- Centro de Investigaciones Biológicas Margarita Salas, Consejo Superior de Investigaciones Científicas, Madrid, Spain
Chemotaxis is a molecular mechanism that confers leukocytes the ability to detect gradients of chemoattractants. Chemokine receptors are well-known regulators of chemotaxis in leukocytes; however, they can regulate several other activities in these cells. This information has been often neglected, probably due to the paramount role of chemotaxis in the immune system and in biology. Therefore, the experimental data available on the mechanisms used by chemokine receptors to regulate other functions of leukocytes is sparse. The results obtained in the study of the chemokine receptor CCR7 in dendritic cells (DCs) provide interesting information on this issue. CCR7 guides the DCs from the peripheral tissues to the lymph nodes, where these cells control T cell activation. CCR7 can regulate DC chemotaxis, survival, migratory speed, cytoarchitecture, and endocytosis. Biochemical and functional analyses show: first, that CCR7 uses in DCs the PI3K/Akt pathway to control survival, the MAPK pathway to control chemotaxis, and the RhoA pathways to regulate actin dynamics, which in turn controls migratory speed, cytoarchitecture, and endocytosis; second, that these three signaling pathways behave as modules with a high degree of independence; and third, that although each one of these routes can regulate several functions in different settings, CCR7 promotes in DCs a functional bias in each pathway. The data uncover an interesting mechanism used by CCR7 to regulate the DCs, entailing multifunctional signaling pathways organized in modules with biased functionality. A similar mechanism could be used by other chemoattractant receptors to regulate the functions of leukocytes.
“Divide and rule” Attributed to Philip II of Macedon
Introduction
Chemokine receptors regulate chemotaxis, a process that allows cells to detect gradients of chemoattractants. Based on this property, chemokine receptors, together with their ligands, serve as “address codes” that guide leukocytes to specific sites in the organism (1–3). Although chemoattraction is an important activity controlled by these receptors, they can regulate additional functions of leukocytes (4–6). This fact has been largely overlooked, probably due to the capital importance of chemoattraction in biology; consequently, the information on other functions of chemokine receptors is sparse (6). It is expected that non-chemotactic functions regulated by chemokine receptors may contribute to the efficient functioning of leukocytes in the immune system. Therefore, getting insight into the molecular mechanisms used to regulate these functions may allow the identification of novel targets to modulate the immune response.
C–C chemokine receptor 7, like all chemokine receptors, is included in the G protein-coupled receptor superfamily (6). CCR7 (ligands CCL19 and CCL21) is one of the chemokine receptors on which more functional information is available (6–8). We have studied the signaling pathways controlling CCR7-mediated functions in dendritic cells (DCs). It was found that, to control specific cellular functions of DCs, this receptor uses well-known signaling pathways that organize as signaling modules with biased functionality and limited crosstalk among them (9–13). Herein we describe the signaling components of these modules and discuss how they may regulate the functions of DCs.
CCR7-Controlled Non-Chemotactic Activities May Contribute to the Efficiency of Dendritic Cells in the Immune System
Dendritic cells are leukocytes that are found in peripheral tissues in a differentiation state called immature, during which they display a high ability to detect, capture, and process antigens (14). After exposure to danger signals, including pathogens, toxic agents, or inflammatory cytokines, immature DCs undergo a complex differentiation program that transforms them into mature DCs (maDCs), which migrate to the lymph nodes (LNs), where they present antigens captured in the immature stage to antigen-specific T cells. As part of their differentiation program, the maDCs upregulate the expression of the chemokine receptor CCR7 that guides the maDCs to the LNs, attracted by CCL21 which is expressed in the afferent lymphatics vessels and by CCL19 and CCL21 which are expressed in stromal cells in the LNs (15, 16). CCR7 is crucial to guide the maDCs to the LNs, implying that its correct expression and function is important for adequate adaptive immune response (15, 17–21). Apart from chemoattraction (8, 22, 23), CCR7 regulates cytoarchitecture (13, 24), endocytosis (13, 25), survival (12), migratory speed (11, 26), adhesion (27), and differentiation in maDCs (28). Predictably, these non-chemotactic activities regulated by CCR7 contribute to the correct functionality of the maDCs in the immune system (6). It is expected that the enhanced survival, migratory speed, and differentiation may increase the number of antigen-loaded maDCs that reach the LNs. The increment in endocytosis may confer the maDCs migrating through the afferent lymphatic vessels, or located in the LNs, the ability to endocyte antigens, e.g., viral particles that can be subsequently presented to T cells (29, 30). Enhanced adhesion facilitates the migration of the maDCs through the afferent lymphatic vessels (27, 31). The induced changes in actin cytoarchitecture can regulate the motility of the maDCs that migrate toward the LNs and confer these cells their dendritic morphology (11, 13, 24). Pseudopod extensions increase the surface-area-to-volume ratio of the maDCs when compared with a spherical cell of equal volume (32), increasing the possibilities of contact with T cells. In summary, the different functions controlled by CCR7 may predictably contribute to a more effective maDCs in the immune system and to a better adaptive immune response (6). An important issue is the identification of the mechanisms used by CCR7 to regulate the cellular activities of the maDCs. In the following sections, we discuss recent experimental data that provide information on the molecules and the signaling mechanism involved in this process.
CCR7-Dependent Survival Is Governed by a PI3K/AKT-Controlled Signaling Module
When maDCs are kept in serum-free medium, they initiate an apoptotic program that leads to their demise (12). This simple experimental setting is useful to identify the receptors that inhibit cellular apoptosis and the intracellular pathways involved (12, 33). The stimulation of maDC kept in serum-free medium with any of the ligands of CCR7, CCL19, or CCL21 slows down the apoptosis of these cells, indicating that this receptor induces anti-apoptotic intracellular signaling (12). Using this experimental strategy, it was also found that the kinases AMPK and GSK3β played pro-apoptotic roles in vitro and in vivo because a forced increase or decrease of their activities enhanced or impaired apoptosis in maDCs (9, 10, 34) (Figure 1). Moreover, it was found that AMPK promotes apoptosis in maDCs by inhibiting the mechanistic target of rapamycin complex 1 (mTORC1), a kinase complex that promotes survival in maDCs (see below) (10). Both AMPK and GSK3β induce the activation of the transcription factors FOXO1/3, which controls the pro-apoptotic Bcl2 family member Bim (9, 10, 12, 35). Moreover, active GSK3β also prevents the activation of anti-apoptotic transcription factor NFκB, which controls the transcription of the anti-apoptotic Bcl2 family member Bclxl (9) (Figure 1). The balance between pro-apoptotic and anti-apoptotic Bcl2 family members determines whether a cell becomes apoptotic or survives (36). An excessive increase in pro-apoptotic (e.g., Bim) over pro-survival (e.g., Bclxl) Bcl2 members induces the activation of the mitochondria gatekeepers Bax/Bak (36, 37), resulting in mitochondrial outer membrane permeabilization and liberation of cytochrome c from the intermembrane space of the mitochondria, which leads to caspase activation and apoptosis (Figure 1) (36).
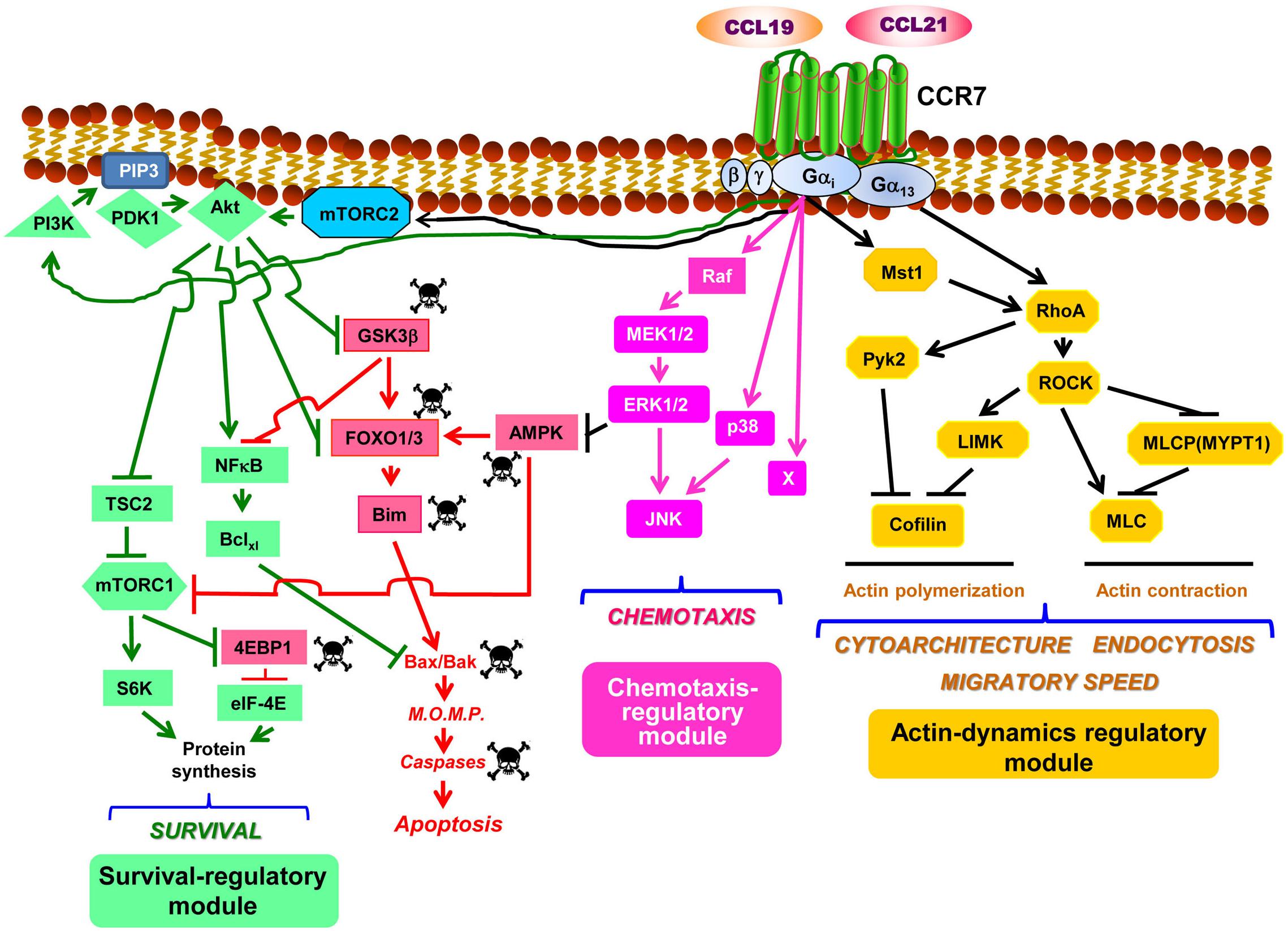
Figure 1. CCR7 uses signaling modules to regulate the functions of dendritic cells. Considering the sparse data within this field, the figure should be considered as a tentative model to be completed as additional experimental information becomes available. The model is largely based on the data presented in Table 1. Downstream of CCR7, the PI3K/Akt, the MAPKs, and the RhoA pathways organize as signaling modules that regulate survival, chemotaxis, and actin dynamics, respectively (see text for details). Abbreviations used: Akt, also known as Protein kinase B (PKB); AMPK, AMP-activated protein kinase; Bclxl, B-cell lymphoma extra-large; Bim, Bcl-2-interacting mediator of cell death; 4EBP1, eukaryotic translation initiation factor 4E (eIF4E)-binding protein 1; MEK1/2, MAPK/ERK kinase 1 and 2; ERK1/2, extracellular signal-regulated kinase 1 and 2; mTORC1, mechanistic target of rapamycin (mTOR) complex 1; FOXO1/3, forkhead box protein O1 and O3; GSK3β, glycogen synthase kinase 3β; JNK, c-Jun N-terminal kinase; LIMK, LIM domain kinase; mTORC2, mTOR complex 2; MLC, myosin light chain; M.O.M.P., mitochondrial outer membrane permeabilization; Mst1, mammalian sterile 20-like kinase 1; MYPT1, myosin phosphatase target subunit 1; NFκB, nuclear factor-κB; PDK1, phosphoinositide-dependent kinase-1; PIP3, phosphatidylinositol (3,4,5)-trisphosphate; PI3K, phosphatidylinositol 3-kinase; Pyk2, proline-rich tyrosine kinase 2; RhoA, Ras homolog family member A; ROCK, Rho-associated protein kinase; S6, ribosomal protein S6; S6K, S6 kinase; TSC2, tuberous sclerosis complex 2. The skull and crossbones symbol indicates molecules that promote apoptosis.
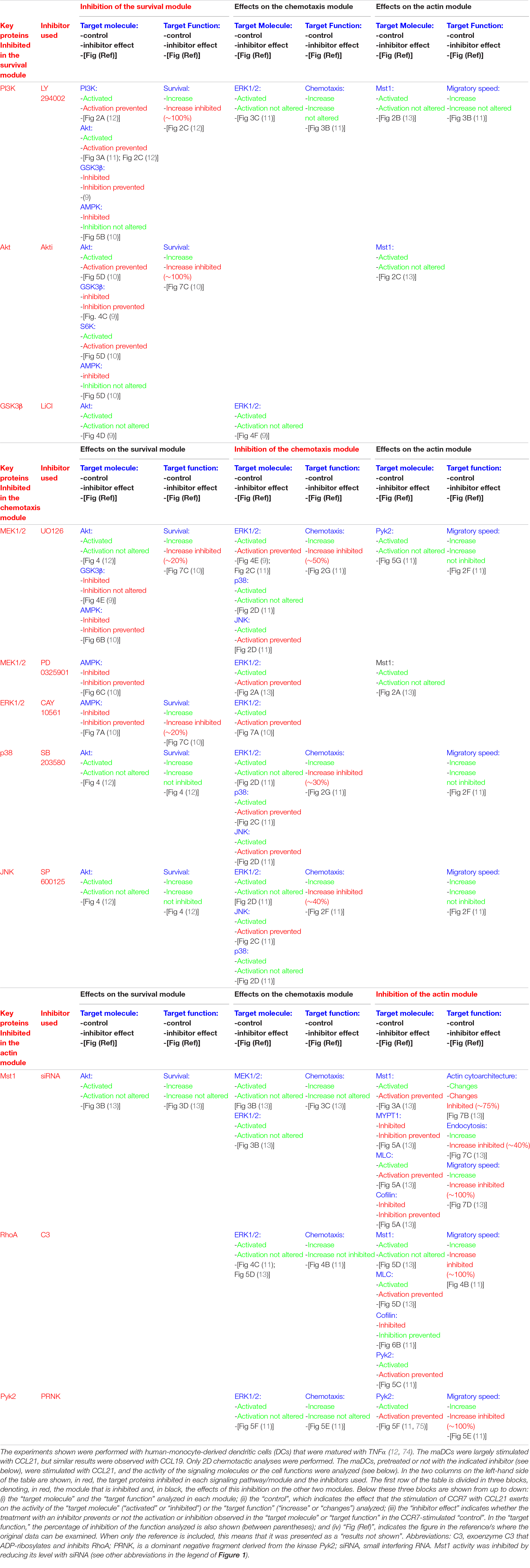
Table 1. Experimental data support a high degree of independence between the different CCR7-regulated modules controlling the functions of the dendritic cells.
When CCR7 was stimulated in the maDCs that were in serum-free medium, it was observed that the pro-apoptotic signaling described above was turned off because this receptor induced the activation of the signaling axis PI3K/Akt (9, 10, 12, 19, 38, 39) which, as shown below, is a core component of a pro-survival pathway in these cells (Figure 1). The Gi family of G proteins and, particularly, the Gβγ complex (12), a dimer associated to the Gα subunit to form a heterotrimeric G protein, mediate the CCR7-dependent activation of the PI3K/Akt pathway (10, 12). This pathway induces survival in multiple cell types due to the ability of its signaling components, particularly Akt, to switch off pro-apoptotic molecules and turn on pro-survival signals (40, 41). Upon the CCR7-dependent activation of Akt in maDCs, this kinase directly phosphorylates and inhibits the transcription factor FOXO1/3 which, as mentioned above, controls the expression of pro-apoptotic Bim (9). Active Akt further prevents apoptosis by phosphorylating/inhibiting GSK3β which, as mentioned before, promotes the activation of pro-apoptotic FOXO1/3 and also the inhibition of pro-survival NFκB (9) (Figure 1). Akt also induces the activation of the transcription factor NFκB, which regulates Bclxl (9, 12) that, as indicated above, protects the cells from apoptosis by opposing pro-apoptotic Bim (Figure 1). Akt can also enhance cell survival by inducing the activation of mTORC1, which stimulates translation, a process that promotes survival in maDCs (42) (Figure 1). Active mTORC1 stimulates translation by inducing phosphorylation/inactivation of the eukaryotic translation initiation factor 4E (eIF4E)-binding protein 1 (4E-BP1), which retains eIF-4E inhibited. After the phosphorylation of 4E-BP1, eIF-4E is released and becomes part of the translation initiation complex (10, 42). Moreover, mTORC1 also activates translation by phosphorylating/activating ribosomal S6 kinase (S6K), which subsequently phosphorylates the protein targets involved in translation, including the ribosomal protein S6 (Figure 1). The combined effects of the activation of Akt, which leads to the up-regulation of Bclxl, the inhibition of Bim and, in addition, the activation of mTORC1, which promotes an increase in protein synthesis, contribute to extend the survival of the maDCs (10, 42). The prior results indicate that the PI3K/Akt pathway mediates CCR7-dependent survival. Regarding the contribution of other pathways to the regulation of survival, a modest contribution of the chemotaxis pathway was observed because the inhibition of MEK1/2/ERK1/2, two key regulators of chemotaxis (see below), reduced by ∼20% the pro-survival effects induced by CCR7 (10). MEK1/2/ERK1/2 may exert this moderate pro-survival effect by inhibiting the pro-apoptotic kinase AMPK (10) (Table 1 and Figure 1).
The inhibition of the PI3K/Akt pathway strikingly failed to affect the CCR7-dependent MAPK pathway (Table 1), the RhoA pathway, or the functions regulated by these two routes in maDCs (Table 1) (9, 10, 12). A lack of effect of PI3K/Akt on CCR7-regulated chemotaxis (19, 39) or endocytosis (see below) has also been reported by other groups (39). These results suggest that the PI3K/Akt pathway constitute a signaling module that largely controls CCR7-induced survival, but no other CCR7-mediated functions in maDCs (see below). This is interesting because the PI3K/Akt pathway also regulates chemotaxis, proliferation, and metabolism in other cells (43, 44). This functional bias is also observed in the other two pathways controlled by CCR7 in maDCs (see below). In summary, the data indicate that CCR7 controls in maDCs a PI3K/Akt-controlled signaling module that regulates survival, but not chemotaxis or actin dynamics (see below).
CCR7-Dependent Chemoattraction Is Governed by a MAPK- Controlled Signaling Module
Experimental evidence indicates that CCR7-dependent chemotaxis is largely regulated by a signaling module formed by Raf and the MAPKs family members MEK1/2, ERK1/2, p38, and JNK in maDCs (11, 39). As shown for CCR7-induced survival, the activation of both the MAPK pathway and chemotaxis is regulated in maDCs by Gi proteins and Gβγ dimers (11). Previously, Gβγ dimers have been shown to regulate chemotaxis in other chemokine receptors and cells (45). Although it has not been experimentally analyzed if CCR7 induces the activation of Ras in maDCs, it is possible that this GTPase may mediate the effects of CCR7 on the MAPK pathway because Ras is an upstream regulator of the Raf-MEK1/2-ERK1/2 pathway (46), and different chemokine receptors can induce the activation of this GTPase (47–49). Moreover, upon G-protein coupled receptor activation, Gi and Gβγ dimers mediate, like in maDCs, the Ras-dependent activation of MAP kinase pathway (50, 51). In summary, the results indicate that the MAPK pathway controls CCR7-dependent chemotaxis in maDCs (11). However, the complete inhibition of ERK1/2, p38, and JNK does not abrogate chemotaxis, suggesting that additional regulator/s, denoted as “X” in Figure 1, may also contribute to the regulation of this pathway (11). The inhibition of key molecular components of the MAPK pathway does not affect dramatically the CCR7-dependent PI3K/Akt pathway and survival or the RhoA pathway and the function associated to it (actin-regulated functions, including cytoarchitecture, endocytosis, and migratory speed; see below) (Table 1). Other authors have also shown that the stimulation of CCR7 induces the activation of JNK and that the inhibition of this MAPK blocks chemotaxis, but not the endocytosis in maDCs (39), supporting the independence of the RhoA pathway (see below) of the chemotaxis regulatory module. In summary, the results suggest that the MAPK pathway may constitute a signaling module that displays a high degree of independence since it seems independent of the module that regulates CCR7-regulated actin dynamics and displays only a very modest contribution to the regulation of CCR7-controlled survival (Figure 1). Another interesting aspect that emerges from these results is the high degree of functional bias of the MAPK pathway, which seems to regulate mainly CCR7-controlled chemotaxis and only modestly survival in maDC, although it is a potent regulator of survival and proliferation in other contexts (52).
The data suggesting that the MAPK pathway mediates CCR7-dependent chemotaxis in maDCs is consistent with prior data showing that Ras, an upstream regulator of the MAPKs (46), is a regulator of chemotaxis in response to N-formyl-L-methionyl-L-leucyl-L-phenylalanine (fMLP) in neutrophils (53, 54) and to cyclic-adenosine monophosphate (cAMP) in Dictyostelium discoideum (55, 56). Moreover, in Dictyostelium, Ras activation takes place independently of PI3K (55), which reminds of the observed independence between the CCR7-regulated activation of MAPK and the PI3K/Akt pathways described in maDCs. In summary, the results indicate that CCR7 controls in maDCs a MAPK-regulated signaling module, which selectively controls chemotaxis, independently of the survival and actin-regulatory modules (see below).
CCR7-Dependent Changes in Actin Dynamics Is Governed by a RhoA-Controlled Signaling Module
Our studies indicate that the CCR7-induced stimulation of migratory speed, endocytosis, and changes in cytoarchitecture in maDCs is mediated by the RhoA pathway (11, 13). Thus RhoA, a key regulator of actin organization in multiple cells, including maDC (57–64), would govern the actin dynamic changes involved in the control of the aforementioned activities. It has been suggested that RhoA does not mediate CCR7-induced morphological changes and endocytosis in murine maDCs, which would be controlled instead by Cdc42 and Rac (24, 25). These discrepancies could be due to species differences [murine maDCs (24, 25) vs. human maDCs (11, 13)] or caused by the maturation stimulus used for the DCs [LPS (24, 25) vs. TNFα (11, 13)]. This issue will have to be settled in future studies. Unlike CCR7-dependent survival and chemotaxis, which depend largely on Gi proteins, CCR7-dependent changes in actin dynamics were found to be regulated both by Gi and G13 family of G proteins (Figure 1). Interestingly, the kinase Mst1 connects Gi with RhoA, which is also downstream of G13 (13) (Figure 1). These data are consistent with prior results indicating that the G12/13 proteins control RhoA (65). RhoA effects are mediated by a pathway that controls actin dynamics, including actin polymerization (ROCK-LIMK-cofilin) and contraction (ROCK/MLCP/MLC) (57, 59, 61) (Figure 1). It was also observed that, downstream of CCR7, RhoA controls the activation of the tyrosine kinase Pyk2 (11) (Figure 1), suggesting that this kinase can also mediate the effects of RhoA on the actin cytoskeleton. Accordingly, other authors have suggested that Pyk2 is activated downstream of G13 and that it is involved in the control of leukocyte motility and cytoarchitecture (66, 67). The selective blocking of the molecular components of the RhoA pathway in mDCs results in the inhibition of CCR7-dependent migratory speed, endocytosis, and alterations of the cytoarchitecture (11, 13), suggesting that RhoA-controlled actin mediates these functions (58, 61). In summary, CCR7 controls two axes, namely, CCR7/G13 and CCR7/Gi/Mst1, that converge on RhoA, which is upstream of a pathway that controls the actin dynamics involved in the regulation of migratory speed, endocytosis, and cytoarchitecture (Figure 1) (11, 13, 57, 60). As shown for the other modules, the inhibition of specific signaling components of this pathway failed to affect the chemotaxis or survival of the signaling components controlling these functions, supporting the independence of the CCR7-dependent RhoA-regulated signaling module (Table 1) (11, 13). Supporting that the actin dynamics regulatory module is independent of the chemotaxis module, it has been shown that the inhibition of the kinase ROCK fails to block the activation of the chemotaxis regulator JNK in maDCs (39). The results together suggest that the CCR7-regulated RhoA pathway behaves as a signaling module that displays a high degree of independence in maDCs. As shown with the other two modules, although in addition to actin dynamics, the RhoA pathway may regulate other cell functions, including survival and proliferation (62); however, CCR7 in maDCs apparently regulates largely actin dynamics. In summary, the RhoA-regulated module controls selectively CCR7-dependent actin dynamics and the cellular activities associated to it, including migratory speed, endocytosis, and cytoarchitecture.
Finally, the described independence between the chemotaxis and actin dynamics regulatory modules suggests that chemotaxis and motility are different functions. The following results further support this concept. Using microfluidic devices, it has been shown that perturbing actin dynamics with actin and myosin inhibitors in mouse maDCs affects the migratory speed, but not the chemotaxis in response to CCL19 (68). The actin-associated protein mDia, which regulates actin dynamics, mediates migratory speed, but it is dispensable for 3D chemotaxis in response to CCL21 in murine maDCs (69, 70). In response to the external gradients of cAMP, in D. discoideum, a polarized localization of Ras is observed, and in neutrophils exposed to the gradients of fMLP, a polarization of PI phosphatidylinositol (3,4,5)-trisphosphate (PIP3) also takes place. However, in Dictyostelium and human neutrophils exposed to these chemoattractants, Ras and PIP3 still polarize, even when the cells were immobilized either on highly adherent substrates or by disrupting their actin cytoskeleton with latrunculin (53, 55). It has been shown that, although the actin-associated leading edge protein Arp2/3, which regulates actin dynamics, is critical for lamellipodial formation and cell motility in fibroblast and cancer cell lines, it is, however, dispensable for chemotaxis (71, 72). These examples suggest that perhaps it is more appropriate to define chemotaxis as “chemoattractant sensing” to separate it from motility, which could be a different cell activity.
Concluding Remarks
Herein we discuss experimental findings indicating that CCR7 activates three signaling pathways in maDCs, namely, the PI3K/Akt, the MAPK, and the RhoA pathways, which largely regulate survival (12), chemotaxis (11), and actin dynamics (11, 13), respectively. The results obtained suggest a high degree of independence between these pathways, although it is not complete because at least the chemotaxis and the survival modules are connected, with the former controlling modestly the latter. Albeit each one of the three pathways can regulate several functions in different contexts (43, 44, 52, 62), CCR7 seems to select only one activity in maDCs. The molecular mechanisms supporting the independence and biased functionality of these pathways are not known. CCR7 regulates in maDCs other signaling molecules not analyzed in this review, e.g., cyclic AMP, calcium, phospholipase C, Src, and others (11, 19, 73). Future studies will determine their roles in the modules described or in others described in the future. Finally, the independent modular organization described could be one among several strategies used by chemokine receptors to regulate leukocyte functions because, for instance, the receptor CXCR4 uses redundant signaling to control survival and chemotaxis in maDCs (33). In summary, the information gathered point out an interesting mechanism that could be used by multifunctional chemokine receptors to regulate the functions of leukocytes.
Author Contributions
JR-F conceived and wrote the manuscript. OC-G performed important contributions to the manuscript and the figure and designed the table as presented.
Funding
This work was supported by grants SAF-2014-53151-R (Ministerio de Economía y Competitividad), SAF2017-83306-R (Ministerio de Ciencia, Innovación y Universidades), and RETICS Program/Instituto de Salud Carlos III (RIER) (RD08/0075).
Conflict of Interest
The authors declare that the research was conducted in the absence of any commercial or financial relationships that could be construed as a potential conflict of interest.
Acknowledgments
We apologize to those researchers that we could not cite due to space constraints.
References
1. Griffith JW, Sokol CL, Luster AD. Chemokines and chemokine receptors: positioning cells for host defense and immunity. Annu Rev Immunol. (2014) 32:659–702. doi: 10.1146/annurev-immunol-032713-120145
2. Olson TS, Ley K. Chemokines and chemokine receptors in leukocyte trafficking. Am J Physiol Regulatory Integrative Comp Physiol. (2002) 283:R7–28. doi: 10.1152/ajpregu.00738.2001
3. Viola A, Luster AD. Chemokines and their receptors: drug targets in immunity and inflammation. Annu Rev Pharmacol Toxicol. (2008) 48:171–97. doi: 10.1146/annurev.pharmtox.48.121806.154841
4. Baggiolini M, Kernen P. Control of shape change, exocytosis, and respiratory burst. News Physiol Sci. (1992) 7:215–9.
5. Baggiolini M, Walz A, Kunkel SL. Neutrophil-activating peptide-1/interleukin 8, a novel cytokine that activates neutrophils. J Clin Invest. (1989) 84:1045–9.
6. López-Cotarelo P, Gómez-Moreira C, Criado-García O, Sánchez L, Rodríguez-Fernández JL. Beyond chemoattraction: multifunctionality of chemokine receptors in leukocytes. Trends Immunol. (2017) 38:927–41. doi: 10.1016/j.it.2017.08.004
7. Comerford I, Harata-Lee Y, Bunting MD, Gregor C, Kara EE, McColl SR. A. myriad of functions and complex regulation of the CCR7/CCL19/CCL21 chemokine axis in the adaptive immune system. Cytokine Growth Factor Rev. (2013) 24:269–83. doi: 10.1016/j.cytogfr.2013.03.001
8. Sanchez-Sanchez N, Riol-Blanco L, Rodriguez-Fernandez JL. The multiple personalities of the chemokine receptor CCR7 in dendritic cells. J Immunol. (2006) 176:5153–9. doi: 10.4049/jimmunol.176.9.5153
9. Escribano C, Delgado-Martin C, Rodriguez-Fernandez JL. CCR7-dependent stimulation of survival in dendritic cells involves inhibition of GSK3β. J Immunol. (2009) 183:6282–95. doi: 10.4049/jimmunol.0804093
10. Lopez-Cotarelo P, Escribano-Diaz C, Gonzalez-Bethencourt IL, Gomez-Moreira C, Deguiz ML, Torres-Bacete J, et al. A novel MEK-ERK-AMPK signaling axis controls chemokine receptor CCR7-dependent survival in human mature dendritic cells. J Biol Chem. (2015) 290:827–40. doi: 10.1074/jbc.M114.596551
11. Riol-Blanco L, Sanchez-Sanchez N, Torres A, Tejedor A, Narumiya S, Corbi AL, et al. The chemokine receptor CCR7 activates in dendritic cells two signaling modules that independently regulate chemotaxis and migratory speed. J Immunol. (2005) 174:4070–80. doi: 10.4049/jimmunol.174.7.4070
12. Sanchez-Sanchez N, Riol-Blanco L, de la Rosa G, Puig-Kroger A, Garcia-Bordas J, Martin D, et al. Chemokine receptor CCR7 induces intracellular signaling that inhibits apoptosis of mature dendritic cells. Blood. (2004) 104:619–25.
13. Torres Bacete J, Delgado-Martín C, Gomez-Moreira C, Simizu S, Rodríguez-Fernández JL. The Mammalian Sterile20-like 1 (Mst1) kinase controls selective CCR7-dependent functions in human dendritic cells. J Immunol. (2015) 195:973–81. doi: 10.4049/jimmunol.1401966
14. Steinman RM. Linking innate to adaptive immunity through dendritic cells. Novartis Found Symp. (2006) 279:101–9.
15. Forster R, Schubel A, Breitfeld D, Kremmer E, Renner-Muller I, Wolf E, et al. CCR7 coordinates the primary immune response by establishing functional microenvironments in secondary lymphoid organs. Cell. (1999) 99:23–33. doi: 10.1016/s0092-8674(00)80059-8
16. Luther SA, Tang HL, Hyman PL, Farr AG, Cyster JG. Coexpression of the chemokines ELC and SLC by T zone stromal cells and deletion of the ELC gene in the plt/plt mouse. Proc Natl Acad Sci USA. (2000) 97:12694–9. doi: 10.1073/pnas.97.23.12694
17. Forster R, Davalos-Misslitz AC, Rot A. CCR7 and its ligands: balancing immunity and tolerance. Nat Rev Immunol. (2008) 8:362–71. doi: 10.1038/nri2297
18. Ohl L, Mohaupt M, Czeloth N, Hintzen G, Kiafard Z, Zwirner J, et al. CCR7 governs skin dendritic cell migration under inflammatory and steady-state conditions. Immunity. (2004) 21:279–88. doi: 10.1016/j.immuni.2004.06.014
19. Scandella E, Men Y, Legler DF, Gillessen S, Prikler L, Ludewig B, et al. CCL19/CCL21-triggered signal transduction and migration of dendritic cells requires prostaglandin E2. Blood. (2004) 103:1595–601. doi: 10.1182/blood-2003-05-1643
20. Cote SC, Pasvanis S, Bounou S, Dumais N. CCR7-specific migration to CCL19 and CCL21 is induced by PGE(2) stimulation in human monocytes: Involvement of EP(2)/EP(4) receptors activation. Mol Immunol. (2009) 46:2682–93. doi: 10.1016/j.molimm.2008.08.269
21. Muthuswamy R, Mueller-Berghaus J, Haberkorn U, Reinhart TA, Schadendorf D, Kalinski PPGE. (2) transiently enhances DC expression of CCR7 but inhibits the ability of DCs to produce CCL19 and attract naive T cells. Blood. (2010) 116:1454–9. doi: 10.1182/blood-2009-12-258038
22. Kellermann SA, Hudak S, Oldham ER, Liu YJ, McEvoy LM. The CC chemokine receptor-7 ligands 6Ckine and macrophage inflammatory protein-3 beta are potent chemoattractants for in vitro- and in vivo-derived dendritic cells. J Immunol. (1999) 162:3859–64.
23. Sozzani S, Allavena P, D’Amico G, Luini W, Bianchi G, Kataura M, et al. Differential regulation of chemokine receptors during dendritic cell maturation: a model for their trafficking properties. J Immunol. (1998) 161:1083–6.
24. Yanagawa Y, Onoe K. CCL19 induces rapid dendritic extension of murine dendritic cells. Blood. (2002) 100:1948–56. doi: 10.1182/blood-2002-01-0260
25. Yanagawa Y, Onoe K. CCR7 ligands induce rapid endocytosis in mature dendritic cells with concomitant up-regulation of Cdc42 and Rac activities. Blood. (2003) 101:4923–9. doi: 10.1182/blood-2002-11-3474
26. Braun A, Worbs T, Moschovakis GL, Halle S, Hoffmann K, Bolter J, et al. Afferent lymph-derived T cells and DCs use different chemokine receptor CCR7-dependent routes for entry into the lymph node and intranodal migration. Nat Immunol. (2011) 12:879–87. doi: 10.1038/ni.2085
27. Eich C, de Vries IJ, Linssen PC, de Boer A, Boezeman JB, Figdor CG, et al. The lymphoid chemokine CCL21 triggers LFA-1 adhesive properties on human dendritic cells. Immunol Cell Biol. (2011) 89:458–65. doi: 10.1038/icb.2010.103
28. Marsland BJ, Battig P, Bauer M, Ruedl C, Lassing U, Beerli RR, et al. CCL19 and CCL21 induce a potent proinflammatory differentiation program in licensed dendritic cells. Immunity. (2005) 22:493–505. doi: 10.1016/j.immuni.2005.02.010
29. Platt CD, Ma JK, Chalouni C, Ebersold M, Bou-Reslan H, Carano RA, et al. Mature dendritic cells use endocytic receptors to capture and present antigens. Proc Natl Acad Sci USA. (2010) 107:4287–92. doi: 10.1073/pnas.0910609107
30. Reynoso GV, Weisberg AS, Shannon JP, McManus DT, Shores L, Americo JL, et al. Lymph node conduits transport virions for rapid T cell activation. Nat Immunol. (2019) 20:602–12. doi: 10.1038/s41590-019-0342-0
31. Johnson LA, Clasper S, Holt AP, Lalor PF, Baban D, Jackson DG. An inflammation-induced mechanism for leukocyte transmigration across lymphatic vessel endothelium. J Exp Med. (2006) 203:2763–77. doi: 10.1084/jem.20051759
32. Miller MJ, Hejazi AS, Wei SH, Cahalan MD, Parker I. T cell repertoire scanning is promoted by dynamic dendritic cell behavior and random T cell motility in the lymph node. Proc Natl Acad Sci USA. (2004) 101:998–1003. doi: 10.1073/pnas.0306407101
33. Delgado-Martin C, Escribano C, Pablos JL, Riol-Blanco L, Rodriguez-Fernandez JL. Chemokine CXCL12 uses CXCR4 and a signaling core formed by bifunctional Akt, extracellular signal-regulated kinase (ERK)1/2, and mammalian target of rapamycin complex 1 (mTORC1) proteins to control chemotaxis and survival simultaneously in mature dendritic cells. J Biol Chem. (2011) 286:37222–36. doi: 10.1074/jbc.M111.294116
34. Gomez-Cabanas L, Delgado-Martin C, Lopez-Cotarelo P, Escribano-Diaz C, Alonso CL, Riol-Blanco L, et al. Detecting apoptosis of leukocytes in mouse lymph nodes. Nat Protoc. (2014) 9:1102–12. doi: 10.1038/nprot.2014.078
35. Riol-Blanco L, Delgado-Martin C, Sanchez-Sanchez N, Alonso CL, Gutierrez-Lopez MD, Del Hoyo GM, et al. Immunological synapse formation inhibits, via NF-kappaB and FOXO1, the apoptosis of dendritic cells. Nat Immunol. (2009) 10:753–60. doi: 10.1038/ni.1750
36. Czabotar PE, Lessene G, Strasser A, Adams JM. Control of apoptosis by the BCL-2 protein family: implications for physiology and therapy. Nat Rev Mol Cell Biol. (2014) 15:49–63. doi: 10.1038/nrm3722
37. Westphal D, Kluck RM, Dewson G. Building blocks of the apoptotic pore: how Bax and Bak are activated and oligomerize during apoptosis. Cell Death Differ. (2014) 21:196–205. doi: 10.1038/cdd.2013.139
38. Ardeshna KM, Pizzey AR, Devereux S, Khwaja A. The PI3 kinase, p38 SAP kinase, and NF-kappaB signal transduction pathways are involved in the survival and maturation of lipopolysaccharide-stimulated human monocyte-derived dendritic cells. Blood. (2000) 96:1039–46.
39. Iijima N, Yanagawa Y, Clingan JM, Onoe K. CCR7-mediated c-Jun N-terminal kinase activation regulates cell migration in mature dendritic cells. Int Immunol. (2005) 17:1201–12. doi: 10.1093/intimm/dxh297
40. Datta SR, Brunet A, Greenberg ME. Cellular survival: a play in three Akts. Genes Dev. (1999) 13:2905–27.
41. Song G, Ouyang G, Bao S. The activation of Akt/PKB signaling pathway and cell survival. J Cell Mol Med. (2005) 9:59–71. doi: 10.1111/j.1582-4934.2005.tb00337.x
42. Lelouard H, Schmidt EK, Camosseto V, Clavarino G, Ceppi M, Hsu HT, et al. Regulation of translation is required for dendritic cell function and survival during activation. J Cell Biol. (2007) 179:1427–39. doi: 10.1083/jcb.200707166
43. Yu JS, Cui W. Proliferation, survival and metabolism: the role of PI3K/AKT/mTOR signalling in pluripotency and cell fate determination. Development. (2016) 143:3050–60. doi: 10.1242/dev.137075
44. Curnock AP, Logan MK, Ward SG. Chemokine signalling: pivoting around multiple phosphoinositide 3-kinases. Immunology. (2002) 105:125–36.
45. Neptune ER, Iiri T, Bourne HR. Galphai is not required for chemotaxis mediated by Gi-coupled receptors. J Biol Chem. (1999) 274:2824–8. doi: 10.1046/j.1365-2567.2002.01345.x
46. McCubrey JA, Steelman LS, Chappell WH, Abrams SL, Wong EW, Chang F, et al. Roles of the Raf/MEK/ERK pathway in cell growth, malignant transformation and drug resistance. Biochim Biophys Acta. (2007) 1773:1263–84. doi: 10.1016/j.bbamcr.2006.10.001
47. Bonacchi A, Romagnani P, Romanelli RG, Efsen E, Annunziato F, Lasagni L, et al. Signal transduction by the chemokine receptor CXCR3: activation of Ras/ERK, Src, and phosphatidylinositol 3-kinase/Akt controls cell migration and proliferation in human vascular pericytes. J Biol Chem. (2001) 276:9945–54. doi: 10.1074/jbc.M010303200
48. Knall C, Young S, Nick JA, Buhl AM, Worthen GS, Johnson GL. Interleukin-8 regulation of the Ras/Raf/mitogen-activated protein kinase pathway in human neutrophils. J Biol Chem. (1996) 271:2832–8.
49. Jimenez-Sainz MC, Fast B, Mayor F Jr., Aragay AM. Signaling pathways for monocyte chemoattractant protein 1-mediated extracellular signal-regulated kinase activation. Mol Pharmacol. (2003) 64:773–82. doi: 10.1124/mol.64.3.773
50. Crespo P, Xu N, Simonds WF, Gutkind JS. Ras-dependent activation of MAP kinase pathway mediated by G-protein beta gamma subunits. Nature. (1994) 369:418–20.
51. Koch WJ, Hawes BE, Allen LF, Lefkowitz RJ. Direct evidence that Gi-coupled receptor stimulation of mitogen-activated protein kinase is mediated by G beta gamma activation of p21ras. Proc Natl Acad Sci USA. (1994) 91:12706–10.
52. Sun Y, Liu WZ, Liu T, Feng X, Yang N, Zhou HF. Signaling pathway of MAPK/ERK in cell proliferation, differentiation, migration, senescence and apoptosis. J Recept Signal Transduct Res. (2015) 35:600–4. doi: 10.3109/10799893.2015.1030412
53. Wang MJ, Artemenko Y, Cai WJ, Iglesias PA, Devreotes PN. The directional response of chemotactic cells depends on a balance between cytoskeletal architecture and the external gradient. Cell Rep. (2014) 9:1110–21. doi: 10.1016/j.celrep.2014.09.047
54. Zheng L, Eckerdal J, Dimitrijevic I, Andersson T. Chemotactic peptide-induced activation of Ras in human neutrophils is associated with inhibition of p120-GAP activity. J Biol Chem. (1997) 272:23448–54.
55. Sasaki AT, Chun C, Takeda K, Firtel RA. Localized Ras signaling at the leading edge regulates PI3K, cell polarity, and directional cell movement. J Cell Biol. (2004) 167:505–18. doi: 10.1083/jcb.200406177
56. Zhang S, Charest PG, Firtel RA. Spatiotemporal regulation of Ras activity provides directional sensing. Curr Biol. (2008) 18:1587–93. doi: 10.1016/j.cub.2008.08.069
57. Agarwal P, Zaidel-Bar R. Principles of actomyosin regulation in vivo. Trends Cell Biol. (2019) 29:150–63. doi: 10.1016/j.tcb.2018.09.006
58. Engqvist-Goldstein AE, Drubin DG. Actin assembly and endocytosis: from yeast to mammals. Annu Rev Cell Dev Biol. (2003) 19:287–332. doi: 10.1146/annurev.cellbio.19.111401.093127
59. Jaffe AB, Hall A. Rho GTPases: biochemistry and biology. Annu Rev Cell Dev Biol. (2005) 21:247–69. doi: 10.1146/annurev.cellbio.21.020604.150721
60. Mooren OL, Galletta BJ, Cooper JA. Roles for actin assembly in endocytosis. Annu Rev Biochem. (2012) 81:661–86. doi: 10.1146/annurev-biochem-060910-094416
61. Pollard TD, Borisy GG. Cellular motility driven by assembly and disassembly of actin filaments. Cell. (2003) 112:453–65. doi: 10.1016/s0092-8674(03)00120-x
62. Zhou X, Zheng Y. Cell type-specific signaling function of RhoA GTPase: lessons from mouse gene targeting. J Biol Chem. (2013) 288:36179–88. doi: 10.1074/jbc.R113.515486
63. Kobayashi M, Azuma E, Ido M, Hirayama M, Jiang Q, Iwamoto S, et al. A pivotal role of Rho GTPase in the regulation of morphology and function of dendritic cells. J Immunol. (2001) 167:3585–91. doi: 10.4049/jimmunol.167.7.3585
64. Xu Y, Pektor S, Balkow S, Hemkemeyer SA, Liu Z, Grobe K, et al. Dendritic cell motility and T cell activation requires regulation of Rho-cofilin signaling by the Rho-GTPase activating protein myosin IXb. J Immunol. (2014) 192:3559–68. doi: 10.4049/jimmunol.1300695
65. Siehler S. Regulation of RhoGEF proteins by G12/13-coupled receptors. Br J Pharmacol. (2009) 158:41–9. doi: 10.1111/j.1476-5381.2009.00121.x
66. Shi CS, Sinnarajah S, Cho H, Kozasa T, Kehrl JH. G13alpha-mediated PYK2 activation. PYK2 is a mediator of G13alpha -induced serum response element-dependent transcription. J Biol Chem. (2000) 275:24470–6. doi: 10.1074/jbc.M908449199
67. Okigaki M, Davis C, Falasca M, Harroch S, Felsenfeld DP, Sheetz MP, et al. Pyk2 regulates multiple signaling events crucial for macrophage morphology and migration. Proc Natl Acad Sci USA. (2003) 100:10740–5. doi: 10.1073/pnas.1834348100
68. Ricart BG, John B, Lee D, Hunter CA, Hammer DA. Dendritic cells distinguish individual chemokine signals through CCR7 and CXCR4. J Immunol. (2011) 186:53–61. doi: 10.4049/jimmunol.1002358
69. Vargas P, Maiuri P, Bretou M, Saez PJ, Pierobon P, Maurin M, et al. Innate control of actin nucleation determines two distinct migration behaviours in dendritic cells. Nat Cell Biol. (2016) 18:43–53. doi: 10.1038/ncb3284
70. Tanizaki H, Egawa G, Inaba K, Honda T, Nakajima S, Moniaga CS, et al. Rho-mDia1 pathway is required for adhesion, migration, and T-cell stimulation in dendritic cells. Blood. (2010) 116:5875–84. doi: 10.1182/blood-2010-01-264150
71. Wu C, Asokan SB, Berginski ME, Haynes EM, Sharpless NE, Griffith JD, et al. Arp2/3 is critical for lamellipodia and response to extracellular matrix cues but is dispensable for chemotaxis. Cell. (2012) 148:973–87. doi: 10.1016/j.cell.2011.12.034
72. Mendoza MC, Vilela M, Juarez JE, Blenis J, Danuser GERK. reinforces actin polymerization to power persistent edge protrusion during motility. Sci Signal. (2015) 8:ra47. doi: 10.1126/scisignal.aaa8859
73. Laufer JM, Hauser MA, Kindinger I, Purvanov V, Pauli A, Legler DF. Chemokine receptor CCR7 triggers an endomembrane signaling complex for spatial rac activation. Cell Rep. (2019) 29:995–1009e6. doi: 10.1016/j.celrep.2019.09.031
74. Sallusto F, Lanzavecchia A. Efficient presentation of soluble antigen by cultured human dendritic cells is maintained by granulocyte/macrophage colony-stimulating factor plus interleukin 4 and downregulated by tumor necrosis factor alpha. J Exp Med. (1994) 179: 1109–18.
Keywords: C–C chemokine receptor 7, signaling, leukocyte, MAPK pathway, RhoA pathway, PI3 K/Akt pathway, Chemotaxis (MeSH ID D002633)
Citation: Rodríguez-Fernández JL and Criado-García O (2020) The Chemokine Receptor CCR7 Uses Distinct Signaling Modules With Biased Functionality to Regulate Dendritic Cells. Front. Immunol. 11:528. doi: 10.3389/fimmu.2020.00528
Received: 21 November 2019; Accepted: 09 March 2020;
Published: 15 April 2020.
Edited by:
Mette M. Rosenkilde, University of Copenhagen, DenmarkReviewed by:
Gertrud Malene Hjortø, University of Copenhagen, DenmarkLuciana D’Apice, Institute of Protein Biochemistry (CNR), Italy
Copyright © 2020 Rodríguez-Fernández and Criado-García. This is an open-access article distributed under the terms of the Creative Commons Attribution License (CC BY). The use, distribution or reproduction in other forums is permitted, provided the original author(s) and the copyright owner(s) are credited and that the original publication in this journal is cited, in accordance with accepted academic practice. No use, distribution or reproduction is permitted which does not comply with these terms.
*Correspondence: José Luis Rodríguez-Fernández, rodrifer@cib.csic.es