Abstract
Conductivity and lithium-ion transference numbers are reported for physically gelled composite electrolytes using lithium hectorite clay as the charge carrier and carbonate solvents (ethylene carbonate, propylene carbonate, and dimethyl carbonate). Results are compared with those of typical lithium-ion battery electrolytes based on lithium hexafluorophosphate and carbonate solvents. Room-temperature conductivities of the composite electrolytes as high as
S/cm were measured. Because of the nature of the anionic clay particulates creating the gel structure, near-unity lithium-ion transference numbers are expected and were observed as high as 0.98, as measured by the dc polarization method using lithium-metal electrodes. Since the carbonates react with lithium and create mobile ionic species that significantly reduce the observed lithium-ion transference number, care must be taken to minimize or eliminate the presence of the reaction-formed ionic species. These hectorite-based composite systems are possible electrolytes for rechargeable lithium-ion batteries requiring high discharge rates. © 2002 The Electrochemical Society. All rights reserved.
Export citation and abstract BibTeX RIS
A viable electrolyte for lithium-ion batteries must meet a number of requirements, for example, high conductivity ( S/cm at 25°C), low electrode-electrolyte interfacial impedance, and high-potential stability window (
V). It is most often the electrolyte conductivity that receives the majority of attention in electrolyte characterization and design. While the conductivity is certainly an important property in determining the success of a particular electrolyte in a lithium-ion cell, the lithium-ion transference number is also an important property and in recent years has started to receive increased attention.1
2
3
4
5 A high lithium-ion transference number can significantly reduce the effects of concentration polarization, thus decreasing this potential loss in a cell. Theoretical work has shown that a transference number of unity can offset a decrease in conductivity by up to an order of magnitude, particularly under high discharge rates.6 Thus, a single-ion conducting electrolyte is particularly attractive for applications requiring high power such as electric vehicles.
Single-ion conducting polymer electrolytes intended for lithium batteries have been reported.7
8
9 For polyelectrolytes gelled with propylene carbonate,7 ethylene carbonate,8 and dimethyl sulfoxide,9 room-temperature conductivities have been reported in the S/cm range. However, lithium-ion transference numbers were either not reported7
8 or were low (
) and explained by reaction of the solvent with the lithium metal electrodes which created other mobile ionic species.9
We have developed single-ion conducting, physically gelled electrolytes based on lithium hectorite clay nanoparticulates dispersed in carbonate solvents suitable for lithium-ion cells. As we report in this communication, room-temperature conductivities of these electrolytes have been measured as high as S/cm and lithium-ion transference numbers have been observed as high as 0.98. With conductivities approximately an order of magnitude less than those of typical lithium-ion electrolytes, these electrolytes appear to be an attractive alternative for cells designed for high-discharge applications.
Hectorite and other 2:1 layered clays (smectites) are characterized by a negatively charged plate-like structure with exchangeable associated cations sandwiched between the plate-like layers,10 as illustrated in Fig. 1. It has long been known that the smectites can be dispersed in water to create physically gelled systems, sometimes described as a "house-of-cards" type of structure.10 We have also been able to create physically gelled systems by dispersing the hectorite in high-dielectric solvents based on ethylene carbonate (EC) and propylene carbonate (PC). With the relatively large anionic platelets (approximately 1 μm for natural clay down to 25 nm for synthetic clay) interacting with one another to create the gelled structure, the anionic platelets are essentially rendered immobile, which should result in cationic transference numbers approaching unity.
Figure 1. Pictorial representation of hectorite structure.
Other investigators have looked at the smectites as possible electrolyte components for lithium-based batteries. However, conductivities of both native-dry and organic-intercalated forms of the smectites are S/cm at room temperature,11
12 even when measurements are performed parallel to the orientation of the clay layers for MEEP intercalated sodium montmorillonite12 (MEEP = poly[bis(2-(2'-methoxyethoxy)ethoxy)phosphazene]). Conductivities exceeding
S/cm at 30°C have been observed for poly(ethylene) oxide (PEO)-intercalated lithium montmorillonite,13 while attempts at dispersing a synthetic hectorite (Laponite®) into PEO-based systems met with limited success with conductivities observed at best at
S/cm at 80°C.14 While each of these novel intercalated or dispersed systems has shown conductivity improvement over native dry clays, they are still orders of magnitude below that typically required for lithium or lithium-ion batteries operating at room temperature (
S/cm).
The focus of this work was the efficient dispersion of the lithium form of hectorite into high-dielectric organic solvents (carbonates) to create a physically gelled nanocomposite electrolyte. The high-dielectric carbonate medium solvates the lithium ions well enough that collapse of the dispersed hectorite is prevented by self-repulsion of the negatively charged platelets. We present an experimental study of conductivity and lithium-ion transference numbers for these electrolyte systems. Results are compared with measurements on typical -based electrolytes presently used in lithium-ion rechargeable batteries.
Experimental
Synthetic sodium hectorite was provided by Hoechst (SKS-21, 88 meq/100 g, approximately 250 nm avg size, clay platelet anionic charge is approximately 60,000). EC, PC, and dimethyl carbonate (DMC) were obtained from Aldrich and dried using 4 Å molecular sieves (Fisher Scientific). Lithium hexafluorophosphate () was obtained from Aldrich and vacuum dried at approximately 120°C for 24 h. Solvents and salts were stored in an argon-filled glove box. The water content of solvents and salts were measured to be below 30 ppm using a Mitsubishi CA-06/VA-06 Karl-Fisher titrator.
Sodium hectorite was first exchanged to the lithium form (Li Hectorite) according to the following procedure. Sodium hectorite (approximately 20 g) was mixed in deionized water (approximately 800 mL) using a blender. A lithium chloride (Fisher Scientific) solution (approximately 30 g LiCl in 200 mL deionized water) was then blended into the dispersion. The sodium hectorite/LiCl mixture was allowed to sit overnight, then centrifuged at 10,000 rpm for approximately 30 min to separate the hectorite from the solution. The exchange/separation process was repeated twice more using the hectorite obtained from the centrifugation step. After the third separation, the hectorite gel ( mL) was diluted with deionized water to 1000 mL and separated twice more. After the final separation the resulting lithium hectorite was dried at 100°C, then rinsed in a glass frit filter with 100 mL methanol to remove any remaining LiCl. A drop (
mL) of 1 M
was added to approximately 3 mL of the rinse solution to qualitatively test for the presence of Cl. The methanol rinse was performed until no AgCl precipitate was observed, or approximately three times total. The lithium hectorite was then dried at 80°C to remove the methanol.
Dispersion of the lithium hectorite into the carbonates was performed using water as a dispersing aid according to the following procedure. Approximately 8 g of the dried lithium hectorite was mixed using a Silverson high-shear mixer into a solution of 40 g deionized water g EC or PC. The majority of the water was removed by drying the resulting gel at 100°C in a conventional oven for approximately 2 days. The gel was then dried in a vacuum oven at 120°C for approximately 1 h. Care was taken not to remove too much of the carbonate, otherwise the hectorite particles would aggregate and require the addition of more water to redisperse. The maximum concentration appears to be approximately 50-60 wt % lithium hectorite before significant aggregation occurs. Qualitatively, significant aggregation occurs when the composite is no longer translucent. The concentrated hectorite/carbonate was transferred to an argon atmosphere glove box where it was diluted with dry (
ppm water) EC or PC to approximately 20-30 wt % hectorite and hand mixed well. The concentrate was transferred back to the vacuum oven and dried again, diluted with EC or PC, and vacuum dried once more (three vacuum drying cycles total). The resulting concentrate composition was approximately 50 wt % lithium hectorite with a water content of approximately 200-300 ppm. Composite electrolytes of various weight percent hectorite were made from the concentrated PC- or EC-based lithium hectorite composites. Equivalent lithium-ion molar concentrations are reported for these systems and are based on the total moles of exchangeable cations (complete exchange of
for
is assumed) per liter of solvent. All sample preparation and cell assembly was performed under an argon atmosphere.
Electrolyte conductivities were measured using two-electrode (0.64 mm platinum wire, Fisher Scientific) cells such as that shown in Fig. 2. The cell constants were found using a standard conductivity solution (Fisher Scientific) prior to and after each measurement. Conductivities were measured over the temperature range of to 100°C. Conductivity measurements were performed using standard ac impedance techniques with a Princeton Applied Research (PAR) 273 potentiostat and 5210 lock-in amplifier.
Figure 2. Schematic design of two-electrode (platinum wire) cell used for conductivity measurements.
Lithium-ion transference numbers were determined using the steady-state current method of Bruce and Vincent.15 Although noted in the literature to have limitations due to its assumption of dilute solution,16 this method was chosen due to its relative simplicity. The method consists of initial measurement of the lithium interfacial resistance (), application of a small voltage (
mV) until a steady current is obtained (
), and final measurement of the interfacial resistance (
). The lithium-ion transference number (
) is calculated using the following equation
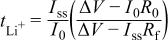
where is the initial current and is calculated from the voltage and the overall cell resistance by
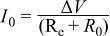
where is the electrolyte resistance.
Measurements were performed using a PAR 273 potentiostat/5210 lock-in amplifier or a BAS-Zahner IM6e impedance analyzer in conjunction with a PAR 173 potentiostat. Cells were constructed using either a stainless steel coin cell configuration (Fig. 3) or a Kel-F™ cylindrical cell configuration (Fig. 4). Both configurations used two lithium metal electrodes that were scraped with a scalpel to expose fresh lithium immediately prior to cell assembly. In the coin cells, Celgard™ 2400 (polypropylene, Hoechst Celanese) or Veratec PowerWeb™ (nonwoven polyolefin, Veratec) separators were used for liquid electrolytes, while polypropylene screen (approximately 500 mm thick/50% open volume, McMaster Carr) was used as a separator for the lithium hectorite-based composite electrolytes. While composite electrolytes of higher clay concentrations (approximately 40 wt % clay and greater) are strong enough to be used without a separator, the separator was used with all composite electrolytes to insure consistency among the various cells. The cylindrical cells used a small piece of Teflon tubing [approx. 0.75 cm i.d. and 1 cm length] as a means to reproducibly position the lithium electrodes. All transference-number cells were allowed to sit for 1 day to allow the lithium metal interface to stabilize to insure no significant change in the interfacial impedance during the course of the measurement and steady state could be achieved. A minimum of three measurements on each cell was performed, with 1 day at open circuit between measurements to allow the concentration polarization to relax.
Figure 3. Schematic design of coin cell used for lithium-ion transference number measurements. SS stainless steel. PP polypropylene.
Figure 4. Schematic design of cylindrical cell used for lithium-ion transference number measurements. SS stainless steel.
Examples of typical steady-state currents and initial and final lithium-electrolyte interfacial impedances obtained for standard electrolyte and composite electrolyte button cells are shown in Fig. 5. Electrolyte and interfacial impedance values were determined by fitting an equivalent circuit to the Nyquist plot. The circuit initially chosen consists of a resistor ( representing the electrolyte resistance) in series with a parallel combination of a resistor (
representing the lithium-electrolyte interfacial resistance and equivalent to either
or
) and a constant-phase element (Q). This circuit is designated on Fig. 5 by
). A constant-phase element was chosen rather than a capacitor to better account for the roughness of the lithium surface. If the fit is not adequate (as determined by visual quality), a second parallel combination of a resistor and a constant phase element are added in series to the original circuit to account for each lithium interface separately. This circuit is represented on Fig. 5 by
). In this case, the lithium-electrolyte interfacial resistance (
or
) is equal to
.
Figure 5. Example of measurements taken for transference number determination by the steady-state current method. Actual applied voltage was approximately 8.2 mV. Parameters obtained for transference number calculation: (a)
μA,
Ω,
Ω,
Ω
); (b)
μA,
Ω,
Ω,
Ω (
).
Results and Discussion
The ideal composite electrolyte is comprised of lithium hectorite dispersed as single platelets in a high-dielectric solvent, resulting from good solvation of the exchangeable lithium cations (Fig. 6). Mobility of the lithium cations results in a high conductivity, while the platelets create a mechanically stable gel structure, most likely through face-to-face electrostatic repulsion and edge-to-edge attraction. Due to their physical interactions, the platelets are essentially immobile, resulting in a near-unity lithium-ion transference number. Results that follow focus on experimentally measured conductivity and lithium-ion transference numbers for lithium hectorite-based composite electrolytes and comparisons with this ideal composite.
Figure 6. Pictorial representation of the desired lithium hectorite composite electrolyte structure. Drawing is not to scale.
Conductivity.—
Conductivities were measured for a variety of systems based on or lithium hectorite and carbonate solvents. Room-temperature conductivities at various lithium-ion molar concentrations are shown in Fig. 7. Room-temperature conductivities of
-based electrolytes are approximately
S/cm at concentrations of approximately 1 M, a typical concentration for commercial electrolytes. In comparison, maximum room-temperature conductivities of the lithium hectorite-based composite electrolytes are approximately
S/cm at a lithium-ion concentration of approximately 0.5 M or about 30 wt % clay.
Figure 7. Effect of lithium-ion concentration on the conductivity of lithium hectorite and -based electrolytes. Solvents: EC, PC, and DMC.
Room-temperature molar conductivity as a function of lithium-ion molar concentration is shown in Fig. 8. Molar conductivity for the -based electrolytes decreases with lithium-ion concentration. In comparison, the molar conductivity for the lithium hectorite-based composite electrolytes increases to a maximum at a lithium-ion concentration of approximately 0.2 M, then decreases with increasing concentration.
Figure 8. Effect of lithium-ion concentration on the molar conductivity of lithium hectorite and -based electrolytes. Solvents: EC, PC, and DMC.
Conductivity is determined by two factors: ion concentration and mobility. In typical high-dielectric liquid electrolytes for lithium-ion batteries, as salt concentration increases, so does conductivity. Electrolyte viscosity increases with salt concentration as well and begins to impede ion mobility. When the effect of electrolyte viscosity becomes the same order as that of increasing ion concentration, the rate of conductivity increase with ion concentration drops (e.g., Fig. 6, electrolytes). Plotting molar conductivity vs. concentration removes the effect of the number of ions on the conductivity, leaving only that of electrolyte viscosity, and molar conductivity is observed to decrease (e.g., Fig. 9,
electrolytes).
Figure 9. Effect of temperature on the conductivity of lithium hectorite and -based electrolytes. Solvents: EC, PC, and DMC.
With the lithium hectorite-based composite electrolytes, the conductivity increases with salt (clay) concentration; however, as the clay concentration increases, not only does the electrolyte viscosity increase, but so does the effective path required for lithium-ion movement due to the large size of the clay particles relative to the free lithium ions. Also, the likelihood of hectorite particle collapse increases as well, thus reducing the number of mobile lithium ions. As a result, conductivity of these composites then decreases. Molar conductivity of the hectorite composites also shows a maximum, in contrast to the molar conductivity of the electrolytes. We believe that the ion motion in these composites occurs primarily near the hectorite particles. At the low clay concentrations, an energy barrier exists for lithium-ion transfer between the hectorite platelets due to their separation. As the molar concentration increases, the energy barrier is reduced, forming more facile ionic pathways for the lithium ions and a maximum in the molar conductivity is observed. With further increase in molar concentration, the tortuosity of the lithium ion pathways increases, as well as the electrolyte viscosity, and molar conductivity decreases.
Low-dielectric solvents such as dimethyl carbonate can be added to the electrolytes to reduce the mixture viscosity and increase lithium-ion mobility, so long as the decrease in dielectric constant does not significantly impact salt solubility. The reduction in solvent dielectric constant is particularly critical for the Li hectorite-based composites. A reduction in the solvent dielectric constant shrinks the double layer surrounding the particles and the repulsive force between the particles is reduced. As van der Waals attractive forces become more significant, the probability of platelet collapse is increased, and the number of highly mobile lithium cations is reduced as lithium cations become trapped between the collapsed platelets.
The effect of temperature on the conductivities of the lithium hectorite- and -based electrolytes is similar, as seen in an Arrhenius plot (Fig. 9). Concentrations yielding the approximate maximum conductivities of each system are shown, i.e., 0.5 M for the lithium hectorite-based electrolytes and 1 M for the
-based electrolytes. Conductivities of the lithium hectorite-based composite electrolytes are approximately 30 times lower than those of molar-equivalent
concentration
electrolytes.
Water content of the electrolytes is of particular concern as it creates adverse reactions in a lithium-ion cell. It is desirable from the standpoint of the adverse reactions to reduce the water content of the composite electrolytes to below 50 ppm. It is not known, however, what role, if any, water plays in the solvation of the lithium cations in the composite and thus the conductivity. The effect of water content of the composite electrolytes on conductivity is shown in Fig. 10. There does not appear to be a significant effect of water content on conductivity from about 75 to 500 ppm. Thus, the parts per million level water content that exists in the composites should not play a significant role in the solvation of the lithium cations, nor is the conductivity observed due to proton conduction. It is anticipated that reduction in the water content below 50 ppm will not significantly affect the composite electrolyte conductivity.
Figure 10. Effect of water content on the conductivity of lithium hectorite based composite electrolytes. Solvent: PC.
At water concentrations ppm, the conductivity decreases, particularly at the lower temperatures. We hypothesize this is related to the hydrophobic nature of propylene carbonate. As the water content increases, the lithium ions become preferentially solvated by the water rather than the PC. As hydration shells form around the lithium ions, the hydrated ions begin to form small clusters. This "clustering" increases the effective mass of the lithium ion and reduces its mobility, and thus the conductivity decreases. At higher temperatures, the hydrated cations are more soluble in the PC and "cluster" to a lower extent, resulting in a reduced effect on the conductivity. However, as this phenomenon is only noticed in a single set of samples, further studies are required to confirm this hypothesis.
Lithium-ion transference number.—
The effect of lithium cation concentration on lithium-ion transference number for and Li hectorite-based electrolytes is shown in Fig. 11. Lithium-ion molar concentration was varied between 0.1 and 1.0 M and these data were collected in the coin cell. Each data point represents three measurements on a single coin cell over the course of 3 days. No specific trends in these consecutive-day measurements were observed. Lithium-ion transference numbers for the
-based electrolytes range between 0.2 and 0.3 and demonstrated a solvent dependency for the sample range investigated. In contrast, lithium-ion transference numbers for the Li hectorite composites increase with lithium-ion concentration to approximately 0.8 in PC and 1:1 (v:v) EC:PC. Li hectorite composites in a 2:1:1 (v:v:v) EC:PC:DMC show a lower lithium-ion transference number with the largest value of 0.65 measured at the highest lithium-ion concentration.
Figure 11. Effect of lithium-ion concentration on the lithium-ion transference number of lithium hectorite and -based electrolytes. Solvents: EC, PC, and DMC.
A few of the samples of the in 2:1:1 (v:v:v) EC:PC:DMC exhibited rather low (
) lithium-ion transference numbers. These samples also exhibited the highest lithium-electrolyte interfacial resistances. In fact, a general trend of decreasing lithium-ion transference number with increasing lithium- electrolyte interfacial resistance was noted in all of the data. The aforementioned trend appears stronger in the
-based electrolytes than in the Li hectorite-based electrolytes. Other researchers have noted a similar trend when measuring lithium-ion transference numbers using the steady-state current method.17 It is unclear at this point whether this is a real physical phenomenon or an artifact of the assumptions that go into the steady-state current method.
The effect of water content on lithium-ion transference numbers of the composite electrolytes is shown in Fig. 12. Above about 400 ppm concentration in button cell configurations, the lithium-ion transference number drops significantly to less than 0.3. It appears that at higher concentrations water reacts with the lithium electrodes to form non- mobile ionic species in the electrolyte. Aurbach et al. reported lithium-water reactions in a tetrahydrofuran solvent creating LiOH at the lithium metal interface, and in addition, evidence that a portion of the LiOH is solubilized.18
Figure 12. Effect of water content on the lithium-ion transference number of lithium hectorite-based composite electrolytes. Solvent: PC.
While the lithium-ion transference numbers for the Li hectorite-based composites are significantly higher than those of standard -based electrolytes, it was expected that they would be near unity. The platelet interactions in forming the gel structure were anticipated to render these anions immobile. In order for the lithium-ion transference numbers to be less than unity, there must be one or more other mobile ionic species present besides lithium. Considering the experimental setup for the transference number measurement, that is, a carbonate solvent in contact with lithium metal, it seems possible that a reaction could occur between the electrolyte and lithium to form other ionic species in the electrolyte. Reaction of the carbonates with lithium metal has been documented, with
R forming with cyclic carbonates (EC and PC) and
R and LiR (alkyl lithium) forming with linear carbonates (DMC).19
20
21 The question then is whether these ionic species are mobile enough and present at high enough concentrations to interfere with the transference-number measurement of the composite electrolytes. The following experiment was performed to address this issue.
Coin cells with two lithium electrodes were assembled that contained only the base solvents (i.e., no salt). Cells with each solvent were assembled both in the "dry" state, less than 30 ppm water, and "wet" state, with purposely added water between 100 and 150 ppm. In a case where the solvents contained no ionic species, the high-frequency impedance of the cells should be very high. However, all cells studied showed rather moderate impedance. The high-frequency impedance, representative of the bulk electrolyte resistance, was approximately 3-4 kΩ for most of the cells, indicating that mobile ionic species were created as a result of reactions between the solvent and the lithium metal. We would expect to see a high-frequency impedance greater than 50 MΩ for a pure carbonate solvent in this cell configuration.
Ionic conductivities resulting after reaction of the solvent with lithium were estimated (Fig. 13). To produce these estimates, a coin cell with two lithium electrodes and a standard electrolyte of 1 M in 1:1 (v:v) EC:DMC was assembled. The conductivity of the standard electrolyte was measured in a platinum wire conductivity cell (Fig. 2). The standard electrolyte conductivity was used with the high-frequency impedance from the standard electrolyte coin cell to calculate an approximate cell constant for the coin cell. The coin cell constant and the impedance measurements from the base solvent coin cells were then used to calculate conductivities for the "pure" solvents. In most cases, the conductivities are near
S/cm, similar to the conductivities of the composite electrolytes (measured in cells with platinum electrodes, Fig. 7). "Pure" carbonate conductivities measured in the platinum electrode cells are approximately
S/cm). Thus, the lithium-solvent reaction could have a significant impact on the composite electrolyte lithium-ion transference number measurements. The wet 2:1:1 EC:PC:DMC solvent was apparently the most reactive and exhibited a conductivity near
S/cm. It is composites with this solvent that show the lowest lithium-ion transference number.
Figure 13. Conductivity and lithium-ion transference number of pure solvents placed in contact with lithium metal. Water content of dry solvents is less than 30 ppm and that of wet solvents is approximately 150 ppm. Solvent ratio (v:v) in the EC:PC solvent is 1:1, while that in the EC:PC:DMC solvent is 2:1:1.
Lithium-ion transference numbers were also measured for these "pure-solvent" cells and are shown in Fig. 13. They are all close to 0.2-0.3. Based upon these data, calculations were performed to estimate what values to expect for the observed lithium-ion transference numbers of the Li hectorite-based composite electrolytes assuming that the actual lithium-ion transference numbers for the composite electrolytes are unity. Conductivities and lithium-ion transference numbers of the composite electrolytes and the pure solvent were used in these calculations, as were conductivities of the composite electrolytes. It is also assumed that the solvent/lithium metal reaction is independent of the presence of the Li hectorite. The following formula is derived from dilute solution theory22

where is the estimated lithium-ion transference number,
is the ideal lithium-ion transference number for the composite electrolyte (assumed to be 1),
is the pure solvent lithium-ion transference number,
is the composite electrolyte conductivity, and
is the pure solvent conductivity. These systems are obviously not at infinite dilution, but for these purposes this method is only being used to evaluate the likelihood that this apparent lithium-electrolyte side reaction is the cause of the nonunity lithium-ion transference numbers for the composites.
Results of the calculation are shown in Fig. 14. The calculated lithium-ion transference numbers (unfilled symbols) are less than the observed values (filled symbols). Thus, it appears likely that at least a significant portion of the nonunity transference number behavior observed for the lithium hectorite composites is a result of additional ionic species created by the solvent/lithium metal reaction.
Figure 14. Comparison of the (-•-,-▾-,-▪-) measured and (-○-,-▿-,-□-) expected lithium-ion transference number for lithium hectorite-based electrolytes. The expected values are calculated using the conductivity and lithium-ion transference number measurements of the pure wet solvents in contact with lithium metal, the conductivities of the lithium hectorite-based electrolytes, and assuming the actual lithium-ion transference number of these electrolytes is unity. Solvent ratios are in volume.
Efforts were made to minimize the effect of the lithium-solvent reaction on the lithium-ion transference number measurements of the composite electrolytes by using cylindrical transference number cells (Fig. 4) with a larger electrolyte volume. The volume is increased by approximately an order of magnitude so as to dilute the reaction-formed salt so that it would not as strongly influence the lithium-ion transference number measurement. The drawback of using a larger electrolyte volume cell is that significantly more sample is needed and the electrolyte polarization requires a significantly longer time to obtain a steady state (approximately 12 h vs. 45 min for the coin cells). With a lithium hectorite/PC electrolyte ( ppm
O), transference numbers are shown to increase to values as high as 0.9, as shown in Fig. 15, compared to previous highs in coin cell configurations around 0.8. The observed transference numbers increased, though still not to unity.
Figure 15. Effect of sample volume on the lithium-ion transference number of lithium hectorite-based electrolytes. Sample volumes in the coin cell and Kel-F™ cylindrical cell assemblies are approximately 0.03 and 0.75 mL, respectively. Solvent: PC.
In order to reduce the effect of water on the lithium-ion transference number measurements of the composites, the water content of the composites was reduced. Composite electrolytes were dried further and the effect of reduced water content on the composite electrolyte lithium-ion transference number measured in the large-volume cells is shown in Fig. 15. Transference numbers have been observed in these cases as high as 0.98, very close to the value of unity that is expected.
Conclusion
We have demonstrated electrolytes based on lithium hectorite and carbonate solvents that are attractive candidates for use in lithium-ion batteries. Dispersion of the hectorite and solvation of the lithium ions is critical to the performance of these electrolytes. Incomplete dispersion leads to poor mobility of the lithium ions as they become trapped between the clay platelets. With good dispersion of the hectorite, room-temperature conductivities have been observed as high as S/cm in these systems. Improved solvation of the lithium cations and subsequently higher conductivities might be achieved through high-dielectric or high-donor number solvent additives. Additives to lower the solvent viscosity may improve conductivity as long as the solvent dielectric constant is not lowered appreciably. In adding any component, it must be compatible with the electrodes under consideration for the full lithium-ion cell.
Lithium-ion transference numbers of these electrolytes are expected to be near unity. DC polarization or the steady-state current method was employed for these measurements, though side reactions of the electrolyte with the lithium metal electrodes appear to create additional ionic species that create problems with this technique. If the concentration and mobility of these reaction-formed species in the electrolyte is of a similar order of magnitude as that of the electrolyte, then the lithium-ion transference number measurement will be compromised. Such is the case with the lithium hectorite-based composite electrolytes. However, by increasing the electrolyte volume and reducing the water content, lithium-ion transference numbers can be observed close to unity at the expense of greater sample volume and longer experiment times.
Acknowledgments
This work is supported, in part, by the NCSU Hoechst-Kenan program and the Air Force Office of Scientific Research through the U.S. DoD National Defense Science and Engineering Fellowship Program. The authors also thank Barrie Davies for his ideas and insight and Timothy LeCroy for his assistance with the water-content conductivity measurements.
North Carolina State University assisted in meeting the publication costs of this article.