GAP-43 and BASP1 in Axon Regeneration: Implications for the Treatment of Neurodegenerative Diseases
- Department of Neurology, Feinberg School of Medicine, Northwestern University, Chicago, IL, United States
Growth-associated protein-43 (GAP-43) and brain acid-soluble protein 1 (BASP1) regulate actin dynamics and presynaptic vesicle cycling at axon terminals, thereby facilitating axonal growth, regeneration, and plasticity. These functions highly depend on changes in GAP-43 and BASP1 expression levels and post-translational modifications such as phosphorylation. Interestingly, examinations of GAP-43 and BASP1 in neurodegenerative diseases reveal alterations in their expression and phosphorylation profiles. This review provides an overview of the structural properties, regulations, and functions of GAP-43 and BASP1, highlighting their involvement in neural injury response and regeneration. By discussing GAP-43 and BASP1 in the context of neurodegenerative diseases, we also explore the therapeutic potential of modulating their activities to compensate for neuron loss in neurodegenerative diseases.
Introduction
Axons integrate external cues to grow toward and arborize their terminals onto their correct targets. Such axonal behavior is critical for establishing proper connections, regenerating injured nerves, and retaining anatomical plasticity in adult brains (Skene, 1989). A group of growth-associated proteins (>100) (Costigan et al., 2002; Xiao et al., 2002), whose expression is upregulated during neuronal development and regeneration, mediates these functions (Jacobson et al., 1986; Widmer and Caroni, 1990; Mason, 2002). Growth-associated protein-43 (GAP-43) and brain acid-soluble protein 1 (BASP1) are two members of this group with many shared structural properties and functions (Mosevitsky, 2005). GAP-43 is solely expressed in the nervous system (Karns et al., 1987), whereas BASP1 is highly expressed in the nervous system and some non-neural tissues including the kidney and testis (Mosevitsky et al., 1997; Mosevitsky and Silicheva, 2011). Within neurons, GAP-43, and BASP1 are enriched in axon terminals (Meiri et al., 1986; Widmer and Caroni, 1990), where they regulate the actin cytoskeleton (Wiederkehr et al., 1997; Laux et al., 2000). By modulating actin dynamics, GAP-43, and BASP1 achieve their physiological functions in neurodevelopment, synaptic function, and nerve regeneration.
Physiological Functions in the Nervous System
Neurodevelopment
Growth-associated protein-43 and BASP1 are highly expressed during periods of active axon growth and synaptogenesis (McGuire et al., 1988; De La Monte et al., 1989; Widmer and Caroni, 1990). Their critical involvement in neurodevelopment has been demonstrated by gene knockout studies. Homozygous knockout of GAP-43 (GAP-43–/–) or BASP1 (BASP1–/–) leads to high neonatal lethality, resulting in 5–10% survival to adulthood (Strittmatter et al., 1995; Frey et al., 2000; Metz and Schwab, 2004). Surviving GAP-43–/– animals exhibit defective pathfinding of retinal and commissural axons (Strittmatter et al., 1995; Shen et al., 2002), as well as an abnormal somatotopic map in the barrel cortex (Maier et al., 1999). In reflection of these anatomical defects, GAP-43–/– animals demonstrate motor, sensory, and behavioral impairments (Metz and Schwab, 2004). Surviving BASP1–/– animals also present evidence of impaired neurodevelopment including enlarged ventricles in the brain, axonal and synaptic abnormalities in the neocortex and hippocampus, and hyperactive behavior (Frey et al., 2000).
Synaptic Function
As axons complete the innervation of their target areas, GAP-43 and BASP1 are downregulated in most brain regions. Interestingly, they remain highly expressed in areas of the adult brain implicated in learning and memory, including the neocortex and hippocampus (Benowitz et al., 1988; McGuire et al., 1988; Neve et al., 1988; Frey et al., 2000). In support of their importance in information storage, heterozygous GAP-43 knockout mice (GAP-43+/–) exhibit a selective impairment in contextual memory (Rekart et al., 2005). This phenotype can be explained by various synaptic functions of GAP-43. GAP-43 was shown to regulate endocytosis via its interaction with rabaptin-5, which functions in endocytic membrane fusion (Neve et al., 1998), and as a substrate of caspase-3, which mediates AMPA receptor endocytosis (Han et al., 2013). Also, antibodies against GAP-43 decreased the release of glutamate and noradrenaline (Dekker et al., 1989a; Hens et al., 1998), indicating its importance in neurotransmitter release. This function is thought to be mediated, at least in part, by its interaction with the presynaptic vesicle fusion complex (Syntaxin, SNAP-25, and VAMP) (Haruta et al., 1997). Moreover, GAP-43 was shown to enhance long-term potentiation (Routtenberg and Lovinger, 1985; Lovinger et al., 1986; Hulo et al., 2002). The presence of BASP1 on synaptic vesicles (Yamamoto et al., 1997) and its identification as a caspase-3 substrate (Han et al., 2013) suggest its potential role in synaptic vesicle cycling, which may have implications for neurotransmission, synaptic plasticity, and information storage.
Nerve Regeneration
Another circumstance under which GAP-43 and BASP1 are upregulated is nerve regeneration following injury (Skene and Willard, 1981; Caroni et al., 1997). An increase in GAP-43 and BASP1 mRNA levels strongly correlates with enhanced regenerative capacity. This is evidenced by their robust upregulation in the regenerating dorsal root ganglion (DRG) axons following sciatic nerve injury but not in the non-regenerating DRG axons following dorsal rhizotomy (Mason, 2002). The upregulation of GAP-43 and BASP1 also correlated with axonal sprouting after stroke in the barrel cortex (Carmichael et al., 2005). Additionally, an increase in GAP-43 was associated with optogenetic-induced functional recovery from stroke in the primary motor cortex (Cheng et al., 2014). In a different rodent model of stroke, antisense oligonucleotides to GAP-43 abolished the enhancement of functional recovery induced by the basic fibroblast growth factor (Kawamata et al., 1999). These observations from rodent stroke models hint at the importance of GAP-43 in neuronal recovery following injury. More importantly, GAP-43 was shown to be essential for the regenerative response through knockdown studies. In adult rodents, climbing fibers retain high levels of GAP-43 and demonstrate structural plasticity after injury (Grasselli and Strata, 2013). Knockdown of GAP-43 in climbing fibers inhibited the sprouting of their axonal branches following laser-axotomy (Allegra Mascaro et al., 2013) and lesion of the inferior olive, where these fibers originate (Grasselli et al., 2011). Overexpression studies involving GAP-43 and BASP1 show these proteins are sufficient for f-actin accumulation and subsequent neurite formation in primary sensory neurons (Aigner and Caroni, 1995) and in Purkinje neurons (Buffo et al., 1997). Moreover, co-overexpression of GAP-43 and BASP1 was sufficient to drive the regeneration of DRG axons following spinal cord lesion in adult mice when peripheral nerve graft was provided (Bomze et al., 2001).
Involvement in Neural Injury Response
In the central (CNS) and peripheral nervous systems (PNS), different external and internal factors lead to disparate outcomes of injury response. In the CNS, glial scar (Silver and Miller, 2004) and inhibitory glial factors such as myelin-associated glycoproteins and Nogo (Filbin, 2003) impede neuronal regeneration. Additionally, the inherent lack of axonal integrins and growth factor receptors limit the regrowth of neurons in the CNS (Koseki et al., 2017). As a result, positive outcomes of CNS injury remain limited to neuroprotection and marginal regenerative response. Neural injury stimulates neurons and glia to release cytokines and neurotrophic factors, which can activate growth-associated proteins such as GAP-43 and BASP1 to promote neuroprotection and regeneration.
Cytokine Signaling
Upon injury, leakage from damaged or dying cells leads to an excess of glutamate in the extracellular space (Bullock et al., 1998). This stimulates astrocytes, microglia, and neurons to secrete cytokines such as interleukin-6 (IL-6) and -10 (IL-10) (Morganti-Kossman et al., 1997; Acarin et al., 2000). Accordingly, IL-6 and -10 were found to be elevated in the cerebrospinal fluid and serum of patients with severe traumatic brain injury (TBI) (Kossmann et al., 1995; Csuka et al., 1999). After spinal cord injury, IL-6 treatment was shown to activate the JAK/STAT3 and PI3K/Akt pathways, upregulate GAP-43 and BASP1, and promote neurite outgrowth in vitro and synaptogenesis in vivo (Yang et al., 2012, 2015; Figure 1). The upregulation of GAP-43 and BASP1 was sensitive to the JAK2 inhibitor AG490 (Yang et al., 2015) but was not examined with a PI3K inhibitor. After oxygen-glucose deprivation, IL-10 treatment was shown to activate the JAK/STAT3 and PI3K/Akt pathways, upregulate GAP-43, and facilitate neuroprotection, neurite outgrowth, and synaptogenesis in vitro (Lin et al., 2015; Chen et al., 2016; Figure 1). The upregulation of GAP-43 was shown to be sensitive to the PI3K inhibitor LY294002 (Lin et al., 2015) but was not examined with a JAK2 inhibitor. The extent to which the neurite outgrowth observed in vitro translates into in vivo regeneration remains unclear.
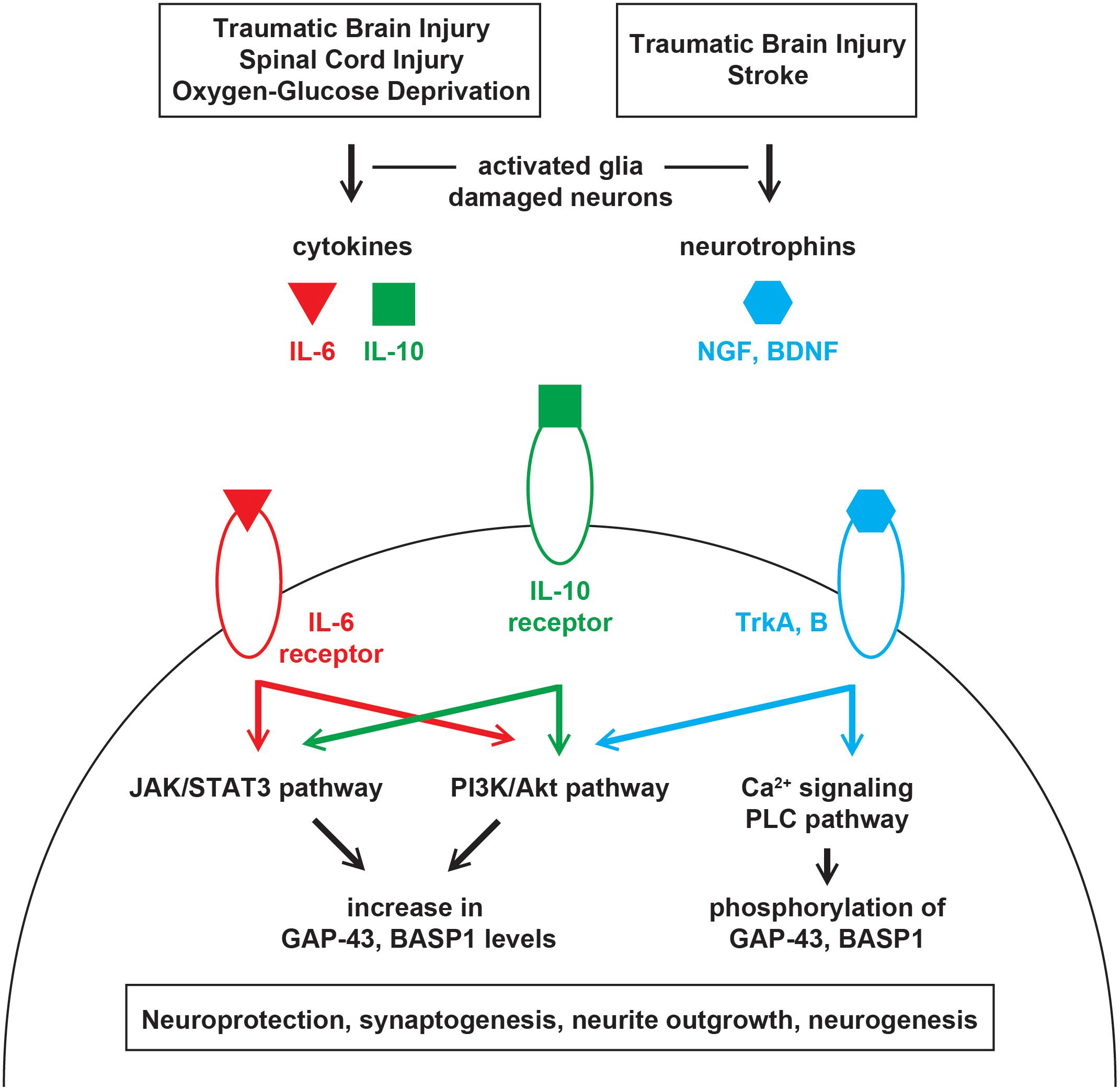
Figure 1. Injury-induced signaling pathways that regulate GAP-43 and BASP1. Injuries in the central nervous system leads to the release of cytokines and neurotrophins from damaged neurons and activated glia. Cytokines and neurotrophins bind their receptors on surviving neurons to activate various signaling cascades that regulate the expression level and phosphorylation of GAP-43 and BASP1. Alterations in GAP-43 and BASP1 levels and phosphorylation are associated with neuroprotection, synaptogenesis, neurite outgrowth, and neurogenesis.
Neurotrophic Factor Signaling
Following injury, neurotrophic factors such as the nerve growth factor (NGF) (DeKosky et al., 1994; Chiaretti et al., 2009) and brain-derived neurotrophic factor (BDNF) (Miyake et al., 2002; Rostami et al., 2014) are upregulated in response to glutamate (Wetmore et al., 1994; Gwag et al., 1997) and cytokines (Kossmann et al., 1996). NGF and BDNF bind tropomyosin receptor kinase A and B, respectively, to initiate cell survival signaling via the PI3K/Akt pathway (Nguyen et al., 2010; Figure 1). In rodent models of stroke and TBI, the activation of NGF and BDNF signaling was shown to promote neuroprotection, synaptogenesis, and neurogenesis (Wu et al., 2008; Qi et al., 2014; Gudasheva et al., 2019). Such beneficial upregulation of NGF and BDNF was accompanied by an increase in GAP-43 levels when optogenetic stimulations were provided after stroke (Cheng et al., 2014). Given that BASP1 is similarly upregulated after stroke (Carmichael et al., 2005), its increase may also be mediated by NGF and BDNF. Moreover, GAP-43 was shown to be an essential effector of BDNF-driven neuroprotection (Gupta et al., 2009).
Structural Properties and Domains
Intrinsic Disorder and Phase Separation
Growth-associated protein-43 and BASP1 are acidic proteins with isoelectric points of 4.4–4.6 (Mosevitsky et al., 1994). Their molecular weights are 23–25 kDa, but they appear at higher molecular weights on SDS-PAGE (Mosevitsky et al., 1994). These proteins are enriched in alanine (22% in GAP-43, 21% in BASP1) and proline (8% in GAP-43, 12% in BASP1), which gives rise to a high content of type II polyproline helix (32 ± 5% in GAP-43, 37 ± 2% in BASP1) characteristic of intrinsically disordered proteins (Forsova and Zakharov, 2016). Nuclear magnetic resonance spectroscopy also indicates that GAP-43 and BASP1 have unordered structures (Zhang et al., 1994; Geist et al., 2013). Intrinsically disordered proteins lack a stable three-dimensional structure and have the ability to engage in multivalent interactions (Haynes et al., 2006). Through multivalent interactions, intrinsically disordered proteins undergo liquid-liquid phase separation and form membraneless compartments that facilitate their functions (Li et al., 2012). Many proteins involved in actin assembly have intrinsically disordered regions, and phase separation mediated by these regions underlies their regulation of actin dynamics (Sun et al., 2017; Miao et al., 2018). Moreover, phosphorylation of intrinsically disordered proteins affects phase separation by modulating their charge and electrostatic interactions (Aumiller and Keating, 2016; Miao et al., 2018). Given that GAP-43 and BASP1 are phosphoproteins, they may transmit signals from kinases and phosphatases to the actin cytoskeleton via phase separation.
PEST Sequence and High Turnover Rate
Growth-associated protein-43 and BASP1 have peptide sequences rich in proline, glutamate, serine, and threonine (PEST) (Barnes and Gomes, 1995; Mosevitsky et al., 1997). While PEST regions vary in their sequences and lengths, they all serve as signals for rapid proteolysis (Rechsteiner and Rogers, 1996). The presence of PEST sequences indicates that GAP-43 and BASP1 are short-lived proteins, however, this has yet to be experimentally verified.
Effector Domain and CaM Binding
Growth-associated protein-43 and BASP1 have regions termed the effector domain (ED) that are enriched in basic and hydrophobic residues, bind calmodulin (CaM), and are phosphorylated by protein kinase C (PKC) (Cimler et al., 1985; Apel et al., 1990; Maekawa et al., 1993). The basic and hydrophobic residues in the ED also contribute to membrane association of these proteins (O’Neil and DeGrado, 1990; Mosevitsky et al., 1997; Mosevitsky, 2005). GAP-43 ED consists of residues 37–52 (KIQASFRGHITRKKLK) (Mosevitsky, 2005; Figure 2), which includes a canonical CaM-binding site termed the IQ motif (IQxxxRGxxxR) (Bähler and Rhoads, 2002). GAP-43 was shown to bind CaM with higher affinity in the absence of or at low Ca2+ and to dissociate at high Ca2+ (Andreasen et al., 1983; Alexander et al., 1987; Gamby et al., 1996). Hence, GAP-43 has been proposed to accumulate CaM at specific sites and release them upon local Ca2+ elevation to sharpen the downstream response (Alexander et al., 1987; Mosevitsky, 2005). Unlike GAP-43, BASP1 ED is located at the N-terminal end (Myristoylation-GGKLSKKKKGY) (Mosevitsky, 2005; Figure 2). BASP1 lacks an IQ motif and instead binds CaM through alternating basic and hydrophobic residues (Takasaki et al., 1999). These alternating parts include the myristoyl moiety, which passes through a tunnel formed by hydrophobic pockets in the N- and C-terminal domains of CaM (Takasaki et al., 1999; Matsubara et al., 2004). BASP1 was shown to bind CaM with stronger affinity than GAP-43 (Maekawa et al., 1994) and in the presence of Ca2+ (Maekawa et al., 1993), suggesting a different mode of action than GAP-43.
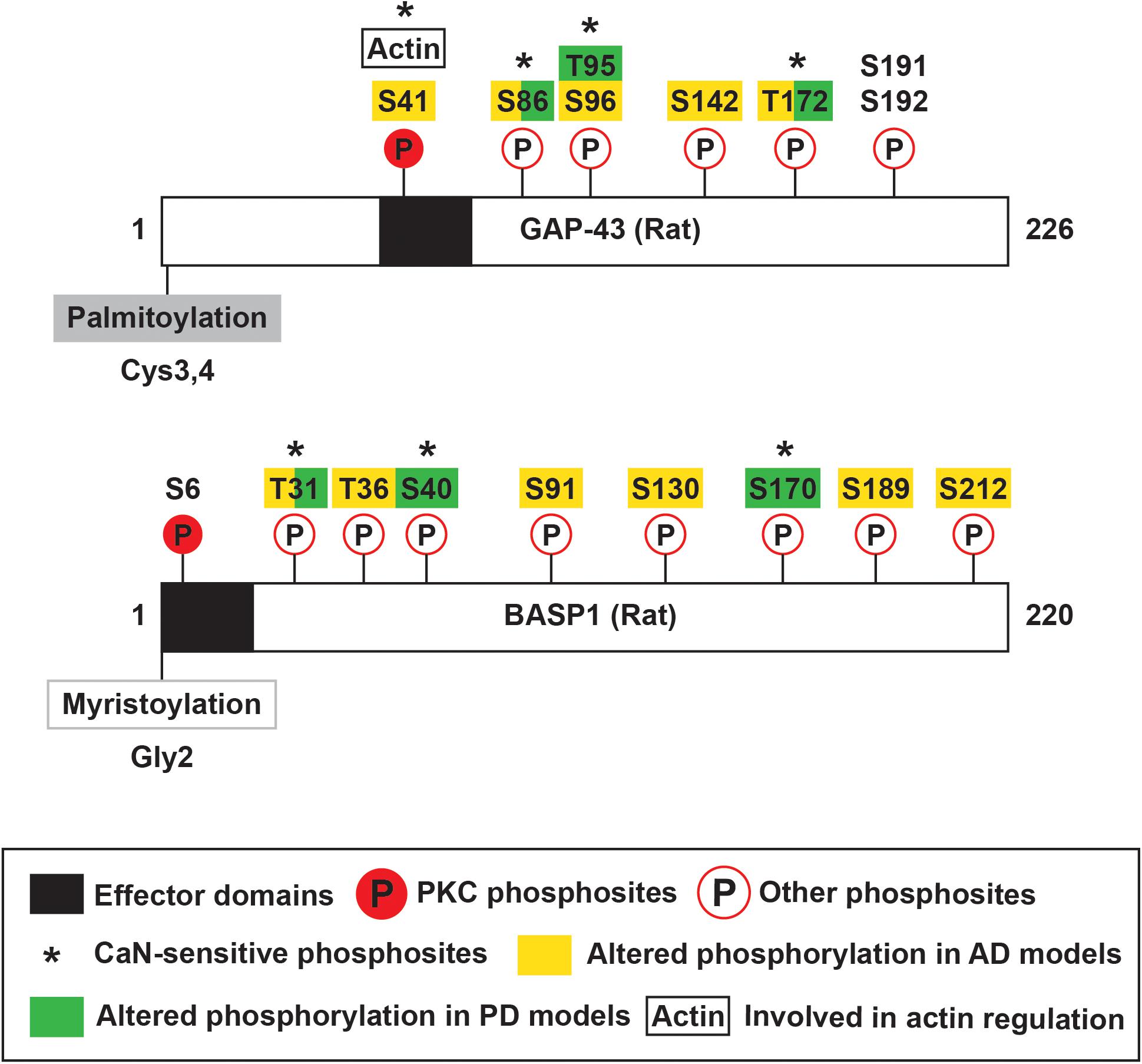
Figure 2. Schematic of rat GAP-43 and BASP1 structure and post-translational modifications. GAP-43 and BASP1 are characterized by N-terminal fatty acylation, effector domains, and multiple phosphosites. GAP-43 is palmitoylated at Cysteine-3 and -4, and BASP1 is myristoylated at the N-terminal end. Their effector domains enable calmodulin binding and are subjected to phosphorylation by PKC. Phosphorylation of GAP-43 by PKC regulates various functions including actin cytoskeleton dynamics. GAP-43 and BASP1 are also regulated by other kinases and phosphatases, and such post-translational modifications are implicated in Alzheimer’s and Parkinson’s Diseases.
Formation of Oligomers
Growth-associated protein-43 and BASP1 form oligomers in the presence of anionic phospholipids or sodium dodecyl sulfate (SDS) (Zakharov and Mosevitsky, 2010). Among anionic phospholipids, phosphatidylinositol 4,5-bisphosphate (PI(4,5)P2) was the most potent driver of oligomerization (Zakharov and Mosevitsky, 2010). Interestingly, GAP-43 and BASP1 accumulate PI(4,5)P2 on the inner surface of the plasma membrane, and this clustering is important for initiating signaling cascades that regulate the actin cytoskeleton (Laux et al., 2000). The role of PI(4,5)P2 in driving oligomerization suggests that PI(4,5)P2-induced oligomers may be important for this process. Oligomerization of GAP-43 and BASP1 results in α-helix formation within their EDs while preserving the overall structural disorder (Forsova and Zakharov, 2016). A significant level of disorder in oligomers has been proposed to enhance the flexibility of interactions and to enable the reversion to monomers (Tompa and Fuxreiter, 2008). In support of the flexibility in binding, GAP-43 and BASP1 were observed to form heterooligomers of different stoichiometries in vitro and in presynaptic membranes (Forsova and Zakharov, 2016). In agreement with the reversibility, GAP-43 and BASP1 oligomers were shown to dissociate into monomers upon removal of SDS or binding of CaM (Zakharov and Mosevitsky, 2010).
Transcriptional and Post-Transcriptional Regulation
In developing and regenerating neurons, the levels of GAP-43 and BASP1 mRNAs and proteins are upregulated (Widmer and Caroni, 1990; Perrone-Bizzozero et al., 1991; Mason, 2002). Studies present conflicting findings regarding the contributions of transcriptional activation to increased GAP-43 levels. The GAP-43 gene has two promoters, distal (P1) and proximal (P2), that are highly conserved between the rat and human genes (Eggen et al., 1994; de Groen et al., 1995). P1 contains classical promoter elements including TATA and CCAAT boxes (Nedivi et al., 1992), as well as a repressive element shown to inhibit GAP-43 expression in non-neuronal cells (Weber and Skene, 1997). P2 lacks classical promoter elements but contains a conserved enhancer box (E1) that can bind basic helix-loop-helix transcription factors (Chiaramello et al., 1996), which have critical roles in neural cell fate specification and differentiation (Dennis et al., 2019). In zebrafish, a 1 kb fragment spanning P1 and P2 of the rat GAP-43 gene was shown to developmentally regulate the expression of a downstream transgene in neurons (Udvadia et al., 2001). In contrast to this finding, developing rat cortical neurons and nerve growth factor-induced PC12 cells showed no change in GAP-43 pre-mRNA levels despite an increase in GAP-43 mRNA levels (Perrone-Bizzozero et al., 1991). This finding indicates that mRNA stability mainly contributes to the observed upregulation of GAP-43. The stabilization of GAP-43 mRNA is dependent on the highly conserved 3′ untranslated region (Kohn et al., 1996; Tsai et al., 1997), where the neural-specific RNA-binding protein HuD binds (Chung et al., 1997). The expression of HuD and GAP-43 are concomitantly increased during neuritogenesis (Anderson et al., 2001), in regenerating nerves (Anderson et al., 2003), and following spatial learning in rodent hippocampi (Quattrone et al., 2001; Pascale et al., 2004). Based on these observations, HuD was hypothesized to stabilize GAP-43 mRNA under physiological conditions. In support of this hypothesis, transgenic mice overexpressing HuD exhibited an increase in GAP-43 mRNA but not pre-mRNA (Bolognani et al., 2006). Also, the half-life of GAP-43 mRNA from these transgenic mice was significantly longer than those from non-transgenic controls (Bolognani et al., 2006). The HuD-dependent stabilization of GAP-43 mRNA is positively regulated by PKC (Perrone-Bizzozero et al., 1993; Sanna et al., 2014) and is inhibited by the KH-type splicing regulatory protein, which competes with HuD to bind and promote the degradation of GAP-43 mRNA (Bird et al., 2013). Compared to GAP-43, little is known about the transcriptional and post-transcriptional control of BASP1. In the chicken BASP1 gene, a 135 bp region in the 5′ end of exon 1 was shown to bind the transcription factors Sp1 and Myc (Hartl et al., 2009). This regulatory region was sufficient to activate transcription and to mediate Myc-induced suppression of BASP1 (Hartl et al., 2009). Additionally, post-transcriptional regulation of BASP1 by its processed pseudogene has been proposed but not experimentally verified (Uzumcu et al., 2009).
Post-Translational Modifications
Fatty Acylation
Growth-associated protein-43 and BASP1 mainly localize to membranes (Skene et al., 1986; Maekawa et al., 1993), and their membrane associations are partially mediated by fatty acylation. GAP-43 is post-translationally palmitoylated at Cysteine-3 and -4 (Skene and Virág, 1989; Figure 2). Its palmitoylation can occur in the endoplasmic reticulum-Golgi intermediate compartment (ERGIC), Golgi apparatus, and plasma membrane (McLaughlin and Denny, 1999). Upon palmitoylation, GAP-43 can be sorted to the tips of growing neurites (Gauthier-Kemper et al., 2014). Palmitoylation of GAP-43 is dynamically regulated, as suggested by the low percentage (∼35%) of fatty acylated GAP-43 at steady state in PC12 and COS-1 cells (Liang et al., 2002). The dynamic regulation of palmitoylation affects GAP-43 functions. Changes in palmitoylation enable GAP-43 to cycle between pathways independent of and involving Go, a heterotrimeric GTP-binding protein enriched in growth cones (Edmonds et al., 1990). N-terminal peptides of GAP-43 produced by the Ca2+-dependent protease m-calpain interact with and activate Go signaling cascade that leads to growth cone collapse (Strittmatter et al., 1994; Zakharov and Mosevitsky, 2007; Figure 3). Palmitoylation reduces the ability of the N-terminal peptides to stimulate Go, thereby blocking Go signaling-induced growth cone collapse (Sudo et al., 1992). Additionally, palmitoylation appears to be important for the switch from promoting axon growth to stabilizing synapses upon successful target innervation (Patterson and Skene, 1999). Experimental evidence shows that palmitoylation of GAP-43, when inhibited, reversibly stalls neurite outgrowth (Hess et al., 1993) and is significantly reduced at the early phase of synapse maturation (Patterson and Skene, 1999). Unlike GAP-43, BASP1 is co-translationally myristoylated at the N-terminal end (Mosevitsky et al., 1997; Takasaki et al., 1999; Figure 2). In the rat brain, BASP1 molecules and N-terminal fragments appear predominantly in the myristoylated form (Mosevitsky et al., 1997; Zakharov et al., 2003). Myristoylation of BASP1 was shown to promote membrane association and to enable CaM binding (Takasaki et al., 1999; Matsubara et al., 2004).
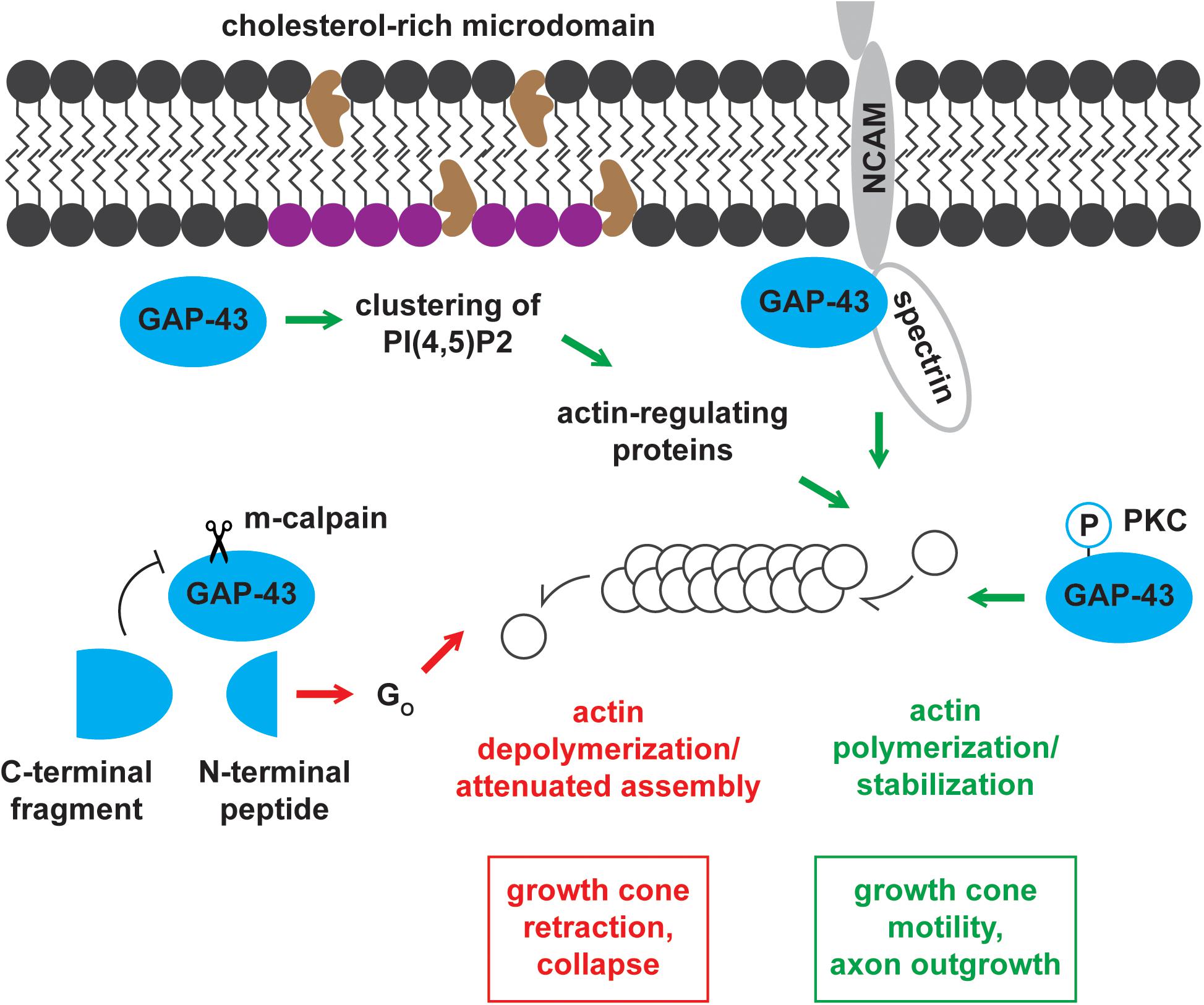
Figure 3. Mechanisms of actin cytoskeleton regulation by GAP-43. GAP-43 positively and negatively regulates the actin cytoskeleton through multiple routes of action. GAP-43 promotes growth cone motility and axon outgrowth by (1) recruiting actin-regulating proteins by forming PI(4,5)P2 clusters, (2) interacting with NCAM and spectrin, and (3) stabilizing actin filaments in a PKC-dependent manner. Conversely, m-calpain cleavage product of GAP-43 drives growth cone retraction and collapse through the Go signaling pathway.
Phosphorylation
Growth-associated protein-43 and BASP1 EDs undergo Ca2+-dependent phosphorylation by PKC. PKC phosphosites in GAP-43 and BASP1 are Serine-41 and Serine-6, respectively (Apel et al., 1990; Maekawa et al., 1994; Figure 2). GAP-43 Serine-41 can also be dephosphorylated by the Ca2+/CaM-dependent phosphatase calcineurin (CaN) (Liu and Storm, 1989). Phosphorylation by PKC abolishes CaM binding to both GAP-43 and BASP1 (Apel et al., 1990; Takasaki et al., 1999). More importantly, PKC-mediated phosphorylation has significant functional consequences. Phosphorylated GAP-43 not only promotes actin polymerization and stabilization (He et al., 1997; Korshunova et al., 2007) but also interacts with presynaptic vesicle fusion complex (Syntaxin, SNAP-25, and VAMP) (Haruta et al., 1997). Through these molecular pathways, phosphorylated GAP-43 facilitates axon guidance (Dent and Meiri, 1998), axon outgrowth (Aigner et al., 1995; Korshunova et al., 2007), neurotransmission (Dekker et al., 1989b; Heemskerk et al., 1990), and synaptic plasticity (Routtenberg and Lovinger, 1985; Lovinger et al., 1986; Hulo et al., 2002). The modulation of BASP1 functions by PKC remains to be studied. In addition to PKC phosphosites, many residues of GAP-43 and BASP1 were found to be phosphorylated. To name a few, GAP-43 was phosphorylated at Serine-96 and Threonine-172 by unknown kinase(s) (Spencer et al., 1992), Serine-191 and -192 by Casein Kinase II (Apel et al., 1991), and Serine-96 by c-Jun N-terminal kinase (JNK) (Kawasaki et al., 2018; Figure 2). Additional phosphosites on GAP-43 (Serine-86, Threonine-95, and Threonine-172) and BASP1 (Threonine-31, Serine-40, Serine-170) were found to be sensitive to CaN (Caraveo et al., 2017; Figure 2). The phosphorylation of GAP-43 and BASP1 at these other residues may be of functional significance. For example, JNK phosphorylates GAP-43 in growth cone membranes, and this modification is associated with axon growth and regeneration (Kawasaki et al., 2018).
Mechanisms of Actin Cytoskeleton Regulation
Regulation of the actin cytoskeleton is important for axon guidance and growth (Gomez and Letourneau, 2014; Blanquie and Bradke, 2018), endocytosis (Smythe and Ayscough, 2006), and exocytosis (Eitzen, 2003). Therefore, understanding how GAP-43 and BASP1 modulate actin dynamics provides insight into the mechanisms through which they achieve their physiological functions. To test if GAP-43 and BASP1 perform their functions through identical pathways, knockin mice expressing GAP-43 in place of BASP1 was generated (Frey et al., 2000). While these mice exhibited no gross abnormality in the brain, they showed small morphological differences in axon sprouts (Frey et al., 2000). This indicates that GAP-43 and BASP1 act through partially redundant pathways, a concept that was reinforced by observations of phenotypic differences between GAP-43- and BASP1-induced neurite outgrowth and of synergistic axon sprouting with co-overexpression of GAP-43 and BASP1 (Caroni et al., 1997).
Signaling Through PI(4,5)P2 Clusters
Growth-associated protein-43 and BASP1 regulate actin dynamics through a shared pathway involving PI(4,5)P2 (Laux et al., 2000; Caroni, 2001). In the intracellular surface of cholesterol-rich rafts, GAP-43 and BASP1 co-distribute with and promote the clustering of PI(4,5)P2 (Laux et al., 2000). Concentrating PI(4,5)P2 in local environments is thought to enhance the recruitment of actin-regulating proteins (Caroni, 2001). These proteins include WASP and ERM proteins known for their roles in actin polymerization and actin cytoskeleton-membrane crosslinking, respectively (Sechi and Wehland, 2000). The functions of WASP and ERM proteins contribute to growth cone motility and outgrowth (Figure 3).
PKC-Dependent Modulation of Actin
Growth-associated protein-43 binds and directly regulates actin filaments in a PKC-dependent manner (He et al., 1997). Phosphorylated GAP-43 binds actin with higher affinity (Kd = 161 nM) compared to the unphosphorylated form (Kd = 1.2 uM) (He et al., 1997). In accordance with this enhanced binding, the cytoskeletal association of GAP-43 increases with phosphorylation (Tejero-Díez et al., 2000). Moreover, phosphorylated GAP-43 stabilizes actin filaments, thereby promoting growth cone extension and motility (He et al., 1997; Figure 3). On the other hand, unphosphorylated GAP-43 inhibits growth cone extension likely by serving as a barbed end-capping protein (He et al., 1997). Capping proteins significantly increase the concentration of actin monomers needed for polymerization and attenuate actin assembly, which is critical for growth cone extension. While BASP1 may similarly regulate the actin cytoskeleton, this hypothesis remains to be tested.
Functional Association With NCAM-180 and Spectrin
Growth-associated protein-43 has also been proposed to modulate actin dynamics through the functional association with the neural cell adhesion molecule-180 (NCAM-180) and the cytoskeletal protein spectrin (Korshunova et al., 2007; Figure 3). NCAMs engage in homophilic and heterophilic binding with components of other cells and the extracellular matrix (ECM) (Ditlevsen et al., 2008). These interactions, in addition to establishing cell-cell and cell-ECM adhesions, initiate intracellular signaling cascades important for neuronal development, synaptic plasticity, and regeneration (Ditlevsen et al., 2008). NCAMs directly associate with intracellular signaling molecules to activate these downstream cascades (Ditlevsen et al., 2008). NCAM-180 has been shown to mediate neurite outgrowth by interacting with spectrin (Leshchyns’ka et al., 2003), and spectrin was shown to bind GAP-43 (Riederer and Routtenberg, 1999). Based on these observations, NCAM-180, spectrin, and GAP-43 have been hypothesized to function in a complex to mediate neurite outgrowth. In support of this hypothesis, NCAM-mediated neurite outgrowth, in the presence of GAP-43, required functional NCAM-180 and spectrin (Korshunova et al., 2007). BASP1 was shown to function independently of this mechanism in PC12 cells and hippocampal neurons, where BASP1 failed to substitute the stimulation of NCAM-mediated neurite outgrowth by GAP-43 (Korshunova et al., 2008).
Properties of GAP-43 and BASP1 and Their Link to Neurodegenerative Diseases
Intrinsic Disorder and Phase Separation in Pathological Protein Aggregation
Growth-associated protein-43 and BASP1 are intrinsically disordered proteins (Zhang et al., 1994; Geist et al., 2013; Forsova and Zakharov, 2016). The functional significance of this property remains understudied in both physiological and pathological contexts. In many neurodegenerative diseases, intrinsically disordered proteins form soluble and fibril aggregates that are central to the pathogenesis (Chiti and Dobson, 2006). The recent discovery of liquid-liquid phase separation, which condensates disordered proteins, gained interest as a potential mechanism of pathological protein aggregation (Elbaum-Garfinkle, 2019). Furthermore, proteins forming aggregates in neurodegenerative diseases such as Tau (Wegmann et al., 2018) and Huntingtin (Peskett et al., 2018) were shown to undergo phase separation. The involvement of disordered proteins in neurodegenerative diseases questions whether GAP-43 and BASP1 also phase separate in this context, whether this process turns aberrant, and what the functional consequences are.
Post-transcriptional Regulation in Neurodegenerative Diseases
Post-transcriptional regulation allows a rapid adjustment of the location and level of protein expression (Bronicki and Jasmin, 2013). This spatiotemporal regulation is particularly important in neurons because of their large and complex morphology (Bronicki and Jasmin, 2013). A key regulator of this process is the neuron-specific RNA-binding protein HuD (Bronicki and Jasmin, 2013). HuD post-transcriptionally regulates GAP-43 during neuritogenesis, regeneration, and learning (Anderson et al., 2001, 2003; Quattrone et al., 2001; Pascale et al., 2004). Interestingly, HuD is associated with various neurodegenerative disorders. The level of HuD was shown to be decreased in the hippocampus of Alzheimer’s Disease patients (Amadio et al., 2009). This decrease correlated with diminished expression of ADAM10, a protein known to reduce the generation of pathogenic amyloid-β peptides (Amadio et al., 2009). From genetic studies, HuD was also identified as a susceptibility gene for Parkinson’s Disease (Noureddine et al., 2005; DeStefano et al., 2008). Additionally, HuD was shown to aberrantly interact with FUS mutant that is causally linked to Amyotrophic Lateral Sclerosis (De Santis et al., 2019). In light of these findings, the post-transcriptional regulation of GAP-43 may be dysregulated in neurodegenerative disorders.
PKC and CaM in Neurodegenerative Diseases
Growth-associated protein-43 and BASP1 engage in intracellular Ca2+ signaling through PKC and CaM (Cimler et al., 1985; Apel et al., 1990; Maekawa et al., 1993). Ca2+ signaling plays a central role in neuronal physiology, and Ca2+ dyshomeostasis contributes to the pathogenesis of neurodegenerative disorders (Bezprozvanny, 2009). As part of Ca2+ signaling cascades, PKC and CaM significantly impact the disease states.
Protein kinase C has a vital role in memory encoding and storage (Sun and Alkon, 2014); therefore, its involvement in Alzheimer’s Disease – a major form of dementia – has been studied extensively. Few studies showed that the activity of PKC is decreased in the brains of Alzheimer’s Disease patients (Wang et al., 1994; Matsushima et al., 1996), suggesting potential adverse effects of its diminished activity. In alignment with this finding, the PKC activator bryostatin reduced the production of amyloid-β peptides and premature mortality in mouse models of Alzheimer’s Disease (Etcheberrigaray et al., 2004). On the other hand, whole genome-sequencing of late-onset Alzheimer’s Disease patients identified gain-of-function mutations in PKC (Alfonso et al., 2016). This study demonstrated that enhanced PKC activity mediates synaptic depression induced by amyloid-β peptides (Alfonso et al., 2016). Other studies also linked PKC activation to synaptic changes such as reduced cell-surface expression of AMPA receptor (Liu et al., 2010) and dysregulated structural plasticity (Calabrese and Halpain, 2005). These findings point to the importance of balanced PKC activity in neuronal physiology. They also raise the possibility that PKC-dependent functions of GAP-43 and BASP1 affect cellular pathways whose dyshomeostasis contribute to neurodegenerative diseases.
Calmodulin connects Ca2+ signals to cellular functions through various effector proteins (Chin and Means, 2000). These effectors include Ca2+/CaM-dependent kinases and phosphatases like CaMKII and CaN. CaMKII and CaN critically regulate synaptic functions (Mulkey et al., 1994; Pang et al., 2010) and contribute to synaptopathy in neurodegenerative diseases (Kuchibhotla et al., 2008; Gu et al., 2009; Teravskis et al., 2018). CaM also affects pathogenic processes such as Ca2+ dysregulation and pathogenic protein fibrillation by directly binding the plasma membrane Ca2+ APTase (PCMA) and amyloid-β peptides (Berrocal et al., 2012; Corbacho et al., 2017). Likewise, CaM binding by GAP-43 and BASP1 may directly and indirectly affect processes involved in neurodegenerative disorders.
Functions of GAP-43 and BASP1 and Their Link to Neurodegenerative Diseases
Growth-associated protein-43 and BASP1 regulate the actin cytoskeleton, which in turn modulates axon outgrowth (Gomez and Letourneau, 2014; Blanquie and Bradke, 2018) and synaptic functions (Eitzen, 2003; Smythe and Ayscough, 2006). Actin dynamics are altered in neurodegenerative disorders, and this change culminates in structural defects and synaptic dysfunctions. Disruption of dendritic actin filaments were observed in Drosophila models of polyglutamine diseases (Lee et al., 2011). This disruption was associated with decreased dendritic complexity – an early deficit that may contribute to the pathogenesis (Lee et al., 2011). Similarly, decreased levels of synaptosomal actin filaments were detected in mouse models and patient brains of Alzheimer’s Disease (Kommaddi et al., 2018). This reduction inversely correlated with dendritic spine density and behavioral performance (Kommaddi et al., 2018). Moreover, in Drosophila, pathogenic tau mutant and amyloid-β promoted abnormal accumulation and bundling of actin filaments, which correlated with neurotoxicity (Fulga et al., 2007).
In addition, actin binding proteins contribute to the pathogenesis of neurodegenerative disorders. For instance, the actin depolymerizing factor cofilin was found to be hyperactivated in Alzheimer’s Disease (Bamburg et al., 2010). The accumulation of hyperactivated cofilin formed cofilin-actin rods that led to impaired synaptic functions and synapse loss (Bamburg et al., 2010; Cichon et al., 2012; Munsie and Truant, 2012). Conversely, the level of the actin stabilizing protein drebrin was dramatically reduced in Alzheimer’s Disease (Harigaya et al., 1996; Hatanpää et al., 1999). A reduction in drebrin levels impaired neuritogenesis (Geraldo et al., 2008), resulted in synaptic dysfunctions (Kojima and Shirao, 2007), and correlated with cognitive impairment (Counts et al., 2006). These findings warrant examination of the actin regulators GAP-43 and BASP1 in neurodegenerative diseases.
Regulation of GAP-43 and BASP1 in Neurodegenerative Diseases
Growth-associated protein-43 and BASP1 were examined in many neurodegenerative diseases – Alzheimer’s Disease (Masliah et al., 1991; De La Monte et al., 1995; Bogdanovic et al., 2000; Rekart et al., 2004; Musunuri et al., 2014; Tagawa et al., 2015), Parkinson’s Disease (Caraveo et al., 2017; Saal et al., 2017; Wang et al., 2019b), Huntington’s Disease (Apostol et al., 2006; Dong and Cong, 2018), Amyotrophic Lateral Sclerosis (Parhad et al., 1992; Ikemoto et al., 1999; Andrés-Benito et al., 2017), and Spinal Muscular Atrophy (Fallini et al., 2016) – through studies of human patients and cellular and animal models. Most of these studies present correlational changes in GAP-43 and BASP1 but do not address their functional implications. In the following sections, GAP-43 and BASP1 will be discussed in the context of Alzheimer’s and Parkinson’s Disease, two neurodegenerative diseases in which they have been studied the most.
Alzheimer’s Disease
Alzheimer’s Disease (AD) is the most common neurodegenerative disorder causing dementia (Barker et al., 2002). AD is neuropathologically characterized by extracellular amyloid beta (Aβ) deposits termed amyloid plaques (Masters et al., 1985) and intracellular neurofibrillary tangles composed of the microtubule-binding protein tau (Goedert et al., 1988). While AD involves a widespread loss of neurons, it primarily affects cholinergic neurons in the basal forebrain (Whitehouse et al., 1982), noradrenergic neurons in the locus coeruleus (Bondareff et al., 1982), and pyramidal neurons in the entorhinal cortex, subiculum, and hippocampal CA1 (Hyman et al., 1984; Morrison and Hof, 2002). These vulnerable neurons have long and thin axons with sparse myelination (Braak and Del Trecidi, 2015). Such axonal properties increase the energetic demand and exposure to pathogenic species that contribute to the vulnerability in AD (Braak and Del Trecidi, 2015). Immunohistochemical studies found that GAP-43 levels were decreased in the neocortex but was preserved or even elevated in the hippocampus of AD patients (Masliah et al., 1991; De La Monte et al., 1995; Bogdanovic et al., 2000; Rekart et al., 2004). The reduction in cortical GAP-43 immunoreactivity likely reflects a profound neuron loss. The preservation or increase in hippocampal GAP-43 immunoreactivity raises the possibility that surviving neurons, by upregulating GAP-43, initiate axon outgrowth to compensate for the lost connections. Hippocampal GAP-43 immunoreactivity was observed in dystrophic neurites associated with plaques and correlated with aberrant sprouting (Masliah et al., 1991; Bogdanovic et al., 2000), which is characteristic of synaptic pathology in AD (Masliah, 1995). Based on this observation, GAP-43-associated axon outgrowth appears to be unsuccessful in establishing functional connections and seems to be contributing to the synaptic pathology instead. Examination of entorhinal fibers in amyloid precursor protein transgenic mice provides evidence that this aberrant sprouting is driven by amyloid deposition (Phinney et al., 1999). This finding highlights the importance of extrinsic factors on the successful regenerative response and emphasizes the need for combined therapy that overcomes both extrinsic and intrinsic barriers to axon remodeling. In agreement with the immunohistochemical data, quantitative mass spectrometry detected a reduction of GAP-43 in the temporal neocortex of AD patients (Musunuri et al., 2014). The same study also measured decreased levels of BASP1 in AD patients (Musunuri et al., 2014). In addition to their changes in expression, the extent of their phosphorylation was altered in AD. The overall phosphorylation of both proteins decreased in the temporal lobe of AD patients compared to non-AD individuals (Tagawa et al., 2015). For a detailed analysis of the phosphoproteome over the course of disease progression, four mouse models of AD at early, middle, and late time points were examined with respect to control mice. This analysis identified 5 phosphosites in GAP-43 and 6 phosphosites in BASP1 (Figure 2 shows equivalent sites in rat proteins based on sequence alignment in Clustal Omega; Tagawa et al., 2015). Phosphorylation at these sites generally increased in the middle stage of pathology and progressively decreased (Tagawa et al., 2015). Although the physiological relevance of these changes remains to be examined, this study prompts us to explore the possibility of modulating GAP-43 and BASP1 phosphorylation for therapeutic interventions in AD.
Parkinson’s Disease
Parkinson’s Disease (PD) is the most common neurodegenerative movement disorder (Tysnes and Storstein, 2017). PD is neuropathologically characterized by the accumulation of α-synuclein inclusions termed Lewy bodies (Spillantini et al., 1997). In PD, dopaminergic neurons in the substantia nigra pars compacta (SNc) primarily degenerate, causing motor symptoms such as bradykinesia, rigidity, and tremor (Tysnes and Storstein, 2017). The axons of the SNc dopaminergic neurons are long, thin, and poorly myelinated (Braak et al., 2004). Additionally, they branch extensively in the striatum and form extraordinarily large numbers of synapses (Bolam and Pissadaki, 2012) with transmitter release sites numbering up to 300,000 (Matsuda et al., 2009). These axonal properties contribute to the selective vulnerability in PD (Braak et al., 2004). A transcriptome-based meta-analysis of multiple studies found that GAP-43 and BASP1 are downregulated in the brains of PD patients. This same study identified BASP1 as an important regulator of other differentially expressed genes associated with synaptic signaling. Immunoreactivity of tyrosine hydroxylase (TH), a marker for dopaminergic neurons, was reduced in the SNc and striatum of PD patients in reflection of a substantial neuron loss (Saal et al., 2017). However, in the remaining TH+ SNc dopaminergic neurons, GAP-43 protein and mRNA levels were likewise decreased. In agreement with these data, reduced GAP-43 expression has also been detected in the cerebral spinal fluid of PD patients (Sjogren et al., 2000). Further evidence implicates the involvement of GAP-43 and BASP1 in PD. In an in vitro scratch lesion model using α-syn mutations causing autosomal-dominant forms of PD, the study found reduced neurite regeneration and subsequent loss of dopaminergic neurons accompanied by a reduction of striatal expression of GAP-43 (Tonges et al., 2014). In one PD patient, infusion with glial cell-derived neurotrophic factor at the putamen provided benefits even after cessation of treatment (Patel et al., 2013). While these reports suggest that increasing GAP-43 expression and therefore the axonal tree would be beneficial, other groups suggest that reduction of the axonal tree, in fact, confers protection in models of PD (Pacelli et al., 2015). The apparent discrepancy between these studies can be addressed if taken into consideration that the effect in neuronal sprouting needs to be regulated. In support of this idea, one study found that modulation of the activity of GAP-43 and BASP1 through CaN can alter the degeneration of axonal trees and confer neuroprotection in a rat model of PD (Caraveo et al., 2017). This model displayed presynaptic and behavioral impairments along with hypophosphorylation at 3 CaN-sensitive sites each in GAP-43 and BASP1 (Figure 2; Caraveo et al., 2017). Treatment with low doses of Tacrolimus, which partially inhibits CaN, ameliorated the presynaptic and behavioral deficits in addition to rescuing phosphorylation at these sites (Caraveo et al., 2017). This correlation suggests a potential involvement of GAP-43 and BASP1 phosphorylation in PD pathogenesis. Interestingly, Tacrolimus is an FDA-approved drug currently in widespread clinical use at high doses to suppress the rejection of organs in transplant patients, a process in which CaN also plays a critical role (Tron et al., 2019). This opens the possibility that Tacrolimus could be repurposed as a potential therapy for the treatment of PD.
Concluding Remarks
Growth-associated protein-43 and BASP1 are essential for developing axons to grow toward their correct targets and form synaptic connections during neuronal development and after neural injury. These axonal functions highly depend on their expression levels and phosphorylation status, which when modulated appropriately, can stimulate mature neurons to re- enter a growth state. This transition, which can protect and enable surviving neurons to re-establish functional connections, has been explored as a therapeutic avenue for neurodegenerative diseases. Specifically, the effects of delivering agents that upregulate GAP-43, such as BDNF, have been tested in animal models of PD (Gupta et al., 2009). BDNF treatment, although unable to revert neurodegeneration, demonstrated protective effects on remaining neurons and ameliorated behavioral impairments (Palasz et al., 2020). Several investigations into understanding the mechanism of potential therapies have identified GAP-43 as either upregulated or essential for its ameliorative effects. Levetiracetam for treatment of retinopathy (Mohammad et al., 2019) as well as for the repair of convulsant- induced cognitive impairment (Wang et al., 2019a) directly signals through the PKC/GAP-43 signaling pathway. Similarly, TGN-020 for treatment of spinal cord injury (Li et al., 2019b), and senegenin for the potential treatment for Aβ-induced neurotoxicity (Jesky and Chen, 2016), involve upregulation of GAP-43 protein levels for both neuroprotection and in vitro regeneration. Low GAP-43 levels in cerebrospinal fluid were associated with a poorer response to treatment of primary progressive multiple sclerosis using fingolimod or alemtuzumab (Sandelius et al., 2019) suggesting that GAP-43 is important in mediating its therapeutic effects. In rat models of PD, treatments with Pilose antler extracts led to an increase in striatal GAP-43 protein expression and less dopaminergic SNc neuronal cell death (Li et al., 2019a). Despite the positive effects of neurotrophic factors in animal models, their short half-life, low bioavailability, and limited permeability through the blood-brain barrier (BBB) imposed challenges in their application to patients (Palasz et al., 2020). Such challenges associated with using neurotrophic factors to increase GAP-43 and BASP1 levels can be avoided by using other pharmacological agents that can cross the BBB and modulate their activities, for instance, via phosphorylation. A potential candidate is the FDA-approved CaN inhibitor Tacrolimus. In a rat model of PD, tacrolimus was shown to cross the BBB, alter phosphorylation of GAP-43 and BASP1, and confer neuroprotection at doses 10-fold lower than the standard immunosuppressive dose (Caraveo et al., 2017). At sub-immunosuppressive doses, the risk of secondary effects, such as opportunistic infections, posterior reversible leukoencephalopathy, and seizures typically achieved at clinical doses would be avoided. Moreover, these lower Tacrolimus doses would finely tune GAP-43 and BASP1 to drive sufficient, but not hyperactive, axonal sprouting in a spatially and temporally confined manner. This regulation is necessary to promote regeneration specifically in the affected neuronal populations at the right time window while preventing potential complications arising from hyperconnectivity. Additionally, external inhibitory factors in the central nervous system (Fournier and Strittmatter, 2001) and other disease-associated factors (Phinney et al., 1999) need to be prevented from blocking axon growth or driving the formation of aberrant connections. Moreover, determining the stages of pathogenesis at which regeneration of remaining axons can be protective will be important. Further exploration of these areas will facilitate the development of GAP-43- and BASP1-targeting therapies for neurodegenerative diseases.
Author Contributions
DC contributed by writing and revising the entire review, as well as generating figures. AS contributed to writing the section “Involvement in Neural Injury Response” and building Figure 1. GC conceived the review, contributed to the concluding remarks, and edited the review. All authors contributed to the article and approved the submitted version.
Funding
This review manuscript was supported by the Parkinson’s Foundation grant PF-JFA-1949 and the National Institute of Mental Health grant 2T32MH067564.
Conflict of Interest
The authors declare that the research was conducted in the absence of any commercial or financial relationships that could be construed as a potential conflict of interest.
Acknowledgments
We would like to thank the members of the laboratory for constructive feedback.
References
Acarin, L., González, B., and Castellano, B. (2000). Neuronal, astroglial and microglial cytokine expression after an excitotoxic lesion in the immature rat brain. Eur. J. Neurosci. 12, 3505–3520. doi: 10.1046/j.1460-9568.2000.00226.x
Aigner, L., Arber, S., Kapfhammer, J. P., Laux, T., Schneider, C., Botteri, F., et al. (1995). Overexpression of the neural growth-associated protein GAP-43 induces nerve sprouting in the adult nervous system of transgenic mice. Cell 83, 269–278. doi: 10.1016/0092-8674(95)90168-x
Aigner, L., and Caroni, P. (1995). Absence of persistent spreading, branching, and adhesion in GAP-43-depleted growth cones. J. Cell Biol. 128, 647–660. doi: 10.1083/jcb.128.4.647
Alexander, K. A., Cimler, B. M., Meier, K. E., and Storm, D. R. (1987). Regulation of calmodulin binding to P-57. A neurospecific calmodulin binding protein. J. Biol. Chem. 262, 6108–6113.
Alfonso, S. I., Callender, J. A., Hooli, B., Antal, C. E., Mullin, K., Sherman, M. A., et al. (2016). Gain-of-function mutations in protein kinase Cα (PKCα) may promote synaptic defects in Alzheimer’s disease. Sci. Signal. 9:ra47. doi: 10.1126/scisignal.aaf6209
Allegra Mascaro, A. L., Cesare, P., Sacconi, L., Grasselli, G., Mandolesi, G., Maco, B., et al. (2013). In vivo single branch axotomy induces GAP-43-dependent sprouting and synaptic remodeling in cerebellar cortex. Proc. Natl. Acad. Sci. U.S.A. 110, 10824–10829. doi: 10.1073/pnas.1219256110
Amadio, M., Pascale, A., Wang, J., Ho, L., Quattrone, A., Gandy, S., et al. (2009). nELAV proteins alteration in Alzheimer’s disease brain: a novel putative target for amyloid-beta reverberating on AbetaPP processing. J. Alzheimers Dis. 16, 409–419. doi: 10.3233/jad-2009-0967
Anderson, K. D., Merhege, M. A., Morin, M., Bolognani, F., and Perrone-Bizzozero, N. I. (2003). Increased expression and localization of the RNA-binding protein HuD and GAP-43 mRNA to cytoplasmic granules in DRG neurons during nerve regeneration. Exp. Neurol. 183, 100–108. doi: 10.1016/s0014-4886(03)00103-1
Anderson, K. D., Sengupta, J., Morin, M., Neve, R. L., Valenzuela, C. F., and Perrone-Bizzozero, N. I. (2001). Overexpression of HuD accelerates neurite outgrowth and increases GAP-43 mRNA expression in cortical neurons and retinoic acid-induced embryonic stem cells in vitro. Exp. Neurol. 168, 250–258. doi: 10.1006/exnr.2000.7599
Andreasen, T. J., Luetje, C. W., Heideman, W., and Storm, D. R. (1983). Purification of a novel calmodulin binding protein from bovine cerebral cortex membranes. Biochemistry 22, 4615–4618. doi: 10.1021/bi00289a001
Andrés-Benito, P., Moreno, J., Aso, E., Povedano, M., and Ferrer, I. (2017). Amyotrophic lateral sclerosis, gene deregulation in the anterior horn of the spinal cord and frontal cortex area 8: implications in frontotemporal lobar degeneration. Aging 9, 823–851. doi: 10.18632/aging.101195
Apel, E. D., Byford, M. F., Au, D., Walsh, K. A., and Storm, D. R. (1990). Identification of the protein kinase C phosphorylation site in neuromodulin. Biochemistry 29, 2330–2335. doi: 10.1021/bi00461a017
Apel, E. D., Litchfield, D. W., Clark, R. H., Krebs, E. G., and Storm, D. R. (1991). Phosphorylation of neuromodulin (GAP-43) by casein kinase II. Identification of phosphorylation sites and regulation by calmodulin. J. Biol. Chem. 266, 10544–10551.
Apostol, B. L., Illes, K., Pallos, J., Bodai, L., Wu, J., Strand, A., et al. (2006). Mutant huntingtin alters MAPK signaling pathways in PC12 and striatal cells: ERK1/2 protects against mutant huntingtin-associated toxicity. Hum. Mol. Genet. 15, 273–285. doi: 10.1093/hmg/ddi443
Aumiller, W. M., and Keating, C. D. (2016). Phosphorylation-mediated RNA/peptide complex coacervation as a model for intracellular liquid organelles. Nat. Chem. 8, 129–137. doi: 10.1038/nchem.2414
Bähler, M., and Rhoads, A. (2002). Calmodulin signaling via the IQ motif. FEBS Lett. 513, 107–113. doi: 10.1016/s0014-5793(01)03239-2
Bamburg, J. R., Bernstein, B. W., Davis, R. C., Flynn, K. C., Goldsbury, C., Jensen, J. R., et al. (2010). ADF/Cofilin-actin rods in neurodegenerative diseases. Curr. Alzheimer Res. 7, 241–250. doi: 10.2174/156720510791050902
Barker, W. W., Luis, C. A., Kashuba, A., Luis, M., Harwood, D. G., Loewenstein, D., et al. (2002). Relative frequencies of Alzheimer disease, Lewy body, vascular and frontotemporal dementia, and hippocampal sclerosis in the State of Florida Brain Bank. Alzheimer Dis. Assoc. Disord. 16, 203–212. doi: 10.1097/00002093-200210000-00001
Barnes, J. A., and Gomes, A. V. (1995). PEST sequences in calmodulin-binding proteins. Mol. Cell. Biochem. 149-150, 17–27. doi: 10.1007/978-1-4615-2015-3_2
Benowitz, L. I., Apostolides, P. J., Perrone-Bizzozero, N., Finklestein, S. P., and Zwiers, H. (1988). Anatomical distribution of the growth-associated protein GAP-43/B-50 in the adult rat brain. J. Neurosci. 8, 339–352. doi: 10.1523/jneurosci.08-01-00339.1988
Berrocal, M., Sepulveda, M. R., Vazquez-Hernandez, M., and Mata, A. M. (2012). Calmodulin antagonizes amyloid-β peptides-mediated inhibition of brain plasma membrane Ca(2+)-ATPase. Biochim. Biophys. Acta 1822, 961–969. doi: 10.1016/j.bbadis.2012.02.013
Bezprozvanny, I. (2009). Calcium signaling and neurodegenerative diseases. Trends Mol. Med. 15, 89–100. doi: 10.1016/j.molmed.2009.01.001
Bird, C. W., Gardiner, A. S., Bolognani, F., Tanner, D. C., Chen, C.-Y., Lin, W.-J., et al. (2013). KSRP modulation of GAP-43 mRNA stability restricts axonal outgrowth in embryonic hippocampal neurons. PLoS One 8:e79255. doi: 10.1371/journal.pone.0079255
Blanquie, O., and Bradke, F. (2018). Cytoskeleton dynamics in axon regeneration. Curr. Opin. Neurobiol. 51, 60–69. doi: 10.1016/j.conb.2018.02.024
Bogdanovic, N., Davidsson, P., Volkmann, I., Winblad, B., and Blennow, K. (2000). Growth-associated protein GAP-43 in the frontal cortex and in the hippocampus in Alzheimer’s disease: an immunohistochemical and quantitative study. J. Neural Transm. 107, 463–478. doi: 10.1007/s007020070088
Bolam, J. P., and Pissadaki, E. K. (2012). Living on the edge with too many mouths to feed: why dopamine neurons die. Mov. Disord. 27, 1478–1483. doi: 10.1002/mds.25135
Bolognani, F., Tanner, D. C., Merhege, M., Deschenes-Furry, J., Jasmin, B., and Perrone-Bizzozero, N. I. (2006). In vivo post-transcriptional regulation of GAP-43 mRNA by overexpression of the RNA-binding protein HuD. J. Neurochem. 96, 790–801. doi: 10.1111/j.1471-4159.2005.03607.x
Bomze, H. M., Bulsara, K. R., Iskandar, B. J., Caroni, P., and Skene, J. H. (2001). Spinal axon regeneration evoked by replacing two growth cone proteins in adult neurons. Nat. Neurosci. 4, 38–43. doi: 10.1038/82881
Bondareff, W., Mountjoy, C. Q., and Roth, M. (1982). Loss of neurons of origin of the adrenergic projection to cerebral cortex (nucleus locus ceruleus) in senile dementia. Neurology 32, 164–168. doi: 10.1212/wnl.32.2.164
Braak, H., and Del Trecidi, K. (2015). Neuroanatomy and pathology of sporadic Alzheimer’s disease. Adv. Anat. Embryol. Cell Biol. 215, 1–162. doi: 10.1007/978-3-540-79850-7_1
Braak, H., Ghebremedhin, E., Rüb, U., Bratzke, H., and Del Tredici, K. (2004). Stages in the development of Parkinson’s disease-related pathology. Cell Tissue Res. 318, 121–134. doi: 10.1007/s00441-004-0956-9
Bronicki, L. M., and Jasmin, B. J. (2013). Emerging complexity of the HuD/ELAVl4 gene; implications for neuronal development, function, and dysfunction. RNA 19, 1019–1037. doi: 10.1261/rna.039164.113
Buffo, A., Holtmaat, A. J., Savio, T., Verbeek, J. S., Oberdick, J., Oestreicher, A. B., et al. (1997). Targeted overexpression of the neurite growth-associated protein B-50/GAP-43 in cerebellar Purkinje cells induces sprouting after axotomy but not axon regeneration into growth-permissive transplants. J. Neurosci. 17, 8778–8791. doi: 10.1523/jneurosci.17-22-08778.1997
Bullock, R., Zauner, A., Woodward, J. J., Myseros, J., Choi, S. C., Ward, J. D., et al. (1998). Factors affecting excitatory amino acid release following severe human head injury. J. Neurosurg. 89, 507–518. doi: 10.3171/jns.1998.89.4.0507
Calabrese, B., and Halpain, S. (2005). Essential role for the PKC target MARCKS in maintaining dendritic spine morphology. Neuron 48, 77–90. doi: 10.1016/j.neuron.2005.08.027
Caraveo, G., Soste, M., Cappelleti, V., Fanning, S., Van Rossum, D. B., Whitesell, L., et al. (2017). FKBP12 contributes to α-synuclein toxicity by regulating the calcineurin-dependent phosphoproteome. Proc. Natl. Acad. Sci. U.S.A. 114, E11313–E11322.
Carmichael, S. T., Archibeque, I., Luke, L., Nolan, T., Momiy, J., and Li, S. (2005). Growth-associated gene expression after stroke: evidence for a growth-promoting region in peri-infarct cortex. Exp. Neurol. 193, 291–311. doi: 10.1016/j.expneurol.2005.01.004
Caroni, P. (2001). New EMBO member’s review: actin cytoskeleton regulation through modulation of PI(4,5)P(2) rafts. EMBO J. 20, 4332–4336. doi: 10.1093/emboj/20.16.4332
Caroni, P., Aigner, L., and Schneider, C. (1997). Intrinsic neuronal determinants locally regulate extrasynaptic and synaptic growth at the adult neuromuscular junction. J. Cell Biol. 136, 679–692. doi: 10.1083/jcb.136.3.679
Chen, H., Lin, W., Zhang, Y., Lin, L., Chen, J., Zeng, Y., et al. (2016). IL-10 promotes neurite outgrowth and synapse formation in cultured cortical neurons after the oxygen-glucose deprivation via JAK1/STAT3 pathway. Sci. Rep. 6, 30459–30416.
Cheng, M. Y., Wang, E. H., Woodson, W. J., Wang, S., Sun, G., Lee, A. G., et al. (2014). Optogenetic neuronal stimulation promotes functional recovery after stroke. Proc. Natl. Acad. Sci. U.S.A. 111, 12913–12918. doi: 10.1073/pnas.1404109111
Chiaramello, A., Neuman, T., Peavy, D. R., and Zuber, M. X. (1996). The GAP-43 gene is a direct downstream target of the basic helix-loop-helix transcription factors. J. Biol. Chem. 271, 22035–22043. doi: 10.1074/jbc.271.36.22035
Chiaretti, A., Barone, G., Riccardi, R., Antonelli, A., Pezzotti, P., Genovese, O., et al. (2009). NGF, DCX, and NSE upregulation correlates with severity and outcome of head trauma in children. Neurology 72, 609–616. doi: 10.1212/01.wnl.0000342462.51073.06
Chin, D., and Means, A. R. (2000). Calmodulin: a prototypical calcium sensor. Trends Cell Biol. 10, 322–328. doi: 10.1016/s0962-8924(00)01800-6
Chiti, F., and Dobson, C. M. (2006). Protein misfolding, functional amyloid, and human disease. Annu. Rev. Biochem. 75, 333–366. doi: 10.1146/annurev.biochem.75.101304.123901
Chung, S., Eckrich, M., Perrone-Bizzozero, N., Kohn, D. T., and Furneaux, H. (1997). The Elav-like proteins bind to a conserved regulatory element in the 3′-untranslated region of GAP-43 mRNA. J. Biol. Chem. 272, 6593–6598. doi: 10.1074/jbc.272.10.6593
Cichon, J., Sun, C., Chen, B., Jiang, M., Chen, X. A., Sun, Y., et al. (2012). Cofilin aggregation blocks intracellular trafficking and induces synaptic loss in hippocampal neurons. J. Biol. Chem. 287, 3919–3929. doi: 10.1074/jbc.m111.301911
Cimler, B. M., Andreasen, T. J., Andreasen, K. I., and Storm, D. R. (1985). P-57 is a neural specific calmodulin-binding protein. J. Biol. Chem. 260, 10784–10788.
Corbacho, I., Berrocal, M., Török, K., Mata, A. M., and Gutierrez-Merino, C. (2017). High affinity binding of amyloid β-peptide to calmodulin: structural and functional implications. Biochem. Biophys. Res. Commun. 486, 992–997. doi: 10.1016/j.bbrc.2017.03.151
Costigan, M., Befort, K., Karchewski, L., Griffin, R. S., D’urso, D., Allchorne, A., et al. (2002). Replicate high-density rat genome oligonucleotide microarrays reveal hundreds of regulated genes in the dorsal root ganglion after peripheral nerve injury. BMC Neurosci. 3:16. doi: 10.1186/1471-2202-3-16
Counts, S. E., Nadeem, M., Lad, S. P., Wuu, J., and Mufson, E. J. (2006). Differential expression of synaptic proteins in the frontal and temporal cortex of elderly subjects with mild cognitive impairment. J. Neuropathol. Exp. Neurol. 65, 592–601. doi: 10.1097/00005072-200606000-00007
Csuka, E., Morganti-Kossmann, M. C., Lenzlinger, P. M., Joller, H., Trentz, O., and Kossmann, T. (1999). IL-10 levels in cerebrospinal fluid and serum of patients with severe traumatic brain injury: relationship to IL-6, TNF-alpha, TGF-beta1 and blood-brain barrier function. J. Neuroimmunol. 101, 211–221. doi: 10.1016/s0165-5728(99)00148-4
de Groen, P. C., Eggen, B. J., Gispen, W. H., Schotman, P., and Schrama, L. H. (1995). Cloning and promoter analysis of the human B-50/GAP-43 gene. J. Mol. Neurosci. 6, 109–119. doi: 10.1007/bf02736770
De La Monte, S. M., Federoff, H. J., Ng, S. C., Grabczyk, E., and Fishman, M. C. (1989). GAP-43 gene expression during development: persistence in a distinctive set of neurons in the mature central nervous system. Brain Res. Dev. Brain Res. 46, 161–168. doi: 10.1016/0165-3806(89)90279-4
De La Monte, S. M., Ng, S. C., and Hsu, D. W. (1995). Aberrant GAP-43 gene expression in Alzheimer’s disease. Am. J. Pathol. 147, 934–946.
De Santis, R., Alfano, V., De Turris, V., Colantoni, A., Santini, L., Garone, M. G., et al. (2019). Mutant FUS and ELAVL4 (HuD) aberrant crosstalk in amyotrophic lateral sclerosis. Cell Rep. 27:3818. doi: 10.1016/j.celrep.2019.05.085
Dekker, L. V., De Graan, P. N., Oestreicher, A. B., Versteeg, D. H., and Gispen, W. H. (1989a). Inhibition of noradrenaline release by antibodies to B-50 (GAP-43). Nature 342, 74–76. doi: 10.1038/342074a0
Dekker, L. V., De Graan, P. N., Versteeg, D. H., Oestreicher, A. B., and Gispen, W. H. (1989b). Phosphorylation of B-50 (GAP43) is correlated with neurotransmitter release in rat hippocampal slices. J. Neurochem. 52, 24–30. doi: 10.1111/j.1471-4159.1989.tb10893.x
DeKosky, S. T., Goss, J. R., Miller, P. D., Styren, S. D., Kochanek, P. M., and Marion, D. (1994). Upregulation of nerve growth factor following cortical trauma. Exp. Neurol. 130, 173–177. doi: 10.1006/exnr.1994.1196
Dennis, D. J., Han, S., and Schuurmans, C. (2019). bHLH transcription factors in neural development, disease, and reprogramming. Brain Res. 1705, 48–65. doi: 10.1016/j.brainres.2018.03.013
Dent, E. W., and Meiri, K. F. (1998). Distribution of phosphorylated GAP-43 (neuromodulin) in growth cones directly reflects growth cone behavior. J. Neurobiol. 35, 287–299. doi: 10.1002/(sici)1097-4695(19980605)35:3<287::aid-neu6>3.0.co;2-v
DeStefano, A. L., Latourelle, J., Lew, M. F., Suchowersky, O., Klein, C., Golbe, L. I., et al. (2008). Replication of association between ELAVL4 and Parkinson disease: the GenePD study. Hum. Genet. 124, 95–99. doi: 10.1007/s00439-008-0526-4
Ditlevsen, D. K., Povlsen, G. K., Berezin, V., and Bock, E. (2008). NCAM-induced intracellular signaling revisited. J. Neurosci. Res. 86, 727–743. doi: 10.1002/jnr.21551
Dong, X., and Cong, S. (2018). Identification of differentially expressed genes and regulatory relationships in Huntington’s disease by bioinformatics analysis. Mol. Med. Rep. 17, 4317–4326.
Edmonds, B. T., Moomaw, C. R., Hsu, J. T., Slaughter, C., and Ellis, L. (1990). The p38 and p34 polypeptides of growth cone particle membranes are the alpha- and beta-subunits of G proteins. Brain Res. Dev. Brain Res. 56, 131–136. doi: 10.1016/0165-3806(90)90172-u
Eggen, B. J., Nielander, H. B., Rensen-De Leeuw, M. G., Schotman, P., Gispen, W. H., and Schrama, L. H. (1994). Identification of two promoter regions in the rat B-50/GAP-43 gene. Brain Res. Mol. Brain Res. 23, 221–234. doi: 10.1016/0169-328x(94)90229-1
Eitzen, G. (2003). Actin remodeling to facilitate membrane fusion. Biochim. Biophys. Acta 1641, 175–181. doi: 10.1016/s0167-4889(03)00087-9
Elbaum-Garfinkle, S. (2019). Matter over mind: liquid phase separation and neurodegeneration. J. Biol. Chem. 294, 7160–7168. doi: 10.1074/jbc.rev118.001188
Etcheberrigaray, R., Tan, M., Dewachter, I., Kuipéri, C., Van Der Auwera, I., Wera, S., et al. (2004). Therapeutic effects of PKC activators in Alzheimer’s disease transgenic mice. Proc. Natl. Acad. Sci. U.S.A. 101, 11141–11146. doi: 10.1073/pnas.0403921101
Fallini, C., Donlin-Asp, P. G., Rouanet, J. P., Bassell, G. J., and Rossoll, W. (2016). Deficiency of the survival of motor neuron protein impairs mRNA localization and local translation in the growth cone of motor neurons. J. Neurosci. 36, 3811–3820. doi: 10.1523/jneurosci.2396-15.2016
Filbin, M. T. (2003). Myelin-associated inhibitors of axonal regeneration in the adult mammalian CNS. Nat. Rev. Neurosci. 4, 703–713. doi: 10.1038/nrn1195
Forsova, O. S., and Zakharov, V. V. (2016). High-order oligomers of intrinsically disordered brain proteins BASP1 and GAP-43 preserve the structural disorder. FEBS J. 283, 1550–1569. doi: 10.1111/febs.13692
Fournier, A. E., and Strittmatter, S. M. (2001). Repulsive factors and axon regeneration in the CNS. Curr. Opin. Neurobiol. 11, 89–94. doi: 10.1016/s0959-4388(00)00178-1
Frey, D., Laux, T., Xu, L., Schneider, C., and Caroni, P. (2000). Shared and unique roles of CAP23 and GAP43 in actin regulation, neurite outgrowth, and anatomical plasticity. J. Cell Biol. 149, 1443–1454. doi: 10.1083/jcb.149.7.1443
Fulga, T. A., Elson-Schwab, I., Khurana, V., Steinhilb, M. L., Spires, T. L., Hyman, B. T., et al. (2007). Abnormal bundling and accumulation of F-actin mediates tau-induced neuronal degeneration in vivo. Nat. Cell Biol. 9, 139–148. doi: 10.1038/ncb1528
Gamby, C., Waage, M. C., Allen, R. G., and Baizer, L. (1996). Analysis of the role of calmodulin binding and sequestration in neuromodulin (GAP-43) function. J. Biol. Chem. 271, 26698–26705. doi: 10.1074/jbc.271.43.26698
Gauthier-Kemper, A., Igaev, M., Sündermann, F., Janning, D., Brühmann, J., Moschner, K., et al. (2014). Interplay between phosphorylation and palmitoylation mediates plasma membrane targeting and sorting of GAP43. Mol. Biol. Cell 25, 3284–3299. doi: 10.1091/mbc.e13-12-0737
Geist, L., Zawadzka-Kazimierczuk, A., Saxena, S., Żerko, S., Koźmiński, W., and Konrat, R. (2013). 1H,13C and 15N resonance assignments of human BASP1. Biomol. NMR Assign. 7, 315–319.
Geraldo, S., Khanzada, U. K., Parsons, M., Chilton, J. K., and Gordon-Weeks, P. R. (2008). Targeting of the F-actin-binding protein drebrin by the microtubule plus-tip protein EB3 is required for neuritogenesis. Nat. Cell Biol. 10, 1181–1189. doi: 10.1038/ncb1778
Goedert, M., Wischik, C. M., Crowther, R. A., Walker, J. E., and Klug, A. (1988). Cloning and sequencing of the cDNA encoding a core protein of the paired helical filament of Alzheimer disease: identification as the microtubule-associated protein tau. Proc. Natl. Acad. Sci. U.S.A. 85, 4051–4055. doi: 10.1073/pnas.85.11.4051
Gomez, T. M., and Letourneau, P. C. (2014). Actin dynamics in growth cone motility and navigation. J. Neurochem. 129, 221–234. doi: 10.1111/jnc.12506
Grasselli, G., Mandolesi, G., Strata, P., and Cesare, P. (2011). Impaired sprouting and axonal atrophy in cerebellar climbing fibres following in vivo silencing of the growth-associated protein GAP-43. PLoS One 6:e20791. doi: 10.1371/journal.pone.0020791
Grasselli, G., and Strata, P. (2013). Structural plasticity of climbing fibers and the growth-associated protein GAP-43. Front. Neural Circuits 7:25. doi: 10.3389/fncir.2013.00025
Gu, Z., Liu, W., and Yan, Z. (2009). {beta}-Amyloid impairs AMPA receptor trafficking and function by reducing Ca2+/calmodulin-dependent protein kinase II synaptic distribution. J. Biol. Chem. 284, 10639–10649. doi: 10.1074/jbc.m806508200
Gudasheva, T. A., Povarnina, P. Y., Volkova, A. A., Kruglov, S. V., Antipova, T. A., and Seredenin, S. B. (2019). A nerve growth factor dipeptide mimetic stimulates neurogenesis and synaptogenesis in the hippocampus and striatum of adult rats with focal cerebral ischemia. Acta Nat. 11, 31–37. doi: 10.32607/20758251-2019-11-3-31-37
Gupta, S. K., Mishra, R., Kusum, S., Spedding, M., Meiri, K. F., Gressens, P., et al. (2009). GAP-43 is essential for the neurotrophic effects of BDNF and positive AMPA receptor modulator S18986. Cell Death Differ. 16, 624–637. doi: 10.1038/cdd.2008.188
Gwag, B. J., Sessler, F. M., Robine, V., and Springer, J. E. (1997). Endogenous glutamate levels regulate nerve growth factor mRNA expression in the rat dentate gyrus. Mol. Cells 7, 425–430.
Han, M.-H., Jiao, S., Jia, J.-M., Chen, Y., Chen, C. Y., Gucek, M., et al. (2013). The novel caspase-3 substrate Gap43 is involved in AMPA receptor endocytosis and long-term depression. Mol. Cell. Proteomics 12, 3719–3731. doi: 10.1074/mcp.m113.030676
Harigaya, Y., Shoji, M., Shirao, T., and Hirai, S. (1996). Disappearance of actin-binding protein, drebrin, from hippocampal synapses in Alzheimer’s disease. J. Neurosci. Res. 43, 87–92. doi: 10.1002/jnr.490430111
Hartl, M., Nist, A., Khan, M. I., Valovka, T., and Bister, K. (2009). Inhibition of Myc-induced cell transformation by brain acid-soluble protein 1 (BASP1). Proc. Natl. Acad. Sci. U.S.A. 106, 5604–5609. doi: 10.1073/pnas.0812101106
Haruta, T., Takami, N., Ohmura, M., Misumi, Y., and Ikehara, Y. (1997). Ca2+-dependent interaction of the growth-associated protein GAP-43 with the synaptic core complex. Biochem. J. 325(Pt 2), 455–463. doi: 10.1042/bj3250455
Hatanpää, K., Isaacs, K. R., Shirao, T., Brady, D. R., and Rapoport, S. I. (1999). Loss of proteins regulating synaptic plasticity in normal aging of the human brain and in Alzheimer disease. J. Neuropathol. Exp. Neurol. 58, 637–643. doi: 10.1097/00005072-199906000-00008
Haynes, C., Oldfield, C. J., Ji, F., Klitgord, N., Cusick, M. E., Radivojac, P., et al. (2006). Intrinsic disorder is a common feature of hub proteins from four eukaryotic interactomes. PLoS Comput. Biol. 2:e100. doi: 10.1371/journal.pcbi.0020100
He, Q., Dent, E. W., and Meiri, K. F. (1997). Modulation of actin filament behavior by GAP-43 (neuromodulin) is dependent on the phosphorylation status of serine 41, the protein kinase C site. J. Neurosci. 17, 3515–3524. doi: 10.1523/jneurosci.17-10-03515.1997
Heemskerk, F. M., Schrama, L. H., Gianotti, C., Spierenburg, H., Versteeg, D. H., De Graan, P. N., et al. (1990). 4-Aminopyridine stimulates B-50 (GAP43) phosphorylation and [3H]noradrenaline release in rat hippocampal slices. J. Neurochem. 54, 863–869. doi: 10.1111/j.1471-4159.1990.tb02331.x
Hens, J. J., Ghijsen, W. E., Weller, U., Spierenburg, H. A., Boomsma, F., Oestreicher, A. B., et al. (1998). Anti-B-50 (GAP-43) antibodies decrease exocytosis of glutamate in permeated synaptosomes. Eur. J. Pharmacol. 363, 229–240. doi: 10.1016/s0014-2999(98)00835-8
Hess, D. T., Patterson, S. I., Smith, D. S., and Skene, J. H. (1993). Neuronal growth cone collapse and inhibition of protein fatty acylation by nitric oxide. Nature 366, 562–565. doi: 10.1038/366562a0
Hulo, S., Alberi, S., Laux, T., Muller, D., and Caroni, P. (2002). A point mutant of GAP-43 induces enhanced short-term and long-term hippocampal plasticity. Eur. J. Neurosci. 15, 1976–1982. doi: 10.1046/j.1460-9568.2002.02026.x
Hyman, B. T., Van Hoesen, G. W., Damasio, A. R., and Barnes, C. L. (1984). Alzheimer’s disease: cell-specific pathology isolates the hippocampal formation. Science 225, 1168–1170. doi: 10.1126/science.6474172
Ikemoto, A., Hirano, A., and Akiguchi, I. (1999). Increased expression of growth-associated protein 43 on the surface of the anterior horn cells in amyotrophic lateral sclerosis. Acta Neuropathol. 98, 367–373. doi: 10.1007/s004010051096
Jacobson, R. D., Virág, I., and Skene, J. H. (1986). A protein associated with axon growth, GAP-43, is widely distributed and developmentally regulated in rat CNS. J. Neurosci. 6, 1843–1855. doi: 10.1523/jneurosci.06-06-01843.1986
Jesky, R., and Chen, H. (2016). The neuritogenic and neuroprotective potential of senegenin against Aβ-induced neurotoxicity in PC 12 cells. BMC Complement. Altern. Med. 16:26. doi: 10.1186/s12906-016-1006-3
Karns, L. R., Ng, S. C., Freeman, J. A., and Fishman, M. C. (1987). Cloning of complementary DNA for GAP-43, a neuronal growth-related protein. Science 236, 597–600. doi: 10.1126/science.2437653
Kawamata, T., Ren, J., Cha, J. H., and Finklestein, S. P. (1999). Intracisternal antisense oligonucleotide to growth associated protein-43 blocks the recovery-promoting effects of basic fibroblast growth factor after focal stroke. Exp. Neurol. 158, 89–96. doi: 10.1006/exnr.1999.7101
Kawasaki, A., Okada, M., Tamada, A., Okuda, S., Nozumi, M., Ito, Y., et al. (2018). Growth cone phosphoproteomics reveals that GAP-43 Phosphorylated by JNK is a marker of axon growth and regeneration. iScience 4, 190–203. doi: 10.1016/j.isci.2018.05.019
Kohn, D. T., Tsai, K. C., Cansino, V. V., Neve, R. L., and Perrone-Bizzozero, N. I. (1996). Role of highly conserved pyrimidine-rich sequences in the 3′ untranslated region of the GAP-43 mRNA in mRNA stability and RNA-protein interactions. Brain Res. Mol. Brain Res. 36, 240–250. doi: 10.1016/0169-328x(95)00239-o
Kojima, N., and Shirao, T. (2007). Synaptic dysfunction and disruption of postsynaptic drebrin-actin complex: a study of neurological disorders accompanied by cognitive deficits. Neurosci. Res. 58, 1–5. doi: 10.1016/j.neures.2007.02.003
Kommaddi, R. P., Das, D., Karunakaran, S., Nanguneri, S., Bapat, D., Ray, A., et al. (2018). Aβ mediates F-actin disassembly in dendritic spines leading to cognitive deficits in Alzheimer’s disease. J. Neurosci. 38, 1085–1099. doi: 10.1523/jneurosci.2127-17.2017
Korshunova, I., Caroni, P., Kolkova, K., Berezin, V., Bock, E., and Walmod, P. S. (2008). Characterization of BASP1-mediated neurite outgrowth. J. Neurosci. Res. 86, 2201–2213. doi: 10.1002/jnr.21678
Korshunova, I., Novitskaya, V., Kiryushko, D., Pedersen, N., Kolkova, K., Kropotova, E., et al. (2007). GAP-43 regulates NCAM-180-mediated neurite outgrowth. J. Neurochem. 100, 1599–1612.
Koseki, H., Donegá, M., Lam, B. Y., Petrova, V., Van Erp, S., Yeo, G. S., et al. (2017). Selective rab11 transport and the intrinsic regenerative ability of CNS axons. eLife 6:e26956.
Kossmann, T., Hans, V., Imhof, H. G., Trentz, O., and Morganti-Kossmann, M. C. (1996). Interleukin-6 released in human cerebrospinal fluid following traumatic brain injury may trigger nerve growth factor production in astrocytes. Brain Res. 713, 143–152. doi: 10.1016/0006-8993(95)01501-9
Kossmann, T., Hans, V. H., Imhof, H. G., Stocker, R., Grob, P., Trentz, O., et al. (1995). Intrathecal and serum interleukin-6 and the acute-phase response in patients with severe traumatic brain injuries. Shock 4, 311–317. doi: 10.1097/00024382-199511000-00001
Kuchibhotla, K. V., Goldman, S. T., Lattarulo, C. R., Wu, H.-Y., Hyman, B. T., and Bacskai, B. J. (2008). Abeta plaques lead to aberrant regulation of calcium homeostasis in vivo resulting in structural and functional disruption of neuronal networks. Neuron 59, 214–225. doi: 10.1016/j.neuron.2008.06.008
Laux, T., Fukami, K., Thelen, M., Golub, T., Frey, D., and Caroni, P. (2000). GAP43, MARCKS, and CAP23 modulate PI(4,5)P(2) at plasmalemmal rafts, and regulate cell cortex actin dynamics through a common mechanism. J. Cell Biol. 149, 1455–1472. doi: 10.1083/jcb.149.7.1455
Lee, S. B., Bagley, J. A., Lee, H. Y., Jan, L. Y., and Jan, Y.-N. (2011). Pathogenic polyglutamine proteins cause dendrite defects associated with specific actin cytoskeletal alterations in Drosophila. Proc. Natl. Acad. Sci. U.S.A. 108, 16795–16800. doi: 10.1073/pnas.1113573108
Leshchyns’ka, I., Sytnyk, V., Morrow, J. S., and Schachner, M. (2003). Neural cell adhesion molecule (NCAM) association with PKCbeta2 via betaI spectrin is implicated in NCAM-mediated neurite outgrowth. J. Cell Biol. 161, 625–639. doi: 10.1083/jcb.200303020
Li, C., Sun, Y., Yang, W., Ma, S., Zhang, L., Zhao, J., et al. (2019a). Pilose Antler Extracts (PAEs) protect against neurodegeneration in 6-OHDA-Induced Parkinson’s Disease Rat Models. Evid. Based. Complement. Altern. Med. 2019:7276407.
Li, J., Jia, Z., Xu, W., Guo, W., Zhang, M., Bi, J., et al. (2019b). TGN-020 alleviates edema and inhibits astrocyte activation and glial scar formation after spinal cord compression injury in rats. Life Sci. 222, 148–157. doi: 10.1016/j.lfs.2019.03.007
Li, P., Banjade, S., Cheng, H.-C., Kim, S., Chen, B., Guo, L., et al. (2012). Phase transitions in the assembly of multivalent signalling proteins. Nature 483, 336–340. doi: 10.1038/nature10879
Liang, X., Lu, Y., Neubert, T. A., and Resh, M. D. (2002). Mass spectrometric analysis of GAP-43/neuromodulin reveals the presence of a variety of fatty acylated species. J. Biol. Chem. 277, 33032–33040. doi: 10.1074/jbc.m204607200
Lin, L., Chen, H., Zhang, Y., Lin, W., Liu, Y., Li, T., et al. (2015). IL-10 Protects neurites in oxygen-glucose-deprived cortical neurons through the PI3K/Akt Pathway. PLoS One 10:e0136959. doi: 10.1371/journal.pone.0136959
Liu, S.-J., Gasperini, R., Foa, L., and Small, D. H. (2010). Amyloid-beta decreases cell-surface AMPA receptors by increasing intracellular calcium and phosphorylation of GluR2. J. Alzheimers Dis. 21, 655–666. doi: 10.3233/jad-2010-091654
Liu, Y. C., and Storm, D. R. (1989). Dephosphorylation of neuromodulin by calcineurin. J. Biol. Chem. 264, 12800–12804.
Lovinger, D. M., Colley, P. A., Akers, R. F., Nelson, R. B., and Routtenberg, A. (1986). Direct relation of long-term synaptic potentiation to phosphorylation of membrane protein F1, a substrate for membrane protein kinase C. Brain Res. 399, 205–211. doi: 10.1016/0006-8993(86)91510-6
Maekawa, S., Maekawa, M., Hattori, S., and Nakamura, S. (1993). Purification and molecular cloning of a novel acidic calmodulin binding protein from rat brain. J. Biol. Chem. 268, 13703–13709.
Maekawa, S., Murofushi, H., and Nakamura, S. (1994). Inhibitory effect of calmodulin on phosphorylation of NAP-22 with protein kinase C. J. Biol. Chem. 269, 19462–19465.
Maier, D. L., Mani, S., Donovan, S. L., Soppet, D., Tessarollo, L., Mccasland, J. S., et al. (1999). Disrupted cortical map and absence of cortical barrels in growth-associated protein (GAP)-43 knockout mice. Proc. Natl. Acad. Sci. U.S.A. 96, 9397–9402. doi: 10.1073/pnas.96.16.9397
Masliah, E. (1995). Mechanisms of synaptic dysfunction in Alzheimer’s disease. Histol. Histopathol. 10, 509–519.
Masliah, E., Mallory, M., Hansen, L., Alford, M., Albright, T., Deteresa, R., et al. (1991). Patterns of aberrant sprouting in Alzheimer’s disease. Neuron 6, 729–739. doi: 10.1016/0896-6273(91)90170-5
Mason, M. (2002). Transcriptional upregulation of SCG10 and CAP-23 Is correlated with regeneration of the axons of peripheral and central neurons in Vivo. Mol. Cell. Neurosci. 20, 595–615. doi: 10.1006/mcne.2002.1140
Masters, C. L., Simms, G., Weinman, N. A., Multhaup, G., Mcdonald, B. L., and Beyreuther, K. (1985). Amyloid plaque core protein in Alzheimer disease and Down syndrome. Proc. Natl. Acad. Sci. U.S.A. 82, 4245–4249. doi: 10.1073/pnas.82.12.4245
Matsubara, M., Nakatsu, T., Kato, H., and Taniguchi, H. (2004). Crystal structure of a myristoylated CAP-23/NAP-22 N-terminal domain complexed with Ca2+/calmodulin. EMBO J. 23, 712–718. doi: 10.1038/sj.emboj.7600093
Matsuda, W., Furuta, T., Nakamura, K. C., Hioki, H., Fujiyama, F., Arai, R., et al. (2009). Single nigrostriatal dopaminergic neurons form widely spread and highly dense axonal arborizations in the neostriatum. J. Neurosci. 29, 444–453. doi: 10.1523/jneurosci.4029-08.2009
Matsushima, H., Shimohama, S., Chachin, M., Taniguchi, T., and Kimura, J. (1996). Ca2+-dependent and Ca2+-independent protein kinase C changes in the brain of patients with Alzheimer’s disease. J. Neurochem. 67, 317–323. doi: 10.1046/j.1471-4159.1996.67010317.x
McGuire, C. B., Snipes, G. J., and Norden, J. J. (1988). Light-microscopic immunolocalization of the growth- and plasticity-associated protein GAP-43 in the developing rat brain. Brain Res. 469, 277–291. doi: 10.1016/0165-3806(88)90189-7
McLaughlin, R. E., and Denny, J. B. (1999). Palmitoylation of GAP-43 by the ER-Golgi intermediate compartment and Golgi apparatus. Biochim. Biophys. Acta 1451, 82–92. doi: 10.1016/s0167-4889(99)00074-9
Meiri, K. F., Pfenninger, K. H., and Willard, M. B. (1986). Growth-associated protein, GAP-43, a polypeptide that is induced when neurons extend axons, is a component of growth cones and corresponds to pp46, a major polypeptide of a subcellular fraction enriched in growth cones. Proc. Natl. Acad. Sci. U.S.A. 83, 3537–3541. doi: 10.1073/pnas.83.10.3537
Metz, G. A., and Schwab, M. E. (2004). Behavioral characterization in a comprehensive mouse test battery reveals motor and sensory impairments in growth-associated protein-43 null mutant mice. Neuroscience 129, 563–574. doi: 10.1016/j.neuroscience.2004.07.053
Miao, Y., Tipakornsaowapak, T., Zheng, L., Mu, Y., and Lewellyn, E. (2018). Phospho-regulation of intrinsically disordered proteins for actin assembly and endocytosis. FEBS J. 285, 2762–2784. doi: 10.1111/febs.14493
Miyake, K., Yamamoto, W., Tadokoro, M., Takagi, N., Sasakawa, K., Nitta, A., et al. (2002). Alterations in hippocampal GAP-43, BDNF, and L1 following sustained cerebral ischemia. Brain Res. 935, 24–31. doi: 10.1016/s0006-8993(02)02420-4
Mohammad, H. M. F., Sami, M. M., Makary, S., Toraih, E. A., Mohamed, A. O., and El-Ghaiesh, S. H. (2019). Neuroprotective effect of levetiracetam in mouse diabetic retinopathy: effect on glucose transporter-1 and GAP43 expression. Life Sci. 232:116588. doi: 10.1016/j.lfs.2019.116588
Morganti-Kossman, M. C., Lenzlinger, P. M., Hans, V., Stahel, P., Csuka, E., Ammann, E., et al. (1997). Production of cytokines following brain injury: beneficial and deleterious for the damaged tissue. Mol. Psychiatry 2, 133–136. doi: 10.1038/sj.mp.4000227
Morrison, J. H., and Hof, P. R. (2002). Selective vulnerability of corticocortical and hippocampal circuits in aging and Alzheimer’s disease. Prog. Brain Res. 136, 467–486. doi: 10.1016/s0079-6123(02)36039-4
Mosevitsky, M., and Silicheva, I. (2011). Subcellular and regional location of “brain” proteins BASP1 and MARCKS in kidney and testis. Acta Histochem. 113, 13–18. doi: 10.1016/j.acthis.2009.07.002
Mosevitsky, M. I. (2005). “Nerve ending “signal” proteins GAP-43, MARCKS, and BASP1. Int. Rev. Cytol. 245, 245–325. doi: 10.1016/s0074-7696(05)45007-x
Mosevitsky, M. I., Capony, J. P., Gyu, S., Novitskaya, V. A., Ayu, P., and Zakharov, V. V. (1997). The BASP1 family of myristoylated proteins abundant in axonal termini. Primary structure analysis and physico-chemical properties. Biochimie 79, 373–384. doi: 10.1016/s0300-9084(97)80032-6
Mosevitsky, M. I., Novitskaya, V. A., Ayu, P., and Gyu, S. (1994). Neuronal protein GAP-43 is a member of novel group of brain acid-soluble proteins (BASPs). Neurosci. Res. 19, 223–228. doi: 10.1016/0168-0102(94)90146-5
Mulkey, R. M., Endo, S., Shenolikar, S., and Malenka, R. C. (1994). Involvement of a calcineurin/inhibitor-1 phosphatase cascade in hippocampal long-term depression. Nature 369, 486–488. doi: 10.1038/369486a0
Munsie, L. N., and Truant, R. (2012). The role of the cofilin-actin rod stress response in neurodegenerative diseases uncovers potential new drug targets. BioArchitecture 2, 204–208. doi: 10.4161/bioa.22549
Musunuri, S., Wetterhall, M., Ingelsson, M., Lannfelt, L., Artemenko, K., Bergquist, J., et al. (2014). Quantification of the brain proteome in Alzheimer’s disease using multiplexed mass spectrometry. J. Proteome Res. 13, 2056–2068. doi: 10.1021/pr401202d
Nedivi, E., Basi, G. S., Akey, I. V., and Skene, J. H. (1992). A neural-specific GAP-43 core promoter located between unusual DNA elements that interact to regulate its activity. J. Neurosci. 12, 691–704. doi: 10.1523/jneurosci.12-03-00691.1992
Neve, R. L., Coopersmith, R., Mcphie, D. L., Santeufemio, C., Pratt, K. G., Murphy, C. J., et al. (1998). The neuronal growth-associated protein GAP-43 interacts with rabaptin-5 and participates in endocytosis. J. Neurosci. 18, 7757–7767. doi: 10.1523/jneurosci.18-19-07757.1998
Neve, R. L., Finch, E. A., Bird, E. D., and Benowitz, L. I. (1988). Growth-associated protein GAP-43 is expressed selectively in associative regions of the adult human brain. Proc. Natl. Acad. Sci. U.S.A. 85, 3638–3642. doi: 10.1073/pnas.85.10.3638
Nguyen, T. L. X., Kim, C. K., Cho, J.-H., Lee, K.-H., and Ahn, J.-Y. (2010). Neuroprotection signaling pathway of nerve growth factor and brain-derived neurotrophic factor against staurosporine induced apoptosis in hippocampal H19-7/IGF-IR [corrected]. Exp. Mol. Med. 42, 583–595. doi: 10.3858/emm.2010.42.8.060
Noureddine, M. A., Qin, X.-J., Oliveira, S. A., Skelly, T. J., Van Der Walt, J., Hauser, M. A., et al. (2005). Association between the neuron-specific RNA-binding protein ELAVL4 and Parkinson disease. Hum. Genet. 117, 27–33. doi: 10.1007/s00439-005-1259-2
O’Neil, K. T., and DeGrado, W. F. (1990). How calmodulin binds its targets: sequence independent recognition of amphiphilic alpha-helices. Trends Biochem. Sci. 15, 59–64. doi: 10.1016/0968-0004(90)90177-d
Pacelli, C., Giguere, N., Bourque, M. J., Levesque, M., Slack, R. S., and Trudeau, L. E. (2015). Elevated mitochondrial bioenergetics and axonal arborization size are key contributors to the vulnerability of dopamine neurons. Curr. Biol. 25, 2349–2360. doi: 10.1016/j.cub.2015.07.050
Palasz, E., Wysocka, A., Gasiorowska, A., Chalimoniuk, M., Niewiadomski, W., and Niewiadomska, G. (2020). BDNF as a promising therapeutic agent in Parkinson’s Disease. Int. J. Mol. Sci. 21:1170. doi: 10.3390/ijms21031170
Pang, Z. P., Cao, P., Xu, W., and Südhof, T. C. (2010). Calmodulin controls synaptic strength via presynaptic activation of calmodulin kinase II. J. Neurosci. 30, 4132–4142. doi: 10.1523/jneurosci.3129-09.2010
Parhad, I. M., Oishi, R., and Clark, A. W. (1992). GAP-43 gene expression is increased in anterior horn cells of amyotrophic lateral sclerosis. Ann. Neurol. 31, 593–597. doi: 10.1002/ana.410310605
Pascale, A., Gusev, P. A., Amadio, M., Dottorini, T., Govoni, S., Alkon, D. L., et al. (2004). Increase of the RNA-binding protein HuD and posttranscriptional up-regulation of the GAP-43 gene during spatial memory. Proc. Natl. Acad. Sci. U.S.A. 101, 1217–1222. doi: 10.1073/pnas.0307674100
Patel, N. K., Pavese, N., Javed, S., Hotton, G. R., Brooks, D. J., and Gill, S. S. (2013). Benefits of putaminal GDNF infusion in Parkinson disease are maintained after GDNF cessation. Neurology 81, 1176–1178. doi: 10.1212/wnl.0b013e3182a55ea5
Patterson, S. I., and Skene, J. H. (1999). A shift in protein S-palmitoylation, with persistence of growth-associated substrates, marks a critical period for synaptic plasticity in developing brain. J. Neurobiol. 39, 423–437. doi: 10.1002/(sici)1097-4695(19990605)39:3<423::aid-neu8>3.0.co;2-z
Perrone-Bizzozero, N. I., Cansino, V. V., and Kohn, D. T. (1993). Posttranscriptional regulation of GAP-43 gene expression in PC12 cells through protein kinase C-dependent stabilization of the mRNA. J. Cell Biol. 120, 1263–1270. doi: 10.1083/jcb.120.5.1263
Perrone-Bizzozero, N. I., Neve, R. L., Irwin, N., Lewis, S., Fischer, I., and Benowitz, L. I. (1991). Post-transcriptional regulation of GAP-43 mRNA levels during neuronal differentiation and nerve regeneration. Mol. Cell. Neurosci. 2, 402–409. doi: 10.1016/1044-7431(91)90027-l
Peskett, T. R., Rau, F., O’driscoll, J., Patani, R., Lowe, A. R., and Saibil, H. R. (2018). A liquid to solid phase transition underlying pathological huntingtin exon1 aggregation. Mol. Cell 70, 588–601.e6.
Phinney, A. L., Deller, T., Stalder, M., Calhoun, M. E., Frotscher, M., Sommer, B., et al. (1999). Cerebral amyloid induces aberrant axonal sprouting and ectopic terminal formation in amyloid precursor protein transgenic mice. J. Neurosci. 19, 8552–8559. doi: 10.1523/jneurosci.19-19-08552.1999
Qi, D., Ouyang, C., Wang, Y., Zhang, S., Ma, X., Song, Y., et al. (2014). HO-1 attenuates hippocampal neurons injury via the activation of BDNF-TrkB-PI3K/Akt signaling pathway in stroke. Brain Res. 1577, 69–76. doi: 10.1016/j.brainres.2014.06.031
Quattrone, A., Pascale, A., Nogues, X., Zhao, W., Gusev, P., Pacini, A., et al. (2001). Posttranscriptional regulation of gene expression in learning by the neuronal ELAV-like mRNA-stabilizing proteins. Proc. Natl. Acad. Sci. U.S.A. 98, 11668–11673. doi: 10.1073/pnas.191388398
Rechsteiner, M., and Rogers, S. W. (1996). PEST sequences and regulation by proteolysis. Trends Biochem. Sci. 21, 267–271. doi: 10.1016/s0968-0004(96)10031-1
Rekart, J. L., Meiri, K., and Routtenberg, A. (2005). Hippocampal-dependent memory is impaired in heterozygous GAP-43 knockout mice. Hippocampus 15, 1–7. doi: 10.1002/hipo.20045
Rekart, J. L., Quinn, B., Mesulam, M. M., and Routtenberg, A. (2004). Subfield-specific increase in brain growth protein in postmortem hippocampus of Alzheimer’s patients. Neuroscience 126, 579–584. doi: 10.1016/j.neuroscience.2004.03.060
Riederer, B. M., and Routtenberg, A. (1999). Can GAP-43 interact with brain spectrin? Brain Res. Mol. Brain Res. 71, 345–348. doi: 10.1016/s0169-328x(99)00179-5
Rostami, E., Krueger, F., Plantman, S., Davidsson, J., Agoston, D., Grafman, J., et al. (2014). Alteration in BDNF and its receptors, full-length and truncated TrkB and p75(NTR) following penetrating traumatic brain injury. Brain Res. 1542, 195–205. doi: 10.1016/j.brainres.2013.10.047
Routtenberg, A., and Lovinger, D. M. (1985). Selective increase in phosphorylation of a 47-kDa protein (F1) directly related to long-term potentiation. Behav. Neural Biol. 43, 3–11. doi: 10.1016/s0163-1047(85)91426-8
Saal, K.-A., Galter, D., Roeber, S., Bähr, M., Tönges, L., and Lingor, P. (2017). Altered expression of growth associated protein-43 and Rho kinase in human patients with Parkinson’s disease. Brain Pathol. 27, 13–25. doi: 10.1111/bpa.12346
Sandelius, Å, Sandgren, S., Axelsson, M., Malmeström, C., Novakova, L., Kostanjevecki, V., et al. (2019). Cerebrospinal fluid growth-associated protein 43 in multiple sclerosis. Sci. Rep. 9:17309.
Sanna, M. D., Quattrone, A., Ghelardini, C., and Galeotti, N. (2014). PKC-mediated HuD-GAP43 pathway activation in a mouse model of antiretroviral painful neuropathy. Pharmacol. Res. 81, 44–53. doi: 10.1016/j.phrs.2014.02.004
Sechi, A. S., and Wehland, J. (2000). The actin cytoskeleton and plasma membrane connection: PtdIns(4,5)P(2) influences cytoskeletal protein activity at the plasma membrane. J. Cell Sci. 113(Pt 21), 3685–3695.
Shen, Y., Mani, S., Donovan, S. L., Schwob, J. E., and Meiri, K. F. (2002). Growth-associated protein-43 is required for commissural axon guidance in the developing vertebrate nervous system. J. Neurosci. 22, 239–247. doi: 10.1523/jneurosci.22-01-00239.2002
Silver, J., and Miller, J. H. (2004). Regeneration beyond the glial scar. Nat. Rev. Neurosci. 5, 146–156. doi: 10.1038/nrn1326
Sjogren, M., Minthon, L., Davidsson, P., Granerus, A. K., Clarberg, A., Vanderstichele, H., et al. (2000). CSF levels of tau, beta-amyloid(1-42) and GAP-43 in frontotemporal dementia, other types of dementia and normal aging. J. Neural Transm. 107, 563–579. doi: 10.1007/s007020070079
Skene, J. H. (1989). Axonal growth-associated proteins. Annu. Rev. Neurosci. 12, 127–156. doi: 10.1146/annurev.ne.12.030189.001015
Skene, J. H., Jacobson, R. D., Snipes, G. J., Mcguire, C. B., Norden, J. J., and Freeman, J. A. (1986). A protein induced during nerve growth (GAP-43) is a major component of growth-cone membranes. Science 233, 783–786. doi: 10.1126/science.3738509
Skene, J. H., and Virág, I. (1989). Posttranslational membrane attachment and dynamic fatty acylation of a neuronal growth cone protein, GAP-43. J. Cell Biol. 108, 613–624. doi: 10.1083/jcb.108.2.613
Skene, J. H., and Willard, M. (1981). Changes in axonally transported proteins during axon regeneration in toad retinal ganglion cells. J. Cell Biol. 89, 86–95. doi: 10.1083/jcb.89.1.86
Smythe, E., and Ayscough, K. R. (2006). Actin regulation in endocytosis. J. Cell Sci. 119, 4589–4598. doi: 10.1242/jcs.03247
Spencer, S. A., Schuh, S. M., Liu, W. S., and Willard, M. B. (1992). GAP-43, a protein associated with axon growth, is phosphorylated at three sites in cultured neurons and rat brain. J. Biol. Chem. 267, 9059–9064.
Spillantini, M. G., Schmidt, M. L., Lee, V. M., Trojanowski, J. Q., Jakes, R., and Goedert, M. (1997). Alpha-synuclein in Lewy bodies. Nature 388, 839–840.
Strittmatter, S. M., Fankhauser, C., Huang, P. L., Mashimo, H., and Fishman, M. C. (1995). Neuronal pathfinding is abnormal in mice lacking the neuronal growth cone protein GAP-43. Cell 80, 445–452. doi: 10.1016/0092-8674(95)90495-6
Strittmatter, S. M., Igarashi, M., and Fishman, M. C. (1994). GAP-43 amino terminal peptides modulate growth cone morphology and neurite outgrowth. J. Neurosci. 14, 5503–5513. doi: 10.1523/jneurosci.14-09-05503.1994
Sudo, Y., Valenzuela, D., Beck-Sickinger, A. G., Fishman, M. C., and Strittmatter, S. M. (1992). Palmitoylation alters protein activity: blockade of G(o) stimulation by GAP-43. EMBO J. 11, 2095–2102. doi: 10.1002/j.1460-2075.1992.tb05268.x
Sun, M.-K., and Alkon, D. L. (2014). The “Memory Kinases”: roles of pkc isoforms in signal processing and memory formation. Prog. Mol. Biol. Transl. Sci. 122, 31–59.
Sun, Y., Leong, N. T., Jiang, T., Tangara, A., Darzacq, X., and Drubin, D. G. (2017). Switch-like Arp2/3 activation upon WASP and WIP recruitment to an apparent threshold level by multivalent linker proteins in vivo. eLife 6:e29140.
Tagawa, K., Homma, H., Saito, A., Fujita, K., Chen, X., Imoto, S., et al. (2015). Comprehensive phosphoproteome analysis unravels the core signaling network that initiates the earliest synapse pathology in preclinical Alzheimer’s disease brain. Hum. Mol. Genet. 24, 540–558. doi: 10.1093/hmg/ddu475
Takasaki, A., Hayashi, N., Matsubara, M., Yamauchi, E., and Taniguchi, H. (1999). Identification of the calmodulin-binding domain of neuron-specific protein kinase C substrate protein CAP-22/NAP-22. Direct involvement of protein myristoylation in calmodulin-target protein interaction. J. Biol. Chem. 274, 11848–11853. doi: 10.1074/jbc.274.17.11848
Tejero-Díez, P., Rodríguez-Sánchez, P., Martín-Cófreces, N. B., and Díez-Guerra, F. J. (2000). bFGF stimulates GAP-43 phosphorylation at ser41 and modifies its intracellular localization in cultured hippocampal neurons. Mol. Cell. Neurosci. 16, 766–780. doi: 10.1006/mcne.2000.0915
Teravskis, P. J., Covelo, A., Miller, E. C., Singh, B., Martell-Martínez, H. A., Benneyworth, M. A., et al. (2018). A53T mutant alpha-synuclein induces Tau-dependent postsynaptic impairment independently of neurodegenerative changes. J. Neurosci. 38, 9754–9767. doi: 10.1523/jneurosci.0344-18.2018
Tompa, P., and Fuxreiter, M. (2008). Fuzzy complexes: polymorphism and structural disorder in protein-protein interactions. Trends Biochem. Sci. 33, 2–8. doi: 10.1016/j.tibs.2007.10.003
Tonges, L., Szego, E. M., Hause, P., Saal, K. A., Tatenhorst, L., Koch, J. C., et al. (2014). Alpha-synuclein mutations impair axonal regeneration in models of Parkinson’s disease. Front. Aging Neurosci. 6:239. doi: 10.3389/fnagi.2014.00239
Tron, C., Lemaitre, F., Verstuyft, C., Petitcollin, A., Verdier, M. C., and Bellissant, E. (2019). Pharmacogenetics of membrane transporters of tacrolimus in solid organ transplantation. Clin. Pharmacokinet. 58, 593–613. doi: 10.1007/s40262-018-0717-7
Tsai, K. C., Cansino, V. V., Kohn, D. T., Neve, R. L., and Perrone-Bizzozero, N. I. (1997). Post-transcriptional regulation of the GAP-43 gene by specific sequences in the 3′ untranslated region of the mRNA. J. Neurosci. 17, 1950–1958. doi: 10.1523/jneurosci.17-06-01950.1997
Tysnes, O.-B., and Storstein, A. (2017). Epidemiology of Parkinson’s disease. J. Neural Transm. 124, 901–905.
Udvadia, A. J., Köster, R. W., and Skene, J. H. (2001). GAP-43 promoter elements in transgenic zebrafish reveal a difference in signals for axon growth during CNS development and regeneration. Development 128, 1175–1182.
Uzumcu, A., Candan, S., Toksoy, G., Uyguner, Z. O., Karaman, B., Eris, H., et al. (2009). Mutational screening of BASP1 and transcribed processed pseudogene TPPsig-BASP1 in patients with Möbius syndrome. J. Genet. Genomics 36, 251–256. doi: 10.1016/s1673-8527(08)60112-5
Wang, H. Y., Pisano, M. R., and Friedman, E. (1994). Attenuated protein kinase C activity and translocation in Alzheimer’s disease brain. Neurobiol. Aging 15, 293–298. doi: 10.1016/0197-4580(94)90023-x
Wang, M. J., Jiang, L., Chen, H. S., and Cheng, L. (2019a). Levetiracetam protects against cognitive impairment of subthreshold convulsant discharge model rats by activating protein kinase C (PKC)-Growth-Associated Protein 43 (GAP-43)-Calmodulin-Dependent Protein Kinase (CaMK) Signal Transduction Pathway. Med. Sci. Monit. 25, 4627–4638. doi: 10.12659/msm.913542
Wang, Q., Zhang, Y., Wang, M., Song, W.-M., Shen, Q., Mckenzie, A., et al. (2019b). The landscape of multiscale transcriptomic networks and key regulators in Parkinson’s disease. Nat. Commun. 10:5234.
Weber, J. R., and Skene, J. H. (1997). Identification of a novel repressive element that contributes to neuron-specific gene expression. J. Neurosci. 17, 7583–7593. doi: 10.1523/jneurosci.17-20-07583.1997
Wegmann, S., Eftekharzadeh, B., Tepper, K., Zoltowska, K. M., Bennett, R. E., Dujardin, S., et al. (2018). Tau protein liquid-liquid phase separation can initiate tau aggregation. EMBO J. 37:e98049.
Wetmore, C., Olson, L., and Bean, A. J. (1994). Regulation of brain-derived neurotrophic factor (BDNF) expression and release from hippocampal neurons is mediated by non-NMDA type glutamate receptors. J. Neurosci. 14, 1688–1700. doi: 10.1523/jneurosci.14-03-01688.1994
Whitehouse, P. J., Price, D. L., Struble, R. G., Clark, A. W., Coyle, J. T., and Delon, M. R. (1982). Alzheimer’s disease and senile dementia: loss of neurons in the basal forebrain. Science 215, 1237–1239. doi: 10.1126/science.7058341
Widmer, F., and Caroni, P. (1990). Identification, localization, and primary structure of CAP-23, a particle-bound cytosolic protein of early development. J. Cell Biol. 111, 3035–3047. doi: 10.1083/jcb.111.6.3035
Wiederkehr, A., Staple, J., and Caroni, P. (1997). The motility-associated proteins GAP-43, MARCKS, and CAP-23 share unique targeting and surface activity-inducing properties. Exp. Cell Res. 236, 103–116. doi: 10.1006/excr.1997.3709
Wu, H., Lu, D., Jiang, H., Xiong, Y., Qu, C., Li, B., et al. (2008). Simvastatin-mediated upregulation of VEGF and BDNF, activation of the PI3K/Akt pathway, and increase of neurogenesis are associated with therapeutic improvement after traumatic brain injury. J. Neurotrauma 25, 130–139. doi: 10.1089/neu.2007.0369
Xiao, H.-S., Huang, Q.-H., Zhang, F.-X., Bao, L., Lu, Y.-J., Guo, C., et al. (2002). Identification of gene expression profile of dorsal root ganglion in the rat peripheral axotomy model of neuropathic pain. Proc. Natl. Acad. Sci. U.S.A. 99, 8360–8365.
Yamamoto, Y., Sokawa, Y., and Maekawa, S. (1997). Biochemical evidence for the presence of NAP-22, a novel acidic calmodulin binding protein, in the synaptic vesicles of rat brain. Neurosci. Lett. 224, 127–130. doi: 10.1016/s0304-3940(97)13482-6
Yang, P., Qin, Y., Bian, C., Zhao, Y., and Zhang, W. (2015). Intrathecal delivery of IL-6 reactivates the intrinsic growth capacity of pyramidal cells in the sensorimotor cortex after spinal cord injury. PLoS One 10:e0127772. doi: 10.1371/journal.pone.0127772
Yang, P., Wen, H., Ou, S., Cui, J., and Fan, D. (2012). IL-6 promotes regeneration and functional recovery after cortical spinal tract injury by reactivating intrinsic growth program of neurons and enhancing synapse formation. Exp. Neurol. 236, 19–27. doi: 10.1016/j.expneurol.2012.03.019
Zakharov, V. V., Capony, J.-P., Derancourt, J., Kropolova, E. S., Novitskaya, V. A., Bogdanova, M. N., et al. (2003). Natural N-terminal fragments of brain abundant myristoylated protein BASP1. Biochim. Biophys. Acta 1622, 14–19. doi: 10.1016/s0304-4165(03)00099-0
Zakharov, V. V., and Mosevitsky, M. I. (2007). M-calpain-mediated cleavage of GAP-43 near Ser41 is negatively regulated by protein kinase C, calmodulin and calpain-inhibiting fragment GAP-43-3. J. Neurochem. 101, 1539–1551. doi: 10.1111/j.1471-4159.2007.04452.x
Zakharov, V. V., and Mosevitsky, M. I. (2010). Oligomeric structure of brain abundant proteins GAP-43 and BASP1. J. Struct. Biol. 170, 470–483. doi: 10.1016/j.jsb.2010.01.010
Keywords: GAP-43, BASP1, phosphorylation, neural injury response, axon regeneration, neurodegenerative diseases
Citation: Chung D, Shum A and Caraveo G (2020) GAP-43 and BASP1 in Axon Regeneration: Implications for the Treatment of Neurodegenerative Diseases. Front. Cell Dev. Biol. 8:567537. doi: 10.3389/fcell.2020.567537
Received: 29 May 2020; Accepted: 14 August 2020;
Published: 03 September 2020.
Edited by:
João M. N. Duarte, Lund University, SwedenReviewed by:
Annalisa Buffo, University of Turin, ItalySol Sotillos, Andalusian Center for Developmental Biology (CABD), Spain
Elisa Tamariz, University of Veracruz, Mexico
Copyright © 2020 Chung, Shum and Caraveo. This is an open-access article distributed under the terms of the Creative Commons Attribution License (CC BY). The use, distribution or reproduction in other forums is permitted, provided the original author(s) and the copyright owner(s) are credited and that the original publication in this journal is cited, in accordance with accepted academic practice. No use, distribution or reproduction is permitted which does not comply with these terms.
*Correspondence: Gabriela Caraveo, gabriela.piso@northwestern.edu