- 1Department of Animal Nutrition and Feed Science, College of Animal Science and Technology, Huazhong Agricultural University, Wuhan, China
- 2The Cooperative Innovation Center for Sustainable Pig Production, Wuhan, China
Paneth cells are a group of unique intestinal epithelial cells, and they play an important role in host-microbiota interactions. At the origin of Paneth cell life, several pathways such as Wnt, Notch, and BMP signaling, affect the differentiation of Paneth cells. After lineage commitment, Paneth cells migrate downward and reside in the base of crypts, and they possess abundant granules in their apical cytoplasm. These granules contain some important substances such as antimicrobial peptides and growth factors. Antimicrobial peptides can regulate the composition of microbiota and defend against mucosal penetration by commensal and pathogenic bacteria to protect the intestinal epithelia. The growth factors derived from Paneth cells contribute to the maintenance of the normal functions of intestinal stem cells. The presence of Paneth cells ensures the sterile environment and clearance of apoptotic cells from crypts to maintain the intestinal homeostasis. At the end of their lives, Paneth cells experience different types of programmed cell death such as apoptosis and necroptosis. During intestinal injury, Paneth cells can acquire stem cell features to restore the intestinal epithelial integrity. In view of the crucial roles of Paneth cells in the intestinal homeostasis, research on Paneth cells has rapidly developed in recent years, and the existing reviews on Paneth cells have mainly focused on their functions of antimicrobial peptide secretion and intestinal stem cell support. This review aims to summarize the approaches to studying Paneth cells and introduce the whole life experience of Paneth cells from birth to death.
Introduction
The intestinal epithelium, which consists of a single layer of intestinal cells, is an important barrier separating intestinal contents from tissues (1). The intestinal epithelium is composed of absorptive cells such as enterocytes (the most abundant cell type) and secretory cells such as Paneth cells (PCs), goblet cells, and enteroendocrine cells (2). The small intestine is structurally divided into two parts, convex villus and concave crypts (3). Unlike other secretory cells, PCs, as a unique type of epithelial cells, are located at the bottom of crypts where they are intercalated between intestinal stem cells (ISCs).
PCs were first identified by Gustav Schwalbe based on the obvious cytoplasmic granules in 1872 (4), and further named by Josef Paneth in 1887 (5). PCs are normally observed in the small intestine, especially ileum, with 5-15 cells per crypt (6, 7). However, in pathological condition, PCs are detected at other tissues such as stomach and colon, and this phenomenon is called PC metaplasia (8). Although metaplastic PCs exhibit a protective effect against infections, they also promote tumorigenesis by secreting growth-promoting factors (8).
In contrast to the 4-5 day lifespan of enterocytes, PCs survive approximately one month in crypts (9). PCs are widely found in the intestine of various species such as humans, mice, pigs, and horses (10–12). However, most studies on PCs are conducted based on the samples from humans and mice, and the results have shown that the development of PCs in humans is different from that in mice. The first emergence of PCs in humans is before birth (13.5 weeks gestational age), while that in mice is after birth (7-10 days old) (13, 14). However, the development and functions of PCs in other species remain largely unclear (12).
Recently, PCs have attracted many researchers due to their beneficial effects on the intestine (1), and recent research highlights the importance of PCs in the regulation of intestinal microbiota and ISCs. To promote a comprehensive understanding of PCs, this review discusses the main materials and methodology for PC study and the events during entire lives of PCs.
Morphological characteristics of PCs
The presence of abundant granules in the cytoplasm is the first clue to PC discovery, and it remains a histological feature used for PC identification now (4). These granules in the apical cytoplasm of PCs mainly contain multiple antibacterial peptides (AMPs) such as lysozyme, α-defensin, secretory phospholipase A2 (sPLA2) and some proteins such as lipopolysaccharide-binding protein (LBP) and norepinephrine (9, 15, 16). The discovery of lysozyme in the granules is the first evidence of the protective role of PCs in the intestine (17). PCs possess extensive endoplasmic reticulum (ER) and trans-Golgi network (TGN) to synthesize and process proteins, which enables them to rapidly supplement the released granules (9). The above morphological characteristics suggest that PCs are a unique type of intestinal epithelial cell with particular structure. Notably, staining identification shows that PCs of some species such as pigs contain no granules (18).
Materials and methods for PC investigation
Multiple materials and methods are required to investigate the alterations of PCs. Selecting appropriate experimental materials is important for PC research. The majority of PC-related studies utilize C57BL/6 mouse strain since this mouse strain possesses more PCs with an abundant AMP profile, compared to 129/SvEv mouse strain (19).
In PC studies, PC interference tends to be conducted by chemical and transgenic methods in mice (20, 21). Dithizone, a zinc chelator, has been reported to selectively eliminate PCs from the small intestine of mice and rats (22). However, the PC-ablation effect of dithizone lasts only for a short time, and then renascent PCs emerge in the crypts at 12 h after ablation and completely restore to normal state at 72 h (22). Intestinal transforming growth factor (TGF)-α and TGF-β1 are responsible for the rapid regeneration of PCs (23). Another method for PC ablation includes two steps: first, a human diphtheria toxin (DTX) receptor is inserted into mouse’s cryptdin-2 (Defa6) promoter to produce transgenic mouse strain PC-DTR (20), and then administration of PC-DTR mice with DTX leads to a decline of PC number (20, 24). Nevertheless, these two methods exhibit different effects in many aspects. In terms of PC-ablation efficiency, lysozyme staining results show that dithizone can reduce PCs by 33%, while DTX administration can reduce PCs by 60% (25). In addition, dithizone also contributes to autophagy-like changes in PCs (25). Tumor necrosis factor (TNF)-α released from dithizone-induced degenerated PCs can activate NF-κB signaling, thus promoting intestinal cell proliferation in rats (26). Furthermore, PC disruption induced by dithizone, rather than by DTX, impairs the small intestinal perfusion by down-regulating nitric oxide signaling (27). DTX administration leads to PC necrosis, thus possibly causing an increase in serum interleukin (IL)-6, IL-10, and TNF, whereas dithizone fails to trigger intestinal inflammation (25). These studies suggest that dithizone and DTX may act on PCs via different mechanisms. Therefore, it is necessary to make the appropriate choice between dithizone and DTX to delete PCs according to the objective of research. Additionally, gene editing makes it convenient to study the influence of certain gene on PCs in C57BL/6 mice. Mice with genes atonal homolog 1 (Atoh1), sex determining region Y-box 9 (Sox9), or growth factor independent 1 transcription repressor (Gfi1) knocked out are usually used as a PC ablation model (28–30). In addition to the methods of complete PC disruption mentioned above, transgenic Defa6-cre mice have been used to generate PC-specific gene knockout mice, which allows the exploration of the functions of certain gene in PCs (31). Notably, transgenic Defa6-cre mouse strain is established by Professor Blumberg (31), and he generously shares this mouse strain with other researchers (32, 33).
With the exception of mice, intestinal organoids, which are similar to the physiological structure and function of the intestinal epithelium in vivo, are also utilized to study PCs (34, 35). The intestinal organoids with a three-dimensional structure contain various intestinal epithelial cells formed from the continuous differentiation of ISCs ex vivo. Hans Clevers and Toshiro Sato first established intestinal organoids based on isolated ISCs in 2009, which lays a foundation for subsequent research on intestinal organoids (34). PCs intermingled with ISCs also exist at the bottom of crypts in organoids. Compared with mouse models, the intestinal organoids make PC studies more convenient, exhibiting multiple advantages such as stability and controllability.
Using various methods such as qPCR, western blot, and immunostaining, the alterations of PCs are examined in the mouse models and intestinal organoids (36, 37). Among them, histochemical staining mainly including periodic acid Schiff’s staining, eosin and phloxine-tartrazine stainings can visualize the abundant granules in the cytoplasm of PCs (38) In addition, transmission electron microscopy (TEM) enables researchers to observe the intracellular components of PCs such as granules and ER (33). Considering that PCs can express several unique genes (Defa1, Defa5, and Lyz1), the detection of these PC markers using qPCR, RNA-seq, and fluorescence in situ hybridization (FISH) usually reflects the changes of PCs at the gene expression level (11, 39). The western blot, immunohistochemistry, and immunofluorescence assays of α-defensin and lysozyme reveal the changes of PCs at the protein level. Notably, it is necessary to examine these PC markers at both mRNA and protein levels since the results at these two levels might be inconsistent due to the translational block caused by unfolded protein response (UPR) activation (40).
Flow cytometry (FACS) with CD24 antibody is used to analyze the PC number (11). FACS in combination with cell sorting allows the isolation of live PCs from the intestine (32). The proportion of PCs in CD24+ cells is extremely low (approximately 1.87%) (32). The isolated PCs can be cultured in vitro, which enables researchers to observe the alterations in PCs directly under different treatments (32). Another method for investigating molecular changes of PCs is laser capture microdissection (LCM). LCM can precisely isolate PCs from embedded tissue sections without impairing tissue structure for subsequent analysis (32, 41). Compared with FACS, LCM is more precise since it reflects the real changes in PCs under physiological condition.
Origin of PC life: Differentiation and migration
Coexistence of multiple cell types in the intestinal epithelium suggests that cell differentiation is a strict and complex process (Figure 1A). Cell differentiation in the intestine is determined by several signaling pathways such as Notch, Wnt, and bone morphogenetic protein (BMP) signaling (2). PC differentiation is conducted under the conditions of Notch signaling off and Wnt signaling on.
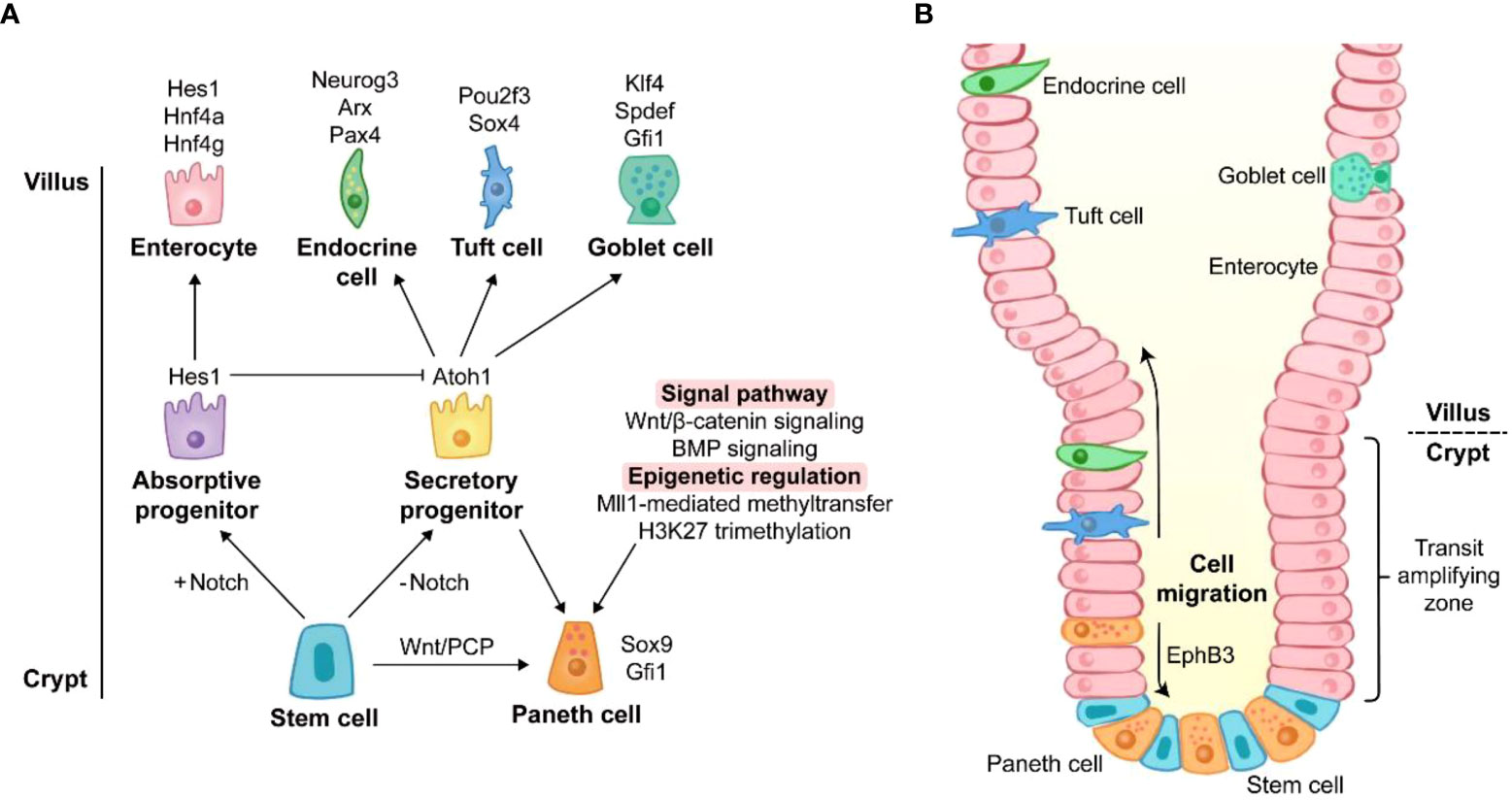
Figure 1 The origin of Paneth cell life. (A) Differentiation of Paneth cells. The differentiation towards secretory cells occurs when Notch signaling is off. Sox9 and Gfi1 are two key transcription factors of Paneth cell lineage. Wnt/PCP signaling directly drives the conversion of intestinal stem cells to Paneth cells. Wnt/β-catenin signaling and BMP signaling promote the lineage commitment of Paneth cells. Recent studies have reported that the differentiation of Paneth cells is also affected by epigenetic regulation such as Mll1-mediated methyltransfer and H3K27 trimethylation. The genes around the cell images are key transcription factors for the cell lineage commitment. (B) Migration of Paneth cells. Unlike other secretory cells, Paneth cells are located at the base of crypts. After differentiation, Paneth cells migrate downward to crypt bottom, and they are intercalated between stem cells. The migration of Paneth cells is mediated by EphB3.
Notch signaling is the key for lineage commitment towards absorptive cells or secretory cells. The 5-day Notch signaling inhibition by dibenzazepine (DBZ), an inhibitor of γ-secretase, leads to PC hyperplasia in mice (42). However, a recent study showed that acute Notch signaling inhibition (1 day after DBZ treatment) induced rapid apoptosis of PCs with a robust rebound of PCs at day 7 post DBZ treatment, suggesting the beneficial role of Notch signaling in PC maintenance rather than in lineage commitment (43). The dominance of Notch signaling in lineage commitment is achieved by its target gene, hairy and enhancer of split 1 (Hes1). Hes1 directly suppresses the transcription of Atoh1 which is responsible for the differentiation of secretory cells (42). Like DBZ-treatment, the deletion of Hes1 promotes PC differentiation in the small intestine (44). Moreover, deletion of Notch ligands Dll1 and Dll4 and their receptors Notch1 and Notch2 also contributes to an increase in PCs (42, 45, 46). It should be noted that Dll1 knockout is accompanied by the elevated expression of Dll4 (45), and Notch1 knockout induces the expression increase of Dll1 and Dll4 (46), which may be attributed to the increased PCs.
Wnt/β-catenin signaling can activate transcription factor 4 (Tcf4)-mediated transcription via nuclear localization of β-catenin, and this signaling plays a crucial role in PC differentiation in the intestine. Transgenic mice overexpressing Dkk1 (a Wnt signaling inhibitor) exhibit the absence of all secretory cell lineages including PCs (47). In contrast, Apclox/loxVilCreERT2 and Catnblox(ex3)Vil-CreERT2 mice (mouse models of Wnt/β-catenin activation) display higher level of PC differentiation, compared with wild type (WT) mice (48). Atoh1, a downstream gene of Wnt/β-catenin signaling, is essential for the maintenance of secretory progenitors. High expression of Atoh1 and nuclear localization of β-catenin/Tcf4 have been reported in PCs (47, 49). Atoh1 deletion results in a decrease in all secretory cell number (50) and the conversion from specified secretory cells into functional enterocytes (51). Furthermore, other factors are also involved in driving the conversion from secretory progenitors to the PC lineage rather than other secretory cells. Sox9 and Gfi1 are the main transcription factors responsible for the differentiation of PCs. Sox9 is also a target gene of Wnt/β-catenin signaling, and Gfi1 functions in the downstream of Atoh1 (29, 30). Lack of Sox9 or Gfi1 leads to PC lineage defects in mice (29, 30). Surprisingly, Atoh1 strengthens its own expression and directly regulates Dll1 and Dll4 which mediate lateral inhibition, a process maintaining a proper proportion of absorptive cells and secretory cells (51). Similar to canonical Wnt/β-catenin signaling, non-canonical Wnt/planar cell polarity (PCP) signaling is also involved in PC lineage differentiation, in spite of the mutual antagonization of their functions in some cases (52). Wnt/PCP signaling drives the differentiation from ISCs directly to PC and enteroendocrine cell lineages without the intermediate step of secretory progenitors (53).
In addition to Notch and Wnt signalings, several signaling pathways also act on PC lineage differentiation. BMP signaling modulates secretory cell maturation without interfering with Wnt/β-catenin signaling. The inhibition of BMP signaling by silencing BMP receptor type IA impairs terminal differentiation of PCs (54). Mammalian mitogen-activated protein kinase (MAPK) signaling disruption leads to a PC maturation defect (55). The nonreceptor tyrosine phosphatase Shp2-mediated MAPK signaling controls the fate between goblet cells and PCs, and Shp2/MAPK signaling weakening causes an increase in PC number meanwhile repressing goblet cell development (56).
In addition to transcription factors and signaling pathways, epigenetic regulation emerges as a novel modulator of PC lineage. The regulation of Wnt and MAPK signalings by histone methyltransferase Mll1 controls the lineage allocation between PCs and goblet cells (57). The inhibition of histone H3 lysine 27 (H3K27) trimethylation impairs PC maturation in the intestinal organoids (58). Thus, these studies indicate that PC lineage differentiation and development are intricate processes under the controls of multiple signaling pathways at both transcriptional and post-transcriptional levels.
After differentiation, most other cells migrate upward to villus, while PCs move to crypt bottom (Figure 1B) (59). Ephrin type-B receptor 3 (EphB3) is highly expressed in PCs and responsible for PC migration (60), and EphB3-knockout mice exhibit the disordered location of PCs along the villus-crypt axis (60). Since EphB3 is also a downstream target of Wnt/β-catenin signaling, Wnt inhibition via Frizzled-5 (a Wnt receptor on membrane) silencing and Dkk1 ectopic expression results in random scatter of PCs, and the absence of EphB3 is found in the disordered PCs (47, 49). Therefore, Wnt signaling is of great importance for both differentiation and migration of PCs.
PC functions in intestine
Located at the bottom of small intestinal crypts, PCs possess multiple functions such as shaping intestinal microbiota, promoting regeneration, and controlling inflammation. PC disruption by chemical and transgenic methods has a negative influence on the intestine as discussed above. AMPs secreted by PCs shape the structure of intestinal microbiota and confer crypts with a sterile environment. The ISC-supporting role of PCs facilitates intestinal regeneration in vivo and organoid formation ex vivo. Clearance of apoptotic cells from crypts by PCs prevents the occurrence of inflammation. Here we discuss the various participations of PCs in the intestinal homeostasis.
AMP secretion
There are trillions of microorganisms in the intestinal lumen, most of which are harmless and commensal. These commensal bacteria protect the intestine against pathogenic bacteria and produce nutrients from the fermentation of intestinal contents (61). However, the pathogenic bacteria with strong virulence can colonize the intestinal epithelium to invade host and exacerbate infections. PCs, as a source of abundant AMPs, play an important role in both controlling composition of commensal bacteria and defending against pathogenic bacteria. AMPs packed in apical cytoplasmic granules are an ancient type of host defense effectors in the intestinal innate immunity. The high AMP secretory characteristics of PC makes it an essential part of the intestinal innate immune system. In addition, the involvements of PCs in the intestinal innate immunity are also demonstrated by their functional proteins such as LBP, MD-2, and integrin α6β4 (15, 62, 63). Therefore, PCs could be called innate immune cells. Recent evidence has revealed that there are two subtypes of PCs which are distinguished by the generation of fucosyltransferase 2 (Fut2) in mice (64). The major source of AMPs is the Fut2+ PCs mainly in ileum, rather than the Fut2- PCs in duodenum, and Fut2+ PCs exhibit the higher granularity and structural complexity (64). PC-derived AMPs mainly include α-defensins (cryptdins in mice), lysozyme, secreted phospholipase A2 (sPLA2), and regenerating islet-derived 3α (REG3α, REG3γ in mice) (38). These AMPs exhibit similar bactericidal ability through bacterial binding and membrane perforation (65).
α-defensins account for 70% in all the AMPs secreted by PCs (66). Mammalian α-defensins exhibit a length of 30-40 amino acids with 6 cysteine residues forming disulfide bridges (38). Human PCs contain 2 α-defensins, HD5 and HD6, while mice PCs possess 6 cryptdin isoforms, cryptdin-1 to -6 (67). PC-specific cryptdin-1 and cryptdin-6 are transcriptionally regulated by β-catenin/Tcf4 signaling, and Frizzled-5 conditional deletion in the intestinal epithelia abolishes the gene expression (49). The mouse model with PC ablation mediated by dithizone treatment or gene knockout exhibits the disorders in intestinal microbiota and the higher susceptibility to bacterial infections, compared to control mice (25, 33, 39). In addition, α-defensin misfolding in abnormal PCs triggers intestinal inflammation and instability of intestinal microbiota (68). Defa1 administration or adenovirus-induced HD5 expression rescues mice with PC defects from severe intestinal injury (39, 69). These studies highlight the important role of α-defensins secreted by PCs in the intestinal homeostasis maintenance.
Additionally, α-defensins do not display antibacterial ability until they are cleaved into their active forms after secretion, and this cleavage process is performed by trypsin in human and matrix metalloproteinase-7 (Mmp7) in mice (70, 71). Mmp7 knockout mice display the alterations in the intestinal microbial community and develop more severe inflammation after infections (70, 72), suggesting the necessity of α-defensin maturation in the intestine. Moreover, the presence of high-level zinc (a cofactor of Mmp7) in PC granules is necessary for Mmp7 activity and AMP stabilization (70, 73, 74). Since zinc transporter 2 facilitates zinc import into PC granules, the ablation of this zinc transporter impairs PC functions and AMP secretion (75). Zinc supplementation alleviates PC necroptosis and intestinal microbiota disorder induced by TNF treatment in mice (76).
Another AMP is lysozyme, a widespread used PC marker in mice, and lysozyme is responsible for peptidoglycan hydrolyzation in the bacterial cell walls (38). There are two types of lysozyme in mice, Lyz1 (expressed by PCs)-encoded lysozyme type P and Lyz2 (expressed by macrophages)-encoded lysozyme type M (38). In human, lysozyme is not unique to PCs, and it is also present in BEST4 cells and follicle associated epithelium (FAE), as demonstrated by single-cell transcriptomic analyses involving duodenum, jejunum, ileum, and colon from three humans (77). Unexpectedly, Lyz1 deficiency has been reported to result in a type 2 immune response to protect mice from DSS (dextran sodium sulfate)-induced colitis via IL-13-IL-4Ra-Stat6 axis (78). Lysozyme-processed and non-processed Ruminococcus gnavus (a lysozyme-sensitive bacterium) induce distinct immune responses in mice, indicating that lysozyme regulates the pro-inflammatory or anti-inflammatory effects of lysozyme-sensitive bacteria (78).
PCs have been confirmed to sense intestinal bacteria directly via cell-autonomous MyD88-dependent toll-like receptor (TLR) activation to induce the expression of AMPs (Figure 2) (79). Toll-IL-1 receptor domain-containing adaptor molecule 1 (TICAM1, also known as TRIF), another downstream protein of TLR, also increases AMP expression under homeostatic condition (80). These findings indicate that the TLR/MyD88 and TLR/TRIF signalings in PCs may explain why specific pathogen-free mice possess more PCs than germ-free mice (81). Nucleotide binding oligomerization domain containing 2 (NOD2), another pattern recognition receptor, also promotes AMP expression (Figure 2). In Caco-2 cells and the ileum of Crohn’s disease patients, NOD2 disruption decreases the gene expression of α-defensin, but not that of lysozyme (82, 83). However, the secretion of lysozyme is affected by NOD2. Lysozyme sorting is a lysozyme-specific process facilitating its secretion (84). After sensing intestinal bacteria, NOD2 promotes lysozyme sorting by recruiting LRRK2, RAB2A, and RIP2 (84, 85). HD5 overexpressing in NOD2-knockout mice can effectively enhance the bactericidal activity and reduce the susceptibility to Helicobacter hepaticus infection (86).
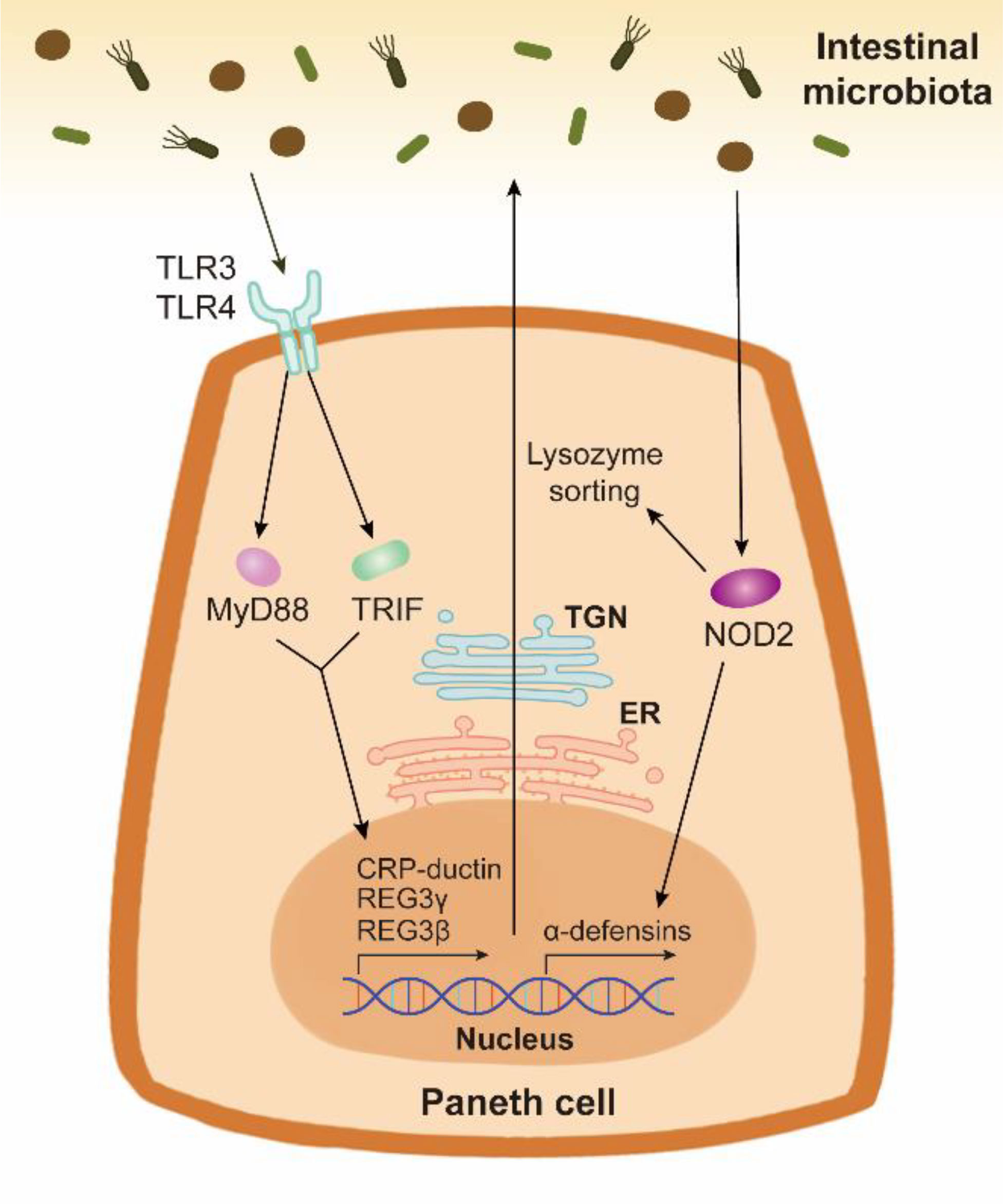
Figure 2 The AMP expression and secretion stimulated by the intestinal bacteria in Paneth cells. Paneth cells can sense the intestinal bacteria through pattern recognition receptors TLR and NOD2. TLR/MyD88 signaling and TLR/TRIF signaling activate the expression and release of AMPs such as CRP-ductin, REG3γ, and REG3β. Bacterium-stimulated NOD2 activation in Paneth cells contributes to the expression of α-defensins. The synthesis and packing of AMPs are respectively mediated by ER and TGN in Paneth cells. NOD2 also promotes lysozyme sorting, thus facilitating the release of lysozyme.
PC degranulation is a key event mediating AMP secretion, which can be induced by cholinergic agonists, bacteria, bacterial cell surface antigens, and cytokines such as IL-17, IL-22 and interferon (IFN)-γ (64, 66, 87, 88). Notably, in the intestinal organoids, PC degranulation can be induced by apical exposure (via microinjection) of lipopolysaccharide (LPS) or Salmonella typhimurium, but not by LPS in culture media (87). PC degranulation is usually accompanied by the increased cytosolic Ca2+ level (66, 89). After degranulation, PCs rapidly replenish their granules in response to subsequent stimulation (87).
ISC niche support
As mentioned above, PCs are derived from ISCs through multiple regulation. Actually, PCs also serve as a supporter in ISC niche. Using individual leucine rich repeat containing G protein-coupled receptor 5 (Lgr5) stem cells, Sato et al. (34) established the small intestinal organoids containing PCs but not mesenchymal niche. The close physiological relationship between PCs and ISCs is demonstrated by co-culturing PCs and Lgr5 stem cells. The existence of PCs significantly enhances the formation efficiency of organoids (90). In vivo study has indicated that PC loss leads to a decrease in the expression of ISC marker olfactomedin 4 (Olfm4) (29). The fact that colonic organoid establishment requires the supplementation of Wnt3a into medium further confirms ISC-supporting role of PCs (91). cKit, as a tyrosine kinase receptor, labels PCs rather than ISCs in the small intestine (92). A subset of colonic goblet cells marked by cKit are adjacent to Lgr5 stem cells. Blocking Notch signaling induces the increase in cKit+ cell number in colon of mice, which is consistent with the above-mentioned observation of PCs in ileum (92). The co-culture of cKit+ cells and colonic Lgr5 stem cells promotes organoid formation ex vivo, suggesting a similar ISC-supporting role of cKit+ cells in colon (92). Stem cell factor (SCF), a ligand of cKit, is highly expressed in the small intestinal crypts under DSS treatment, and after the activation of SCF/cKit signaling in PCs, the co-culture of PCs and ISCs further enhances ISC functions (93). Numerous studies have demonstrated that IL-22 also improves ISC functions in both mice and organoids (94). IL-22Ra1 signaling in PCs is required for organoid growth under IL-22 treatment, suggesting that the beneficial effect of IL-22 on ISCs depends on PCs (33). In contrast, a recent study of the optimized human intestinal organoids has shown that IL-22 inhibits the growth of human intestinal organoids and boosts PC number and functions (95), which indicates that IL-22 signaling should be cautiously controlled. These findings imply that PCs facilitate the function of ISCs under both homeostasis and injury conditions.
The ISC niche is comprised of epithelial niche and mesenchymal niche (2). Gene expression profiling reveals that epithelial niche characterized by PCs provides Wnt3, Dll4, epidermal growth factor (EGF), TGF-α, and R-spondin1 for ISC niche (90). Notably, the ISC-supporting role of PCs might be different in murine and human since the first emergence of PCs in murine is at 7-10 day after birth and that in human is before birth. The formation of intestinal organoids at the absence of mesenchymal niche highlights the importance of epithelial niche. Pericryptal stromal cells are major sources of Wnt2b, Wnt5a, EGF, and R-spondin3 in mesenchymal niche, and enteric glia cells secrete proEGF (EGF precursor form) to promote the intestinal regeneration (Figure 3) (96–100).
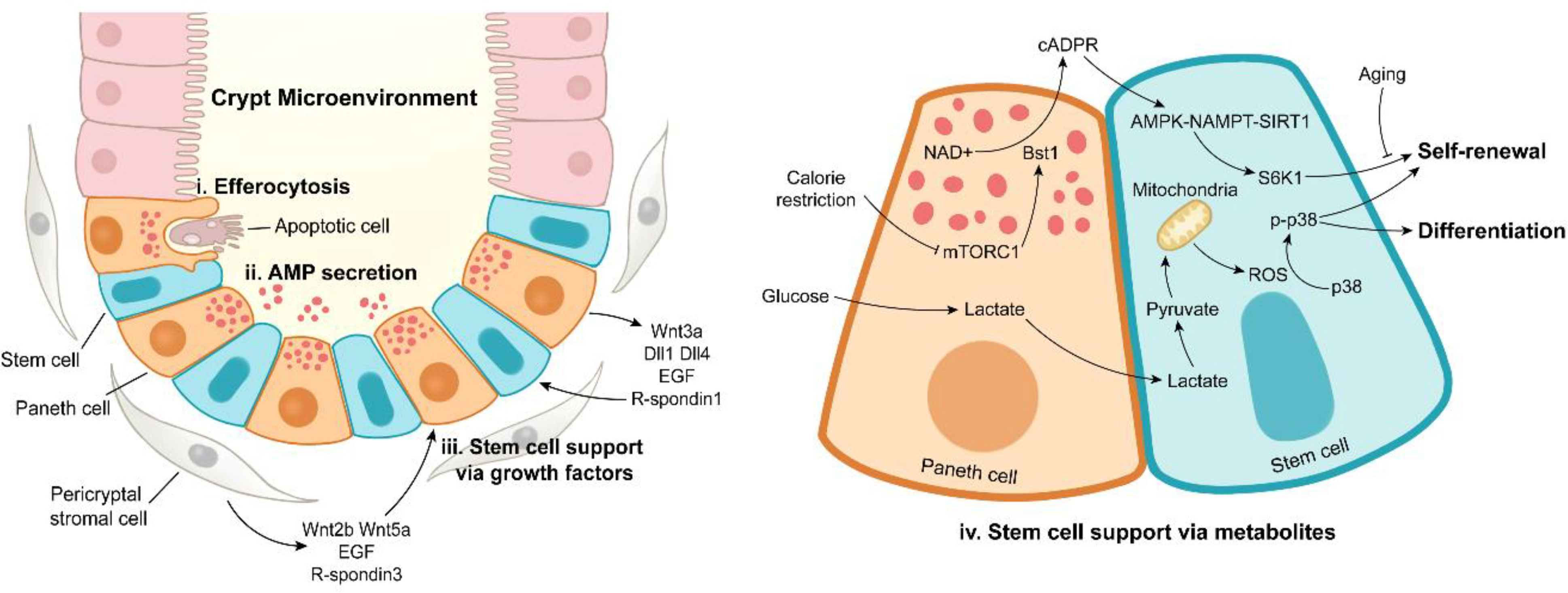
Figure 3 Paneth cell functions under intestinal homeostasis. Paneth cells maintain the stabilization of crypt microenvironment via efferocytosis of apoptotic cells (i), antimicrobial peptide secretion (ii), stem cell support by releasing growth factors (iii) or metabolites (iv). In addition to Paneth cells, pericryptal stromal cells are another important source of growth factors.
ISC markers Lgr5 and Ascl2 are target genes of Wnt signaling (101, 102). The transfer of Wnt signaling needs direct contact between PCs and ISCs through the membrane receptors composed of frizzled protein and low-density lipoprotein receptor-related protein 5/6 (LRP5/6) (103). The aged PCs exhibit the weakened ISC-supporting role due to the deficient Wnt3 supply (104) and the excessive release of Notum (105), an extracellular Wnt inhibitor. Although both PCs and mesenchymal components provide Wnt ligands, the two sources of Wnt signalings are redundant for ISC functions (106). Wnt3 deficiency has no effect on ISC maintenance in mice, but Wnt3 is required for organoid formation, implying a compensatory role of mesenchymal niche for ISC functions (106). In addition, the disruption of Wnt ligands secreted by PCs and myofibroblasts had no influence on ISC functions, suggesting the complexity of the ISC regulation (107). As an enhancer of Wnt signaling, R-spondin directly acts on Lgr5 to boost Wnt signaling activity, and it is necessary for Wnt ligand-mediated ISC maintenance (108). R-spondin3 has a more powerful effect on Wnt activation and organoid growth than R-spondin1 (98).
In addition to Wnt signaling, Notch signaling also plays a positive role in ISC niche. Notch inactivation leads to a reduction in Lgr5 stem cell number and Olfm4 expression level (a Notch-regulated gene, another ISC marker) (42, 46). Notch ligands are transferred only to adjacent cells via cell-cell contact, and the transfer condition of Notch ligands is more strict than that of Wnt ligands (2). The expression of Dll4 is confirmed in PCs, whereas that of Dll1 is still under controversy. Mice with lacZ inserted at the Dll1 locus exhibit β-galactosidase expression in the small intestinal lysozyme positive cells (45). By contrast, Dll1/lysozyme double fluorescent staining reveals that there is no Dll1 expression in lysozyme positive cells (109). In addition, high-level radical fringe proteins in PCs can increase Notch ligands on PC surface, thus elevating the number of adjacent Lgr5 stem cells (110). These findings further confirm that PCs promote ISC maintenance via Notch signaling. Notably, after PC ablation, enteroendocrine and tuft cells emerge at crypt bottoms, thus promoting ISC stabilization by providing Notch ligands, suggesting the flexibility of intestinal epithelium for ISC maintenance (111).
EGF signaling is responsible for regulating cell proliferation rather than ISC maintenance through EGF receptor (EGFR) highly expressed in ISCs (112). In ISC niche, EGF is secreted by PCs, enteric glia cells, and pericryptal stromal cells (via extracellular vesicles) (90, 96, 100). EGFR deletion leads to cell-cycle exit by disturbing MAPK/ERK signaling in organoids (113). Apart from MAPK/ERK signaling, PI3K/AKT signaling (a downstream signaling of EGFR) is also down-regulated after EGFR inhibition (113), but its role in ISC support requires further investigation. However, EGF signaling must be controlled discreetly, since ablation of leucine rich repeats and immunoglobulin like domains 1 (Lrig1), an ISC marker and negative regulator of EGFR, induces ISC expansion or even duodenal adenomas and superficially invasive carcinomas in mice (112, 114).
The ISC-supporting role of PCs is also achieved by metabolic regulation (Figure 3). Calorie restriction inhibits the activity of mammalian target of rapamycin complex 1 (mTORC1) to induce the expression of bone stromal antigen 1 (Bst1) converting NAD+ into cyclic ADP ribose (cADPR) in PCs (115). Once released, PC-derived cADPR paracrinically acts on ISCs and dramatically enhances their functions (115). A subsequent study has reported that calorie restriction-induced cADPR in PCs activates AMPK-Nampt-Sirt1 axis in ISCs to deacetylate S6K1, thus enhancing phosphorylation of S6K1 by mTORC1, eventually boosting ISC functions (116). Surprisingly, aging eliminates the calorie restriction-induced expansion of ISCs, which is associated with decreased number of PCs (117). In PCs, glycolysis provides lactate for ISCs, and the latter converts lactate into pyruvate, thus triggering mitochondrial oxidative phosphorylation (OXPHOS). OXPHOS-derived reactive oxygen species (ROS) facilitates p38 phosphorylation, thereby enhancing ISC function and differentiation (118). These studies emphasize the metabolic support effect of PCs on ISCs, and the metabolic interaction between PCs and ISCs may be an interesting topic to be further explored.
Crypt microenvironment stabilization
It is generally accepted that the intestinal crypt is a sterile microenvironment. The intestinal epithelium is covered with a chemical layer mainly consisting of mucus (from goblet cells) and AMPs (primarily from PCs) (1). Although mucus is penetrable to bacteria, mucus in combination with AMPs maintains the sterility of intestinal crypts (119), suggesting the importance of PCs for crypt microenvironment stabilization. PC disruption potentially results in bacterial colonization in crypts or even bacterial penetration into mucosa in the intestine. Gene ablation such as X-linked inhibitor of apoptosis protein (XIAP), autophagy-related gene 7 (ATG7), and caspase-8 leads to the loss and abnormality of PCs as well as subsequent bacterial colonization in crypts (39, 120–123). Under the condition of unimpaired intestinal epithelium, PC disruption exacerbates the bacterial translocation to mesenteric lymph nodes (MLN) and other sites such spleen and liver (79, 124). These findings suggest that crypts are in a contaminated state under PC disruption.
The bacterial colonization in crypts may trigger ISC apoptosis. Chemotherapy such as 5-fluorouracil and doxorubicin (Dox) treatments can induce intestinal bacterial translocation and the apoptosis of PCs and ISCs in mice (125). Antimicrobial administration rescues Dox-induced ISC loss (126), implying that sterile crypts protect ISCs from excessive apoptosis. Based on these findings, it could be speculated that PC disruption-mediated bacterial colonization in crypts might bring about ISC apoptosis and loss. This speculation is confirmed by one report that ATG7 knockout causes PC dysfunction and bacterial colonization in crypts, thus potentially inducing ISC apoptosis in mice, while ATG7 knockout has no effect on organoid growth ex vivo (127). Antimicrobial treatment prevents ISC loss in ATG7 knockout mice (127), further demonstrating the indispensable role of PCs in crypt microenvironment stabilization.
The intestinal epithelium possesses high turnover rate with a large number of apoptotic cells rapidly supplemented to sustain optimal barrier and absorptive functions (1). CD95, a member of TNF receptor family, is expressed on the basolateral surface of intestinal epithelial cells (IECs), and it can trigger cell apoptosis in the intestine (128). PCs secrete CD95 ligand to drive apoptosis of IECs, suggesting the potential role of PCs in regulating epithelial integrity (129). The apoptotic cells at villus tip are shed into the intestinal lumen (130), and other apoptotic cells on the intestinal epithelium are engulfed by efficient phagocytes (macrophages and dendritic cells) and inefficient phagocytes (IECs) (131, 132). The accumulation of excessive apoptotic cells engenders a pro-inflammatory microenvironment (133, 134). Recently, an unanticipated phagocytic role of PCs has been reported (Figure 3) (21). PCs mediate the uptake of neighboring apoptotic cells in vivo and ex vivo, and PC deletion leads to apoptotic cell increase and efferocytosis disappearance in crypts under homeostasis and irradiation conditions (21), suggesting a novel apoptotic cell phagocytic function by PCs, which needs to be further explored.
End of PC life: Death and dedifferentiation
PCs possess prolonged lifespan, relative to enterocytes, and they undergo spontaneous cell death in the small intestinal crypts (Figure 4) (135). After approximately one-month hard work, PCs end their lives via apoptosis which represents an immunologically silent form of programmed cell death (PCD), as demonstrated by the abundant expression of pro-apoptotic protein ARTS (apoptosis-related protein in the TGF-β signaling pathway) in PCs (136). ARTS deficiency prevents apoptosis of PCs and increases the number of PCs (136). In addition, PC apoptosis can be accelerated in some cases. Gene ablation of XIAP and XBP1 (X-box-binding protein 1) and ischemia-reperfusion cause apoptotic PC loss (39, 124, 137). PC disruption in DTX-treated PC-DTR mice leads to robust apoptosis of PCs (24). However, dithizone selectively induces the degeneration to destroy PCs without triggering inflammation (22).
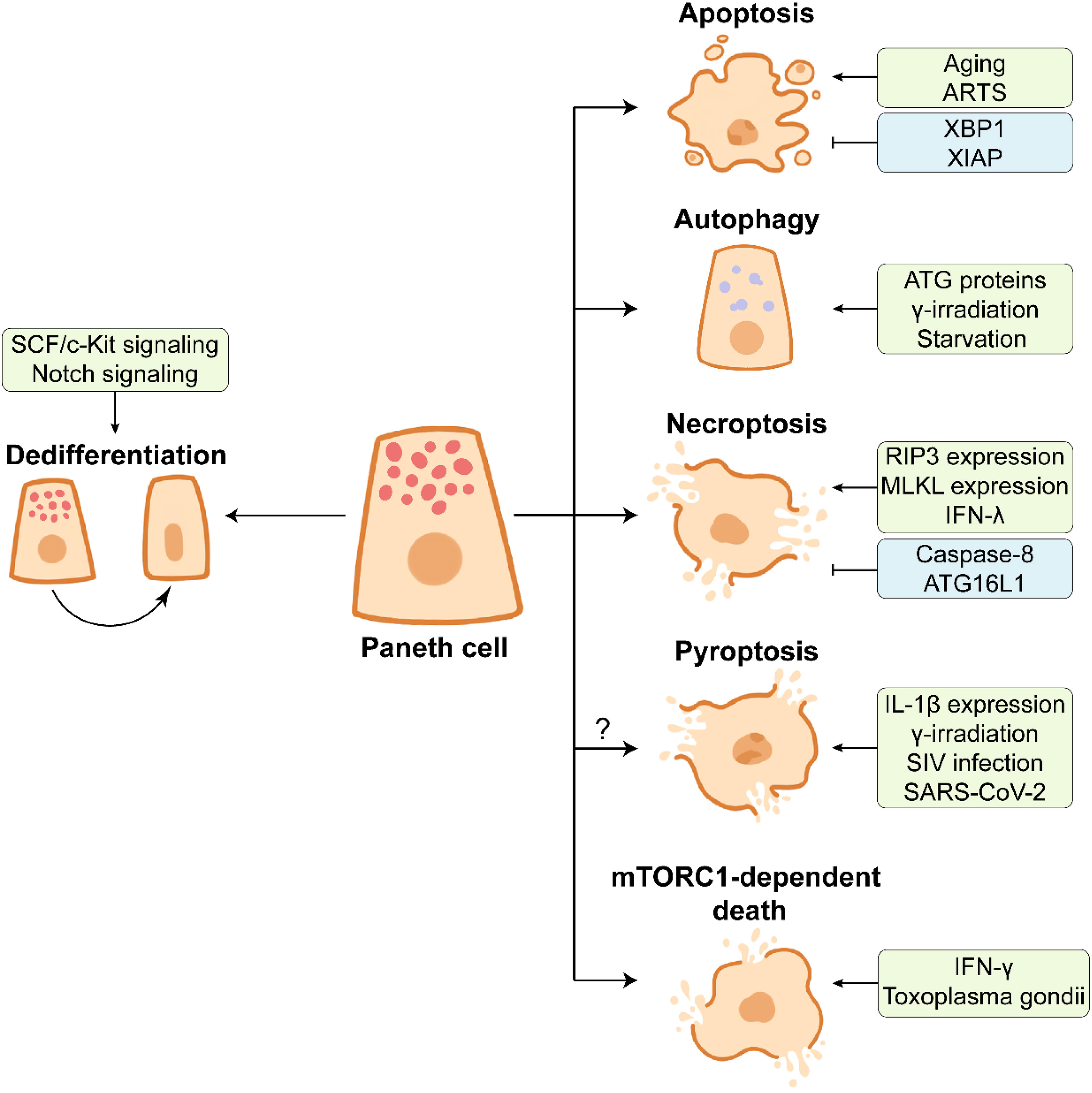
Figure 4 The end of Paneth cell life. Paneth cells dedifferentiate into stem-like cells by activating SCF/c-Kit signaling or Notch signaling in response to the intestinal injury, which facilitates intestinal proliferation and repair. Aging or ARTS-expressing Paneth cells undergo apoptosis under the intestinal homeostasis. XBP1 and XIAP have opposite effects on apoptosis of Paneth cells. Paneth cells can remove the damaged organelles and proteins by autophagy, which contributes to the maintenance of cellular homeostasis. ATG proteins, γ-irradiation, and starvation are associated with the activation of autophagy in Paneth cells. The necroptosis of Paneth cells is frequently observed in the inflamed small intestine. The necroptosis of Paneth cells is promoted by highly expressed RIP3 and MLKL and pro-inflammatory cytokine IFN-λ, and it is inhibited by caspase-8 and ATG16L1. Multiple factors such as high IL-1β expression in Paneth cells, γ-irradiation, SIV infection, and SARS-CoV-2 infection may trigger the pyroptosis of Paneth cells. In Toxoplasma gondii infection, IFN-γ induces mTORC1-dependent death of Paneth cells.
Considering that PCs possess a prolonged lifespan in the intestine, autophagy is essential for the removal of the broken organelles and redundant proteins from PCs (9). γ-irradiation and starvation treatments significantly activate autophagy of PCs and up-regulate the expression of LC3 II (138–140). ATG family proteins play an important role in PC autophagy. The abnormality of ATG family proteins such as autophagy related 16 like 1 (ATG16L1) and ATG7 leads to the defects in AMPs expression in PCs from mice and Crohn’s disease patients (31, 141, 142).
Necroptosis is a pro-inflammatory type of PCD, accompanied by the release of immuno-stimulating DAMPs (damage-associated molecular patterns) such as mtDNA and ATP (143). In addition to apoptosis, necroptosis frequently occurs in PCs under inflammatory conditions. PCs from human and mouse exhibit an abundance of receptor-interacting protein 3 (RIP3), a key regulator of necroptosis, which renders PCs susceptible to necroptosis (120). Crohn’s disease and mouse intestinal inflammation are closely associated with strong necroptosis of PCs (120, 144), and PC necroptosis is further associated with gene ablation and cytokines. Caspase-8 deletion leads to necroptotic PC loss (120). ATG16L1 deficiency-induced disruption of mitochondrial homeostasis triggers PC necroptosis in response to TNF-α challenge (145). In addition, pro-inflammatory cytokine IFN-λ induces PC necroptosis via caspase-8/mixed lineage kinase domain-like (MLKL) signaling (146). The elevated level of IFN-γ induced by Toxoplasma gondii infection results in PC death which is mTORC1-dependent and different from canonical PCD (147). Necroptotic PC loss can be rescued by RIP3 knockout or Necrostatin-1 (an inhibitor of necroptosis) administration (39, 148). Whether PC necroptosis is associated with the intestinal inflammation still remains to be further investigated.
Pyroptosis, another form of PCD, may also occur during the end of PC life. Pyroptosis is mediated by caspase-1 protease promoting IL-1β maturation (143). The expression of IL-1β is much higher in PCs than in lamina propria under intestinal homeostasis (149). The activation of caspase-1 and IL-1β as well as the release of IL-1β are observed in PCs at day 7 post γ-irradiation, suggesting the potential pyroptosis in PCs (138). A large amount of IL-1β is produced by PCs before the type i IFN response, thus impairing epithelial barrier in early simian immunodeficiency virus (SIV) infection (149). It should be noted that the expression of NLRP3, a key component of NLRP3 inflammasome facilitating pyroptosis, is increased in PCs from SARS-CoV-2-infected rhesus macaques (150). More research needs to be conducted so as to understand the importance of PC pyroptosis in the intestine.
As mentioned above, PCs are differentiated from ISCs through a series of lineage commitments, and PCs can dedifferentiate into multipotent stem cells in some injury cases (Figure 4). In the DSS-induced intestinal inflammation, the differentiated and post-mitotic PCs dedifferentiate into stem-like cells, thus promoting tissue regenerative response by activating the SCF/cKit signaling and its downstream PI3K/Akt and Wnt signalings (93). The functions of Notch signaling in PC maintenance has been discussed above, and another function of Notch signaling in PCs is to facilitate PC dedifferentiation. Notch signaling activation drives Defa4-labelled PCs to dedifferentiate into ISCs, which benefits the intestinal regeneration in Dox-induced injury model (151). Irradiation activates Notch signaling to endow PCs with stem cell features (152). Surprisingly, the intact intestinal organoids are established based on the isolated PCs with stem cell features, implying the plasticity of dedifferentiated PCs (152). These findings suggest that the end of PC life is a complicated process which is affected by many factors.
Conclusion
With the increasing attention to the multiple functions of PCs, a brief summary of materials and methods used for PC study is badly needed. PCs are devoted to maintaining the intestinal homeostasis during their entire lives. PC, a long-lived intestinal epithelial cell, is differentiated from adjacent Lgr5 positive ISCs, and it migrates downward to the bottom of crypts after maturation. The presence of abundant granules in the cytoplasm is the most striking feature of PCs, which is used to identify the presence of PCs in the intestine. Although it has been 150 years (1872–2022) since the first identification of PCs, the multiple functions of PCs in the intestine are not completely understood. In recent years, the novel functions of PCs have been continuously reported. In the intestine, PCs endow the intestinal crypts with sterile environment by secreting AMPs, and they support ISC functions by providing growth factors and metabolites. Apoptotic cells in crypts can be promptly engulfed by PCs, thus preventing inflammation. At the end of life, PCs undergo canonical PCD and heroic dedifferentiation. Considering that PCs are located at the crypt bottom above the lamina propria, it is worth exploring the influence of pro-inflammatory PC death on the intestinal inflammation in future work.
Author contributions
CC (conceptualization: lead; writing - original draft: lead; visualization: supporting; writing - review & editing: supporting). FW (visualization: lead). YZ (writing - original draft: supporting). HW (writing - review & editing: supporting). JP (funding acquisition: lead; writing - original draft: supporting; writing - review & editing: lead). All authors contributed to the article and approved the submitted version.
Funding
This work was financially supported by the Joint Funds of the National Natural Science Foundation of China (U22A20511) and China Agriculture Research System (CARS-36).
Conflict of interest
The authors declare that the research was conducted in the absence of any commercial or financial relationships that could be construed as a potential conflict of interest.
Publisher’s note
All claims expressed in this article are solely those of the authors and do not necessarily represent those of their affiliated organizations, or those of the publisher, the editors and the reviewers. Any product that may be evaluated in this article, or claim that may be made by its manufacturer, is not guaranteed or endorsed by the publisher.
References
1. Allaire JM, Crowley SM, Law HT, Chang S-Y, Ko H-J, Vallance BA. The intestinal epithelium: Central coordinator of mucosal immunity. Trends Immunol (2018) 39(9):677–96. doi: 10.1016/j.it.2018.04.002
2. Beumer J, Clevers H. Cell fate specification and differentiation in the adult mammalian intestine. Nat Rev Mol Cell Biol (2021) 22(1):39–53. doi: 10.1038/s41580-020-0278-0
3. Spence JR, Lauf R, Shroyer NF. Vertebrate intestinal endoderm development. Dev Dynamics (2011) 240(3):501–20. doi: 10.1002/dvdy.22540
4. Schwalbe G. Beiträge zur kenntniss der drüsen in den darmwandungen, in’s besondere der brunner’schen drüsen. Archiv für mikroskopische Anatomie (1872) 8(1):92–140. doi: 10.1007/BF02955835
5. Paneth J. Ueber die secernirenden zellen des dünndarm-epithels. Archiv für mikroskopische Anatomie (1887) 31(1):113–91. doi: 10.1007/BF02955706
6. Porter EM, Bevins CL, Ghosh D, Ganz T. The multifaceted paneth cell. Cell And Mol Life Sci (2002) 59(1):156–70. doi: 10.1007/s00018-002-8412-z
7. Karlsson J, Putsep K, Chu H, Kays RJ, Bevins CL, Andersson M. Regional variations in paneth cell antimicrobial peptide expression along the mouse intestinal tract. BMC Immunol (2008) 9:37. doi: 10.1186/1471-2172-9-37
8. Singh R, Balasubramanian I, Zhang L, Gao N. Metaplastic paneth cells in extra-intestinal mucosal niche indicate a link to microbiome and inflammation. Front In Physiol (2020) 11:280. doi: 10.3389/fphys.2020.00280
9. Clevers HC, Bevins CL. Paneth cells: Maestros of the small intestinal crypts. Annu Rev Of Physiol (2013) 75:289–311. doi: 10.1146/annurev-physiol-030212-183744
10. Bruhn O, Groetzinger J, Cascorbi I, Jung S. Antimicrobial peptides and proteins of the horse - insights into a well-armed organism. Vet Res (2011) 42:98. doi: 10.1186/1297-9716-42-98
11. Wu A, Yu B, Zhang K, Xu Z, Wu D, He J, et al. Transmissible gastroenteritis virus targets paneth cells to inhibit the self-renewal and differentiation of Lgr5 intestinal stem cells Via notch signaling. Cell Death Dis (2020) 11(1):40. doi: 10.1038/s41419-020-2233-6
12. Lueschow SR, McElroy SJ. The paneth cell: The curator and defender of the immature small intestine. Front Immunol (2020) 11:587. doi: 10.3389/fimmu.2020.00587
13. Rumbo M, Schiffrin EJ. Ontogeny of intestinal epithelium immune functions: Developmental and environmental regulation. Cell And Mol Life Sci (2005) 62(12):1288–96. doi: 10.1007/s00018-005-5033-3
14. Stanford AH, Gong H, Noonan M, Lewis AN, Gong Q, Lanik WE, et al. A direct comparison of mouse and human intestinal development using epithelial gene expression patterns. Pediatr Res (2020) 88(1):66–76. doi: 10.1038/s41390-019-0472-y
15. Hansen GH, Rasmussen K, Niels-Christiansen L-L, Danielsen EM. Lipopolysaccharide-binding protein: Localization in secretory granules of paneth cells in the mouse small intestine. Histochem And Cell Biol (2009) 131(6):727–32. doi: 10.1007/s00418-009-0572-6
16. Han SJ, Kim M, D'Agati VD, Lee HT. Norepinephrine released by intestinal paneth cells exacerbates ischemic aki. Am J Of Physiology-Renal Physiol (2020) 318(1):F260–F72. doi: 10.1152/ajprenal.00471.2019
17. Deckx RJ, Vantrappen GR, Parein MM. Localization of lysozyme activity in a paneth cell granule fraction. Biochim Et Biophys Acta (1967) 139(1):204–7. doi: 10.1016/0005-2744(67)90136-2
18. Verdile N, Mirmahmoudi R, Brevini TAL, Gandolfi F. Evolution of pig intestinal stem cells from birth to weaning. Animal (2019) 13(12):2830–9. doi: 10.1017/s1751731119001319
19. Gulati AS, Shanahan MT, Arthur JC, Grossniklaus E, von Furstenberg RJ, Kreuk L, et al. Mouse background strain profoundly influences paneth cell function and intestinal microbial composition. PloS One (2012) 7(2):e32403. doi: 10.1371/journal.pone.0032403
20. White JR, Gong H, Pope B, Schlievert P, McElroy SJ. Paneth-Cell-Disruption-Induced necrotizing enterocolitis in mice requires live bacteria and occurs independently of Tlr4 signaling. Dis Models Mech (2017) 10(6):727–36. doi: 10.1242/dmm.028589
21. Shankman LS, Fleury ST, Evans WB, Penberthy KK, Arandjelovic S, Blumberg RS, et al. Efferocytosis by paneth cells within the intestine. Curr Biol (2021) 31(11):2469–76. doi: 10.1016/j.cub.2021.03.055
22. Sawada M, Takahashi K, Sawada S, Midorikawa O. Selective killing of paneth cells by intravenous administration of dithizone in rats. Int J Of Exp Pathol (1991) 72(4):407–21.
23. Seno H, Sawada M, Fukuzawa H, Morita Y, Takaishi S, Hiai H, et al. Enhanced expression of transforming growth factor (Tgf) -alpha precursor and tgf-Beta(1) during paneth cell regeneration. Digestive Dis Sci (2001) 46(5):1004–10. doi: 10.1023/a:1010797609041
24. Garabedian EM, Roberts LJJ, McNevin MS, Gordon JI. Examining the role of paneth cells in the small intestine by lineage ablation in transgenic mice. J Of Biol Chem (1997) 272(38):23729–40. doi: 10.1074/jbc.272.38.23729
25. Lueschow SR, Stumphy J, Gong H, Kern SL, Elgin TG, Underwood MA, et al. Loss of murine paneth cell function alters the immature intestinal microbiome and mimics changes seen in neonatal necrotizing enterocolitis. PloS One (2018) 13(10):e0204967. doi: 10.1371/journal.pone.0204967
26. Seno H, Sawada M, Fukuzawa H, Morita-Fujisawa Y, Takaishi S, Hiai H, et al. Involvement of tumor necrosis factor alpha in intestinal epithelial cell proliferation following paneth cell destruction. Scand J Of Gastroenterol (2002) 37(2):154–60. doi: 10.1080/003655202753416803
27. Berger JN, Gong H, Good M, McElroy SJ. Dithizone-induced paneth cell disruption significantly decreases intestinal perfusion in the murine small intestine. J Of Pediatr Surg (2019) 54(11):2402–7. doi: 10.1016/j.jpedsurg.2019.02.021
28. Durand A, Donahue B, Peignon G, Letourneur F, Cagnard N, Slomianny C, et al. Functional intestinal stem cells after paneth cell ablation induced by the loss of transcription factor Math1 (Atoh1). Proceedings of the National Academy of Sciences of the United States of America (2012) 109(23):8965–70. doi: 10.1073/pnas.1201652109
29. Mori-Akiyama Y, Van den Born M, Van Es JH, Hamilton SR, Adams HP, Zhang J, et al. Sox9 is required for the differentiation of paneth cells in the intestinal epithelium. Gastroenterology (2007) 133(2):539–46. doi: 10.1053/j.gastro.2007.05.020
30. Shroyer NF, Wallis D, Venken KJT, Bellen HJ, Zoghbi HY. Gfi1 functions downstream of Math1 to control intestinal secretory cell subtype allocation and differentiation. Genes Dev (2005) 19(20):2412–7. doi: 10.1101/gad.1353905
31. Adolph TE, Tomczak MF, Niederreiter L, Ko HJ, Böck J, Martinez-Naves E, et al. Paneth cells as a site of origin for intestinal inflammation. Nature (2013) 503(7475):272–6. doi: 10.1038/nature12599
32. Lu R, Y-g Z, Xia Y, Zhang J, Kaser A, Blumberg R, et al. Paneth cell alertness to pathogens maintained by vitamin d receptors. Gastroenterology (2021) 160(4):1269–83. doi: 10.1053/j.gastro.2020.11.015
33. Gaudino SJ, Beaupre M, Lin X, Joshi P, Rathi S, McLaughlin PA, et al. Il-22 receptor signaling in paneth cells is critical for their maturation, microbiota colonization, Th17-related immune responses, and anti-salmonellaimmunity. Mucosal Immunol (2021) 14(2):389–401. doi: 10.1038/s41385-020-00348-5
34. Sato T, Vries RG, Snippert HJ, van de Wetering M, Barker N, Stange DE, et al. Single Lgr5 stem cells build crypt-villus structures in vitro without a mesenchymal niche. Nature (2009) 459(7244):262–5. doi: 10.1038/nature07935
35. Almeqdadi M, Mana MD, Roper J, Yilmaz OH. Gut organoids: Mini-tissues in culture to study intestinal physiology and disease. Am J Of Physiology-Cell Physiol (2019) 317(3):C405–C19. doi: 10.1152/ajpcell.00300.2017
36. Hou Q, Dong Y, Yu Q, Wang B, Le S, Guo Y, et al. Regulation of the paneth cell niche by exogenousl-arginine couples the intestinal stem cell function. FASEB J (2020) 34(8):10299–315. doi: 10.1096/fj.201902573RR
37. Lin X, Gaudino SJ, Jang KK, Bahadur T, Singh A, Banerjee A, et al. Il-17ra-Signaling in Lgr(5+) intestinal stem cells induces expression of transcription factor Atoh1 to promote secretory cell lineage commitment. Immunity (2022) 55(2):237–53. doi: 10.1016/j.immuni.2021.12.016
38. Bevins CL, Salzman NH. Paneth cells, antimicrobial peptides and maintenance of intestinal homeostasis. Nat Rev Microbiol (2011) 9(5):356–68. doi: 10.1038/nrmicro2546
39. Strigli A, Gopalakrishnan S, Zeissig Y, Basic M, Wang J, Schwerd T, et al. Deficiency in X-linked inhibitor of apoptosis protein promotes susceptibility to microbial triggers of intestinal inflammation. Sci Immunol (2021) 6(65):eabf7473. doi: 10.1126/sciimmunol.abf7473
40. Hodin CM, Verdam FJ, Grootjans J, Rensen SS, Verheyen FK, Dejong CHC, et al. Reduced paneth cell antimicrobial protein levels correlate with activation of the unfolded protein response in the gut of obese individuals. J Of Pathol (2011) 225(2):276–84. doi: 10.1002/path.2917
41. Park SW, Kim M, Brown KM, D'Agati VD, Lee HT. Paneth cell-derived interleukin-17a causes multiorgan dysfunction after hepatic ischemia and reperfusion injury. Hepatology (2011) 53(5):1662–75. doi: 10.1002/hep.24253
42. VanDussen KL, Carulli AJ, Keeley TM, Patel SR, Puthoff BJ, Magness ST, et al. Notch signaling modulates proliferation and differentiation of intestinal crypt base columnar stem cells. Dev (Cambridge England) (2012) 139(3):488–97. doi: 10.1242/dev.070763
43. Bohin N, Keeley TM, Carulli AJ, Walker EM, Carlson EA, Gao J, et al. Rapid crypt cell remodeling regenerates the intestinal stem cell niche after notch inhibition. Stem Cell Rep (2020) 15(1):156–70. doi: 10.1016/j.stemcr.2020.05.010
44. Suzuki K, Fukui H, Kayahara T, Sawada M, Seno H, Hiai H, et al. Hes1-deficient mice show precocious differentiation of paneth cells in the small intestine. Biochem And Biophys Res Commun (2005) 328(1):348–52. doi: 10.1016/j.bbrc.2004.12.174
45. Stamataki D, Holder M, Hodgetts C, Jeffery R, Nye E, Spencer-Dene B, et al. Delta1 expression, cell cycle exit, and commitment to a specific secretory fate coincide within a few hours in the mouse intestinal stem cell system. PloS One (2011) 6(9):e24484. doi: 10.1371/journal.pone.0024484
46. Carulli AJ, Keeley TM, Demitrack ES, Chung J, Maillard I, Samuelson LC. Notch receptor regulation of intestinal stem cell homeostasis and crypt regeneration. Dev Biol (2015) 402(1):98–108. doi: 10.1016/j.ydbio.2015.03.012
47. Pinto D, Gregorieff A, Begthel H, Clevers H. Canonical wnt signals are essential for homeostasis of the intestinal epithelium. Genes Dev (2003) 17(14):1709–13. doi: 10.1101/gad.267103
48. Andreu P, Peignon G, Slomianny C, Taketo MM, Colnot S, Robine S, et al. A genetic study of the role of the Wnt/Beta-catenin signalling in paneth cell differentiation. Dev Biol (2008) 324(2):288–96. doi: 10.1016/j.ydbio.2008.09.027
49. van Es JH, Jay P, Gregorieff A, van Gijn ME, Jonkheer S, Hatzis P, et al. Wnt signalling induces maturation of paneth cells in intestinal crypts. Nat Cell Biol (2005) 7(4):381–6. doi: 10.1038/ncb1240
50. Yang Q, Bermingham NA, Finegold MJ, Zoghbi HY. Requirement of Math1 for secretory cell lineage commitment in the mouse intestine. Science (2001) 294(5549):2155–8. doi: 10.1126/science.1065718
51. Kim T-H, Li F, Ferreiro-Neira I, Ho L-L, Luyten A, Nalapareddy K, et al. Broadly permissive intestinal chromatin underlies lateral inhibition and cell plasticity. Nature (2014) 506(7489):511–5. doi: 10.1038/nature12903
52. Grumolato L, Liu G, Mong P, Mudbhary R, Biswas R, Arroyave R, et al. Canonical and noncanonical wnts use a common mechanism to activate completely unrelated coreceptors. Genes Dev (2010) 24(22):2517–30. doi: 10.1101/gad.1957710
53. Boettcher A, Buettner M, Tritschler S, Sterr M, Aliluev A, Oppenlaender L, et al. Non-canonical Wnt/Pcp signalling regulates intestinal stem cell lineage priming towards enteroendocrine and paneth cell fates. Nat Cell Biol (2021) 23(1):23–31. doi: 10.1038/s41556-020-00617-2
54. Auclair BA, Benoit YD, Rivard N, Mishina Y, Perreault N. Bone morphogenetic protein signaling is essential for terminal differentiation of the intestinal secretory cell lineage. Gastroenterology (2007) 133(3):887–96. doi: 10.1053/j.gastro.2007.06.066
55. de Jong PR, Taniguchi K, Harris AR, Bertin S, Takahashi N, Duong J, et al. Erk5 signalling rescues intestinal epithelial turnover and tumour cell proliferation upon Erk1/2 abrogation. Nat Commun (2016) 7:11551. doi: 10.1038/ncomms11551
56. Heuberger J, Kosel F, Qi J, Grossmann KS, Rajewsky K, Birchmeier W. Shp2/Mapk signaling controls Goblet/Paneth cell fate decisions in the intestine. Proceedings of the National Academy of Sciences of the United States of America (2014) 111(9):3472–7. doi: 10.1073/pnas.1309342111
57. Grinat J, Kosel F, Goveas N, Kranz A, Alexopoulou D, Rajewsky K, et al. Epigenetic modifier balances mapk and wnt signalling in differentiation of goblet and paneth cells. Life Sci alliance (2022) 5(4):1–14. doi: 10.26508/lsa.202101187
58. Baba R, Kokubu K, Nakamura K, Fujita M, Morimoto H. Paneth cell maturation is related to epigenetic modification during neonatal-weaning transition. Histochem And Cell Biol (2022) 158(1):5–13. doi: 10.1007/s00418-022-02110-3
59. Krndija D, El Marjou F, Guirao B, Richon S, Leroy O, Bellaiche Y, et al. Active cell migration is critical for steady-state epithelial turnover in the gut. Science (2019) 365(6454):705–10. doi: 10.1126/science.aau3429
60. Batlle E, Henderson JT, Beghtel H, van den Born MMW, Sancho E, Huls G, et al. Beta-catenin and tcf mediate cell positioning in the intestinal epithelium by controlling the expression of Ephb/Ephrinb. Cell (2002) 111(2):251–63. doi: 10.1016/s0092-8674(02)01015-2
61. Barreto e Barreto L, Rattes IC, da Costa AV, Gama P. Paneth cells and their multiple functions. Cell Biol Int (2022) 46(5):701–10. doi: 10.1002/cbin.11764
62. Wolfs TGAM, Derikx JPM, Hodin CMIM, Vanderlocht J, Driessen A, de Bruine AP, et al. Localization of the lipopolysaccharide recognition complex in the human healthy and inflamed premature and adult gut. Inflammatory Bowel Dis (2010) 16(1):68–75. doi: 10.1002/ibd.20995
63. Dydensborg AB, Teller IC, Basora N, Groulx J-F, Auclair J, Francoeur C, et al. Differential expression of the integrins alpha 6a beta 4 and alpha 6b beta 4 along the crypt-villus axis in the human small intestine. Histochem And Cell Biol (2009) 131(4):531–6. doi: 10.1007/s00418-008-0547-z
64. Kamioka M, Goto Y, Nakamura K, Yokoi Y, Sugimoto R, Ohira S, et al. Intestinal commensal microbiota and cytokines regulate Fut2(+) paneth cells for gut defense. Proceedings of the National Academy of Sciences of the United States of America (2022) 119(3):e211530119. doi: 10.1073/pnas.2115230119
65. Guani-Guerra E, Santos-Mendoza T, Lugo-Reyes SO, Teran LM. Antimicrobial peptides: General overview and clinical implications in human health and disease. Clin Immunol (2010) 135(1):1–11. doi: 10.1016/j.clim.2009.12.004
66. Ayabe T, Satchell DP, Wilson CL, Parks WC, Selsted ME, Ouellette AJ. Secretion of microbicidal alpha-defensins by intestinal paneth cells in response to bacteria. Nat Immunol (2000) 1(2):113–8. doi: 10.1038/77783
67. Nakamura K, Sakuragi N, Takakuwa A, Ayabe T. Paneth cell alpha-defensins and enteric microbiota in health and disease. Bioscience Of Microbiota Food And Health (2016) 35(2):57–67. doi: 10.12938/bmfh.2015-019
68. Shimizu Y, Nakamura K, Yoshii A, Yokoi Y, Kikuchi M, Shinozaki R, et al. Paneth cell alpha-defensin misfolding correlates with dysbiosis and ileitis in crohn's disease model mice. Life Sci alliance (2020) 3(6):e201900592. doi: 10.26508/lsa.201900592
69. Hu X, Deng J, Yu T, Chen S, Ge Y, Zhou Z, et al. Atf4 deficiency promotes intestinal inflammation in mice by reducing uptake of glutamine and expression of antimicrobial peptides. Gastroenterology (2019) 156(4):1098–111. doi: 10.1053/j.gastro.2018.11.033
70. Wilson CL, Ouellette AJ, Satchell DP, Ayabe T, Lopez-Boado YS, Stratman JL, et al. Regulation of intestinal alpha-defensin activation by the metalloproteinase matrilysin in innate host defense. Science (1999) 286(5437):113–7. doi: 10.1126/science.286.5437.113
71. Ghosh D, Porter E, Shen B, Lee SK, Wilk D, Drazba J, et al. Paneth cell trypsin is the processing enzyme for human defensin-5. Nat Immunol (2002) 3(6):583–90. doi: 10.1038/ni797
72. Shim D-H, Ryu S, Kweon M-N. Defensins play a crucial role in protecting mice against oral shigella flexneri infection. Biochem And Biophys Res Commun (2010) 401(4):554–60. doi: 10.1016/j.bbrc.2010.09.100
73. Chakraborti S, Chatterjee T, Joshi P, Poddar A, Bhattacharyya B, Singh SP, et al. Structure and activity of lysozyme on binding to zno nanoparticles. Langmuir (2010) 26(5):3506–13. doi: 10.1021/la903118c
74. Zhang Y, Cougnon FBL, Wanniarachchi YA, Hayden JA, Nolan EM. Reduction of human defensin 5 affords a high-affinity zinc-chelating peptide. ACS Chem Biol (2013) 8(9):1907–11. doi: 10.1021/cb400340k
75. Podany AB, Wright J, Lamendella R, Soybel DI, Kelleher SL. Znt2-mediated zinc import into paneth cell granules is necessary for coordinated secretion and paneth cell functioninmice. Cell Mol Gastroenterol Hepatol (2016) 2(3):369–83. doi: 10.1016/j.jcmgh.2015.12.006
76. Souffriau J, Timmermans S, Vanderhaeghen T, Wallaeys C, Van Looveren K, Aelbrecht L, et al. Zinc inhibits lethal inflammatory shock by preventing microbe-induced interferon signature in intestinal epithelium. EMBO Mol Med (2020) 12(10):e11917. doi: 10.15252/emmm.201911917
77. Burclaff J, Bliton RJ, Breau KA, Ok MT, Gomez-Martinez I, Ranek JS, et al. A proximal-to-Distal survey of healthy adult human small intestine and colon epithelium by single-cell transcriptomics. Cell Mol Gastroenterol Hepatol (2022) 13(5):1554–89. doi: 10.1016/j.jcmgh.2022.02.007
78. Yu S, Balasubramanian I, Laubitz D, Tong K, Bandyopadhyay S, Lin X, et al. Paneth cell-derived lysozyme defines the composition of mucolytic microbiota and the inflammatory tone of the intestine. Immunity (2020) 53(2):398–416. doi: 10.1016/j.immuni.2020.07.010
79. Vaishnava S, Behrendt CL, Ismail AS, Eckmann L, Hooper LV. Paneth cells directly sense gut commensals and maintain homeostasis at the intestinal host-microbial interface. Proceedings of the National Academy of Sciences of the United States of America (2008) 105(52):20858–63. doi: 10.1073/pnas.0808723105
80. Stockinger S, Duerr CU, Fulde M, Dolowschiak T, Pott J, Yang I, et al. Trif signaling drives homeostatic intestinal epithelial antimicrobial peptide expression. J Of Immunol (2014) 193(8):4223–34. doi: 10.4049/jimmunol.1302708
81. Tsang DKL, Wang RJ, De Sa O, Ayyaz A, Foerster EG, Bayer G, et al. A single cell survey of the microbial impacts on the mouse small intestinal epithelium. Gut Microbes (2022) 14(1):2108281. doi: 10.1080/19490976.2022.2108281
82. Tan G, Zeng B, Zhi F-C. Regulation of human enteric alpha-defensins by Nod2 in the paneth cell lineage. Eur J Of Cell Biol (2015) 94(1):60–6. doi: 10.1016/j.ejcb.2014.10.007
83. Wehkamp J, Harder J, Weichenthal M, Schwab M, Schaffeler E, Schlee M, et al. Nod2 (Card15) mutations in crohn's disease are associated with diminished mucosal alpha-defensin expression. Gut (2004) 53(11):1658–64. doi: 10.1136/gut.2003.032805
84. Zhang Q, Pan Y, Yan R, Zeng B, Wang H, Zhang X, et al. Commensal bacteria direct selective cargo sorting to promote symbiosis. Nat Immunol (2015) 16(9):918–26. doi: 10.1038/ni.3233
85. Wang H, Zhang X, Zuo Z, Zhang Q, Pan Y, Zeng B, et al. Rip2 is required for Nod2-mediated lysozyme sorting in paneth cells. J Of Immunol (2017) 198(9):3729–36. doi: 10.4049/jimmunol.1601583
86. Biswas A, Liu Y-J, Hao L, Mizoguchi A, Salzman NH, Bevins CL, et al. Induction and rescue of Nod2-dependent Th1-driven granulomatous inflammation of the ileum. Proceedings of the National Academy of Sciences of the United States of America (2010) 107(33):14739–44. doi: 10.1073/pnas.1003363107
87. Yokoi Y, Nakamura K, Yoneda T, Kikuchi M, Sugimoto R, Shimizu Y, et al. Paneth cell granule dynamics on secretory responses to bacterial stimuli in enteroids. Sci Rep (2019) 9:2710. doi: 10.1038/s41598-019-39610-7
88. Tanabe H, Ayabe T, Bainbridge B, Guina T, Ernst RK, Darveau RP, et al. Mouse paneth cell secretory responses to cell surface glycolipids of virulent and attenuated pathogenic bacteria. Infection And Immun (2005) 73(4):2312–20. doi: 10.1128/iai.73.4.2312-2320.2005
89. Satoh Y, Habara Y, Ono K, Kanno T. Carbamylcholine-induced and catecholamine-induced intracellular calcium dynamics of epithelial-cells in mouse ileal crypts. Gastroenterology (1995) 108(5):1345–56. doi: 10.1016/0016-5085(95)90681-9
90. Sato T, van Es JH, Snippert HJ, Stange DE, Vries RG, van den Born M, et al. Paneth cells constitute the niche for Lgr5 stem cells in intestinal crypts. Nature (2011) 469(7330):415–8. doi: 10.1038/nature09637
91. Sato T, Stange DE, Ferrante M, Vries RGJ, van Es JH, van den Brink S, et al. Long-term expansion of epithelial organoids from human colon, adenoma, adenocarcinoma, and barrett's epithelium. Gastroenterology (2011) 141(5):1762–72. doi: 10.1053/j.gastro.2011.07.050
92. Rothenberg ME, Nusse Y, Kalisky T, Lee JJ, Dalerba P, Scheeren F, et al. Identification of a ckit(+) colonic crypt base secretory cell that supports Lgr5(+) stem cells in mice. Gastroenterology (2012) 142(5):1195–205. doi: 10.1053/j.gastro.2012.02.006
93. Schmitt M, Schewe M, Sacchetti A, Feijtel D, de Geer WSv, Teeuwssen M, et al. Paneth cells respond to inflammation and contribute to tissue regeneration by acquiring stem-like features through Scf/C-kit signaling. Cell Rep (2018) 24(9):2312–28. doi: 10.1016/j.celrep.2018.07.085
94. Keir ME, Yi T, Lu TT, Ghilardi N. The role of il-22 in intestinal health and disease. J Of Exp Med (2020) 217(3):e20192195. doi: 10.1084/jem.20192195
95. He G-W, Lin L, DeMartino J, Zheng X, Staliarova N, Dayton T, et al. Optimized human intestinal organoid model reveals interleukin-22-Dependency of paneth cell formation. Cell Stem Cell (2022) 29(9):1333–45.e6. doi: 10.1016/j.stem.2022.08.002
96. Van Landeghem L, Chevalier J, Mahe MM, Wedel T, Urvil P, Derkinderen P, et al. Enteric glia promote intestinal mucosal healing via activation of focal adhesion kinase and release of proegf. Am J Of Physiology-Gastrointestinal And Liver Physiol (2011) 300(6):G976–G87. doi: 10.1152/ajpgi.00427.2010
97. Shoshkes-Carmel M, Wang YJ, Wangensteen KJ, Toth B, Kondo A, Massassa EE, et al. Subepithelial telocytes are an important source of wnts that supports intestinal crypts. Nature (2018) 557(7704):242–6. doi: 10.1038/s41586-018-0084-4
98. Greicius G, Kabiri Z, Sigmundsson K, Liang C, Bunte R, Singh MK, et al. Pdgfr alpha(+) pericryptal stromal cells are the critical source of wnts and Rspo3 for murine intestinal stem cells in vivo. Proceedings of the National Academy of Sciences of the United States of America (2018) 115(14):E3173–E81. doi: 10.1073/pnas.1713510115
99. Stzepourginski I, Nigro G, Jacob J-M, Dulauroy S, Sansonetti PJ, Eberl G, et al. Cd34(+) mesenchymal cells are a major component of the intestinal stem cells niche at homeostasis and after injury. Proceedings of the National Academy of Sciences of the United States of America (2017) 114(4):E506–E13. doi: 10.1073/pnas.1620059114
100. Oszvald A, Szvicsek Z, Sandor GO, Kelennen A, Soos AA, Paloczi K, et al. Extracellular vesicles transmit epithelial growth factor activity in the intestinal stem cell niche. Stem Cells (2020) 38(2):291–300. doi: 10.1002/stem.3113
101. Barker N, van Es JH, Kuipers J, Kujala P, van den Born M, Cozijnsen M, et al. Identification of stem cells in small intestine and colon by marker gene Lgr5. Nature (2007) 449(7165):1003–7. doi: 10.1038/nature06196
102. van der Flier LG, van Gijn ME, Hatzis P, Kujala P, Haegebarth A, Stange DE, et al. Transcription factor achaete scute-like 2 controls intestinal stem cell fate. Cell (2009) 136(5):903–12. doi: 10.1016/j.cell.2009.01.031
103. Farin HF, Jordens I, Mosa MH, Basak O, Korving J, Tauriello DVF, et al. Visualization of a short-range wnt gradient in the intestinal stem-cell niche. Nature (2016) 530(7590):340–3. doi: 10.1038/nature16937
104. Nalapareddy K, Nattamai KJ, Kumar RS, Karns R, Wikenheiser-Brokamp KA, Sampson LL, et al. Canonical wnt signaling ameliorates aging of intestinal stem cells. Cell Rep (2017) 18(11):2608–21. doi: 10.1016/j.celrep.2017.02.056
105. Pentinmikko N, Iqbal S, Mana M, Andersson S, Cognetta AB III, Suciu RM, et al. Notum produced by paneth cells attenuates regeneration of aged intestinal epithelium. Nature (2019) 571(7765):398–402. doi: 10.1038/s41586-019-1383-0
106. Farin HF, Van Es JH, Clevers H. Redundant sources of wnt regulate intestinal stem cells and promote formation of paneth cells. Gastroenterology (2012) 143(6):1518–29. doi: 10.1053/j.gastro.2012.08.031
107. Shaker A, Swietlicki EA, Wang L, Jiang S, Onal B, Bala S, et al. Epimorphin deletion protects mice from inflammation-induced colon carcinogenesis and alters stem cell niche myofibroblast secretion. J Of Clin Invest (2010) 120(6):2081–93. doi: 10.1172/jci40676
108. de Lau W, Barker N, Low TY, Koo B-K, Li VSW, Teunissen H, et al. Lgr5 homologues associate with wnt receptors and mediate r-spondin signalling. Nature (2011) 476(7360):293–7. doi: 10.1038/nature10337
109. Shimizu H, Okamoto R, Ito G, Fujii S, Nakata T, Suzuki K, et al. Distinct expression patterns of notch ligands, Dll1 and Dll4, in normal and inflamed mice intestine. Peerj (2014) 2:e370. doi: 10.7717/peerj.370
110. Murthy PKL, Srinivasan T, Bochter MS, Xi R, Varanko AK, Tung K-L, et al. Radical and lunatic fringes modulate notch ligands to support mammalian intestinal homeostasis. eLife (2018) 7:e35710. doi: 10.7554/eLife.35710
111. van Es JH, Wiebrands K, Lopez-Iglesias C, de Wetering Mv, Zeinstra L, van den Born M, et al. Enteroendocrine and tuft cells support Lgr5 stem cells on paneth cell depletion. Proceedings of the National Academy of Sciences of the United States of America (2019) 116(52):26599–605. doi: 10.1073/pnas.1801888117
112. Wong VWY, Stange DE, Page ME, Buczacki S, Wabik A, Itami S, et al. Lrig1 controls intestinal stem-cell homeostasis by negative regulation of erbb signalling. Nat Cell Biol (2012) 14(4):401–8. doi: 10.1038/ncb2464
113. Basak O, Beumer J, Wiebrands K, Seno H, van Oudenaarden A, Clevers H. Induced quiescence of Lgr5+Stem cells in intestinal organoids enables differentiation of hormone-producing enteroendocrine cells. Cell Stem Cell (2017) 20(2):177–90. doi: 10.1016/j.stem.2016.11.001
114. Powell AE, Wang Y, Li Y, Poulin EJ, Means AL, Washington MK, et al. The pan-erbb negative regulator Lrig1 is an intestinal stem cell marker that functions as a tumor suppressor. Cell (2012) 149(1):146–58. doi: 10.1016/j.cell.2012.02.042
115. Yilmaz OH, Katajisto P, Lamming DW, Gueltekin Y, Bauer-Rowe KE, Sengupta S, et al. Mtorc1 in the paneth cell niche couples intestinal stem-cell function to calorie intake. Nature (2012) 486(7404):490–5. doi: 10.1038/nature11163
116. Igarashi M, Guarente L. Mtorc1 and Sirt1 cooperate to foster expansion of gut adult stem cells during calorie restriction. Cell (2016) 166(2):436–50. doi: 10.1016/j.cell.2016.05.044
117. Annunziata F, Rasa SMM, Krepelova A, Lu J, Minetti A, Omrani O, et al. Paneth cells drive intestinal stem cell competition and clonality in aging and calorie restriction. Eur J Cell Biol (2022) 101(4):151282. doi: 10.1016/j.ejcb.2022.151282
118. Rodriguez-Colman MJ, Schewe M, Meerlo M, Stigter E, Gerrits J, Pras-Raves M, et al. Interplay between metabolic identities in the intestinal crypt supports stem cell function. Nature (2017) 543(7645):424–7. doi: 10.1038/nature21673
119. Dolan B, Ermund A, Martinez-Abad B, Johansson MEV, Hansson GC. Clearance of small intestinal crypts involves goblet cell mucus secretion by intracellular granule rupture and enterocyte ion transport. Sci Signaling (2022) 15(752):eabl5848. doi: 10.1126/scisignal.abl5848
120. Guenther C, Martini E, Wittkopf N, Amann K, Weigmann B, Neumann H, et al. Caspase-8 regulates tnf-Alpha-Induced epithelial necroptosis and terminal ileitis. Nature (2011) 477(7364):335–9. doi: 10.1038/nature10400
121. Guenther C, Buchen B, He G-W, Hornef M, Torow N, Neumann H, et al. Caspase-8 controls the gut response to microbial challenges by tnf-Alpha-Dependent and independent pathways. Gut (2015) 64(4):601–10. doi: 10.1136/gutjnl-2014-307226
122. Levy J, Cacheux W, Bara MA, L'Hermitte A, Lepage P, Fraudeau M, et al. Intestinal inhibition of Atg7 prevents tumour initiation through a microbiome-influenced immune response and suppresses tumour growth. Nat Cell Biol (2015) 17(8):1062–73. doi: 10.1038/ncb3206
123. Wittkopf N, Guenther C, Martini E, Waldner M, Amann KU, Neurath MF, et al. Lack of intestinal epithelial Atg7 affects paneth cell granule formation but does not compromise immune homeostasis in the gut. Clin Dev Immunol (2012) 2012:278059. doi: 10.1155/2012/278059
124. Grootjans J, Hodin CM, de Haan J-J, Derikx JPM, Rouschop KMA, Verheyen FK, et al. Level of activation of the unfolded protein response correlates with paneth cell apoptosis in human small intestine exposed to Ischemia/Reperfusion. Gastroenterology (2011) 140(2):529–39. doi: 10.1053/j.gastro.2010.10.040
125. Zhan Y, Xu C, Liu Z, Yang Y, Tan S, Yang Y, et al. Beta-Arrestin1 inhibits chemotherapy-induced intestinal stem cell apoptosis and mucositis. Cell Death Dis (2016) 7(5):e2229. doi: 10.1038/cddis.2016.136
126. Sheahan BJ, Theriot CM, Cortes JE, Dekaney CM. Prolonged oral antimicrobial administration prevents doxorubicin-induced loss of active intestinal stem cells. Gut Microbes (2022) 14(1):2018898. doi: 10.1080/19490976.2021.2018898
127. Trentesaux C, Fraudeau M, Pitasi CL, Lemarchand J, Jacques S, Duche A, et al. Essential role for autophagy protein Atg7 in the maintenance of intestinal stem cell integrity. Proceedings of the National Academy of Sciences of the United States of America (2020) 117(20):11136–46. doi: 10.1073/pnas.1917174117
128. Strater J, Moller P. Expression and function of death receptors and their natural ligands in the intestine. Epithelial Transport And Barrier Function: Pathomechanisms In Gastrointestinal Disord (2000) 915:162–70. doi: 10.1111/j.1749-6632.2000.tb05239.x
129. Moller P, Walczak H, Riedl S, Strater J, Krammer PH. Paneth cells express high levels of Cd95 ligand transcripts - a unique property among gastrointestinal epithelia. Am J Of Pathol (1996) 149(1):9–13.
130. Blander JM. Death in the intestinal epithelium-basic biology and implications for inflammatory bowel disease. FEBS J (2016) 283(14):2720–30. doi: 10.1111/febs.13771
131. Arandjelovic S, Ravichandran KS. Phagocytosis of apoptotic cells in homeostasis. Nat Immunol (2015) 16(9):907–17. doi: 10.1038/ni.3253
132. Cummings RJ, Barbet G, Bongers G, Hartmann BM, Gettler K, Muniz L, et al. Different tissue phagocytes sample apoptotic cells to direct distinct homeostasis programs. Nature (2016) 539(7630):565–9. doi: 10.1038/nature20138
133. Vince JE, Silke J. The intersection of cell death and inflammasome activation. Cell And Mol Life Sci (2016) 73(11-12):2349–67. doi: 10.1007/s00018-016-2205-2
134. Gregory CD, Ford CA, Voss JJLP. Microenvironmental effects of cell death in malignant disease. Adv Exp Med Biol (2016) 930:51–88. doi: 10.1007/978-3-319-39406-0_3
135. Guenther C, Neumann H, Neurath MF, Becker C. Apoptosis, necrosis and necroptosis: Cell death regulation in the intestinal epithelium. Gut (2013) 62(7):1062–71. doi: 10.1136/gutjnl-2011-301364
136. Koren E, Yosefzon Y, Ankawa R, Soteriou D, Jacob A, Nevelsky A, et al. Arts mediates apoptosis and regeneration of the intestinal stem cell niche. Nat Commun (2018) 9:4582. doi: 10.1038/s41467-018-06941-4
137. Kaser A, Lee A-H, Franke A, Glickman JN, Zeissig S, Tilg H, et al. Xbp1 links er stress to intestinal inflammation and confers genetic risk for human inflammatory bowel disease. Cell (2008) 134(5):743–56. doi: 10.1016/j.cell.2008.07.021
138. Gorbunov NV, Garrison BR, Kiang JG. Response of crypt paneth cells in the small intestine following total-body gamma-irradiation. Int J Of Immunopathol. And Pharmacol (2010) 23(4):1111–23. doi: 10.1177/039463201002300415
139. Gorbunov NV, Kiang JG. Up-regulation of autophagy in small intestine paneth cells in response to total-body gamma-irradiation. J Of Pathol (2009) 219(2):242–52. doi: 10.1002/path.2591
140. Hodin CM, Lenaerts K, Grootjans J, de Haan JJ, Hadfoune Mh, Verheyen FK, et al. Starvation compromises paneth cells. Am J Of Pathol (2011) 179(6):2885–93. doi: 10.1016/j.ajpath.2011.08.030
141. Cadwell K, Liu JY, Brown SL, Miyoshi H, Loh J, Lennerz JK, et al. A key role for autophagy and the autophagy gene Atg16l1 in mouse and human intestinal paneth cells. Nature (2008) 456(7219):259–63. doi: 10.1038/nature07416
142. Cadwell K, Patel KK, Komatsu M, Virgin HW, Stappenbeck TS. A common role for Atg16l1, Atg5 and Atg7 in small intestinal paneth cells and crohn disease. Autophagy (2009) 5(2):250–2. doi: 10.4161/auto.5.2.7560
143. Patankar JV, Becker C. Cell death in the gut epithelium and implications for chronic inflammation. Nat Rev Gastroenterol Hepatol (2020) 17(9):543–56. doi: 10.1038/s41575-020-0326-4
144. Hui G-Q, Yang Y-J, Qin X-X, Qi S, Zhang J, Yu S-X, et al. Salmonella outer protein b suppresses colitis development Via protecting cell from necroptosis. Front In Cell And Infection Microbiol (2019) 9:87. doi: 10.3389/fcimb.2019.00087
145. Matsuzawa-Ishimoto Y, Shono Y, Gomez LE, Hubbard-Lucey VM, Cammer M, Neil J, et al. Autophagy protein Atg16l1 prevents necroptosis in the intestinal epithelium. J Of Exp Med (2017) 214(12):3687–705. doi: 10.1084/jem.20170558
146. Guenther C, Ruder B, Stolzer I, Dorner H, He G-W, Chiriac MT, et al. Interferon lambda promotes paneth cell death Via Stat1 signaling in mice and is increased in inflamed ileal tissues of patients with crohn's disease. Gastroenterology (2019) 157(5):1310–22. doi: 10.1053/j.gastro.2019.07.031
147. Araujo A, Safronova A, Burger E, Lopez-Yglesias A, Giri S, Camanzo ET, et al. Ifn-gamma mediates paneth cell death Via suppression of mtor. eLife (2021) 10:e60478. doi: 10.7554/eLife.60478
148. Simmons AN, Kajino-Sakamoto R, Ninomiya-Tsuji J. Tak1 regulates paneth cell integrity partly through blocking necroptosis. Cell Death Dis (2016) 7(4):e2196. doi: 10.1038/cddis.2016.98
149. Hirao LA, Grishina I, Bourry O, Hu WK, Somrit M, Sankaran-Walters S, et al. Early mucosal sensing of siv infection by paneth cells induces il-1 beta production and initiates gut epithelial disruption. PloS Pathog (2014) 10(8):e1004311. doi: 10.1371/journal.ppat.1004311
150. Zheng H, Chen Y, Li J, Li H, Zhao X, Li J, et al. Longitudinal analyses reveal distinct immune response landscapes in lung and intestinal tissues from sars-Cov-2-Infected rhesus macaques. Cell Rep (2022) 39(8):111014. doi: 10.1016/j.celrep.2022.110864
151. Jones JC, Brindley CD, Elder NH, Myers MG Jr., Rajala MW, Dekaney CM, et al. Cellular plasticity of Defa4(Cre)-expressing paneth cells in response to notch activation and intestinal injury. Cell And Mol Gastroenterol And Hepatol (2019) 7(3):533–54. doi: 10.1016/j.jcmgh.2018.11.004
Keywords: Paneth cell, cell differentiation, intestinal stem cell, antibacterial peptide, cell death
Citation: Cui C, Wang F, Zheng Y, Wei H and Peng J (2023) From birth to death: The hardworking life of Paneth cell in the small intestine. Front. Immunol. 14:1122258. doi: 10.3389/fimmu.2023.1122258
Received: 12 December 2022; Accepted: 28 February 2023;
Published: 10 March 2023.
Edited by:
Eduard F. Stange, University of Tübingen, GermanyReviewed by:
Markus Tschurtschenthaler, Technical University of Munich, GermanyClaudia Günther, University Hospital Erlangen, Germany
Copyright © 2023 Cui, Wang, Zheng, Wei and Peng. This is an open-access article distributed under the terms of the Creative Commons Attribution License (CC BY). The use, distribution or reproduction in other forums is permitted, provided the original author(s) and the copyright owner(s) are credited and that the original publication in this journal is cited, in accordance with accepted academic practice. No use, distribution or reproduction is permitted which does not comply with these terms.
*Correspondence: Jian Peng, pengjian@mail.hzau.edu.cn