- 1State Key Laboratory of Freshwater Ecology and Biotechnology, Institute of Hydrobiology, The Innovative Academy of Seed Design, Chinese Academy of Sciences, Wuhan, China
- 2College of Life Sciences, University of Chinese Academy of Sciences, Beijing, China
- 3Hubei Hongshan Laboratory, Chinese Academy of Sciences, Wuhan, China
- 4Fisheries Institute, Sichuan Academy of Agricultural Sciences, Chengdu, China
Src homology region 2 domain-containing phosphatase 1 (SHP1), encoded by the protein tyrosine phosphatase nonreceptor type 6 (ptpn6) gene, belongs to the family of protein tyrosine phosphatases (PTPs) and participates in multiple signaling pathways of immune cells. However, the mechanism of SHP1 in regulating fish immunity is largely unknown. In this study, we first identified two gibel carp (Carassius gibelio) ptpn6 homeologs (Cgptpn6-A and Cgptpn6-B), each of which had three alleles with high identities. Then, relative to Cgptpn6-B, dominant expression in adult tissues and higher upregulated expression of Cgptpn6-A induced by polyinosinic-polycytidylic acid (poly I:C), poly deoxyadenylic-deoxythymidylic (dA:dT) acid and spring viremia of carp virus (SVCV) were uncovered. Finally, we demonstrated that CgSHP1-A (encoded by the Cgptpn6-A gene) and CgSHP1-B (encoded by the Cgptpn6-B gene) act as negative regulators of the RIG-I-like receptor (RLR)-mediated interferon (IFN) response via two mechanisms: the inhibition of CaTBK1-induced phosphorylation of CaMITA shared by CgSHP1-A and CgSHP1-B, and the autophagic degradation of CaMITA exclusively by CgSHP1-A. Meanwhile, the data support that CgSHP1-A and CgSHP1-B have sub-functionalized and that CgSHP1-A overwhelmingly dominates CgSHP1-B in the process of RLR-mediated IFN response. The current study not only sheds light on the regulative mechanism of SHP1 in fish immunity, but also provides a typical case of duplicated gene evolutionary fates.
Introduction
SHP1, which is encoded by the ptpn6 gene, is a member of the family of PTPs that catalyze the dephosphorylation of tyrosyl residues in proteins phosphorylated by protein tyrosine kinases (PTKs) (1, 2). PTPs and PTKs participate in cell-cycle progression, cell motility and invasion, as well as in cell death and apoptosis (3, 4). SHP1 has two N-terminal SH2 domains (N-SH2 and C-SH2), followed by a classical PTP domain and a C-terminal tail (C-tail), and contains two tyrosyl phosphorylation sites. In response to the activation signal, SHP1 is recruited to membrane-bound inhibitory receptors through the binding of its SH2 domain to tyrosine-phosphorylated immunoreceptor tyrosine-based inhibitory motifs (ITIMs) (5, 6). It is a critical regulator of immune cell development and function and has been considered as an immune checkpoint (1). Studies on natural mouse mutants (motheaten and motheatenviable) and conditional cell-type-specific Shp1 mutants (Ptpn6f/fCD19Cre/+) both showed that Shp1 plays critical roles in regulating the differentiation and/or activation of B cells (7, 8), T cells (9), dendritic cells (10), and neutrophils (11). Moreover, it is required to establish life-long protective humoral immunity (8). Once recruited to the inhibitory receptors through ITIMs, SHP1 controls multiple signaling pathways to obtain an ideal immune response (12–16).
Although the roles of SHP1 have been well documented in mammals (17, 18), the research in fish is still scarce. Several studies have demonstrated that SHP1 can be recruited by different immune-type receptors, such as channel catfish (Ictalurus punctatus) leukocyte immune-type receptors (IpLITRs) (19, 20), sea lamprey (Petromyzon marinus) T-cell receptor-like molecule (TCRL) (21) and gibel carp diverse immunoglobulin domain-containing protein (DICP) (22). In addition, only a few studies have reported the expression changes of SHP1 and its role in the immune reaction after bacterial challenge (23, 24). Morpholino knockdown of ptpn6 in zebrafish embryo was shown to lead to the hyperinduction of innate immune response genes such as ifnφ1, il1b, il8, tnfa, and tnfb during Salmonella typhimurium or Mycobacterium marinum infection (23). The expression of ptpn6 in Nile tilapia was upregulated after Streptococcus agalactiae infection and may involve in the B cell receptor (BCR) signaling pathway (24). However, to date, little is known about the function of fish SHP1 in regulating IFN mechanism.
Most extant vertebrates have evolved from polyploid ancestors. It is now universally accepted that two rounds (2R) of whole-genome duplication (WGD) occurred at the root of vertebrates, and a subsequent teleost fish-specific (Ts3R) WGD took place after the divergence of tetrapods and teleosts (25–30). More recent WGD events have also occurred in some actinopteriygiian families, including Acipenseridae, Cyprinidae, and Salmonidae (31, 32). In general, WGD are thought to increase genetic complexity and variability, which would in turn give rise to evolutionary novelties and broader adaptabilities (28, 33). During the subsequent post-polyploid diploidization (PPD), the duplicated genes experience divergent evolutionary trajectories and undergo partitioning under relaxed purification options. Their evolutionary fates include retention/loss, non-(pseudogenization), sub- or neo-functionalization (33, 34). Although the evolutionary fates of duplicated genes have been well elaborated in plant polyploids, only a few studies on recurrent animal polyploids have been reported due to the difficulties in discriminating the different homeologs/alleles of duplicated genes. We had recently elaborated the divergent functions of duplicated foxl2 and viperin homeologs in gibel carp (35, 36). However, no single model or ideal could explain all evolutionary ways and fates of duplicate genes (30, 33, 37). Therefore, it is necessary to investigate more cases to deepen our understanding of the evolutionary “rules” in animal polyploids.
Gibel carp, which is widely distributed across the Eurasian continent (38–43), has been recognized as an evolutional hexaploid with over 150 chromosomes in comparison with tetraploid goldfish (C. auratus) with 100 chromosomes (44–46). Analyses of several conserved genes suggests that two extra rounds of polyploidy, an early allopolyploidy and a later autopolyploidy, had taken place during gibel carp evolution (35, 36, 47–50). Disease resistance breeding has become an important hotspot in the current research landscape. In our previous study, we identified several candidate resistant-related genes (50–53) and found that gibel carp DICPs recruit SHP1 through the ITIM motif to inhibit the induction of IFN and interferon-stimulated gene (ISGs) (22). However, the molecular mechanism between SHP1 and IFN is still unknown. In this study, we first analyzed the diversification, evolution, and biased expression pattern of two ptpn6 homeologs (Cgptpn6-A and Cgptpn6-B) in hexaploid gibel carp. Then, we explored the roles of CgSHP1-A and CgSHP1-B in the immune response regulation underlying their biased expression. Finally, we investigated the divergent mechanisms of CgSHP1-A and CgSHP1-B in regulating IFN through in vitro over-expression functional analysis.
Materials and Methods
Cells and Virus
Epithelioma papulosum cyprini (EPC) cells for western blotting and subcellular localization, Human embryonic kidney (HEK) 293T cells for coimmunoprecipitation (Co-IP) and dephosphorylation assays were cultured as described previously (54). Gibel carp brain (GiCB) cells for quantitative real-time PCR (qPCR) and viral infection were kindly provided by Prof. Zeng (Yangtze River Fisheries Research Institute, Chinese Academy of Fishery Sciences) (55). SVCV, a negative sense single-stranded RNA virus in the family Rhabdoviridae that could infect crucian carp and gibel carp (56) was propagated in GiCB cells until cytopathic effects (CPE) were observed, and then the culture media with cells were harvested and stored at -80°C until needed.
Amplification of CgSHP1 and Sequence Analysis
According to the genome sequences of gibel carp clone F, Cgptpn6-A and Cgptpn6-B cDNAs were amplified from gibel carp head kidney cDNA library by Rapid Amplification of cDNA Ends Polymerase Chain Reaction (RACE-PCR). PCR products amplified by a high-fidelity polymerase (TransGen Biotech) were purified and cloned into Trans5α Chemically Competent Cells. About 30 clones of each sample were sequenced and classified according to the specific SNPs among the sequences. The complete cDNA sequences of six Cgptpn6 transcripts were deposited in GenBank (accession numbers from OK142786-OK142791). Amino acid sequences and domains were predicted by open reading frame (ORF) Finder (https://www.ncbi.nlm.nih.gov/orffinder/) and SMART (http://smart.embl-heidelberg.de/), multiple amino acid sequence alignment was performed by DNAman version 7.0 software. Phylogenetic tree was constructed by bootstrap analysis (1000 replicates) using the neighbor-joining method (NJ) in MEGA 7.0 software (57).
All the amino acid sequences used in this study were obtained from GenBank (http://www.ncbi.nlm.nih.gov/) and Ensembl (http://www.ensembl.org). The accession numbers are as following: Homo sapiens SHP1, NP_002822.2; Mus musculus SHP1, NP_038573.2; Gallus gallus SHP1, NP_001026655.1; Lepisosteus oculatus SHP1, ENSLOCT00000009309.1; Danio rerio SHP1, NP_956254.1; Carassius auratus SHP1-A, XP_026109501.1; Carassius auratus SHP1-B, XP_026139710.1. The exon-intron structure was determined by aligning cDNA and genomic sequences. Syntenic analyses were conducted by comparing the chromosomic regions around ptpn6 genes in gibel carp chromosomes (CgA16 and CgB16) and crucian carp chromosomes (CaA16 and CaB16) with corresponding regions in H. sapiens chromosome 12, M. musculus chromosome 6, G. gallus chromosome 1, L. oculatus chromosome LG26, D. rerio chromosome 16. The genome information was obtained from the Ensembl genome database.
Chromosome Preparation and Fluorescence In Situ Hybridization (FISH)
Chromosome preparation was performed as described previously (58). Five individuals of gibel carp clone F were injected phytohemagglutinin (PHA) (15-20 μg/g) in vivo and the head kidney cells were harvested by conventional hypotonic and fixation treatments. Briefly, the cells were exposed to a hypotonic solution for 30 min at room temperature and fixed for 30 min (with replacement of the fixative every 10 min without resuspension) in 3 ml of a 3:1 mixture of methanol and acetic acid. Finally, the cells were resuspended in 0.5 ml of fresh fixative and were spread on clean slides. The slides were prepared by the air-drying technique and storied at -20°C for FISH.
The bacterial artificial chromosome (BAC) clones containing Cgptpn6-A and Cgptpn6-B were screened by PCR. Then, Cgptpn6-A-BAC-DNA and Cgptpn6-B-BAC-DNA labeled by DIG-Nick Translation Mix and Biotin-Nick Translation Mix (Roche) respectively were used to perform FISH as described previously (35, 47). 4’, 6-diamidino-2-phenylindole (DAPI) was used to counterstain metaphase chromosomes. The results were acquired by Carl Zeiss upright fluorescence microscope Axio imager M2 (Analytical & Testing Center, IHB, CAS).
RNA Extraction, Reverse Transcription, and Quantitative Real-Time PCR (qPCR)
Total RNAs from 12 adult tissues, including brain, kidney, intestine, skin, gill, heart, liver, muscle, spleen, thymus, ovary and head kidney, and GiCB cells were extracted by Trizol reagent (Invitrogen). RNase-free DNase was used to purify RNA by removing all contaminating genomic DNA. The first-strand cDNA was synthesized by using a GoScript Reverse Transcription System (Promega) according to the manufacturer’s instructions. qPCR was performed with Fast SYBR Green master mix (BioRad) on a CFX96 Real-Time System (BioRad). PCR conditions were as follows: 95°C for 5 min, then 40 cycles of 95°C for 20 s, 60°C for 20 s, 72°C for 20 s. Eukaryotic translation elongation factor 1 alpha 1, like 1 (eef1a1l1) (M value = 0.74 < 1.5) was selected as the optimal reference gene for qPCR analysis according to the previous study (52). The primers of other IFN-related genes were also synthetized for qPCR (Supplementary Table 1). The specificity of the PCR amplification for all primer pairs was verified from the dissociation curves. The relative gene expression levels were calculated with 2-△△CT method. All the samples were analyzed in triplicates.
Plasmid Construction
For Coimmunoprecipitation assay (Co-IP) and Western blotting, the ORFs of CgSHP1-A and CgSHP1-B were cloned into pCMV-Myc, pCMV-HA (Clontech) and pcDNA3.1(+), respectively. For subcellular localization, the ORFs of CgSHP1-A and CgSHP1-B were inserted into pEGFP-N3 (Clontech) vector. Owing the extremely high amino acid sequence identities (98.46%-100.00%) of the IFN-related genes and autophagy-related genes between gibel carp and crucian carp (C. auratus) used in this study, we chose the corresponding plasmids from crucian carp constructed previously. The ORF of C. auratus mediator of IFN regulatory factor 3 (IRF3) activation (CaMITA) [also called stimulator of interferon genes (STING)] (Gene accession number: MZ172421) and kinase TANK-binding kinase 1 (CaTBK1) (Gene accession number: MZ172419) were inserted into pCS2-mCherry vector (Clontech Laboratories). The ORFs of mitochondrial antiviral signaling protein (CaMAVS) (Gene accession number: MZ170793), CaMITA, CaTBK1, CaIRF3 (Gene accession number: MZ172420), microtubule-associated Protein 1A/1B-Light Chain 3 (CaLC3) (Gene accession number: XM_026238864.1), CaBeclin1 (Gene accession number: XM_026249455.1), and autophagy-related gene 14 (CaATG14) (Gene accession number: XM_026286484.1) were cloned into pCMV-HA, pCMV-Myc and pCMV-Tag2c vector. Compared to the crucian carp genome, CaMAVS, CaMITA and CaIRF3 localize in A subgenome, while CaTBK1 and CaATG14 belong to B subgenome. The plasmids containing CaIFN-luc and ISRE-Luc in pGL3-Basic luciferase reporter vectors were constructed as described previously (36). The primers including the restriction enzyme cutting sites used for plasmid construction were also listed in Supplementary Table 1. These primers were designed with Oligo Calc (Oligonucleotide Properties Calculator) (http://biotools.nubic.northwestern.edu/OligoCalc.html).
Transient Transfection, Subcellular Localization and Virus Infection
Transient transfections were performed in EPC and GiCB cells seeded in 6-well or 24-well plates by using FishTrans Transfection Reagent (MeiSenTe Biotechnology) according to the manufacturer’s protocol [Total plasmid dosage (μg) and FishTrans (μl) dosage is at the ratio of 1:2]. For subcellular localization, EPC cells were plated onto coverslips in 6-well plates and transfected with indicated plasmids for 24 h. Following this, the cells were washed twice with phosphate-buffered saline (PBS) and fixed with 4% paraformaldehyde (PFA) for 1 h. After draining the fixative, the cells were stained with DAPI (1 μg/ml; Beyotime) for 5 min in a dark at room temperature. Finally, the coverslips were washed and observed with a Leica confocal microscope under a × 63 oil immersion objective (SP8; Leica Microsystems). Fluorescence intensity was analyzed with Image J.
For the antiviral assay, GiCB cells were seeded to 24-well plates and were transfected with 0.5 μg CgSHP1-A and CgSHP1-B or pcDNA3.1(+) vector, separately. At 24 h post-transfection, the GiCB cells were infected with SVCV at a multiplicity of infection (MOI = 0.01) and incubated at 28°C. At 48 h post-infection, the cell monolayers were washed with PBS, fixed with 4% PFA for 1 h, and stained with 0.05% crystal violet overnight to observe the CPE. The supernatants were subjected to 10-fold serial dilutions and then added (100 μl) onto a monolayer of GiCB cells cultured in a 96-well plate. After 48 h, the medium was removed and the cells were washed with PBS, fixed by 4% PFA and stained with 1% crystal violet. The virus titer was expressed as 50% tissue culture infective dose (TCID50/ml). Results are the representative of three independent experiments.
Luciferase Activity Assay
EPC cells were seeded in 24-well plates, and 24 h later co-transfected with 250 ng luciferase reporter plasmid (CaIFNpro-luc or ISRE-Luc), 250 ng CgSHP1-A, CgSHP1-B or pcDNA3.1(+), and 50 ng Renilla luciferase internal control vector (pRL-TK, Promega). Then, the cells were transfected again with a mimic of viral dsRNA poly I:C or the double-stranded DNA mimetic poly dA:dT at 24 h post-transfection, and the cells were infected by SVCV. To further explore the role of SHP1 in the RLR-induced interferon reaction, EPC cells were co-transfected with plasmids as described above, then transfected 250 ng CaMAVS-, CaMITA-, CaTBK1- or CaIRF3-Myc expressing plasmid or pCMV-Myc empty vector respectively. At 24 h post-transfection or infected, the cells were washed in PBS and lysed for measuring luciferase activity by Dual-Luciferase Reporter Assay System, according to the manufacturer’s instructions (Promega). Fireflyluciferase activities were normalized on the basis of Renilla luciferaseactivity. The results were the representative of more than three independent experiments, each performed in triplicate. Luciferase and qPCR assay data are expressed as the mean ± standard error of the mean (SEM). Error bars indicate the SEM (n = 3, biologically independent samples). Data were analyzed using a Student’s unpaired t-tests. A probability (p) < 0.05 was considered statistically significant (∗), and p < 0.01 was considered extremely significant (∗∗).
In Vitro Protein Dephosphorylation Assay and Western Blotting
Transfected HEK 293T cells were lysed in radioimmuno-precipitation (RIPA) lysis buffer [1% NP-40, 50 mM Tris-HCl (pH 7.5), 150 mM NaCl, 1 mM EDTA, 1 mM NaF, 1 mM sodium orthovanadate,1 mM phenyl-methylsulfonyl fluoride, and 0.25% sodium deoxycholate] without phosphatase inhibitors. Protein dephosphorylation was carried out in 100 μl reaction mixtures consisting of 100 μg of cell protein and 10 U of calf intestinal phosphatase (CIP) (Sigma-Aldrich) (59). The reaction mixtures were incubated at 37°C for 1 h, then separated by 10% SDS-PAGE and transferred to polyvinylidene difluoride (PVDF) membrane (Bio-Rad). The membranes were blocked and incubated with indicated primary antibodies (Abs) at an appropriate dilution overnight at 4°C, washed three times with TBST buffer [25 mM Tris-HCl, 150 mM NaCl, 0.1% Tween 20 (pH 7.5)] and then incubated with secondary Abs. After additional three washes with TBST, the membranes were stained with Immobilon TM Western Chemiluminescent HRP Substrate (Millipore) and detected using an Image Quant LAS4000 system (GE Healthcare). Abs were diluted as follows: anti-β-actin (Cell Signaling Technology) at 1:3,000, anti-Flag (Sigma-Aldrich) at 1:3,000, anti-Myc (Santa Cruz Biotechnology) at 1:3,000, HRP-conjugated anti-mouse IgG or anti-rabbit IgG (Thermo Scientific) at 1:5,000. The results were the representative of three independent experiments.
Coimmunoprecipitation Assay
HEK 293T cells seeded in 10 cm2 dishes overnight were transfected with a total of 10 µg of the plasmids. At 24 h post-transfection, medium was removed carefully and cell monolayer was washed twice with 10 ml ice-cold PBS. Then the cells were lysed in 1 ml of RIPA lysis buffer containing protease inhibitor mixture (Sigma-Aldrich) at 4°C for 1 h on a rocker platform. The cellular debris was removed by centrifugation at 12,000 × g for 15 min at 4°C. The 100 μl supernatant was transferred to a fresh tube and the rest was incubated with 30 μl of anti-Flag or anti-Myc affinity gel (Sigma-Aldrich) overnight at 4°C with constant agitation. Immunoprecipitated proteins were collected by centrifugation at 5,000 × g for 1 min at 4°C, washed three times with lysis buffer, and resuspended in 100 μl SDS sample buffer (59). The immunoprecipitates and whole cell lysates were analyzed by western blotting with the indicated Abs.
Results
Two Divergent Cgptpn6 Homeologs With Conserved Genomic Structure in Gibel Carp
Six Cgptpn6 transcripts cloned from gibel carp head kidney were clearly clustered into two homeologs (Cgptpn6-A and Cgptpn6-B), and each of them clearly possessed three alleles (Supplementary Figure 1). The average identities among the three alleles (99.87% ± 0.09% for Cgptpn6-A and 99.73% ± 0.05% for Cgptpn6-B) were higher than that between Cgptpn6-As and Cgptpn6-Bs (89.28% ± 0.07%). The major differences between the Cgptpn6-As and Cgptpn6-Bs homeologs were observed in the 3′ untranslated region (UTR), where the sequence identity was only 73.10%. The ORFs of the three Cgptpn6-A alleles were all 1761 bp, encoding two CgSHP1-A proteins [CgSHP1-A1 and CgSHP1-A2/A3, 586 amino acids (aa)] with one aa difference at the 29th. The ORFs of the three Cgptpn6-B genes were also 1761 bp, encoding the same CgSHP1-B protein (586 aa, CgSHP1-B1/B2/B3). All gibel carp SHP1 proteins possessed three conserved domains (two SH2-domains [N-SH2, C-SH2] and a PTPc domain). The PTP signature motif (I/VHCSAGIGRTG) is identical among mammals and fish SHP1 (Supplementary Figure 2A). Multiple sequence alignments and phylogenetic analysis showed that almost identical (99.08% and 100.00%) gibel carp and crucian carp SHP1-A and SHP1-B were grouped into two separate branches, implying the duplication of the ptpn6 gene in the common ancestor of gibel carp and crucian carp, which then clustered with zebrafish SHP1 and spotted gar SHP1 (Supplementary Figure 2B).
Subsequently, the genomic structure and syntenic alignment of gibel carp ptpn6-A and ptpn6-B and other vertebrates were identified. Except chicken Ptpn6 (15 exons), both Cgptpn6-A and Cgptpn6-B, as well as other vertebrate ptpn6, were composed of 16 exons (Figure 1A). Cgptpn6-A and Cgptpn6-B possess almost identical lengths of exons except the first and last exons, which are the loci for transcribing the 5′ and 3′ UTR. However, the lengths of their introns are varied and their identity is only 52.70%. The identities between the corresponding introns of Cgptpn6-A and Cgptpn6-B ranged from 19.82% (11th intron) to 90.74% (9th intron). Similarities in the genomic structure between crucian carp ptpn6-A and ptpn6-B were also observed. In addition, only the lengths of the 1st, 7th, and 16th exons of Cgptpn6-A and Cgptpn6-B are different from zebrafish ptpn6, implying a highly conserved genomic structure in Cyprinidae fish. Human PTPN6 and mouse Ptpn6 have similar exon lengths, which are different from chicken and fish ptpn6 genes.
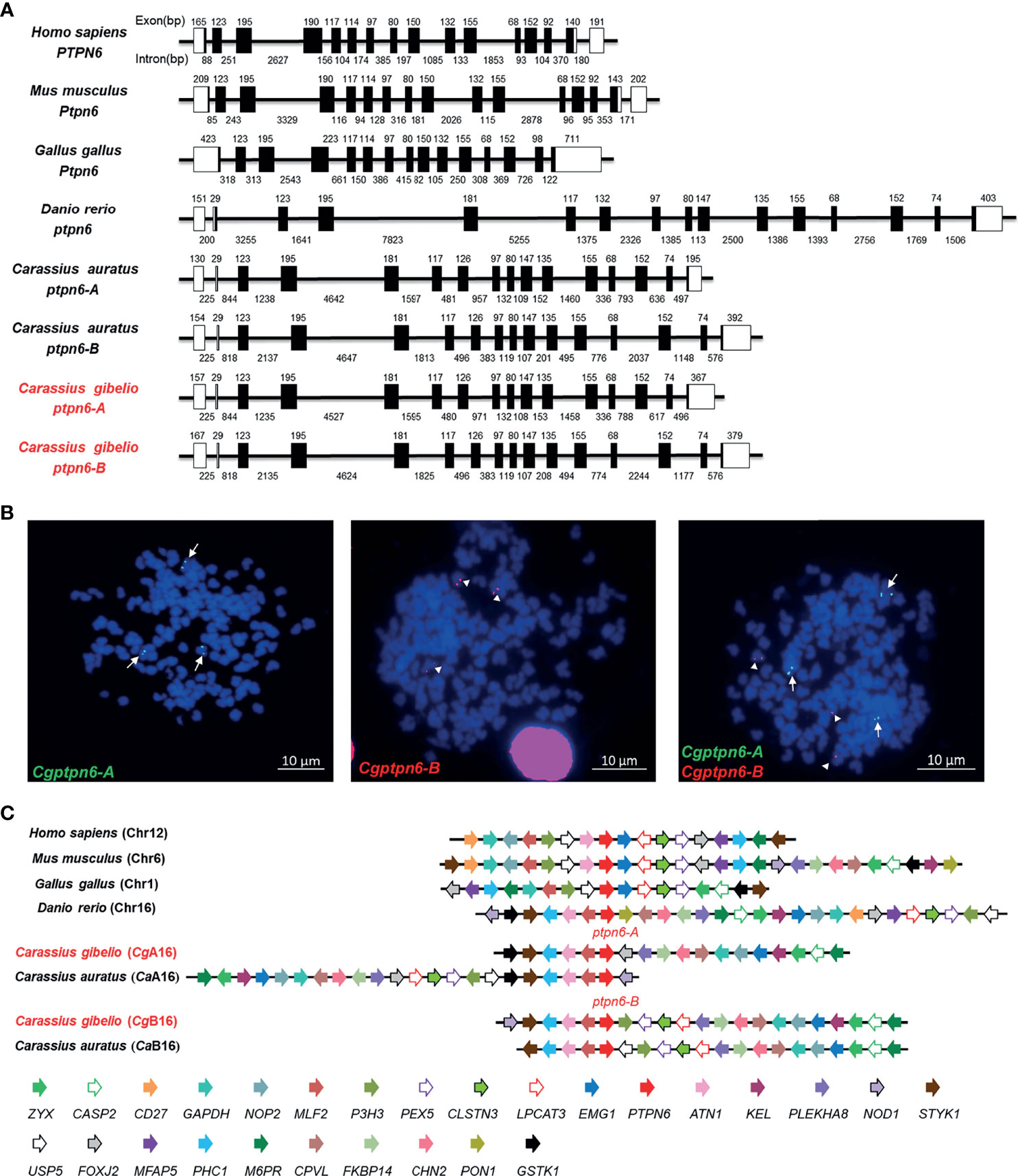
Figure 1 Molecular characterization of Cgptpn6-A and Cgptpn6-B in gibel carp. (A) Genomic structure of ptpn6 genes. Exons and introns are shown by boxes and horizontal lines, respectively. ORFs are highlighted by black boxes. The exon and intron size are indicated upon or below themselves as base pairs (bp). (B) Localization of Cgptpn6-A (green, indicated by arrows) and Cgptpn6-B (red, indicated by arrowheads) on metaphase chromosomes (blue). Scale bars = 10 μm (C) Syntenic alignment of chromosomal regions around vertebrate ptpn6 genes. Cgptpn6-A and Cgptpn6-B are located on the chromosome CgA16 and CgB16 respectively. Chromosome segments are represented as thick lines. The conserved gene blocks are shown in matching colors and the transcription orientation are indicated by arrows.
Each Cgptpn6 homeolog with three alleles was confirmed by FISH. Consistent with our previous studies (35, 36), three green Cgptpn6-A signals and three red Cgptpn6-B signals were located on the three different chromosomes when simultaneously using Cgptpn6-A-BAC-DNA and Cgptpn6-B-BAC-DNA as probes respectively (Figure 1B). Syntenic alignment showed that gibel carp chromosome CgA16 and CgB16 both retained approximately 60% of the analyzed homologous genes in zebrafish chromosome 16 and had a conserved gene block (styk1-phc1-atn1-mlf2-ptpn6). One homeolog of the other duplicated genes in CgA16 and CgB16 seemed to be deleted, and became singletons. For example, p3h3, pex5, clstns, lpcat3, and nod1 were mapped only in CgB16, while foxj2 and gstk1 ware located only in CgA16 (Figure 1C).
Dominant Expression of Cgptpn6-A in Gibel Carp Adult Tissues
The distributions of Cgptpn6-A and Cgptpn6-B in 12 adult tissues of gibel carp were analyzed by qPCR. Two specific pairs of primers were designed to amplify Cgptpn6-A and Cgptpn6-B, respectively. Cgptpn6-A and Cgptpn6-B were abundantly expressed in the immune-related tissues, such as spleen, head kidney, kidney, and thymus (Figure 2). Cgptpn6-A expression was remarkably higher (20-1726 folds) than that of Cgptpn6-B in all tissues, suggesting that Cgptpn6-A may play a dominant role in immune regulation.
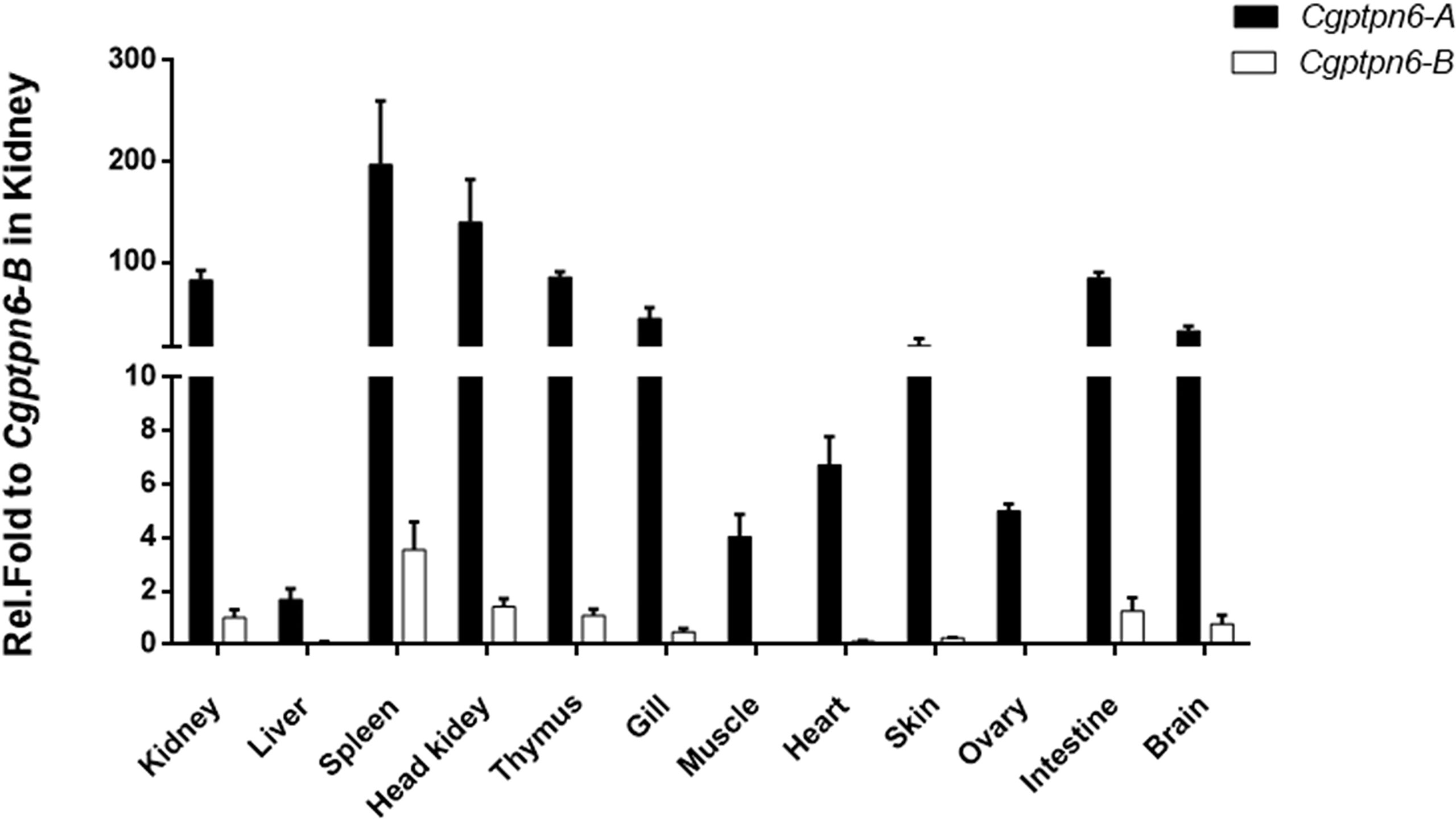
Figure 2 qPCR analysis of Cgptpn6-A and Cgptpn6-B expression in healthy adult tissues. eef1a1l1 was used as control. Each bar represents mean ± standard deviation (SD) (n = 3).
Higher Upregulated Expression of Cgptpn6-A Induced by Poly I:C, Poly dA:dT, and SVCV
Subsequently, the dynamic expression changes of Cgptpn6-A and Cgptpn6-B were investigated after stimulation with poly I:C, poly dA:dT, and SVCV. Cgptpn6-A and Cgptpn6-B expression increased up to 9.5-fold and 3-fold, respectively, at 24 h after poly I:C treatment (Figure 3A). Poly dA:dT showed a weaker stimulation (4.7-fold at 72 h) for the upregulation of Cgptpn6-A expression and showed no effect on the expression of Cgptpn6-B (Figure 3D). In contrast, Cgptpn6-A and Cgptpn6-B expression were remarkably up-regulated (2293- and 68-fold respectively) at 48 h after SVCV infection (Figure 3G). These results indicate that Cgptpn6-A and Cgptpn6-B may both participate in the host immune response, with Cgptpn6-A playing a dominant role. Similar to Cgptpn6, the other IFN-related genes, such as Cgifn, Cgirf3, Cgrig-i, and Cgviperin, all have two homeologs (52). Cgifn-A and Cgifn-B, as well as Cgirf3-A and Cgirf3-B, showed similar dynamic expression changes (Figures 3B, C, E, F, H, I) as Cgptpn6-A and Cgptpn6-B, implying potential association between Cgptpn6 and IFN response.
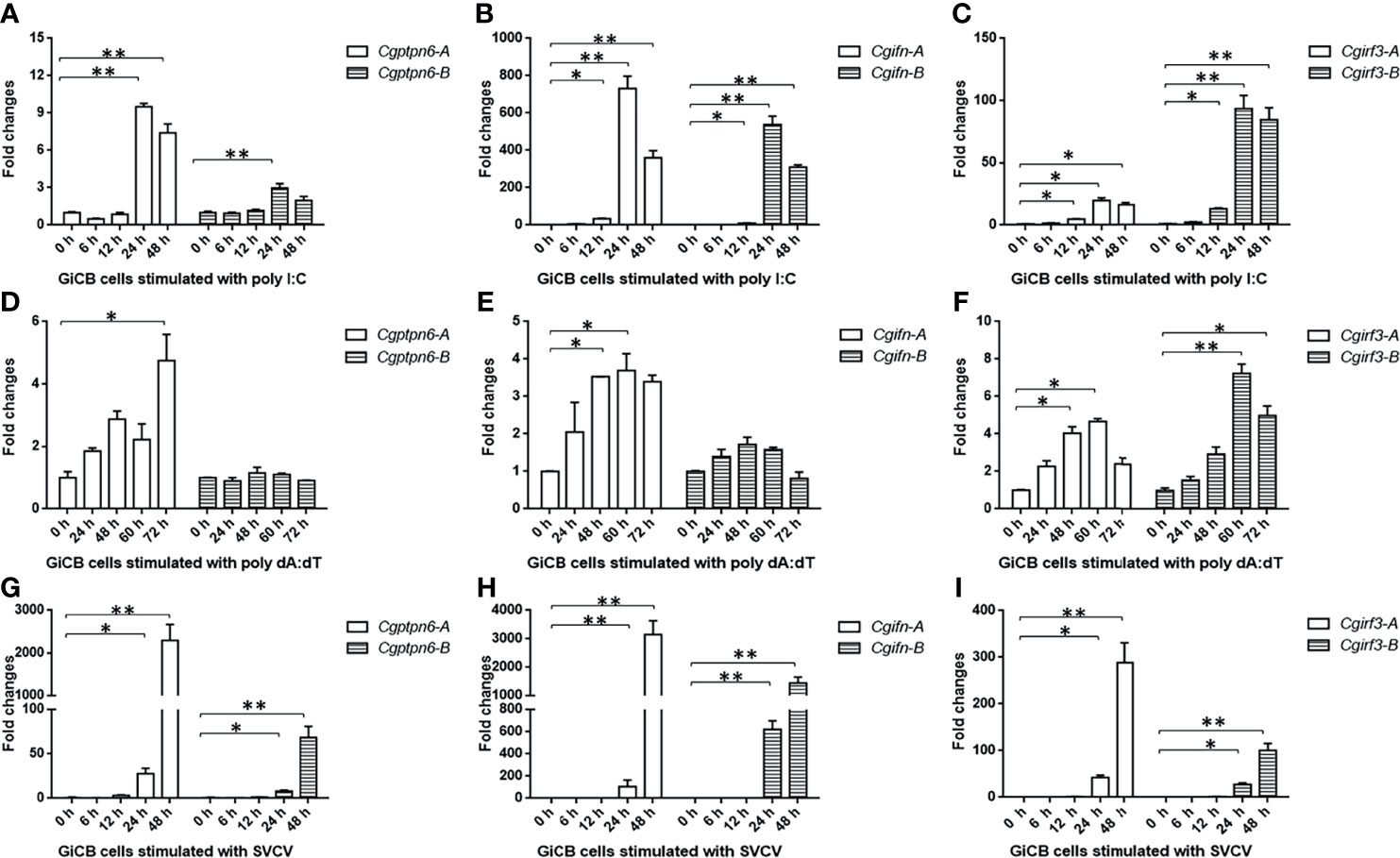
Figure 3 Dynamic expression changes of Cgptpn6-A and Cgptpn6-B, Cgifn-A and Cgifn-B, Cgirf3-A and Cgirf3-B stimulated by 1 μg poly I:C (A–C), poly dA:dT (D–F) or SVCV (MOI = 1) in GiCB cells (G–I) by qPCR analyses. eef1a1l1 was used as an internal control for normalization and the relative expression is represented as fold induction relative to the expression level in control cells. Each bar represents mean ± standard deviation (SD) (n = 3). Asterisks indicate significant differences from control (*p < 0.05, **p < 0.01).
CgSHP1-A and CgSHP1-B Both Negatively Regulate IFN Response
To explore the association between Cgptpn6 and innate immunity, the effects of Cgptpn6-A and Cgptpn6-B on IFN regulation were examined. The overexpression of CgSHP1-A and CgSHP1-B both remarkably inhibited CaIFN promoters and ISRE activities induced by poly I:C, poly dA:dT, and SVCV (Figures 4A–C). In comparison with CgSHP1-B, only a slightly stronger inhibition of CgSHP1-A was observed. Previous studies showed that fish IFN response could be triggered through the RLR signaling pathway (60). As shown in Figure 4D, the co-transfection of CgSHP1-A or CgSHP1-B can obviously inhibit the activities of CaIFNpro and ISRE promoted by CaMAVS, CaMITA, and CaTBK1. The repression effects seemed to be stronger by the co-transfection of CgSHP1-A relative to CgSHP1-B. In addition, both CgSHP1-A and CgSHP1-B had no significant effect on the activities of CaIFNpro and ISRE induced by CaIRF3.
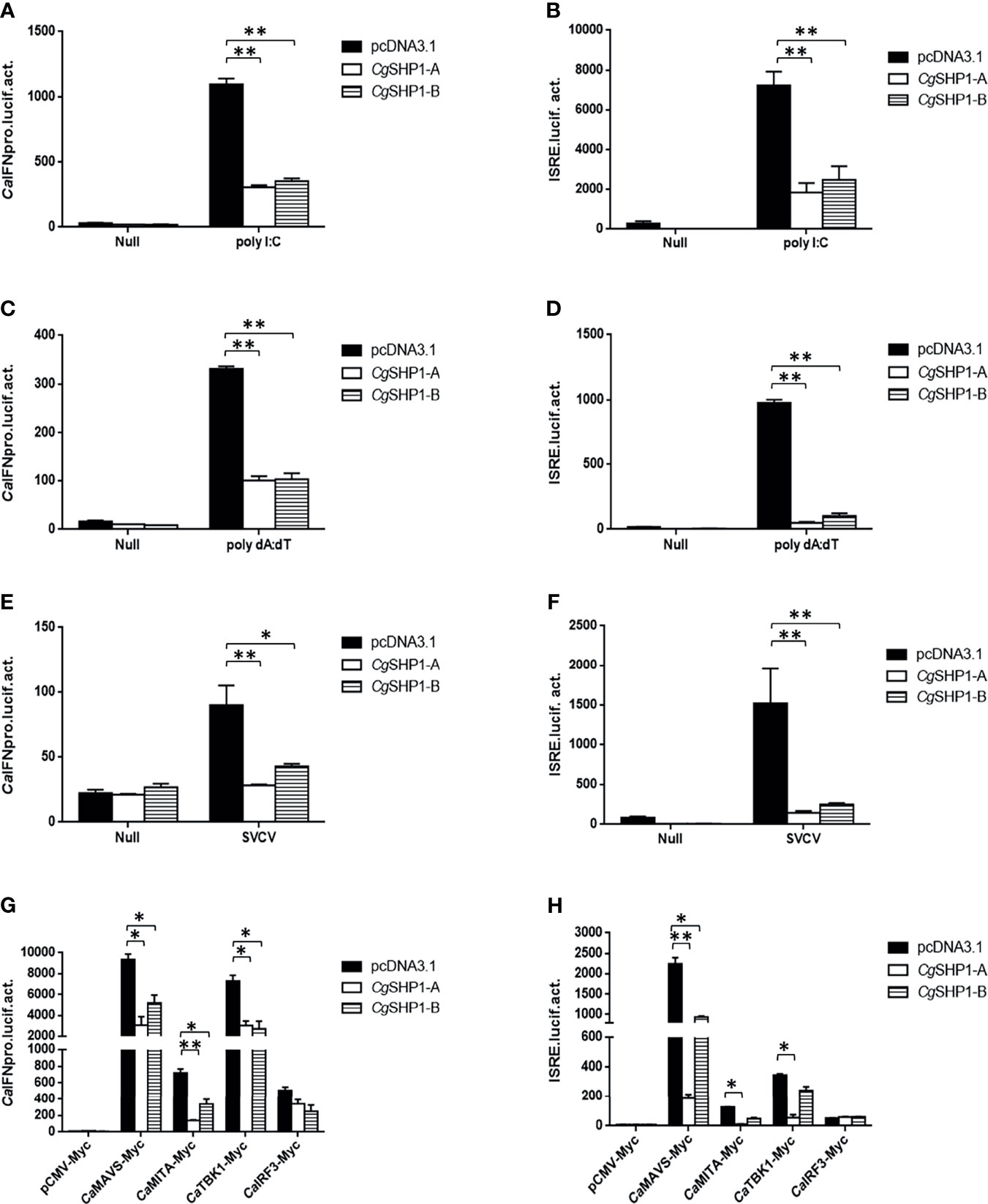
Figure 4 CgSHP1-A and CgSHP1-B inhibit IFN response induced by 1 μg poly I:C (A, B), poly dA:dT (C, D), SVCV (MOI = 1) (E, F) in EPC cells. CgSHP1-A and CgSHP1-B inhibit CaMAVS, CaMITA and CaTBK1-mediated activation of CaIFNpro and ISRE (G, H). Data are expressed as mean ± SEM, n = 3. Asterisks indicate significant differences from control (*p < 0.05, **p < 0.01).
These results were further supported by qPCR findings. The upregulated expression of RLR molecules (Cgrig-i-A and Cgrig-i-B), ifn (Cgifn-A and Cgifn-B), and ISGs (Cgviperin-A and Cgviperin-B) induced by poly I:C or poly dA:dT were remarkably reduced by CgSHP1-A or CgSHP1-B overexpression. Similarly, the inhibitory effect of CgSHP1-A was more significant than that of CgSHP1-B (Figures 5A, B, E, F). Similar to the results of the luciferase activity assay, the increased expression of Cgirf3-A and Cgirf3-B were rarely influenced by the CgSHP1-A or CgSHP1-B overexpression induced by poly I:C (Figures 5C, D), while the upregulated expression of Cgirf3-A and Cgirf3-B were decreased by the CgSHP1-A or CgSHP1-B overexpression induced by poly dA:dT (Figures 5G, H). These data demonstrate that CgSHP1-A and CgSHP1-B may negatively regulate IFN response through the RLR signaling pathway.
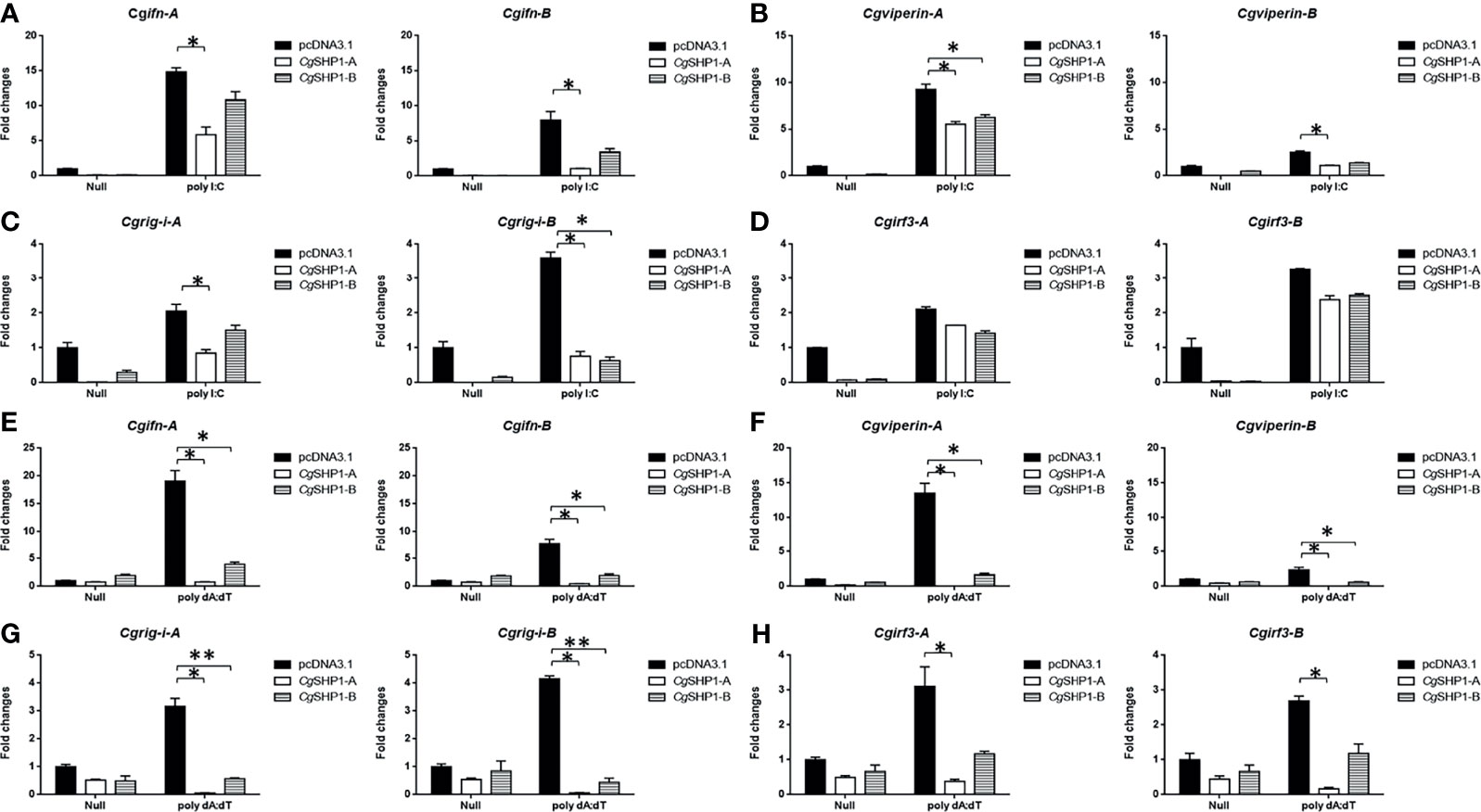
Figure 5 CgSHP1-A and CgSHP1-B inhibit the expression of gibel carp IFN and IFN-related genes induced by 1 μg/ml poly I:C (A–D) or poly dA:dT (E–H) in GiCB cells. eef1a1l1 was used as control and the relative expression is represented as fold induction relative to the expression level in control cells (set to 1). Each bar represents mean ± SEM (n = 3). The asterisks indicate the significant differences (*p < 0.05, **p < 0.01).
CgSHP1-A and CgSHP1-B Are Both Associated With CaTBK1 and Inhibit CaTBK1-Induced Phosphorylation of CaMITA
To further decipher the relationship between Cgptpn6 and the RLR signaling pathway, Co-IP experiments were performed using CgSHP1-A-Flag and RLR cascades with the Myc tag (CaMAVS-Myc, CaMITA-Myc, CaTBK1-Myc and CaIRF3-Myc). The results clearly showed that CgSHP1-A was efficiently associated with CaMITA and CaTBK1, not with CaMAVS and CaIRF3 (Figure 6A). However, CgSHP1-B-Myc could only be efficiently pulled down by CaTBK1-Flag (Figure 6B). The interactions between CgSHP1-A and CgSHP1-B with CaTBK1 were confirmed by the reverse assays (Figures 6C, D). The subcellular locations of CgSHP1s and CaMITA or CaTBK1 were also monitored in EPC cells. Consistent with the findings of a previous report (61), CgSHP1-A and CgSHP1-B both localized in the cytosol, implying that SHP1 is an intracellular cytoplasmic signaling enzyme (Figures 6E, F). The colocalization results showed that the green fluorescence signals of CgSHP1-A or CgSHP1-B were uniformly overlapped with the red signals of CaTBK1, and partly overlapped with the CaMITA signals in the cytosol (Figures 6E, F).
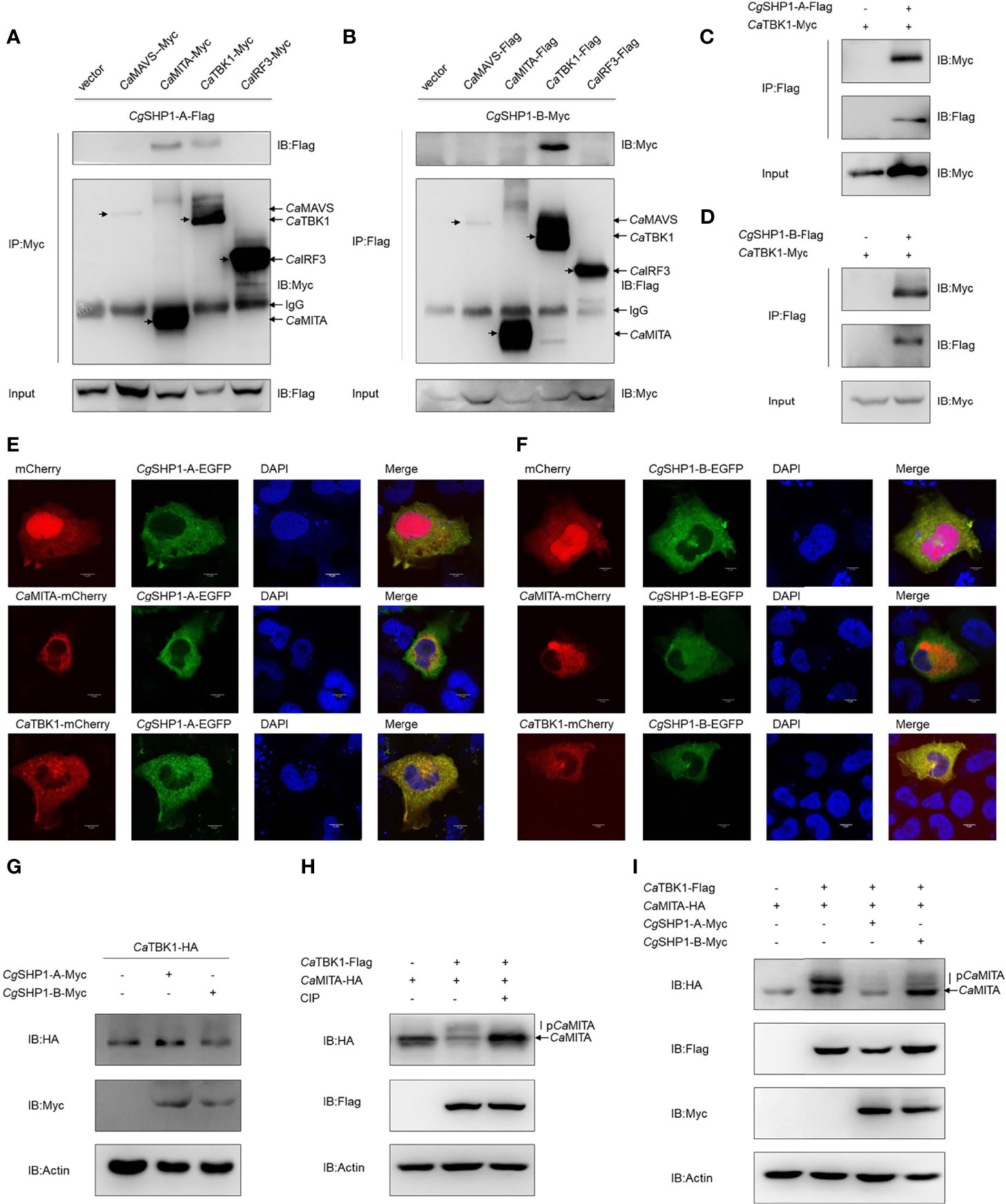
Figure 6 Interaction of CgSHP1s with CaTBK1 and CaMITA. (A–D) Co-IP analyses between CgSHP1s and CaTBK1, CaMITA in HEK 293T cells. (E, F) Subcellular localization of CgSHP1-A and CgSHP1-B with CaTBK1 and CaMITA in EPC cells. Scale bars = 5 μm. (G) CgSHP1-A and CgSHP1-B do not influence the expression of CaTBK1 in EPC cells. (H, I) Co-IP analyses reveal CaTBK1-mediated phosphorylation of CaMITA was inhibited by CIP (10 U) in HEK 293T cells (H), CgSHP1-A and CgSHP1-B decrease the phosphorylation of CaMITA induced by CaTBK1 (I). All experiments were repeated for at least three times with similar results.
Next, we investigated the protein changes to determine the effect of CgSHP1-A and CgSHP1-B on the CaTBK1. Overexpression of CgSHP1-A or CgSHP1-B exerted little influence on the expression of CaTBK1 (Figure 6G). Since SHP1 is known as protein tyrosine phosphatase, we speculated that CgSHP1-A and CgSHP1-B may affect the post-translational status of some downstream molecules phosphorylated by TBK1. We first confirmed that CaMITA was indeed phosphorylated by CaTBK1. When CaMITA was co-transfected with CaTBK1, weakly shifted bands with higher molecular weights were detected. As expected, these bands disappeared after treatment with CIP (Figure 6H). We subsequently investigated the role of CgSHP1-A and CgSHP1-B in CaTBK1 kinase activity. The phosphorylated CaMITA was reduced with overexpression of CgSHP1-A or CgSHP1-B. Interestingly, CgSHP1-A degrades unphosphorylated CaMITA (Figure 6I). Taken together, these data demonstrate that both CgSHP1-A and CgSHP1-B inhibit CaTBK1-induced phosphorylation of CaMITA.
CgSHP1-A Degrades CaMITA via an Autophagy Pathway
The interaction between CgSHP1-A and CaMITA was further confirmed by the reverse assay (Figure 7A). To determine the effect of CgSHP1-A on CaMITA, CgSHP1-A was cotransfected with CaMITA. Overexpression of CgSHP1-A caused a significant reduction of CaMITA in a dose-dependent manner (Figures 7B, C). Consistent with the no or very weak interaction between CgSHP1-B and CaMITA (Figure 6B), the overexpression of CgSHP1-B did not reduce CaMITA level (Figure 7B). A proteasome inhibitor (MG132) and an autophagy–lysosomal pathway inhibitor 3-methyladenine (3-MA) were used to examine the process underlying the CgSHP1-A-mediated CaMITA degradation. In comparison with the control (DMSO treatment) and MG132 groups, 3-MA could effectively block the degradation of CaMITA induced by CgSHP1-A in a dose-dependent manner (Figures 7D, E), implying that CgSHP1-A can degrade CaMITA via an autophagy-lysosomal pathway. To test this speculation, we preliminarily evaluated several autophagic components to identify which one could interact with CgSHP1-A. Co-IP assays showed that CgSHP1-A interacted with CaATG14 (Figure 7F). Similarly, the interaction between CaMITA and CaATG14 was also confirmed (Figure 7G). These data demonstrate that CgSHP1-A can degrade CaMITA probably through CaATG14-mediated autophagy signaling pathway.
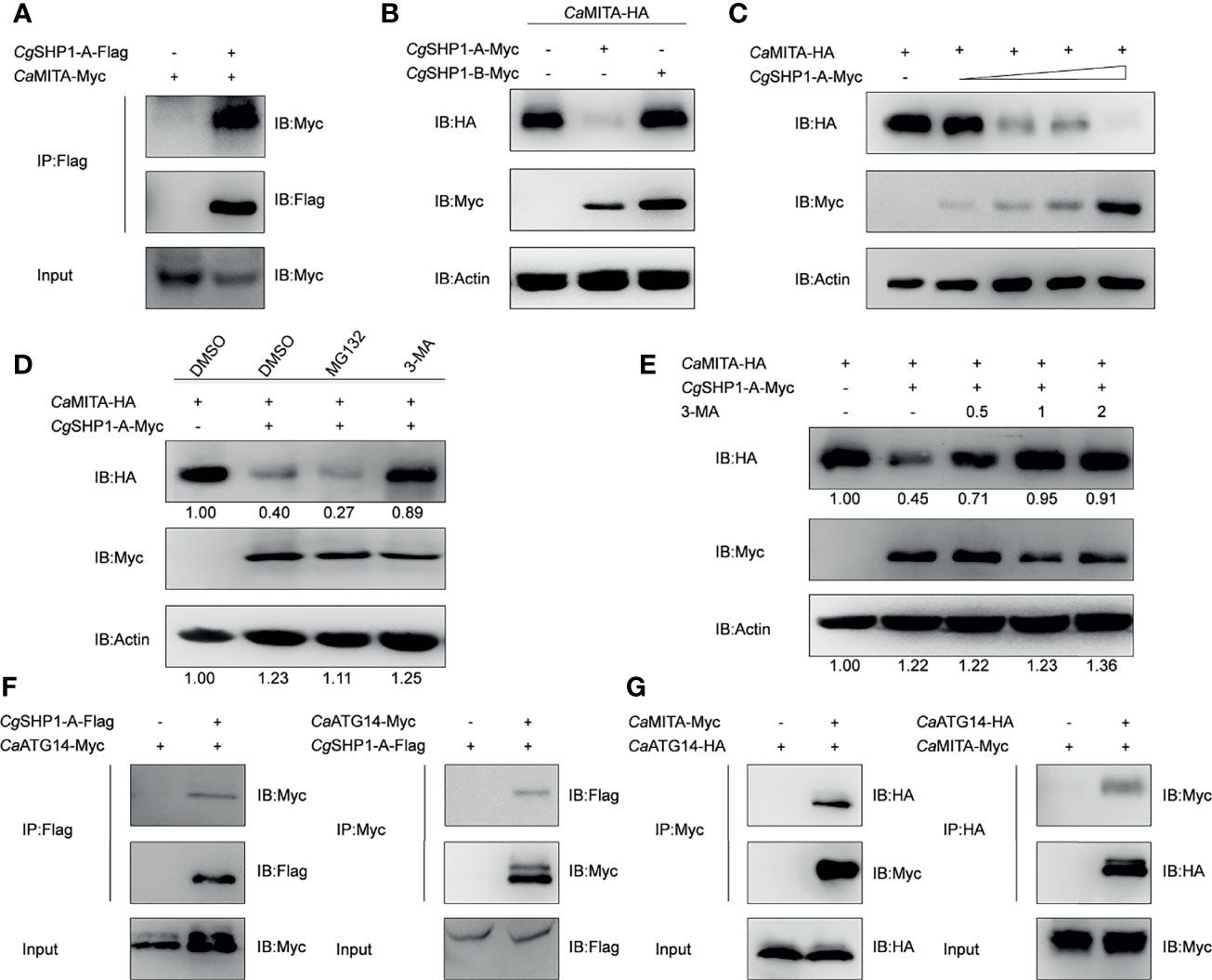
Figure 7 CgSHP1-A interacts with and degrades CaMITA by autophagy pathway. (A) Co-IP analysis between CgSHP1-A and CaMITA. (B–D) CgSHP1-A degrades CaMITA in a dose-dependent manner by autophagy pathway. 1.5 μg CaMITA-HA co-transfected with 1.5 µg CgSHP1s-Myc (B) and various concentration of CgSHP1-A-Myc (0.5 μg, or 1 μg, or 1.5 μg or 2 μg, empty vector was used to make up the rest) (C) in EPC cells. At 18 h post-transfection, the cells were treated with DMSO, MG132 and 3-MA for 6 h (D). The cell lysates were subjected to IB. Experiments were repeated for at least three times with similar results. (E) Effects of 3-MA on CgSHP1-A mediated destabilization of CaMITA. Transfection with the indicated expression vectors (2 μg/well) and treated with DMSO or 3-MA (0.5, 1, or 2 mM) for 6 h at 18 h post-transfection, the WCLs were analyzed by IB. (F, G) Co-IP of CgSHP1-A-Flag with CaATG14-Myc (F) or CaMITA-Myc with CaATG14-HA (G) in HEK 293T cells.
Both CgSHP1-A and CgSHP1-B Attenuate the Cellular Antiviral Response
Since CgSHP1-A and CgSHP1-B negatively regulate the IFN response, the modulation of CgSHP1 to the antiviral innate immune response was evaluated. In comparison with empty vector control infected with SVCV (MOI = 0.01), the overexpression of CgSHP1-A or CgSHP1-B in GiCB cells both resulted in an enhanced CPE (Figure 8A) and the viral titers increased about 104.5- and 103.94-fold respectively at 2 days post-infection (Figure 8B). In addition, the upregulated expression of Cgifn-A, Cgifn-B, Cgviperin-A and Cgviperin-B induced by SVCV were remarkably repressed by overexpression of CgSHP1-A or CgSHP1-B (Figure 8C). The inhibition effects of CgSHP1-A transfection were stronger than those of CgSHP1-B transfection. Meanwhile, more abundant transcripts of SVCV genes, n, p, m and g were detected in the CgSHP1-A overexpressed group (Figure 8D). These data indicate that both CgSHP1-A and CgSHP1-B negatively regulate the cellular antiviral response, in which CgSHP1-A is potentially dominant.
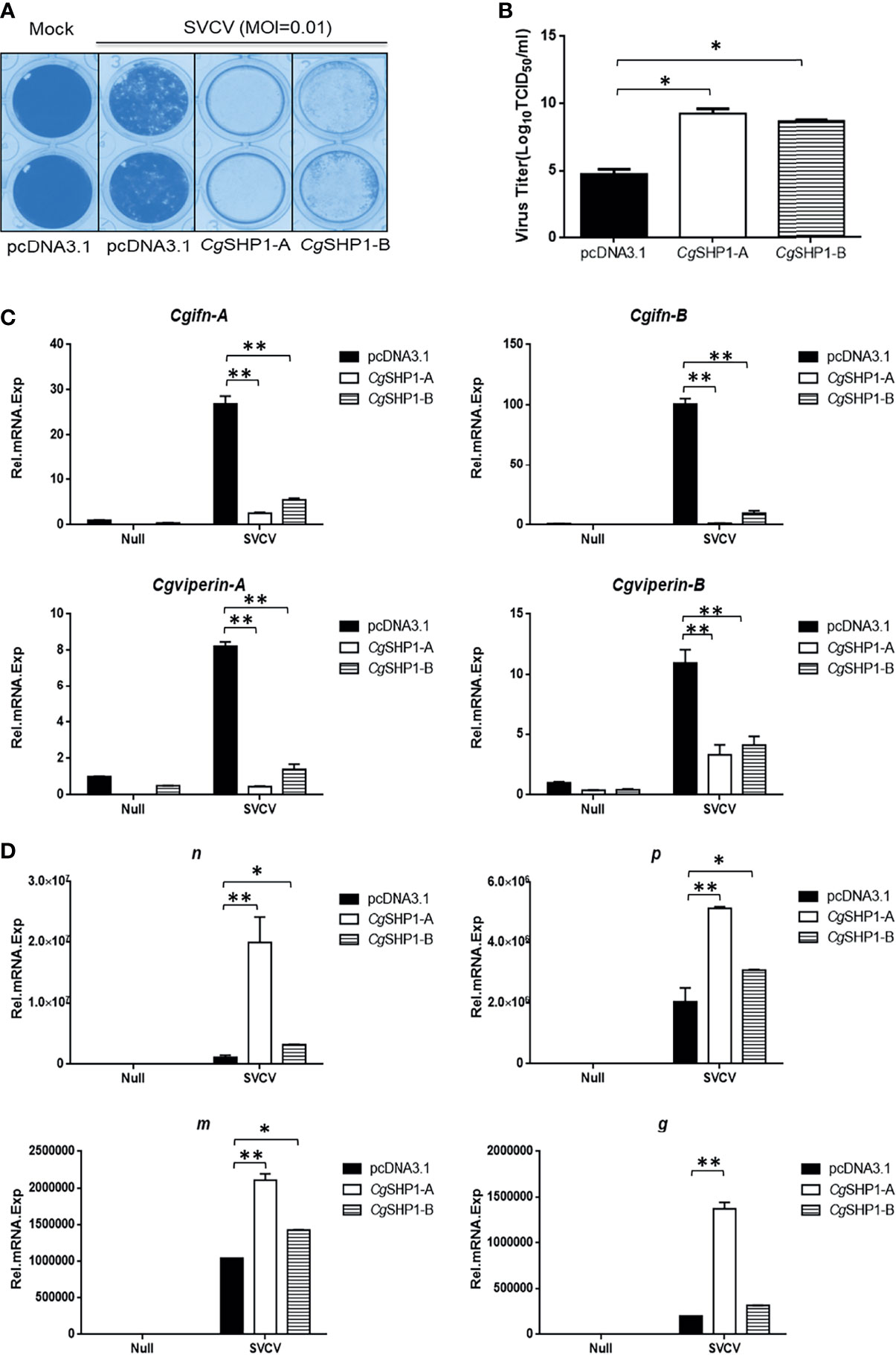
Figure 8 CgSHP1-A and CgSHP1-B attenuate the cellular antiviral response. (A, B) Enhance of virus replication by overexpression of CgSHP1-A or CgSHP1-B. GiCB cells were transfected with 0.5 μg pcDNA3.1-CgSHP1-A or CgSHP1-B or empty vector. At 24 h post-transfection, cells were infected with SVCV (MOI = 0.01) for 48 h (A). Viral titer was measured according to the method of Karber (B). (C) CgSHP1-A and CgSHP1-B inhibit the expression of Cgifn-A and Cgifn-B, Cgviperin-A and Cgviperin-B. GiCB cells were transfected with 2 μg pcDNA3.1-CgSHP1-A or CgSHP1-B or empty vector. At 24 h post-transfection, cells were untreated or infected with SVCV (MOI = 1). After 24 h infection, the WCLs were detected for qPCR analysis. (D) The mRNA levels of cellular n, p, m and g. The same samples were prepared similarly as described above for (C). The relative transcriptional levels were normalized to the transcriptional level of the eef1a1l1 gene and were represented as fold induction relative to the transcriptional level in the control cells. Data are expressed as mean ± SEM, n = 3. Asterisks indicate significant differences from control values (* p < 0.05, **p < 0.01).
Discussion
Protein tyrosine phosphorylation, an important post-translational modification, is necessary for normal immune regulation and occurs under the strict control of PTKs-PTPs (1, 62, 63). Because of the variable activities and poor substrate specificity of PTPs, research on these molecules has seriously lagged behind PTKs, and they have only recently begun to attract considerable attention as potential therapeutic targets (4). One of our previous studies on gibel carp disease resistance breeding showed that SHP1 can be recruited to inhibitory immune receptor DICPs, which could inhibit the expression of IFN and ISGs (22). In this study, we first identified two diverged gibel carp ptpn6 homeologs and observed the dominant expression of Cgptpn6-A. Then, we revealed that CgSHP1-A and CgSHP1-B both negatively regulate the IFN response through the RLR signaling pathway. Finally, we identified the dominant role of CgSHP1-A in negatively regulating cellular antiviral response.
One of the most interesting consequences of hybridization and polyploidization is the diversification of duplicated genes. Allopolyploids, which arise from interspecific hybridization, possess duplicated gene copies (64). Because gibel carp is derived from a common allotetraploid ancestor by autotriploidy about 0.8 million years ago, it possesses the same haplotype (A+B) as curcian carp. It means that gibel carp and crucian carp generally have the very high similar genes but the former has one more allele. Consistently, gibel carp also showed two ptpn6 homeologs with about 90% identity, and each of homeologs possesses three alleles with identities above 99% (Supplementary Figure 1). Together with other conserved genes (i.e., dmrt1, foxl2, viperin, nanos2 and bmp15), the phylogeny of Cgptpn6 confirmed the assumption that gibel carp and crucian carp are derived from a common allotetraploid ancestor, and a subsequent autotriploidy event drove the speciation of gibel carp.
Under relaxed purifying selection, duplicated homeologs may step into different evolutionary trajectories: co-retained or fractionated (one of the duplicated genes is either retained or deleted) (33). Approximately 60% of the analyzed homologous genes in zebrafish chromosome 16 were co-retained both in gibel carp chromosome CgA16 and CgB16, while the others had fractionated. In addition, pon1, cd27, and mfap5 were also located neither in CaA16 nor in CaB16, implying that they might have been lost in the ancestor of Carassius complex after an allotetraploidy event. Relative to CaA16, a gene block (lpcat3-clstns-pex5-p3h3) is not in CgA16 (Figure 1C), which suggests that it might have been deleted in gibel carp after divergence from crucian carp. Similar to the results observed in zebrafish and Nile tilapia (23, 24), Cgptpn6s is ubiquitously expressed in the analyzed tissues and abundantly in immune tissues (i.e., spleen, head kidney, and thymus) (Figure 2). Numerous examples have proven that homeolog biased expression seems to be a rule rather than an exception (30). In our previous studies, homeolog bias occurred differently in different tissues (35, 36, 50); for example, Cgviperin-A was expressed higher than Cgviperin-B in the spleen and liver, whereas in the gill, the transcripts of Cgviperin-B were more abundant than Cgviperin-A (36). However, Cgptpn6-A was dominant in the 12 adult tissues (Figure 2), and poly I:C, poly dA:dT, and SVCV all induced higher upregulated expression of Cgptpn6-A relative to Cgptpn6-B (Figure 3). The expression dominance of Cgptpn6-A implies its leading role in immune regulation.
As the first defense line, the innate immune system, including IFNs, plays vital roles against invasive pathogens (65, 66) and is tightly regulated by complex mechanisms that prevent excessive inflammation and autoimmunity (67–69). SHP1 is known to be a major regulator in this process (70, 71). For example, the inducible deletion of Ptpn6 led to an increase in IFNγ expression in the Ptpn6fl/flERT2-Cre mouse (72). Epstein-Barr virus (EBV) Tegument protein BGLF2 facilitates the recruitment of SHP1 to STAT1, which reduces STAT1 phosphorylation and thereby the induction of IFN and ISGs in HEK293 cells (73). The overexpression of mouse SHP-1 in L929 cells markedly reduced the phosphorylation of several critical signaling regulators (i.e., TBK1, IRF3, STAT1, p65, p38, and Erk) and thereby inhibited type I IFN production in response to vesicular stomatitis virus infection (74). However, IFN-β induced by poly (I:C) was significantly impaired in the splenocytes of SHP-1-deficient mouse both in vitro and in vivo (75). In this study, the overexpression of CgSHP1-A or CgSHP1-B both inhibited the IFN response stimulated by poly I:C, poly dA:dT, and SVCV (Figure 4), indicating that fish SHP1 is also a critical negative factor for IFN. The upregulated expression of Cgirf3s were more significantly decreased by the CgSHP1-A or CgSHP1-B overexpression induced by poly dA:dT than poly I:C (Figure 5). Poly dA:dT has been reported to trigger not only the RIG-I pathway but also the cGAS-STING/MITA pathway to induce type I IFN (76). Besides, MITA as the target of CgSHP1-A participates in both cytoplasmic RNA- and DNA-triggered signaling pathways that converge on the TBK1-IRF3 axis in different molecular mechanisms (77). Therefore, we speculate that overexpression of CgSHP1-A or CgSHP1-B could more significantly reduce the increased expression of Cgirf3s triggered by poly dA:dT through two pathways. Moreover, overexpression of CgSHP1-A or CgSHP1-B both promoted SVCV proliferation, and the overexpression of CgSHP1-A had a more powerful effect on the suppression of IFN response than that of CgSHP1-B, while CPE in the GiCB transfected with CgSHP1-A was more obvious than that of CgSHP1-B (Figure 8). These results indicate that CgSHP1-A and CgSHP1-B negatively regulate gibel carp antiviral activities, in which the former plays a dominant role.
The importance of SHP1 has been implicated in various signaling events in mammals, including adaptive immunity pathways such as the T cell receptor (TCR) and BCR signaling pathway (78, 79), and innate immunity pathways, including Janus kinase-signal transducer and activator of transcription (JAK-STAT), phosphatidylinositol 3-kinase (PI3-K)/activation of protein kinase B (Akt), mitogen-activated protein kinases (MAPKs), and transcription nuclear factor (NF-κB) pathways, and Toll-like receptor (TLR) signaling pathway (13, 15, 73, 75, 80–82). For example, SHP-1 inhibited the TLR-mediated proinflammatory cytokine production by repressing the activation of MAPKs and NF-κB, but it increased TLR- and RIG-I-activated IFN-β production by inhibiting IRAK1 activation in mouse splenocytes after VSV infection (75). In this study, we found that both CgSHP1-A and CgSHP1-B were associated with CaTBK1 and could inhibit CaTBK1-induced phosphorylation of CaMITA, and CgSHP1-A degrades unphosphorylated CaMITA (Figure 6). The activation of the fish IFN response has been well-characterized (65, 83, 84). Similar to the process in mammals, viral products are recognized by TLRs or RLRs and then trigger an IRF3/7-dependent IFN response. In the RLR-activated IFN signaling cascade, members of the RLR family, such as RIG-I, interacts with MAVS that subsequently associates with TBK1 and MITA, which enables the phosphorylation of IRF3/7 for translocating into the nucleus and then triggering the production of IFNβ (60, 65). TBK1 and MITA are strictly regulated to achieve a coordinated response, and several negative regulatory molecules for these factors have been identified. For example, zebrafish major vault protein (MVP) inhibits IFN production through recruitment and degradation of TBK1 in a lysosome-dependent manner (85), and transmembrane protein 33 (TMEM33) acts as a competitive substrate of TBK1 to reduce MITA/IRF3 phosphorylation (86). According to previous report, the C-terminal domain of SHP2, which has a similar structure to SHP1, directly bounds TBK1 by interacting with the kinase domain of TBK1 (87). Therefore, we speculate that CgSHP1-A and CgSHP1-B might also interact with CaTBK1 via the kinase domain of CaTBK1 and inhibit the kinase domain of CaTBK1-induced phosphorylation of CaMITA. Since TBK1 is a serine/threonine-kinase and its induced phosphorylation is not tyrosine phosphorylation (88, 89), the inhibition of CaTBK1-induced phosphorylation by CgSHP1s may be independent of its tyrosine phosphatase activity, which requires further investigation. Interestingly, we observed that only CgSHP1-A could degrade CaMITA via an autophagy pathway (Figure 7). Autophagy is one of major cellular protein degradation pathways to decompose misfolded/unfolded proteins or invading cytoplasmic organisms in eukaryotes (90, 91). Many recent studies have reported that autophagy has a negative influence on type I IFN signaling pathways (92–95). Taken together, the differential expression pattern and regulative mechanisms indicate that CgSHP1-A and CgSHP1-B might have sub-functionalized and that CgSHP1-A overwhelmingly dominates CgSHP1-B. Besides the coding sequences, mutations in cis-elements may have also led to the subfunctionalization of duplicated genes (33, 96). Further research will be required to identify the distinct motifs or sites between Cgptpn6-A and Cgptpn6-B, including coding sequences and promoters, which will result in their differential expression and regulative mechanisms.
On the basis of these results, we propose a schematic diagram for the cooperatively and negatively regulative mechanisms of CgSHP1-A and CgSHP1-B in RLR-mediated IFN response (Figure 9). In response to SVCV infection, more abundant CgSHP1-A is expressed in relative to CgSHP1-B. In addition to the inhibition of CaTBK1-induced phosphorylation of CaMITA shared with CgSHP1-A and CgSHP1-B, CgSHP1-A also interacts with CaMITA and triggers autophagic degradation of CaMITA. In the fight between fish and aquatic viruses, the ability to mount a properly strong immune reaction is crucial for host survival and health (97, 98). The current findings support that fish SHP1 acts as a negative regulator of RLR-mediated IFN response, which not only sheds light on the functions and regulative mechanism of fish SHP1, but also provides a target gene to breed gibel carp with higher disease-resistance through CRISPR/Cas9 editing. Meanwhile, the above data also provide a typical case of homeolog/allele diversification, biased expression, and sub-functionalization in the evolution of duplicated genes.
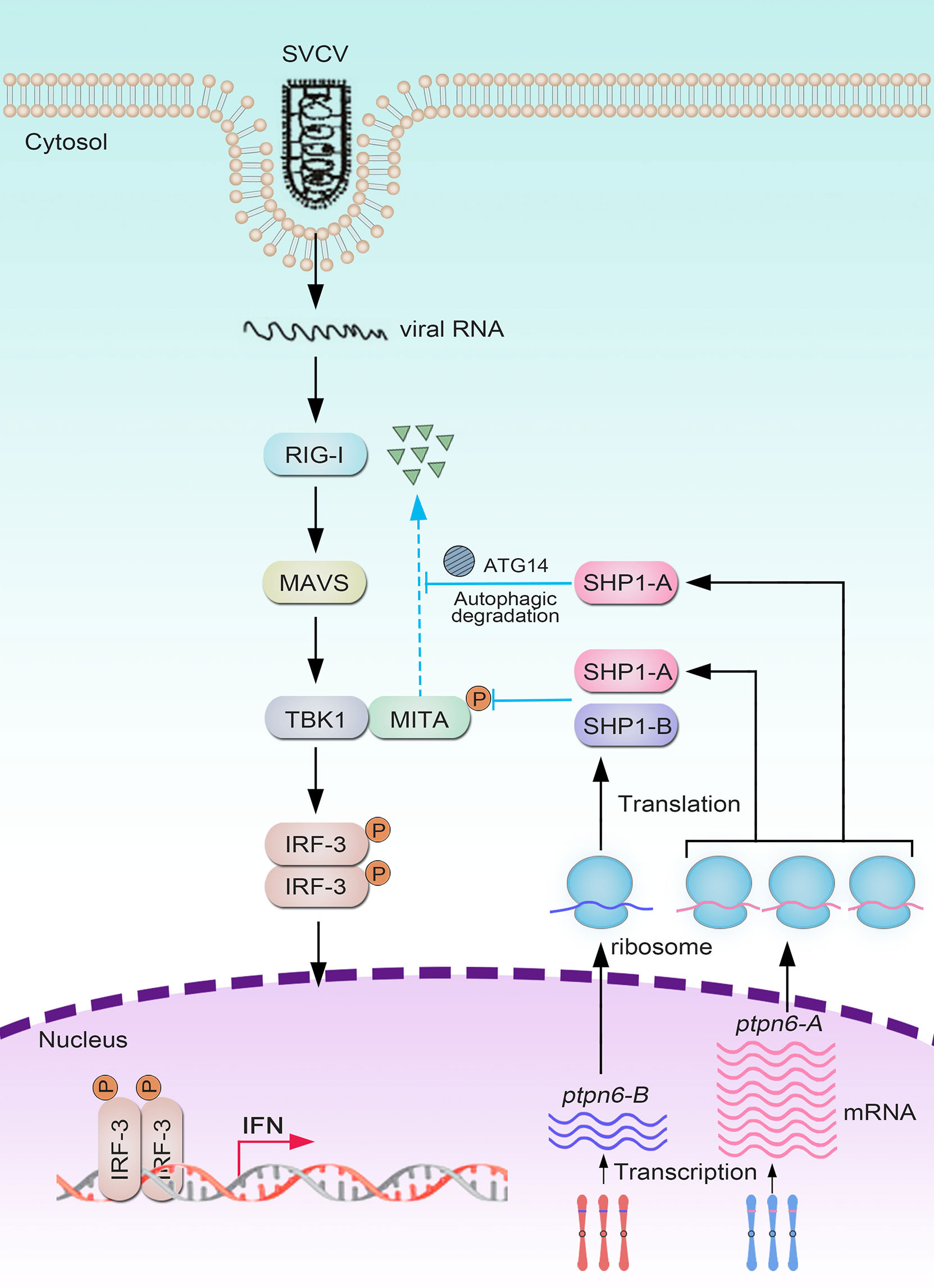
Figure 9 Divergent mechanisms of Cgptpn6-A and Cgptpn6-B in negatively regulating RLR-mediated signaling pathways.
Data Availability Statement
The original contributions presented in the study are included in the article/Supplementary Material. Further inquiries can be directed to the corresponding authors.
Ethics Statement
The animal study was reviewed and approved by the Institutional Animal Care and Use Committee of IHB, CAS (protocol number 2016-018).
Author Contributions
J-FG, YW, LZ, and J-FT designed the study. J-FT, SL, L-FL, Z-CL, ZL, R-HG, C-YM, Q-YZ, Z-WW, and X-JZ prepared the samples and carried out the experiments. J-FT, LZ, J-FG, YW, and SL analyzed and discussed the results. LZ, J-FG, SL, YW, and J-FT wrote the paper. All authors contributed to the article and approved the submitted version.
Funding
This work was supported by the Strategic Priority Research Program of the Chinese Academy of Sciences (XDB31000000, XDA24030203 and XDA24030104), the National Natural Science Foundation (31930111) and China Agriculture Research System of MOF and MARA. The funding bodies had no role in the design of the study and collection, analysis, and interpretation of data and in writing the manuscript.
Conflict of Interest
The authors declare that the research was conducted in the absence of any commercial or financial relationships that could be construed as a potential conflict of interest.
Publisher’s Note
All claims expressed in this article are solely those of the authors and do not necessarily represent those of their affiliated organizations, or those of the publisher, the editors and the reviewers. Any product that may be evaluated in this article, or claim that may be made by its manufacturer, is not guaranteed or endorsed by the publisher.
Acknowledgments
The research was supported by the Analytical & Testing Center, IHB, CAS and Wuhan Branch, Supercomputing Centre, CAS, China.
Supplementary Material
The Supplementary Material for this article can be found online at: https://www.frontiersin.org/articles/10.3389/fimmu.2021.780667/full#supplementary-material
Supplementary Figure 1 | Multiple nucleotide sequence alignment of six Cgptpn6 transcripts from gibel carp clone F. ORF is highlighted by red box (A). Phylogenetic tree of six Cgptpn6 transcripts in gibel carp (B).
Supplementary Figure 2 | Multiple amino acid sequence alignment of CgSHP1 from gibel carp clone F with other vertebrate SHP1 proteins (A). Phylogenetic tree of SHP1 proteins in vertebrates (B).
Supplementary Table 1 | Primers used in this study.
References
1. Garg M, Wahid M, Khan F. Regulation of Peripheral and Central Immunity: Understanding the Role of Src Homology 2 Domain-Containing Tyrosine Phosphatases, SHP-1 & SHP-2. Immunobiology (2020) 225:151847. doi: 10.1016/j.imbio.2019.09.006
2. Poole AW, Jones ML. A SHPing Tale: Perspectives on the Regulation of SHP-1 and SHP-2 Tyrosine Phosphatases by the C-Terminal Tail. Cell Signal (2005) 17:1323–32. doi: 10.1016/j.cellsig.2005.05.016
3. Mustelin T, Vang T, Bottini N. Protein Tyrosine Phosphatases and the Immune Response. Nat Rev Immunol (2005) 5:43–57. doi: 10.1038/nri1530
4. Varone A, Spano D, Corda D. Shp1 in Solid Cancers and Their Therapy. Front Oncol (2020) 10:935. doi: 10.3389/fonc.2020.00935
5. Neel BG, Gu H, Pao L. The 'Shp'ing News: SH2 Domain-Containing Tyrosine Phosphatases in Cell Signaling. Trends Biochem Sci (2003) 28:284–93. doi: 10.1016/S0968-0004(03)00091-4
6. Tsui FWL, Martin A, Wang J, Tsui HW. Investigations Into the Regulation and Function of the SH2 Domain-Containing Protein-Tyrosine Phosphatase, SHP-1. Immunol Res (2006) 35:127–36. doi: 10.1385/IR:35:1:127
7. Pao LI, Lam KP, Henderson JM, Kutok JL, Alimzhanov M, Nitschke L, et al. B Cell-Specific Deletion of Protein-Tyrosine Phosphatase Shp1 Promotes B-1a Cell Development and Causes Systemic Autoimmunity. Immunity (2007) 27:35–48. doi: 10.1016/j.immuni.2007.04.016
8. Li YF, Xu S, Ou X, Lam KP. Shp1 Signalling is Required to Establish the Long-Lived Bone Marrow Plasma Cell Pool. Nat Commun (2014) 5:4273. doi: 10.1038/ncomms5273
9. Johnson DJ, Pao LI, Dhanji S, Murakami K, Ohashi PS, Neel BG. Shp1 Regulates T Cell Homeostasis by Limiting IL-4 Signals. J Exp Med (2013) 210:1419–31. doi: 10.1084/jem.20122239
10. Kaneko T, Saito Y, Kotani T, Okazawa H, Iwamura H, Sato-Hashimoto M, et al. Dendritic Cell-Specific Ablation of the Protein Tyrosine Phosphatase Shp1 Promotes Th1 Cell Differentiation and Induces Autoimmunity. J Immunol (2012) 188:5397–407. doi: 10.4049/jimmunol.1103210
11. Abram CL, Roberge GL, Pao LI, Neel BG, Lowell CA. Distinct Roles for Neutrophils and Dendritic Cells in Inflammation and Autoimmunity in Motheaten Mice. Immunity (2013) 38:489–501. doi: 10.1016/j.immuni.2013.02.018
12. Huang TT, Su JC, Liu CY, Shiau CW, Chen KF. Alteration of SHP-1/P-STAT3 Signaling: A Potential Target for Anticancer Therapy. Int J Mol Sci (2017) 18:1234. doi: 10.3390/ijms18061234
13. Chong ZZ, Maiese K. The Src Homology 2 Domain Tyrosine Phosphatases SHP-1 and SHP-2: Diversified Control of Cell Growth, Inflammation, and Injury. Histol Histopathol (2007) 22:1251–67. doi: 10.14670/HH-22.1251
14. Geraldes P, Hiraoka-Yamamoto J, Matsumoto M, Clermont A, Leitges M, Marette A, et al. Activation of PKC-Delta and SHP-1 by Hyperglycemia Causes Vascular Cell Apoptosis and Diabetic Retinopathy. Nat Med (2009) 15:1298–306. doi: 10.1038/nm.2052
15. Jiang M, Ye J, Wang X, Li N, Wang Y, Shi Y. Phosphatase SHP1 Impedes Mesenchymal Stromal Cell Immunosuppressive Capacity Modulated by JAK1/STAT3 and P38 Signals. Cell Biosci (2020) 10:65. doi: 10.1186/s13578-020-00428-w
16. Zhang J, Somani AK, Siminovitch KA. Roles of the SHP-1 Tyrosine Phosphatase in the Negative Regulation of Cell Signalling. Semin Immunol (2000) 12:361–78. doi: 10.1006/smim.2000.0223
17. Neel M BG, Chen HE, Goelz S, Larner AC, Neel BG. Differential Regulation of the Alpha/Beta Interferon-Stimulated Jak/Stat Pathway by the SH2 Domain-Containing Tyrosine Phosphatase SHPTP1. Mol Cell Biol (1995) 15:7050–8. doi: 10.1128/mcb.15.12.7050
18. Kim M, Morales LD, Jang IS, Cho YY, Kim DJ. Protein Tyrosine Phosphatases as Potential Regulators of STAT3 Signaling. Int J Mol Sci (2018) 19:2708. doi: 10.3390/ijms19092708
19. Montgomery BC, Cortes HD, Burshtyn DN, Stafford JL. Channel Catfish Leukocyte Immune-Type Receptor Mediated Inhibition of Cellular Cytotoxicity is Facilitated by SHP-1-Dependent and -Independent Mechanisms. Dev Comp Immunol (2012) 37:151–63. doi: 10.1016/j.dci.2011.09.005
20. Montgomery BC, Mewes J, Davidson C, Burshtyn DN, Stafford JL. Cell Surface Expression of Channel Catfish Leukocyte Immune-Type Receptors (IpLITRs) and Recruitment of Both Src Homology 2 Domain-Containing Protein Tyrosine Phosphatase (SHP)-1 and SHP-2. Dev Comp Immunol (2009) 33:570–82. doi: 10.1016/j.dci.2008.10.006
21. Yu C, Ehrhardt GR, Alder MN, Cooper MD, Xu A. Inhibitory Signaling Potential of a TCR-Like Molecule in Lamprey. Eur J Immunol (2009) 39:571–9. doi: 10.1002/eji.200838846
22. Gao FX, Lu WJ, Wang Y, Zhang QY, Zhang YB, Mou CY, et al. Differential Expression and Functional Diversification of Diverse Immunoglobulin Domain-Containing Protein (DICP) Family in Three Gynogenetic Clones of Gibel Carp. Dev Comp Immunol (2018) 84:396–407. doi: 10.1016/j.dci.2018.03.013
23. Kanwal Z, Zakrzewska A, den Hertog J, Spaink HP, Schaaf MJ, Meijer AH. Deficiency in Hematopoietic Phosphatase Ptpn6/Shp1 Hyperactivates the Innate Immune System and Impairs Control of Bacterial Infections in Zebrafish Embryos. J Immunol (2013) 190:1631–45. doi: 10.4049/jimmunol.1200551
24. Wu L, Gao A, Lei Y, Li J, Mai K, Ye J. SHP1 Tyrosine Phosphatase Gets Involved in Host Defense Against Streptococcus Agalactiae Infection and BCR Signaling Pathway in Nile Tilapia (Oreochromis Niloticus). Fish Shellfish Immun (2020) 99:562–71. doi: 10.1016/j.fsi.2020.02.026
25. Amores A, Force A, Yan YL, Joly L, Amemiya C, Fritz A, et al. Zebrafish Hox Clusters and Vertebrate Genome Evolution. Science (1998) 282:1711–4. doi: 10.1126/science.282.5394.1711
26. Dehal P, Boore JL. Two Rounds of Whole Genome Duplication in the Ancestral Vertebrate. PloS Biol (2005) 3:e314. doi: 10.1371/journal.pbio.0030314
27. Van de Peer Y, Maere S, Meyer A. The Evolutionary Significance of Ancient Genome Duplications. Nat Rev Genet (2009) 10:725–32. doi: 10.1038/nrg2600
28. Van de Peer Y, Mizrachi E, Marchal K. The Evolutionary Significance of Polyploidy. Nat Rev Genet (2017) 18:411–24. doi: 10.1038/nrg.2017.26
29. Fox DT, Soltis DE, Soltis PS, Ashman TL, Van de Peer Y. Polyploidy: A Biological Force From Cells to Ecosystems. Trends Cell Biol (2020) 30:688–94. doi: 10.1016/j.tcb.2020.06.006
30. Nieto FG, Casacuberta J, Wendel JF. Genomics of Evolutionary Novelty in Hybrids and Polyploids. Front Genet (2020) 11:792. doi: 10.3389/fgene.2020.00792
31. Mable BK, Alexandrou MA, Taylor MI. Genome Duplication in Amphibians and Fish: An Extended Synthesis. J Zoology (2011) 284:151–82. doi: 10.1111/j.1469-7998.2011.00829.x
32. Zhou L, Gui JF. Natural and Artificial Polyploids in Aquaculture. Aquaculture Fisheries (2017) 2:103–11. doi: 10.1016/j.aaf.2017.04.003
33. Cheng F, Wu J, Cai X, Liang J, Freeling M, Wang X. Gene Retention, Fractionation and Subgenome Differences in Polyploid Plants. Nat Plants (2018) 4:258–68. doi: 10.1038/s41477-018-0136-7
34. Liu S, Li Z, Gui JF. Fish-Specific Duplicated Dmrt2b Contributes to a Divergent Function Through Hedgehog Pathway and Maintains Left-Right Asymmetry Establishment Function. PloS One (2009) 4:e7261. doi: 10.1371/journal.pone.0007261
35. Gan RH, Wang Y, Li Z, Yu ZX, Li XY, Tong JF, et al. Functional Divergence of Multiple Duplicated Foxl2 Homeologs and Alleles in a Recurrent Polyploid Fish. Mol Biol Evol (2021) 38:1995–2013. doi: 10.1093/molbev/msab002
36. Mou CY, Li S, Lu LF, Wang Y, Yu P, Li Z, et al. Divergent Antiviral Mechanisms of Two Viperin Homeologs in a Recurrent Polyploid Fish. Front Immunol (2021) 12:702971. doi: 10.3389/fimmu.2021.702971
37. Conant GC, Birchler JA, Pires JC. Dosage, Duplication, and Diploidization: Clarifying the Interplay of Multiple Models for Duplicate Gene Evolution Over Time. Curr Opin Plant Biol (2014) 19:91–8. doi: 10.1016/j.pbi.2014.05.008
38. Liu XL, Jiang FF, Wang ZW, Li XY, Li Z, Zhang XJ, et al. Wider Geographic Distribution and Higher Diversity of Hexaploids Than Tetraploids in Carassius Species Complex Reveal Recurrent Polyploidy Effects on Adaptive Evolution. Sci Rep (2017) 7:5395. doi: 10.1038/s41598-017-05731-0
39. Liu XL, Li XY, Jiang FF, Wang ZW, Li Z, Zhang XJ, et al. Numerous Mitochondrial DNA Haplotypes Reveal Multiple Independent Polyploidy Origins of Hexaploids in Carassius Species Complex. Ecol Evol (2017) 7:10604–15. doi: 10.1002/ece3.3462
40. Sakai H, Iguchi K, Yamazaki Y, Sideleva VG, Goto A. Morphological and mtDNA Sequence Studies on Three Crucian Carps (Carassius: Cyprinidae) Including a New Stock From the Ob River System, Kazakhstan. J Fish Biol (2009) 74:1756–73. doi: 10.1111/j.1095-8649.2009.02203.x
41. Takada M, Tachihara K, Kon T, Yamamoto G, Iguchi K, Miya M, et al. Biogeography and Evolution of the Carassius Auratus-Complex in East Asia. BMC Evol Biol (2010) 10:7. doi: 10.1186/1471-2148-10-7
42. Gui JF, Zhou L. Genetic Basis and Breeding Application of Clonal Diversity and Dual Reproduction Modes in Polyploid Carassius Auratus Gibelio. Sci China Life Sci (2010) 53:409–15. doi: 10.1007/s11427-010-0092-6
43. Ding M, Li X, Zhu Z, Chen J, Lu M, Shi Q, et al. Genomic Anatomy of Male-Specific Microchromosomes in a Gynogenetic Fish. PloS Genet (2021) 17:e1009760. doi: 10.1371/journal.pgen.1009760
44. Lu M, Li XY, Li Z, Du WX, Zhou L, Wang Y, et al. Regain of Sex Determination System and Sexual Reproduction Ability in a Synthetic Octoploid Male Fish. Sci China Life Sci (2021) 64:77–87. doi: 10.1007/s11427-020-1694-7
45. Yu P, Wang Y, Yang WT, Li Z, Zhang XJ, Zhou L, et al. Upregulation of the PPAR Signaling Pathway and Accumulation of Lipids are Related to the Morphological and Structural Transformation of the Dragon-Eye Goldfish Eye. Sci China Life Sci (2021) 64:1031–49. doi: 10.1007/s11427-020-1814-1
46. Gui JF LZ, Li XY. Rethinking Fish Biology and Biotechnologies in the Challenge Era for Burgeoning Genome Resources and Strengthening Food Security. Water Biol Secur (2022) 1:1–17. doi: 10.1016/j.watbs.2021.11.001
47. Li XY, Zhang XJ, Li Z, Hong W, Liu W, Zhang J, et al. Evolutionary History of Two Divergent Dmrt1 Genes Reveals Two Rounds of Polyploidy Origins in Gibel Carp. Mol Phylogenet Evol (2014) 78:96–104. doi: 10.1016/j.ympev.2014.05.005
48. Zhang QQ, Zhou L, Li Z, Gan RH, Yu ZX, Gui JF, et al. Allelic Diversification, Syntenic Alignment and Expression Patterns of Nanos2 in Polyploid Gibel Carp. Acta Hydrobiologica Sinica (2020) 44:1087–96. doi: 10.7541/2020.126
49. Jiang SY, Wang Y, Zhou L, Chen F, Li Z, Gui JF. Molecular Characteristics, Genomic Structure and Expression Patterns of Diverse Bmp15 Alleles in Polyploid Gibel Carp Clone F. Acta Hydrobiologica Sinica (2020) 44:518–27. doi: 10.7541/2020.063
50. Lu WJ, Zhou L, Gao FX, Zhou YL, Li Z, Zhang XJ, et al. Dynamic and Differential Expression of Duplicated Cxcr4/Cxcl12 Genes Facilitates Antiviral Response in Hexaploid Gibel Carp. Front Immunol (2020) 11:2176. doi: 10.3389/fimmu.2020.02176
51. Gao FX, Wang Y, Zhang QY, Mou CY, Li Z, Deng YS, et al. Distinct Herpesvirus Resistances and Immune Responses of Three Gynogenetic Clones of Gibel Carp Revealed by Comprehensive Transcriptomes. BMC Genomics (2017) 18:561. doi: 10.1186/s12864-017-3945-6
52. Mou CY, Wang Y, Zhang QY, Gao FX, Li Z, Tong JF, et al. Differential Interferon System Gene Expression Profiles in Susceptible and Resistant Gynogenetic Clones of Gibel Carp Challenged With Herpesvirus. CaHV Dev Comp Immunol (2018) 86:52–64. doi: 10.1016/j.dci.2018.04.024
53. Lu WJ, Gao FX, Wang Y, Zhang QY, Li Z, Zhang XJ, et al. Differential Expression of Innate and Adaptive Immune Genes in the Survivors of Three Gibel Carp Gynogenetic Clones After Herpesvirus Challenge. BMC Genomics (2019) 20:432. doi: 10.1186/s12864-019-5777-z
54. Chen J, Zhou XY, Li P, Li ZC, Zhang C, Sun YH, et al. Molecular Characterization of a Cyprinid Fish (Ancherythroculter Nigrocauda) TBK1 and its Kinase Activity in IFN Regulation. Dev Comp Immunol (2021) 114:103805. doi: 10.1016/j.dci.2020.103805
55. Ma J, Jiang N, LaPatra SE, Jin L, Xu J, Fan Y, et al. Establishment of a Novel and Highly Permissive Cell Line for the Efficient Replication of Cyprinid Herpesvirus 2 (CyHV-2). Vet Microbiol (2015) 177:315–25. doi: 10.1016/j.vetmic.2015.04.006
56. Ashraf U, Lu Y, Lin L, Yuan J, Wang M, Liu X. Spring Viraemia of Carp Virus: Recent Advances. J Gen Virol (2016) 97:1037–51. doi: 10.1099/jgv.0.000436
57. Kumar S, Stecher G, Tamura K. MEGA7: Molecular Evolutionary Genetics Analysis Version 7.0 for Bigger Datasets. Mol Biol Evol (2016) 33:1870–4. doi: 10.1093/molbev/msw054
58. Zhu HP, Ma DM, Gui JF. Triploid Origin of the Gibel Carp as Revealed by 5S rDNA Localization and Chromosome Painting. Chromosome Res (2006) 14:767–76. doi: 10.1007/s10577-006-1083-0
59. Chen J, Li ZC, Lu LF, Li P, Li XY, Li S. Functional Characterization of Dark Sleeper (Odontobutis Obscura) TBK1 on IFN Regulation. Front Immunol (2019) 10:985. doi: 10.3389/fimmu.2019.00985
60. Chen SN, Zou PF, Nie P. Retinoic Acid-Inducible Gene I (RIG-I)-Like Receptors (RLRs) in Fish: Current Knowledge and Future Perspectives. Immunology (2017) 151:16–25. doi: 10.1111/imm.12714
61. Abram CL, Lowell CA. Shp1 Function in Myeloid Cells. J Leukoc Biol (2017) 102:657–75. doi: 10.1189/jlb.2MR0317-105R
62. Simoncic PD, Lee-Loy A, Barber DL, Tremblay ML, McGlade CJ. The T Cell Protein Tyrosine Phosphatase is a Negative Regulator of Janus Family Kinases 1 and 3. Curr Biol (2002) 12:446–53. doi: 10.1016/S0960-9822(02)00697-8
63. Motiwala T, Jacob ST. Role of Protein Tyrosine Phosphatases in Cancer. Prog Nucleic Acid Res Mol Biol (2006) 81:297–329. doi: 10.1016/S0079-6603(06)81008-1
64. Kuo TCY, Hatakeyama M, Tameshige T, Shimizu KK, Sese J. Homeolog Expression Quantification Methods for Allopolyploids. Brief Bioinform (2020) 21:395–407. doi: 10.1093/bib/bby121
65. Zhang YB, Gui JF. Molecular Regulation of Interferon Antiviral Response in Fish. Dev Comp Immunol (2012) 38:193–202. doi: 10.1016/j.dci.2012.06.003
66. Feng H, Zhang YB, Gui JF, Lemon SM, Yamane D. Interferon Regulatory Factor 1 (IRF1) and Anti-Pathogen Innate Immune Responses. PloS Pathog (2021) 17:e1009220. doi: 10.1371/journal.ppat.1009220
67. Gall A, Treuting P, Elkon KB, Loo Y, Gale M, Barber GN, et al. Autoimmunity Initiates in Nonhematopoietic Cells and Progresses via Lymphocytes in an Interferon-Dependent Autoimmune Disease. Immunity (2012) 36:120–31. doi: 10.1016/j.immuni.2011.11.018
68. Theofilopoulos AN, Baccala R, Beutler B, Kono DH. Type I Interferons (Alpha/Beta) in Immunity and Autoimmunity. Annu Rev Immunol (2005) 23:307–36. doi: 10.1146/annurev.immunol.23.021704.115843
69. Turnier JL, Kahlenberg JM. The Role of Cutaneous Type I IFNs in Autoimmune and Autoinflammatory Diseases. J Immunol (2020) 205:2941–50. doi: 10.4049/jimmunol.2000596
70. Markovics A, Toth DM, Glant TT, Mikecz K. Regulation of Autoimmune Arthritis by the SHP-1 Tyrosine Phosphatase. Arthritis Res Ther (2020) 22:160. doi: 10.1186/s13075-020-02250-8
71. Croker BA, Lawson BR, Rutschmann S, Berger M, Eidenschenk C, Blasius AL, et al. Inflammation and Autoimmunity Caused by a SHP1 Mutation Depend on IL-1, MyD88, and a Microbial Trigger. Proc Natl Acad Sci U S A (2008) 105:15028–33. doi: 10.1073/pnas.0806619105
72. Myers DR, Abram CL, Wildes D, Belwafa A, Welsh A, Schulze CJ, et al. Shp1 Loss Enhances Macrophage Effector Function and Promotes Anti-Tumor Immunity. Front Immunol (2020) 11:576310. doi: 10.3389/fimmu.2020.576310
73. Jangra S, Bharti A, Lui WY, Chaudhary V, Botelho MG, Yuen KS, et al. Suppression of JAK-STAT Signaling by Epstein-Barr Virus Tegument Protein BGLF2 Through Recruitment of SHP1 Phosphatase and Promotion of STAT2 Degradation. J Virol (2021) 95:e0102721. doi: 10.1128/JVI.01027-21
74. Hao D, Wang Y, Li L, Qian G, Liu J, Li M, et al. SHP-1 Suppresses the Antiviral Innate Immune Response by Targeting TRAF3. FASEB J (2020) 34:12392–405. doi: 10.1096/fj.202000600RR
75. An H, Hou J, Zhou J, Zhao W, Xu H, Zheng Y, et al. Phosphatase SHP-1 Promotes TLR- and RIG-I-Activated Production of Type I Interferon by Inhibiting the Kinase IRAK1. Nat Immunol (2008) 9:542–50. doi: 10.1038/ni.1604
76. Ablasser A, Bauernfeind F, Hartmann G, Latz E, Fitzgerald KA, Hornung V. RIG-I-Dependent Sensing of Poly(Da:Dt) Through the Induction of an RNA Polymerase III–transcribed RNA Intermediate. Nat Immunol (2009) 10:1065–72. doi: 10.1038/ni.1779
77. Ran Y, Shu HB, Wang YY. MITA/STING: A Central and Multifaceted Mediator in Innate Immune Response. Cytokine Growth Factor Rev (2014) 25:631–9. doi: 10.1016/j.cytogfr.2014.05.003
78. Plas DR, Johnson R, Pingel JT, Matthews RJ, Dalton M, Roy G, et al. Direct Regulation of ZAP-70 by SHP-1 in T Cell Antigen Receptor Signaling. Science (1996) 272:1173–6. doi: 10.1126/science.272.5265.1173
79. Plas DR, Thomas ML. Negative Regulation of Antigen Receptor Signaling in Lymphocytes. J Mol Med (Berl) (1998) 76:589–95. doi: 10.1007/s001090050254
80. Massa PT, Wu C. The Role of Protein Tyrosine Phosphatase SHP-1 in the Regulation of IFN-Gamma Signaling in Neural Cells. J Immunol (1996) 157:5139–44. doi: 10.1084/jem.184.6.2445
81. Sharma Y, Ahmad A, Bashir S, Elahi A, Khan F. Implication of Protein Tyrosine Phosphatase SHP-1 in Cancer-Related Signaling Pathways. Future Oncol (2016) 12:1287–98. doi: 10.2217/fon-2015-0057
82. Minchenberg SB, Massa PT. The Control of Oligodendrocyte Bioenergetics by Interferon-Gamma (IFN-Gamma) and Src Homology Region 2 Domain-Containing Phosphatase-1 (SHP-1). J Neuroimmunol (2019) 331:46–57. doi: 10.1016/j.jneuroim.2017.10.015
83. Langevin C, Aleksejeva E, Passoni G, Palha N, Levraud JP, Boudinot P. The Antiviral Innate Immune Response in Fish: Evolution and Conservation of the IFN System. J Mol Biol (2013) 425:4904–20. doi: 10.1016/j.jmb.2013.09.033
84. Secombes CJ, Zou J. Evolution of Interferons and Interferon Receptors. Front Immunol (2017) 8:209. doi: 10.3389/fimmu.2017.00209
85. Li S, Lu LF, Li ZC, Zhang C, Zhou XY, Zhou Y, et al. Zebrafish MVP Recruits and Degrades TBK1 to Suppress IFN Production. J Immunol (2019) 202:559–66. doi: 10.4049/jimmunol.1801325
86. Lu LF, Zhang C, Li ZC, Zhou XY, Jiang JY, Chen DD, et al. A Novel Role of Zebrafish TMEM33 in Negative Regulation of Interferon Production by Two Distinct Mechanisms. PloS Pathog (2021) 17:e1009317. doi: 10.1371/journal.ppat.1009317
87. An H, Zhao W, Hou J, Zhang Y, Xie Y, Zheng Y, et al. SHP-2 Phosphatase Negatively Regulates the TRIF Adaptor Protein-Dependent Type I Interferon and Proinflammatory Cytokine Production. Immunity (2006) 25:919–28. doi: 10.1016/j.immuni.2006.10.014
88. Fitzgerald KA, McWhirter SM, Faia KL, Rowe DC, Latz E, Golenbock DT, et al. IKKepsilon and TBK1 are Essential Components of the IRF3 Signaling Pathway. Nat Immunol (2003) 4:491–6. doi: 10.1038/ni921
89. Tanaka Y, Chen ZJ. STING Specifies IRF3 Phosphorylation by TBK1 in the Cytosolic DNA Signaling Pathway. Sci Signal (2012) 5:a20. doi: 10.1126/scisignal.2002521
90. Levine B, Mizushima N, Virgin HW. Autophagy in Immunity and Inflammation. Nature (2011) 469:323–35. doi: 10.1038/nature09782
91. Deretic V, Saitoh T, Akira S. Autophagy in Infection, Inflammation and Immunity. Nat Rev Immunol (2013) 13:722–37. doi: 10.1038/nri3532
92. Chen M, Meng Q, Qin Y, Liang P, Tan P, He L, et al. TRIM14 Inhibits cGAS Degradation Mediated by Selective Autophagy Receptor P62 to Promote Innate Immune Responses. Mol Cell (2016) 64:105–19. doi: 10.1016/j.molcel.2016.08.025
93. Jin S, Cui J. BST2 Inhibits Type I IFN (Interferon) Signaling by Accelerating MAVS Degradation Through CALCOCO2-Directed Autophagy. Autophagy (2018) 14:171–2. doi: 10.1080/15548627.2017.1393590
94. Jin S, Tian S, Luo M, Xie W, Liu T, Duan T, et al. Tetherin Suppresses Type I Interferon Signaling by Targeting MAVS for NDP52-Mediated Selective Autophagic Degradation in Human Cells. Mol Cell (2017) 68:308–22. doi: 10.1016/j.molcel.2017.09.005
95. Ma Y, Galluzzi L, Zitvogel L, Kroemer G. Autophagy and Cellular Immune Responses. Immunity (2013) 39:211–27. doi: 10.1016/j.immuni.2013.07.017
96. Freeling M, Scanlon MJ, Fowler JE. Fractionation and Subfunctionalization Following Genome Duplications: Mechanisms That Drive Gene Content and Their Consequences. Curr Opin Genet Dev (2015) 35:110–8. doi: 10.1016/j.gde.2015.11.002
97. Workenhe ST, Rise ML, Kibenge MJ, Kibenge FS. The Fight Between the Teleost Fish Immune Response and Aquatic Viruses. Mol Immunol (2010) 47:2525–36. doi: 10.1016/j.molimm.2010.06.009
Keywords: Gibel carp, SHP1, negative regulator, autophagy, MITA, interferon
Citation: Tong J-F, Zhou L, Li S, Lu L-F, Li Z-C, Li Z, Gan R-H, Mou C-Y, Zhang Q-Y, Wang Z-W, Zhang X-J, Wang Y and Gui J-F (2021) Two Duplicated Ptpn6 Homeologs Cooperatively and Negatively Regulate RLR-Mediated IFN Response in Hexaploid Gibel Carp. Front. Immunol. 12:780667. doi: 10.3389/fimmu.2021.780667
Received: 21 September 2021; Accepted: 11 November 2021;
Published: 26 November 2021.
Edited by:
Tiehui Wang, University of Aberdeen, United KingdomReviewed by:
Xinhua Chen, Fujian Agriculture and Forestry University, ChinaChengyu Hu, Nanchang University, China
Copyright © 2021 Tong, Zhou, Li, Lu, Li, Li, Gan, Mou, Zhang, Wang, Zhang, Wang and Gui. This is an open-access article distributed under the terms of the Creative Commons Attribution License (CC BY). The use, distribution or reproduction in other forums is permitted, provided the original author(s) and the copyright owner(s) are credited and that the original publication in this journal is cited, in accordance with accepted academic practice. No use, distribution or reproduction is permitted which does not comply with these terms.
*Correspondence: Yang Wang, wangyang@ihb.ac.cn; Jian-Fang Gui, jfgui@ihb.ac.cn