Abstract
A good durability of concrete under nuclear conditions is essential for the safe operating of nuclear powerplants. In this case, concrete is generally exposed to a relatively high range of temperature compared to ordinary situations, and the understanding of its thermomechanical behavior becomes required for a fair safety assessment. To achieve this objective, the thermomechanical behavior of the concrete components should be understood. Among these components, this paper focuses on aggregates due to their high proportion in concrete and the high heterogeneity. A granite sample is selected, and it is composed of quartz, plagioclase, K-feldspar, biotite and some minor phases of muscovite, zircon, chlorite, and fluorite. The CTE according to three orthogonal directions is measured and small difference is observed. According to the XRCT examination, the samples do not show any visible cracks. However, some voids surrounding heavy phases are detected before the heating process. To identify these heavy phases, SEM–EDS is used, and some micro cracks have been detected and linked to biotite and pargasite. The numerical estimation of the average of the high and low bounds underestimates the CTE compared to the experimental value. It is consistent with the pre-damaged state providing a higher strain for an equivalent thermic stress. Two-phase model composed from an inclusion inside a predominant phase is used to calculate the hydrostatic pressure. The combinations giving high pressure allow to identify the zones of weakness in the granite. It also shows a higher probability of cracking for the biotite and pargasite inclusions inside quartz.









Similar content being viewed by others
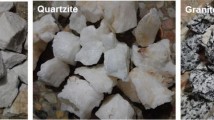
Abbreviations
- BSW:
-
Biological shield wall
- CBS:
-
Concrete biological shield
- CTE:
-
Coefficient of thermal expansion
- HCP:
-
Hardened cement paste
- NPP:
-
Nuclear powerplant
- PPL:
-
Plane polarized light
- RIVE:
-
Radiation-induced volumetric expansion
- RVE:
-
Representative volume element
- VRH:
-
Voigt reuss hill
- XPL:
-
Cross polarized light
- XRCT:
-
X-ray computed tomography
- \({D}_{i}\) :
-
The diameter of the phase i (μm)
- \({K}_{i}\) :
-
The bulk modulus of the phase i (GPa)
- \({\mathrm{\alpha }}_{i}\) :
-
Coefficient of thermal expansion of the phase i (10–6/ °C)
- \({\Phi }_{i}\) :
-
The volume fraction of the phase i
- \(\Delta T\) :
-
The temperature increment (°C)
- GoF:
-
Goodness of Fit defined as the ratio of Rwp and Rexp
- Rexp :
-
The Expected R-factor corresponding to the best possible Rwp
- RWP :
-
The Weighted Profile R-factor
References
Naus DJ (2012) “Concrete,” in Comprehensive Nuclear Materials, pp. 407–431
Samouh H, Soive A, Rozière E, Loukili A (2016) Experimental and numerical study of size effect on long-term drying behavior of concrete: influence of drying depth. Mater Struct 49(10):4029–4048
Samouh H, Rozière E, Wisniewski V, Loukili A (2017) Consequences of longer sealed curing on drying shrinkage, cracking and carbonation of concrete. Cem Concr Res 95:117–131
van Aardt JHP, Visser S (1977) Formation of hydrogarnets: Calcium hydroxide attack on clays and feldspars. Cem Concr Res 7(1):39–44
van Aardt JHP, Visser S (1977) Calcium hydroxide attack on feldspars and clays: possible relevance to cement-aggregate reactions. Cem Concr Res 7(6):643–648
Rymes J et al (2019) Long-term material properties of a thick concrete wall exposed to ordinary environmental conditions in a nuclear reactor building:the contribution of cement hydrates and feldspar interaction. J Adv Concr Technol 17(May):195–215
Samouh H, Rozière E, Loukili A (2018) Shape effect on drying behavior of cement-based materials: mechanisms and numerical analysis. Cem Concr Res 110(May):42–51
Samouh H, Rozière E, Loukili A (2017) The differential drying shrinkage effect on the concrete surface damage: experimental and numerical study. Cem Concr Res 102(December):212–224
Samouh H, Rozière E, Loukili A (2019) Experimental and numerical study of the relative humidity effect on drying shrinkage and cracking of self-consolidating concrete. Cem Concr Res 115(January):519–529
Maruyama I, Sasano H, Nishioka Y, Igarashi G (2014) Strength and Young’s modulus change in concrete due to long-term drying and heating up to 90°c. Cem Concr Res 66:48–63
Rozière E, Loukili A, El Hachem R, Grondin F (2009) Durability of concrete exposed to leaching and external sulphate attacks. Cem Concr Res 39(12):1188–1198
Rozière E, Loukili A, Cussigh F (2009) A performance based approach for durability of concrete exposed to carbonation. Constr Build Mater 23(1):190–199
Fillmore DL (2004) “Literature review of the effects of radiation and temperature on the aging of concrete,”
Hilsdorf H, Kropp J, Koch H (1978) The effects of nuclear radiation on the mechanical properties of concrete. ACI Sp 55:223–251
Rosseel TM et al (2016) Review of the current state of knowledge on the effects of radiation on concrete. J Adv Concr Technol 14(7):368–383
Field KG, Remec I, Le Pape Y (2015) Radiation effects in concrete for nuclear power plants - Part I: quantification of radiation exposure and radiation effects. Nucl Eng Des 282:126–143
Kontani O, Sawada S, Maruyama I, Takizawa M, Sato O (2013) “Evaluation of irradiation effects on concrete structure-Gamma-ray irradiation tests on cement paste-,” in Proceedings of the ASME 2013 Power Conference POWER2013, Boston, Massachusetts, USA
Golewski GL (2018) Evaluation of morphology and size of cracks of the interfacial transition zone (ITZ) in concrete containing fly ash (FA). J Hazard Mater 357:298–304
Akçaoǧlu T, Tokyay M, Çelik T (2005) Assessing the ITZ microcracking via scanning electron microscope and its effect on the failure behavior of concrete. Cem Concr Res 35(2):358–363
Maruyama I, Sasano H, Lin M (2016) Impact of aggregate properties on the development of shrinkage-induced cracking in concrete under restraint conditions. Cem Concr Res 85:82–101
Igarashi G, Maruyama I, Nishioka Y, Yoshida H (2015) Influence of mineral composition of siliceous rock on its volume change. Constr Build Mater 94:701–709
Maruyama I, Muto S (2016) Change in relative density of natural rock minerals due to electron irradiation. J Adv Concr Technol 14(11):706–716
Vu NL et al (2020) (2020) “Swelling of alpha-quartz induced by MeV ions irradiation: critical dose and swelling mechanism.” J Nucl Mater 539:152266
Luu VN et al (2021) Changes in properties of alpha-quartz and feldspars under 3 MeV Si-ion irradiation. J Nucl Mater 545:152734
Maruyama I et al (2017) Development of soundness assessment procedure for concrete members affected by neutron and gamma-ray irradiation. J Adv Concr Technol 15(9):440–523
Ishikawa S, Maruyama I, Takizawa M, Etoh J, Kontani O, Sawada S (2019) Hydrogen production and the stability of hardened cement paste under gamma irradiation. J Adv Concr Technol 17(12):673–685
Maruyama I et al (2017) Impact of gamma-ray irradiation on hardened white Portland cement pastes exposed to atmosphere. Cem Concr Res 108:59–71
Maruyama I, Igarashi G (2015) Numerical approach towards aging management of concrete structures: material strength evaluation in a massive concrete structure under one-sided heating. J Adv Concr Technol 13(11):500–527
Maruyama I, Igarashi G (2014) Cement reaction and resultant physical properties of cement paste. J Adv Concr Technol 12(6):200–213
Krishnan NMA, Le Pape Y, Sant G, Bauchy M (2018) Effect of irradiation on silicate aggregates’ density and stiffness. J Nucl Mater 512:126–136
Maruyama I, Lura P (2019) “Properties of early-age concrete relevant to cracking in massive concrete,” Cem Concr Res, 123, p. 105770
Mindess S, Young JF, Darwin D (2002) Concrete
Plevova E, Vaculikova L, Kozusnikova A, Ritz M, Simha MG (2016) Thermal expansion behaviour of granites. J Therm Anal Calorim 123(2):1555–1561
Zhao P, Feng ZJ (2019) Thermal deformation of granite under different temperature and pressure pathways. Adv Mater Sci Eng. https://doi.org/10.1155/2019/7869804
Hockman A, Kessler DW (1934) Thermal and moisture expansion studies of some domestic granites. J Res Natl Bur Stand 44(4):395–410
Wong T-F, Brace WF (1979) Thermal expansion of rocks: some measurements at high pressure. Tectonophysics 57(2–4):95–117
Hobbs DW (1971) The dependence of the bulk modulus, Young’s modulus, creep, shrinkage and thermal expansion of concrete upon aggregate volume concentration. Matériaux Constr 4(2):107–114
CEN- EN 12407(2019) Natural stone test methods—Petrographic examination
Larrea ML, Martig SR, Castro SM, Aliani PA, Bjerg EA, “Rock.AR—a point counting application for petrographic Thin Sections.”
Electric Power Research Institute (2016) “Long-term operations: impact of radiation heating on pwr biological shield concrete, EPRI Report 3002008129,”
Bruck PM, Esselman TC, Elaidi BM, Wall JJ, Wong EL (2019) Structural assessment of radiation damage in light water power reactor concrete biological shield walls. Nucl Eng Des 350(February):9–20
Osnes JD, Coates GK, Delong KB, Loken MC, WagnerRA (1984) “Expected repository environments in granite: Thermal environment,”
Van der Molen I (1981) The shift of the α-β transition temperature of quartz associated with the thermal expansion of granite at high pressure. Tectonophysics 73(4):323–342
Sprunt ES, Brace WF (1974) Direct observation of microcavities in crystalline rocks. Int J Rock Mech Min Sci 11(4):139–150
Samouh H et al (2021) Modal analysis of rock forming minerals: contribution of XRD/rietveld analysis compared to the classic point counting method. J Adv Concr Technol 19(5):395–413
Lin W (2002) Permanent strain of thermal expansion and thermally induced microcracking in Inada granite. J Geophys Res Solid Earth. https://doi.org/10.1029/2001JB000648
Homand-Etienne F, Houpert R (1989) Thermally induced microcracking in granites: characterization and analysis. Int J Rock Mech Min Sci 26(2):125–134
Vázquez P, Shushakova V, Gómez-Heras M (2015) Influence of mineralogy on granite decay induced by temperature increase: Experimental observations and stress simulation. Eng Geol 189:58–67
Calleja L, Ruiz De Argandoña VM (1985) Variación de la expansión térmica en rocas cristalinas. Trab Geol 15(12):307–315
Richter D, Simmons G (1974) Thermal expansion behavior of igneous rocks. Int J Rock Mech Min Sci 11(10):403–411
Walsh JB, Decker ER (1966) Effect of pressure and saturating fluid on the thermal conductivity of compact rock. J Geophys Res 71(12):3053–3061
Adams LH, Williamson ED (1923) On the compressibility of minerals and rocks at high pressures. J Franklin Inst 195(4):475–529
Wanne TS, Young RP (2008) Bonded-particle modeling of thermally fractured granite. Int J Rock Mech Min Sci 45(5):789–799
Huotari T, Kukkonen I (2004) “Thermal expansion properties of rocks:Literature survey and estimation of thermal expansion coefficient for Olkiluoto Mica Gneiss,”
Adie AJ (1835) On the expansion of different kinds of stone from an increase of temperature with a description of the pyrometer used in making experiments. Prog Civ Eng 24(3):200–211
Dwivedi RD, Goel RK, Prasad VVR, Sinha A (2008) Thermo-mechanical properties of Indian and other granites. Int J Rock Mech Min Sci 45(3):303–315
Ramana YV, Sarma LP (1980) Thermal expansion of a few Indian granitic rocks. Phys Earth Planet Inter 22(1):36–41
Heyliger P, Ledbetter H, Kim S (2003) Elastic constants of natural quartz. J Acoust Soc Am 114(2):644–650
Brown JM (2015) Determination of Hashin-Shtrikman bounds on the isotropic effective elastic moduli of polycrystals of any symmetry. Comput Geosci 80:95–99
Angel RJ, Zhao J, Kaminsky W, Ross N, Waeselmann N, Brown JM (2016) The elastic tensor of monoclinic alkali feldspars. Am Mineral 101(5):1228–1231
Alexandrov (1974) “Microcline,” [Online]. Available: http://www.gm.univ-montp2.fr/PERSO/mainprice/W_data/CareWare_Unicef_Databases/MTEX_Elastic_Tensor_Files/Microcline_1974.m
Moos D, Dvorkin J (1997) Application of theoretically derived rock physics relationships for clastic rocks to log data from the Wilmington Field, CA. Geophys Res Lett 24(3):329–332
Comboni D, Lotti P, Gatta GD, Merlini M, Liermann HP, Frost DJ (2018) Pargasite at high pressure and temperature. Phys Chem Miner 45(3):259–278
Roberts RB, White GK (1986) Thermal expansion of fluorites at high temperatures. J Phys C Solid State Phys 19(36):7167–7172
Speziale S, Duffy TS (2002) Single-crystal elastic constants of fluorite (CaF2) to 9.3 GPa. Phys Chem Miner 29(7):465–472
Peter Watt J (1987) POLYXSTAL a FORTRAN program to calculate average elastic properties of minerals from single-crystal elasticity data. Comput Geosci. https://doi.org/10.1016/0098-3004(87)90050-1
Zanazzi P, Francesco Comodi P, Nazzareni S, Andreozzi G (2009) Thermal behaviour of chlorite: an in situ single-crystal and powder diffraction study. Eur J Mineral. https://doi.org/10.1127/0935-1221/2009/0021-1928
Mookherjee M, Mainprice D (2014) Unusually large shear wave anisotropy for chlorite in subduction zone settings. Geophys Res Lett 10(April):1506–1513
Subbarao EC, Agrawal DK, McKinstry HA, Sallese CW, Roy R (1990) Thermal expansion of compounds of zircon structure. J Am Ceram Soc 73(5):1246–1252
Momenzadeh L, Belova IV (2020) Murch GE (2019) “Prediction of the lattice thermal conductivity of zircon and the cubic and monoclinic phases of zirconia by molecular dynamics simulation.” Comput Mater Sci 176:109522
Bruner WM (1979) Crack growth and the thermoelastic behaviour of rocks. J Geophys Res 84(B10):5578–5590
Thirumalai K, Demou SG (1974) Thermal expansion behavior of intact and thermally fractured mine rocks. AIP Conf Proc 17(60):60–71
Cooper HW, Simmons G (1977) The effect of cracks on the thermal expansion of rocks. Earth Planet Sci Lett 36(3):404–412
Wang F, Konietzky H (2019) Thermo-mechanical properties of granite at elevated temperatures and numerical simulation of thermal cracking. Rock Mech Rock Eng 52(10):3737–3755
Subbarao EC, Gokhale KVGK (1968) Thermal expansion of zircon. Jpn J Appl Phys 7:1126
Acknowledgements
The authors gratefully acknowledge the support of Ministry of Economy, Trade, and Industry (METI) in Japan, through the Japan Concrete Aging Management Program on Irradiation Effects (JCAMP).
Funding
Ministry of Economy,Trade,and Industry (METI) in Japan,Japan Concrete Aging Management Program on Irradiation Effects (JCAMP).
Author information
Authors and Affiliations
Corresponding author
Ethics declarations
Conflicts of interest
The authors declared that there is no conflict of interest.
Additional information
Publisher's Note
Springer Nature remains neutral with regard to jurisdictional claims in published maps and institutional affiliations.
Rights and permissions
About this article
Cite this article
Samouh, H., Ishikawa, S., Kontani, O. et al. Thermomechanical behavior of granite under 150 °C: experimental and numerical analysis. Mater Struct 54, 239 (2021). https://doi.org/10.1617/s11527-021-01830-7
Received:
Accepted:
Published:
DOI: https://doi.org/10.1617/s11527-021-01830-7