor, 3,4-dimethylpyrazole phosphate (DMPP), has many advantages, including application in small dosages, a long aging time, and non-toxicity, and it does not pollute the environment (Zerulla et al., 2001; Macadam et al., 2003). Therefore, DMPP has the potential to be a good nitrification inhibitor in agricultural management practices. A previous study found that DMPP decreased gross soil autotrophic nitrification rates and reduced gross mineralization rates through feedback regulation (Zhu et al., 2019). DMPP application reduced the risk of nitrate leaching and N losses due to denitrification and did not increase NH3 volatilization (Zerulla et al., 2001; Li et al., 2008). Yin et al. (2012) also found that the inhibition effects and associated time of DMPP on nitrification increased as the DMPP dosage increased, but when the dosage was >2%, the enhancement of the inhibition effect was no longer obvious.
Currently, researches on nitrification inhibitor DMPP are mainly focused on soil N transformation and N losses (Chen et al., 2019; Li et al., 2020b; Mateo-Marin et al., 2020; Vilarrasa-Nogue et al., 2020). Moreover, its effects on fruit quality and its application in apple orchards are rarely reported. Therefore, in this study, the effects of DMPP on apple soil mineral N, C–N nutrition of tree, and fruit quality were investigated to provide a reference for reducing N losses and improving fruit quality.
Materials and Methods
Experimental Site and Materials
This study was performed from 2017 to 2018 in an apple orchard located in Laishan, Yantai City, Shandong Province, Northeast China (121°43′00″E, 37°50′47″N). The climate is semi-humid with an annual average precipitation of 672.5 mm, of which nearly 70% occurs from June to September. The mean monthly rainfall and soil temperature at the 5 cm soil depth during the study are presented in Figure 1.
Trees were planted in 2012 in rows spaced 1.5 m apart with 4 m between rows and were trained as a slender spindle. The commercially important ‘Red Fuji’ apple cultivar (Malus × domestica Borkh.) was grafted on the dwarfing interstock, M.26, then grafted on M. hupehensis Rehd. rootstocks (‘Red Fuji’/M.26/M. hupehensis Rehd.). The basic physicochemical properties of the soil are presented in Table 1.
Experimental Design and Sampling
In this study, 30 trees with similar growth potential were selected and treated with repeated applications of five treatments in 2017 and 2018. The treatments included Treatment 1: Control (0 mg DMPP kg–1 soil; water as control), Treatment 2: T1 (0.5 mg DMPP kg–1 soil), Treatment 3: T2 (1 mg DMPP kg–1 soil), Treatment 4: T3 (2 mg DMPP kg–1 soil), and Treatment 5: T4 (4 mg DMPP kg–1 soil). In each treatment, 0, 0.42, 0.85, 1.70, and 3.39 g DMPP plant–1 was applied. The dosage to each tree was calculated by the mass of soil at the 60 cm depth within the projected area of the tree crown. Treatments were conducted during the later stage of fruit expansion (105 days after blooming). The application method was as follows: DMPP was dissolved in 4 L of water and evenly distributed in 12 spots within the projected area occupied by the crown of a single tree, then DMPP was applied to 12 spots using a fertilizer gun at the 10, 30, and 50 cm soil depths.
Based on isotope labeling, each treatment was divided into two groups with three replicates per group and two trees per replicate as follows. Group 1: 340 g normal urea (CO(NH2)2), 210 g ammonium phosphate ((NH4)2HPO4), and 120 g potassium sulfate (K2SO4) were applied to each tree as the non-labeled group, where 50% of the fertilizer was applied at the germination stage and 50% as fruit setting fertilizer (40 days after blooming). Group 2: 10 g 15N-urea (CO(15NH2)2 produced by the Shanghai Research Institute of Chemical Industry, 10.22% abundance), 330 g normal CO(NH2)2, 210 g (NH4)2HPO4, and 120 g K2SO4 were applied to each tree as the labeled group, where 50% of the fertilizer was applied at the germination stage and 50% as fruit setting fertilizer (40 days after blooming). Subsequently, 13C pulse labeling was performed in a labeling chamber 182 days after blooming in 2017 and 2018. Fertilizer was applied by digging a circular trench with a 30-cm radius around each tree and a width and depth of 20 cm. The growth conditions, cultivation, and management of all treatments were consistent across treatments and years.
Soil samples were obtained 20, 40, 60, and 80 days after DMPP application. The soil sampling method was as follows: 12 sampling points were evenly distributed throughout the projected area of the tree canopy occupied by a single tree; the soil sample depths of 0–20, 20–40, 40–60, 60–80, and 80–100 cm were retrieved in the vertical direction of each soil extraction point; 12 soil samples per layer were evenly mixed as one replicate. After collection, soil samples were immediately transferred to the laboratory to determine gene abundance, mineral N (NH4+-N and NO3–-N) contents and 15N residues (calculated to the 60 cm depth). All plants were subjected to destructive sampling at the fruit maturity stage (185 days after blooming). Fruits were selected from four directions in the middle of the outer part of the crown with 12 fruits in each tree. Fruit peels and flesh were immediately frozen in liquid N and stored at −80°C for further analysis.
13C Labeling Method
The 13C labeling method used in this study was previously described by Wang et al. (2020a). Each tree of Group 2 was individually covered and sealed by a labeling chamber, which was composed of 0.1-mm-thick Mylar plastic bags and brackets. Put fans and beaker contained with 10 g of Ba13CO3 (13C abundance is 98%) into the labeling room, turned on the fans and sealed the labeling chamber. Labeling work started at 8:00 am (182 days after blooming). 1 mL of hydrochloric acid (1 mol L–1) was injected into the beaker with a syringe every 0.5 h in order to maintain the concentration of 13CO2, 13C labeling process lasted for 4 h. In order to prevent excessive temperature during the labeling process, appropriate amount of ice bag was added to the bottom of labeling chamber to control the temperature in the range of 28–37°C. All trees were destructively sampled after 72 h (185 days after blooming). The trees of Group 1 were destructively sampled and used as a blank for 13C labeling (natural abundance of 13C).
DNA Extraction and Quantitative PCR of AOA and AOB amoA Genes
The 0–60 cm soil sample of each tree is mixed as one replicate to measure the abundance of AOA and AOB amoA genes. DNA was extracted using the FastDNA SPIN Kit for soil (Bio101, Vista, CA, United States) according to the manufacturer’s instructions. Real-time quantitative PCR of amoA genes was according to Chen et al. (2019). The details of primers, reaction mixture compositions, and thermal cycling conditions are listed in Supplementary Table S1.
Concentrations of Soil Ammonium and Nitrate
Soil ammonium and nitrate were extracted with 0.01 M KCl and analyzed using a San++ continuous flow analyzer (Skalar Analytical, Breda, Netherlands) (Duan et al., 2015).
Ammonia Volatilization
Ammonia volatilization was measured every 10 days after DMPP application. Twelve ammonia volatilization measurement points were evenly distributed in each tree disk. The average of 12 results was used as one replicate. Ammonia volatilization was measured using the ventilation method (Li et al., 2020a). A PVC collection tube (0.20 m diameter, 0.25 m height) was inserted into the soil at a depth of 0.05 m with a phosphoglycerol-soaked sponge placed inside as an absorbent, which was collected (and replaced) daily (10:00 am) throughout the experiment period. The phosphoglycerol-soaked sponges bearing the collected samples were transported to the laboratory and immediately immersed in 500 mL 1.0 mol L–1 KCl solution in 1 L polyethylene bottles. Bottles were sealed and shaken at 200 rpm for 1 h on a reciprocating shaker. The NH4+-N concentrations of the extracted solutions from each bottle were measured by colorimetry (λ = 630 nm) using a UV-VIS spectrophotometer (Unico, Shanghai, China). The NH3 volatilization rates were calculated as follows: RAV = M/(A × D) × 10–2, where RAV is the NH3 volatilization rate (kg N ha–1 d–1), M is the amount of NH3-N collected in the sponge (mg), which is equal to the NH4+-N contents of the extracted solutions, A is the cross-sectional area of the sponge (m2), and D is the interval of sample collection (d).
Contents of 15N and 13C
The whole plant samples were divided into fruits, leaves, annual branches, perennial branches, trunk, and roots. The samples were heated at 105°C for 30 min and then dried at 80°C, followed with homogenization by an electric grinder and filtration with a 0.25 mm mesh screen (Liu et al., 2017). The samples of Group 2 were used to determine the abundance of 15N and 13C and the content of N, and those of Group 1 were used to determine the natural abundance of 13C as a blank control of the corresponding organs of Group 2. The content of N was determined by the Kjeldahl method (Wang et al., 2019), and the abundance of 15N was measured with a ZHT-03 mass spectrometer made in the Beijing Analytical Instrument Factory (Chinese Academy of Agricultural Sciences). The abundance of 13C was measured with a DELTAVplusXP advantage isotope ratio mass spectrometer and analyzed by the China Academy of Forestry Sciences Stable Isotope Laboratory. Three replicates were conducted for each treatment.
Calculation of 15N
Calculation of 13C
Fnl: no 13C labeling, natural abundance of 13C of each organ
Fruit Quality
The total anthocyanin content of apple peels was measured according to (Sun et al., 2019) with minor modifications. Each sample (0.5 g) was ground to a powder in liquid N and incubated in 5 mL 1% (v/v) HCl-methanol for 24 h at 4°C in total darkness. After centrifugation, KCl and NaAc buffers were added to the supernatant aliquots, which were mixed and incubated for 20 min at 4°C in total darkness. Solutions were centrifuged at 8000 rcf (× g) for 15 min. The absorbance of the supernatant was measured using a UV-2450 spectrophotometer (Shimadzu, Kyoto, Japan) at 510 and 700 nm (i.e., OD510 and OD700).
The content of soluble sugars was measured using Anthrone colorimetry (Liu et al., 2018). Samples were placed in a test tube, to which 5 mL distilled water was added and mixed after cutting samples into pieces. After 30 min boiling in a water bath, the supernatant was collected. This step was repeated twice, and the volume of the solution was adjusted to 10 mL using distilled water. The absorbance of the solution was determined at 630 nm after adding sulfuric acid and anthrone. The contents of titratable acid were measured by the NaOH titration method (Wang X. et al., 2018). Each treatment had a total of three replicates.
Statistical Analysis
All the graphs were plotted by Origin 8.0 (OriginLab Corporation, Northampton, MA, United States). Data were analyzed with the IBM SPSS Statistics for Windows Version 19.0 (IBM Corporation, Armonk, NY, United States) by using one-way factorial analysis of variance (ANOVA). In all cases, differences were considered significant at a probability level of P < 0.05.
Results
Soil N Transformation
Abundance of AOA and AOB amoA Genes
The copy numbers of AOA and AOB were assessed via qPCR of their respective amoA genes in Figure 2. In general, the abundance of AOB amoA gene was higher (2.84 × 108 to 8.01 × 108) than that of AOA amoA gene (3.41 × 106 to 7.19 × 106). Over time, the abundance of AOA and AOB amoA genes increased first and then decreased, and reached the highest at 40 days. The AOA amoA gene abundance increased as the DMPP dosage increased at 20 days and 40 days. DMPP decreased AOB amoA gene abundance in different degrees compared to the control at 20 and 40 days, and decreased as the DMPP dosage increased. After 60 days, DMPP had no significant effect on the abundance of AOA and AOB amoA genes in each treatment (p > 0.05). The results showed that AOB population size was greater than AOA in the apple orchard, and DMPP inhibits the ammoxidation process by decreasing the abundance of AOB amoA gene.
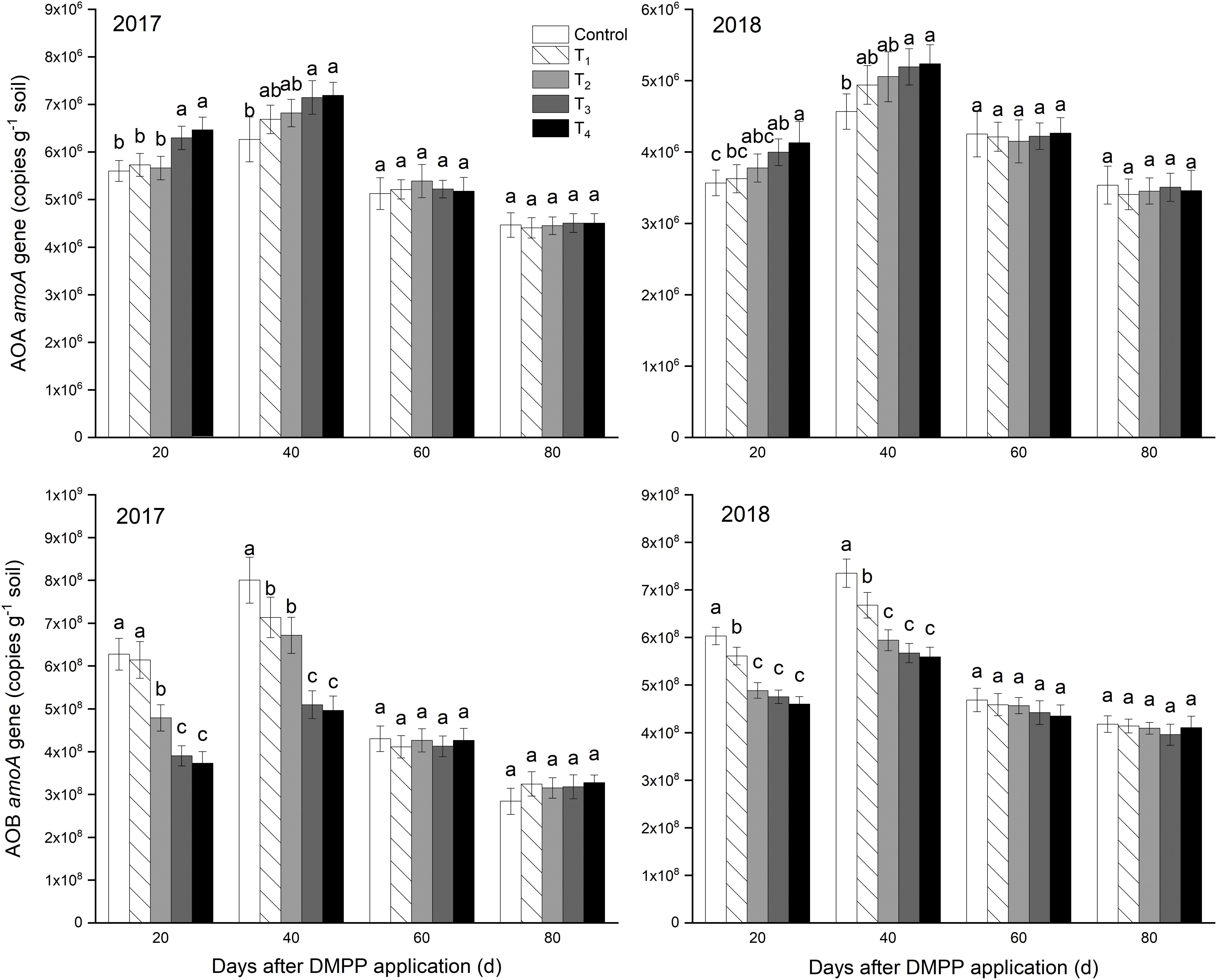
Figure 2. Abundances of amoA genes of ammonia-oxidizing archaea (AOA) and bacteria (AOB). The vertical bar indicates the standard deviation of three replications. Different letters indicate statistically significant differences (P < 0.05).
Concentrations of Soil Mineral N (NH4+-N and NO3–-N)
The trends of NH4+-N concentrations in each treatment were similar (Figure 3). In 2017 and 2018, the NH4+-N concentrations of the 0–60 cm soil layer were high, while those of the 60–100 cm soil layer were low, exhibiting a high to low distribution. The concentrations of NH4+-N in the soil increased within 60 days after DMPP application. The concentrations of NH4+-N increased as the DMPP dosage increased in the 0–60 cm soil layer, but no obvious differences were detected among the treatments in the 60–100 cm soil layer. No significant differences were detected in the NH4+-N concentrations among treatments after 60 days (p > 0.05; Figure 3).
Over time, NO3–-N concentrations of control exhibited a vertical migration trend in 2017 and 2018 (Figure 4). The NO3–-N concentrations of the 0–60 cm soil layer decreased within 60 days after DMPP application and decreased as the DMPP dosage increased. No obvious differences were detected in the NO3–-N concentrations among treatments after 60 days. Therefore, DMPP treatment effectively inhibited the production of NO3–-N in the 0–60 cm soil layer and reduced the risk of NO3–-N vertical migration.
NH3 Volatilization Rates and Cumulative NH3 Volatilization
Over time, NH3 volatilization rates increased at first then decreased (Figure 5). Differences in the NH3 volatilization rates among treatments gradually decreased over time, and these differences were not obvious after 60 days. DMPP application increased NH3 volatilization rates and cumulative NH3 volatilization compared to the control, and both increased as the DMPP dosage increased (Figure 5). Compared to the control, the differences in cumulative NH3 volatilization were not significant (p > 0.05) when the DMPP dosage was low (T1 and T2), but that was significant (p < 0.05) when the DMPP dosage was high (T3 and T4).
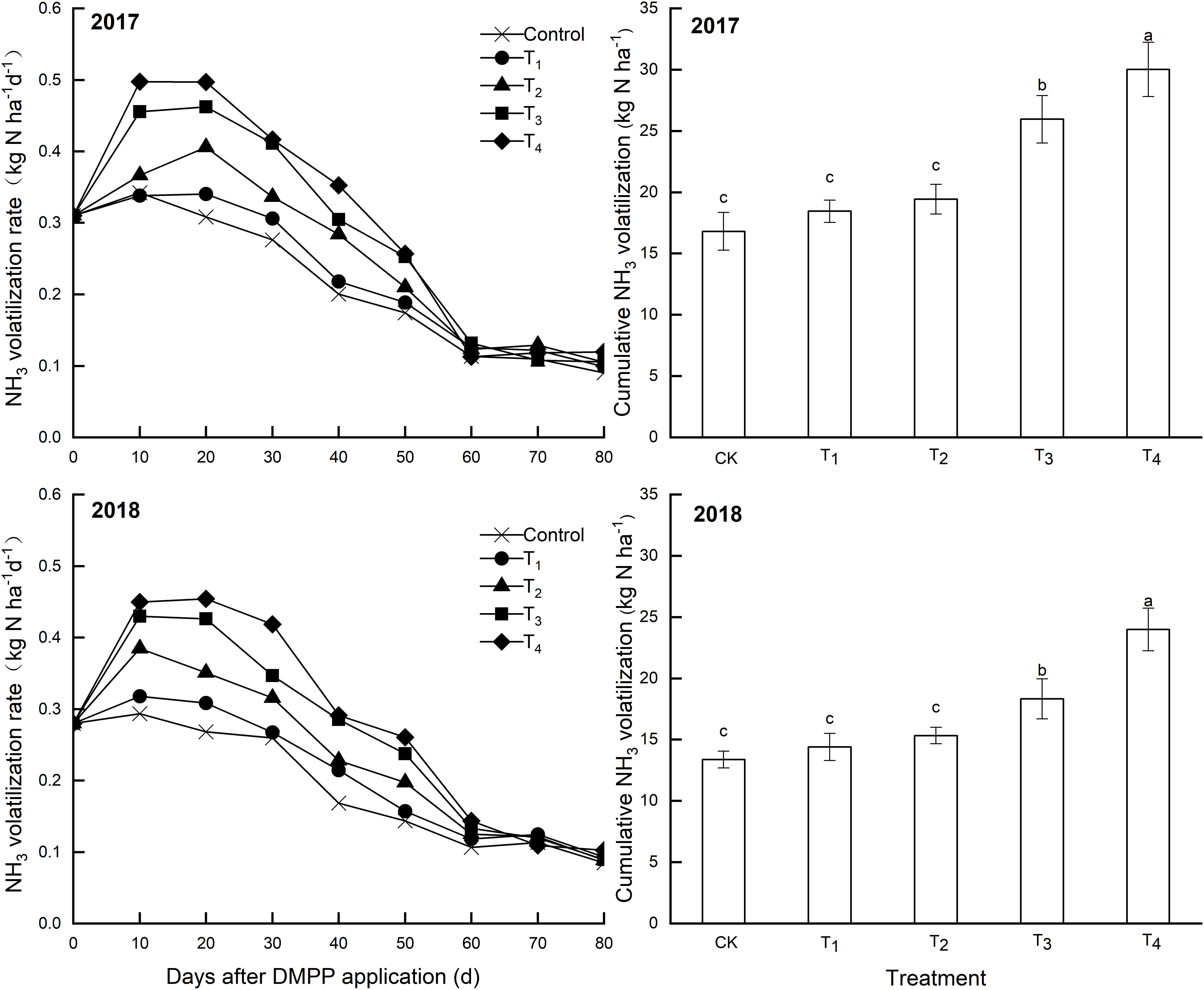
Figure 5. Effects of DMPP on the NH3 volatilization rate and cumulative NH3 volatilization in 2017 and 2018. The vertical bar indicates the standard deviation of three replications. Different letters indicate statistically significant differences (P < 0.05).
The Utilization, Residue and Loss of 15N
According to the roots distribution of dwarf apple, 15N within the 0–60 cm soil layers was considered to be soil 15N residue, while 15N in other soil layers means 15N loss. DMPP reduced the 15N utilization rate to varying degrees (Table 2). As the DMPP dosage increased, the 15N utilization rate exhibited a downward trend. DMPP application increased the 15N residual and recovery rates, both of which reached the highest in T2. Compared to the control, the application of DMPP reduced 15N loss rate and the lowest value appeared in T2.
Plant Organ Ndff and N Content
Ndff refers to the 15N contribution rate absorbed from fertilizer and distributed by plant organs relative to the total N of plant organs, and reflects the ability of plant organs to absorb and regulate 15N fertilizer. The Ndff of organs subjected to different treatments were consistent at the fruit maturity stage across both years (Figure 6). In each treatment, the Ndff values were ordered as follows: fruits > annual branches > leaves > roots > perennial branches > trunk. Compared to the control, DMPP application reduced the Ndff of fruits, annual branches, leaves, and roots, which decreased as the DMPP dosage increased. No significant differences were detected on the Ndff of perennial branches and trunk among different treatments (p > 0.05). Results revealed that fruits absorbed and regulated 15N the most at the fruit maturity stage, while the annual branches and leaves also exhibited strong competitiveness. DMPP application reduced the ability of newborn organs to absorb and regulate 15N.
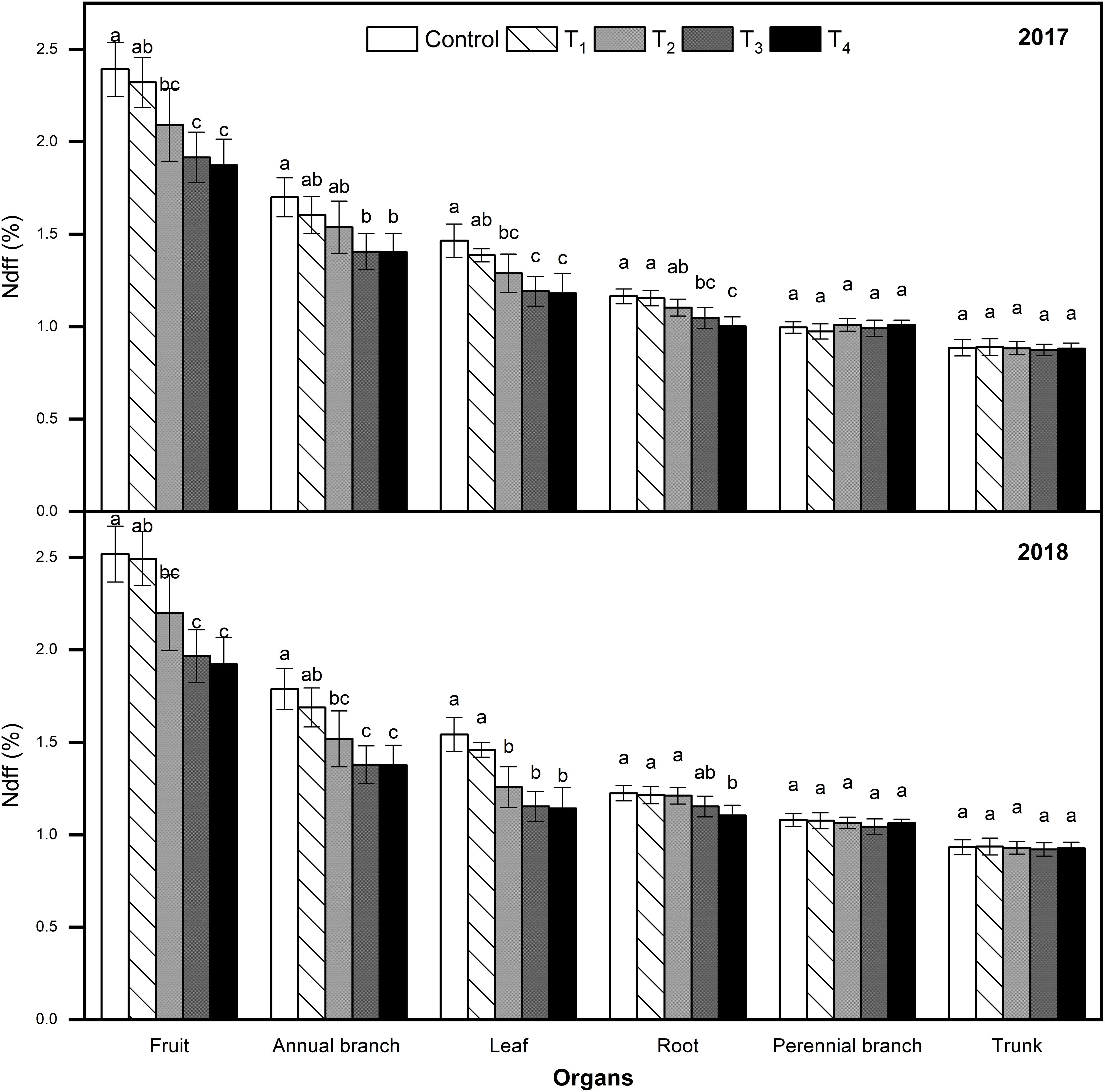
Figure 6. Effects of DMPP on Ndff value at the fruit maturity stage in 2017 and 2018. The vertical bar indicates the standard deviation of three replications. Different letters indicate statistically significant differences (P < 0.05).
Accumulated 15N in the whole plant and fruits decreased gradually as the DMPP dosage increased (Table 3). Compared to the control, fruit 15N accumulation with DMPP application decreased by 10.53%–26.32% and 5.26%–15.79% in 2017 and 2018, respectively. Meanwhile, N contents in leaves and fruits also decreased gradually as the DMPP dosage increased (Table 3).
13C Distribution Rate and 13C Accumulation in Fruits
The proportion of 13C assimilates assigned to each organ is related to its competitive ability, which refers to the ability to absorb 13C from the leaves of active metabolic and growth organs. The 13C distribution rates for each treatment were consistent across both years, among which fruits had the highest value followed by leaves, roots, perennial branches, and trunk (Figure 7). DMPP increased the 13C distribution rate in fruits, which increased first and then decreased as the DMPP dosage increased. The highest 13C distribution rate in fruits appeared in T2, and the value increased by 10.36% and 10.87% compared to the control in 2017 and 2018, respectively. With an increasing of DMPP application rate, the 13C distribution rate in leaves and annual branches initially decreased and then increased, and the lowest value appeared in T2. No significant effect was observed on the 13C distribution rate in storage organs (roots, perennial branches, and trunk) (p > 0.05). Therefore, DMPP improved the competitiveness of fruits with respect to 13C and promoted 13C transportation from the vegetative organs (leaves and annual branches) to the fruits (Figure 7). Additionally, DMPP increased the 13C accumulation in fruits, and the highest value appeared in T2 treatment (Table 3).
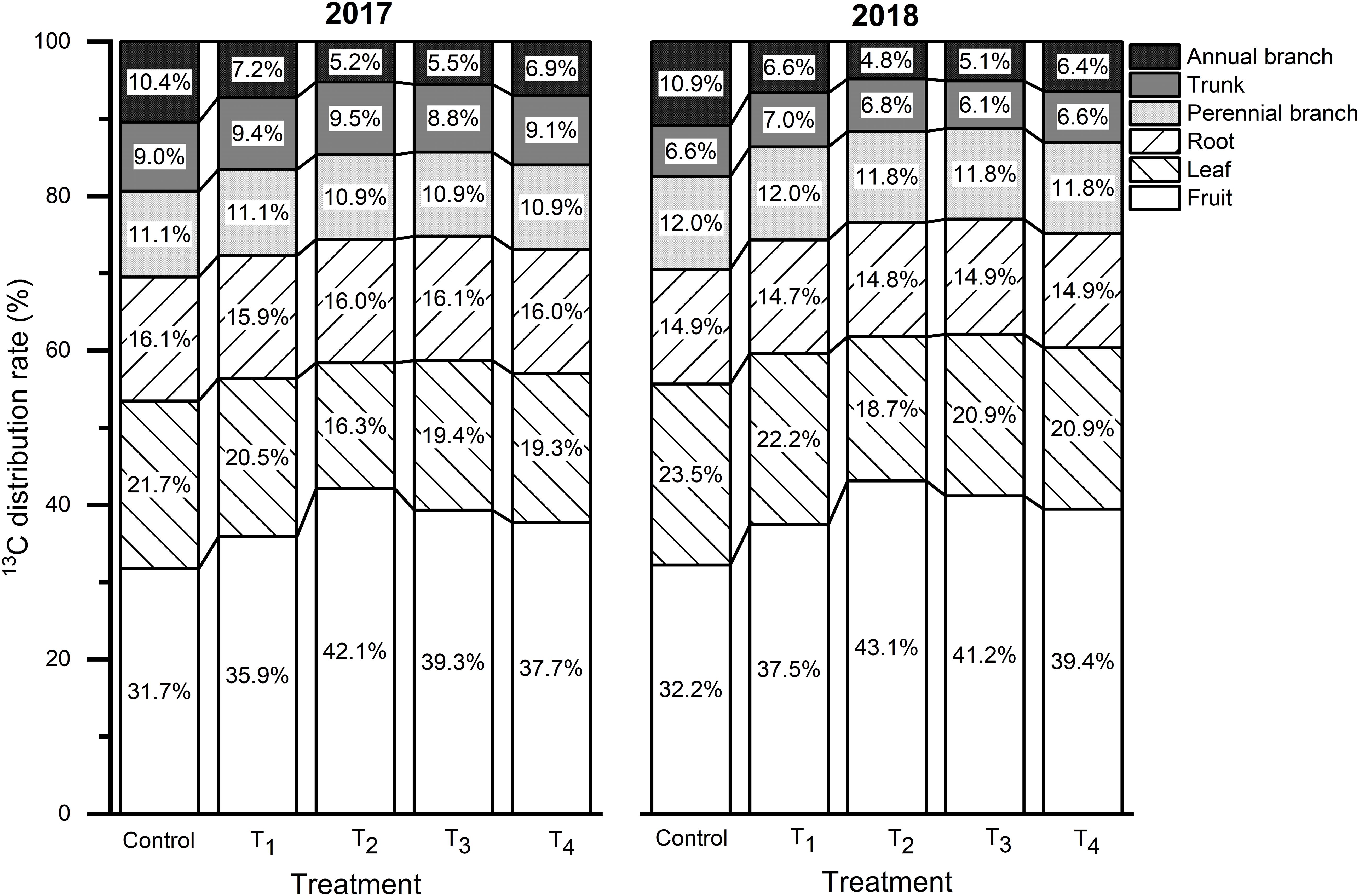
Figure 7. Effects of DMPP on the 13C distribution rate at the fruit maturity stage in 2017 and 2018 (13C distribution rate refers to the ratio of the 13C content of each organ to the amount of net 13C absorbed by the plant).
Fruit Yield and Quality
No significant effect was observed on fruit yield after DMPP application (p > 0.05; Table 4). However, DMPP had a positive effect on fruit quality. With an increasing of DMPP application rate, the anthocyanin contents of apple peels increased at first then decreased (Table 4). Compared to the control, the anthocyanin contents of T2 were the highest, which increased by 50.06% and 49.58% in 2017 and 2018, respectively. The trends of soluble sugar contents were consistent with anthocyanin contents. For titratable acids contents in fruits, the value decreased as the DMPP dosage increased. The sugar-acid ratio of T2 was the highest, and was 32.38% and 34.45% higher compared to the control in 2017 and 2018, respectively. Overall, appropriate DMPP application rate significantly improved fruit quality.
Discussion
Effects of DMPP on Soil N Transformation and N Loss
Li et al. (2008) found that DMPP enhanced NH4+-N concentrations but reduced NO3–-N concentrations in the leachate and soil, as well as decreased the AOB population and soil nitrate reductase activities. This study found that DMPP inhibited the ammoxidation process by decreasing the abundance of AOB amoA gene. DMPP increased the abundance of AOA amoA gene, which is consistent with the results of Kleineidam et al. (2011). This may be related to DMPP changing the soil pH and microbial community structure (Li et al., 2011; Cao et al., 2018). The NH4+-N concentrations of the 0–60 cm soil layer were higher than that in the 60–100 cm soil layer, exhibiting a high to low distribution. This result was mainly due to the strong NH4+-N adsorption abilities of soil organic matter and colloidal particles; however, NH4+-N adsorption generally does not occur during vertical migration. Compared to the control, DMPP application decreased the concentration of NO3–-N and its vertical migration. Therefore, it was determined that DMPP could be used as an effective nitrification inhibitor to control ammonium oxidation and decrease soil NO3–-N concentration and its vertical migration, thereby minimizing shallow groundwater pollution risk. These findings are consistent with the results of Yu et al. (2007).
Nitrification inhibitors effectively prevent the occurrence of nitrification reactions. The mineralization of soil N is also enhanced by soil microorganisms, which results in the maintenance of soil ammonia N concentrations at higher levels. Therefore, the ammonia concentration gradient at the soil air interface is large, the ammonia diffusion ability is strong, and the rate of ammonia gas runaway is fast (Pinheiro et al., 2018). In this study, DMPP application increased NH3 volatilization rates and cumulative NH3 volatilization, and both increased as the DMPP dosage increased. Cumulative NH3 volatilization at high DMPP dosages was significantly different compared to the control. Overall, results revealed that the risk of soil ammonia volatilization significantly increased after DMPP reached a certain dosage. The prevention and control measures of ammonia volatilization after DMPP application need further study.
Previous studies found that DMPP increased fertilizer N and soil N recovery and decreased N runoff loss, which was beneficial to the ecological environment (Li et al., 2008; Yu et al., 2015; Alonso-Ayuso et al., 2016). Quemada et al. (2013) conducted a meta-analysis on irrigated agricultural systems and found that the use of nitrification inhibitors reduced nitrate leaching by 27% compared to conventional fertilizers. We found that DMPP reduced 15N loss rates, as well as increased 15N residual and recovery rates. DMPP application reduced 15N utilization rates but increased 15N residual rates during the growing season. Over time, DMPP was beneficial to the maintenance of the soil N pool, sustainable soil N supply capacity, and soil N absorption and utilization by trees during the subsequent growing season. However, a previous study found that DMPP significantly increased urea-N loss, which was due to the abundant 15NH4+-N retention and absence of plants in the soil condition with a high pH (Xu et al., 2019). These findings suggest that different environmental parameters (e.g., moisture, temperature, soil texture, pH, and the quality and quantity of soil organic matter) are modulated by the climate and agricultural management strategies, which should be considered when applying nitrification inhibitors (Niu et al., 2018; Zhang et al., 2019).
Effects of DMPP on Tree C–N Nutrition and Fruit Quality
During the later stage of apple fruit expansion, the nutrients absorbed by trees mainly supplied fruit development. If the autumn shoots grew too much during this time, it led to nutrient dispersion, which thereby affected fruit quality. In this study, DMPP reduced autumn shoot length compared to the control (Supplementary Table S2). As the DMPP dosage increased, autumn shoot length gradually decreased. The growth of autumn shoots of control was too large, and the excessive growth of autumn shoots consumed several nutrients, which led to limited reproductive growth and was not conducive to fruit development.
C and N metabolism is the most basic metabolic process during fruit growth and development. C metabolism serves as a C source and provides energy for N metabolism, while N metabolism provides enzymes and photosynthetic pigments for C metabolism. The degree of coordination between C and N metabolism and their transformation directly and indirectly affect fruit quality. The late growth stage of apple trees is the key time when fruits convert from N to C nutrients. However, high temperature and rainy weather during this time lead to large soil NO3–-N supplies, resulting in vigorous N metabolism in tree. In this study, fruit N contents of control were 2.72 and 2.75 g kg–1 in 2017 and 2018, respectively (Table 3), which were higher than the optimal N contents of high-quality apple fruits (Zhang et al., 2017). High fruit N could reduce the activity of sugar metabolism enzymes in fruits, decrease the strength of fruit sink, and affect the transportation of carbohydrates to fruits, thus detrimentally affecting fruit quality (Kühn et al., 2011; Sha et al., 2019; Wang et al., 2020a). Therefore, coordinating C–N nutrition would benefit and improve fruit quality. In this study, the 13C and 15N double isotope labeling technology results revealed that DMPP reduced the capacity of 15N absorption and regulation in fruits, decreased 15N accumulation in fruits and whole plant, and improved the distribution of 13C from vegetative organs to fruits. Martínez et al. (2017) found that DMPP increased strawberry antioxidant compound contents, including vitamin C and total phenolics, and increased fruit quality. Yu et al. (2018) also reported that DMPP could improve pakchoi cabbage quality by regulating N transformation and heavy metal absorption. Consistent with previous results, we found that appropriate DMPP application dosages significantly improved fruit anthocyanin contents, soluble sugar contents, and sugar-acid ratios.
Nitrogen contents affect the distribution and accumulation of C in plant organs (Wang et al., 2020a). Our results found that higher leaves and fruits N contents in control and T1 inhibited C accumulation in fruits, which negatively affected fruit quality. Leaves and fruits N contents of T3 and T4 were lower, which resulted in deficient C metabolism precursors and affected the formation of fruit quality. T2 was more conducive to the transformation of C and N nutrition, C assimilate accumulation, and fruit quality improvement (Tables 3, 4 and Figures 6, 7).
Conclusion
Appropriate DMPP application dosages could decrease the abundance of AOB amoA gene and the vertical migration of nitrate, thereby minimizing the risk of shallow groundwater pollution. Moreover, DMPP application reduced extravagant absorption of N in newborn organs, which in turn regulated the distribution and accumulation of C in fruits, so as to promote fruit quality. On the basis of our results, the application of 1 mg DMPP kg–1 soil during the later stage of fruit expansion could regulate soil N transfer and transformation, the C–N nutrition of trees, and effectively address the problems of N losses and fruit quality degradation caused by excessive N fertilizer application.
Data Availability Statement
All datasets presented in this study are included in the article/Supplementary Material.
Author Contributions
FW and YJ conceived and designed the experiments. FW, XX, XH, and ZJ performed the experiments. FW, SG, and ZZ wrote the manuscript. All authors have read and approved the final version of the manuscript.
Funding
This work was supported by the Special Fund for the National Key R&D Program of China (2016YFD0201100), National Natural Science Foundation of China (31501713), China Agriculture Research System (CARS-27), and Taishan Scholar Assistance Program from Shandong Provincial Government.
Conflict of Interest
The authors declare that the research was conducted in the absence of any commercial or financial relationships that could be construed as a potential conflict of interest.
Acknowledgments
The authors would like to thank Dr. Han Jiang for improving the English of the manuscript.
Supplementary Material
The Supplementary Material for this article can be found online at: https://www.frontiersin.org/articles/10.3389/fpls.2020.00764/full#supplementary-material
References
Alonso-Ayuso, M., Gabriel, J. L., and Quemada, M. (2016). Nitrogen use efficiency and residual effect of fertilizers with nitrification inhibitors. Eur. J. Agron. 80, 1–8. doi: 10.1016/j.eja.2016.06.008
An, J. P., An, X. H., Yao, J. F., Wang, X. N., You, C. X., Wang, X. F., et al. (2018). BTB protein MdBT2 inhibits anthocyanin and proanthocyanidin biosynthesis by triggering MdMYB9 degradation in apple. Tree Physiol. 38, 1578–1587. doi: 10.1093/treephys/tpy063
Bell, M. J., Cloy, J. M., Topp, C. F. E., Ball, B. C., Bagnall, A., Rees, R. M., et al. (2016). Quantifying N2O emissions from intensive grassland production: the role of synthetic fertilizer type, application rate, timing and nitrification inhibitors. J. Agr. Sci. 154, 812–827. doi: 10.1017/s0021859615000945
Cao, H., Feng, F., Xun, M., Huang, P., Li, Y. G., Ji, T., et al. (2018). Effect of carbonized apple wood on nitrogen-transforming microorganisms and nitrogen oxides in soil of apple tree root zone. Eur. J. Soil Sci. 69, 545–554. doi: 10.1111/ejss.12532
Chen, D., Suter, H. C., Islam, A., and Edis, R. (2010). Influence of nitrification inhibitors on nitrification and nitrous oxide (N2O) emission from a clay loam soil fertilized with urea. Soil Biol. Biochem. 42, 660–664. doi: 10.1016/j.soilbio.2009.12.014
Chen, H., Yin, C., Fan, X., Ye, M., Peng, H., Li, T., et al. (2019). Reduction of N2O emission by biochar and/or 3,4-dimethylpyrazole phosphate (DMPP) is closely linked to soil ammonia oxidizing bacteria and nosZI-N2O reducer populations. Sci. Total Environ. 694:133658. doi: 10.1016/j.scitotenv.2019.133658
Chen, Q., Qi, L., Bi, Q., Dai, P., Sun, D., Sun, C., et al. (2015). Comparative effects of 3,4-dimethylpyrazole phosphate (DMPP) and dicyandiamide (DCD) on ammonia-oxidizing bacteria and archaea in a vegetable soil. Appl. Microbiol. Biotechnol. 99, 477–487. doi: 10.1007/s00253-014-6026-7
Dessureault-Rompré, J., Zebarth, B. J., Georgallas, A., Burton, D. L., Grant, C. A., and Drury, C. F. (2010). Temperature dependence of soil nitrogen mineralization rate: comparison of mathematical models, reference temperatures and origin of the soils. Geoderma 157, 97–108. doi: 10.1016/j.geoderma.2010.04.001
Ding, N., Chen, Q., Zhu, Z., Peng, L., Ge, S., and Jiang, Y. (2017). Effects of crop load on distribution and utilization of 13C and 15N and fruit quality for dwarf apple trees. Sci. Rep. 7:14172. doi: 10.1038/s41598-017-14509-3
Duan, W., Shi, Y., Zhao, J., Zhang, Y., and Yu, Z. (2015). Depth of nitrogen fertiliser placement affects nitrogen accumulation, translocation and nitrate-nitrogen content in soil of rainfed wheat. Int. J. Plant Prod. 9, 237–256.
FAOSTAT (2018). Statistical Database of the Food and Agricultural Organization of the United Nations. Rome: FAOSTAT.
Friedl, J., Scheer, C., Rowlings, D. W., Mumford, M. T., and Grace, P. R. (2017). The nitrification inhibitor DMPP (3,4-dimethylpyrazole phosphate) reduces N2 emissions from intensively managed pastures in subtropical Australia. Soil Biol. Biochem. 108, 55–64. doi: 10.1016/j.soilbio.2017.01.016
Ge, S. F., Jiang, Y. M., and Wei, S. C. (2015). Gross nitrification rates and nitrous oxide emissions in an apple orchard soil in northeast China. Pedosphere 25, 622–630. doi: 10.1016/s1002-0160(15)30042-4
Gilsanz, C., Báez, D., Misselbrook, T. H., Dhanoa, M. S., and Cárdenas, L. M. (2016). Development of emission factors and efficiency of two nitrification inhibitors. DCD and DMPP. Agric. Ecosyst. Environ. 216, 1–8. doi: 10.1016/j.agee.2015.09.030
Guntiñas, M. E., Leirós, M. C., Trasar-Cepeda, C., and Gil-Sotres, F. (2012). Effects of moisture and temperature on net soil nitrogen mineralization: a laboratory study. Eur. J. Soil Biol. 48, 73–80. doi: 10.1016/j.ejsobi.2011.07.015
Ju, X. (2014). Direct pathway of nitrate produced from surplus nitrogen inputs to the hydrosphere. Proc. Natl. Acad. Sci. U.S.A. 111:E416. doi: 10.1073/pnas.1321334111
Kleineidam, K., Kosmrlj, K., Kublik, S., Palmer, I., Pfab, H., Ruser, R., et al. (2011). Influence of the nitrification inhibitor 3,4-dimethylpyrazole phosphate (DMPP) on ammonia-oxidizing bacteria and archaea in rhizosphere and bulk soil. Chemosphere 84, 182–186. doi: 10.1016/j.chemosphere.2011.02.086
Kühn, B. F., Bertelsen, M., and Sorensen, L. (2011). Optimising quality-parameters of apple cv. ‘Pigeon’ by adjustment of nitrogen. Sci. Hortic. 129, 369–375. doi: 10.1016/j.scienta.2011.03.033
Li, H., Liang, X., Chen, Y., Lian, Y., Tian, G., and Ni, W. (2008). Effect of nitrification inhibitor DMPP on nitrogen leaching, nitrifying organisms, and enzyme activities in a rice-oilseed rape cropping system. J. Environ. Sci. 20, 149–155. doi: 10.1016/s1001-0742(08)60023-6
Li, J., Jiang, Y., Men, Y., Li, H., Zhou, L., and Wei, S. (2013). Effects of ammonium and nitrate nitrogen on growth and properties of 15N distribution of apple trees. Sci. Agric. Sin. 46, 3818–3825. doi: 10.3864/j.issn.0578-1752.2013.18.010
Li, X., Ying, J., Chen, Y., Zhang, L., Gao, Y., and Bai, Y. (2011). Effects of nitrogen addition on the abundance and composition of soil ammonia oxidizers in Inner Mongolia Grassland. Acta Ecol. Sin. 31, 174–178. doi: 10.1016/j.chnaes.2011.03.009
Li, Y., Shah, S. H. H., and Wang, J. Y. (2020a). Modelling of nitrification inhibitor and its effects on emissions of nitrous oxide (N2O) in the UK. Sci. Total Environ. 709:136156. doi: 10.1016/j.scitotenv.2019.136156
Li, Y., Xu, J., Liu, S., Qi, Z., Wang, H., Wei, Q., et al. (2020b). Salinity-induced concomitant increases in soil ammonia volatilization and nitrous oxide emission. Geoderma 361:114053. doi: 10.1016/j.geoderma.2019.114053
Liu, H., Fu, Y., Hu, D., Yu, J., and Liu, H. (2018). Effect of green, yellow and purple radiation on biomass, photosynthesis, morphology and soluble sugar content of leafy lettuce via spectral wavebands “knock out”. Sci. Hortic. 236, 10–17. doi: 10.1016/j.scienta.2018.03.027
Liu, L., Peng, F., and Wang, X. (2010). Effects of bag-controlled release fertilizer on nitrogen utilization rate, growth and fruiting of the ‘Fuji’ apple. J. Plant Nutr. 33, 1904–1913. doi: 10.1080/01904167.2010.512050
Liu, L., Xiao, W., Li, L., Li, D. M., Gao, D. S., Zhu, C. Y., et al. (2017). Effect of exogenously applied molybdenum on its absorption and nitrate metabolism in strawberry seedlings. Plant Physiol. Biochem. 115, 200–211. doi: 10.1016/j.plaphy.2017.03.015
Macadam, X. M., Prado, A., Merino, P., Estavillo, J. M., Pinto, M., and Gonzalez-Murua, C. (2003). Dicyandiamide and 3,4-dimethyl pyrazole phosphate decrease N2O emissions from grassland but dicyandiamide produces deleterious effects in clover. J. Plant Physiol. 160, 1517–1523. doi: 10.1078/0176-1617-01006
Martínez, F., Palencia, P., Alonso, D., and Oliveira, J. A. (2017). Advances in the study of nitrification inhibitor DMPP in strawberry. Sci. Hortic. 226, 191–200. doi: 10.1016/j.scienta.2017.07.046
Mateo-Marin, N., Quilez, D., Guillen, M., and Isla, R. (2020). Feasibility of stabilised nitrogen fertilisers decreasing greenhouse gas emissions under optimal management in sprinkler irrigated conditions. Agric., Ecosyst. Environ. 290:106725. doi: 10.1016/j.agee.2019.106725
Ni, K., Kage, H., and Pacholski, A. (2018). Effects of novel nitrification and urease inhibitors (DCD/TZ and 2-NPT) on N2O emissions from surface applied urea: an incubation study. Atmos. Environ. 175, 75–82. doi: 10.1016/j.atmosenv.2017.12.002
Niu, Y., Luo, J., Liu, D., Müller, C., Zaman, M., Lindsey, S., et al. (2018). Effect of biochar and nitrapyrin on nitrous oxide and nitric oxide emissions from a sandy loam soil cropped to maize. Biol. Fertil. Soils 54, 645–658. doi: 10.1007/s00374-018-1289-2
Pinheiro, P. L., Recous, S., Dietrich, G., Weiler, D. A., Giovelli, R. L., Mezzalira, A. P., et al. (2018). Straw removal reduces the mulch physical barrier and ammonia volatilization after urea application in sugarcane. Atmos. Environ. 194, 179–187. doi: 10.1016/j.atmosenv.2018.09.031
Quemada, M., Baranski, M., Nobel-De Lange, M. N. J., Vallejo, A., and Cooper, J. M. (2013). Meta-analysis of strategies to control nitrate leaching in irrigated agricultural systems and their effects on crop yield. Agric. Ecosyst. Environ. 174, 1–10. doi: 10.1016/j.agee.2013.04.018
Sha, J. C., Jia, Z. H., Xu, X. X., Hou, X., Li, B. Y., Ge, S. F., et al. (2019). Effects of nitrogen application levels on translocation and distribution of 13C-photosynthate and 15N to fruit from leaves of apple tree. J. Appl. Ecol. 30, 1373–1379. doi: 10.13287/j.1001-9332.201904.011
Sun, Q., Jiang, S., Zhang, T., Xu, H., Fang, H., Zhang, J., et al. (2019). Apple NAC transcription factor MdNAC52 regulates biosynthesis of anthocyanin and proanthocyanidin through MdMYB9 and MdMYB11. Plant Sci. 289, 110286. doi: 10.1016/j.plantsci.2019.110286
Vilarrasa-Nogue, M., Teira-Esmatges, M. R., Pascual, M., Villar, J. M., and Rufat, J. (2020). Effect of N dose, fertilisation duration and application of a nitrification inhibitor on GHG emissions from a peach orchard. Sci. Total Environ. 699:134042. doi: 10.1016/j.scitotenv.2019.134042
Vinzent, B., Fuß, R., Maidl, F. X., and Hülsbergen, K. J. (2018). N2O emissions and nitrogen dynamics of winter rapeseed fertilized with different N forms and a nitrification inhibitor. Agric. Ecosyst. Environ. 259, 86–97. doi: 10.1016/j.agee.2018.02.028
Wang, F., Sha, J. C., Chen, Q., Xu, X. X., Zhu, Z. L., Ge, S. F., et al. (2020a). Exogenous abscisic acid regulates distribution of 13C and 15N and anthocyanin synthesis in ‘Red Fuji’ apple fruit under high nitrogen supply. Front. Plant Sci. 10:1738. doi: 10.3389/fpls.2019.01738
Wang, F., Yue, Y., Li, M., Luo, J., Ge, S., and Jiang, Y. (2020b). Effects of Na2WO4 on 15N absorption and utilization, 13C accumulation of apple seedling and fruit quality at mature stage. Chin. J. Appl. Ecol. 31, 182–188. doi: 10.13287/j.1001-9332.202001.024
Wang, H., Köbke, S., and Dittert, K. (2020c). Use of urease and nitrification inhibitors to reduce gaseous nitrogen emissions from fertilizers containing ammonium nitrate and urea. Glob. Ecol. Conserv. 22:e00933. doi: 10.1016/j.gecco.2020.e00933
Wang, W., Hao, Q., Wang, W., Li, Q., Chen, F., Ni, F., et al. (2019). The involvement of cytokinin and nitrogen metabolism in delayed flag leaf senescence in a wheat stay-green mutant, tasg1. Plant Sci. 278, 70–79. doi: 10.1016/j.plantsci.2018.10.024
Wang, X., Fu, X., Chen, M., Huan, L., Liu, W., Qi, Y., et al. (2018). Ultraviolet B irradiation influences the fruit quality and sucrose metabolism of peach (Prunus persica L.). Environ. Exp. Bot. 153, 286–301. doi: 10.1016/j.envexpbot.2018.04.015
Wang, X. F., An, J. P., Liu, X., Su, L., You, C. X., and Hao, Y. J. (2018). The nitrate-responsive protein MdBT2 regulates anthocyanin biosynthesis by interacting with the MdMYB1 transcription factor. Plant Physiol. 178, 890–906. doi: 10.1104/pp.18.00244
Wang, Y., Wang, N., Xu, H., Jiang, S., Fang, H., Zhang, T., et al. (2017). Nitrogen affects anthocyanin biosynthesis by regulating MdLOB52 downstream of MdARF19 in callus cultures of red-fleshed apple (Malus sieversii f. niedzwetzkyana). J. Plant Growth Regul. 37, 719–729. doi: 10.1007/s00344-017-9766-7
Wen, B., Li, C., Fu, X., Li, D., Li, L., Chen, X., et al. (2019). Effects of nitrate deficiency on nitrate assimilation and chlorophyll synthesis of detached apple leaves. Plant Physiol. Biochem. 142, 363–371. doi: 10.1016/j.plaphy.2019.07.007
Xu, J., Zhu, T., Xue, W., Ni, D., Sun, Y., Yang, J., et al. (2019). Influences of nitrification inhibitor 3,4-dimethylpyrazole phosphate (DMPP) and application method on nitrogen dynamics at the centimeter-scale. Eur. J. Soil Biol. 90, 44–50. doi: 10.1016/j.ejsobi.2018.12.004
Yin, J., Yu, Q., Fu, J., Ma, J., Ye, J., and Tang, Q. (2012). Influence of different DMPP addition level on organic nitrogen transformation in the soil. J. Soil Water Conserv. 26, 111–115. doi: 10.13870/j.cnki.stbcxb.2012.06.011
Yu, Q., Ma, J., Sun, W., Zou, P., Lin, H., Fu, C., et al. (2018). Evaluations of the DMPP on organic and inorganic nitrogen mineralization and plant heavy metals absorption. Geoderma 312, 45–51. doi: 10.1016/j.geoderma.2017.10.007
Yu, Q., Ma, J., Zou, P., Lin, H., Sun, W., Yin, J., et al. (2015). Effects of combined application of organic and inorganic fertilizers plus nitrification inhibitor DMPP on nitrogen runoff loss in vegetable soils. Environ. Sci. Pollut. Res. Int. 22, 472–481. doi: 10.1007/s11356-014-3366-x
Yu, Q. G., Chen, Y. X., Ye, X. Z., Tian, G. M., and Zhang, Z. J. (2007). Influence of the DMPP (3,4-dimethyl pyrazole phosphate) on nitrogen transformation and leaching in multi-layer soil columns. Chemosphere 69, 825–831. doi: 10.1016/j.chemosphere.2007.05.047
Zerulla, W., Barth, T., Dressel, J., Erhardt, K., Horchler Von Locquenghien, K., Pasda, G., et al. (2001). 3,4-Dimethylpyrazole phosphate (DMPP)-a new nitrification inhibitor for agriculture and horticulture. Biol. Fertil. Soils 34, 79–84. doi: 10.1007/s003740100380
Zhang, Q., Li, X., Li, M., Zhou, B., Zhang, J., and Wei, Q. (2017). Correlation analysis between quality characteristics and fruit mineral element contents in ‘Fuji’ apples. Agric. Sci. Technol. 18, 212–218.
Zhang, Q. Y., Gu, K. D., Cheng, L., Wang, J. H., Yu, J. Q., Wang, X. F., et al. (2020). BTB-TAZ domain protein MdBT2 modulates malate accumulation and vacuolar acidification in response to nitrate. Plant Physiol. doi: 10.1104/pp.20.00208 [Epub ahead of print].
Zhang, Y., Liu, S., Cheng, Y., Cai, Z., Müller, C., and Zhang, J. (2019). Composition of soil recalcitrant C regulates nitrification rates in acidic soils. Geoderma 337, 965–972. doi: 10.1016/j.geoderma.2018.11.014
Zhu, G., Ju, X., Zhang, J., Müller, C., Rees, R. M., Thorman, R. E., et al. (2019). Effects of the nitrification inhibitor DMPP (3,4-dimethylpyrazole phosphate) on gross N transformation rates and N2O emissions. Biol. Fertil. Soils 55, 603–615. doi: 10.1007/s00374-019-01375-6
Zhu, T., Zhang, J., Huang, P., Suo, L., Wang, C., Ding, W., et al. (2015). N2O emissions from banana plantations in tropical China as affected by the application rates of urea and a urease/nitrification inhibitor. Biol. Fertil. Soils 51, 673–683. doi: 10.1007/s00374-015-1018-z
Keywords: apple, DMPP, mineral nitrogen, 15N, 13C, fruit quality
Citation: Wang F, Xu X, Jia Z, Hou X, Chen Q, Sha J, Liu Z, Zhu Z, Jiang Y and Ge S (2020) Nitrification Inhibitor 3,4-Dimethylpyrazole Phosphate Application During the Later Stage of Apple Fruit Expansion Regulates Soil Mineral Nitrogen and Tree Carbon–Nitrogen Nutrition, and Improves Fruit Quality. Front. Plant Sci. 11:764. doi: 10.3389/fpls.2020.00764
Received: 02 April 2020; Accepted: 14 May 2020;
Published: 03 June 2020.
Edited by:
Antonio Ferrante, University of Milan, ItalyReviewed by:
Mingjun Li, Northwest A&F University, ChinaDong Zhang, Northwest A&F University, China
Yuncong Yao, China Agricultural University, China
Copyright © 2020 Wang, Xu, Jia, Hou, Chen, Sha, Liu, Zhu, Jiang and Ge. This is an open-access article distributed under the terms of the Creative Commons Attribution License (CC BY). The use, distribution or reproduction in other forums is permitted, provided the original author(s) and the copyright owner(s) are credited and that the original publication in this journal is cited, in accordance with accepted academic practice. No use, distribution or reproduction is permitted which does not comply with these terms.
*Correspondence: Zhanling Zhu, zhlzh@sdau.edu.cn; Yuanmao Jiang, ymjiang@sdau.edu.cn; Shunfeng Ge, geshunfeng210@126.com