The Diel and Seasonal Heterogeneity of Carbonate Chemistry and Dissolved Oxygen in Three Types of Macroalgal Habitats
- 1Department of Aquaculture and Aquatic Science, Kunsan National University, Gunsan, South Korea
- 2East Sea Research Institute, Korea Institute of Ocean Science & Technology, Uljin, South Korea
- 3Division of Environmental Science and Engineering, Pohang University of Science & Technology, Pohang, South Korea
- 4Korea Marine Environment & Ecology Research Institute, Bucheon, South Korea
- 5Department of Marine Science, Incheon National University, Incheon, South Korea
- 6Ecological Restoration Division, Korea Fisheries Resources Agency (East Sea Branch), Pohang, South Korea
- 7Department of Biology, San Diego State University, San Diego, CA, United States
As concerns about ocean acidification continue to grow, the importance of macroalgal communities in buffering coastal seawater biogeochemistry through their metabolisms is gaining more attention. However, studies on diel and seasonal fluctuations in seawater chemistry within these communities are still rare. Here, we characterized the spatial and temporal heterogeneity in diel and seasonal dynamics of seawater carbonate chemistry and dissolved oxygen (DO) in three types of macroalgal habitats (UAM: ulvoid algal mat dominated, TAM: turf algal mat dominated, and SC: Sargassum horneri and coralline algae dominated). Our results show that diel fluctuations in carbonate parameters and DO varied significantly among habitat types and seasons due to differences in their biological metabolisms (photosynthesis and calcification) and each site’s hydrological characteristics. Specifically, carbonate parameters were most affected by biological metabolisms at the SC site, and by environmental variables at the UAM site. Also, we demonstrate that macroalgal communities reduced ocean acidification conditions when ocean temperatures supported photosynthesis and thereby the absorption of dissolved inorganic carbon. However, once temperatures exceeded the optimum ranges for macroalgae, respiration within these communities exceeded photosynthesis and increased CO2 concentrations, thereby exacerbating ocean acidification conditions. We conclude that the seawater carbonate chemistry is strongly influenced by the metabolisms of the dominant macroalgae within these different habitat types, which may, in turn, alter their buffering capacity against ocean acidification.
Introduction
Macroalgae provide numerous ecological functions such as enhancing the primary production and providing food and shelter for other organisms (Kim et al., 2015; Mineur et al., 2015; Metzger et al., 2019; Edwards et al., 2020; Sullaway and Edwards, 2020). With increasing impacts of ocean acidification (OA) on marine ecosystems (Doney et al., 2009; IPCC, 2019), the role of macroalgae, and more specifically their metabolisms, in buffering coastal ecosystems against OA has received considerable attention because they can alter seawater carbonate parameters through photosynthesis and respiration, and to a lesser extent calcification and dissolution (Comeau and Cornwall, 2016). Indeed, macroalgae can reduce seawater pCO2 within some coastal waters, and thereby temporarily mitigate the effects of OA (Murie and Bourdeau, 2020; Xiao et al., 2021). They can also influence diel and seasonal cycles in seawater chemistry because their metabolisms are strongly influenced by patterns of solar irradiance. These cycles, however, likely also vary among habitats that are characterized by different macroalgae with different metabolisms. As a result, examining diel and seasonal variations in seawater chemistry within and around different types of macroalgal communities can help discern the importance of biological and chemical interactions between macroalgae and seawater chemistry parameters (Gonzales et al., 2017; Carrano et al., 2020; Carrano et al., 2021).
The coastal ocean experiences large fluctuations in its carbonate chemistry due to both hydrodynamic and biological activities (Lee et al., 2011; Cai et al., 2020; Kim et al., 2020b). In fact, variability in seawater pH within some coastal oceans can be greater than that in the open ocean (Hofmann et al., 2011). Several studies have shown that substantial diel fluctuations in pH and pCO2 occur in the waters around macroalgal communities (e.g., Delille et al., 2000; Middelboe and Hansen, 2007; Delille et al., 2009; Krause-Jensen et al., 2015). In general, photosynthesis and respiration strongly contribute to diel variations in inorganic carbon by absorbing CO2 during the day and releasing CO2 at night (Chou et al., 2018), with the net effects of these being manifested in net community production (NCP) (Cyronak et al., 2018; Edwards et al., 2020). To a lesser extent, the production and dissolution of CaCO3 by calcifying organisms (e.g., coralline algae, epiphytes and benthic invertebrates) also alter seawater carbonate chemistry, with the net effects of these being quantified as net community calcification (NCC) (Turk et al., 2015; Ragazzola et al., 2021). Both NCP and NCC can regulate seawater total alkalinity (AT) and total dissolved inorganic carbon (CT) following a well-established stoichiometry, where the ratio of AT-CT for NCP is –0.2 and the ratio for NCC is 2.0 (Krumins et al., 2013; Sippo et al., 2016). Hence, measuring the relationship between AT and CT could be an effective way to identify the dominant metabolism within these communities. For example, while NCP is the dominant metabolism within some shallow coastal regions of the Mediterranean Sea throughout the year, NCC can lead to decreases in AT during the summer within areas of the coast that are dominated by coralline algae whose metabolisms alter ΔAT/ΔCT ratios (Ragazzola et al., 2021). Another potential metric for evaluating the biogeochemical effects of macroalgal communities on seawater chemistry is the relationship between CO2 partial pressure (pCO2) and dissolved oxygen (DO), as photosynthesis lowers pCO2 but increases DO, while respiration releases CO2 but consumes DO (Zhai et al., 2009; Vachon et al., 2020). Hence, the coupling pCO2 and DO can help discern the status of ocean metabolism, explicitly the ratio of primary production and respiration (Kuss et al., 2006; Zhai and Dai, 2009; Vachon et al., 2020).
At present, the ability of macroalgae to mitigate OA by altering seawater chemistry has been studied in kelp beds (Frieder et al., 2012; Britton et al., 2016; Pfister et al., 2019; Edwards et al., 2020) and seaweed farms (Li et al., 2021; Xiao et al., 2021). In contrast, other macroalgal communities in coastal oceans have seldom been studied (Middelboe and Hansen, 2007; Wahl et al., 2018). The types of macroalgal communities are diverse, and their influence on the biogeochemical interactions within the surrounding environment can vary depending on their community structures (Kim et al., 2015; Spector and Edwards, 2020). For example, in habitats dominated by opportunistic macroalgae, fluctuations in biogeochemical parameters may be large because of high rates of photosynthesis and respiration, whereas at the same time the absence of calcifying organisms may result in only small changes in AT (e.g., Deng et al., 2018). On the other hand, habitats dominated by canopy-forming macroalgae such as kelp beds exhibit high rates of respiration due to their strong ability to capture organic matter (Edwards et al., 2020; Spector and Edwards, 2020). However, the coralline algae that inhabit the rocky bottom within these kelp beds can create unexpected biogeochemical responses because of their integrated metabolisms (Edwards et al., 2020). In the turf algal mats, lower metabolism appears than that in the blooming area of the canopies, but more complex variations in AT and CT occur in the turf algae because of the higher species diversity. However, studies on the ability of different macroalgal communities to alter seawater carbonate parameters have not been conducted. In particular, it is necessary to better understand diel fluctuations in carbonate parameters within these communities because changes in these parameters can reveal the influence of their biological metabolisms, which are regulated by changes in irradiance between the daytime and nighttime.
Anthropogenic activities are leading to widespread changes in macroalgal communities along the temperate coast of Korea, and acclimation patterns of the dominant macrophytes (i.e., their phenology) are clearly observed according to the seasons. For example, in Pohang new port, Korea, coralline algae are dominant in all seasons, but the canopy-forming alga, Sargassum horneri is abundant only in winter (Yoo et al., 2006). Indeed, total macroalgal biomass is high in winter and spring, and low in summer and autumn. In addition, opportunistic macroalgae have become abundant in coastal areas with intensive nutrient inputs (Lee and Kang, 2020; Kim et al., 2020b; Kang et al., 2021), while coralline algae have become dominant in other areas because of climate change and increased herbivore pressure on fleshy macroalgae (Kim et al., 2020a). Over time, these macroalgal communities are turning into tiny mosaics with strong spatial heterogeneity. Hence, it is important to investigate fluctuations in seawater chemistry in and around these different communities throughout the year if we are to evaluate seasonal variation in their abilities to buffer against changes in pH (Delille et al., 2009; Koweek et al., 2017). In this study, we investigate diel and seasonal heterogeneity in carbonate chemistry and dissolved oxygen within different macroalgal habitats, and attempt to understand how diel metabolic activities within various macroalgal habitats are affecting seawater chemistry in different seasons, and their implications for OA mitigation.
Materials and Methods
Study Area
Seawater carbonate parameters and DO were measured in three macroalgal habitats along the east coast of the Korean peninsula near the Odo, Heunghae-eup, Pohang-si (36°9’15’’N, 129°24’3’’E) (Figure 1). This area is located in the East Sea (a marginal sea in the northwestern Pacific), which takes up substantial amounts of anthropogenic CO2 that subsequently changes the carbonate chemistry of the East Sea (Park et al., 2006). Otherwise, the study area has no large-scale sources of pollutants, with the only sewage flowing from nearby villages (Heunghae-eup: with a population of less than 40,000) and harbors. The water mass at the sampling area is physically quite stable. Specifically, the tidal range is less than 30 cm, and there are nearly no tidal currents. In addition, depending on the weather conditions, the mixing of seawater occurs actively by waves from the open ocean. Our previous study showed that the concentrations of nitrate and phosphate were maintained at relatively low and stable levels, up to 12 µmol kg-1 and 1 µmol kg-1 respectively (Unpublished data). Three macroalgal habitats were chosen within the study area as sampling sites, namely ulvoid algal mats (UAM), turf algal mats (TAM), and a coexisting habitat of the canopy-forming macroalga, Sargassum horneri, and crustose coralline algae (SC). Specifically, the UAM site, which covers approximately 2,500 m2, is located inside of the harbor where the sewage from villages is input directly and ulvoid green algae (e.g., Ulva australis) are predominant, showing close to 100% coverage. Also, small individuals of Undaria pinnatifida are attached to the harbor’s concrete wall. Metabolism at the UAM site is expected to be dominated by photosynthesis/respiration of ulvoid seaweed and microalgae, and by microbial respiration. The TAM site, which covers approximately 3,550 m2, is located near the outer harbor and has more than 30 species of understory and canopy-forming macrophytes that are distributed haphazardly (e.g., Chlorophyta: Ulva spp., Phaeophyta: S. horneri, Saccharina japonica, U. pinnatifida; Rhodophyta: Portieria japonica, Callophyllis japonica, Plocamium telfairiae; Vascular plants: Zostera marina, Phyllospadix iwatensis, etc.). Since the exchange of seawater at the TAM site is relatively active compared to the UAM site, photosynthesis and respiration by the benthic community are balanced, and microbial respiration is expected to be relatively low. The SC site, which is connected to an open ocean and not semi-enclosed, is located at the outside of the harbor and is a transitional habitat to an urchin barren ground where most of the foliose macroalgae have disappeared, except for S. horneri, and where the crustose coralline algae (CCA) species have become dominant due to the intense herbivore pressure. At the SC site, we can expect intense photosynthesis/respiration by S. horneri and CCA, and calcification/dissolution by CCA. Also, the biomass of benthic macrofauna was less than 16% compared to that of macroalgae near the TAM and SC sites (see Supplementary Tables S1, S2). The distance between three sampling sites is very close (UAM to SC: 310 m; UAM to TAM: 120 m; TAM to SC: 270 m). Salinity (during the carbonate chemistry analysis) and temperature data (during the in-situ monitoring) are presented in Supplementary Table S3.
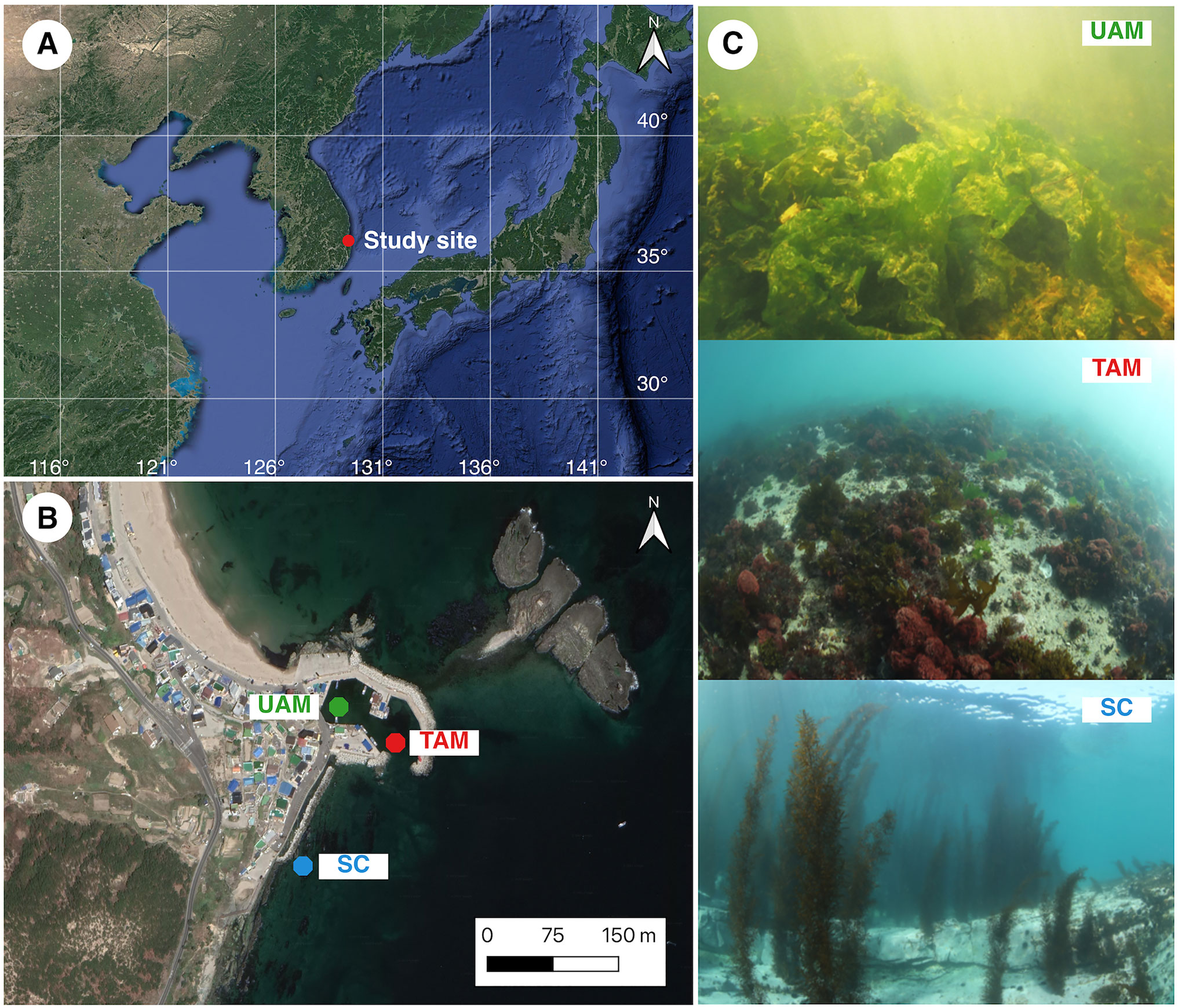
Figure 1 (A) The location of study area where the three sites with different macroalgal habitats were located in Odo beach of Pohang on the east coast of the Korea peninsula, (B) locations of the three study sites with different macroalgal habitats (UAM, ulvoid algal mat; TAM, turf algal mat; SC, coexisting Sargassum horneri and crustose coralline algae) and (C) photographs of three macroalgal habitats (UAM: top, TAM: mid, and SC: bottom). Maps were generated using a Google satellite map and QGIS 3.20 Odense software (QGIS Development Team, 2021).
Seawater Sampling and Determination of Carbonate Parameters (nAT, nCT, and npHT)
To characterize diel fluctuations in seawater carbonate parameters, seawater was collected from each of the three macroalgal habitats (UAM, TAM, and SC) during four seasonal events in 2019: winter (from Feb. 12 at 9:00 AM to Feb. 13 at 7:00 AM), spring (from May 30 at 9:00 AM to May 31 at 6:00 AM), summer (from Aug. 8 at 9:00 AM to Aug. 9 at 6:00 AM), and autumn (from Oct. 10 at 9:00 AM to Oct. 11 at 6:00 AM). At each site, seawater was collected every 2 to 3 hours over a 24-hour period to determine total alkalinity (AT), total inorganic carbon (CT), and pH on the total hydrogen ion concentration scale (total scale, pHT). As three sites were close to each other, we collected the samples for each site at similar times on the same day. All seawater sampling procedures and carbonate chemistry analyses followed the standard operation procedures for ocean CO2 measurement as described by Dickson et al. (2007). The seawater samples were collected from about 0.5 m above the bottom of macroalgal habitats using a custom-made sampler (2 L capacity) made of transparent PC material, and quickly transferred to two 500 ml borosilicate airtight glass bottles respectively (1,500-500 Pyrex; Corning, NY, USA) without introducing air bubbles. The bottles were immediately closed with vacuum grease (Apiezon M grease; M&I Materials Ltd., Manchester, UK) after adding 100 µL saturated mercuric chloride (HgCl2) to poison all organisms and stop the metabolism. These bottoles were then stored cold in the dark until measurement.
Seawater AT, CT, and pHT were measured within two weeks after sampling. Concentrations of AT and CT were measured using potentiometric and coulometric titration methods, respectively, within the VINDTA system (Versatile Instrument for the Determination of Titration Alkalinity, Marianda, Kiel, Germany). For AT measurements, the samples were titrated with weak HCl (0.2 M). The volume of added acid was recorded at least 25 points until the titration to the endpoint [Electromotive force (EMF) ≈ 400 mV]. The values of AT were determined from the resulting titration data using a non-linear curve fitting within the VINDTA LabView-software (Millero et al., 1993; Dickson et al., 2007). Note that AT errors associated with organic acids and particulate organic matter were found to be negligible in this environment (Ko et al., 2016; Lee et al., 2021). To analyze CT, the coulometer cell was filled with ethanol amine and a colorimetric indicator. When the gas stream passes through the solution, CO2 is absorbed quantitatively. Seawater pHT was determined spectrophotometrically (Clayton and Byrne, 1993) by adding the sulfonephthalein indicator m-Cresol Purple (mCP, Sigma-Aldrich Chemical Co., St. Louis, MO, USA) at the room temperature of 25°C. The absorbances of samples with and without the mCP indicator were measured using a 10 cm path length cuvette and a spectrophotometer (Agilent 8453 UV-Visible Spectrophotometer; Agilent Technologies, Pal Alto, CA, USA) at wavelengths of 730, 578, and 434 nm (Clayton and Byrne, 1993; Dickson, 1993). Specifically, the tube and cuvette were rinsed with sample before each measurement, and then the cuvette full of sample was placed in the sample compartment of the spectrophotometer. After the absorbance measurement, 80 µl mCP indicator was injected into the cuvette and mixed with sample by gently inverting. Then the cuvette was returned to the spectrophotometer to measure again the absorbances of the sample with indicator. Prior to measurements, the precision and performance of the instruments were verified using certified reference materials (CRMs, supplied by A. G. Dickson, Scripps Institute of Oceanography, U.S.A., batch number 180, 183 and 184). The accuracy of these measurements was ± 1.5 µmol kg-1 for AT, ± 2 µmol kg-1 for CT and ± 0.004 unit for pHT. The salinity of samples was measured using a salinometer (Portasal™ 8410A; Guideline Instruments Ltd., USA). To consider only the effects of biological metabolism on the carbonate chemistry, the values of AT and CT were normalized to salinity (by multiplying by a factor of the averaged salinity value/S, where S is the measured salinity value of each sample) to remove the effect of salinity variations on AT and CT. Also, values of pHT were normalized to temperature of 25°C to remove the effect of temperature on pHT. The normalized values are expressed as nAT, nCT, npHT hereafter.
In-Situ Monitoring Seawater Biogeochemical Parameters (pCO2, DO, and pHNIST)
Various data logger sensors were deployed at each of the three macroalgal habitats to monitor in-situ seawater biogeochemical parameters, including partial pressure of carbon dioxide (pCO2), dissolved oxygen (DO) and pH on the scale of National Institute of Standards and Technology (NIST scale, pHNIST) for two to six days each in winter (from Feb. 18 to Apr. 2), spring (from May 24 to Jun. 3), summer (from Aug. 9 to 19), and autumn (from Oct. 15 to 23) in 2019. The in-situ measurements at three sites were conducted on different dates during the monitoring period since we needed to put the sensors at one site after another. The number of monitoring days at each site was different, from at least two to six days, because of the weather and other practical considerations. In particular, the UAM site was monitored for only 34 hours in summer due to the interruption by a typhoon. Although the winter monitoring continued until Apr. 2 (spring), these data were included in winter data because there was no significant change in water temperature during monitoring (approximately 11°C). A CONTROS HydroC CO2 sensor (Kongsberg Maritime Contros GmbH, Kiel, Germany) equipped with a non-dispersive infrared (NDIR) analyzer was used to measure pCO2, and data were stored every 1 hour. This instrument and 16.8V lithium battery were fixed together on a stainless-steel frame and placed at 0.2 m above the bottom of each macroalgal habitat. A HOBO datalogger U26-001 and a HOBO datalogger MX2501 (onset computer corporation, Bourne, MA, USA) were used to monitor DO and pHNIST with temperature respectively, which were fastened on a stainless-steel frame and deposited at 0.2 m above the bottom of each macroalgal habitat. The caps of DO dataloggers were replaced every six months to obtain reliable results. Before the in-situ pH monitoring, dataloggers were calibrated using the NIST standard reference buffer (pH 4.0, 7.0, and 10.0; YSI 3821~3823, YSI Inc./Xylem Inc., USA). After in-situ monitoring, pHNIST values showed stable signal when re-calibration with the reference buffer. Data of DO and pHNIST were stored every 10 minutes.
Data Analysis
Statistical analyses were performed using SPSS software version 25, and PRIMER/PERMANOVA version 6.1.13. All data were checked for normality and equality of variances prior to testing. Data for normalized carbonate chemistry (nAT, nCT, and npHT), and in-situ monitoring data (pCO2, DO, and pHNIST) were all non-normal and heteroscedastic, and could not be fixed by transformation. Therefore, differences in each parameter among seasons and macroalgal sites were analyzed with separate two-way Model I PERMANOVAs on Euclidean distance-based resemblance matrices, as described by Anderson and Walsh (2013). Data were square root transformed prior to analyses. Due to very large sample sizes (100’s to 1,000’s depending on variable), our analyses had an abundance of statistical power to resolve even very small differences in our response variables. Consequently, we acknowledge p-values alone may not be entirely informative when evaluating the effects of each explanatory factor, as described for ANOVA by Graham and Edwards (2001). We therefore include the amount of variation in our response variables (% of total) that was explained by each explanatory factor by calculating their magnitudes of effect (ω2) (Graham and Edwards, 2001). Following this, a priori hypotheses regarding general differences among the four seasons and/or three habitats were examined using permutation pairwise comparisons as post hoc tests on the season and site main effects. All data are presented as mean ± standard deviation unless otherwise stated. All data figures and fitting curves were generated using DataGraph 4.7.1 software (Visual Data Tools, Inc., Chapel Hill, North Carolina, USA). The extreme outliers were excluded from the statistical analysis and subsequent figures. To further study the ratio of net organic metabolism (net community production; NCP) and net inorganic metabolism [net community calcification; NCC; (Cyronak et al., 2018)], nAT values and nCT values at three sites in four seasons were plotted separately to create a least squares fitting curve using a linear regression model. The obtained slope was grouped with corresponding temperature and generated a least squares fitting curve using the linear regression model to study the relationship of the nAT - nCT slope and temperature. As the relative variation of nAT and nCT follows a well-established stoichiometry that is specific to the respective biogeochemical process, where the ratio for photosynthesis/respiration is –0.2 and the ratio for carbonate dissolution/calcification is 2.0 (Krumins et al., 2013; Sippo et al., 2016), the relative percent influence of NCP on changes in nCT was calculated according to the following formula (Cyronak et al., 2018):
where the slope is the slope of nAT - nCT vector.
Since the correlation of pCO2 and DO has been shown to have implications for upper ocean metabolic status, i.e., primary production/respiration and their history (Zhai et al., 2009), the pCO2 and DO values were grouped together, creating a least squares fitting curve using the non-linear exponential regression model.
Results
Seawater Carbonate Chemistry (nAT, nCT, and npHT)
The means with standard deviations and the variation ranges of seawater nAT, nCT, and npHT at three macroalgal sits in four seasons are presented in Table 1, and the diel patterns of these three parameters are shown in Figure 2. In general, nAT ranged from 2203.4 to 2318.9 µmol kg-1 at the UAM site, and from 2202.0 to 2329.4 µmol kg-1 at the TAM site, besides from 2189.0 to 2304.0 µmol kg-1 at the SC site within four seasons. nCT values had a range from 1793.7 to 2161.3 µmol kg-1 among all collected data. The highest value of npHT (8.23) was at the SC site in winter, and the lowest value (7.63) was at the SC site in spring. Salinity values were relatively stable in winter and spring (around 34 ‰), then decreased to approximately 32 ‰ in summer and autumn (Supplementary Table S3). Overall, nAT values differed among seasons (PERMANOVA: Pseudo-F3,199 = 566.280, P(perm) < 0.001) and the macroalgal habitat types (Pseudo-F2,199 = 13.429, P(perm) < 0.001), and these two factors interacted with each other (Season × Site interaction: Pseudo-F6,199 = 11.467, P(perm) < 0.001) (Table 2). However, this interaction explained very little (5%) of the total variation in nAT values. In contrast, differences among seasons explained most of the total variation in nAT (86%), with the mean values in winter (2212.1 ± 11.8 µmol kg-1), spring (2225.9 ± 13.8 µmol kg-1), summer (2219.0 ± 18.7 µmol kg-1), and autumn (2299.2 ± 10.8 µmol kg-1) all being significantly different from one another (permutation post hoc tests: P ≤ 0.015 for each comparison). In contrast, differences among sites explained only 1% of the variation in nAT, with the mean values at the UAM site (2241.2 ± 34.4 µmol kg-1) being significantly higher than values at the TAM (2233.4 ± 38.5 µmol kg-1) and the SC (2230.0 ± 35.0 µmol kg-1) sites (permutation post hoc tests: P < 0.001 for each comparison). nAT values did not differ between at the TAM and SC sites (P = 0.193). nCT values also varied among seasons (PERMANOVA: Pseudo-F3,203 = 33.837, P(perm) < 0.001) and sites with different macroalgal habitat types (Pseudo-F2,203 = 4.276, P(perm) = 0.015), and these factors interacted with each other (Season × Site interaction: Pseudo-F6,203 = 3.263, P(perm) = 0.007) (Tables 1, 2 and Figure 2). However, this interaction again explained very little (7%) of the total variation in nCT. In contrast, differences among seasons explained far more of the variation in nCT (34%), with mean values in winter (1968.5 ± 50.8 µmol kg-1 SW), spring (2019.4 ± 77.4 µmol kg-1), summer (1982.1 ± 47.0 µmol kg-1), and autumn (2072.3 ± 66.1 µmol kg-1) all being significantly different from one another (permutation post hoc tests: P < 0.01 for each comparison), with an exception of the difference between winter and summer, which was not statistically different from one another (P = 0.104). Differences among sites again explained very little (3%) of the variation in nCT, with the values at the UAM site (2015.4 ± 64.7 µmol kg-1) being significantly higher than those at the SC site (1990.8 ± 93.3 µmol kg-1) (permutation post hoc test: P = 0.013). Otherwise, nCT values did not differ between the TAM site (2011.9 ± 51.2 µmol kg-1) and either the SC site (P = 0.065) or the UAM site (P = 0.232). Lastly, npHT values varied among seasons (PERMANOVA: Pseudo-F3, 201 = 6.589, P(perm) = 0.002) but they did not vary among sites with different macroalgal habitat types (Pseudo-F2,201 = 1.336, P(perm) = 0.265) (Tables 1, 2 and Figure 2). As with nAT and nCT, these factors interacted with each other (Season × Site interaction: Pseudo-F6,201 = 2.301, P(perm) = 0.033), but this interaction again explained very little (7%) of the variation in npHT. Unlike with nAT and nCT, however, differences among seasons only explained 9% of the total variation in npHT, but values in spring (7.89 ± 0.14) were significantly lower than values in winter (7.97 ± 0.10), summer (7.96 ± 0.09), and autumn (7.94 ± 0.07) (permutation post hoc tests: P = 0.001, 0.028, 0.003, respectively). Otherwise, npHT values did not vary among the other seasons (P > 0.08 for each comparison).
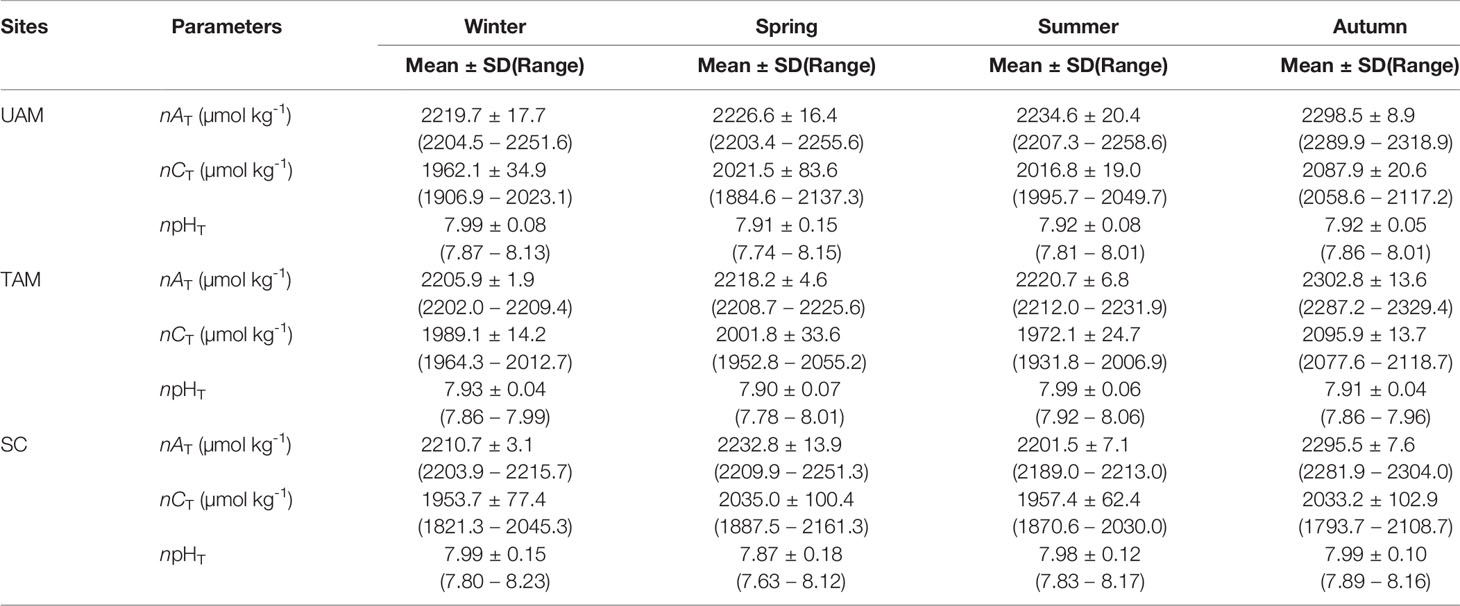
Table 1 The mean ± standard deviation (SD) and variation range of seawater carbonate parameters (salinity normalized total alkalinity: nAT, salinity normalized total dissolved inorganic carbon: nCT, and temperature normalized pH total scale: npHT,) of three macroalgal habitats (UAM: ulvoid algal mat; TAM: turf algal mat; SC: coexisting Sargassum horneri and crustose coralline algae) in four seasons.
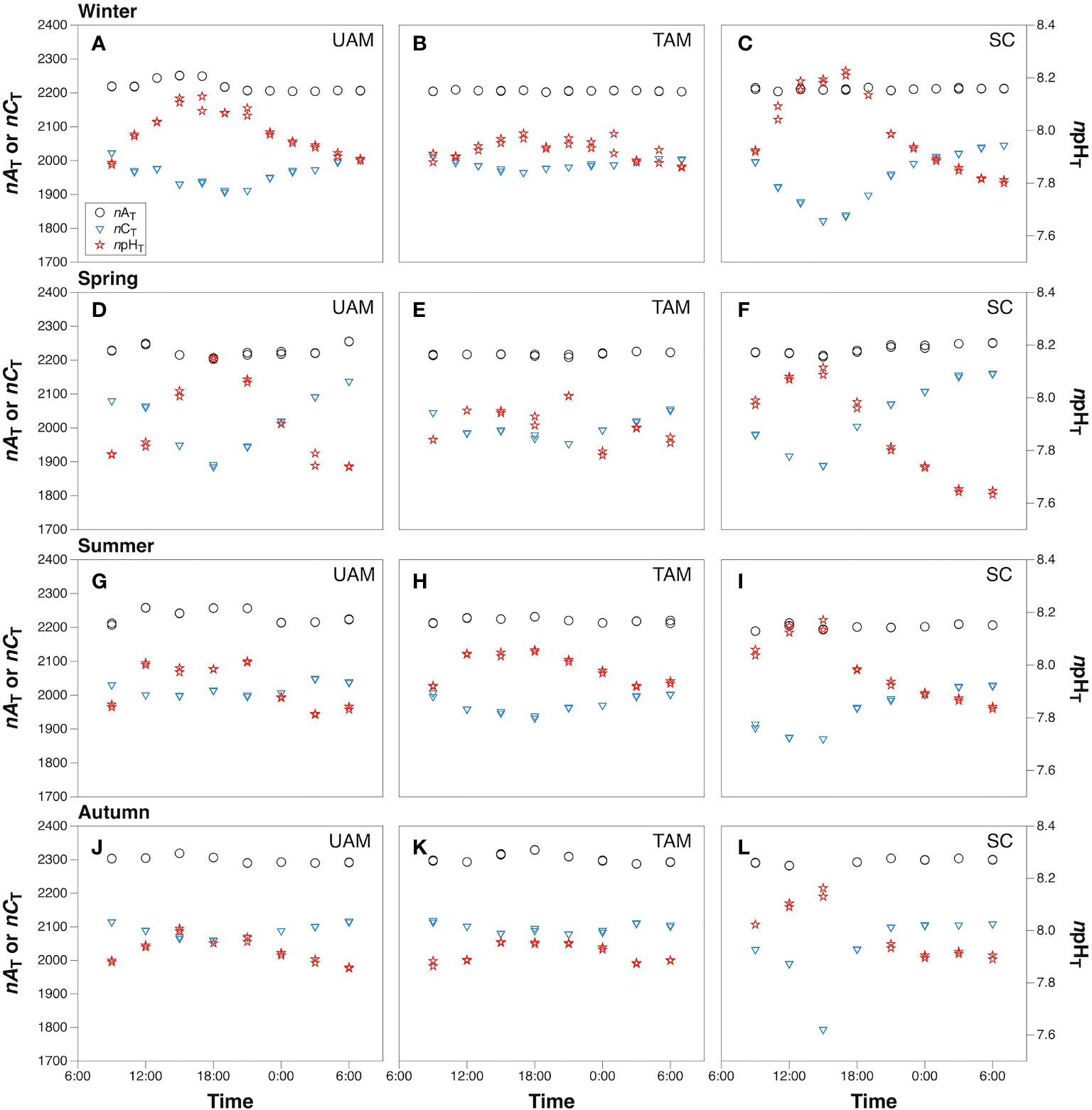
Figure 2 The diel variation of salinity normalized total alkalinity (nAT, µmol kg-1), salinity normalized total inorganic carbon (nCT, µmol kg-1) and temperature normalized pH total scale (npHT) at three sites with different macroalgal habitats (UAM, ulvoid algal mat; TAM, turf algal mat; SC, coexisting Sargassum horneri and crustose coralline algae) in winter (A–C), spring (D–F), summer (G–I) and autumn (J–L). Black circles represent values of nAT, black triangles represent values of nCT, and red stars represent values of npHT.
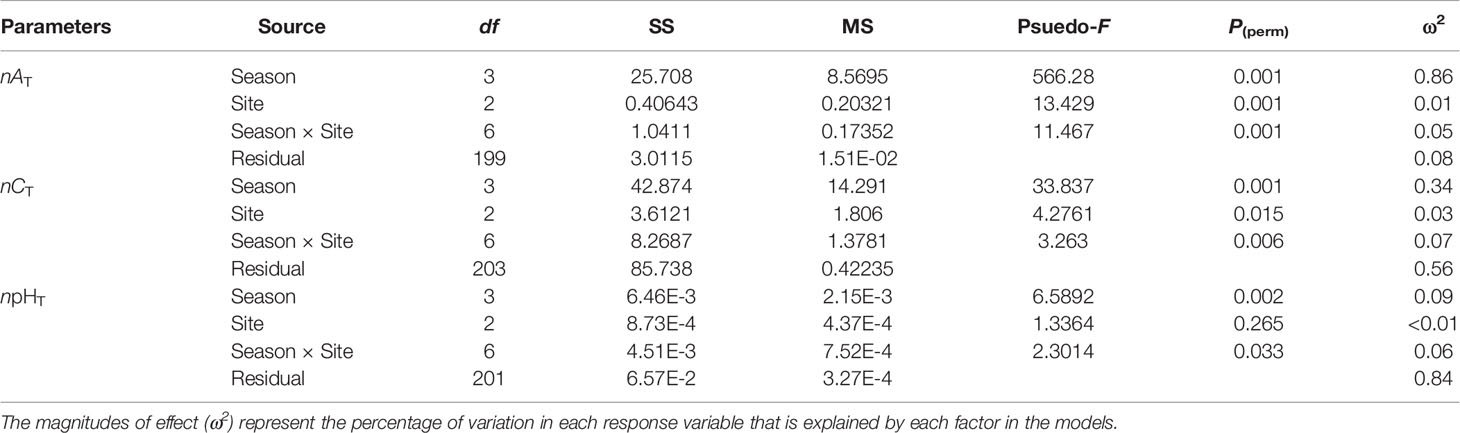
Table 2 Results of two factor Model I PERMANOVAs testing the effects of season and site on seawater carbonate parameters (salinity normalized total alkalinity: nAT, salinity normalized total dissolved inorganic carbon: nCT, and temperature normalized pH total scale: npHT).
The values of nCT and npHT covaried but in opposite directions in terms of diel variations, with nCT decreasing during the day and increasing at night, while npHT increased during the day and decreased at night (Figure 2). The largest nCT fluctuation occurred at the SC site in autumn (1793.7 to 2108.7 µmol kg-1), and the largest npHT fluctuations occurred at the SC site in spring (7.63 to 8.12, Table 1). The smallest nCT and npHT fluctuation occurred at the TAM site in autumn (2077.6 to 2118.7 µmol kg-1, 7.86 to 7.96 respectively). nAT showed similar patterns with nCT at the SC site, though the range of nAT variations was smaller than that of nCT. In contrast, nAT had opposite patterns to nCT at the UAM and TAM sites except in spring.
The relationships of nAT and nCT at three sites in four seasons are shown in Figure 3. The winter nAT/nCTslopes for all three habitats were generally low (UAM: –0.124, TAM: –0.021, and SC: 0.017), leading to the high relative percent influence of NCP on changes in nCT from 90.14% to 96.56% (Table 3). The spring nAT/nCT slopes increased slightly compared to winter slopes (UAM: 0.156, TAM: 0.077, and SC: 0.134), with an averaged % NCP value of 85.33 ± 1.85%. The nAT/nCT slopes in summer and autumn showed values lower than –0.2% (i.e., summer: –0.724 at UAM site and –0.219 at TAM site; autumn: –0.593 at TAM site), which led to the % NCP values over than 100%. The linear regression models (Figure 4) showed that the relationships of nAT/nCT slopes with temperature were negative at the UAM and TAM sites (slopes: –0.051 at the UAM site and –0.035 at the TAM site), whereas slightly positive at the SC site (slope: 0.002). However, none of these three regressions were statistically significant.
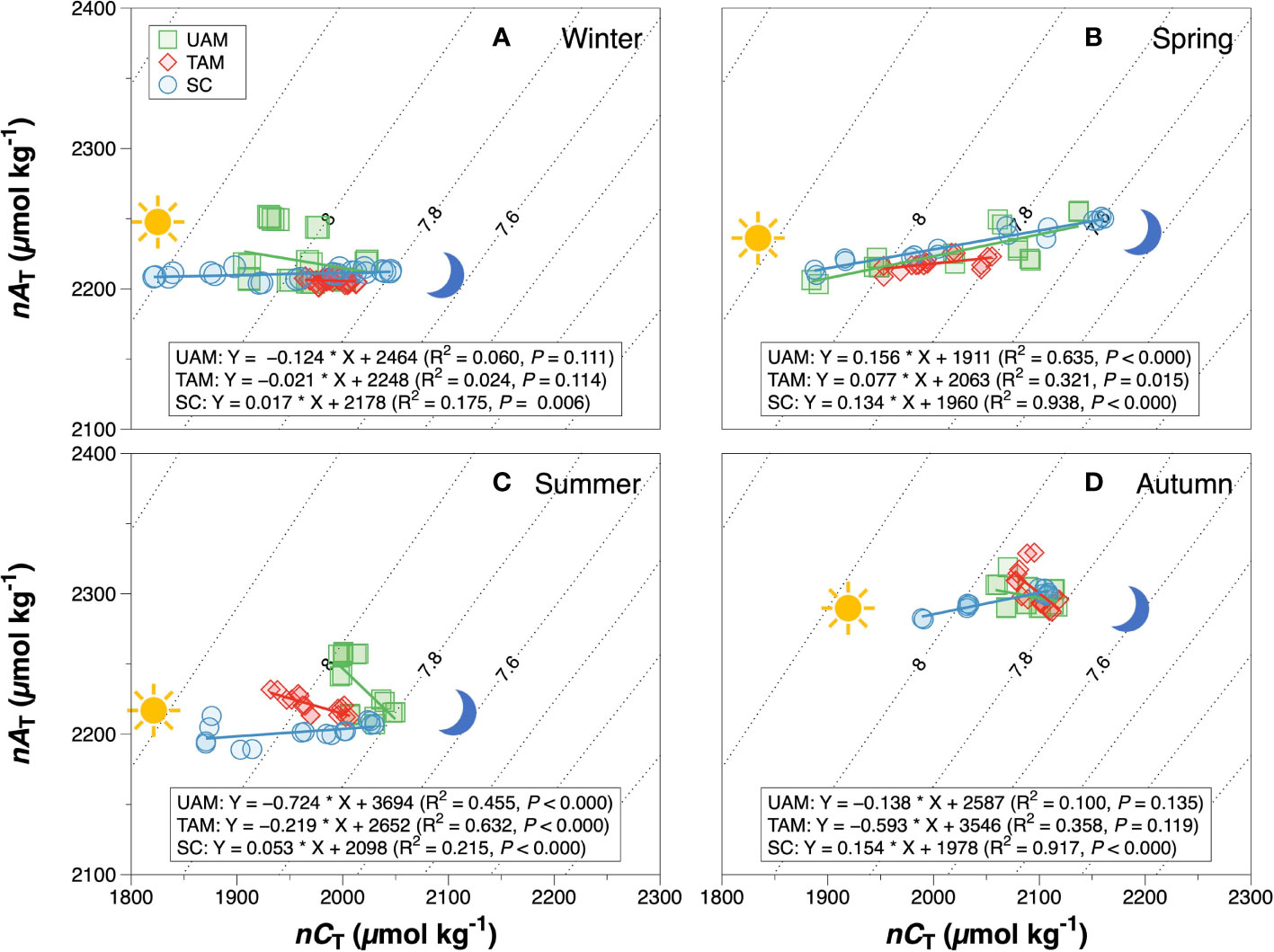
Figure 3 The relationship between salinity normalized total alkalinity (nAT) and salinity normalized total inorganic carbon (nCT) at three macroalgal habitats with different macroalgal habitats in winter (A), spring (B), summer (C) and autumn (D). The solid lines are the least square fitting curve of data using the linear regression model. The dashed lines are pH isolines at 0.2 units increments with the 7.6 – 8.0 pH isoline indicated. pH contours were calculated using the averaged seawater temperature and salinity in each season. Values for different macroalgal habitats: ulvoid algal mat (UAM, green square); turf algal mat (TAM, red diamond); coexisting Sargassum horneri and crustose coralline algae (SC, blue circle).

Table 3 The slope of the salinity normalized total alkalinity (nAT) – salinity normalized total inorganic carbon (nCT) vector and the relative percent influence of net community production (%NCP) on changes in nCT at three habitat sites (UAM, ulvoid algal mat; TAM, turf algal mat; SC, coexisting Sargassum horneri and crustose coralline algae) in four seasons.
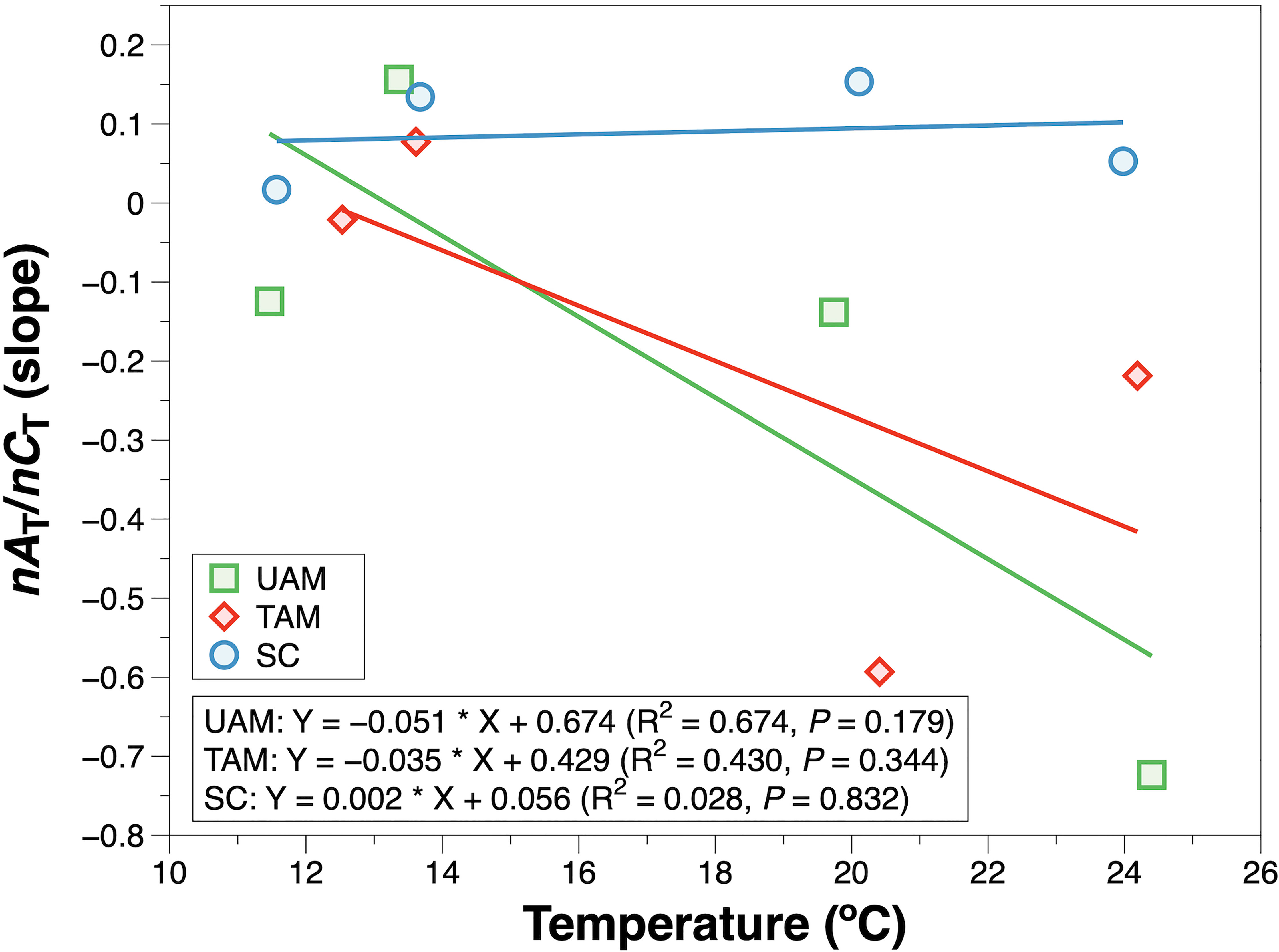
Figure 4 The slope of salinity normalized total alkalinity (nAT) and salinity normalized total inorganic carbon (nCT) in response to temperature. The solid line is the least square fitting curve of data using the linear regression model. Values for different macroalgal habitats: ulvoid algal mat (UAM, green square); turf algal mat (TAM, red diamond); coexisting Sargassum horneri and crustose coralline algae (SC, blue circle).
In-Situ Monitoring Seawater Biogeochemical Parameters (pCO2, DO, and pHNIST)
In-situ monitoring of biogeochemical parameters (pCO2, DO, and pHNIST) at the three sites characterized by different macroalgal types and four seasons are presented in Table 4, and their diel patterns are shown in Figure 5. Generally, pCO2 values, paralleled temperature values (Supplementary Table S3), being lowest in winter, increasing in spring, and then remaining high in summer and autumn at all three macroalgal sites. In contrast, DO and pHNIST values had relatively high values in winter and spring, then decreased in summer and autumn. Further statistical analysis showed that pCO2 values varied among seasons (PERMANOVA: Pseudo-F3,978 = 586.43, P(perm) < 0.001) and sites with different macroalgal habitat types (Pseudo-F2,978 = 59.93, P(perm) < 0.001), and these two factors interacted with each other (Season × Site interaction: Pseudo-F6,978 = 77.40, P(perm) < 0.001) (Table 5). However, differences among seasons explained the largest amount of variation in pCO2 values (54%), while differences among sites explained only 4% of this variation and the interaction between season and site explained 21% of this variation. Overall, pCO2 values were highest in autumn (522.33 ± 91.79 µatm), followed by summer (465.13 ± 95.01 µatm), then by spring (398.78 ± 152.47 µatm), and then by winter (256.64 ± 71.36 µatm), all of which were significantly different from each other (permutation post hoc tests: P < 0.01 for each comparison). The mean pCO2 value at the TAM site (394.82 ± 110.50 µatm) was significantly higher than the mean values at both the UAM site (376.01 ± 214.89 µatm) and the SC site (339.92 ± 85.18 µatm), all of which were significantly different from each other (P < 0.01 for each comparison). Similarly, DO values also varied among seasons (PERMANOVA: Pseudo-F3,6106 = 2614.3, P(perm) < 0.001) and sites with different macroalgal habitat types (Pseudo-F2,6106 = 185.64, P(perm) < 0.001), and these two factors interacted with each other (Season × Site interaction: Pseudo-F6,6106 = 226.31, P(perm) < 0.001) (Table 5). As with pCO2, differences among seasons explained the larges amount of the total variations in DO (54%), while differences among sites explained only 3% and the interaction between season and site explained 14% of this variation. Overall, mean DO values were greatest in winter (11.70 ± 2.49 mg L-1), followed by spring (10.99 ± 2.48 mg L-1), autumn (7.71 ± 0.72 mg L-1), and then summer (7.37 ± 1.25 mg L-1), all of which were significantly different from each other (permutation post hoc tests: P < 0.01 for each comparison). Further, the mean DO value was greatest at the SC site (10.26 ± 1.94 mg L-1), followed by UAM site (10.77 ± 4.12 mg L-1), and then the TAM site (9.16 ± 1.57 mg L-1), all of which were significantly different from each other (P < 0.01 for each comparison). As with DO and pCO2, pHNIST varied among seasons (PERMANOVA: Pseudo-F3,6043 = 4890.40, P(perm) < 0.001) and sites with different macroalgal habitat types (Pseudo-F2,6043 = 246.58, P(perm) < 0.001), and these two factors interacted with each other (Season × Site interaction: Pseudo-F6,6043 = 379.29, P(perm) < 0.001) (Table 5). Also, as with DO and pCO2, differences among seasons explained the largest amount of variation in pHNIST (64%), while differences among sites explained only 3% of this variation and the interaction between season and site explained only 15% of this variation. Overall, pHNIST was highest in winter (8.41 ± 0.12), then decreased in spring (8.21 ± 0.14) and summer (8.13 ± 0.07), and reached their lowest values in autumn (8.05 ± 0.07), all of which were significantly different from each other (permutation post hoc tests: P < 0.01 for each comparison). Furthermore, although pHNIST values appeared very similar, pHNIST at the SC site (8.27 ± 0.12), the UAM site (8.27 ± 0.26), and the TAM site (8.21 ± 0.14) were each significantly different from one another (P < 0.05 for each comparison).
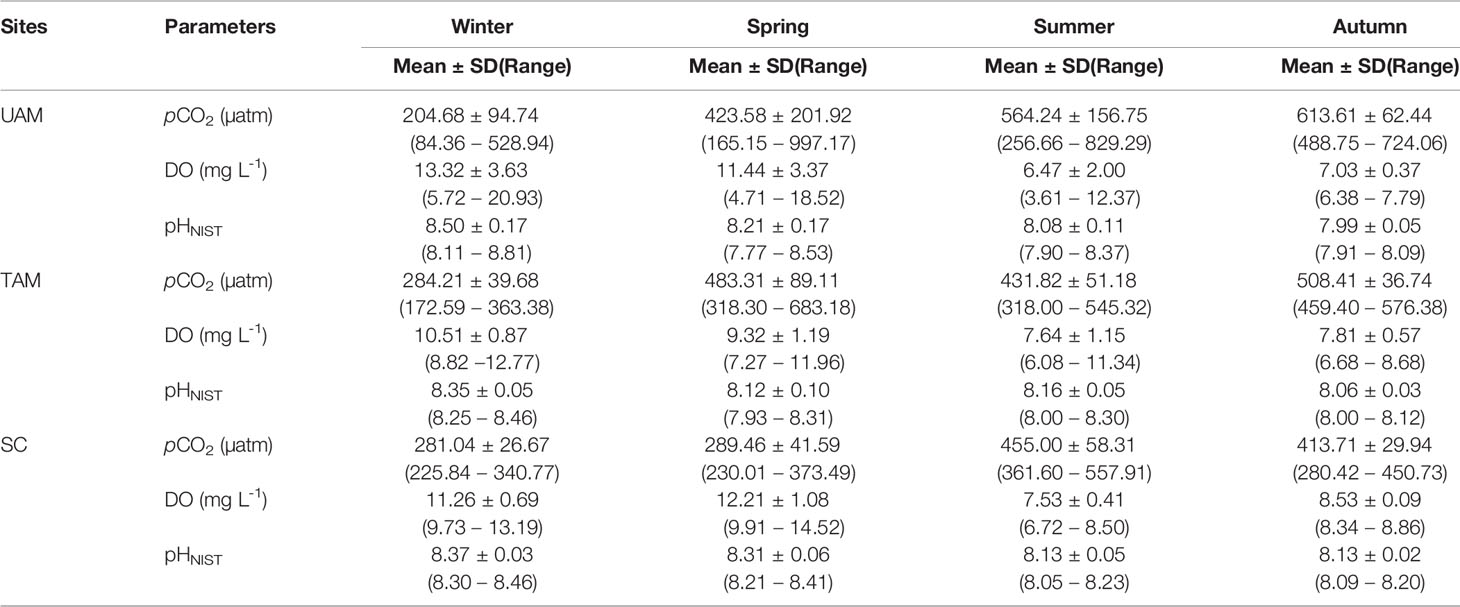
Table 4 The mean ± standard deviation (SD) and variation range of the in-situ monitoring parameters (partial pressure of CO2: pCO2, dissolved oxygen: DO, and pH on the scale of National Institute of Standards and Technology: pHNIST) of three macroalgal habitats (UAM: ulvoid algal mat; TAM: turf algal mat; SC: coexisting Sargassum horneri and crustose coralline algae) in four seasons.
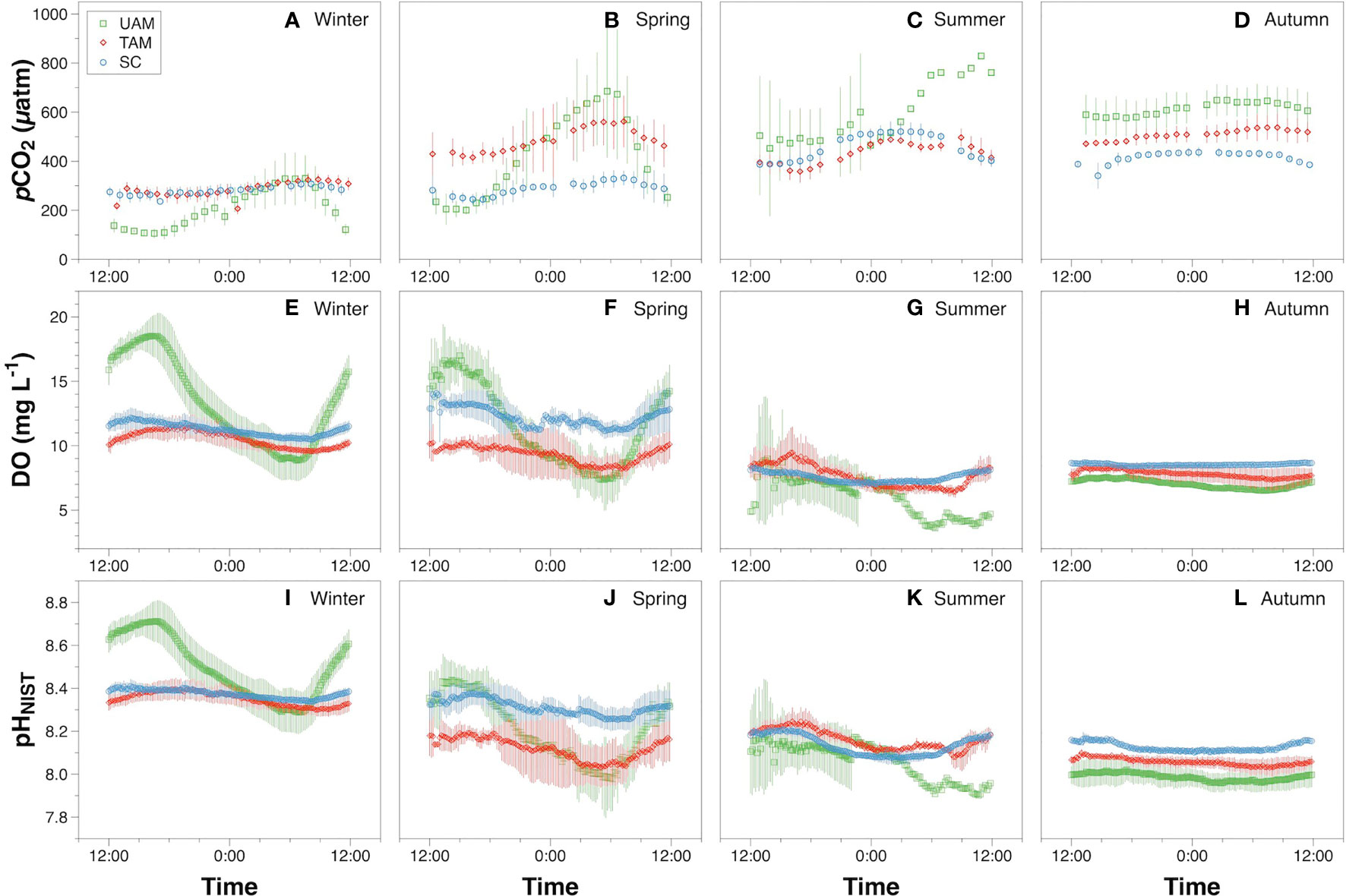
Figure 5 The diel variation of pCO2 (A–D), dissolved oxygen (DO) (E–H) and pHNIST (I–L) at three macroalgal habitats (UAM, ulvoid algal mat; TAM, turf algal mat; SC, coexisting Sargassum horneri and crustose coralline algae) in four seasons. Values are means ± standard deviation. Green squares, red diamonds, and blue circles represent mean values over days 1~7 at the UAM, TAM, SC sites, respectively.
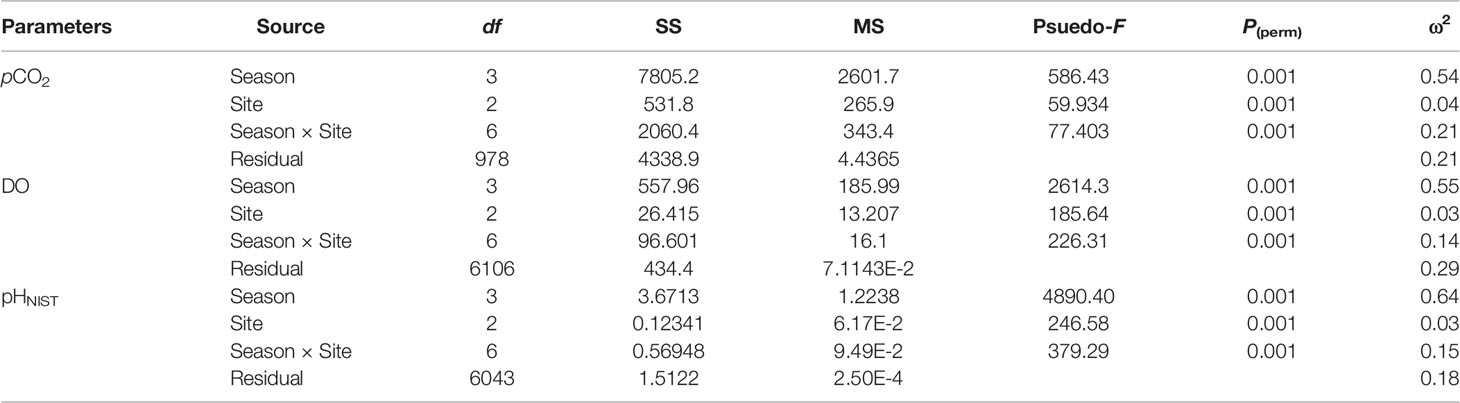
Table 5 Results of two factor Model I PERMANOVAs testing the effects of season and site on the in-situ monitoring parameters (partial pressure of CO2: pCO2, dissolved oxygen concentration: DO, and pH on the scale of National Institute of Standards and Technology: pHNIST). The magnitudes of effect (ω2) represent the percentage of variation in each response variable that is explained by each factor in the models.
The diel variation of DO, pCO2 and pHNIST within the three habitat sites and four seasons all varied significantly among seasons and habitats (Tables 4, 5 and Figure 5). Generally, DO and pHNIST exhibited similar patterns of diel fluctuations (i.e., increasing during the day and decreasing during the night), which were opposite to the fluctuation patterns in pCO2. The UAM site in summer had abnormal patterns of these three parameters since a typhoon occurred during the monitoring period. DO in winter at the UAM site had the largest fluctuation, from 5.72 to 20.93 mg L-1 and with an average value of 13.32 ± 3.63 mg L-1. In contrast, DO in autumn at the SC site had the smallest fluctuation, from 8.34 to 8.86 mg L-1. Both lowest and highest pHNIST values occurred at the UAM site in spring (7.77) and winter (8.81). The largest and smallest fluctuation of pCO2 was at the UAM site in spring (165.15 to 997.17 µatm) and at the SC site in winter (225.84 to 340.77 µatm). Furthermore, pCO2 and DO values in all groups had significantly negative exponential correlations (Figure 6). The UAM site had higher regression coefficients (r2) over 0.9 among four seasons except in autumn (r2 = 0.33). The TAM site had r2 over 0.9 in spring and autumn. The SC site generally had a small range of DO and pCO2, as well as low r2 compared to UAM and TAM sites.
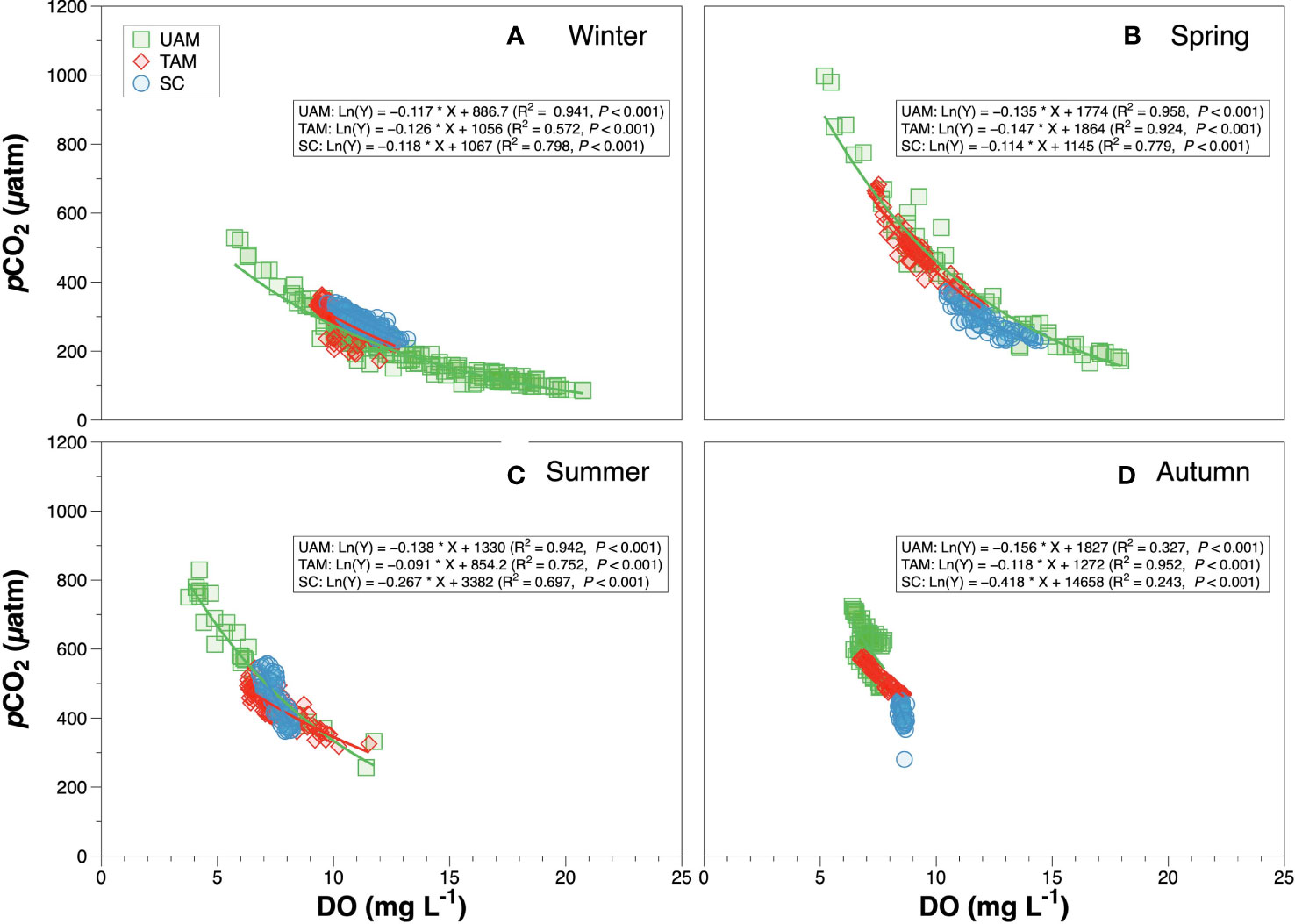
Figure 6 The relationship between pCO2 and dissolved oxygen (DO) of three macroalgal habitats in winter (A), spring (B), summer (C) and autumn (D). Solid lines are the least square fitting curves of data using the non-linear exponential regression model. Values for different macroalgal habitats: ulvoid algal mat (UAM, green square); turf algal mat (TAM, red diamond); coexisting Sargassum horneri and crustose coralline algae (SC, blue circle).
Discussion
We utilized two experimental approaches to investigate diel and seasonal variations in seawater chemistry in order to evaluate how macroalgal community metabolism or/and habitat characters per se influence the seawater chemistry signatures. First, the normalized carbonate chemistry parameters (i.e., nAT, nCT and npHT) were used to compare the effects of biological metabolism after the influences of temperature and salinity were removed by normalization. Hence, we believe that these results could represent the heterogeneity mainly induced by the metabolism of different macroalgal communities, even though they might not show the representative characters of each season. On the other hand, the DO, pCO2, and pHNIST results were used to evaluate the combined effects of both environmental parameters and biological activities on biogeochemical features, which are more appropriate for the ecological implications. Unlike direct carbonate chemistry analysis, simultaneous in-situ monitoring between habitats was not possible because of the limitation in the number of sensors, but this limitation could be compensated by monitoring a wide range of fluctuations for several days. The limitations of above two approaches are complementary and their combined results are sufficient to explain the diel and seasonal heterogeneity among different types of macroalgal habitats.
Diel and Seasonal Variations in Seawater Chemistry
The significant diel variations in seawater chemistry observed in our study were broadly consistent with previous investigations in macrophyte habitats (Chou et al., 2018; Murie and Bourdeau, 2020). Values of pH and DO shared similar diel patterns (i.e., increasing during the daytime and decreasing at nighttime), which were opposite to the diel patterns of nCT and pCO2. Such diel variation followed the metabolic patterns expected for macroalgae, with photosynthesis absorbing and releasing CO2 during the daytime and nighttime, respectively. Besides, macroalgae also serve as habitats for numerous organisms (e.g., meiofauna, macrofauna, and fish) (Christie et al., 2009) and support higher densities of microbes (Weigel and Pfister, 2019). Hence, when evaluating the diel fluctuations of seawater chemistry, it should be kept in mind the contribution of fauna and microbes to community respirations, as well as calcifying organisms to calcification. In our study, the benthic macroflora accounted for over 85% of the total biomass of macroflora and macrofauna (Supplementary Tables 1, 2) at the TAM and SC sites. Moreover, each site has distinguished typical macroalgal communities as described in the study area of methods. Hence, even though macroalgae are not the sole drivers of seawater chemistry variation, it is meaningful to compare the heterogeneity of seawater chemistry from the view of different macroalgal communities. When only the biological metabolic effects of macroalgae were considered, the range of fluctuations in nCT and npHT was the most extensive at the SC site. Wide fluctuations in nCT and npHT at the SC site suggest that high rates of photosynthesis and calcification by CCA, and high productivity of canopy forming algae significantly affect carbonate chemistry parameters. However, the strong biological metabolism at the SC site was offset by hydrological characteristics. Specifically, when hydrological properties were considered, the ranges of DO, pCO2, pHNIST at the UAM site were higher than the ranges at the TAM and SC sites. This discrepancy suggests that diel fluctuations in carbonate chemistry may be affected by interactions between biological metabolism and environmental parameters. At first, ulvoid algae are opportunistic algae due to their high rates of nutrient uptake and then growth (Cohen and Fong, 2006; Bews et al., 2021), which could lead to a high amount of CO2 influx via high rates of photosynthesis and respiration. Previous studies have shown that Ulva spp. had higher values of chlorophyll contents and the maximal quantum yield of photosystem II (Fv/Fm), an indicator of photosynthetic potential, than values of brown algae (e.g., Sargassum spp.) and red algae (e.g., Grateloupia livida) (Shi et al., 2021; Zhao et al., 2021). Carvalho and Eyre (2011) found that Ulva sp. had higher respiration rates than both brown and red algae (i.e., Sargassum sp. and Pterocladia capillacea Bornet). They believed it was because the thinner thallus enables Ulva to have more active cells per unit of carbon, as thinner primary producers have been observed to have higher respiration rates (Enríquez et al., 1996; Sand-Jensen and Nielsen, 2004). In addition, the UAM site is located in the inner harbor where there are direct sewage inputs. Hence, the relatively high nutrient concentrations might contribute to the high rates of metabolic activity (photosynthesis and respiration) in ulvoid algae, resulting in the largest fluctuation of DO and pCO2 at UAM site (Bews et al., 2021). Furthermore, higher nutrient concentrations increased the biomass of microbes (Lebaron et al., 2001), and DO is consumed and pCO2 is released due to microbial respiration as part of the overall degradation process of organic matter in the blooming area (Chen et al., 2020). Therefore, biogeochemical fluctuations are more dynamically driven by photosynthesis of autotrophs, and respiration of autotrophs and microbial community. Another factor is that the seawater exchange with the open ocean is less at the UAM and TAM sites than at the SC site. The low rate of water exchange better highlighted the metabolic-induced variations (Semesi et al., 2009). In addition, the mixing between seawater and sewage containing high organic carbon also affected the carbonate chemistry of seawater (Kim et al., 2006). This might cause the abnormal ratios of nAT and nCT at the UAM and TAM sites in summer and autumn, which differed from the ratios at the SC site (Figure 3). Consequently, the heterogeneity of seawater chemistry across the three macroalgal habitats can be affected by both biological metabolisms and hydrological characteristics within the site.
The seasonal variation in seawater carbonate parameters among macroalgae habitats were also observed in other studies, though their patterns differed from those found in our study (Delille et al., 2000; Delille et al., 2009). Specifically, pCO2 values in our study were lowest in winter and then increased in spring and summer, meanwhile DO values followed the opposite patterns. In contrast, Delille et al. (2009) found that pCO2 values around kelp beds in the sub-Antarctic coast were relatively low in summer and spring, reached a high oversaturation of pCO2 in autumn, and then decreased thereafter. These seasonal changes in the study of Delille et al. (2009) could be induced by the higher photosynthetic rates in spring and summer, and the decomposition of organic matter in autumn. A possible explanation for the difference of pCO2 patterns between our study and Delille et al. (2009) might be the different ranges in seawater temperatures over seasons, and different growth patterns of the dominant macroalgae. In our study area, the variation of seawater temperature throughout the year was from 7.75 to 26.63°C, which was higher than the range (about 2 to 8°C) of sub-Antarctic coast in the study of Delille et al. (2009). In our study, the high temperature in summer (UAM: 23.03 to 24.93°C, TAM: 17.50 to 24.71°C, SC: 24.16 to 26.63°C) might inhibit the photosynthesis of macroalgae. For example, Ulva spp. has its highest growth rates at 15-20°C, and decreases in temperatures over 20°C (Taylor et al., 2001). Additionally, Ulva australis biomass along the south coast of Korean peaks in May and drops significantly in June, meanwhile photosynthetic rates are highest from January to March during the growth period and lowest from May to July when the biomass peaks or declines (Kim et al., 2004). Thus, our results indicate that the growth optimal temperature can be one of the potent factors for influencing the seawater biogeochemical parameters. However, it should be noted that our study focused on the investigation of seawater carbonate parameters without the measurements of biomass and photosynthetic performances of the corresponding macroalgae. Our data identified seasonal variation in seawater chemistry within macroalgal communities, but further studies could test the physiological performance of seaweed in different seasons to help understand how activities of seaweed affect the seawater carbon dynamics (Spector and Edwards, 2020). Furthermore, apart from the growth rhythms of macroalgae themselves, the abiotic factors also had important impacts on the metabolism of macroalgae. Hence, besides temperature and salinity, water depth, light intensity, and nutrient concentration, the physical condition of seawater should be recorded in the further study to fully understand the process of seaweed communities altering the seawater carbonate conditions in a varying environment.
Relationship of nAT-nCT
The relationship between AT and CT has been used as a tool to quantify the relative contributions of ecosystem metabolism to seawater chemistry changes occurring in various aquatic habitats (Dutta et al., 2019; Baldry et al., 2020; Saderne et al., 2020). The variation in stoichiometry between these two carbonate parameters represents relative intensities of biological activities including photosynthesis (and respiration), calcification (and dissolution), nitrification (and denitrification), sulfate reduction, and fermentation (Saderne et al., 2020). In our study, the slope values (relationships) in winter were from –0.12 to 0.02, indicating that photosynthesis and respiration were governing factors for carbon chemistry variations. Especially, the UAM and TAM sites had high % NCP values exceeding 90%. In spring, even though the slopes increased slightly compared to winter slopes, the activity of photosynthesis and respiration still were the major drivers of the carbonate chemistry dynamics. However, the slope values in summer and autumn were lower than –0.2, which appeared to violate the stoichiometry nAT/nCT ratios predicted for photosynthesis/respiration. The abnormal phenomenon might be caused by the inflow of external water with different values of nAT in summer and autumn. As the UAM and TAM sites are located in the harbor, their seawater conditions are easy to be changed by the inflow of wastewater, especially in summer. The field investigation (Kim et al., 2004), in which the photosynthetic rate of ulvoid green algae was lowest in July, suggested that the low nAT/nCT slopes in summer found in our study were not mainly caused by the photosynthesis of macroalgae. Actually, previous studies have shown that the magnitude of change along this AT-CT ratio is driven by metabolic rates that are strongly dependent on physical factors including light intensity (Gattuso et al., 1996; Takeshita et al., 2016), residence time, and water depth (Falter et al., 2013). Our study further strengthened such viewpoint and highlighted that the wastewater might affect the seawater carbon dynamics within the short time scale.
Relationship of pCO2-DO
The relationship of pCO2-DO depends on several physical (e.g., temperature, salinity and air-sea exchange) and biological (e.g., photosynthesis, respiration, and calcification) processes (DeGrandpre et al., 1998). Studies have evaluated the ocean metabolic status via the analysis of combined CO2 and O2 data (DeGrandpre et al., 1997; DeGrandpre et al., 1998; Zhai et al., 2009). In our study, pCO2 and DO values were negatively correlated (Figure 5), especially in winter and spring, which are consistent with previous findings (Noriega and Araujo, 2014). The oversaturation of DO corresponding to the low pCO2 values in winter and spring, indicated that macroalgae possessed high photosynthesis rates during this period, which agrees with our nAT-nCT data and the growth patterns of macroalgae in the Korean coast (Kim et al., 2004). In addition, the UAM site had high pCO2 values with unsaturated DO, suggesting that the aerobic respiration rates were high. Such condition might occur because the UAM site in the inner harbor was directly influenced by sewage inputs. Meanwhile, the rates of organic matter decomposition increased with rising temperature, leading to the low DO and high pCO2 values in summer. Previous studies have shown that the nutrient and organic matter inputs from riverine outflows and sewage effluents have increased the occurrences of hypoxia and high CO2 concentrations in many coastal waters (Borges et al., 2006; Diaz and Rosenberg, 2008). In autumn, the narrow ranges of pCO2 and DO might be related to the decrease in macroalgae biomass after several storms. Even though the accurate investigation is lacking, we observed the obvious decline of macroalgae during the set-up of sensors in autumn. Altogether, results of pCO2 and DO are important for understanding the variation in biological processes among different types of macroalgal habitats, and they could be used as an important biochemical indicator to understand the fate of carbon dioxide within macroalgal communities (e.g., possibly convert DO-based NCP to CO2 based NCP).
Ecological Implication
The average value of atmospheric CO2 has risen to 415.48 ppm June 2021 due to anthropogenic activities (Dlugokencky and Tans, 2021), and is predicted to reach 936 ppm for the representative concentration pathway (RCP) 8.5 by 2100 (IPCC, 2013). As 20-30% of global emissions of CO2 are absorbed by the ocean, seawater pH has decreased to 8.1 (Caldeira and Wickett, 2003) and will drop by 0.036 to 0.291 units for the RCPs 2.6-8.5 by 2081-2100 (IPCC, 2019). According to our pCO2 and pHNIST data, averaged values of pCO2 in winter and spring were lower than the current concentrations and pHNIST were higher than 8.1, which means seaweed communities could mitigate OA stress during their growth seasons when photosynthesis rates are high. In summer and autumn, however, seaweed communities might exacerbate the effects of OA through respiration, as average values of pCO2 were higher than current average values. Specifically, the average pCO2 increased to 522.33 ± 91.79 µatm in autumn, resulting the low pHNIST value of 8.05 ± 0.07. Again, such situations should consider both the condition of the macroalgae and ambient environmental factors, because as temperature increase, macroalgae began to die and decompose, resulting in decreased photosynthesis and increased aerobic respiration. Our investigation highlights that macroalgae serve dual roles in OA mitigation by decreasing OA stress during the winter and spring (the growing season), but worsening OA in summer and autumn.
Conclusion
This study investigated the dynamics of carbonate chemistry and dissolved oxygen in the benthic macroalgal habitats, which in turn reflected how the metabolic activities of macroalgae regulated seawater chemistry and its implications for mitigating OA stress. Our results illustrated that macroalgal photosynthesis could largely mitigate the OA stress in winter and spring when photosynthesis is high. Meanwhile the high photosynthesis rates by the dominant macroalgae also result in large diel fluctuations in seawater carbonate parameters in winter and spring. However, these mitigation effects were dependent on temperature, which determined the growth condition and photosynthetic rates of the macroalgae. Once temperature exceeds the optimum ranges for these macroalgae in summer and autumn, respiration rates can surpass photosynthesis rates, and macroalgal communities could reversely increase CO2 concentrations, thereby enhancing OA stress. Our study highlights that the role of seaweeds in regulating the seawater chemistry, especially acidification, should be evaluated in a long-term scale considering their metabolic interactions with multi-environmental factors.
Data Availability Statement
The raw data supporting the conclusions of this article will be made available by the authors, without undue reservation.
Author Contributions
HL analyzed data and wrote the draft manuscript. HM conducted the experiment, sample collection and analysis, collected data, wrote the draft manuscript. EJK, J-MK, MK, KL, C-WK, HK, I-NK, KYP, YKL, JWJ and ME designed the field experiment, sample collection and analysis, data discussion. J-HK supervised the project and reviewed the draft manuscript. All authors reviewed and approved the manuscript.
Funding
This research was supported by following research grants: Basic Science Research Program through the National Research Foundation of Korea (NRF) funded by the Korean government (MIST) NRF-2021R1A2C4002298 to J-HK, NRF-2022R1C1C2008739 to EJK, and NRF-2020R1A4A1018818 to KL. Also, this work was partially supported by Ministry of Oceans and Fisheries (MOF) Korea (project title: Techniques development for management and evaluation of biofouling on ship hull; No. 20210651), Korea Institute of Ocean Science and Technology (grant number PEA 0016) to J-HK and HK and Korea Fisheries Resources Agency (FIRA) to J-HK and KL.
Conflict of Interest
The authors declare that the research was conducted in the absence of any commercial or financial relationships that could be construed as a potential conflict of interest.
Publisher’s Note
All claims expressed in this article are solely those of the authors and do not necessarily represent those of their affiliated organizations, or those of the publisher, the editors and the reviewers. Any product that may be evaluated in this article, or claim that may be made by its manufacturer, is not guaranteed or endorsed by the publisher.
Acknowledgments
The authors thank to Sukyeon Lee, Nahyun Kim, and Min Ji Park for their field assistance and technical support.
Supplementary Material
The Supplementary Material for this article can be found online at: https://www.frontiersin.org/articles/10.3389/fmars.2022.857153/full#supplementary-material
References
Anderson M. J., Walsh D. C. (2013). PERMANOVA, ANOSIM, and the Mantel Test in the Face of Heterogeneous Dispersions: What Null Hypothesis are You Testing? Ecol. Monogr. 83, 557–574. doi: 10.1890/12-2010.1
Baldry K., Saderne V., McCorkle D. C., Churchill J. H., Agusti S., Duarte C. M. (2020). Anomalies in the Carbonate System of Red Sea Coastal Habitats. Biogeosciences 17, 423–439. doi: 10.5194/bg-17-423-2020
Bews E., Booher L., Polizzi T., Long C., Kim J.-H., Edwards M. S. (2021). Effects of Salinity and Nutrients on Metabolism and Growth of Ulva lactuca: Implications for Bioremediation of Coastal Watersheds. Mar. Pollut. Bull. 166, 112199. doi: 10.1016/j.marpolbul.2021.112199
Borges A. V., Schiettecatte L. S., Abril G., Delille B., Gazeau F. (2006). Carbon Dioxide in European Coastal Waters. Estuar. Coast. Shelf. Sci. 70, 375–387. doi: 10.1016/j.ecss.2006.05.046
Britton D., Cornwall C. E., Revill A. T., Hurd C. L., Johnson C. R. (2016). Ocean Acidification Reverses the Positive Effects of Seawater pH Fluctuations on Growth and Photosynthesis of the Habitat-Forming Kelp, Ecklonia radiata. Sci. Rep. 6, 26036. doi: 10.1038/srep26036
Cai W. J., Xu Y. Y., Feely R. A., Wanninkhof R., Jonsson B., Alin S. R. (2020). Controls on Surface Water Carbonate Chemistry Along North American Ocean Margins. Nat. Commun. 11, 2691. doi: 10.1038/s41467-020-16530-z
Caldeira K., Wickett M. E. (2003). Anthropogenic Carbon and Ocean pH. Nature 425, 365. doi: 10.1038/425365a
Carrano M. W., Carrano C. J., Edwards M. S., Al-Adilah H., Fontana Y., Sayer M. D. J., et al. (2021). Laminaria Kelps Impact Iodine Speciation Chemistry in Coastal Seawater. Estuar. Coast. Shelf. Sci. 262, 107531. doi: 10.1016/j.ecss.2021.107531
Carrano M. W., Yarimizu K., Conzales J. L., Cruz-López R., Edwards M. S., Tymon T., et al. (2020). The Influence of Marine Algae on Iodine Speciation in the Coastal Ocean. Algae 35, 167–176. doi: 10.4490/algae.2020.35.5.25
Carvalho M. C., Eyre B. D. (2011). Carbon Stable Isotope Discrimination During Respiration in Three Seaweed Species. Mar. Ecol. Prog. Ser. 437, 41–49. doi: 10.3354/meps09300
Chen J., Li H., Zhang Z., He C., Shi Q., Jiao N., et al. (2020). DOC Dyanmics and Bacterial Community Succession During Long-Term Degradation of Ulva prolifera and Their Implications for the Legacy Effect of Green-Tides on Refractory DOC Pool in Seawater. Water Res. 185, 116268. doi: 10.1016/j.watres.2020.116268
Chou W.-C., Chu H.-C., Chen Y.-H., Syu R.-W., Hung C.-C., Soong K. (2018). Short-Term Variability of Carbon Chemistry in Two Contrasting Seagrass Meadows at Dongsha Island: Implications for pH Buffering and CO2 Sequestration. Estuar. Coast. Shelf. Sci. 210, 36–44. doi: 10.1016/j.ecss.2018.06.006
Christie H., Norderhaug K. M., Fredriksen S. (2009). Macrophytes as Habitat for Fauna. Mar. Ecol. Prog. Ser. 396, 221–233. doi: 10.3354/meps08351
Clayton T. D., Byrne R. H. (1993). Spectrophotometric Seawater pH Measurements: Total Hydrogen Ion Concentration Scale Calibration of M-Cresol Purple and at-Sea Results. Dee. Sea. Res. Part I. Oceanogr. Res. Pap. 40, 2115–2129. doi: 10.1016/0967-0637(93)90048-8
Cohen R. A., Fong P. (2006). Using Opportunistic Green Macroalgae as Indicators of Nitrogen Supply and Sources to Estuaries. Ecol. Appl. 16, 1405–1420. doi: 10.1890/1051-0761(2006)016[1405:UOGMAI]2.0.CO;2
Comeau S., Cornwall C. E. (2016). “Contrasting Effects of Ocean Acidification on Coral Reef “Animal Forests” Versus Seaweed “Kelp Forests”, in Marine Animal Forests. eds. Rossi S., Bramanti L., Gori A., Orejas Saco del Valle C. (Cham: Springer), 1–25. doi: 10.1007/978-3-319-17001-5_29-1
Cyronak T., Andersson A. J., Langdon C., Albright R., Bates N. R., Caldeira K., et al. (2018). Taking the Metabolic Pulse of the World’s Coral Reefs. PloS One 13, e0190872. doi: 10.1371/journal.pone.0190872
DeGrandpre M. D., Hammar T. R., Wallace D. W. R., Wirick C. D. (1997). Simultaneous Mooring-Based Measurements of Seawater CO2 and O2 Off Cape Hatteras, North Carolina. Limnol. Oceanogr. 42, 21–28. doi: 10.4319/lo.1997.42.1.0021
DeGrandpre M. D., Hammar T. R., Wirick C. D. (1998). Short-Term pCO2 and O2 Dynamics in California Coastal Waters. Deep. Sea. Res. Part II Top. Stud. Oceanogr. 45, 1557–1575. doi: 10.1016/S0967-0645(98)80006-4
Delille B., Borges A. V., Delille D. (2009). Influence of Giant Kelp Beds (Macrocystis pyrifera) on Diel Cycles of pCO2 and DIC in the Sub-Antarctic Coastal Area. Estuar. Coast. Shelf. Sci. 81, 114–122. doi: 10.1016/j.ecss.2008.10.004
Delille B., Delille D., Fiala M., Prevost C., Frankignoulle M. (2000). Seasonal Changes of pCO2 Over a Subantarctic Macrocystis Kelp Bed. Polar. Biol. 23, 706–716. doi: 10.1007/s003000000142
Deng X., Liu T., Liu C.-Y., Liang S.-K., Hu Y.-B., Jin Y.-M., et al. (2018). Effects of Ulva prolifera Blooms on the Carbonate System in the Coastal Waters of Qingdao. Mar. Ecol. Prog. Ser. 605, 73–86. doi: 10.3354/meps12739
Diaz R. J., Rosenberg R. (2008). Spreading Dead Zones and Consequences for Marine Ecosystems. Science 321, 926–929. doi: 10.1126/science.1156401
Dickson A. G. (1993). The Measurement of Sea Water pH. Mar. Chem. 44, 131–142. doi: 10.1016/0304-4203(93)90198-W
Dickson A. G., Sabine C. L., Christian J. R. (eds.) (2007). Guide to Best Practices for Ocean CO2 Measurements Vol. 3 (Sidney, BC: PICES Special Publication). doi: 10.25607/OBP-1342
Dlugokencky E., Tans P. (2021) Trends in Atmospheric Carbon Dioxide (NOAA/GML). Available at: https://gml.noaa.gov/ccgg/trends/global.html (accessed Sep. 28, 2021).
Doney S. C., Fabry V. J., Feely R. A., Kleypas J. A. (2009). Ocean Acidification: The Other CO2 Problem. Ann. Rev. Mar. Sci. 1, 169–192. doi: 10.1146/annurev.marine.010908.163834
Dutta M. K., Kumar S., Mukherjee R., Sharma N., Acharya A., Sanyal P., et al. (2019). Diurnal Carbon Dynamics in a Mangrove-Dominated Tropical Estuary (Sundarbans, India). Estuar. Coast. Shelf. Sci. 229, 106426. doi: 10.1016/j.ecss.2019.106426
Edwards M. S., Kornar B., Kim J.-H., Gabara S., Sullaway G., McHugh T. A., et al. (2020). Marine Deforestation Leads to Widespread Loss of Ecosystem Function. PloS One 15, e0226173. doi: 10.1371/journal.pone.0226173
Enríquez S., Duarte C. M., Sand-Jensen K., Nielsen S. L. (1996). Broad-Scale Comparison of Photosynthetic Rates Across Phototrophic Organisms. Oecologia 108, 197–206. doi: 10.1007/BF00334642
Falter J. L., Lowe R. J., Zhang Z., McCulloch M. (2013). Physical and Biological Controls on the Carbonate Chemistry of Coral Reef Waters: Effects of Metabolism, Wave Forcing, Sea Level, and Geomorphology. PloS One 8, e53303. doi: 10.1371/journal.pone.0053303
Frieder C. A., Nam S. H., Martz T. R., Levin L. A. (2012). High Temporal and Spatial Variability of Dissolved Oxygen and pH in a Nearshore California Kelp Forest. Biogeosciences 9, 3917–3930. doi: 10.5194/bg-9-3917-2012
Gattuso J. P., Pichon M., Delesalle B., Canon C., Frankignoulle M. (1996). Carbon Fluxes in Coral Reefs. I. Lagrangian Measurement of Community Metabolism and Resulting Air-Sea CO2 Disequilibrium. Mar. Ecol. Prog. Ser. 145, 109–121. doi: 10.3354/meps145109
Gonzales J., Tymon T., Küpper F. C., Edwards M. S., Carrano C. J. (2017). The Potential Role of Kelp Forests on Iodine Speciation in Coastal Seawater. PloS One 12, e0180755. doi: 10.1371/journal.pone.0180755
Graham M. H., Edwards M. S. (2001). Statistical Significance Versus Factor Fit: Estimating the Importance of Individual Factor in Ecological Analysis of Variance. Oikos 93, 505–513. doi: 10.1034/j.1600-0706.2001.930317.x
Hofmann G. E., Smith J. E., Johnson K. S., Send U., Levin L. A., Micheli F., et al. (2011). High-Frequency Dynamics of Ocean pH: A Multi-Ecosystem Comparison. PloS One 6, e28983. doi: 10.1371/journal.pone.0028983
IPCC (2013). Climate Change 2013: The Physical Science Basis. Contribution of Working Group I to the Fifth Assessment Report of the Intergovernmental Panel on Climate Change, eds. Stocker T. F., Qin D., Plattner G.-K., Tignor M., Allen S. K., Boschung J., et al. (Cambridge: Cambridge University Press), 1535. doi: 10.1017/CBO9781107415324
IPCC (2019). IPCC Special Report on the Ocean and Cryosphere in a Changing Climate. eds. Pörtner H.-O., Roberts D. C., Masson-Delmotte V., Zhai P., Tignor M., Poloczanska E., et al. Available at: https://www.ipcc.ch/report/srocc/ (accessed Oct. 25, 2021).
Kang E. J., Han A.-R., Kim J.-H., Kim I.-N., Lee S., Min J.-O., et al. (2021). Evaluating Bloom Potential of the Green-Tide Forming Alga Ulva ohnoi Under Ocean Acidification and Warming. Sci. Total. Environ. 769, 144443. doi: 10.1016/j.scitotenv.2020.144443
Kim K. Y., Choi T. S., Kim J. H., Han T., Shin H. W., Garbary D. J. (2004). Physiological Ecology and Seasonality of Ulva pertusa on a Temperate Rocky Shore. Phycologia 43, 483–492. doi: 10.2216/i0031-8884-43-4-483.1
Kim J.-H., Kang E. J., Kim K., Jeong H. J., Lee K., Edwards M. S., et al. (2015). Evaluation of Carbon Fluxes in Vegetative Bay Based on Ecosystem Production and CO2 Exchange Driven by Coastal Autotrophs. Algae 30, 121–137. doi: 10.4490/algae.2015.30.2.121
Kim J.-H., Kim N., Moon H., Lee S., Jeong S. Y., Diaz-Pulido G., et al. (2020a). Global Warming Offsets the Ecophysiological Stress of Ocean Acidification on Temperate Crustose Coralline Algae. Mar. Pollut. Bull. 157, 111324. doi: 10.1016/j.marpolbul.2020.111324
Kim H.-C., Lee K., Choi W. (2006). Contribution of Phytoplankton and Bacterial Cells to the Measured Alkalinity of Seawater. Limnol. Oceanogr. 51, 331–338. doi: 10.4319/lo.2006.51.1.0331
Kim J.-M., Lee K., Han I.-S., Lee J.-S., Choi Y. H., Lee J.-H., et al. (2020b). Anthropogenic Nitrogen-Induced Changes in Seasonal Carbonate Dynamics in a Productive Coastal Environment. Geophys. Res. Lett. 47, e2020GL088232. doi: 10.1029/2020GL088232
Ko Y. H., Lee K., Noh J. H., Lee C. M., Kleypas J. A., Jeong H. J., et al. (2016). Influence of Ambient Water Intrusion on Coral Reef Acidification in the Chuuk Lagoon, Located in the Coral-Rich Western Pacific Ocean. Geophys. Ress. Lett. 43, 3830–3838. doi: 10.1002/2016gl068234
Koweek D. A., Nickols K. J., Leary P. R., Litvin S. Y., Bell T. W., Luthin T., et al. (2017). A Year in the Life of a Central California Kelp Forest: Physical and Biological Insights Into Biogeochemical Variability. Biogeosciences 14, 31–44. doi: 10.5194/bg-14-31-2017
Krause-Jensen D., Duarte C. M., Hendriks I. E., Meire L., Blicher M. E., Marbà N., et al. (2015). Macroalgae Contribute to Nested Mosaics of pH Variability in a Subarctic Fjord. Biogeosciences 12, 4895–4911. doi: 10.5194/bg-12-4895-2015
Krumins V., Gehlen M., Arndt S., Van Cappellen P., Regnier P. (2013). Dissolved Inorganic Carbon and Alkalinity Fluxes From Coastal Marine Sediments: Model Estimates for Different Shelf Environments and Sensitivity to Global Change. Biogeosciences 10, 371–398. doi: 10.5194/bg-10-371-2013
Kuss J., Roeder W., Wlost K. P., DeGrandpre M. D. (2006). Time-Series of Surface Water CO2 and Oxygen Measurements on a Platform in the Central Arkona Sea (Baltic Sea): Seasonality of Uptake and Release. Mar. Chem. 101, 220–232. doi: 10.1016/j.marchem.2006.03.004
Lebaron P., Servais P., Troussellier M., Courties C., Muyzer G., Bernard L., et al. (2001). Microbial Community Dynamics in Mediterranean Nutrient-Enriched Seawater Mesocosms: Changes in Abundances, Activity and Composition, FEMS Microbiol. Ecol 34, 255–266. doi: 10.1016/S0168-6496(00)00103-3
Lee J. E., Kang J. W. (2020). The Interactive Effects of Elevated Temperature and Nutrient Concentrations on the Physiological Responses of Ulva linza Linnaeus (Ulvales, Chlorophyta). J. Appl. Phycol. 32, 2459–2467. doi: 10.1007/s10811-019-02031-0
Lee C.-H., Lee K., Ko Y. H., Lee J.-S. (2021). Contribution of Marine Phytoplankton and Bacteria to Alkalinity: An Uncharacterized Component. Geophys. Res. Lett. 48, e2021GL093738. doi: 10.1029/2021GL093738
Lee K., Sabine C. L., Tanhua T., Kim T.-W., Feely R. A., Kim H.-C. (2011). Roles of Marginal Seas in Absorbing and Storing Fossil Fuel CO2. Energy Environ. Sci. 4, 1133–1146. doi: 10.1039/c0ee00663g
Li J., Zhang W., Ding J., Xue S., Huo E., Ma Z., et al. (2021). Effect of Large-Scale Kelp and Bivalve Farming on Seawater Carbonate System Variations in the Semi-Enclosed Sanggou Bay. Sci. Total. Environ. 753, 142065. doi: 10.1016/j.scitotenv.2020.142065
Metzger J. R., Konar B., Edwards M. S. (2019). Assessing a Macroalgal Foundation Species: Community Variation With Shifting Algal Assemblages. Mar. Biol. 166, 156. doi: 10.1007/s00227-019-3606-1
Middelboe A. L., Hansen P. J. (2007). High pH in Shallow-Water Macroalgal Habitats. Mar. Ecol. Prog. Ser. 338, 107–117. doi: 10.3354/meps338107
Millero F. J., Zhang J. Z., Lee K., Campbell D. M. (1993). Titration Alkalinity of Seawater. Mar. Chem. 44, 153–165. doi: 10.1016/0304-4203(93)90200-8
Mineur F., Arenas F., Assis J., Davies A. J., Engelen A. H., Fernandes F., et al. (2015). European Seaweeds Under Pressure: Consequences for Communities and Ecosystem Functioning. J. Sea. Res. 98, 91–108. doi: 10.1016/j.seares.2014.11.004
Murie K. A., Bourdeau P. E. (2020). Fragmented Kelp Forest Canopies Retain Their Ability to Alter Local Seawater Chemistry. Sci. Rep. 10, 11939. doi: 10.1038/s41598-020-68841-2
Noriega C., Araujo M. (2014). Carbon Dioxide Emissions From Estuaries of Northern and Northeastern Brazil. Sci. Rep. 4, 6164. doi: 10.1038/srep06164
Park G.-H., Lee K., Tishchenko P., Min D.-H., Warner M. J., Talley L. D., et al. (2006). Large Accumulation of Anthropogenic CO2 in the East (Japan) Sea and its Significant Impact on Carbonate Chemistry. Glob. Biogeochem. Cycle. 20, GB4013. doi: 10.1029/2005gb002676
Pfister C. A., Altabet M. A., Weigel B. L. (2019). Kelp Beds and Their Local Effects on Seawater Chemistry, Productivity, and Microbial Communities. Ecology 100, e02798. doi: 10.1002/ecy.2798
QGIS Development Team (2021) QGIS Geographic Information System. Available at: http://qgis.osgeo.org.
Ragazzola F., Kolzenburg R., Adani M., Bordone A., Cantoni C., Cerrati G., et al. (2021). Carbonate Chemistry and Temperature Dynamics in an Alga Dominated Habitat. Reg. Stud. Mar. Sci. 44, 101770. doi: 10.1016/j.rsma.2021.101770
Saderne V., Fusi M., Thomson T., Dunne A., Mahmud F., Roth F., et al. (2020). Total Alkalinity Production in a Mangrove Ecosystem Reveals an Overlooked Blue Carbon Component. Limnol. Oceanogr. Lett. 6, 61–67. doi: 10.1002/lol2.10170
Sand-Jensen K., Nielsen S. L. (2004). “Estuarine Primary Producers,” in Estuarine Nutrient Cycling: The Influence of Primary Producers. Aquatic Ecology Book Series. eds. Nielsen S. L., Banta G. T., Pedersen M. F. (Dordrecht: Springer), 17–57. doi: 10.1007/978-1-4020-3021-5_2
Semesi I. S., Beer S., Björk M. (2009). Seagrass Photosynthesis Controls Rates of Calcification and Photosynthesis of Calcareous Macroalgae in a Tropical Seagrass Meadow. Mar. Ecol. Prog. Ser. 382, 41–47. doi: 10.3354/meps07973
Shi X., Zou D., Hu S., Mai G., Ma Z., Li G. (2021). Photosynthetic Characteristics of Three Cohabitated Macroalgae in the Daya Bay, and Their Responses to Temperature Rises. Plants 10, 2441. doi: 10.3390/plants10112441
Sippo J. Z., Maher D. T., Tait D. R., Holloway C., Santos I. R. (2016). Are Mangroves Drivers or Buffers of Coastal Acidification? Insights From Alkalinity and Dissolved Inorganic Carbon Export Estimates Across a Latitudinal Transect. Global Biogeochem. Cycle. 30, 753–766. doi: 10.1002/2015gb005324
Spector M., Edwards M. S. (2020). Species-Specific Biomass Drives Macroalgal Benthic Primary Production on Temperate Recky Reefs. Algae 35, 237–252. doi: 10.4490/algae.2020.35.8.19
Sullaway G. H., Edwards M. S. (2020). Impacts of the non-Native Algal Sargassum horneri on Benthic Primary Production in a California Kelp Forest. Mar. Ecol. Prog. Ser. 637, 45–57. doi: 10.3354/meps13231
Takeshita Y., McGillis W., Briggs E. M., Carter A. L., Donham E. M., Martz T. R., et al. (2016). Assessment of Net Community Production and Calcification of a Coral Reef Using a Boundary Layer Approach. J. Geophys. Res. Ocean. 121, 5655–5671. doi: 10.1002/2016jc011886
Taylor R., Fletcher R. L., Raven J. A. (2001). Preliminary Studies on the Growth of Selected ‘Green Tide’ Algae in Laboratory Culture: Effects of Irradiance, Temperature, Salinity and Nutrients on Growth Rate. Bot. Mar. 44, 327–336. doi: 10.1515/BOT.2001.042
Turk D., Yates K. K., Vega-Rodriguez M., Toro-Farmer G., L’Esperance C., Melo N., et al. (2015). Community Metabolism in Shallow Coral Reef and Seagrass Ecosystems, Lower Florida Keys. Mar. Ecol. Prog. Ser. 538, 35–52. doi: 10.3354/meps11385
Vachon D., Sadro S., Bogard M. J., Lapierre J.-F., Baulch H. M., Rusak J. A., et al. (2020). Paired O2-CO2 Measurements Provide Emergent Insights Into Aquatic Ecosystem Function. Limnol. Oceanogr. Lett. 5, 287–294. doi: 10.1002/lol2.10135
Wahl M., Schneider Covachã S., Saderne V., Hiebenthal C., Müller J. D., Pansch C., et al. (2018). Macroalgae may Mitigate Ocean Acidification Effects on Mussel Calcification by Increasing pH and its Fluctuations. Limnol. Oceanogr. 63, 3–21. doi: 10.1002/lno.10608
Weigel B. L., Pfister C. A. (2019). Successional Dynamics and Seascape-Level Patterns of Microbial Communities on the Canopy-Forming Kelps Nereocystis luetkeana and Macrocystis pyrifera. Front. Microbiol. 10, 346. doi: 10.3389/fmicb.2019.00346
Xiao X., Agusti S., Yu Y., Huang Y., Chen W., Hu J., et al. (2021). Seaweed Farms Provide Refugia From Ocean Acidification. Sci. Total. Environ. 776, 145192. doi: 10.1016/j.scitotenv.2021.145192
Yoo J. S., Park I. S., Song Y. C., Seo Y. W., Doe G. Y., Lee J. W., et al. (2006). Characteristics and Structure of Benthic Algal Community in Pohang New Port Area. J. Navig. Port. Res. 30, 309–314. doi: 10.5394/KINPR.2006.30.4.309
Zhai W., Dai M. (2009). On the Seasonal Variation of Air – Sea CO2 Fluxes in the Outer Changjiang (Yangtze River) Estuary, East China Sea. Mar. Chem. 117, 2–10. doi: 10.1016/j.marchem.2009.02.008
Zhai W. D., Dai M., Cai W. J. (2009). Coupling of Surface pCO2 and Dissolved Oxygen in the Northern South China Sea: Impacts of Contrasting Coastal Processes. Biogeosciences 6, 2589–2598. doi: 10.5194/bg-6-2589-2009
Zhao X., Zhong Y., Zhang H., Qu T., Hou C., Guan C., et al. (2021). Comparison of Environmental Responding Strategies Between Ulva prolifera and Sargassum horneri: An In-Situ Study During the Co-Occurrence of Green Tides and Golden Tides in the Yellow Sea, China in 2017. J. Oceanol. Limnol. 39, 2252–2266. doi: 10.1007/s00343-021-0397-2
Keywords: biogeochemical interaction, carbonate chemistry, dissolved oxygen, diel fluctuation, macroalgal habitat
Citation: Li H, Moon H, Kang EJ, Kim J-M, Kim M, Lee K, Kwak C-W, Kim H, Kim I-N, Park KY, Lee YK, Jin JW, Edwards MS and Kim J-H (2022) The Diel and Seasonal Heterogeneity of Carbonate Chemistry and Dissolved Oxygen in Three Types of Macroalgal Habitats. Front. Mar. Sci. 9:857153. doi: 10.3389/fmars.2022.857153
Received: 18 January 2022; Accepted: 21 March 2022;
Published: 02 May 2022.
Edited by:
Pablo P Leal, Instituto de Fomento Pesquero (IFOP), ChileReviewed by:
Rebecca K. James, Wageningen University & Research, NetherlandsGlen Lee Wheeler, Marine Biological Association of the United Kingdom, United Kingdom
Copyright © 2022 Li, Moon, Kang, Kim, Kim, Lee, Kwak, Kim, Kim, Park, Lee, Jin, Edwards and Kim. This is an open-access article distributed under the terms of the Creative Commons Attribution License (CC BY). The use, distribution or reproduction in other forums is permitted, provided the original author(s) and the copyright owner(s) are credited and that the original publication in this journal is cited, in accordance with accepted academic practice. No use, distribution or reproduction is permitted which does not comply with these terms.
*Correspondence: Ju-Hyoung Kim, juhyoung@kunsan.ac.kr
†These authors have contributed equally to this work and share first authorship