Abstract
The tubular flow-through electrode (FTE) is a facile electroanalytical tool for investigating electrochemical reaction kinetics; however, its suitability for this purpose requires careful design and operation under conditions that guarantee uniform current distribution. In this perspective article, we provide a scaling analysis of the transport and reaction processes within a tubular FTE leading to quantitative guidelines for the FTE design and operation. Two dimensionless parameters (classical Wagner number WaI and modified Wagner number incorporating the effect of mass transport WaII) are utilized to compare the magnitudes of the ohmic, activation and transport resistances of the tubular FTE, and to quantify its current distribution uniformity. With the aid of analytical modeling of the current distribution, optimal ranges for these two dimensionless parameters are defined so as to ascertain uniform current distribution. Application of these guidelines to a graphite tubular FTE is shown to enable the precise determination of the charge-transfer kinetics of the ferri/ferrocyanide redox reaction.
Export citation and abstract BibTeX RIS
Tubular flow-through electrodes (FTEs), consisting of a single cylindrical pore through which electrolyte is passed, have been extensively used as analytical tools in applications such as electrochemical sensing. 1,2 FTEs are easy to fabricate and use, and they facilitate electrochemical measurements in the presence of convective flow. 3,4 Generally, the direction of convective flow is parallel to the electrical field in FTEs as opposed to the flow-by configuration where flow is perpendicular to the electrical field. 5,6 Due to the high rates of convective mass transfer, FTEs enable detection of electroactive species below 10–8 M. 3,7 The growing interest in "flow-through" and "flow-by" porous electrodes in modern flow batteries and electrochemical reactors has resurrected the interest in FTEs, which represent an idealized single-pore embodiment of an otherwise complex pore network. 8,9 The transport characteristics of tubular FTEs are well-understood, 4 and they more closely mimic the transport characteristics of a practical porous flow-through electrode than the widely used rotating disk electrode (RDE). 10–12
The tubular FTE, much like other electrode configurations, can be a reliable analytical tool for precise measurement of electrochemical kinetics only if the electrode provides a sufficiently uniform distribution of the reaction current (rate) over its surface. Current distribution non-uniformity can result from preferential transport (via migration, diffusion or convection) of the reacting species to more accessible portions of the tubular FTE. Alkire and Mirarefi computed the current distribution within FTEs by solving the Laplace's equation and the convection–diffusion equation. 9,13 They showed that, in a long tubular electrode with negligible mass transfer resistance, non-uniform current distribution results when the ohmic resistance is more dominant than the kinetic resistance. This is particularly evident when using resistive electrolytes and studying highly reversible charge-transfer reactions. Ben-Porat et al. extended the analysis to also consider the effect of electrode resistance and showed that uniformity in electroplating can be achieved when the cathodic charge-transfer resistance is more dominant than the metal electrode resistance. 14 Blaedel et al. investigated the use of platinum flow-through electrodes for electroanalytical studies, 3,7,15–17 and recommended the use of FTEs only at low operating currents so as to maintain uniform current distribution. The recent resurgence of tubular FTEs for electroanalytical studies and applications, 18–20 and the general lack of user-friendly guidelines on how to design and operate a tubular FTE for this purpose, motivated us to take a fresh look at this classical electrode geometry and analyze its salient features.
The goal of this perspective article is to develop guidelines for the design and operation of a tubular FTE for its use as an electroanalytical tool for reliable measurement of electrochemical kinetics. This goal is accomplished through a combination of scaling analysis and analytical modeling of the current distribution, which leads to quantitative criterion (based on two dimensionless parameters: WaI and WaII) for selecting the tubular FTE dimensions and operating average current density. We apply the developed guidelines to the design of a graphite tubular FTE and show that they lead to kinetics parameters for the ferri/ferrocyanide redox reaction that are in excellent agreement with those obtained using RDE.
Experimental
Fabrication of graphite tubular FTE
A simple graphite tubular flow-through electrode (FTE) was prepared by drilling a 1-mm-diameter hole (d = 1 mm) through a graphite rod (6.15 mm diameter, 99.9995% metals basis, Alfa Aesar). The rod was cut to 4.5 mm in length (L = 4.5 mm). A conductive silver wire was glued with conductive epoxy to the outer surface of the graphite electrode in order to make proper electrical contact. Non-conductive epoxy (thickness ≈ 0.02 cm) was applied on all outer surfaces of the electrode. Thus, only the interior surface of the FTE was exposed to the electrolyte. The electrode was glued with non-conductive epoxy to the end of a glass tube (ID ≈ 5.6 mm), which was used in a three-electrode cell described in Fig. 1. Before electrochemical experiments, the FTE was cured in the oven at 100 °C for > 12 h and then soaked in isopropyl alcohol (IPA) for 10 min. The IPA was allowed to evaporate in the oven for 5 min.
Figure 1. (a) Schematic representation of a tubular FTE experimental setup. (b) 3-D cross-section showing flow of electrolyte within the FTE in the axial direction. (c) Schematic representation of the various transport and reaction processes within the tubular FTE, assuming a ferri/ferrocyanide redox reaction.
Download figure:
Standard image High-resolution imageFabrication of graphite RDE
The RDE was manufactured from a graphite rod. The graphite rod was shaped with 600-grit silicon carbide paper, polished with 5, 0.3, and 0.05-μm alumina polishing solutions on a micro-fiber polishing cloth, and washed with DI water. The diameter of the RDE was 0.5 cm.
Steady-state polarization using FTE and RDE
As shown in Fig. 1a, the graphite tubular FTE was tested in a custom two-compartment cell with a three-electrode configuration consisting of the FTE as the working electrode, a coiled platinum (Pt) counter electrode, and a saturated Ag/AgCl reference electrode (Pine Research Instrumentation, Inc.). The two compartments were separated by a Nafion membrane to avoid re-oxidation and re-reduction of species electrochemically generated at the Pt counter electrode. The electrolytes consisted of varying equimolar concentrations (1, 0.5, 0.25, and 0.1 mM) of potassium hexacyanoferrate (II) trihydrate (K4[Fe(CN)6]·3H2O, Sigma Aldrich) and potassium hexacyanoferrate (III) (K3Fe(CN)6, >99.0% purity, Sigma Aldrich) supported by 1 M KCl. The equilibrium potential (Eeq) of the working electrode was well-defined, measurable and stable in the presence of equimolar amounts of [Fe(CN)6]4– and [Fe(CN)6]3– in the electrolytes. The electrolyte flow direction was upward through the graphite tubular FTE at a flow rate (V0) of 5 cm3 min−1 controlled by a syringe pump (KDS200, KD Scientific). A SP-300 Potentiostat (Bio-Logic Science Instruments, France) was used for electrochemical measurements. Before steady-state polarization experiments were performed on the FTE and RDE, cyclic voltammetry (potential range of −0.4 to 0.2 V vs Ag/AgCl) at a scan rate of 0.02 V s−1 was applied to the FTE for 5 cycles in 1 M KCl to reactivate the electrode surface. To obtain steady-state polarization curves, chronoamperometry experiments were performed as follows: the working electrode potential was changed stepwise in increments of 0.01 V and the transient current response at each potential was recorded until steady-state current was achieved. Applied potentials were within the electrochemical stability window of water. Electrochemical impedance spectroscopy (EIS) was performed to determine the ohmic resistance, which was needed for iRΩ correction. Steady-state polarization measurements and EIS were also performed on the RDE at a rotation rate of 500 rpm.
Results and Discussion
Dimensionless parameters representing transport and reaction processes in a tubular FTE
We first perform scaling analysis for a simplified one-dimensional tubular FTE geometry shown in Fig. 1 to assess the current distribution uniformity over the FTE surface. For clarity, Table I lists definitions of all the symbols used in this work. For scaling analysis, we assume: (i) A high aspect ratio FTE with L ≫ d; (ii) Negligible potential and concentration gradients in the radial direction within the tubular FTE; and (iii) Current density on the interior surface of the FTE depends only on the axial position z.
Table I. List of symbols and definitions used in this work.
Ac | Cross-sectional area of FTE, cm2 |
As | Interior surface area of FTE, cm2 |
Cb | Concentration of equimolar redox species in the bulk electrolyte, M |
Cj | Concentration of ferrocyanide (j = II) and ferricyanide (j = III), M |
Dj | Diffusion coefficient of ferrocyanide (j = II) and ferricyanide (j = III), cm2/s |
d | Diameter of FTE, cm |
Eeq | Equilibrium potential of [Fe(CN)6]4–/[Fe(CN)6]3– redox couple, V |
F | Faraday's constant (= 96485 C mol−1) |
I | Total current at the FTE-electrolyte interface, mA |
Ic | Total axial current flowing through the electrolyte within FTE [= Ac·ic], mA |
Is | Total reaction current density on FTE surface [= As·is], mA |
i | Current density, mA cm−2 |
iavg | Average current density at the FTE-electrolyte interface, mA/cm2 |
ic | Axial current density flowing through the electrolyte within FTE, mA/cm2 |
ik | Kinetic current density, mA/cm2 |
iL | Transport-limited current density, mA/cm2 |
is | Reaction current density on FTE surface, mA/cm2 |
i0 | Exchange current density of the redox reaction, mA/cm2 |
L | Length of FTE, cm |
m | A parameter characterizing the current distribution [= WaI −0.5], dimensionless |
n | Number of electrons transferred in the redox reaction, dimensionless |
R | universal gas constant (= 8.314 J mol−1 K−1) |
Ra | Activation resistance, Ω |
Rconv | Convective mass transport resistance, Ω |
Rdiff | Diffusional mass transport resistance, Ω |
Rmt | Total mass transport resistance, Ω |
RΩ | Ohmic resistance of the electrolyte, Ω |
rRDE | Radius of RDE, cm |
T | Temperature, K |
Vapp | Applied electrode potential, V |
V0 | Flow rate in FTE, cm3/min |
v0 | Average axial flow velocity in FTE [= 4V0 /πd2], cm/s |
WaI | First Wagner number, dimensionless |
WaII | Second Wagner number, dimensionless |
z | Axial position coordinate in FTE (inlet: z = 0; outlet: z = L), cm |
αa | Anodic charge transfer coefficient, dimensionless |
ηa | Activation overpotential, V |
ηc | Concentration overpotential, V |
ηΩ | Ohmic overpotential, V |
κ | Electrolyte conductivity, S/cm |
μ | A parameter characterizing the current distribution, dimensionless |
For scaling analysis, the activation, ohmic and mass transport resistances associated with the tubular FTE were first calculated. The activation resistance (Ra) is the reaction resistance due to irreversible charge transfer at the FTE-electrolyte interface. The ohmic resistance (RΩ) is the resistance of the electrolyte through which ionic current flows in the presence of an electric field. Finally, the mass transport resistance (Rmt) is the resistance associated with diffusional or convective transport of species to the reacting interface.
By definition, the activation resistance (Ra) is the gradient of the activation overpotential vs current curve calculated at the average value of the current. If we assume Tafel kinetics, 21 then

Similarly, the ohmic resistance (RΩ) of the tubular FTE can be calculated as:

In a well-supported electrolyte, the resistance associated with transport of reacting species (Rmt) may be attributed to diffusional or convective transport. Since diffusional or convective transport may occur in tandem in a tubular FTE (resistances in parallel), we can write:

The diffusional transport resistance (Rdiff) is the gradient of the concentration overpotential vs current curve:
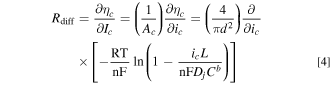
For small values of ic, Rdiff can be approximated as:

Similarly, the convective mass transport resistance (Rconv) can be determined:


We now define two dimensionless parameters. Here, we refer to these as WaI, i.e., the classical Wagner number widely used to analyze electrochemical systems, 22–24 and WaII, i.e., a modified version of the Wagner number incorporating the effect of mass transport. WaI represents the ratio of the activation resistance to the electrolyte ohmic resistance, and WaII represents the ratio of the activation resistance to the mass transport resistance: 22,25,26


For any practical tubular FTE operating at typical flow rates, Lv0 is of the order of 1 cm2 s−1 and thus Lv0 ≫ Dj implying that convection is significantly more effective at transporting reactant species compared to diffusion. Thus, for a typical tubular FTE with convection, Eq. 9 simplifies to:

Note that WaII is inversely correlated to the Thiele modulus (Th) and Damköhler number (Da) commonly found in literature on chemical kinetics. 27,28 From Eqs. 8 and 9, it can be concluded that for uniform current distribution in a tubular FTE whereby the overall process is activation controlled (ohmic and transport resistances are small in comparison to the activation resistance), WaI and WaII must be large quantities.
Figure 2 shows WaI and WaII values plotted as a function of the average current density (iavg). For this plot, parameter values, e.g., Cb, n, v0, αa, and κ, are reported in Table II. These values represent closely the ferri/ferrocyanide reaction on graphite, as discussed below. To achieve large values of WaI and WaII, i.e., uniform current distribution, Fig. 2 shows that the tubular FTE must be operated at a low average current density because WaI and WaII both depend inversely on iavg. At typical flow rates through the FTE, e.g., v0 = 10.6 cm s−1 used in Fig. 2, we observe that WaII is very large (WaII > 100 over the entire range of current densities shown) and that WaII ≫ WaI. This is a manifestation of the fact that convective transport through the tubular FTE considerably lowers its transport resistance compared to its typical ohmic resistance.
Figure 2. WaI and WaII calculated for a tubular FTE (using conditions listed in Table II) as a function of the average current density. Plot shows that WaII ≫ WaI and that large WaI (uniform current distribution) requires operation at low average current density. Sign convention used for this figure is that negative currents imply cathodic reaction, and positive currents imply anodic reaction.
Download figure:
Standard image High-resolution imageTable II. Parameters used in scaling analysis and analytical modeling.
Parameter | Value |
---|---|
Cb | 1 mM |
DII | 0.677 × 10–5 cm2 s−1 |
DIII | 0.726 × 10–5 cm2 s−1 |
d | 0.1 cm |
L | 0.45 cm |
n | 1 |
v0 | 10.6 cm s−1 |
αa | 0.5 |
κ | 0.109 s cm−1 |
Parameters represent typical values for ferri/ferrocyanide reaction on a graphite tubular FTE. Diffusion coefficients were taken from Ref. 29 and electrolyte conductivity was taken from Ref. 30. Quantifying current distribution non-uniformity via analytical modeling.
In this section, we perform analytical modeling to quantify the extent of current distribution non-uniformity as a function of WaI and WaII. The current distribution over the tubular FTE surface was modeled for the simplified axisymmetric geometry shown in Fig. 3a. An analytical expression for the secondary current distribution (small WaI, large WaII) for this geometry, assuming linearization of the kinetics near iavg, 22 was provided by Akolkar: 30

Here, m = WaI −0.5. When ohmic resistance is negligibly small (large WaI) but diffusion resistance is large (v0 = 0 providing small WaII), the current distribution obeys: 31

where μ is related to WaII as: μ·tanh(μ) = (αa·WaII)−1 for a one-electron transfer reaction (n = 1). The analytically modeled current profiles over the FTE surface at various WaI and WaII values are shown in Fig. 3b. In the absence of flow (v0 = 0 cm s−1) and at very low average current density, WaI is large (negligible ohmic resistance compared to activation resistance) but WaII is small (substantial diffusional mass transport resistance compared to activation resistance). This leads to significant non-uniformity in the current distribution (Fig. 3b) with less current able to penetrate deep into the FTE due to diffusion limitations in the absence of flow. The presence of flow through an FTE is thus of paramount importance in eliminating diffusion limitations and achieving large WaII as seen in Eq. 9. In the presence of flow through the tubular FTE (v0 = 10.6 cm s−1), WaI and WaII values decrease as iavg increases. Increase in iavg makes the current increasingly non-uniform (Fig. 3b). In all cases, WaII ≫ WaI due to the fact that mass transport resistance is nearly eliminated by the flow attributes of the FTE. Notably, the ratio is/iavg (which is maximum at the FTE entrance z/L = 0) is equal to or less than 1.05 for WaI > 6. We take is/iavg ≤ 1.05 to represent relatively uniform current distribution and thus the criterion WaII ≫ WaI > 6 becomes the key design criterion to ensure reasonably uniform current distribution across the tubular FTE.
Figure 3. (a) Schematic representation of the 1-D FTE geometry used for analytical modeling of the current distribution (Eqs. 11 and 12 in the text). (b) Current distribution across the tubular FTE surface at various v0 and iavg. Current distribution is uniform (is/iavg ≤ 1.05) at WaII ≫ WaI > 6.
Download figure:
Standard image High-resolution imageFTE operating conditions that yield reliable kinetics data
The above evaluation of current distribution using scaling analysis and analytical modeling provided key design criterion for tubular FTEs: WaII ≫ WaI > 6. Plots of WaI and WaII at various iavg, d, and L values are shown in Fig. 4. Analysis presented in Fig. 4 assumes a constant flow velocity (v0 = 10.6 cm s−1). To achieve large values of WaI and WaII, FTE must be operated at a small average current density (iavg) and must have a large diameter (d) or a small length (L). Figure 4 shows that, to achieve uniform current distribution, i.e., WaII ≫ WaI > 6, the FTE must be designed to have a diameter d ≥ 0.2 cm and be operated at an average current density iavg ≤ 0.23 mA cm−2 when L is fixed at 0.45 cm. Alternatively, the tubular FTE must have a length L ≤ 1 cm and be operated at iavg ≤ 0.02 mA cm−2 when d is fixed at 0.1 cm. In Fig. 4, conditions below the horizontal solid line (WaII = WaI = 6) should be avoided as these would lead to non-uniform current distribution.
Figure 4. Effect of FTE parameters (iavg, d, and L) on WaI and WaII: (a) Constant L, varying d and (b) Constant d, varying L. Thicker lines represent design regime where WaII ≫ WaI > 6.
Download figure:
Standard image High-resolution imageGuided by Fig. 4, we now investigate the ferri/ferrocyanide reaction kinetics on a graphite tubular FTE. We constructed a tubular FTE setup as described under "methods" with L = 0.45 cm and d = 0.1 cm. Figure 5a shows steady-state polarization curves measured on a graphite FTE at various equimolar concentrations of K3Fe(CN)6 and K2Fe(CN)6. A stable equilibrium potential (Eeq) was established at each [Fe(CN)6]4–/[Fe(CN)6]3– concentration (e.g., Eeq = 0.33 V vs Ag/AgCl at CII = CIII = 1 mM). At large overpotentials (Vapp—IRΩ—Eeq > 0.2 V), mass transport limitations are observed in Fig. 5a. Below the mass transport limit, the anodic voltammogram in the Tafel range can be described by:

Tafel plots (Fig. 5b) were constructed where the kinetic current density ik = ∣iavg [iL/(iL − iavg)]∣ was plotted vs Vapp—IRΩ—Eeq. For Tafel kinetics, semi-log plots of ik vs Vapp—IRΩ—Eeq show a region with linearity because:

In Fig. 5b, the data points chosen from the raw polarization plot (Fig. 5a) meet the design criterion: WaII >> WaI > 6. This ensures that non-uniform current distribution does not convolute Tafel analysis. As seen in Eq. 14 and its application to Fig. 5b, the Tafel slope provided the anodic charge transfer coefficient: αa = 0.58, and the Y-intercept at Vapp = Eeq provided the exchange current density: i0 = 0.046 mA cm−2 (at equimolar concentration of 0.25 mM). Similar analyses were performed on a graphite RDE: the raw polarization data (Fig. 5c) was screened for uniform current distribution, followed by Tafel analysis using Eq. 14. Analysis yielded αa = 0.56 and i0 = 0.052 mA cm−2 for equimolar concentration of 0.25 mM. Good agreement between the two methods is observed in Table III which compares the kinetics parameters αa and i0 determined using FTE and RDE over a range of concentrations. Note that, for the RDE (Fig. 5c), the conventional Wagner number takes the form: 25

Figure 5. (a) Steady-state polarization for the [Fe(CN)6]4–
[Fe(CN)6]3– redox reaction on a graphite tubular FTE at various equimolar concentrations of [Fe(CN)6]4– and [Fe(CN)6]3–. Filled data points meet the criterion WaII >> WaI > 6 and were selected for Tafel plots. (b) Tafel analysis of data selected from (a) leading to electrochemical kinetics parameters. The Tafel regime was selected such that i0 < iavg < 0.9iL. (c) and (d) represent similar analysis as (a) and (b) but using RDE at 500 rpm. Table III summarizes kinetics parameters i0 and αa determined using FTE and RDE.
Download figure:
Standard image High-resolution imageTable III. Kinetics parameters (αa, i0) at various concentrations (Cb) of [Fe(CN)6]4– and [Fe(CN)6]3– measured using FTE and RDE.
FTE | RDE | |||
---|---|---|---|---|
Cb (mM) | αa | i0 (mA/cm2) | αa | i0 (mA/cm2) |
1 | – | 0.195 | 0.50 | 0.208 |
0.5 | – | 0.082 | 0.52 | 0.100 |
0.25 | 0.58 | 0.046 | 0.56 | 0.052 |
0.1 | 0.55 | 0.021 | 0.60 | 0.025 |
αa was not available at Cb = 1 and 0.5 mM for the FTE due to unavailability of data points that satisfy WaI > 6 and lie within the Tafel regime. Therefore, i0 at Cb = 1 and 0.5 mM was determined in the "linear" regime (Vapp—IRΩ—Eeq < 0.04 V) where iavg = i0 ·nF(Vapp—IRΩ—Eeq)/RT.
From the dependence of the exchange current density on Cb (Table III), the standard rate constant can be determined: 21

Figure 6 shows a plot of i0 vs Cb and contains kinetics data acquired on the tubular FTE as well as the RDE following the design criterion (WaI > 6). From the slopes in Fig. 6, the standard rate constants (k0) were extracted: for FTE, k0 = 1.95 × 10–3 cm s−1, and for RDE, k0 = 2.14 × 10–3 cm s−1. While these kinetics constants are already in very good agreement (within 10%), the slightly higher apparent rate constant measured on the RDE may be attributed to its elevated roughness and thus higher electroactive surface area confirmed by Atomic Force Microscopy (discussed in detail in the Supporting Information available online at stacks.iop.org/JES/168/043505/mmedia). Our rate constant value is in the same general range as values reported previously. 32,33 The differing electrocatalytic properties of graphite electrodes due to oxygenation 34 and edge effects 35 result in a wide range of rate constant values. Therefore, consistent preparation and treatment of graphite electrodes is crucial in obtaining reproducible kinetics parameters.
Figure 6. Exchange current density (i0) as a function of equimolar concentrations of [Fe(CN)6]4– and [Fe(CN)6]3– for graphite tubular FTE (blue) and RDE (red). While FTE and RDE based kinetics are in reasonable agreement when using WaI > 6 as the FTE design criterion, significant error (14 − 24%) is introduced in i0 when applying a weaker design criterion of WaI > 2.
Download figure:
Standard image High-resolution imageTo demonstrate the importance of strict adherence to the design criterion (WaI > 6) when conducting kinetics analysis, we evaluated the error in i0 introduced when deviating from the design criterion. In Fig. 5, if WaI > 2 is followed as the selection criterion for Tafel analysis, we get i0 = 0.167 mA cm−2 at Cb = 1 mM and 0.067 mA cm−2 at Cb = 0.5 mM. As seen in Fig. 6, these values are 14%–24% lower than those for the correct criterion (WaI > 6, which ensures highly uniform current distribution). Analogous to well-established analysis of the disk electrode, 36 a mathematical treatment of the magnitude of error in kinetics measurement using FTE and its dependence on the FTE current distribution non-uniformity will be reported in a subsequent contribution.
Conclusions
Quantitative guidelines for the design and operation of a tubular FTE are developed. It is demonstrated that, an FTE with WaII ≫ WaI > 6 provides a uniform current distribution. This condition can be satisfied by choosing appropriate FTE dimensions (large d, small L), and by operating the FTE at low average current densities and high flow rates. The process for choosing the correct parameters for the FTE is summarized in Fig. 7. Such optimized FTE design and operation guarantees that its use as an electroanalytical tool leads to reliable electrochemical kinetics data. This is demonstrated for the [Fe(CN)6]4–
[Fe(CN)6]3– reaction over a graphite FTE, and results are compared rigorously to those obtained on a conventional RDE. Operation away from the design criterion (WaII ≫ WaI > 6) are shown to introduce significant errors.
Figure 7. Process flow chart for the design and operation of a tubular flow-through electrode for electrochemical kinetics studies.
Download figure:
Standard image High-resolution imageAcknowledgments
This work was performed under the auspices of the U.S. Department of Energy by Lawrence Livermore National Laboratory under Contract DE-AC52-07NA27344 and LDRD 20-ERD-056. lLNL-JRNL-819093.