- 1Tumor Microenvironment Laboratory, UMR3347 CNRS/U1021 INSERM, Institut Curie, Orsay, France
- 2Human Neurosciences Department, Neurosurgery Division, Sapienza University, Rome, Italy
Glioblastoma is a highly aggressive and invasive tumor that affects the central nervous system (CNS). With a five-year survival rate of only 6.9% and a median survival time of eight months, it has the lowest survival rate among CNS tumors. Its treatment consists of surgical resection, subsequent fractionated radiotherapy and concomitant and adjuvant chemotherapy with temozolomide. Despite the implementation of clinical interventions, recurrence is a common occurrence, with over 80% of cases arising at the edge of the resection cavity a few months after treatment. The high recurrence rate and location of glioblastoma indicate the need for a better understanding of the peritumor brain zone (PBZ). In this review, we first describe the main radiological, cellular, molecular and biomechanical tissue features of PBZ; and subsequently, we discuss its current clinical management, potential local therapeutic approaches and future prospects.
Introduction
Glioblastoma (GB) is the most common malignant primary tumor of the central nervous system (CNS). It represents 50.1% of malignant brain tumors and 14.2% of all brain tumors, with an approximate incidence rate of 3 per 100,000 people and five-year relative survival rate of 6.9% (1). The current treatment consists of surgery followed by radiotherapy with concurrent and adjuvant temozolomide (2, 3). However, despite this aggressive standard of care, the prognosis for these patients is poor and the median survival time after diagnosis is 8 months (1).
Histological analysis has been the established approach for GB diagnosis, but nowadays, this main approach is supported by novel diagnostic technologies, such as DNA methylome profiling and advances in molecular diagnostics, especially at the single-cell level, which have reshaped CNS tumor classification. According to the new classification, GB is classified as isocitrate dehydrogenase (IDH) wild-type, representing the most aggressive form of diffuse gliomas (4). Genetic and epigenetic profiling has proven its molecular heterogeneity at inter- and intra-tumor levels. First, bulk RNA-seq studies showed inter-tumor heterogeneity defining three main GB subtypes: proneural (PN), classical (CL), and mesenchymal (MES) (5). Later, scRNA-seq emerged to characterize intratumor heterogeneity, and genetic alterations in CDK4, PDGFRA, EGFR, and NF1 can favor four different cellular states that may coexist in the same tumor and recapitulate neural-progenitor-like (NPC-like), oligodendrocyte-progenitor-like (OPC-like), astrocyte-like (AC-like), and mesenchymal-like (MES-like) cellular states (6). The plasticity between these cell states allows tumor cells to adapt to different treatments, thus evading or resisting the current therapeutic approaches (7). Furthermore, intrinsic phenotypic adaptation also exists in the microenvironment of the tumor core and peritumor brain zone (PBZ), which adds even more complexity to our understanding of this dynamic biological disease (8, 9).
However, despite significant progress in understanding the biology of GB, it remains incurable and there have been no significant therapeutic advances over the past two decades (10). Many biological factors have contributed to this relative lack of progress. The current therapeutic approach involves micro-neurosurgical resection, followed by chemoradiotherapy. The objective of surgical removal is gross total resection (GTR) of the tumor, which has a major effect on the overall survival (OS), progression-free survival (PFS), and quality of life (QoL) of patients (11–13). A balance between surgical cytoreduction and the preservation of neurological function is required to achieve the true benefit of neurosurgical resection. Despite significant technical improvements and contrast agents for enhanced fluorescence-guided surgery, macroscopic GTR is achieved in only 50% of cases (14–17). This is mainly due to the involvement of functional areas, which makes it impossible to perform tumor resection with safe margins without the risk of neurological impairment (12). Furthermore, GB is an infiltrative disease that can extend beyond the contrast-enhancing portion (2, 18). The extent of resection (EOR) is evaluated using early postoperative magnetic resonance imaging (MRI) performed within 72 hours after tumor resection (3, 11). Despite complete surgical resection of the contrast-enhancing portion of the tumor, chemoradiation is not sufficient to avoid regrowth of resistant cells that were not removed by surgery. Therefore, in most cases, recurrence occurs at the margin of the resection cavity, where substantial invading tumor cells are located (19, 20). This poorly characterized area, whose microenvironment interacts with the tumor recurrence-initiating cells, is called the PBZ (14, 21–23). There is a need to biologically characterize the tissues surrounding GB tumors (24, 25); however, preclinical and clinical multicenter studies are lacking in this regard.
The PBZ refers to an area of several centimeters around the tumor that contains specific molecular, radiological, and cellular alterations that are not limited to the immediate area surrounding the contrast-enhanced tumor, which promotes GB cell proliferation, invasion, and recurrence (26, 27). The radiological definition of the PBZ corresponds to the brain area surrounding the tumor without contrast enhancement in T1 gadolinium-enhanced MRI (24). A lack of contrast enhancement with gadolinium-based contrast agents during MRI of the PBZ does not necessarily indicate the absence of tumor cells in this area (24). PBZ radiological and macroscopic analyses have revealed that the PBZ resembles normal brain tissue; however, few cellular, molecular or histopathological studies have been performed in this area (14, 28–30). The biological and molecular characteristics of PBZ may be highly relevant as therapeutic targets, accordingly, proper diagnostic approaches for identifying these areas are needed. Therefore, through an examination of the scientific production from various research groups focused on this topic (24, 31), this article provides an in-depth exploration of the cellular spatial heterogeneity within the PBZ of GB and explains the relevance of the tissue mechanics as an influencer of GB biological niches. In addition, we describe the main radiological features of PBZ and discuss the clinical management of it, with novel local therapies, research approaches and treatment perspectives.
Cell spatial heterogeneity in the PBZ
In addition to this vast intertumor heterogeneity, which profoundly affects treatment outcomes and patient prognosis, well-defined intratumor heterogeneity has been reported in the last few years. The histological features exhibit a significant discrepancy between the bulk tumor core and the PBZ surrounding the tumor mass (32) (Figure 1). The GB core is distinguished by high cellular proliferation, inflammation, hypoxia, and pseudopalisading areas, i.e. peculiar necrotic zones surrounded by GB cells (31). While the periphery of the GB is primarily composed of brain parenchymal tissue with isolated tumor-infiltrating cells.
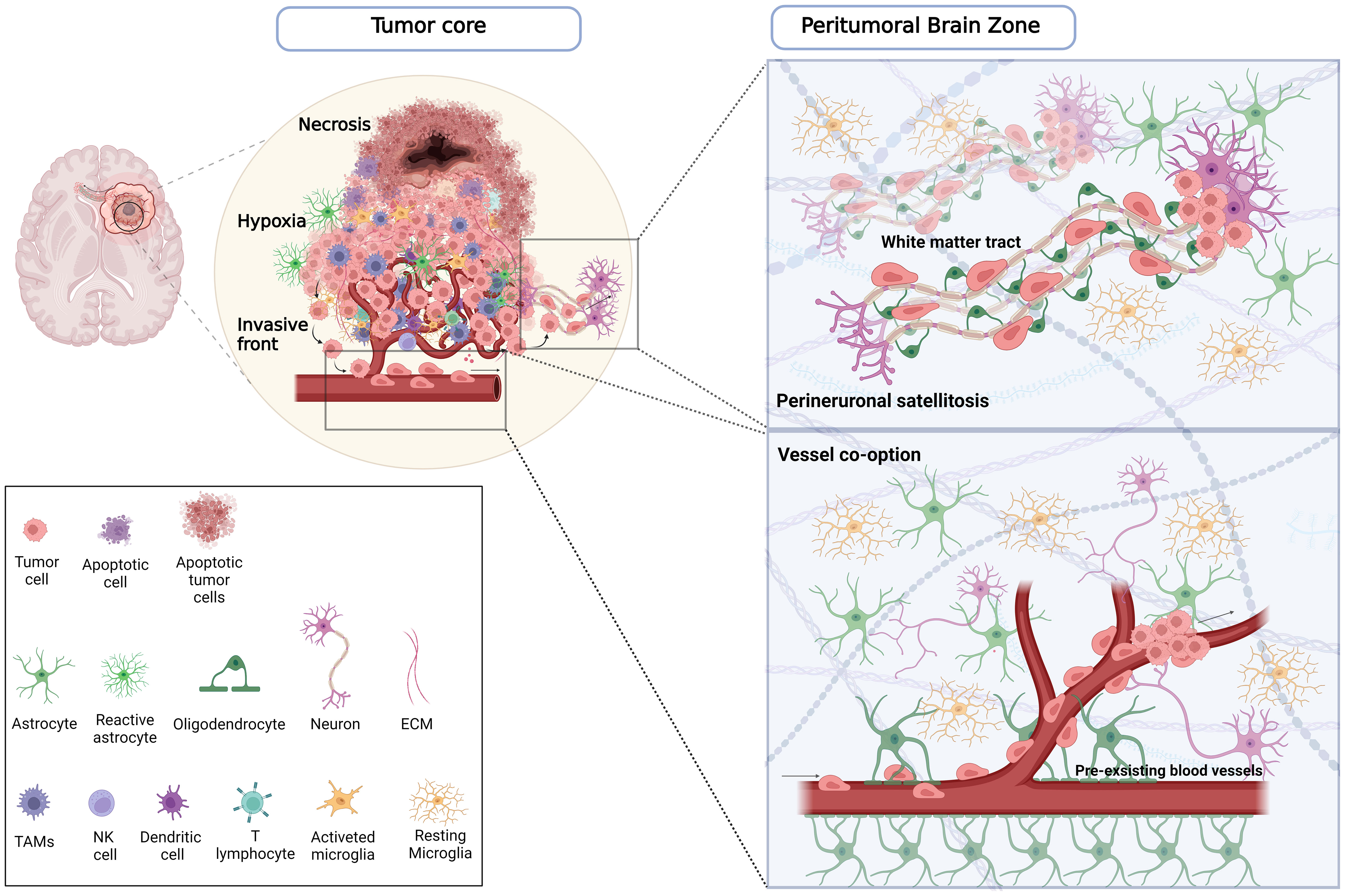
Figure 1 Comprehensive schematic of the distribution of tumoral and non-tumoral cells across the tumor core and PBZ. On the left, the tumor core is characterized in the inner part by a necrotic region in which apoptotic tumor cells and immune cells reside. A hypoxic region encloses the necrotic area surrounded by pseudopalisading cells, which promotes tumor growth, angiogenesis, migration and invasion, and recruits innate immune cells, including macrophages and activated microglia. At the invasive front, endothelial cells, pericytes, activated microglia, reactive astrocytes, and neurons are among the major cell types that make up the GB microenvironment in this region, where glioma cells infiltrate the brain parenchyma in PBZ. On the right, the illustrations of the PBZ, represent the main migration routes by which infiltrating tumor cells invade healthy parenchyma. In the panel below, tumor cells co-opting pre-existing blood vessels outside the tumor core are able to migrate and penetrate the surrounding tissue. In the panel above, the tumor cells, in a process called perineuronal satellitosis, can also take advantage of the nerve fibres and white matter bundles that connect the two hemispheres (corpus callosum), and different brain regions using them as a highway to invade far away from the primary tumor lesion making its surgical resection and treatment almost impossible. Created with www.BioRender.com.
Infiltrating GB cells in PBZ
The presence of GB cells with self-renewal and multi-lineage differentiation properties, called GB stem-like cells (GSCs), has been proposed as the main cause of tumor initiation, growth, and recurrence during GB progression (33). GB mimics developmental-like lineage hierarchies at the cellular level (6, 34). GSCs are at the top of this hierarchy, and are capable of self-renewal and differentiation into non-stem GB cells (33, 35). GSCs with varying stemness have a significant impact on treatment success rates. Different types of GSC niches have been characterized in the tumor core and PBZ, and these distinctions contribute to the progression and therapeutic resistance of GB (36, 37).
According to Neftel et al., GB cells exist in four main cellular states that recapitulate the distinct neural cell types. GB cell lineage progression proceeds towards neural-like fates, such as mesenchymal-like (MES-like), astrocyte-like (AC-like), and neural progenitor-like (NPC-like), or oligodendrocyte progenitor cell (OPC-like) states (6). However, our understanding of lineage evolution in invasive GB cells remains limited. Differences in stemness and molecular characteristics between GB cells isolated from the tumor core and proximal margin regions of patient-derived xenograft models and GB patients have been reported. Anyway, results have been inconsistent and sometimes contradictory because of the heterogeneity in the brain and tumor regions, which highlights the necessity for a more consistent analysis (8, 38–40).
The PBZ is profoundly affected by molecular and metabolic changes of the tumor bulk, making it very different from the normal brain and much closer to the tumor core (41, 42). Therefore, distinguishing GB cells from normal cells in the PBZ is challenging (43), and one of the main problems is the collection of samples from a PBZ area in GB patients compared to a collection of healthy glia or subject to other pathologies (16, 21). Thus, to identify biological markers capable of discriminating and recognizing cancer cells in the PBZ, it is vital to explore the differences between the PBZ and the tumor core.
Different GSC niche localizations across tumor tissues affect their proliferation, self-renewal, molecular subtyping, and radioresistance characteristics (8). The hypercellular hypoxic/peri-necrotic niche is crucial for maintaining the stemness of GSCs while promoting self-renewal, which expands the GSC pool throughout the tumor until it reaches healthy parenchyma and retains its GSC signature (44, 45). Moreover, the peri-necrotic niche in the tumor core is characterized by greater proliferation in contrast to the PBZ, where a more invasive and less proliferating GSCs phenotype is determined (46).
Although a definite distinction in molecular spatial heterogeneity remains unclear, according to Li et al., CD109 (MES marker) and CD133 (PN marker) are mutually exclusive when expressed within a single GSC and their expression appears to indicate a dynamic molecular state (47). The presence of CD133low/CD109high GB cells (linked to MES) was associated with the tumor core, whereas that of CD133high/CD109low GB cells (linked to PN) was associated with PBZ. However, their expression levels can change after the treatment. These results revealed a significant association between the CD133low/CD109high signature and poor prognosis in patients with GB, associated with a substantial transition toward the mesenchymal subtype during recurrence (47). Furthermore, the same group showed that Olig2high GB cells, GSCs typical of PBZ, coexist with CD109high cells, which are typical of tumor core, suggesting that the PBZ and tumor core signature constitute a combination of different GB subtypes. The authors also noted that Olig2 expression was markedly reduced and CD109 expression was higher in MES tumors. When researchers compared the distribution of GB cells from the PBZ and the tumor core in various tumor areas, they discovered that the tumor core was enriched in CD109high cells, whereas the PBZ had a higher concentration of Olig2high GB cells. Additionally, they highlighted a unique marker signature attributable to the tumor core (CD44, MYC, HIF1α, VIM, ANXA1, CDK6, and JAG1) and PBZ (OLIG1, TC2, SRRM2, ERBB3, PHGDH, and RAP1GAP) (8). These gene markers are part of different transcriptional subtypes, implying that the PBZ is a mixture of subtypes. This suggests that beyond the well-known PN-MES axis, there are also spatial implications pointing to a core-to-periphery axis.
The clinical relevance of GSCs that express high levels of CD44 in disease development is not well understood. According to a recent study by Nishikawa et al., higher CD44 expression in the PBZ than in the tumor core was associated with a highly invasive feature, which was correlated with early tumor development and a worse prognosis for survival rates, whereas lower CD44 expression in the PBZ was associated with low invasion and a longer survival rate (48). High CD44 expression is associated with the GB mesenchymal subtype (49), and low survival has been shown to be associated with CD44 expression (50). However, CD44 was preferentially expressed together with CD109 in the tumor core, where a mesenchymal signature in the perinecrotic and pseudopalisading tumor regions was found, compared with the PBZ, where a predominant proneural signature is established (46). Thus, GB cells exhibiting CD44 are not only characterized by a mesenchymal subtype and marked proliferation but are also able to migrate and infiltrate the PBZ, thus causing tumor relapse. Such GB cells behavior may need a phenotypic shift from invasive to proliferative tumor forms and viceversa, in accordance with the ‘Go or Grow’ dichotomy of cancer cells. According to this theory, alterations in the microenvironment, including hypoxia or nutrient depletion, drive a tumor cell to “Go” in search of a more favourable environment and re-establish there, or to “Grow” if the environment provides adequate oxygen and nourishment (51). Hence the possibility, as already proposed by Herrlich et al., that CD44 may exhibit two different functions that encourage the growth or invasion of tumor cells (52). Given this evidence, CD44 could play an essential role in tumor cells adapting to microenvironmental, therapy-induced, oxygen, and nutrient gradient conditions. Hence, a deeper knowledge of its role in GB could guide research towards innovative therapeutic strategies.
The invasive strategies of GB cells in the brain parenchyma
One of the key causes of GB recurrence and poor prognosis is the infiltration of GB cells into the healthy brain (53). Even with the most advanced imaging tools, detecting solitary cells that have migrated into healthy brain parenchyma is extremely challenging. GB cells can spread widely and even enter the contralateral hemisphere, rendering complete surgical excision of the GB unfeasible (54). GB cells not only move away from hypoxic zones within the tumor core but also have the propensity to infiltrate normal tissues. GB cells are known to penetrate the brain parenchyma via important Scherer pathways, including white matter tracts and blood vessel structures (27, 55). In particular, invasive GB cells prefer to move along myelinated fiber tracts, such as the corpus callosum and internal capsule, meninges, ventricular lining, or on basement membranes found in blood vessels and perivascular regions of the subependymal space (56). The morphology of the brain blood vessels has a diameter ranging from 5 to 50 µm, on which GB cell collections might be concentrated (57), whereas the architecture of the white matter tracts corresponds to high aspect ratio fibers (nanosized diameter and significant length fibers) that prompt cells to detect and stimulate migration (58). These extended, uninterrupted pathways are ideal for encouraging effective cell migration in the PBZ (59). As blood vessels in the perivascular region supply oxygen and nutrients, GB cells are drawn into this area. Indeed, several studies have demonstrated the importance of blood vessels in glioma cell invasion routes (60). Most notably, it has been demonstrated that when GB cells are injected into the brain, more than 85% come in contact with blood vessels (61). Moreover, beyond their function in blood supply, endothelial cell chemoattractants can also encourage the migration of GSCs and invasive GB cells to the perivascular space around blood arteries, enabling them to withstand therapeutic hits, including radiation, facilitating their infiltration into the PBZ (62–64). The perivascular niche allows GB cells to infiltrate healthy brain parenchyma via two distinct mechanisms. A well-known phenomenon that affects nearly all types of tumors is called tumor angiogenesis, which is lead by pro-angiogenic substances such as fibroblast growth factor (FGF), vascular endothelial growth factor (VEGF), angiopoietins, and others synthesised in hypoxic tumor cells (65). At the same time, tumor cells can travel towards and then along pre-existing blood vessels through a process known as vessel co-option, which massively occurs in the PBZ. Vessel co-option (66, 67), refers to the process by which GB cells infiltrate around normal brain vessels to form perivascular cuffs, absorbing the preexisting capillaries into the tumor (68).
On the other hand, white matter, which accounts for approximately 60% of the brain, is the other major route of infiltration (60), and it is composed of tracts or bundles of myelinated axons. Often, GBs originate in white matter and use myelinated fibers as scaffolds for cell migration to expand throughout the brain (69). This includes propagation to the other hemisphere, which only occurs along the corpus callosum’s white matter tracts (60). Surprisingly, very little is known about the cellular and molecular mechanisms underlying white matter invasion. Recently, Brooks et al. identified the white matter as a differentiation niche for GBs with oligodendrocyte lineage competency (70). They found that GB cells that come into contact with the white matter take on the fate of oligodendrocytes, which inhibits their proliferation and invasion. This study emphasizes that differentiation is a reaction to white matter damage caused by tumor infiltration, which creates a feedback loop that suppresses tumor growth via SOX10 making it a potential therapeutic target. Hence, even though invasion along the white matter is a typical infiltration route towards and within the PBZ, myelin damage results in tumor growth suppression, slowing the spread of GB (70).
Non tumor cells in the PBZ
The tumor ecosystem in the GB consists of neurons, astrocytes, oligodendrocytes, glioma-associated stromal cells, endothelial cells, pericytes, microglia, tumor-infiltrating immune cells – such as tumor-associated macrophages (TAMs) and tumor-infiltrating lymphocytes (TILs) – as well as extracellular matrix (ECM) components, extracellular vesicles, and soluble secreted ECM remodelling enzymes (71). To maintain their growth, GB cells establish multiple communication routes with brain-resident cells via cellular secretion, gap junctions, and tunnelling nanotubes (25, 72, 73). Recently, some of these mechanisms have been identified and used to develop novel therapeutic strategies (74). However, the intricate cell-cell interactions between the GB and stromal cells in the PBZ remain partly unknown. In addition to maintaining brain homeostasis, neurons and astrocytes play a significant role in tumor development and contribute to their survival in the PBZ. They increase Ca2+ activity of the GB cells followed by a shift towards a more MES-like state as a result of plastic changes to surgery, radiotherapy, and chemotherapy, leading to increased resistance (75). However, the role of astrocytes and, to a lesser extent, oligodendrocytes in the GB context and their interactions with tumor cells remain elusive. Although studies on the neural regulation of GB formation have made significant progress, some issues remain unresolved.
Neuronal cells in PBZ
GB cells can exploit the mechanisms that normally underlie brain development and the plasticity of neural circuits to ensure growth and proliferation. Throughout normal development, electrical activity affects central and peripheral nervous system formation (76). Furthermore, recent studies have revealed that GB cell behavior is influenced by brain activity in a region-specific manner (77). Moreover, glutamate, which causes seizures and cytotoxicity in PBZ networks, is released by GB cells and acts in a paracrine and autocrine manner, promoting the spread of GB cells into the brain parenchyma (78). In addition, the identification of neuronal activity-dependent paracrine signalling of neuroligin-3 (NLGN3) and BDNF led to the first understanding of the mechanisms of activity-regulated paracrine signalling (79, 80). GB cells can also structurally integrate into neural circuits, forming neuron-glioma synapses that facilitate GB invasion within the PBZ of the tumor in a calcium-dependent manner (81). In this study, the authors first examined the direct gap junction-mediated interactions among themselves and with astrocytes to then investigate the tumor network concept in the brain tumor microenvironment. They identified both connected and unconnected GB cell subtypes and showed that the highly connected GB cells, which are present in the tumor core, are more stationary, have a larger density of tumor microtubules (TMs) among cells, and are stable over time. In contrast, the unconnected GB cells present in the PBZ were more invasive and had higher TM turnover. According to Humphries et al., TM turnover is crucial, because it resembles a peculiar searching pattern typical of animals called Levy-like movements (82), which also reflects an optimal search strategy of TM protrusions in the tumor ecosystem to increase their invasiveness (81), reminiscent of the dynamics typical of the migration of immature neurons (83). Moreover, they found that unconnected GB cells in the PBZ were enriched in neuronal and oligodendrocyte precursor-like cell states, and showed neurodevelopmental transcriptional signatures. In contrast, connected GB cells exhibit an MES-like and an injury response transcriptional signature in the tumor core. Additionally, they discovered that higher neural activity in the PBZ resulted in more TM branching and turnover, suggesting that neuronal inputs drive TM dynamics during invasion. Along with these results from gene and pathway analyses, AMPAR gene expression has also been found to be enriched and associated with higher invasiveness at the tumor rim (81).
This novel interplay between neurons and GB cells raises questions about the implications in terms of therapy resistance, as well as the impact that surgery has on tumor recurrence. Moreover, it invites the consideration of how these differently connected regional differences respond differently to surgical and therapeutic interventions.
Lastly, it has been shown that the GB cell network includes cells with rhythmic Ca2+ activity that influences cell growth and proliferation in a MAPK and NF-ĸB pathway-dependent manner and is related to the calcium-activated potassium channel KCa3.1. The presence of the channel is related and restricted only to a small group of GB cells with spontaneous and oscillatory activity that communicate and interconnect via gap-junctions with the rest of the GB cell network, and the direct ablation of these “peacemaker cells”, profoundly compromised the Ca2+ activity, leading to increased cell deaths (84). Interestingly, the presence of these tumor cells is restricted to the tumor core and is largely absent in the PBZ (84), which, as mentioned previously, is characterized by an unconnected GB cell distribution pattern (81). Thus, even the extent of surgical resection in the non-enhancing fluid-attenuated inversion recovery (FLAIR) zone could affect how quickly the tumor recurs, not only based on how many GB cells are removed but also on how this pattern of connections is perturbed. Hence, in conclusion, therapy should be reconsidered in terms of region-based therapy and flanked by systemic therapy.
Astrocytes and reactive astrogliosis
Astrocytes are the most common glial cells in the CNS. They carry out crucial tasks related to growth and homeostasis, including maintaining the blood-brain barrier (BBB), storing and supplying energetic substrates to neurons, assisting in the growth of neural cells and synaptogenesis (85). Additionally, astrocytes can regulate microglial activity, attract inflammatory cells to the CNS, and even inhibit neurodegeneration and CNS inflammation through a variety of mechanisms such as neurotoxicity, metabolic cascades, and modulation of microglial activities (86–88).
As a result, it is not surprising that they also react with the GB cells. GB cells successfully co-opt astrocytes to maintain their proliferation, survival, migration, and therapeutic resistance (89). Cancer patients with high reactive astrocyte gene expression levels have a worse prognosis, and tumor cells co-transplanted with astrocytes in mice result in more aggressive tumors (90).
GB cells communicate with astrocytes via the gap junction protein connexin-43 (CX-43), which increases chemotherapy resistance and GB cell proliferation and migration (91, 92). Astrocytes and GB cells express CX-43 via gap junction proteins. The role of astrocytes in the GB microenvironment is partially explained by CX-43-mediated gap junction coupling between GB cells and astrocytes, which alters their phenotype and hence fosters a more pro-invasion environment for GB cells (93, 94).
A subpopulation of astrocytes specifically localized in perivascular areas, where a population of CD44+ GB cells also resides, was identified by comparing the gene expression patterns of tumor microenvironment-associated astrocytes in low- and high-grade gliomas. Osteopontin, a CD44 ligand that promotes stemness of GB cells, is highly expressed in astrocytes. The correlation among osteopontin, CD44, and poor OS in patients with GB raises the possibility that perivascular-niche astrocytes foster GCSs (95, 96). In addition, astrocytes in the PBZ express glial cell line-derived neurotrophic factor (GDNF) to promote invasive tumor growth (97).
As astrocytes cannot respond to inflammatory stimuli, one of the mechanisms for their activation is their interaction with microglia (98). Tenascin-C, osteopontin, lactadherin, and fibulin-3, which are secreted by tumor cells to promote tumor development and invasion, modify ECM and tumor microenvironment components, which in turn alter the complex microglia-astrocytes-tumor cell interactions (99). Reactive astrocytes build a thick network in the area of tumor invasion in the PBZ as a result of aberrant plasticity of the CNS and consequent alterations in the composition of the microenvironment (100). Reactive astrocytes secrete high levels of growth factors, cytokines, and other chemicals that are then exposed to the GB milieu, triggering various pathways to promote tumor growth. Released by astrocytes near the tumor, TNF-α, TGF-β, IGF-1, and VEGF enhance GB cell proliferation and invasion (101). Astrocytes support tumor growth and nutrient supply (102). Given that, it has been reported that reactive astrocyte depletion regresses GB and increases mice survival (103). In addition, IL-6 produced by astrocytes appears to participate in many processes that drive GB development, including promoting proliferation and invasion, as well as modulating the immune response to tumor progression (104, 105). Therefore, this important cytokine may be taken into account as a target for novel treatments. Therefore, focusing on particular environmental elements may enhance the effectiveness of immune therapies in addition to regular treatment with targeted immunotherapy alone.
Although reactive astrocytes are key players in promoting GB invasion, proliferation and resistance to therapy, they remain under-studied. Hence, the effectiveness of therapeutic approaches for GB may be increased by a deeper understanding of the pathogenic mechanisms that drive interactions between GB cells and reactive astrocytes in the PBZ.
Endothelial cells in PBZ
Endothelial cells (ECs) in the brain are characterized by specific features and properties, given their role as the interface between the CNS and blood stream. ECs in the brain develop constant complexes of tight and adherent junctions between them, creating a tight and size-selective barrier. By controlling vascular permeability ECs are the primary cellular constituents of the blood-brain barrier (BBB) (106). However, the EC phenotypic and functional characteristics in GB differ from the ones in normal tissues. In GB context, ECs form a close connection with tumor cells to change tissue homeostasis towards a tumor-supporting microenvironment (107). A clear difference exists between normal and tumor ECs which present biomarkers such as vWF, CD31, and CD105 (107). Tumor ECs loose CD144 (VE-cadherin), a protein that is crucial for vascular integrity (108). Furthermore, it has been shown that almost all CD31- and CD34-positive ECs co-express VEGFR-1 and -2 within the tumor core of GB. In contrast, the majority of CD31 and CD34-positive ECs in the PBZ lacked VEGFR expression, expressing Nestin but not Factor VIII. In the PBZ, vessels that are CD31, CD34, and nestin-positive but lack VEGFRs and Factor VIII may be signs that tumor vasculature is starting to proliferate. Furthermore, low VEGFR expression in PBZ ECs appears to correlate with the therapeutic failure of VEGFR-targeting drugs, which could explain why this area is frequently the source of recurrence after surgical resection (30). In addition, Xie et al. discovered five distinct EC phenotypes that corresponded to different anatomical regions: the tumor core and PBZ, representing various degrees of ECs activity and BBB disruption. Using scRNA-seq, they discovered a cluster in the PBZ, characterized by elevated expression of genes typical of the BBB, such as KLF2 and SLC2A1. KLF2 is a crucial transcription factor that controls the gene networks that promote ECs quiescence. Furthermore, they discovered ECs with an angiogenic phenotype that expressed a high concentration of genes related to endothelial tip cells, angiogenesis, cytoskeleton rearrangement, and basement membrane remodelling. The authors identified CD93 as a critical regulator of the cytoskeleton and ECM organization in ECs during angiogenesis, collagen (COL4A1 and COL4A2), collagen-modifying enzyme (PXDN), and other basement membrane components (LAMB1 and HSPG2). In addition, numerous BBB transporters, including SLC2A1, ABCG2, ABCB1, SLCO1A2, and ATP10A, were overexpressed in the PBZ ECs compared to the tumor core (109).
Spatial immune heterogeneity within PBZ
GB has been extensively characterized as an immune-cold tumor, and its immunosuppressive microenvironment promotes tumor development and growth by hampering an effective antitumor immune response (110). GB is supported by tumor-associated microglia and peripheral myeloid cells (111). In addition to CNS-resident microglia, which regulate homeostasis and safeguard the CNS against injury and infections (111), infiltrating immune cells in the GB include peripheral macrophages, granulocytes, neutrophils, myeloid-derived suppressor cells (MDSCs), and TILs (112), which infiltrate tumor tissue to different extents across the tumor core and PBZ.
Tumor-associated macrophages and microglia within PBZ
TAMs, which make up to 40% of the tumor mass, are a combination of microglia and infiltrating macrophages, and represent an essential component of the tumor microenvironment. TAMs are crucial for the development, recurrence, and therapeutic response of tumors (113). Their differentiation has historically been based on different CD45 expression levels; while infiltrating macrophages show high levels of CD45 (CD11b+CD45high), microglia express low levels of CD45 (CD11b+CD45low). These include P2RY12, Sall1, Tmem119, and Hexb, which are expressed only by CNS-resident microglia (114–117), whereas CD49d and CXCR4 are preferentially expressed by infiltrating macrophages (118, 119). However, a comprehensive understanding of the spatial dynamics within the tumor core or PBZ and its clinical relevance has been poorly investigated. Data from a meta-analysis of single-cell and bulk RNA-seq of materials from patients with GB revealed that TAMs have a dynamic identity (with M0, M1, and M2 states). TAMs are more pro-inflammatory and have enhanced PD-1 signalling activity in the tumor core, while they are more anti-inflammatory and have higher NF-kB signalling in the PBZ (120). Based on this evidence, it has recently been shown that TAMs with anti-phagocytic actions express PD-1, which can be counteracted by PD-L1 blockage (121). Moreover, anti-PD-1 antibodies can directly activate macrophages, which leads to an increase in cytokine production, a pro-inflammatory phenotype, and improved tumor cell phagocytosis (122). Additionally, NF-κB has been shown to encourage polarization towards M1-like macrophages under normal conditions (123). However, in cancer, NF-κB signalling in tumor-associated macrophages inhibits M1 polarization and promotes M2 anti-inflammatory responses (124). Thus, inhibition of NF-κB signalling in myeloid cells promotes M1-like TAM polarisation in mice models of GB, resulting in increased cytotoxic T cell infiltration and decreased tumor growth (125, 126).
On the other hand, microglia have been reported to be predominant in the PBZ regions (127, 128). Conversely, Darmanis et al. highlighted that TAMs express more pro-inflammatory cytokine genes, such as IL1, in the PBZ than in the tumor core, where anti-inflammatory genes, such as TGFβ, are elevated (129). TGFβ expression by TAMs in the tumor core may be beneficial for tumor growth and dissemination, as TGFβ has been shown to inhibit cell adhesion, promote survival of cells with DNA damage, and act as a potential angiogenic factor. In contrast, the tumor core showed increased levels of IL1RN, a significant anti-inflammatory regulator that negatively interacts with IL1R1 to actively inhibit immune activation. These results have implications for therapeutic immunotherapy strategies against GB. However, these observations imply that the effectiveness of targeted immune therapies may significantly differ across different patients and that tumor phenotyping is essential for understanding the local distribution of immune cells and developing more effective and beneficial treatments. Moreover, according to Friebel et al., there is a subset of CD206+CD169+CD209+ macrophages in the tumor core that has been directly linked to blood vessels (130). Furthermore, Koshkaki et al. suggested that CD163+ TAMs are the most common cell type in both the PBZ and tumor core. Additionally, IDO and PDL-1 were nearly absent in the PBZ but were expressed in the majority of tumor core samples, frequently in conjunction with TIGIT expression. According to the authors, the significant immunosuppression seen in the GB microenvironment is a result of many inhibitory mechanisms that might work simultaneously in the tumor core and PBZ. Nevertheless, compared to the tumor core, PBZ exhibited significantly less immunosuppression (131). Nonetheless, unlike other neurodegenerative and inflammatory diseases, for which the different stages of activation and the different spatial distributions along different brain regions have been extensively described (117), the temporal and spatial dynamics of TAMs in brain tumors, as well as the functional differences among different subpopulations in the tumor core and PBZ are still largely unknown, and their clinical relevance remains poorly defined.
Different T-cell subsets as key players across GB tumor tissue
The development of adaptive immunity against cancer is a self-replicating, cyclical process that begins with the antigens released by cancer cells and ends with the infiltration of activated effector T-cells (also known as TILs) into the tumor bed which recognize and targets tumor cells (132). Increased intratumoral effector cytotoxic and helper T-cell populations are substantially associated with improved survival (133). Nevertheless, as observed for TAMs, very few studies have compared the spatial and temporal differences in the different T cell types in the GB between the tumor core and PBZ. This aspect is crucial when considering T-cell exhaustion, where the tumor core is more prone to induce it and is characterized by decreased granzyme B, TNFα, IFNγ (134), and IL-2 (135) levels, thus impacting immunotherapy (136). Conversely, the PBZ contains a greater number of effector T-cells that are not exhausted (137). Moreover, the tumor core has more Foxp3+CD25+CD127loCD4+ Tregs and myeloid-derived suppressor cells than the PBZ (138), indicating that the milieu in the core of the tumor is more immunosuppressive than the PBZ (137). Furthermore, it has been shown that tumor cores have more exhausted CD8+ T cells than the PBZ, and that hypoxia directly enhances CD8+ T-cell exhaustion (139). It was also discovered that a higher frequency of PD-1+CTLA-4+CD8+ T-cells in the tumor core than in the PBZ was strongly associated with a decreased PFS in patients with GB. CD4+ and NK cells are significantly higher in the tumor core than in the PBZ, whereas the number of dendritic cells is higher in the PBZ than in the core region (139). This study showed that immunosuppression and hypoxia were higher in the tumor core than in the PBZ. Moreover, a Phase I clinical trial assessed efficacy and feasibility of employing neural stem cells, already known to cross the BBB and infiltrate the tumor tissue (140), in order to delivery engineered oncolytic viruses in the PBZ. The treatment demonstrated that an antitumor response was associated with an increase in CD8+ T cells in the tumor (141).
Thus, based on evidence from the literature, it is possible to reconstruct a different spatial distribution between the tumor core and PBZ, which shows a more immunosuppressive and hypoxic environment in the tumor core together with a high number of Tregs, exhausted CD8+ T cells, and TAMs M2, which may suggest a much less susceptible environment for immunotherapy efficacy. Hence, there is an urgent need to better understand the spatial immune microenvironment in GB to develop novel immunotherapies for more efficacious treatments.
Tissue mechanics in PBZ
Physical stress caused by GB plays a fundamental role in progression, immune evasion, and response to treatment, affecting both GB cells and the stroma of the PBZ. As GBs grow, they modify the structure of the brain and affect the function of the surrounding PBZ through several physical forces mechanisms, such as elevated solid stress, elevated interstitial fluid pressure (IFP), modified tissue stiffness and altered tissue microarchitecture (142).
Interstitial fluid pressure in PBZ
IFP represents the isotropic stress exerted by the fluid phase. Stylianopoulos et al. discovered that the growth of tumors leads to an increase in fluid pressure, which is attributed to the increased permeability of blood vessels and the impairment of lymphatic function. This increase in fluid pressure is regulated by the pressure in the microvascular system. However, it is important to note that fluid pressure does not result in compression of tumor blood vessels (143). This presence of leaky blood vessels and a compromised drainage system in GB contributes to a high IFP of approximately 7.5 mmHg (142).
A high IFP hinders the convection of drugs from the vasculature into the tumor core and drives interstitial flow in the PBZ, exposing extravascular cells to shear stress (144). The pronounced IFP gradient in the PBZ propels the movement of interstitial fluid from the tumor core towards the PBZ. This fluid flow can promote tumor invasion and growth by facilitating the transport of released substances and GB cells to the PBZ.
Solid stress in PBZ
The term “solid stress” refers to the pressure experienced by the solid components of tumors and the surrounding brain tissue, that is, the ECM and cells. IFP and solid stress are two separate types of mechanical stress, each with its own origin and impact on the tissue studied (142).
The elevated compressive solid stress within the tumor core leads to the collapse of blood vessels, which consequently results in a reduced growth rate of GB cells compared to those found in the periphery. Solid stress in the periphery of the GB causes blood vessels in the surrounding normal tissue to deform into elliptical shapes (143).
The growth pattern of the GB, which can be nodular or infiltrative, is a determining factor for intratumor and peritumor solid stress. Solid stresses are larger around more nodular GBs than around infiltrative GBs. Nodular GBs deform the surrounding PBZ and neuronal nuclei, thus reducing peritumor vascular perfusion and inducing neural loss (145). In addition, surgical resection modifies tissue mechanics in the PBZ adjacent to the resection cavity, which suddenly reduces solid stress and mechanical pressure, thus favoring GB cell proliferation towards the cavity, which is repopulated by GB cells over time (146).
ECM stiffness in the tumor core and in the PBZ
GB develops within a microenvironment that is mechanically challenged and is characterized by a dense and stiff ECM, which leads to compromised vascular integrity and subsequently induces hypoxia (147).
Early investigations began to map and measure GB rigidity. However, disparities persist among research groups who emploied varying methodologies, such as magnetic resonance elastography (MRE) and atomic force microscopy (AFM), in both preclinical and clinical specimens.
The general consensus seems to be that the GB is stiffer than brain tissue. Additionally, it appeared that the GB stiffness in the tumor core was greater than that in the PBZ. However, it should be noted that the range of stiffness among the different regions of the GB is quite large, which suggests that there may be varying biological and mechanical features across different regions of the GB.
Stiffer environments may alter the GB cell biology. By employing a hydrogel platform with tunable stiffness that is degradable by matrix metalloproteinases for three-dimensional culture, it has been demonstrated that reducing the stiffness of the hydrogel enhances the proliferation of GB cells, whereas increasing the stiffness of the hydrogel enhances drug resistance in GB cells.
GB tissue stiffness may also modulate blood vessel perfusion. A prospective study aimed to map differences in biomechanical and functional properties between GB and healthy brain tissue, measuring the mechanical properties with MRE has been found to be a useful predictor of blood vessel perfusion. Specifically, tissue stiffness has been observed to be inversely correlated with the values obtained for perfusion (148).
In addition to a direct impact on blood perfusion and oxygen supply, mechanical changes and ECM produced by GB can also promote local immune dysfunction, by inhibiting T cell migration into the tumor core and PBZ (149). Furthermore, immune response and immunotherapy treatment effects not only require the generation of cancer-specific T-cells but also that these T-cells physically contact cancer cells (150).
All of the previously cited physical modifications favor the compression and disruption of blood and lymphatic vessels, hindering blood flow, supply of oxygen, proper functioning of immune cells, and impeding correct drug delivery. Therefore, a broader understanding of the relationship between the biology and physics of GB and its PBZ might provide opportunities for the discovery of new therapies.
Insights into tissue mechanics in the PBZ may offer a pivotal avenue for practical interventions to enhance the clinical outcomes in patients with GB. Understanding the role of elevated IFP and compressive solid stress within the tumor core and surrounding PBZ reveals critical factors influencing GB progression, treatment response, and immune evasion. ECM stiffness influences vascular integrity and induces hypoxia, thereby contributing to local immune dysfunction and hindering effective immunotherapy. Therefore, strategies focusing on modulating IFP, alleviating solid stress, and manipulating ECM stiffness present promising avenues for optimizing drug delivery, enhancing treatment response, and addressing immune-related challenges in GB patients, marking a significant step towards translating biomechanical knowledge into clinical advancements.
Importantly, to improve immunotherapy strategies, targeting and reducing IFP would promote better drug penetration and enhance immune cell access to the tumor core and PBZ. In the same way, strategies to alleviate compressive solid stress and modulate the stiff ECM may enhance immune cell mobility and functionality. Combining these tissue mechanics-targeted approaches with immunotherapies holds promise for synergistic benefits. Due to the mechanical heterogeneity of tissues among GB patients, it is important to think that personalized immunotherapeutic interventions adapted to individual biomechanical profiles should be considered.
Clinical imaging
GB growth is characterized by diffuse infiltration of normal brain tissue. However, techniques based on the identification of regions with disrupted BBB cannot accurately detect tumor infiltration beyond the apparent borders of the enhancing region (151, 152). Therefore, new MRI techniques may be useful for evaluating tumor cell infiltration in the PBZ; abnormal signal regions are frequently observed adjacent to or along the surgical cavity in clinical practice (8, 9, 18, 153). The main imaging techniques described in the literature are presented here (Figure 2): different contrast images in magnetic resonance imaging (MRI), such as T1- and T2-weighted imaging, FLAIR, diffusion-weighted imaging (DWI), apparent diffusion coefficient (ADC), diffusion tensor imaging (DTI), restriction spectrum imaging (RSI), and positron emission tomography and computed tomography (PET-CT) scans (Supplementary Table 1).
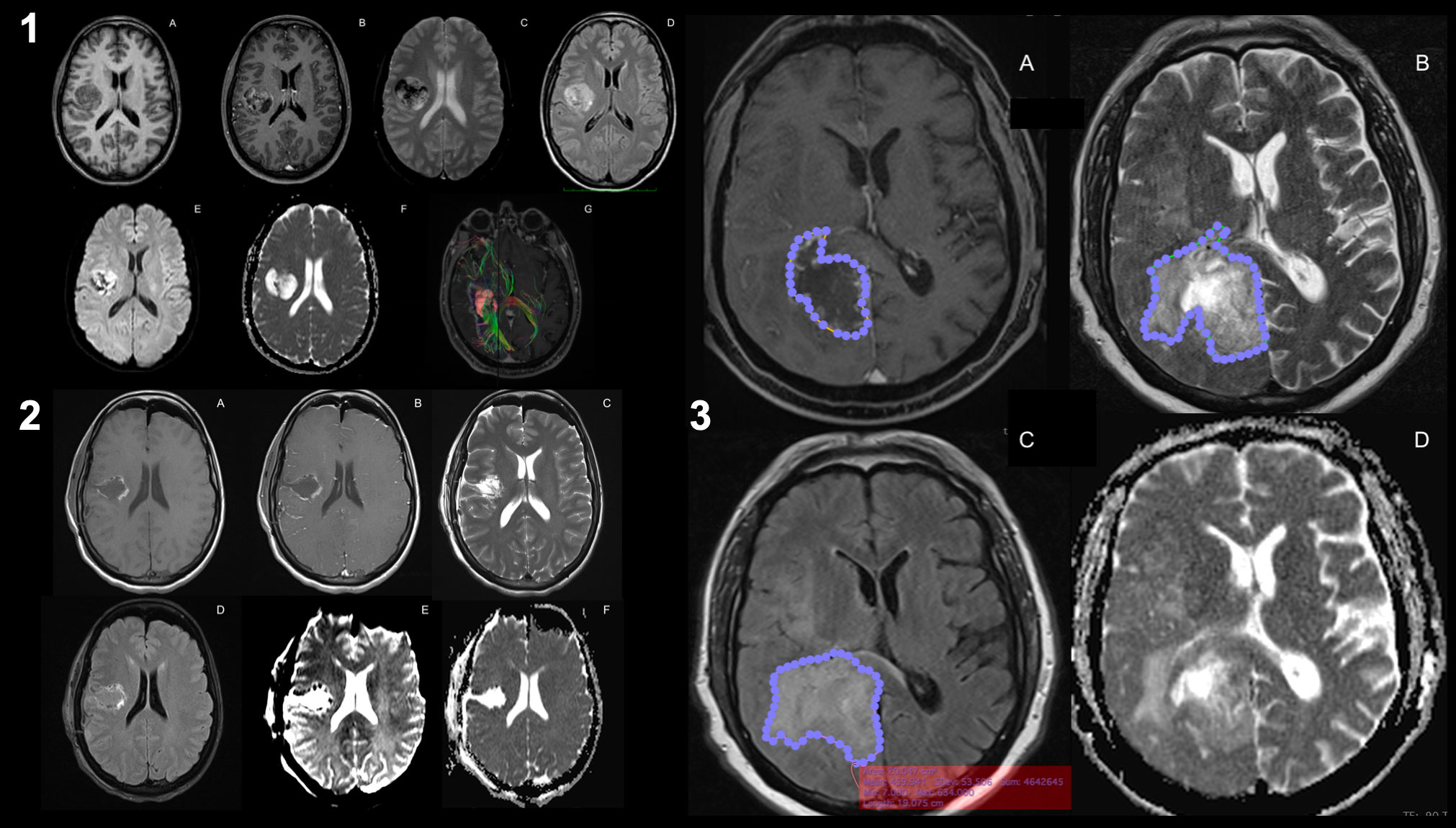
Figure 2 Magnetic resonance imaging of glioblastoma. Preoperative MRI-imaging sequences used for the study of glioblastoma (1): T1-weighted images (1.A), T1-weighted contrast-enhanced images (1.B), T2-weighted images (1.C), FLAIR images (1.D), perfusion imaging (PWI, 1.E), diffusion imaging (DWI, 1.F), and DTI with intra- and peri-lesional fiber-tracking study (1.G). Same sequences for the postoperative study of resection margins after the surgical procedure (2). Areas of the identified altered signals (3): the contrast-enhanced signal margin highlighted by a blue-dotted line (3.A), the signal margin in T2 associated with the presence of peri-lesional edema (3.B), the margin in the same lesion in FLAIR (3.C) and the DWI (3.D). It can be seen that there is a discrepancy between the signal area in FLAIR versus T2 (known as T2-FLAIR mismatch), which may imply that there are areas of PBZ within healthy edematous tissue.
T1-weighted-imaging
Contrast enhancement on standard brain MRI qualitatively reflects the disrupted state of the BBB. The PBZ displays a homogeneous dynamic contrast enhancement (DCE) profile, comparable to that of a healthy brain, but different from that of other GB sampling zones (14). This radiological profile is characterized by low permeability and low extracellular volume fraction that reflect the maintenance of the BBB in the PBZ, which would explain the absence of contrast enhancement (154). Tumor infiltration can occur in the absence of angiogenesis or vascular abnormalization. The tumor cell density threshold to modify the MRI signal is approximately 500 cells/mm3, which limits radiological examination of GB infiltration in the brain parenchyma. This was highlighted by the results of a histopathological examination that found GB cell infiltration in one-third of radiologically normal PBZ areas (14). Similarly, drug access to a viable contrast-enhanced tumor core is likely significantly higher than that of PBZ, which usually does not exhibit contrast enhancement (155).
T2-weighted-imaging
Extensive edema in the PBZ is a characteristic feature of the GB. It is believed that when hyperintensity is found on T2 weighted imaging surrounding a tumor, it hosts infiltrating tumor cells (156). However, with this type of MRI sequence, it is currently impossible to distinguish edema related to the lesion from edema caused by GB cells. Furthermore, distinguishing among gliosis, radiation effects, tumor-containing tissue after recurrence, impaired blood flow due to injury to normal vessels and cytotoxic edema can be difficult (157).
Combination of FLAIR and T2-weighted MRI
The measurement of the residual T2/FLAIR abnormal signal region surrounding the surgical cavity defined as the postoperative peritumor edema zone (Figure 3), coupled with a high ratio of choline/N-acetyl-aspartate (Cho/NAA) ≥ 1.31 is associated with early recurrence and poor prognosis, proving this approach to be a useful prognostic tool to predict GB relapse (18).
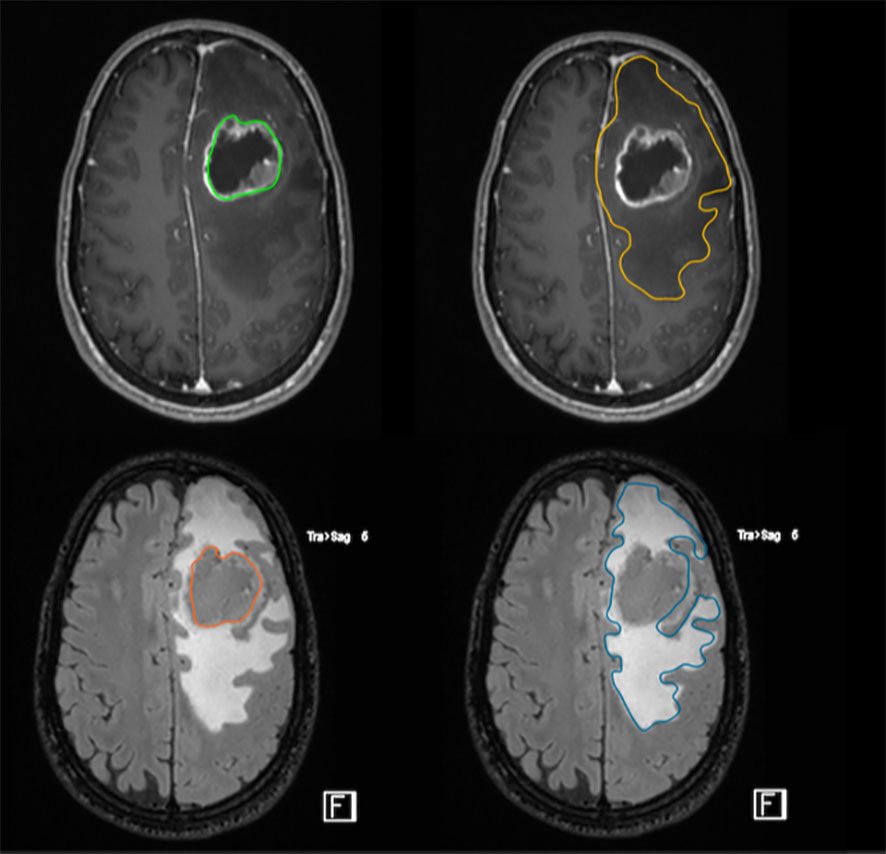
Figure 3 FLAIR-high lesions showing peritumoral brain zone in glioblastoma. The size of the peritumoral edema is one index to predict the degree of tumor infiltration through imaging. The surgical resection of FLAIR-high lesions is important to improve postoperative outcome.
On T2‐weighted (T2W) FLAIR, peritumoral hyperintensity usually fades toward the normal-appearing brain tissue, making it very difficult to identify a clear‐cut border. Some studies on MRI‐guided biopsies report features of diffusively infiltrating gliomas intermixed with apparently normal brain tissue on MRI. Thus, most imaging studies define the PBZ as the whole area of T2W hyperintensity surrounding the contrast-enhanced T1W brain tissue identified as the border of the tumor. A wide peritumoral T2W FLAIR hyperintensity is associated with elevated microvessel density and MIB‐1 index, whereas a narrow peritumoral FLAIR hyperintensity correlates with both low microvessel density and low MIB‐1 index. FLAIR signal could correspond to high CD163+ cells expression at bioptic analysis. CD163+ GB cell are involved in invasiveness, tumor cell migration, angiogenesis, immune evasion, edema formation, and a worse survival rate (158).
Moreover, the MIB-1 index (used as a complementary method to differentiate better and worse prognostic groups of astrocytic tumors) of the PBZ correlates with the degree of the FLAIR-high area. Low MIB-1 index in the PBZ correlated with narrow FLAIR-high areas, and high MIB-1 index in the PBZ correlated with broad FLAIR-high areas. In addition, the microvessel density (MVD) ratio of the PBZ to the tumor core also correlates with the degree of FLAIR-high areas; however, the association between postoperative edema and the prognosis of patients with GB is not well understood (159).
Importantly, patients with complete resection (>95%) who had greater than 53% of FLAIR abnormality resected in addition to the contrast-enhancing tumor showed improved survival compared with those who had less than 53% of FLAIR abnormalities resected (160, 161).
Diffusion-weighted-imaging (DWI)
The apparent diffusion coefficient (ADC) derived from DWI, which represents the measurement of the magnitude of diffusion (of water molecules) within tissues, is inversely related to tissue cellularity and has been proposed as a noninvasive imaging biomarker for the detection of intratumor high-density areas. Postoperative edema associated with inflammation, infiltration, and necrosis increases extracellular diffusion. This increased diffusion may be a combined consequence of surgical injury, tumor cell invasion, or demyelination; however, these cannot be differentiated using routine MRI (18).
Novel MRI techniques
Several studies have shown an association between preoperative GB MRI features and clinical prognosis, but few studies have focused on the imaging configuration of the PBZ. Moreover, radiological identification of the PBZ remains problematic. Indeed, the tumor boundary, which includes the necrotic area, can be easily identified by delineating the contrast enhancement area visible on post‐gadolinium T1‐weighted (T1W) images. Conversely, the no‐enhancing regions, beyond these limits, may show radiological features that are not specific to tumor tissue and may be indistinguishable from vasogenic edema and other non‐tumor tissue alterations. Multidirectional diffusion‐weighted imaging can be used to compute tensor‐based quantitative maps of water diffusion, such as apparent diffusion coefficient (ADC), fractional anisotropy, mean diffusivity, radial diffusivity, and axial diffusivity, all of which help distinguish vasogenic edema from tumor infiltration and high‐cellularity regions.
Novel diffusion-weighted MRI techniques, such as DTI and RSI (151) sequences, separate the relative contributions of hindered and restricted signals originating from the extracellular and intracellular water compartments. These techniques were used to assess PBZ infiltration and showed that vasogenic edema and tumor-infiltrated edema are characterized by distinct imaging patterns (152). They can be used to explore regional cerebral blood volume (CBV), cerebral blood flow, and vascular permeability indices, all of which are expected to be relatively higher in tumor areas associated with neoangiogenesis than in normal brain tissues. More recently, a relatively new functional MRI technique has been developed that allows the identification of brain areas associated with glycolytic metabolism leading to lactate production and pH reduction, which can be identified using MRI signal modifications induced by chemical exchange saturation transfer (CEST) resulting in acidity maps. Considering the highly hypoxic metabolism of GB, these MRI sequences may differentiate between IDH wild‐type and IDH‐mutant gliomas, which exhibit different pH distribution gradients (162). Therefore, these technologies can be used to differentiate peritumor edema from what could instead be the nest of a PBZ that is capable of generating recurrence (163). 1H magnetic resonance spectroscopy (1H-MRS) is widely used to non-invasively detect biochemical indices and metabolic changes in intracranial lesions, which can reinforce diagnostic confidence in distinguishing neoplastic and non-neoplastic intracranial lesions and distinguishing tumor recurrence from radiation necrosis (164).
Further improvements in the MRI diagnosis of the GB and its periphery are possible owing to active targeting. Specific targeting groups conjugated with contrast agents can improve recognition and accumulation within a particular tissue. For example, enhanced GB visualization has been observed for paramagnetic liposomes coupled with monoclonal antibodies (mAbs) against endoglin (CD105), a key protein involved in tumor angiogenesis. Under this aspect, radiomics could offer in coming future new tools for defining and identifying PBZ by capturing subtle quantitative measurements on MRI by computing local macro-and microscale morphological changes in texture patterns within the lesion. Peritumor radiomic features have already been found to be predictive of survival in GB across T2w and FLAIR sequences when compared with tumor enhancement and tumor necrosis features (165). The need for improved imaging is given by the importance of establishing proper preoperative planning to ensure total and supramarginal GB resection because it is known that tumor cells that have not yet developed a characteristic radiologic phenotype are present at the margins of the lesion and from the nests of cells (the PBZ) in more than 70% of recurrences.
Apart from radiomics studies, all other imaging techniques have entered the routine MRI protocols of primary brain tumors and are rapidly generating a huge amount of high‐definition structural and functional MRI multiparametric data, which can be correlated with histopathological, genetic, and molecular data. These types of analyses require high computing power and new imaging analysis methods to improve identification of the PBZ (166).
Treating residual GB within the PBZ
Most GB recurrences are located near the resection cavity (167), to treat or prevent them, several local therapies have been developed and tested in preclinical and clinical trials. These therapeutic approaches aim to treat residual and infiltrating GB cells in the postoperative PBZ (Figure 4). These therapies also aim to avoid drug delivery problems in the tumor niche due to the BBB, vessel dysfunction, local fluid pressure, hematomas or hard-to-reach poorly perfused hypoxic areas. At the same time, these local approaches represent an opportunity, since aggressive treatments can be administered locally without significant risks of systemic toxicity.
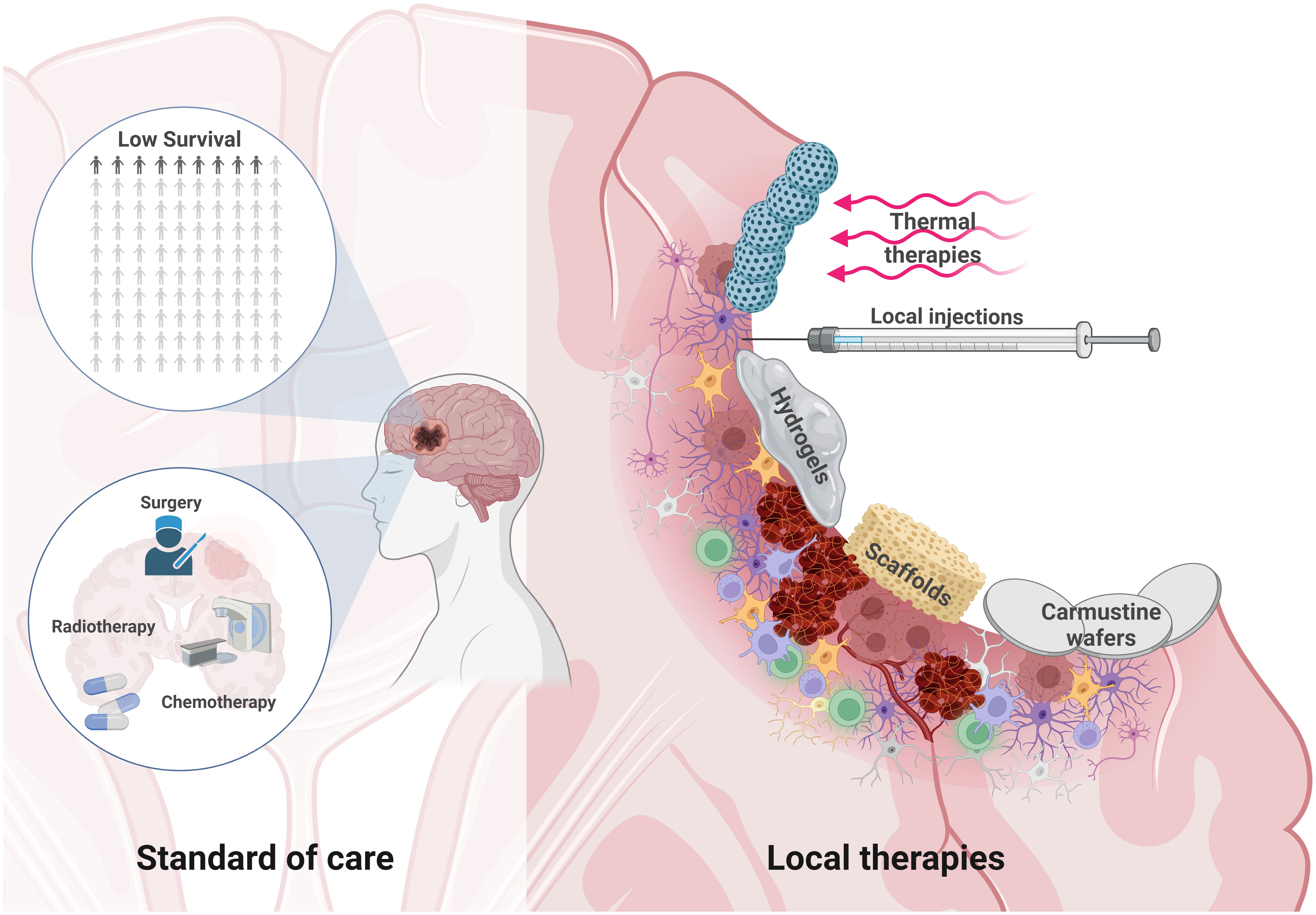
Figure 4 Local therapies to treat residual GB within the PBZ. The survival of glioblastoma patients is low despite the standard of care therapeutic approach based on microsurgical tumor resection, radiotherapy and chemotherapy (Left). Microsurgical resection opens a window of possibilities for local therapies. Brain injury can develop in the form of hematoma formation due to microbleeds, edema, neuroinflammation, and even hemorrhage. The PBZ is characterized by a heterogeneous and dynamic microenvironment where the interaction between the resident immune, vascular and cellular components takes place. Some local therapeutic approaches in this resected area are being studied, such as the use thermal therapies and nanoparticles that are stimulated by external magnetic fields, local injections, hydrogels, carmustine wafers or various scaffolds to deliver drugs locally, among other therapeutic approaches (Right). Created with www.BioRender.com.
Achieving precise drug delivery to the PBZ confronts multifaceted challenges in the complex tumor microenvironment. The heterogeneity of GB, marked by diverse cell types and genetic variations, demands nuanced strategies to ensure comprehensive coverage within the PBZ. BBB requires an inventive approach to facilitate optimal drug penetration. Tumor vasculature irregularities and perfusion variations introduce complexities in uniform drug distribution, whereas the infiltrative nature of GB mandates addressing the surrounding infiltrated regions. The immunosuppressive microenvironment of GB adds an additional layer of complexity, requiring interventions to overcome immune evasion for enhanced treatment efficacy. Challenges persist in accurately monitoring drug distribution in real time, limiting our ability to precisely track therapeutic agents within the PBZ. Furthermore, the spatial and temporal heterogeneity of the tumor microenvironment underscores the need for adaptable strategies to accommodate dynamic changes.
Several approaches have been explored for local delivery of drugs using hydrogels (168–170) and other drug-impregnated biodegradable polymers (171–173). Biodegradable carmustine wafers have been used to administer high doses of chemotherapy to the resection cavity (174, 175). Carmustine functions as an alkylating agent by forming interstrand crosslinks in the DNA, which prevents DNA replication and transcription. Its use in trials with patients has shown improvements in overall survival when used with the Stupp protocol (176); however, its use has been associated with adverse events, such as seizures, weakness on one side of the body, nausea, vomiting, and fever.
Implantable Ommaya reservoirs connected to the resection cavity and/or brain ventricles with a catheter have been used to deliver high doses of chemotherapy over time (177, 178). These reservoirs are implanted under the skin and can be accessed through brief interventions when the doctor considers them appropriate, allowing personalized adaptation of the patient’s treatment. However, to date, patient improvement in clinical trials has been minimal.
Direct intraoperative injections after surgical resection (179, 180) and stereotactic injections with the aid of neuronavigation coupled with computed tomography (CT) or MRI have been studied (181, 182); however, poor diffusion of compounds into the brain parenchyma has been described. Convection-enhanced delivery (CED) method has been explored to improve drug delivery (183, 184). This establishes a positive pressure gradient that improves the spatial distribution of treatment and can be left in place for a prolonged period of time. Nevertheless, the use of this method is challenging; precise cannula placement is crucial but difficult, and learning how to use it appropriately by surgeons requires a steep learning curve (185). In addition, the distribution of the infused treatment varies because the GB and PBZ are heterogeneous among patients, with different foci, degrees of necrosis, and variation in vascular anatomy and metabolism. Furthermore, it varies by area even within the same patient. Future optimization of CED may revolve around refining the cannula design, personalizing treatment plans, and adopting monitoring techniques with advanced imaging to track the therapeutic agent distribution, thereby minimizing variability and ensuring consistent outcomes.
Thermal therapies, such as laser interstitial thermal therapy (LITT) (155, 186–188), focused ultrasound (FUS) (189, 190), and magnetic hyperthermia (191, 192) have also been developed to treat residual GB cells in the surgical cavity because GB cells are sensitive to temperature, leading to apoptosis and/or necrosis.
LITT involves inserting an optical fiber into the tumor and heating it with laser light. The extent of tissue ablation during LITT is a critical factor that influences its efficacy. Aggressive treatment may lead to higher rates of adverse effects, including permanent neurological symptoms, cerebral edema, seizures, and hydrocephalus (193).
Under MRI guidance, transcranial FUS has emerged as a tool for transiently disrupting the BBB and improving drug delivery and penetration. However, its application in GB is limited, and ongoing trials are exploring its potential. The main limitations are attenuation owing to skull thickness and ablation of healthy tissue in the path of ultrasound waves (194).
Magnetic hyperthermia involves the generation of heat by magnetic nanoparticles. In addition to its direct effects, hyperthermia can sensitize GB cells to radiotherapy and chemotherapy (185, 195). Although slight improvements have been achieved in clinical trials using these thermal treatments, undesired side effects related to worsening of neurological symptoms in patients, with edema and seizures, have also been reported. Hence, further investigations are required, emphasizing the need for well-designed trials.
Photodynamic therapy (PDT) is a local therapeutic approach that uses photosensitizing agents in the resection cavity. After activation by light of a certain wavelength, they generate reactive oxygen species that cause extensive DNA damage, leading to cell death (196, 197). One of the main drawbacks of this therapy is that light must reach the sensitizing agent. However, with the wavelengths currently used, tissue penetration is minimal, typically a few millimeters, although some studies have attempted to improve penetration into brain tissues (198). Alternative light sources with deeper penetration capabilities and innovative delivery systems should be explored.
Recently, local viral gene therapies for GB have attracted significant interest. Notably, some patients exhibit impressive responses to these treatments. One extensively researched virus is the replication-incompetent adenoviral vector AdvHSV-tk, delivering the herpes simplex virus type 1 (HSV-1) thymidine kinase gene (199), that by administering an antiviral (like ganciclovir) leads to thymidine kinase phosphorylating the prodrug, causing it to bind to DNA during double-strand break repair, disrupting mitosis and DNA repair mechanisms, inducing cell apoptosis and improving sensitivity to chemoradiation. Recently, in a Phase III clinical trial, AdvHSV-tk under the control of the promoter of the early growth response gene 1 (EGR-1) was employed to intraoperative transduce GB cells by local administration in the PBZ area. Following surgical resection, patients were intravenously treated with ganciclovir to target the sensitive HSV-tk expressing GB cells (200). Furthermore, gene therapy-based immunotherapeutic strategies that harness the host immune system’s ability to specifically target and eliminate glioma cells, while also developing immunological memory have demonstrated significant progress. Two adenoviral vectors expressing Ad-HSV1-TK/GCV and Ad-Flt3L have shown promising preclinical data (201), which has led to FDA phase I human trial that have shown safety and feasibility in high-grade glioma patients (202).
Many of these local approaches have shown great potential for improving PFS and/or OS in several phase I and II trials; however, adverse effects have also been described, not yet allowing the translation of these promising results and the addition of these therapeutic tools to the standard treatment. However, promising results have been obtained, offering potential avenues to enhance patient outcomes. Innovative techniques, such as LITT, magnetic hyperthermia, FUS, PDT, and CED have demonstrated encouraging prospects. However, translating these promises into standard clinical practice requires addressing the key challenges. These include the need for conclusive clinical trials to validate the safety and efficacy of emerging modalities, refine precision in cannula placement and infusate distribution, overcome hurdles in implantable technologies, and establish standardized or individualized protocols. As we navigate through these challenges, the evolving landscape of local therapies for residual GB shows exciting potential, emphasizing the importance of continued research and strategic integration into routine clinical practice.
Future perspectives and impact of PBZ research
The molecular and cellular heterogeneity of PBZ in GB presents a formidable challenge in the field of neuro-oncology. This heterogeneity extends beyond genetic variations to encompass diverse cellular phenotypes and distinct spatial microenvironments within tumors. The tumor microenvironment, intricately woven with the ECM, immune cells, and blood vessels, plays a crucial role in GB progression and therapeutic response. Tissue mechanics within the PBZ further contribute to this complexity, with solid stress, IFP, and ECM stiffness influencing tumor behavior. Understanding these mechanical aspects will open new avenues for novel therapeutic intervention. Cutting-edge imaging techniques have emerged as powerful tools to dissect this heterogeneity and explore the intricacies of the PBZ. However, there remains a need for more precise imaging methodologies that can capture dynamic changes within the PBZ and guide localized therapeutic strategies. Local therapeutic options (hydrogels, carmustine wafers, implantable reservoirs, CED, LITT, FUS, etc.) have been developed to address spatial and temporal heterogeneity within the infiltrative areas of GB, especially around the surgical resection cavity. As neuro-oncology navigates the intricate landscape of GB and its PBZ heterogeneity, the convergence of insights from tissue mechanics, advancements in imaging technologies, and the development of localized therapeutic modalities collectively define the trajectory toward precision medicine for individuals afflicted by this pathology.
Author contributions
AB: Writing – original draft, Writing – review & editing. DA: Writing – original draft, Writing – review & editing. VR: Writing – original draft, Writing – review & editing. GS: Writing – original draft, Writing – review & editing.
Funding
The author(s) declare that financial support was received for the research, authorship, and/or publication of this article. This work was supported by the European Research Council (ERC; Vessel-coopt, Grant Agreement No. 805225), by the Marie Sklodowska-Curie Postdoctoral Fellowship (GlioSurg, Grant Agreement ID: 101061921, DOI: 10.3030/101061921) under the European Union’s Horizon 2020, Institut National du Cancer (INCa; INCa-2020-1-PLBIO-01-ICR-1 and INCa-2021-1-PAIR-CEREB-01-1), and the INSERM-CNRS ATIP-Avenir grant.
Conflict of interest
The authors declare that the research was conducted in the absence of any commercial or financial relationships that could be construed as a potential conflict of interest.
The author(s) declared that they were an editorial board member of Frontiers, at the time of submission. This had no impact on the peer review process and the final decision.
Publisher’s note
All claims expressed in this article are solely those of the authors and do not necessarily represent those of their affiliated organizations, or those of the publisher, the editors and the reviewers. Any product that may be evaluated in this article, or claim that may be made by its manufacturer, is not guaranteed or endorsed by the publisher.
Supplementary material
The Supplementary Material for this article can be found online at: https://www.frontiersin.org/articles/10.3389/fimmu.2024.1347877/full#supplementary-material
Supplementary Table 1 | Primary outcomes revealed by different imaging techniques and limitations in the identification of the peritumoral brain zone (PBZ).
References
1. Ostrom QT, Price M, Neff C, Cioffi G, Waite KA, Kruchko C, et al. CBTRUS statistical report: primary brain and other central nervous system tumors diagnosed in the United States in 2015-2019. Neuro Oncol. (2022) 24:v1–v95. doi: 10.1093/neuonc/noac202
2. Stupp R, Hegi ME, Mason WP, van den Bent MJ, Taphoorn MJ, Janzer RC, et al. Effects of radiotherapy with concomitant and adjuvant temozolomide versus radiotherapy alone on survival in glioblastoma in a randomised phase III study: 5-year analysis of the EORTC-NCIC trial. Lancet Oncol. (2009) 10:459–66. doi: 10.1016/S1470-2045(09)70025-7
3. Stupp R, Mason WP, van den Bent MJ, Weller M, Fisher B, Taphoorn MJ, et al. Radiotherapy plus concomitant and adjuvant temozolomide for glioblastoma. N Engl J Med. (2005) 352:987–96. doi: 10.1056/NEJMoa043330
4. Louis DN, Perry A, Wesseling P, Brat DJ, Cree IA, Figarella-Branger D, et al. The 2021 WHO classification of tumors of the central nervous system: a summary. Neuro Oncol. (2021) 23:1231–51. doi: 10.1093/neuonc/noab106
5. Verhaak RG, Hoadley KA, Purdom E, Wang V, Qi Y, Wilkerson MD, et al. Integrated genomic analysis identifies clinically relevant subtypes of glioblastoma characterized by abnormalities in PDGFRA, IDH1, EGFR, and NF1. Cancer Cell. (2010) 17:98–110. doi: 10.1016/j.ccr.2009.12.020
6. Neftel C, Laffy J, Filbin MG, Hara T, Shore ME, Rahme GJ, et al. An integrative model of cellular states, plasticity, and genetics for glioblastoma. Cell. (2019) 178:835–49 e21. doi: 10.1016/j.cell.2019.06.024
7. Yabo YA, Niclou SP, Golebiewska A. Cancer cell heterogeneity and plasticity: A paradigm shift in glioblastoma. Neuro Oncol. (2021) 24(5):669–82. doi: 10.1093/neuonc/noab269
8. Bastola S, Pavlyukov MS, Yamashita D, Ghosh S, Cho H, Kagaya N, et al. Glioma-initiating cells at tumor edge gain signals from tumor core cells to promote their Malignancy. Nat Commun. (2020) 11:4660. doi: 10.1038/s41467-020-18189-y
9. Jin X, Kim LJY, Wu Q, Wallace LC, Prager BC, Sanvoranart T, et al. Targeting glioma stem cells through combined BMI1 and EZH2 inhibition. Nat Med. (2017) 23:1352–61. doi: 10.1038/nm.4415
10. Bagley SJ, Kothari S, Rahman R, Lee EQ, Dunn GP, Galanis E, et al. Glioblastoma clinical trials: current landscape and opportunities for improvement. Clin Cancer Res. (2022) 28:594–602. doi: 10.1158/1078-0432.CCR-21-2750
11. Chaichana KL, Jusue-Torres I, Navarro-Ramirez R, Raza SM, Pascual-Gallego M, Ibrahim A, et al. Establishing percent resection and residual volume thresholds affecting survival and recurrence for patients with newly diagnosed intracranial glioblastoma. Neuro Oncol. (2014) 16:113–22. doi: 10.1093/neuonc/not137
12. Orringer D, Lau D, Khatri S, Zamora-Berridi GJ, Zhang K, Wu C, et al. Extent of resection in patients with glioblastoma: limiting factors, perception of resectability, and effect on survival. J Neurosurg. (2012) 117:851–9. doi: 10.3171/2012.8.JNS12234
13. Lacroix M, Abi-Said D, Fourney DR, Gokaslan ZL, Shi W, DeMonte F, et al. A multivariate analysis of 416 patients with glioblastoma multiforme: prognosis, extent of resection, and survival. J Neurosurg. (2001) 95:190–8. doi: 10.3171/jns.2001.95.2.0190
14. Lemee JM, Clavreul A, Aubry M, Com E, de Tayrac M, Eliat PA, et al. Characterizing the peritumoral brain zone in glioblastoma: a multidisciplinary analysis. J Neurooncol. (2015) 122:53–61. doi: 10.1007/s11060-014-1695-8
15. Stummer W, Reulen HJ, Meinel T, Pichlmeier U, Schumacher W, Tonn JC, et al. Extent of resection and survival in glioblastoma multiforme: identification of and adjustment for bias. Neurosurgery. (2008) 62:564–76. doi: 10.1227/01.neu.0000317304.31579.17
16. McGirt MJ, Chaichana KL, Gathinji M, Attenello FJ, Than K, Olivi A, et al. Independent association of extent of resection with survival in patients with Malignant brain astrocytoma. J Neurosurg. (2009) 110:156–62. doi: 10.3171/2008.4.17536
17. Brown TJ, Brennan MC, Li M, Church EW, Brandmeir NJ, Rakszawski KL, et al. Association of the extent of resection with survival in glioblastoma: A systematic review and meta-analysis. JAMA Oncol. (2016) 2:1460–9. doi: 10.1001/jamaoncol.2016.1373
18. Cui Y, Zeng W, Jiang H, Ren X, Lin S, Fan Y, et al. Higher cho/NAA ratio in postoperative peritumoral edema zone is associated with earlier recurrence of glioblastoma. Front Neurol. (2020) 11:592155. doi: 10.3389/fneur.2020.592155
19. Kruthika BS, Jain R, Arivazhagan A, Bharath RD, Yasha TC, Kondaiah P, et al. Transcriptome profiling reveals PDZ binding kinase as a novel biomarker in peritumoral brain zone of glioblastoma. J Neurooncol. (2019) 141:315–25. doi: 10.1007/s11060-018-03051-5
20. Luo X, Xu S, Zhong Y, Tu T, Xu Y, Li X, et al. High gene expression levels of VEGFA and CXCL8 in the peritumoral brain zone are associated with the recurrence of glioblastoma: A bioinformatics analysis. Oncol Lett. (2019) 18:6171–9. doi: 10.3892/ol
21. Sherriff J, Tamangani J, Senthil L, Cruickshank G, Spooner D, Jones B, et al. Patterns of relapse in glioblastoma multiforme following concomitant chemoradiotherapy with temozolomide. Br J Radiol. (2013) 86:20120414. doi: 10.1259/bjr.20120414
22. Petrecca K, Guiot MC, Panet-Raymond V, Souhami L. Failure pattern following complete resection plus radiotherapy and temozolomide is at the resection margin in patients with glioblastoma. J Neurooncol. (2013) 111:19–23. doi: 10.1007/s11060-012-0983-4
23. Dimou J, Beland B, Kelly J. Supramaximal resection: A systematic review of its safety, efficacy and feasibility in glioblastoma. J Clin Neurosci. (2020) 72:328–34. doi: 10.1016/j.jocn.2019.12.021
24. Lemee JM, Clavreul A, Menei P. Intratumoral heterogeneity in glioblastoma: don’t forget the peritumoral brain zone. Neuro Oncol. (2015) 17:1322–32. doi: 10.1093/neuonc/nov119
25. D’Alessio A, Proietti G, Sica G, Scicchitano BM. Pathological and molecular features of glioblastoma and its peritumoral tissue. Cancers (Basel). (2019) 11(4):469. doi: 10.3390/cancers11040469
26. Nagashima G, Suzuki R, Hokaku H, Takahashi M, Miyo T, Asai J, et al. Graphic analysis of microscopic tumor cell infiltration, proliferative potential, and vascular endothelial growth factor expression in an autopsy brain with glioblastoma. Surg Neurol. (1999) 51:292–9. doi: 10.1016/S0090-3019(98)00056-1
27. Scherer HJ. THE FORMS OF GROWTH IN GLIOMAS AND THEIR PRACTICAL SIGNIFICANCE. Brain. (1940) 63:1–35. doi: 10.1093/brain/63.1.1
28. Nimbalkar VP, Kruthika BS, Sravya P, Rao S, Sugur HS, Verma BK, et al. Differential gene expression in peritumoral brain zone of glioblastoma: role of SERPINA3 in promoting invasion, stemness and radioresistance of glioma cells and association with poor patient prognosis and recurrence. J Neurooncol. (2021) 152:55–65. doi: 10.1007/s11060-020-03685-4
29. Giambra M, Messuti E, Di Cristofori A, Cavandoli C, Bruno R, Buonanno R, et al. Characterizing the genomic profile in high-grade gliomas: from tumor core to peritumoral brain zone, passing through glioma-derived tumorspheres. Biol (Basel). (2021) 10(11):1157. doi: 10.3390/biology10111157
30. Tamura R, Ohara K, Sasaki H, Morimoto Y, Yoshida K, Toda M. Histopathological vascular investigation of the peritumoral brain zone of glioblastomas. J Neurooncol. (2018) 136:233–41. doi: 10.1007/s11060-017-2648-9
31. Giambra M, Di Cristofori A, Valtorta S, Manfrellotti R, Bigiogera V, Basso G, et al. The peritumoral brain zone in glioblastoma: where we are and where we are going. J Neurosci Res. (2023) 101:199–216. doi: 10.1002/jnr.25134
32. Vollmann-Zwerenz A, Leidgens V, Feliciello G, Klein CA, Hau P. Tumor cell invasion in glioblastoma. Int J Mol Sci. (2020) 21(6):1932. doi: 10.3390/ijms21061932
33. Lathia JD, Mack SC, Mulkearns-Hubert EE, Valentim CL, Rich JN. Cancer stem cells in glioblastoma. Genes Dev. (2015) 29:1203–17. doi: 10.1101/gad.261982.115
34. Couturier CP, Ayyadhury S, Le PU, Nadaf J, Monlong J, Riva G, et al. Single-cell RNA-seq reveals that glioblastoma recapitulates a normal neurodevelopmental hierarchy. Nat Commun. (2020) 11:3406. doi: 10.1038/s41467-020-17186-5
35. Lan X, Jorg DJ, Cavalli FMG, Richards LM, Nguyen LV, Vanner RJ, et al. Fate mapping of human glioblastoma reveals an invariant stem cell hierarchy. Nature. (2017) 549:227–32. doi: 10.1038/nature23666
36. Garnier D, Renoult O, Alves-Guerra MC, Paris F, Pecqueur C. Glioblastoma stem-. Front Oncol. (2019) 9:118. doi: 10.3389/fonc.2019.00118
37. Ou A, Ott M, Fang D, Heimberger AB. The role and therapeutic targeting of JAK/STAT signaling in glioblastoma. Cancers (Basel). (2021) 13(3):437. doi: 10.3390/cancers13030437
38. Glas M, Rath BH, Simon M, Reinartz R, Schramme A, Trageser D, et al. Residual tumor cells are unique cellular targets in glioblastoma. Ann Neurol. (2010) 68:264–9. doi: 10.1002/ana.22036
39. Molina JR, Hayashi Y, Stephens C, Georgescu MM. Invasive glioblastoma cells acquire stemness and increased Akt activation. Neoplasia. (2010) 12:453–63. doi: 10.1593/neo.10126
40. Smith SJ, Diksin M, Chhaya S, Sairam S, Estevez-Cebrero MA, Rahman R. The invasive region of glioblastoma defined by 5ALA guided surgery has an altered cancer stem cell marker profile compared to central tumour. Int J Mol Sci. (2017) 18(6):453–63. doi: 10.3390/ijms18112452
41. Fazi B, Felsani A, Grassi L, Moles A, D’Andrea D, Toschi N, et al. The transcriptome and miRNome profiling of glioblastoma tissues and peritumoral regions highlights molecular pathways shared by tumors and surrounding areas and reveals differences between short-term and long-term survivors. Oncotarget. (2015) 6:22526–52. doi: 10.18632/oncotarget.v6i26
42. Mangiola A, Saulnier N, De Bonis P, Orteschi D, Sica G, Lama G, et al. Gene expression profile of glioblastoma peritumoral tissue: an ex vivo study. PloS One. (2013) 8:e57145. doi: 10.1371/journal.pone.0057145
43. Nakajima S, Morii K, Takahashi H, Fujii Y, Yamanaka R. Prognostic significance of S-phase fractions in peritumoral invading zone analyzed by laser scanning cytometry in patients with high-grade glioma: A preliminary study. Oncol Lett. (2016) 11:2106–10. doi: 10.3892/ol.2016.4205
44. Harland A, Liu X, Ghirardello M, Galan MC, Perks CM, Kurian KM. Glioma stem-like cells and metabolism: potential for novel therapeutic strategies. Front Oncol. (2021) 11:743814. doi: 10.3389/fonc.2021.743814
45. Uribe D, Niechi I, Rackov G, Erices JI, San Martín R, Quezada C. Adapt to persist: glioblastoma microenvironment and epigenetic regulation on cell plasticity. Biol (Basel). (2022) 11(2):313. doi: 10.3390/biology11020313
46. Minata M, Audia A, Shi J, Lu S, Bernstock J, Pavlyukov MS, et al. Phenotypic plasticity of invasive edge glioma stem-like cells in response to ionizing radiation. Cell Rep. (2019) 26:1893–905.e7. doi: 10.1016/j.celrep.2019.01.076
47. Li C, Cho HJ, Yamashita D, Abdelrashid M, Chen Q, Bastola S, et al. Tumor edge-to-core transition promotes Malignancy in primary-to-recurrent glioblastoma progression in a PLAGL1/CD109-mediated mechanism. Neurooncol Adv. (2020) 2:vdaa163. doi: 10.1093/noajnl/vdaa163
48. Nishikawa M, Inoue A, Ohnishi T, Kohno S, Ohue S, Matsumoto S, et al. Significance of glioma stem-like cells in the tumor periphery that express high levels of CD44 in tumor invasion, early progression, and poor prognosis in glioblastoma. Stem Cells Int. (2018) 2018:5387041. doi: 10.1155/2018/5387041
49. Bhat KPL, Balasubramaniyan V, Vaillant B, Ezhilarasan R, Hummelink K, Hollingsworth F, et al. Mesenchymal differentiation mediated by NF-kappaB promotes radiation resistance in glioblastoma. Cancer Cell. (2013) 24:331–46. doi: 10.1016/j.ccr.2013.08.001
50. Si D, Yin F, Peng J, Zhang G. High expression of CD44 predicts a poor prognosis in glioblastomas. Cancer Manag Res. (2020) 12:769–75. doi: 10.2147/CMAR
51. Hatzikirou H, Basanta D, Simon M, Schaller K, Deutsch A. ‘Go or grow’: the key to the emergence of invasion in tumour progression? Math Med Biol. (2012) 29:49–65. doi: 10.1093/imammb/dqq011
52. Herrlich P, Morrison H, Sleeman J, Orian-Rousseau V, Konig H, Weg-Remers S, et al. CD44 acts both as a growth- and invasiveness-promoting molecule and as a tumor-suppressing cofactor. Ann N Y Acad Sci. (2000) 910:106–18. doi: 10.1111/j.1749-6632.2000.tb06704.x
53. Demuth T, Berens ME. Molecular mechanisms of glioma cell migration and invasion. J Neurooncol. (2004) 70:217–28. doi: 10.1007/s11060-004-2751-6
54. Bonosi L, Marrone S, Benigno UE, Buscemi F, Musso S, Porzio M, et al. Maximal safe resection in glioblastoma surgery: A systematic review of advanced intraoperative image-guided techniques. Brain Sci. (2023) 13(2):216. doi: 10.3390/brainsci13020216
55. Louis DN. Molecular pathology of Malignant gliomas. Annu Rev Pathol. (2006) 1:97–117. doi: 10.1146/annurev.pathol.1.110304.100043
56. de Gooijer MC, Guillen Navarro M, Bernards R, Wurdinger T, van Tellingen O. An experimenter’s guide to glioblastoma invasion pathways. Trends Mol Med. (2018) 24:763–80. doi: 10.1016/j.molmed.2018.07.003
57. Burden-Gulley SM, Qutaish MQ, Sullivant KE, Lu H, Wang J, Craig SE, et al. Novel cryo-imaging of the glioma tumor microenvironment reveals migration and dispersal pathways in vivid three-dimensional detail. Cancer Res. (2011) 71:5932–40. doi: 10.1158/0008-5472.CAN-11-1553
58. Hanahan D, Weinberg RA. Hallmarks of cancer: the next generation. Cell. (2011) 144:646–74. doi: 10.1016/j.cell.2011.02.013
59. Griveau A, Seano G, Shelton SJ, Kupp R, Jahangiri A, Obernier K, et al. A glial signature and wnt7 signaling regulate glioma-vascular interactions and tumor microenvironment. Cancer Cell. (2018) 33:874–89 e7. doi: 10.1016/j.ccell.2018.03.020
60. Cuddapah VA, Robel S, Watkins S, Sontheimer H. A neurocentric perspective on glioma invasion. Nat Rev Neurosci. (2014) 15:455–65. doi: 10.1038/nrn3765
61. Montana V, Sontheimer H. Bradykinin promotes the chemotactic invasion of primary brain tumors. J Neurosci. (2011) 31:4858–67. doi: 10.1523/JNEUROSCI.3825-10.2011
62. Diksin M, Smith SJ, Rahman R. The molecular and phenotypic basis of the glioma invasive perivascular niche. Int J Mol Sci. (2017) 18(11):2342. doi: 10.3390/ijms18112342
63. Calabrese C, Poppleton H, Kocak M, Hogg TL, Fuller C, Hamner B, et al. A perivascular niche for brain tumor stem cells. Cancer Cell. (2007) 11:69–82. doi: 10.1016/j.ccr.2006.11.020
64. Brooks LJ, Parrinello S. Vascular regulation of glioma stem-like cells: a balancing act. Curr Opin Neurobiol. (2017) 47:8–15. doi: 10.1016/j.conb.2017.06.008
65. Li Z, Bao S, Wu Q, Wang H, Eyler C, Sathornsumetee S, et al. Hypoxia-inducible factors regulate tumorigenic capacity of glioma stem cells. Cancer Cell. (2009) 15:501–13. doi: 10.1016/j.ccr.2009.03.018
66. Seano G, Jain RK. Vessel co-option in glioblastoma: emerging insights and opportunities. Angiogenesis. (2020) 23:9–16. doi: 10.1007/s10456-019-09691-z
67. Seano G. Targeting the perivascular niche in brain tumors. Curr Opin Oncol. (2018) 30:54–60. doi: 10.1097/CCO.0000000000000417
68. Kuczynski EA, Vermeulen PB, Pezzella F, Kerbel RS, Reynolds AR. Vessel co-option in cancer. Nat Rev Clin Oncol. (2019) 16:469–93. doi: 10.1038/s41571-019-0181-9
70. Brooks LJ, Clements MP, Burden JJ, Kocher D, Richards L, Devesa SC, et al. The white matter is a pro-differentiative niche for glioblastoma. Nat Commun. (2021) 12:2184. doi: 10.1038/s41467-021-22225-w
71. Sharma P, Aaroe A, Liang J, Puduvalli VK. Tumor microenvironment in glioblastoma: Current and emerging concepts. Neurooncol Adv. (2023) 5:vdad009. doi: 10.1093/noajnl/vdad009
72. Jung E, Alfonso J, Osswald M, Monyer H, Wick W, Winkler F. Emerging intersections between neuroscience and glioma biology. Nat Neurosci. (2019) 22:1951–60. doi: 10.1038/s41593-019-0540-y
73. Osswald M, Jung E, Sahm F, Solecki G, Venkataramani V, Blaes J, et al. Brain tumour cells interconnect to a functional and resistant network. Nature. (2015) 528:93–8. doi: 10.1038/nature16071
74. Venkatesh HS, Tam LT, Woo PJ, Lennon J, Nagaraja S, Gillespie SM, et al. Targeting neuronal activity-regulated neuroligin-3 dependency in high-grade glioma. Nature. (2017) 549:533–7. doi: 10.1038/nature24014
75. Heuer S, Winkler F. Glioblastoma revisited: from neuronal-like invasion to pacemaking. Trends Cancer. (2023) 9:887–96. doi: 10.1016/j.trecan.2023.07.009
76. Gafarov FM. Neural electrical activity and neural network growth. Neural Netw. (2018) 101:15–24. doi: 10.1016/j.neunet.2018.02.001
77. Tantillo E, Vannini E, Cerri C, Spalletti C, Colistra A, Mazzanti CM, et al. Differential roles of pyramidal and fast-spiking, GABAergic neurons in the control of glioma cell proliferation. Neurobiol Dis. (2020) 141:104942. doi: 10.1016/j.nbd.2020.104942
78. de Groot J, Sontheimer H. Glutamate and the biology of gliomas. Glia. (2011) 59:1181–9. doi: 10.1002/glia.21113
79. Pan Y, Hysinger JD, Barron T, Schindler NF, Cobb O, Guo X, et al. NF1 mutation drives neuronal activity-dependent initiation of optic glioma. Nature. (2021) 594:277–82. doi: 10.1038/s41586-021-03580-6
80. Venkatesh HS, Johung TB, Caretti V, Noll A, Tang Y, Nagaraja S, et al. Neuronal activity promotes glioma growth through neuroligin-3 secretion. Cell. (2015) 161:803–16. doi: 10.1016/j.cell.2015.04.012
81. Venkataramani V, Yang Y, Schubert MC, Reyhan E, Tetzlaff SK, Wißmann N, et al. Glioblastoma hijacks neuronal mechanisms for brain invasion. Cell. (2022) 185:2899–917.e31. doi: 10.1016/j.cell.2022.06.054
82. Humphries NE, Weimerskirch H, Queiroz N, Southall EJ, Sims DW. Foraging success of biological Levy flights recorded in situ. Proc Natl Acad Sci U.S.A. (2012) 109:7169–74. doi: 10.1073/pnas.1121201109
83. Bressan C, Saghatelyan A. Intrinsic mechanisms regulating neuronal migration in the postnatal brain. Front Cell Neurosci. (2020) 14:620379. doi: 10.3389/fncel.2020.620379
84. Hausmann D, Hoffmann DC, Venkataramani V, Jung E, Horschitz S, Tetzlaff SK, et al. Autonomous rhythmic activity in glioma networks drives brain tumour growth. Nature. (2023) 613:179–86. doi: 10.1038/s41586-022-05520-4
85. Allen NJ, Eroglu C. Cell biology of astrocyte-synapse interactions. Neuron. (2017) 96:697–708. doi: 10.1016/j.neuron.2017.09.056
86. Linnerbauer M, Wheeler MA, Quintana FJ. Astrocyte crosstalk in CNS inflammation. Neuron. (2020) 108:608–22. doi: 10.1016/j.neuron.2020.08.012
87. Mayo L, Trauger SA, Blain M, Nadeau M, Patel B, Alvarez JI, et al. Regulation of astrocyte activation by glycolipids drives chronic CNS inflammation. Nat Med. (2014) 20:1147–56. doi: 10.1038/nm.3681
88. Wheeler MA, Jaronen M, Covacu R, Zandee SEJ, Scalisi G, Rothhammer V, et al. Environmental control of astrocyte pathogenic activities in CNS inflammation. Cell. (2019) 176:581–96.e18. doi: 10.1016/j.cell.2018.12.012
89. Buffo A, Rolando C, Ceruti S. Astrocytes in the damaged brain: molecular and cellular insights into their reactive response and healing potential. Biochem Pharmacol. (2010) 79:77–89. doi: 10.1016/j.bcp.2009.09.014
90. Mega A, Hartmark Nilsen M, Leiss LW, Tobin NP, Miletic H, Sleire L, et al. Astrocytes enhance glioblastoma growth. Glia. (2020) 68:316–27. doi: 10.1002/glia.23718
91. Guan X, Hasan MN, Maniar S, Jia W, Sun D. Reactive astrocytes in glioblastoma multiforme. Mol Neurobiol. (2018) 55:6927–38. doi: 10.1007/s12035-018-0880-8
92. Noronha C, Ribeiro AS, Taipa R, Castro DS, Reis J, Faria C, et al. Cadherin expression and EMT: A focus on gliomas. Biomedicines. (2021) 9(10):1328. doi: 10.3390/biomedicines9101328
93. Zhang W, Couldwell WT, Simard MF, Song H, Lin JH, Nedergaard M. Direct gap junction communication between Malignant glioma cells and astrocytes. Cancer Res. (1999) 59:1994–2003.
94. Soroceanu L, Manning TJ, Sontheimer H. Reduced expression of connexin-43 and functional gap junction coupling in human gliomas. Glia. (2001) 33:107–17. doi: 10.1002/(ISSN)1098-1136
95. Katz AM, Amankulor NM, Pitter K, Helmy K, Squatrito M, Holland EC. Astrocyte-specific expression patterns associated with the PDGF-induced glioma microenvironment. PloS One. (2012) 7:e32453. doi: 10.1371/journal.pone.0032453
96. Pietras A, Katz AM, Ekström EJ, Wee B, Halliday JJ, Pitter KL, et al. Osteopontin-CD44 signaling in the glioma perivascular niche enhances cancer stem cell phenotypes and promotes aggressive tumor growth. Cell Stem Cell. (2014) 14:357–69. doi: 10.1016/j.stem.2014.01.005
97. Shabtay-Orbach A, Amit M, Binenbaum Y, Na’ara S, Gil Z. Paracrine regulation of glioma cells invasion by astrocytes is mediated by glial-derived neurotrophic factor. Int J Cancer. (2015) 137:1012–20. doi: 10.1002/ijc.29380
98. Henrik Heiland D, Ravi VM, Behringer SP, Frenking JH, Wurm J, Joseph K, et al. Tumor-associated reactive astrocytes aid the evolution of immunosuppressive environment in glioblastoma. Nat Commun. (2019) 10:2541. doi: 10.1038/s41467-019-10493-6
99. Ellert-Miklaszewska A, Wisniewski P, Kijewska M, Gajdanowicz P, Pszczolkowska D, Przanowski P, et al. Tumour-processed osteopontin and lactadherin drive the protumorigenic reprogramming of microglia and glioma progression. Oncogene. (2016) 35:6366–77. doi: 10.1038/onc.2016.55
100. Eder K, Kalman B. The dynamics of interactions among immune and glioblastoma cells. Neuromol Med. (2015) 17:335–52. doi: 10.1007/s12017-015-8362-x
101. Matias D, Balça-Silva J, da Graça GC, Wanjiru CM, Macharia LW, Nascimento CP, et al. Microglia/astrocytes-glioblastoma crosstalk: crucial molecular mechanisms and microenvironmental factors. Front Cell Neurosci. (2018) 12:235. doi: 10.3389/fncel.2018.00235
102. Brandao M, Simon T, Critchley G, Giamas G. Astrocytes, the rising stars of the glioblastoma microenvironment. Glia. (2019) 67:779–90. doi: 10.1002/glia.23520
103. Perelroizen R, Philosof B, Budick-Harmelin N, Chernobylsky T, Ron A, Katzir R, et al. Astrocyte immunometabolic regulation of the tumour microenvironment drives glioblastoma pathogenicity. Brain: J neurology. (2022) 145:3288–307. doi: 10.1093/brain/awac222
104. Chen Q, Boire A, Jin X, Valiente M, Er EE, Lopez-Soto A, et al. Carcinoma-astrocyte gap junctions promote brain metastasis by cGAMP transfer. Nature. (2016) 533:493–8. doi: 10.1038/nature18268
105. Jiang Y, Han S, Cheng W, Wang Z, Wu A. NFAT1-regulated IL6 signalling contributes to aggressive phenotypes of glioma. Cell Commun Signal. (2017) 15:54. doi: 10.1186/s12964-017-0210-1
106. Daneman R, Prat A. The blood-brain barrier. Cold Spring Harb Perspect Biol. (2015) 7:a020412. doi: 10.1101/cshperspect.a020412
107. Testa E, Palazzo C, Mastrantonio R, Viscomi MT. Dynamic interactions between tumor cells and brain microvascular endothelial cells in glioblastoma. Cancers (Basel). (2022) 14(13):3128. doi: 10.3390/cancers14133128
108. Charalambous C, Chen TC, Hofman FM. Characteristics of tumor-associated endothelial cells derived from glioblastoma multiforme. Neurosurg Focus. (2006) 20:E22. doi: 10.3171/foc.2006.20.4.e22
109. Xie Y, He L, Lugano R, Zhang Y, Cao H, He Q, et al. Key molecular alterations in endothelial cells in human glioblastoma uncovered through single-cell RNA sequencing. JCI Insight. (2021) 6(15):e150861. doi: 10.1172/jci.insight.150861
110. Luoto S, Hermelo I, Vuorinen EM, Hannus P, Kesseli J, Nykter M, et al. Computational characterization of suppressive immune microenvironments in glioblastoma. Cancer Res. (2018) 78:5574–85. doi: 10.1158/0008-5472.CAN-17-3714
111. Uriarte Huarte O, Richart L, Mittelbronn M, Michelucci A. Microglia in health and disease: the strength to be diverse and reactive. Front Cell Neurosci. (2021) 15:660523. doi: 10.3389/fncel.2021.660523
112. Gieryng A, Pszczolkowska D, Walentynowicz KA, Rajan WD, Kaminska B. Immune microenvironment of gliomas. Lab Invest. (2017) 97:498–518. doi: 10.1038/labinvest.2017.19
113. Buonfiglioli A, Hambardzumyan D. Macrophages and microglia: the cerberus of glioblastoma. Acta Neuropathol Commun. (2021) 9:54. doi: 10.1186/s40478-021-01156-z
114. Butovsky O, Jedrychowski MP, Moore CS, Cialic R, Lanser AJ, Gabriely G, et al. Identification of a unique TGF-β-dependent molecular and functional signature in microglia. Nat Neurosci. (2014) 17:131–43. doi: 10.1038/nn.3599
115. Buttgereit A, Lelios I, Yu X, Vrohlings M, Krakoski NR, Gautier EL, et al. Erratum: Sall1 is a transcriptional regulator defining microglia identity and function. Nat Immunol. (2017) 18:246. doi: 10.1038/ni0217-246c
116. Bennett ML, Bennett FC, Liddelow SA, Ajami B, Zamanian JL, Fernhoff NB, et al. New tools for studying microglia in the mouse and human CNS. Proc Natl Acad Sci U S A. (2016) 113:E1738–46. doi: 10.1073/pnas.1525528113
117. Masuda T, Sankowski R, Staszewski O, Prinz M. Microglia heterogeneity in the single-cell era. Cell Rep. (2020) 30:1271–81. doi: 10.1016/j.celrep.2020.01.010
118. Bowman RL, Klemm F, Akkari L, Pyonteck SM, Sevenich L, Quail DF, et al. Macrophage ontogeny underlies differences in tumor-specific education in brain Malignancies. Cell Rep. (2016) 17:2445–59. doi: 10.1016/j.celrep.2016.10.052
119. Werner Y, Mass E, Ashok Kumar P, Ulas T, Händler K, Horne A, et al. Cxcr4 distinguishes HSC-derived monocytes from microglia and reveals monocyte immune responses to experimental stroke. Nat Neurosci. (2020) 23:351–62. doi: 10.1038/s41593-020-0585-y
120. Landry AP, Balas M, Alli S, Spears J, Zador Z. Distinct regional ontogeny and activation of tumor associated macrophages in human glioblastoma. Sci Rep. (2020) 10:19542. doi: 10.1038/s41598-020-76657-3
121. Gordon SR, Maute RL, Dulken BW, Hutter G, George BM, McCracken MN, et al. PD-1 expression by tumour-associated macrophages inhibits phagocytosis and tumour immunity. Nature. (2017) 545:495–9. doi: 10.1038/nature22396
122. Ene CI, Kreuser SA, Jung M, Zhang H, Arora S, White Moyes K, et al. Anti-PD-L1 antibody direct activation of macrophages contributes to a radiation-induced abscopal response in glioblastoma. Neuro Oncol. (2020) 22:639–51. doi: 10.1093/neuonc/noz226
123. Lawrence T, Natoli G. Transcriptional regulation of macrophage polarization: enabling diversity with identity. Nat Rev Immunol. (2011) 11:750–61. doi: 10.1038/nri3088
124. Porta C, Rimoldi M, Raes G, Brys L, Ghezzi P, Di Liberto D, et al. Tolerance and M2 (alternative) macrophage polarization are related processes orchestrated by p50 nuclear factor kappaB. Proc Natl Acad Sci U S A. (2009) 106:14978–83. doi: 10.1073/pnas.0809784106
125. Achyut BR, Angara K, Jain M, Borin TF, Rashid MH, Iskander ASM, et al. Canonical NFkappaB signaling in myeloid cells is required for the glioblastoma growth. Sci Rep. (2017) 7:13754. doi: 10.1038/s41598-017-14079-4
126. Barberi T, Martin A, Suresh R, Barakat DJ, Harris-Bookman S, Drake CG, et al. Absence of host NF-kappaB p50 induces murine glioblastoma tumor regression, increases survival, and decreases T-cell induction of tumor-associated macrophage M2 polarization. Cancer Immunol Immunother. (2018) 67:1491–503. doi: 10.1007/s00262-018-2184-2
127. Chen Z, Feng X, Herting CJ, Garcia VA, Nie K, Pong WW, et al. Cellular and molecular identity of tumor-associated macrophages in glioblastoma. Cancer Res. (2017) 77:2266–78. doi: 10.1158/0008-5472.CAN-16-2310
128. Chen Z, Ross JL, Hambardzumyan D. Intravital 2-photon imaging reveals distinct morphology and infiltrative properties of glioblastoma-associated macrophages. Proc Natl Acad Sci U S A. (2019) 116:14254–9. doi: 10.1073/pnas.1902366116
129. Darmanis S, Sloan SA, Croote D, Mignardi M, Chernikova S, Samghababi P, et al. Single-cell RNA-seq analysis of infiltrating neoplastic cells at the migrating front of human glioblastoma. Cell Rep. (2017) 21:1399–410. doi: 10.1016/j.celrep.2017.10.030
130. Friebel E, Kapolou K, Unger S, Núñez NG, Utz S, Rushing EJ, et al. Single-cell mapping of human brain cancer reveals tumor-specific instruction of tissue-invading leukocytes. Cell. (2020) 181:1626–42.e20. doi: 10.1016/j.cell.2020.04.055
131. Rahimi Koshkaki H, Minasi S, Ugolini A, Trevisi G, Napoletano C, Zizzari IG, et al. Immunohistochemical characterization of immune infiltrate in tumor microenvironment of glioblastoma. J Pers Med. (2020) 10(3):112. doi: 10.3390/jpm10030112
132. Chen DS, Mellman I. Oncology meets immunology: the cancer-immunity cycle. Immunity. (2013) 39:1–10. doi: 10.1016/j.immuni.2013.07.012
133. Lohr J, Ratliff T, Huppertz A, Ge Y, Dictus C, Ahmadi R, et al. Effector T-cell infiltration positively impacts survival of glioblastoma patients and is impaired by tumor-derived TGF-β. Clin Cancer Res. (2011) 17:4296–308. doi: 10.1158/1078-0432.CCR-10-2557
134. Jiang Y, Li Y, Zhu B. T-cell exhaustion in the tumor microenvironment. Cell Death Dis. (2015) 6:e1792. doi: 10.1038/cddis.2015.162
135. Saeidi A, Zandi K, Cheok YY, Saeidi H, Wong WF, Lee CYQ, et al. T-cell exhaustion in chronic infections: reversing the state of exhaustion and reinvigorating optimal protective immune responses. Front Immunol. (2018) 9:2569. doi: 10.3389/fimmu.2018.02569
136. Watowich MB, Gilbert MR, Larion M. T cell exhaustion in Malignant gliomas. Trends Cancer. (2023) 9:270–92. doi: 10.1016/j.trecan.2022.12.008
137. Tamura R, Ohara K, Sasaki H, Morimoto Y, Kosugi K, Yoshida K, et al. Difference in immunosuppressive cells between peritumoral area and tumor core in glioblastoma. World Neurosurg. (2018) 120:e601–e10. doi: 10.1016/j.wneu.2018.08.133
138. Hasmim M, Noman MZ, Messai Y, Bordereaux D, Gros G, Baud V, et al. Cutting edge: Hypoxia-induced Nanog favors the intratumoral infiltration of regulatory T cells and macrophages via direct regulation of TGF-β1. J Immunol. (2013) 191:5802–6. doi: 10.4049/jimmunol.1302140
139. Kim AR, Choi SJ, Park J, Kwon M, Chowdhury T, Yu HJ, et al. Spatial immune heterogeneity of hypoxia-induced exhausted features in high-grade glioma. Oncoimmunology. (2022) 11:2026019. doi: 10.1080/2162402X.2022.2026019
140. Aboody KS, Brown A, Rainov NG, Bower KA, Liu S, Yang W, et al. Neural stem cells display extensive tropism for pathology in adult brain: evidence from intracranial gliomas. Proc Natl Acad Sci U S A. (2000) 97:12846–51. doi: 10.1073/pnas.97.23.12846
141. Fares J, Ahmed AU, Ulasov IV, Sonabend AM, Miska J, Lee-Chang C, et al. Neural stem cell delivery of an oncolytic adenovirus in newly diagnosed Malignant glioma: a first-in-human, phase 1, dose-escalation trial. Lancet Oncol. (2021) 22:1103–14. doi: 10.1016/S1470-2045(21)00245-X
142. Nia HT, Munn LL, Jain RK. Physical traits of cancer. Science. (2020) 370(6516):eaaz0868. doi: 10.1126/science.aaz0868
143. Stylianopoulos T, Martin JD, Snuderl M, Mpekris F, Jain SR, Jain RK. Coevolution of solid stress and interstitial fluid pressure in tumors during progression: implications for vascular collapse. Cancer Res. (2013) 73:3833–41. doi: 10.1158/0008-5472.CAN-12-4521
144. Heldin CH, Rubin K, Pietras K, Ostman A. High interstitial fluid pressure - an obstacle in cancer therapy. Nat Rev Cancer. (2004) 4:806–13. doi: 10.1038/nrc1456
145. Seano G, Nia HT, Emblem KE, Datta M, Ren J, Krishnan S, et al. Solid stress in brain tumours causes neuronal loss and neurological dysfunction and can be reversed by lithium. Nat BioMed Eng. (2019) 3:230–45. doi: 10.1038/s41551-018-0334-7
146. Weil S, Osswald M, Solecki G, Grosch J, Jung E, Lemke D, et al. Tumor microtubes convey resistance to surgical lesions and chemotherapy in gliomas. Neuro Oncol. (2017) 19:1316–26. doi: 10.1093/neuonc/nox070
147. Miroshnikova YA, Mouw JK, Barnes JM, Pickup MW, Lakins JN, Kim Y, et al. Tissue mechanics promote IDH1-dependent HIF1alpha-tenascin C feedback to regulate glioblastoma aggression. Nat Cell Biol. (2016) 18:1336–45. doi: 10.1038/ncb3429
148. Flogstad Svensson S, Fuster-Garcia E, Latysheva A, Fraser-Green J, Nordhoy W, Isam Darwish O, et al. Decreased tissue stiffness in glioblastoma by MR elastography is associated with increased cerebral blood flow. Eur J Radiol. (2022) 147:110136. doi: 10.1016/j.ejrad.2021.110136
149. Huang JY, Cheng YJ, Lin YP, Lin HC, Su CC, Juliano R, et al. Extracellular matrix of glioblastoma inhibits polarization and transmigration of T cells: the role of tenascin-C in immune suppression. J Immunol. (2010) 185:1450–9. doi: 10.4049/jimmunol.0901352
150. Joyce JA, Fearon DT. T cell exclusion, immune privilege, and the tumor microenvironment. Science. (2015) 348:74–80. doi: 10.1126/science.aaa6204
151. Latysheva A, Geier OM, Hope TR, Brunetti M, Micci F, Vik-Mo EO, et al. Diagnostic utility of Restriction Spectrum Imaging in the characterization of the peritumoral brain zone in glioblastoma: Analysis of overall and progression-free survival. Eur J Radiol. (2020) 132:109289. doi: 10.1016/j.ejrad.2020.109289
152. Martirosyan NL, Cavalcanti DD, Eschbacher JM, Delaney PM, Scheck AC, Abdelwahab MG, et al. Use of in vivo near-infrared laser confocal endomicroscopy with indocyanine green to detect the boundary of infiltrative tumor. J Neurosurg. (2011) 115:1131–8. doi: 10.3171/2011.8.JNS11559
153. Neska-Matuszewska M, Bladowska J, Sasiadek M, Zimny A. Differentiation of glioblastoma multiforme, metastases and primary central nervous system lymphomas using multiparametric perfusion and diffusion MR imaging of a tumor core and a peritumoral zone-Searching for a practical approach. PloS One. (2018) 13:e0191341. doi: 10.1371/journal.pone.0191341
154. Zhang N, Zhang L, Qiu B, Meng L, Wang X, Hou BL. Correlation of volume transfer coefficient Ktrans with histopathologic grades of gliomas. J Magn Reson Imaging. (2012) 36:355–63. doi: 10.1002/jmri.23675
155. Leuthardt EC, Duan C, Kim MJ, Campian JL, Kim AH, Miller-Thomas MM, et al. Hyperthermic laser ablation of recurrent glioblastoma leads to temporary disruption of the peritumoral blood brain barrier. PloS One. (2016) 11:e0148613. doi: 10.1371/journal.pone.0148613
156. Yamahara T, Numa Y, Oishi T, Kawaguchi T, Seno T, Asai A, et al. Morphological and flow cytometric analysis of cell infiltration in glioblastoma: a comparison of autopsy brain and neuroimaging. Brain Tumor Pathol. (2010) 27:81–7. doi: 10.1007/s10014-010-0275-7
157. Csutak C, Stefan PA, Lenghel LM, Morosanu CO, Lupean RA, Simonca L, et al. Differentiating high-grade gliomas from brain metastases at magnetic resonance: the role of texture analysis of the peritumoral zone. Brain Sci. (2020) 10(9):638. doi: 10.3390/brainsci10090638
158. Hagiwara A, Yao J, Raymond C, Cho NS, Everson R, Patel K, et al. “Aerobic glycolytic imaging” of human gliomas using combined pH-, oxygen-, and perfusion-weighted magnetic resonance imaging. NeuroImage Clin. (2021) 32:102882. doi: 10.1016/j.nicl.2021.102882
159. Thiex R, Hans FJ, Krings T, Sellhaus B, Gilsbach JM. Technical pitfalls in a porcine brain retraction model. The impact of brain spatula on the retracted brain tissue in a porcine model: a feasibility study and its technical pitfalls. Neuroradiology. (2005) 47:765–73. doi: 10.1007/s00234-005-1426-0
160. Sanai N, Polley MY, McDermott MW, Parsa AT, Berger MS. An extent of resection threshold for newly diagnosed glioblastomas. J Neurosurg. (2011) 115:3–8. doi: 10.3171/2011.2.JNS10998
161. Bander ED, Magge R, Ramakrishna R. Advances in glioblastoma operative techniques. World Neurosurg. (2018) 116:529–38. doi: 10.1016/j.wneu.2018.04.023
162. Akbari H, Kazerooni AF, Ware JB, Mamourian E, Anderson H, Guiry S, et al. Quantification of tumor microenvironment acidity in glioblastoma using principal component analysis of dynamic susceptibility contrast enhanced MR imaging. Sci Rep. (2021) 11:15011. doi: 10.1038/s41598-021-94560-3
163. Deilami T, Hadizadeh Kharrazi H, Seddighi AS, Tanzifi P, Tayebivaljouzi R, Zamani F, et al. Evaluating the possibility of defining cut-off points for deltaFA% in order to differentiate four major types of peri-tumoral white matter tract involvement. Iran J Radiol. (2015) 12:e9567. doi: 10.5812/iranjradiol.9567v2
164. Noth U, Tichy J, Tritt S, Bahr O, Deichmann R, Hattingen E. Quantitative T1 mapping indicates tumor infiltration beyond the enhancing part of glioblastomas. NMR Biomed. (2020) 33:e4242. doi: 10.1002/nbm.4242
165. Prasanna P, Patel J, Partovi S, Madabhushi A, Tiwari P. Radiomic features from the peritumoral brain parenchyma on treatment-naive multi-parametric MR imaging predict long versus short-term survival in glioblastoma multiforme: Preliminary findings. Eur Radiol. (2017) 27:4188–97. doi: 10.1007/s00330-016-4637-3
166. Furtak J, Rakowska J, Szylberg T, Harat M, Malkowski B. Glioma biopsy based on hybrid dual time-point FET-PET/MRI-A proof of concept study. Front Neurol. (2021) 12:634609. doi: 10.3389/fneur.2021.634609
167. Brandes AA, Tosoni A, Franceschi E, Sotti G, Frezza G, Amista P, et al. Recurrence pattern after temozolomide concomitant with and adjuvant to radiotherapy in newly diagnosed patients with glioblastoma: correlation With MGMT promoter methylation status. J Clin Oncol. (2009) 27:1275–9. doi: 10.1200/JCO.2008.19.4969
168. Wang X, Ye L, He W, Teng C, Sun S, Lu H, et al. In situ targeting nanoparticles-hydrogel hybrid system for combined chemo-immunotherapy of glioma. J Control Release. (2022) 345:786–97. doi: 10.1016/j.jconrel.2022.03.050
169. Puente P, Fettig N, Luderer MJ, Jin A, Shah S, Muz B, et al. Injectable hydrogels for localized chemotherapy and radiotherapy in brain tumors. J Pharm Sci. (2018) 107:922–33. doi: 10.1016/j.xphs.2017.10.042
170. Bastiancich C, Bianco J, Vanvarenberg K, Ucakar B, Joudiou N, Gallez B, et al. Injectable nanomedicine hydrogel for local chemotherapy of glioblastoma after surgical resection. J Control Release. (2017) 264:45–54. doi: 10.1016/j.jconrel.2017.08.019
171. Chaichana KL, Pinheiro L, Brem H. Delivery of local therapeutics to the brain: working toward advancing treatment for Malignant gliomas. Ther Deliv. (2015) 6:353–69. doi: 10.4155/tde.14.114
172. Gallia GL, Brem S, Brem H. Local treatment of Malignant brain tumors using implantable chemotherapeutic polymers. J Natl Compr Canc Netw. (2005) 3:721–8. doi: 10.6004/jnccn.2005.0042
173. Brem H, Piantadosi S, Burger PC, Walker M, Selker R, Vick NA, et al. Placebo-controlled trial of safety and efficacy of intraoperative controlled delivery by biodegradable polymers of chemotherapy for recurrent gliomas. The Polymer-brain Tumor Treatment Group. Lancet. (1995) 345:1008–12. doi: 10.1016/S0140-6736(95)90755-6
174. Lin SH, Kleinberg LR. Carmustine wafers: localized delivery of chemotherapeutic agents in CNS Malignancies. Expert Rev Anticancer Ther. (2008) 8:343–59. doi: 10.1586/14737140.8.3.343
175. Westphal M, Hilt DC, Bortey E, Delavault P, Olivares R, Warnke PC, et al. A phase 3 trial of local chemotherapy with biodegradable carmustine (BCNU) wafers (Gliadel wafers) in patients with primary Malignant glioma. Neuro Oncol. (2003) 5:79–88. doi: 10.1215/S1522851702000236
176. De Bonis P, Anile C, Pompucci A, Fiorentino A, Balducci M, Chiesa S, et al. Safety and efficacy of Gliadel wafers for newly diagnosed and recurrent glioblastoma. Acta Neurochir (Wien). (2012) 154:1371–8. doi: 10.1007/s00701-012-1413-2
177. Han D, Teng L, Wang X, Zhen Y, Chen X, Yang M, et al. Phase I/II trial of local interstitial chemotherapy with arsenic trioxide in patients with newly diagnosed glioma. Front Neurol. (2022) 13:1001829. doi: 10.3389/fneur.2022.1001829
178. Patchell RA, Regine WF, Ashton P, Tibbs PA, Wilson D, Shappley D, et al. A phase I trial of continuously infused intratumoral bleomycin for the treatment of recurrent glioblastoma multiforme. J Neurooncol. (2002) 60:37–42. doi: 10.1023/A:1020291229317
179. Duerinck J, Schwarze JK, Awada G, Tijtgat J, Vaeyens F, Bertels C, et al. Intracerebral administration of CTLA-4 and PD-1 immune checkpoint blocking monoclonal antibodies in patients with recurrent glioblastoma: a phase I clinical trial. J Immunother Cancer. (2021) 9(6):e002296. doi: 10.1136/jitc-2020-002296
180. Ursu R, Carpentier A, Metellus P, Lubrano V, Laigle-Donadey F, Capelle L, et al. Intracerebral injection of CpG oligonucleotide for patients with de novo glioblastoma-A phase II multicentric, randomised study. Eur J Cancer. (2017) 73:30–7. doi: 10.1016/j.ejca.2016.12.003
181. Markert JM, Razdan SN, Kuo HC, Cantor A, Knoll A, Karrasch M, et al. A phase 1 trial of oncolytic HSV-1, G207, given in combination with radiation for recurrent GBM demonstrates safety and radiographic responses. Mol Ther. (2014) 22:1048–55. doi: 10.1038/mt.2014.22
182. Hassenbusch SJ, Nardone EM, Levin VA, Leeds N, Pietronigro D. Stereotactic injection of DTI-015 into recurrent Malignant gliomas: phase I/II trial. Neoplasia. (2003) 5:9–16. doi: 10.1016/S1476-5586(03)80012-X
183. Han Y, Park JH. Convection-enhanced delivery of liposomal drugs for effective treatment of glioblastoma multiforme. Drug Delivery Transl Res. (2020) 10:1876–87. doi: 10.1007/s13346-020-00773-w
184. Vogelbaum MA, Aghi MK. Convection-enhanced delivery for the treatment of glioblastoma. Neuro Oncol. (2015) 17 Suppl 2:ii3–8. doi: 10.1093/neuonc/nou354
185. van Solinge TS, Nieland L, Chiocca EA, Broekman MLD. Advances in local therapy for glioblastoma - taking the fight to the tumour. Nat Rev Neurol. (2022) 18:221–36. doi: 10.1038/s41582-022-00621-0
186. de Groot JF, Kim AH, Prabhu S, Rao G, Laxton AW, Fecci PE, et al. Efficacy of laser interstitial thermal therapy (LITT) for newly diagnosed and recurrent IDH wild-type glioblastoma. Neurooncol Adv. (2022) 4:vdac040. doi: 10.1093/noajnl/vdac040
187. Montemurro N, Anania Y, Cagnazzo F, Perrini P. Survival outcomes in patients with recurrent glioblastoma treated with Laser Interstitial Thermal Therapy (LITT): A systematic review. Clin Neurol Neurosurg. (2020) 195:105942. doi: 10.1016/j.clineuro.2020.105942
188. Thomas JG, Rao G, Kew Y, Prabhu SS. Laser interstitial thermal therapy for newly diagnosed and recurrent glioblastoma. Neurosurg Focus. (2016) 41:E12. doi: 10.3171/2016.7.FOCUS16234
189. Roberts JW, Powlovich L, Sheybani N, LeBlang S. Focused ultrasound for the treatment of glioblastoma. J Neurooncol. (2022) 157:237–47. doi: 10.1007/s11060-022-03974-0
190. Liu HL, Hsu PH, Lin CY, Huang CW, Chai WY, Chu PC, et al. Focused ultrasound enhances central nervous system delivery of bevacizumab for Malignant glioma treatment. Radiology. (2016) 281:99–108. doi: 10.1148/radiol.2016152444
191. Silva AC, Oliveira TR, Mamani JB, Malheiros SM, Malavolta L, Pavon LF, et al. Application of hyperthermia induced by superparamagnetic iron oxide nanoparticles in glioma treatment. Int J Nanomed. (2011) 6:591–603. doi: 10.2147/IJN
192. Cheng Y, Muroski ME, Petit D, Mansell R, Vemulkar T, Morshed RA, et al. Rotating magnetic field induced oscillation of magnetic particles for in vivo mechanical destruction of Malignant glioma. J Control Release. (2016) 223:75–84. doi: 10.1016/j.jconrel.2015.12.028
193. Maraka S, Asmaro K, Walbert T, Lee I. Cerebral edema induced by laser interstitial thermal therapy and radiotherapy in close succession in patients with brain tumor. Lasers Surg Med. (2018) 50:917–23. doi: 10.1002/lsm.22946
194. Hersh AM, Bhimreddy M, Weber-Levine C, Jiang K, Alomari S, Theodore N, et al. Applications of focused ultrasound for the treatment of glioblastoma: A new frontier. Cancers (Basel). (2022) 14(19):4920. doi: 10.3390/cancers14194920
195. Hurwitz M, Stauffer P. Hyperthermia, radiation and chemotherapy: the role of heat in multidisciplinary cancer care. Semin Oncol. (2014) 41:714–29. doi: 10.1053/j.seminoncol.2014.09.014
196. Schipmann S, Muther M, Stogbauer L, Zimmer S, Brokinkel B, Holling M, et al. Combination of ALA-induced fluorescence-guided resection and intraoperative open photodynamic therapy for recurrent glioblastoma: case series on a promising dual strategy for local tumor control. J Neurosurg. (2020) 24:1–11. doi: 10.3171/2019.11.JNS192443
197. Castano AP, Demidova TN, Hamblin MR. Mechanisms in photodynamic therapy: part one-photosensitizers, photochemistry and cellular localization. Photodiagnosis Photodyn Ther. (2004) 1:279–93. doi: 10.1016/S1572-1000(05)00007-4
198. Inglut CT, Gaitan B, Najafali D, Lopez IA, Connolly NP, Orsila S, et al. Predictors and limitations of the penetration depth of photodynamic effects in the rodent brain. Photochem Photobiol. (2020) 96:301–9. doi: 10.1111/php.13155
199. Harsh GR, Deisboeck TS, Louis DN, Hilton J, Colvin M, Silver JS, et al. Thymidine kinase activation of ganciclovir in recurrent Malignant gliomas: a gene-marking and neuropathological study. J Neurosurg. (2000) 92:804–11. doi: 10.3171/jns.2000.92.5.0804
200. Westphal M, Yla-Herttuala S, Martin J, Warnke P, Menei P, Eckland D, et al. Adenovirus-mediated gene therapy with sitimagene ceradenovec followed by intravenous ganciclovir for patients with operable high-grade glioma (ASPECT): a randomised, open-label, phase 3 trial. Lancet Oncol. (2013) 14:823–33. doi: 10.1016/S1470-2045(13)70274-2
201. Faisal SM, Castro MG, Lowenstein PR. Combined cytotoxic and immune-stimulatory gene therapy using Ad-TK and Ad-Flt3L: Translational developments from rodents to glioma patients. Mol Ther. (2023) 31:2839–60. doi: 10.1016/j.ymthe.2023.08.009
Keywords: glioblastoma, peritumoral brain zone, recurrence, tumor microenvironment, local therapy, cell plasticity and cell reprogramming, brain tumor, surgical resection
Citation: Ballestín A, Armocida D, Ribecco V and Seano G (2024) Peritumoral brain zone in glioblastoma: biological, clinical and mechanical features. Front. Immunol. 15:1347877. doi: 10.3389/fimmu.2024.1347877
Received: 01 December 2023; Accepted: 14 February 2024;
Published: 29 February 2024.
Edited by:
Syed M. Faisal, University of Michigan Medical School, United StatesReviewed by:
Chao Wei, Northwestern University, United StatesShwetal Mehta, Barrow Neurological Institute (BNI), United States
Anzar Mujeeb, University of Michigan, United States
Copyright © 2024 Ballestín, Armocida, Ribecco and Seano. This is an open-access article distributed under the terms of the Creative Commons Attribution License (CC BY). The use, distribution or reproduction in other forums is permitted, provided the original author(s) and the copyright owner(s) are credited and that the original publication in this journal is cited, in accordance with accepted academic practice. No use, distribution or reproduction is permitted which does not comply with these terms.
*Correspondence: Giorgio Seano, giorgio.seano@curie.fr
†These authors have contributed equally to this work and share first authorship
‡ORCID: Alberto Ballestín, orcid.org/0000-0002-7150-9758
Daniele Armocida, orcid.org/0000-0001-6550-5534
Valentino Ribecco, orcid.org/0000-0001-9348-4320
Giorgio Seano, orcid.org/0000-0002-7294-302X