Abstract
The electroplating of Ni/W alloys in ammoniacal citrate baths was studied, in an effort to evaluate the mechanism of the synergetic effect of ions on the deposition of W, commonly referred to as induced codeposition. The extensive review of the field, published by Brenner in 1963, was summarized and updated. The partial current densities for deposition of Ni and W, as a function of the composition of the solution, the current density, and the rate of mass transport, were determined. There is strong evidence to support the hypothesis that the intermediate, which serves as the precursor for deposition of a Ni/W alloy, is a complex such as
where Cit is the triply deprotonated anion of citric acid. Analysis of the data indicates that the concentration of this intermediate is in the range of 2-4 mM. Most experiments where done at pH 8.0 and in the presence of a large excess of ammonium hydroxide. The concentration of W in the alloy declines sharply when the pH is increased to a value of 9.0. Alloys rich in W can be deposited in the absence of ammonia. The chemistry of the plating bath, in particular, the relative abundance of the different complexes of nickel and tungstate ions with citrate and
as a function of pH and the concentrations of the ligands, is discussed. © 2002 The Electrochemical Society.JES All rights reserved.
Export citation and abstract BibTeX RIS
Interest in electrodeposition of W alloys has increased in recent years, due to their excellent properties and potential applications. Tungsten alloys exhibit high corrosion resistance and good mechanical properties, which make them suitable for many engineering applications. Recently they have been deposited as barrier layers used for ultralarge-scale integrated and microelectromechanical systems applications.1 2
The term induced codeposition was first coined by Brenner3 to describe a situation in which a metal that cannot be electrodeposited alone from aqueous solution, is codeposited in the presence of another metal, forming an alloy. The best-known example for this kind of process is the formation of Ni/P and Co/P alloys during electroless deposition. Other documented cases induced the codeposition of W with Ni, Co, or Fe. Similar behavior was observed when tungsten was replaced by molybdenum or rhenium.4 5 6
It is widely known that W cannot be electrodeposited from an aqueous solution of sodium tungstate or any other soluble compound containing this element. Nevertheless, it is quite easy to deposit Ni/W alloys, if a suitable nickel compound, such as nickel sulfate, is added.
The role of Ni in assisting the deposition of W has not been explained so far. A great deal of work has been done, and several explanations for the induced codeposition phenomenon have been proposed in the past. However, no real experimental evidence was given to support the proposed mechanisms.
The central goal of the present study is to provide a satisfactory explanation for the mechanism of the induced codeposition of W in Ni/W alloys. Specifically, we have concentrated on the effect of the experimental variables on the composition of the alloy. The crystal structure and the surface morphology as a function of the concentration of tungsten in the alloy were also investigated, using several techniques, and will be reported in Part II of this series.
Electrodeposition of Tungsten Alloys
The electrodeposition of tungsten is of considerable interest because of the unusual properties of the metal. Of all the metals, tungsten possesses the highest melting point the lowest coefficient of linear thermal expansion
the highest tensile strength (4.0 MPa), the highest Young's modulus of elasticity (34 GPa), high thermal conductivity
and one of the highest densities of all metals
7
Because of this unusual combination of properties, tungsten has many engineering and industrial applications. For example, its good mechanical properties at high temperatures make it useful for filaments of incandescent lamps, for nozzles of rocket engines, and high-speed tool steels. The metal is also used for electrical contacts and for high temperature corrosion-resistant alloys.
Numerous attempts have been made to electrodeposit tungsten in its pure state from aqueous or organic solutions. A number of claims have been made for the deposition of tungsten from alkaline media, but none could be substantiated.8 9 10
The electrodeposition of tungsten alloys was first observed by Fink and Jones, while attempting to electrodeposit pure tungsten.11 It was shown later by Holt12 that the deposit obtained was an iron-tungsten alloy. The iron was present in the solution as an impurity.
Most important in the early development of W alloy plating is the ammoniacal plating bath, introduced first by Gol'tz and Kharlamov.9 10 This bath contained ammonium salts, ammonium hydroxide, and ions of the relevant metals. The plating was poor, because the deposits were porous and weak. The concentration of the relevant salts was limited by their solubility, and the current densities employed were too high.
The next stage in the development of tungsten alloy plating baths was the introduction of organic hydroxy acids, such as citrate, tartrate, and malate, into the ammoniacal baths.13 The organic hydroxy acids, together with itself a known complexing agent for the iron group metals, improved the faradaic efficiency and the solubility of the metal ions in the bath. In this way, smooth, hard, and thick deposits of the alloy could be deposited at lower current densities and at current efficiencies approaching 100%.
The deposition of tungsten alloys from alkaline baths has been studied and developed by several authors, in an attempt to find the optimum conditions to obtain high tungsten content and better quality of the alloy. A great deal of work has been done to explain the reasons that a metal that cannot be electrodeposited alone from aqueous solution, is readily codeposited in the presence of another metal, forming an alloy.
An extensive review, covering the literature up to 1963, was published by Brenner.3 This review summarizes the influence of the composition of the bath and the operating conditions on the properties of the alloys and their W content.
Understandably, the most important variable determining the tungsten content of the electrodeposited alloy is the concentration of tungstate ions in solution.14 It was shown that, as the concentration of tungstate increased, the tungsten content of the deposits increased and approached a limit, which was about 50 to 60 wt % (23 to 32 atom %) of tungsten for the Fe/W and Co/W alloys, and about 30 wt % (12 atom %) for the Ni/W alloy. Similar limiting values for the tungsten content were obtained in our work for Ni/W alloys deposited from ammoniacal citrate bath.15 However, in solutions without ammonia, we observed a very high tungsten content of 76 wt % (50 atom %) as the concentration of tungstate was increased.16 As the total metal concentration in the bath is increased, at constant metal ratio, the tungsten content in the alloy is increased, whereas the faradaic efficiency decreases.
The concentration of
or
is also of major importance. On one hand, W needs the synergetic effect of one of the above iron group metals. On the other hand, the iron group metals can be readily deposited in the absence
The present work shows that increasing the concentration of
may increase the rate of deposition of W, while decreasing the W content of the alloy, since the rate of deposition of Ni is increased even more. Thus, an optimum ratio of
that will give rise to the highest concentration of W in the alloy can, in general, be found for any composition of the other components of the plating bath.
The tungsten content of the alloy also depends on the type of the complexing agent used in the bath. Citrate baths yielded higher tungsten content than those containing tartrate or malate. However, a decrease in W content and in the faradaic efficiency with increasing concentration of citrate was reported.13
The current density applied effects the tungsten content in the alloy and the faradaic efficiency. In the case of Co/W, the tungsten content increased slightly with increasing current density and the current efficiency decreased.17 For Ni/W deposited from ammoniacal citrate bath, a significant increase of tungsten content was observed with increasing current density.14
Temperature is an important variable in the operation of the plating baths, not because of its effect on the tungsten content of the alloy, which is rather small, but due to its effect on the hardness of the alloy and on the current efficiency. Alloys deposited at room temperature were found to be weak with microscopic cracks. It was shown that the W content and the faradaic efficiency obtained from ammoniacal citrate baths increased with increasing temperature.18 The effect of temperature on the W content of alloys electrodeposited from ammonia-free baths was small.19 Note that these baths contained tartaric acid and boric acid. In ammonia-free solutions containing only citrate, used in our work, almost no effect of temperature on the tungsten content was observed, but the faradaic efficiency increased with temperature.15
Considerable attention has been given to the study of the relationship between the deposition potential and the current density, in an effort to understand the mechanism of the induced codeposition of tungsten with the iron group metals. In ammoniacal citrate solutions, the deposition potentials observed were positive, compared to those measured during deposition of the iron group metal itself.10 20 21 This observation refers to relatively high current densities and is not found in measurements at low current densities.
Despite the large number of studies of the current/potential relationship, new insight about the mechanism of induced codeposition was not gained. The interpretation of this phenomenon is complicated, as the deposition of the alloy involves the discharge of hydrogen as a side reaction, and since both Ni and W can be deposited along several parallel pathways.
Several theories were proposed to explain the mechanism of induced codeposition of tungsten, but none could be confirmed experimentally. The different mechanisms proposed in the past by several authors are discussed below.
Holt and Vaaler22 proposed in 1948 that alternative layers of W and Ni are deposited. When a layer of W has been deposited, further deposition of this metal is inhibited, but Ni can be formed on top of it. Once a fresh layer of nickel has been plated, a second layer of tungsten can be formed, and so on. The reason for this self-inhibition effect was not explained. In modern terminology, it would be the equivalent of assuming that W is deposited as an underpotential deposited (UPD) layer on Ni, hence deposition is terminated as soon as a complete monolayer of this metal has been formed, but the concept of UPD had not yet been introduced at the time. The difficulty of this explanation is that the X-ray diffraction (XRD) studies showed that a solid solution of W in Ni was formed, which is not consistent with the layered structure. Moreover, the concentration of W in the alloy was usually found to be below 20 atom %, at variance with the above model.
A somewhat similar mechanism was proposed by Davis and Gentry,23 who claimed that the potential for the deposition of W was shifted to more positive value in the presence of Ni, due to the energy of interaction between the metals in the alloy. This was based on the assumption that the deposition potential of tungsten was too negative to be attained in aqueous solutions. However, measurements of the heat of solution of the two metals showed that it was too small to explain the shift in potential.24
The failure of tungsten to be deposited by itself was attributed to a low overvoltage for the hydrogen evolution reaction on tungsten. It was considered that the rate of hydrogen evolution on tungsten was very high, while its rate on the Ni/W alloy was considerably lower, so deposition of tungsten alone could not be sustained. This hypothesis was discredited by Jackson et al. ,25 who showed that tungsten deposition did not occur on mercury, even though the rate of hydrogen evolution on this metal is very low.
Clark and Lietzke,26 assumed that an oxide of tungsten was formed first, and was subsequently reduced by hydrogen. The role of nickel would then be to catalyze the reduction of this oxide film. There was, however, no independent experimental evidence to support this hypothesis.
A different role was assumed for the tungsten oxide film by Nielsen and Holt.27 They proposed that this film could act as a semipermeable membrane, permitting only penetration of protons, followed by hydrogen evolution. In the presence of nickel ions, this oxide film would be disrupted, making it possible for tungstate ions to reach the cathode and be discharged. This hypothesis is not consistent with the fact that the oxide film is readily soluble in alkaline media.
A similar mechanism was suggested by Vasko,28 who assumed that nickel forms an adsorbed hydroxide layer on the electrode, which reacts with tungstate ions to form a mixed oxide. This mixed oxide is then reduced to yield the alloy. However, the existence of the adsorbed hydroxide or the mixed oxide during deposition was not demonstrated.
None of the hypotheses mentioned above can provide full details of the mechanism of deposition of tungsten alloys with the iron group metal, and no evidence was presented to determine which was the correct mechanism.
Several studies were published in recent years on tungsten alloy deposition, but the mechanism is still not understood. Most studies concentrated on increasing the tungsten content and improving the properties of the alloy, rather than on evaluating the mechanism of induced codeposition.
Donten and Stojek29 used pulse electroplating to increase the tungsten content in amorphous Co/W alloys. These alloys contained small amounts of boron or phosphorous. They showed that, if a symmetrical current pulse was used, the tungsten content in the alloys reached a maximum value of 41.4 atom %, which is higher than in the case of constant current deposition. When employing asymmetrical current wave of any form, the tungsten content in the alloy decreases. No mechanism was proposed to explain the effect of pulsing in general or that of the pulse shape on the composition of the alloy. Pulsed deposition was also found to improve the smoothness and the resistance to corrosion, and to reduced the brittleness of the alloy.
Isaev and Osteryoung30 studied the electrodeposition of Ni/W/B amorphous alloys plated from tungstate-rich solutions. Partial polarization curves were calculated for nickel and tungsten deposition. A limiting current was observed for both metals in solutions with 0.025 M and 0.234 M
In the case of nickel, the results indicated that nickel was reduced under diffusion control. The limiting current observed for deposition of W could not be attributed to diffusion limitation, because the concentration of tungstate was an order of magnitude higher than that of nickel. Furthermore, the composition of the alloy was found to be independent of potential. Therefore, it was assumed that a supersaturated solid solution was formed and the partial current for W deposition was due to the stoichiometric limitations imposed by the alloy.
Wikiel and Osteryoung31 used anodic stripping voltammetry for in situ monitoring of the concentration of cobalt ions during electrodeposition of Co/W alloys on platinum microdisk electrodes. This may turn out to be a useful way to monitor and control the composition in the plating bath.
A recent study of the mechanism of electrodeposition of Co/W alloy, using cyclic voltammetry, was reported by Aravinda et al.32 They suggested that deposition occurred from a cobalt-tungstate complex, while the deposition of occurred in two steps involving the
ion as an intermediate.
Recently, we proposed a mechanism that can explain the synergetic effect of on the deposition of W, forming an alloy.15
16 This mechanism is discussed in detail in the present paper and in Part II of this series.
Chemical and Electrochemical Properties of Tungstate
Chemical properties.—
The chemistry of tungstate in aqueous solution is rather complicated.33 When tungsten is dissolved in an aqueous alkaline solution, the resulting solution contains tetrahedral ions and simple tungstates, such as
can be crystallized from it. If this solution is acidified strongly, a white precipitate of tungstic acid is formed. At intermediate pH values, polymeric anions, made up almost invariably of
octahedra are formed. In aqueous solution, equilibration among the tungstate species is rather slow, and can take several weeks.
The predominant species produced by the progressive acidification of tungstate solutions at pH values below 6 are the paratungstates, which are followed, upon further acidification by metatungstates
Metal tungstates of the type can also be formed in solutions containing divalent cations. The solubility of these salts in water is rather low. Complexes of tungstate with anions of organic acids such as citrate,34 tartarate,35 and oxalate35 are well known. In the case of citrate, complexes in the form of
are formed, where Cit stands for the triply deprotonated anion of citric acid, and the number of protons,
, depends on the pH.
Electrochemical properties.—
The main reaction studied in this work is the reduction of tungstate ions to metallic W

The standard potential given in the Pourbaix diagrams36 for this reaction is

In a typical system used in the present work, at pH 8.0 and a tungstate concentration of 0.1 M, the reversible potential is

where SHE is standard hydrogen electrode and RHE is reversible hydrogen electrode. The reversible potential is not very sensitive to the concentration of tungstate. Thus, if the concentration of this ion is increased to 0.5 M, for example, the reversible potential will shift by less than 7 mV. It should be borne in mind, however, that Eq. 3 does not necessarily represent the reversible potential in the system studies here. In this case, both nickel and tungstate ions are complexed, and the stability constant of the ternary complex of the two metal ions with citrate, which we believe is the precursor for deposition of the W/Ni alloy, is not known.
Experimental
Solutions.—
Deposition of W/Ni alloys was studied using solutions that contain and
as the electroactive species.
was used as the complexing agent. Ammonium hydroxide and sulfuric acid were used to adjust the pH. No additives were used, to keep the system as simple as possible. The concentrations of the above species were varied over a wide range, to test the effect of each on the concentration of W, and on the crystal structure of the alloy.
Plating conditions.—
Experiments were conducted at A constant current density of
and a pH of 8 were used, except when their effects were being tested. Mass transport was controlled with a rotating cylinder working electrode The rotation rate was adjusted to ensure turbulent flow at the surface. It was maintained at 2,000 rpm, except when the effect of mass transport was tested. A one-compartment cell, deaerated with purified nitrogen, was used. The faradaic efficiency was calculated from the charge passed and the added weight.
Electrodes.—
A gold cylinder, 0.2 cm diam and 1 cm long, was used as the working electrode. A platinum wire counter electrode and Ag/AgCl/1 M KCl reference electrode were used.
Instrumentation.—
The composition of the alloy was measured using an energy dispersive spectroscopy (EDS) probe (Link Corp.) attached to a JOEL, model 6300 SEM. ZAF calculation was used to determine the composition. Each sample was measured at five different points along the cylinder, to confirm uniformity. The thickness of the deposit was large enough (more than 1 μm), so that no signal from the underlying gold substrate was observed. In some of the samples the composition was also confirmed by secondary ion mass spectrometry and by X-ray photoelectron spectroscopy measurements.
The current density was controlled with a Pine potentiostat model AFCBP. An analytical rotator (Pine instruments) was used to control the rotation rate of the cylindrical working electrode.
Results
The ammoniacal citrate bath for the deposition of Ni/W alloys was developed in the 1940s. It consists of an aqueous solution of and
to which
and
are added. The former is used as a complexing agent for both
and
and the latter is added to increase the faradaic efficiency of metal deposition. The pH of the solution is usually adjusted to 8.0, where the citrate ion exists almost exclusively in the form
and is a strong complexing agent for
It also forms a series of complexes with the
ion. The predominant complex at pH 8.0 is
The more protonated form,
is predominant in the range of pH of 5 to 7. The highly charged, nonprotonated complex
is not stable. Thus, at
the predominant species is the free tungstate ion.
The faradaic efficiency was calculated directly from the added weight following passage of a given charge. This was used to calculate the partial current density for hydrogen evolution. The partial current densities for the two metals were calculated using the composition of the alloy, as determined by EDS. It should be noted that, since the total current density applied was fixed by the external circuit, the sum of partial current densities for metal deposition is the difference between the total current density applied and the partial current density for hydrogen evolution. Thus, it is possible (as we have observed) that the partial current density for tungsten deposition would increase with increasing faradaic efficiency, while its concentration in the alloy would decrease. It should also be noted that, in the figures that follow, the partial current density was calculated taking into account that six electrons are needed to deposit one atom of W while only two are needed per Ni atom. Thus, the ratio of partial current densities, should be divided by three, in order to calculate the atom percent concentration of W in the alloy. Each sample was plated for several hours. The potential during plating is the steady state potential at a given set of experimental conditions.
The effect of solution composition on the W content of the alloy.—
In Fig. 1 we show the effect of the concentration of in solutions containing 0.10 M
and 0.40 M citrate. At equal molar concentrations of nickel and tungstate ions, the concentration of W in the alloy is quite low, about 5 atom %. As the concentration of nickel in solution is decreased, the W content of the alloy increases, but the faradaic efficiency decreases dramatically. The deposition potential changes in the positive direction (i.e., to lower overpotential for hydrogen evolution), in parallel with the increase in faradaic efficiency, as expected. Note that the partial current density for W deposition increases by a factor of about 10 with increasing concentration of nickel in solution, while its concentration in the alloy decreases by a factor of about 7.5 over the same range of Ni concentration. This lends very strong support to the reaction pathway suggested here and in Part II of this paper, claiming that W is deposited from a complex with Ni, while Ni itself can also be deposited in a parallel route, independent of the existence of tungstate in solution.
Figure 1. The effect of the concentration of Excess of
0.1 M
0.4 M
pH 8,
2000 rpm.
The effect of changing the concentration of tungstate ion in solution is shown in Fig. 2. The results of two sets of experiments are shown, in which the concentrations of were 0.1 and 0.2 M. The concentration of citrate was 0.60 M in both cases. The W content of the alloy initially increases sharply with increasing concentration of tungstate in the solution, but seems to level off at about 12 and 8 atom %, in 0.1 and 0.2 M
respectively. The faradaic efficiency seems to decrease first and then to increase with increasing tungstate concentration. The deposition potential changes in the positive direction over the whole range. The partial current for W deposition increases with the concentration of tungstate, which is to be expected. When the concentration of nickel in solution is doubled, the W content of the alloy decreases, but the partial current for W deposition increases, as seen in Fig. 2d, supporting the behavior shown in Fig. 1d and the conclusions derived from it. The faradaic efficiency is significantly higher and the plating potential is more positive in 0.2 M
solutions, except at very low tungstate concentrations.
Figure 2. The effect of the concentration of Excess of
0.6 M
pH 8,
2000 rpm. ••• 0.1 M
○○○ 0.2 M
The effect of changing the concentration of citrate, in a solution containing equal amounts of nickel and tungstate ions, is shown in Fig. 3. The W content of the alloy increases with increasing concentration of citrate up to 0.50 M, but then starts to decrease. The faradaic efficiency decreases dramatically when the concentration of citrate exceeds 0.20 M, i.e., when the molar concentration of citrate exceeds the sum of concentrations of nickel and tungstate ions. It levels off in the region of 0.50-0.80 M, and seems to increase a little above that. The deposition potential changes in the negative direction with increasing citrate concentration, first sharply, and then moderately. Note that the partial current density for the deposition of both metals decreases with increasing concentration of citrate. It can be concluded qualitatively that citrate, being a strong complexing agent for both metal ions in solution, tends to sequester these ions in stable complexes, making it more difficult to deposit the metal. The relative effect on Ni is apparently stronger up to a concentration of 0.50 M citrate and weaker at higher concentrations, leading to a maximum W content of the alloy at a citrate concentration of about 0.5 M.
Figure 3. The effect of the concentration of citrate. Excess of 0.1 M
0.1 M
pH 8,
2000 rpm.
Partial control by mass transport.—
The effect of mass transport can be examined in two ways: by varying the rotation rate at constant current density, or changing the current density at constant rotation rate. In Fig. 4, we show the effect of rotation rate on the deposition process. Two solutions were examined: one in which the ratio was 10 and the other in which it was 0.1, in order to determine which of the two ions is limiting the rate of W deposition in this system. The limiting current at a rotating cylinder electrode, operating in the region of turbulent flow, is given by

where is the radius of the rotating cylinder,
and
are the diffusion coefficient and bulk concentration of the electroactive species, respectively, ν is the kinematic viscosity, and ω is the angular velocity.
Figure 4. The effect of rotation rate. Excess of 0.4 M
pH 8,
2000 rpm. ••• 0.4 M
0.04 M
○○○ 0.04 M
0.4 M
For the systems used in our experiments, the lowest rotation rate ensuring turbulence is 2,000 rpm. At this rotation rate, the limiting current densities for 0.04 M and
are approximately 24 and
respectively, using
The corresponding limiting current densities at 5,000 rpm, (the highest value shown in Fig. 4) is 90% higher (since
), namely, 46 and
respectively. Note that plating was conducted at a current density of
in this set of experiments (compared to
in the previous sets), to enhance the effect of mass-transport limitation, if any.
The W content of the alloy is higher in the tungstate-rich solution, as expected, but its dependence on rotation rate is rather small in both solutions. The faradaic efficiency and the deposition potential are essentially constant in Ni-rich solutions. In tungstate-rich solutions, the faradaic efficiency increased somewhat with increasing rate of mass transport, and the deposition potential shifts in the positive direction.
The most important information obtained from this series of experiments is in the examination of the partial currents for the deposition of the two metals. The rate of W deposition increases with increasing rotation rate in both solutions by almost the same amount (about 40%). Increasing the concentration of tungstate by a factor of ten, leads to a moderate increase in the rate of deposition of this metal, by a factor of about two. The data for the tungstate-rich solution are particularly significant for the following reasons: (i) While the rate of mass transport is increased by a factor of 1.90 in the range of rotation rates shown, the partial current for W deposition increases by a factor of 1.4 in both solutions. (ii) In the solution containing 0.040 M tungstate the calculated limiting current densities are 72 and at 2,000 and 5,000 rpm, respectively. The corresponding values of
are 0.03 and 0.02, respectively. This is too small to justify a 40% increase in current density with increasing rotation rate, indicating that a different species (probably a ternary complex containing both
and
with citrate, as is discussed below) is determining the rate of reaction. (iii) The results for the tungstate-rich solution shown in Fig. 4d are even more striking. Here, the calculated values of
are 0.0057 and 0.0034, respectively, and one would not expect to observe any mass-transport limitation, based on the concentration of tungstate. If the species limiting mass transport for the deposition of W is the
ion in solution (which makes sense, considering that W cannot be deposited from a solution of tungstate in the absence of
ions), the calculated values of the ratio of
are ten times larger, namely, 0.057 and 0.034, respectively, enough to explain some dependence of the partial current of W deposition on the rotation rate, but not all of it.
The effect of rotation rate on the partial current for nickel deposition is very minor, as seen in Fig. 4e. It is also noted, however, that as the concentration of nickel in solution is increased tenfold, the partial current density for its deposition is only increased by a factor of about three. This behavior is addressed in detail in the Discussion section.
We now turn to describe the effect of current density, shown in Fig. 5. Comparison was made in the same two solutions as shown in Fig. 4, one rich in tungstate and the other rich in nickel. All measurements were taken at a rotation rate of 2,000 rpm. The W content of the alloy is found to decrease with increasing current density, which is consistent with the increase of the same quantity with increasing rotation rate, both showing some degree of mass transport limitation. For the Ni-rich solution, the dependence of W content in the alloy disappears above about consistent with an apparent plateau in the partial current density for W deposition. In the tungstate-rich solution, the concentration of W in the alloy is almost independent of current density.
Figure 5. The effect of the applied current density. Excess of 0.4 M
pH 8, 2000 rpm. ••• 0.4 M
0.04 M
○○○ 0.04 M
0.4 M
The potential is shifting in the cathodic direction with increasing current density, as expected. The potential is found to change by about 0.35 V over an increase of current density by a factor of 20. This could formally lead to an average Tafel slope of about 0.27 V. However, we do not think that one should attempt to interpret these results in terms of the apparent Tafel slopes observed, since the system is very complex and at least four reactions can occur in parallel, as is explained also in the Discussion section.
The effect of ammonia and of pH.—
Ammonia is commonly added to plating baths for W/Ni alloys, to increase the faradaic efficiency, and sometimes to fine-tune the pH of the solution to a desired value, usually around pH 8. However, it is well known that is a strong ligand for
and can form a series of complexes of the type
where
can assume several values in the range of 2-6. The role of ammonia as a complexing agent, in addition to citrate, was not considered until recently.15
16 In Fig. 6, we show the effect of the concentration of ammonia as the parameter in the electroplating on Ni/W alloys. In all experiments shown above, there was a large excess of ammonia in solution, of the order of 2 M, hence its effect of W/Ni deposition was studied in the same range.
Figure 6. The effect of concentration of ammonia. 0.1 M 0.5 M
0.6 M
pH 8,
2000 rpm.
The first observation is that adding ammonia decreases the concentration of W in the alloy dramatically, from about 30 atom % in the absence of ammonia to 20 atom % in a solution that is 0.3 M with respect to ammonia. Increasing the concentration of ammonia further had only a moderate effect, decreasing the W content of the alloy to about 15 atom % in 2 M solution of ammonia. In parallel, the faradaic efficiency is more than doubled in the particular solution composition shown in Fig. 6, and the deposition potential shifts in the positive direction. The partial current densities for both metals first increases and then decreases again with increasing concentration of ammonia.
There is no doubt that the marked effect of ammonia on the deposition of W/Ni alloys is associated with its ability to form complexes with This apparently reduces the concentration of the ternary Ni/W/citrate complex, which is the precursor for W deposition. It may also influence the rate of Ni deposition, as is discussed below.
Since eliminating ammonia from the plating bath allows the plating of W/Ni alloys having a high concentration of W, this system is of interest both from the fundamental and the practical point of view. The detailed behavior of plating baths from which ammonia has been eliminated will be discussed in detail in a following paper (Part II).
The ammoniacal citrate bath for deposition of W/Ni alloys is commonly operated at pH 8.0. It is well known in the literature, however, that increasing the pH can increase the faradaic efficiency. It was therefore of interest to determine the effect of pH on this process in more alkaline media. In Fig. 7, we show the effect of pH on deposition of W/Ni alloys, in the range of pH 8.0 to 12.0. The solutions chosen have equal amounts of nickel and tungstate ions, and an excess of citrate. A dramatic decrease of the W content of the alloy (from 9 to about 3 atom %) is detected as the pH is increased from 8.0 to 9.0. Beyond that, the decrease is moderate, reaching about 1 atom % at a pH of 12.0. The faradaic efficiency is indeed found to increase, from about 38% at pH 8.0 to 83% at a pH of 9.0, and approaches 100% at a pH of 12.0. The partial current density for W deposition declines almost linearly with increasing pH, while that for Ni rises sharply between 8.0 and 9.0 and moderately at higher pH values. It should be noted that this experiment was not performed under ideal conditions, since the pH was increased by adding Thus, the variation with pH of the measured parameters is also affected by the added ammonia. Nevertheless, the sharp changes between pH values of 8.0 and 9.0 must be attributed mainly to the effect of pH, since more
is needed to change the pH from, say 10.0 to 11.0 than from 8.0 to 9.0, yet the effect on the concentration of W in the alloy is much larger in the latter case.
Figure 7. The effect of the pH. 0.1 M 0.1 M
0.4 M
pH 8,
2000 rpm.
The effect of pH on the W content and other parameters of W/Ni alloy deposition in ammoniacal citrate plating baths can be explained on the basis of the equilibrium between
and
as is shown in the Discussion section below. We note, however, that a strong dependence on the pH is observed, even in solutions where ammonia has been excluded. This effect will be discussed in detail in our next paper on this subject.
Discussion
In our attempt to understand the mechanism of deposition of W/Ni alloys, in particular, the synergetic effect of Ni on the deposition of W, it is necessary first to understand the solution chemistry of this system. The ammoniacal citrate bath is quite complicated, in that many complex ions may coexist and can serve as the precursor for the deposition of Ni alone or a W/Ni alloy. In this section, we show the results of numerical calculations of the relative abundance of the different species, as a function of pH or of the concentration of the ligands in solution. A detailed description of the calculations used is given elsewhere.37 38
Complexes of citric acid.—
This acid has three active hydrogen atoms on acidic groups and a less active hydrogen on an alcohol group. The corresponding values are 2.96, 4.38, 5.68, and 10.82.35 The distribution of the different ions as a function of pH is shown in Fig. 8a. The important point is that in the range of pH 7-10, the predominant species is the ion with three negative charges,
which is assumed to be the main ligand in all the complexes discussed here. This anion forms two types of complexes with nickel,
and
The distribution of these species as a function of the concentration of citrate is shown in Fig. 8b. We note that the concentration of free
ions falls to very low values as soon as the concentration of citrate reaches that of
The
ion exists only in a fairly narrow range of citrate concentration. When the ratio of
about 95% of the nickel ions are in the highly charged complex
It should be mentioned that the nickel citrate complex
is partially deprotonated to form
The concentrations of these two complexes are equal over a range of concentrations of
hence the concentration of
in Fig. 8b reaches a maximum of only 50%.
Figure 8. (a) The dissociation of citric acid as a function of pH. (b) Concentration distribution of complexes as a function of citrate concentration. 0.1 M
pH 8. (c) Concentration distribution of
complexes
as a function of pH, at
In the shorthand form
the value of
represents the number of protons in the complex. (d) Concentration distribution of
complexes as a function of concentration of ammonia. 0.1 M
pH 8.
Citrate also forms a complex with the tungstate ion, in spite of the fact that they are both negatively charged.34 In the simplest case, this ion should have the formula This is, however, unstable. Several protonated forms of this complex are known, such as
where
can assume values of 1-3. The
values for the equilibria

are 10.21, 17.21, and 21.67 for 2, and 3, respectively. The distribution of different complexes as a function of pH is given in Fig. 8c. Note that around pH 8, the predominant species is the complex containing only one proton, which can be written as
for short. Since
is not protonated at this pH, as seen in Fig. 8a, it may be assumed that in
the hydrogen atom originates from the partially protonated
ion. The first dissociation constant of tungstic acid is not given in the literature, probably because the corresponding
value is low, and formation of polyions makes measurements difficult.
It is usual to ignore the effect of ammonia on the composition of the alloy, considering this compound only as a mild base, used to adjust the pH of the solution and to increase the faradaic efficiency of plating in this system. However, it is well known that which is at equilibrium with
in alkaline media, is a ligand, forming several complex ions with nickel, all having the composition
where
can assume values of 2-6. The
values for the equilibria of these complexes are 5.0, 6.82, 7.14, and 8.55 for
3, 4, and 6, respectively.35 The concentration of the different complexes, as a function of the concentration of
is shown in Fig. 8d. At the concentration of about 2 M used in the present work, the predominant species is
and the concentration of free
is close to zero. It is obvious that
will compete with citrate for nickel ions in solution.
The precursor for deposition of tungsten.—
In previous publications,15
16 we have shown that it is possible (in a citrate bath from which ammonia was excluded) to deposit a Ni/W alloy with equal amounts of the two metals. It is reasonable to assume that the precursor for the deposition of such an alloy is a ternary complex of the type The overall reduction of this complex requires eight electrons, and can be written as


The ternary complex shown in Eq. 6 could be formed in the reaction


Unfortunately, this complex has not been detected directly, either in solution or as an adsorbed species, even though its existence is strongly implied by the deposition of a 1:1 Ni/W alloy. The rate of the reaction shown in Eq. 7 is expected to be quite low, in view of the fact that it involves interaction between two negative ions, one of them highly charged, and requires the removal of a citrate ligand from one of the two complexes. This may also lead to a low concentration of the ternary complex at steady state, which is the reason that this complex has not yet been detected. The likelihood of a similar reaction involving the complex of nickel with two citrate ions is low, because it would be difficult to get two anions, each having a charge of
to interact with each other.
The fact that, under most circumstances, the concentration of Ni in the alloy is much higher than that of W, and in ammoniacal citrate baths an alloy with 50 atom % W cannot be obtained at all, is easily understood if we remember that there are parallel paths through which Ni can be deposited alone, from its complexes with citrate or with ammonia, as shown in Fig. 8b and d, respectively.
The effect of ammonia and of pH.—
The addition of ammonia causes a sharp decrease in the concentration of W in the alloy, as shown in Fig. 6. This is easy to understand, in terms of the model suggested above. Since competes with citrate as a ligand for nickel ions, the concentration of
will be lower in the presence of ammonia. This shifts the reaction shown in Eq. 7 to the left, decreasing the concentration of the ternary complex from which W is plated. On the other hand, the rate of deposition of Ni may not be decreased at all; since it can be deposited from its complexes with
as well as from its complexes with citrate. Indeed, we notice in Fig. 6 that the partial current for Ni deposition increases in the same region where the concentration of W in the alloy decreases drastically.
It should be pointed out that the addition of ammonia should decrease the concentration of W in the alloy for any mechanism, as long as there is a synergistic effect of in solution upon the deposition of W from aqueous
which is a well-established experimental fact. In the presence of
in solution, some of the
ions are sequestered in the form of one or more of the complexes shown in Fig. 8d, and less will be available to assist in the deposition of W, whatever the mechanism of that assistance may be. The model given above is a highly plausible model, but it may not be the only one that explains the behavior observed.
The effect of pH is shown in Fig. 7. A sharp decline of the concentration of W in the alloy, and a corresponding increase in the faradaic efficiency, are observed as the pH is increased from 8 to 9. The partial current density for deposition of W declines almost linearly, while that for Ni rises sharply between these two pH values. Thus, the sharp decline in W content of the alloy is caused by the combined effects of the decrease in the rate of deposition of W and the concurrent increase in the rate of deposition of Ni.
This change in the rate of deposition of W can be associated with the complexing of with
The
for the dissociation ammonium hydroxide, corresponding to the equilibrium

is 9.25. Hence, the concentration of equals that of
at pH 9.25. At a pH of 8, only 5.3% of the ammonium hydroxide remains undissociated, while at pH 9, this is increased to 36%. Thus, in a 2 M solution of
the concentration of undissociated ammonium hydroxide will increase from about 0.1 to 0.7 M as the pH is increased from 8 to 9. The ligand
is formed in the reaction

Hence, increasing the pH in this range has an effect similar to increasing the concentration of ammonium hydroxide from 0.1 to 0.7 M, thus decreasing the concentration of W in the alloy.
The effect of mass transport.—
The effect of rotation rate was studied in the range of 2,000 to 5,000 rpm, which represents a 90% increase in the rate of mass transport for a rotating cylinder electrode. The partial current densities for deposition of both W and Ni show a rather irregular behavior. In Fig. 4d, we noted that increasing the concentration of tungstate tenfold, from 0.04 to 0.4 M increases the current density by a factor of only two. The limiting current, calculated on the basis of the concentration of the ion in solution, is much higher than the partial currents for deposition of this metal (
1, cf. the Results section), so that one would not expect a 40% increase of the rate of deposition of W with the increase of the rate of mass transport, as found experimentally.
The explanation of these anomalous observations lies in the formation of the ternary complex shown in Eq. 7 above. The concentration of the ternary complex is low and its rate of formation is also expected to be low, because it entails the reaction between two negatively charged species, one having a high charge of From the dependence of the partial current density for W deposition shown in Fig. 4d, we can estimate the activation-controlled and the mass-transport-limited current densities, using the well-known relationship
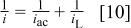
bearing in mind that the limiting current at a rotating cylinder electrode is proportional to
For solutions containing 0.04 and 0.4 M the values of the activation controlled current increases from 5.0 to
a ratio of only 1.8 for a tenfold increase in concentration. The corresponding limiting currents at 2,000 rpm increase in the same proportion, from 4.1 to
Moreover, we recall that the limiting current for deposition of W from a 0.04 M solution was calculated to be
Thus, the limiting current estimated from the data in Fig. 4d is only 5.7% of that calculated for a concentration of 0.04 M.
From the above value of the limiting current, it is possible to estimate the concentration of the electroactive species that is the precursor for deposition of W. Neglecting the difference in diffusion coefficients, this turns out to be 2.3 mM. This is the order of magnitude of the concentration of the ternary complex in a solution containing 0.04 M
0.4 M
0.4 M
and about 2.0 M
Considering that the diffusion coefficient of this ternary complex is probably smaller than that of the complex
the above concentrations of the ternary complex is probably a slight underestimate.
When the concentration of tungstate is increased tenfold and that of nickel is decreased by the same factor, both the activation controlled current density and the limiting current density increase by a factor of 1.8. It may be concluded that the concentration of the precursor for the deposition of W increased by the same factor, to a value of 4.1 mM. Considering only the equilibrium shown in Eq. 7, there would seem to be no reason for the concentration of the ternary complex to change. However, it must be remembered that ions are also in equilibrium with complexes containing
and the concentration of the nickel-citrate complex shown in Eq. 7 is not necessarily a linear function of the nominal concentration of
in solution.
The partial current density for Ni deposition was found to be essentially independent of the rotation rate. An anomaly was observed in this case too, since increasing the concentration of in solution by a factor of 10 causes an increase of partial current density only by a factor of 2-3, as seen in Fig. 4e. We recall, however, that the nickel ion can exist in solution in many different forms. There are four possible complexes with
and two complexes with citrate, in addition to the ternary complex with tungstate and citrate. The overpotential for deposition depends on the nature of each complex, and the relative abundance of the various complexes depends on the concentration of nickel. Hence, the resulting partial current density for Ni deposition may depend on concentration in a complex manner, which we shall not discuss here.
The plots of partial current density vs. the applied current density indicate a limiting current behavior for W deposition, as shown in Fig. 5d. For 0.04 and 0.4 M solutions of tungstate, the values of the limiting current densities observed are about 2.5 and respectively. These are in fairly good agreement with the values of 4.1 and
estimated above from the data given in Fig. 4d.
Conclusions
The electrodeposition of Ni/W alloys from an aqueous bath containing citrate and ammonia at room temperature has been studied. The chemistry of the bath has been discussed, and the influence of the pH, the concentration of the different species in solution, and the effect of current density and rotation rate were evaluated. In this bath, which contains an excess of ammonia, the concentration of W in the alloy is limited to about 12-15 atom %. When ammonia is removed, this concentration can be readily increased to the range of 35 atom %, at the cost of a decrease in faradaic efficiency, as shown elsewhere.15 16 The properties of citrate plating baths without ammonia will be discussed in detail in our next paper, Part II in this series.
Strong, albeit indirect, evidence points to the existence of a ternary complex of
and
which, we believe, is the precursor for the deposition of tungsten. The dependence of the partial current density for the deposition of W on the rotation rate and on the total current density applied allowed us to estimate the concentration of this precursor. Values of 2.3 and 4.1 mM where obtained for solutions containing 0.04 and 0.4 M tungstate, respectively. If the ternary complex was at equilibrium with its components (cf. Eq. 7), its steady-state concentration would not influence the limiting current for W deposition. However, the limiting current density calculated is much lower than that expected for the concentration of 0.04 M
used. This indicates that, either the formation of the ternary complex or its activation-controlled rate of discharge is the slow process, and may be the origin of the low values of the limiting currents observed.
Two sets of experiments were performed. In one, the ratio of concentrations of and
was 0.1 and in the other it was 10. Increasing the concentration of
by a factor of 10 increased the limiting current only by a factor of 1.8, showing that, of the above two ions in solution, the one that is the minority component controls the rate of the reaction. The activation controlled current density increased by the same factor. This is exactly the behavior one would expect when the limiting current is controlled by the steady-state concentration of a complex formed at relatively low concentration and at a low rate.
It should be noted that the existence of complexes containing more than one metal ion is not uncommon, and has been studied in detail in the literature.39 40 41
The heterogeneous rate constant can be calculated in two ways. If it is assumed that discharge of the ternary complex is the rate-determining step, one has

hence the first-order rate constant is If, on the other hand, it is assumed that formation of the ternary complex is the rate-determining step, the reaction will be quasi first order, since one of the reactants is in great excess. In this case one has

which can be rewritten as

where and
represent the concentrations of tungstate and the nickel ions. This leads to a value of
This low value of the quasi-first-order rate constant is consistent with the mechanism proposed above, where it was postulated that a complex containing nickel and tungstate ions, stabilized by citrate (and possibly one or two protons) is the precursor for deposition of a Ni/W alloy. The relatively low values of the concentration of this complex may be the reason that it has not yet been detected directly.
The effect of adding ammonium hydroxide to the plating bath was interpreted in terms of the competition of the corresponding ligand, with citrate for nickel ions in solution. The nickel-citrate complex is the one participating in the formation of the ternary complex, which is the precursor for the deposition of W. Consequently, the partial current for W deposition decreases with increasing concentration of ammonia.
The effect of increasing the pH on the composition of the alloy was related indirectly to the presence of Thus, for a nominal concentration of 2 M
the concentration of this species in solution increases from about 0.1 to 0.7 M, as the pH is changed from 8.0 to 9.0, and the concentration of free
changes accordingly. It should be noted, however, that a pH-dependence was also observed in a citrate plating bath in the absence of ammonia. This is related to the different degrees of protonation of the citrate-tungstate complexes, and will be discussed in detail in our next paper (Part II).
Acknowledgments
The authors wish to thank Professor E. Kirowa-Eisner and Professor V. Tsionsky for useful discussions and advice. Thanks are due to the staff of the Wolfson Center for Materials Research for performing the elemental analysis of the alloys. Financial support for this work by the Ministry of Science, Culture and Sports is gratefully acknowledged.