Abstract
Ionization chamber dosimetry is predominantly used for determination of the absorbed dose to water in 60Co and high-energy radiotherapy photon beams. The most widespread ionization chambers employed for absolute or reference dose determinations in reference conditions are the Farmer-type cylindrical ionization chambers. The Farmer-type ionization chambers have a variety of constructions and materials and their responses vary in the radiation beam. Clinical accelerators, in addition to conventional photon beams with flattening-filter, can also deliver flattening-filter-free (FFF) photon beams. The responses of five different Farmer-type cylindrical ionization chambers were experimentally examined with reference to absorbed dose determination in reference conditions when using the International Atomic Energy Agency (IAEA) - American Association of Physicists in Medicine (AAPM) Technical Reports Series no. 483 (TRS-483) and the IAEA TRS-398 dosimetry protocol in the present investigation. The irradiations were performed using 60Co and megavoltage photon beams with 6 MV, 15 MV, 6 MV FFF and 10 MV FFF nominal photon energies. The chamber calibrations were performed at different Secondary Standard Dosimetry Laboratories and are traceable to primary standards at different Primary Standard Dosimetry Laboratories. The chambers were also cross-calibrated at our laboratory using 60Co γ-beam. The variation found in the data regarding the reference dose determination using the various Farmer-type chambers in the photon beams employed was about 1% at maximum. Thus, the selection of the ionization chamber in reference dose determinations may affect the outcomes. The differences in the absorbed dose values were similar in the conventional as well as in the FFF photon beams. For the FFF photon beams the absorbed dose computations were performed using the IAEA-AAPM TRS-483 dosimetry protocol. Two of the ionization chambers used had identical construction but different central electrodes, i.e. graphite versus aluminium. The results obtained using these two chambers show that, in the photon beams examined, the employed correction for the central electrode (pcel) regarding these two chambers is associated with an inaccuracy which is larger than the calculated uncertainty for this correction. The outcomes found in the present experimental investigation using the various ionization chambers also indicate possible inaccuracy in the employed beam quality correction factors (kQ) and imply the need for a revision of these factors.
Export citation and abstract BibTeX RIS

Original content from this work may be used under the terms of the Creative Commons Attribution 4.0 licence. Any further distribution of this work must maintain attribution to the author(s) and the title of the work, journal citation and DOI.
1. Introduction
Among other methods, ionization chamber dosimetry can be used for the determination of absorbed dose to water. Farmer-type cylindrical ionization chambers are frequently employed, in reference conditions, for absolute or reference dose determination in 60Co and high-energy radiotherapy photon beams. The absorbed dose determinations are performed using dosimetry protocols, i.e. International Atomic Energy Agency (IAEA) Technical Reports Series no. 398 (TRS-398) (Andreo et al 2000), the American Association of Physicists in Medicine (AAPM) Task Group 51 (TG-51) (Almond et al 1999) and IAEA-AAPM TRS-483 (Palmans et al 2017). The currently available Farmer-type cylindrical ionization chambers have a variety of constructions and materials and their responses vary in the radiation beam. The presence of ionization chambers in the radiation beam is associated with perturbation effects on the photon and electron fluence and the absorbed dose determination is carried out using different perturbation correction factors (px). These correction factors are the displacement (pdis), cavity (pcav), wall (pwall) and central electrode (pcel) correction factor. In the AAPM notation, the pdis and pcav correction factors correspond to the gradient (pgr) and the fluence (pfl) correction factor, respectively. In the kQ-based dosimetry formalism the various perturbation correction factors are integrated in the kQ,Q0 beam quality correction factor. For a given beam quality (Q) the kQ,Q0 factor may differ for different ionization chambers due to differences in chamber construction and materials. The determinations of the absorbed dose to water are made employing the chamber specific absorbed dose to water calibration factor, ND,w,Q0, where Q0 is usually a 60Co reference beam. This calibration factor is traceable to a primary standard at a Primary Standard Dosimetry Laboratory (PSDL) and can be provided via factory- or Secondary Standard Dosimetry Laboratory (SSDL) calibration. For a given chamber the ND,w,Q0 calibration factor can also be determined using a 60Co γ-beam at the user's site by cross-calibration against a calibrated reference chamber (Andreo et al 2000).
Linear accelerators can deliver high-energy flattening-filter-free (FFF) photon beams. Using dosimetry protocols (TRS-398, TG-51 and TRS-483), the kQ,Q0 correction factor for a given megavoltage (MV) photon beam is calculated using the beam specific quality index, i.e. TPR20/10 and %dd(10)x. In the determination of the absorbed dose to water with air-filled cylindrical ionization chambers, the mass stopping power ratio for water relative to air (s/ρ)w,air is employed (i.e. included in the kQ,Q0 correction). It has been reported by others that the spectral distribution of the photon and electron fluence in FFF photon beams at the point of interest in a phantom differs compared with conventional photon beams with the same beam quality index (TPR20/10 or %dd(10)x) and consequently the mass stopping power ratio, (s/ρ)w,air, is different between the FFF and the conventional beams. The kQ,Q0 correction factor is dependent on the (s/ρ)w,air and the difference in the spectral distribution for the FFF and the conventional beams thus influences the determination of the absorbed dose to water, i.e. producing a deviation between 0.4%–1% for these beams (Xiong and Rogers 2008, Dalaryd et al 2014). In the theoretical studies presented by Xiong and Rogers (2008) and Dalaryd et al (2014) Monte Carlo simulations were used. Recently de Prez et al (2018) showed that the differences in the beam quality correction factors (kQ) between conventional and FFF photon beams are small and are within the uncertainties of the kQ determinations. An investigation by Lye et al (2016) has been made to examine the determination of the absorbed dose to water using the TRS-398 and TG-51 dosimetry protocols with FFF beams to monitor any differences between these two dosimetry protocols with regard to the possible change in the kQ,Q0 correction. In this study by Lye et al (2016), no difference was found in absorbed dose determination when using the TRS-398 and TG-51 dosimetry protocols with FFF beams.
Recently, IAEA and AAPM introduced a new dosimetry protocol, TRS-483, for determination of absorbed dose to water in small (non-reference) as well as FFF beams (Palmans et al 2017).
The aim of the present investigation was to experimentally study the response of Farmer-type cylindrical ionization chambers with a variety of constructions and materials, in reference conditions, in a 60Co γ-beam and high-energy radiotherapy photon beams including FFF beams. It is also of interest to experimentally examine the effects on the determination of the absorbed dose to water in FFF beams when using the new dosimetry protocol (TRS-483) with different ionization chambers.
2. Materials and methods
Six Farmer-type air-filled cylindrical ionization chambers were included in the present investigation. This was done to study the chambers responses to the determination of the absorbed dose to water in an inter-chamber comparison. Two chambers were of the NE2571 type (manufactured by Nuclear Enterprises, U.K.). Two other chambers were of PTW30011 and PTW30012 type with central electrode made of graphite and aluminium, respectively (manufactured by Physikalisch-Technische Werkstätten, Germany). In other words, these two PTW chambers had identical construction but different central electrode. The remaining chambers were: one ExradinA12 (manufactured by Standard Imaging, USA) and one IBA FC65-G (manufactured by IBA Dosimetry, Germany). The two NE2571 chambers were equipped with BNC and banana connectors and the four other chamber types had TNC-triaxial connectors. The different types of ionization chambers have different air cavity diameter and length. Except for the ExradinA12 and IBA FC65-G chambers, the chambers were not waterproof and were placed in a waterproof PMMA sleeve during the measurements in the water phantom. The geometrical centre of the chambers air cavity was defined as the point of measurement throughout the investigation. The physical parameters of the chambers employed in the present investigation are summarized in table 1.
Table 1. Physical and operating parameters of the ionization chambers used in the present investigation.
Ionization chamber | Cavity diameter (mm) | Cavity length (mm) | Wall material | Central electrode diameter (mm) | Central electrode material | Bias voltage (V) |
---|---|---|---|---|---|---|
ExradinA12a | 6.1 | 24.06 | Plasticb | 1.0 | Plasticb | +400c |
IBA FC65-Ga | 6.2 | 23.1 | Graphite | 1.0 | Aluminium | +300c |
NE2571 | 6.3 | 24.1 | Graphite | 1.0 | Aluminium | +400c |
PTW30011 | 6.1 | 23 | Graphite | 1.0 | Graphite | +400c |
PTW30012 | 6.1 | 23 | Graphite | 1.1 | Aluminium | +400c |
aWaterproof. bC552 Shonka air-equivalent plastic. cPositive potential is connected to the central electrode collecting negative charge.
The Farmer-type chambers used in the present study were calibrated at different SSDLs. The ND,w,Q0 calibration factor for the two NE2571 chambers is traceable to a primary standard at the BIPM (Bureau International des Poids et Mesures, France). For the PTW chambers as well as for the IBA chamber, this calibration factor is traceable to PTB (Physikalisch-Technische Bundesanstalt, Braunschweig, Germany). For the ExradinA12 chamber, the ND,w,Q0 calibration factor is traceable to NIST (National Institute of Standards and Technology, Gaithersburg, MD, USA). Thus, three primary standards at three different PSDLs take part in the present investigation. Note, the degree of equivalence with reference to the absorbed dose to water standards may differ between the various PSDLs. The difference in this respect is −0.06% for the NIST compared with the BIPM. The corresponding difference for the PTB is larger, −0.39% (Kessler et al 2013). In other words, the calibration of the ionization chambers (i.e. the PTW chambers and the IBA chamber) with traceability to the primary standard at the PTB may have an intrinsic difference of about 0.3%–0.4% compared with the calibrations of the other chambers used. Consequently, this can affect the outcomes in the comparisons of the absorbed dose to water obtained using the different chambers.
The chambers used were also cross-calibrated against one of the NE2571 chamber in the 60Co γ-beam at our laboratory. For this reference chamber, the notation RefNE2571 will be used. To reduce the uncertainties, these calibrations were performed twice. The chambers were removed and set up again for each series of measurements. The set-up of the water phantom and the collimation of the 60Co γ-beam were unchanged during these two cross-calibration sequences. The sequences were performed on different days. The calibration factor was calculated as mean from these two cross-calibration sequences.
A teletherapy unit (TEM Mobaltron, U.K.) with a 60Co-source was used in this study. All the measurements were performed using the reference field, i.e. a source-surface distance (SSD) of 800 mm, a 100 × 100 mm2 field size at the phantom surface and a depth of 50 mm in water. The TPR20/10 value in water was 0.574. At the time of the measurements with the Farmer-type chambers for the inter-chamber comparison of the chamber responses and also the cross-calibration of the chambers the dose rate using the reference geometry was 0.21 Gy min−1.
A Varian TrueBeam linear accelerator (Varian Medical Systems, USA) with 6 MV, 15 MV, 6 MV FFF and 10 MV FFF nominal photon energies was utilized throughout this investigation. These MV photon beams had quality indices (TPR20/10) of 0.669, 0.762, 0.632 and 0.705, respectively. The determination of the TPR20/10 values was performed using one of the Farmer-type chambers employed in the present investigation. The reference irradiation geometry consisted of a 100 × 100 mm2 field size, a depth of 100 mm in water and a source-surface distance of 900 mm.
An in-house water phantom (200 × 200 × 200 mm3) was utilized during all the irradiations. This was done to reduce the positioning uncertainties of the ionization chambers. The chambers were fixed at the same depth in water in each irradiation series. The 60Co γ-beam and the MV photon beam irradiations were performed using horizontal beams. Thus, the gantry angle was 90° in the MV photon beam irradiations. The front wall of the water phantom consisted of a 5 mm layer of PMMA with a 1 mm thick 100 × 100 mm2 PMMA window.
The ionization chambers were biased (high-voltage) and submerged in the water phantom for 60 min prior to the measurements and were pre-irradiated with 5 Gy. Every measurement consisted of 120 monitor units (approximately 1 Gy at a depth of 100 mm) in the MV photon beams and 5 min (approximately 1 Gy at a depth of 50 mm) in the 60Co γ-beam. For each series of measurements, ten consecutive measurements were performed from which the mean value of the charge was computed.
A SuperMax reference-class electrometer from Standard Imaging was used to measure the charge. A chamber specific polarity and normal bias voltage was used (table 1) in the 60Co γ-beam as well as in the MV photon beam measurements, i.e. positive polarity on the central electrode of the chamber collecting negative charge. The charge (M), expressed in nC, was corrected for the influence quantities, i.e. leakage current, ion recombination, temperature and pressure. The ion recombination correction was determined for the MV photon beams using the two-voltage method (Andreo et al 2000). The bias voltage ratio was 4 in all cases except for the IBA chamber; in this case the bias voltage ratio was 3. For the 60Co γ-beam measurements the factor 1 was used for ion recombination. For the determination of the MV photon beams quality indices (TPR20/10) the ion recombination correction was determined at both depths of interest, i.e. 200 mm and 100 mm, as recommended by Palmans et al (2017).
The notation SSDLND,w,Q0 and CrossND,w,Q0 will be used for the SSDL calibration and the cross-calibration, respectively. The determination of the absorbed dose to water in the MV photon beams were performed using both these two calibration factors, i.e. SSDLND,w,Q0 and CrossND,w,Q0. For the determination of the absorbed dose in the 60Co γ-beam, the SSDLND,w,Q0 factor was utilized. The MV photon beam measurements using the various ionization chambers were performed on the same day. The set-up of the water phantom, as well as the accelerator settings (i.e. gantry-, collimator angle and jaw setting), was fixed or unchanged during these measurements. In the in-house water phantom, at the depth of measurement and at a distance of 40 mm from the beam axis in the transverse plane, an external monitor chamber, connected to a Fluke-35040 reference-class electrometer (Fluke Biomedical, USA), was positioned to monitor any drift in the accelerator output. The chamber signal from this external monitor chamber was employed to correct for the variation in the accelerator output.
The coefficient of variation, CV, expressed as a percentage, was utilized to describe the variation in the values of charge obtained with the different chambers in each measurement series in the 60Co γ-beam as well as the MV photon beams. The CV is defined as the ratio of the standard deviation to the mean. The long-term stability (expressed as the CV) of the ionization chambers used in this study were checked using the 60Co γ-beam to monitor any drift in the chamber response.
The absorbed dose to water is defined as the dose at a point. The lateral absorbed dose profile on the central axis of the beam at the depth of interest is peaked for the 6 MV FFF and the 10 MV FFF beam. Thus, when using the Farmer-type chambers there is an erroneous or incomplete measurement of the charge, with reference to the point dose determination, due to volume averaging effect (McEwen et al 2014). In other words, correction must be made to correct for volume averaging effect in FFF beams (Palmans et al 2017, de Prez et al 2018). In the TRS-483 dosimetry protocol correction for volume averaging effect is included in the chamber specific beam quality correction factor (kQ).
For a given beam quality (Q) the formula for determination of the absorbed dose to water (Dw) using air-filled cylindrical ionization chambers in reference conditions can be written (Andreo et al 2000, Palmans et al 2017):

where: MQ is the mean charge corrected for influence quantities and disintegration of the 60Co-source or the variation in the accelerator output.
The kQ,Q0 correction factor can be computed (Andreo et al 2000):
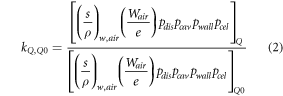
where: (Wair/e) is the average energy required to form an ion pair in air. It is assumed that the values of Wair/e are identical for the beam quality Q and Q0.
The pdis perturbation correction factor for air-filled cylindrical ionization chambers is calculated in dosimetry protocols by using the radius of the air cavity of the chamber (Andreo et al 2000). The difference in the radius of the air cavity of the chambers used in the present investigation is small (table 1). Consequently, the pdis correction factor is approximately the same for these chambers.
The pdis perturbation corrections employed in dosimetry protocols (Andreo et al 2000) are larger than those reported by Wang and Rogers (2009) and Swanpalmer and Johansson (2012). However, these differences will have little effect on the absorbed dose values.
As mentioned above, the PTW chambers had identical construction but a different central electrode, graphite (C) and aluminium (Al). Assuming that pcel = 1 for the PTW chamber with the central electrode made of graphite in the 60Co γ-beam as well as the MV photon beams (Andreo et al 2000) the ratio Alpcel,MV/Alpcel,Co can be written for the PTW chamber with the central electrode made of aluminium by using equations (1) and (2) above:

where: MV is representing megavoltage photon beams.
This ratio can be calculated using the SSDLND,w,Q0 as well as the CrossND,w,Q0 calibration factors. In dosimetry protocols for Farmer-type cylindrical ionization chambers with the central electrode made of aluminium (diameter of 1 mm) the pcel = 0.993 is used for 60Co γ-beams. The corresponding pcel is assumed to vary linearly between 0.9925 and 0.9957 for MV photon beam qualities (TPR20,10) of 0.58 and 0.80, respectively (Andreo et al 2000). Thus, a comparison of the ratio Alpcel,MV/Alpcel,Co for each MV photon beam quality employed in the present study can be made by computing this ratio via: (1) using the SSDLND,w,Q0 factors, (2) using the CrossND,w,Q0 factors and (3) using the data given by Andreo et al (2000), i.e. pcel between 0.9925 and 0.9957 for the MV photon beams and 0.993 for the 60Co γ-beam.
3. Results
The values of the ion recombination correction (at a depth of 100 mm) for the different chambers and MV photon beam qualities used are given in table 2 together with the dose per radiation pulse and the number of radiation pulses during 120 monitor units corresponding to 1 Gy absorbed dose to water. The ion recombination correction is dependent on the dose per radiation pulse, the higher the dose per radiation pulse the higher the ion recombination correction. Thus, a larger number of radiation pulses for 1 Gy gives a lower dose per radiation pulse and a lower ion recombination correction (and vice versa). This effect is seen in table 2 where the ion recombination correction decreased for all the chambers in the MV photon beams as a function of decreasing dose per radiation pulse, i.e. as expected. The same ion recombination corrections are obtained for the ExradinA12 and the PTW30011 chamber. These chambers had the central electrode made of plastic and graphite, respectively, and were biased with the same high-voltage (table 1). The smallest corrections were obtained for the PTW30012 chamber. The results also show that the largest corrections were found for the IBA FC65-G and the NE2571 chamber. The IBA FC65-G chamber was biased with +300 V (table 1).
Table 2. The ion recombination corrections (at a depth of 100 mm) in the MV photon beams together with the dose per radiation pulse and the number of radiation pulses during 120 monitor units.
Beam parameter and ionization chamber | 6 MV | 15 MV | 6 MV FFF | 10 MV FFF |
---|---|---|---|---|
Dose/pulse (mGy) | 0.23 | 0.47 | 0.60 | 1.10 |
Number of pulses | 4292 | 2147 | 1679 | 910 |
ExradinA12 | 1.003 | 1.005 | 1.006 | 1.010 |
IBA FC65-G | 1.004 | 1.007 | 1.009 | 1.015 |
NE2571 | 1.004 | 1.007 | 1.008 | 1.014 |
PTW30011 | 1.003 | 1.005 | 1.006 | 1.010 |
PTW30012 | 1.002 | 1.004 | 1.005 | 1.009 |
The SSDLND,w,Q0 and CrossND,w,Q0 calibration factors used for the absorbed dose determinations in the photon beams employed in this study are given in table 3. For the SSDLND,w,Q0 calibrations, performed at different SSDLs, the traceability to primary standards are included. The difference between the SSDLND,w,Q0 and CrossND,w,Q0 calibration factors is also included in table 3. Relatively good agreement is found between the SSDLND,w,Q0 and CrossND,w,Q0 factors for the ExradinA12, NE2571 and the PTW30012 chamber, this difference is 0.1% at maximum. For the IBA FC65-G and PTW30011 chamber this difference is within 0.5%. As described above, the cross-calibration of the chambers was performed twice and the CrossND,w,Q0 factor was calculated as mean from these two cross-calibration sequences. The difference in these two CrossND,w,Q0 factors was less than 0.2% for all the chambers used. It should be remembered, that the SSDLND,w,Q0 calibration factor for the PTW chambers and the IBA chamber has traceability to PTB. The primary standard at the PTB may differ somewhat from the standards at the BIPM and NIST as described above.
Table 3. SSDLND,w,Q0 and CrossND,w,Q0 calibration factors for determination of absorbed dose to water. The difference between the SSDLND,w,Q0 and CrossND,w,Q0 factors, expressed as a percentage, is included.
Ionization chamber | SSDLND,w,Q0 (mGy nC−1) | CrossND,w,Q0 (mGy nC−1) | Diff. (SSDL/Cross) (%) |
---|---|---|---|
ExradinA12 | 47.74 ± 1.19 (NIST) | 47.73 ± 0.57 | 0.02 |
IBA FC65-G | 47.89 ± 1.05 (PTB) | 48.12 ± 0.58 | −0.48 |
NE2571 | 45.41 ± 0.45 (BIPM) | 45.43 ± 0.55 | −0.04 |
PTW30011 | 53.91 ± 0.59 (PTB) | 54.11 ± 0.65 | −0.37 |
PTW30012 | 52.86 ± 0.58 (PTB) | 52.92 ± 0.64 | −0.11 |
The beam quality correction factors (kQ,Q0) used for determination of the absorbed dose to water using the various ionization chambers in the MV photon beams are given in table 4. The SSDL calibrations (SSDLND,w,Q0) of the ionization chambers utilized in the present work were made in 60Co γ-beams. The spectral distribution of these 60Co γ-beams could be slightly different from each other and also from the beam used at our laboratory. However, it is assumed that the effect of this is negligible and thus kQ,Q0 = 1 for the 60Co γ-beam measurements.
Table 4. Beam quality correction factors (kQ,Q0) used in the absorbed dose determinations for the various ionization chambers and MV photon beams.
Ionization chamber | 6 MVa | 15 MVb | 6 MV FFFa | 10 MV FFFa |
---|---|---|---|---|
ExradinA12 | 0.995 | 0.980 | 0.998 | 0.993 |
IBA FC65-G | 0.996 | 0.980 | 0.997 | 0.992 |
NE2571 | 0.993 | 0.981 | 0.996 | 0.992 |
PTW30011 | 0.992 | 0.979 | 0.995 | 0.990 |
PTW30012 | 0.994 | 0.982 | 0.997 | 0.992 |
The results of the absorbed dose determinations in the 60Co γ-beam using the Farmer-type chambers with the SSDLND,w,Q0 calibration factor are shown in figure 1. The error bars represent the uncertainty of the absorbed dose determination. This uncertainty was calculated using the estimations of the uncertainties specified by Andreo et al (2000) for 60Co γ-beams with the exception of the uncertainty for the ND,w calibration factor. For this quantity the uncertainty given in table 3 for the SSDLND,w,Q0 factor was used. As can be seen from figure 1, the absorbed dose values found with the ExradinA12, PTW30012 and the two NE2571 chambers are very close to each other. The difference in the results for these four chambers is less than 0.15%. The spread in the data for all the chambers used is within 0.5%. As also seen from figure 1, each of the results obtained using the Farmer-type chambers is within the uncertainties of all of the other results.
Figure 1. Results of the absorbed dose determinations in the 60Co γ-beam using the Farmer-type chambers with the SSDLND,w,Q0 calibration factor. The error bars represent the uncertainty of the absorbed dose determination for each chamber used.
Download figure:
Standard image High-resolution imageThe outcomes of the Dw determinations in the MV photon beams using the Farmer-type chambers with the SSDLND,w,Q0 as well as the CrossND,w,Q0 calibration factors are shown in figure 2. The error bars represent the uncertainty of the absorbed dose determination. Similarly as for the 60Co γ-beam (figure 1), the uncertainty of the Dw was calculated using the estimations of the uncertainties given by Andreo et al (2000) for high-energy photon beams. The uncertainty of the ND,w factor in these estimations was replaced with the uncertainty of the SSDLND,w,Q0 and CrossND,w,Q0 calibration factors (table 3) when computing the error bars. The spread in the data for the chambers included in this study is within 1% (absorbed dose determination with both the SSDLND,w,Q0 and CrossND,w,Q0 calibration factors). In a comparison using the results obtained with the SSDLND,w,Q0 as well as the CrossND,w,Q0 calibration factors, the smallest difference in Dw is found between the PTW30012 and the NE2571 chambers. This deviation is 0.1% at maximum in each MV photon beam. As can be seen from figure 2, the Dw determinations with the SSDLND,w,Q0 and the CrossND,w,Q0 calibration factors are close to each other (0.1% difference at maximum) for the ExradinA12, PTW30012 and the NE2571 chambers reflecting the small difference between the SSDLND,w,Q0 and the CrossND,w,Q0 calibration factors for these chambers, as shown above (table 3).
Figure 2. Values of the Dw determinations in the MV photon beams using the Farmer-type chambers with the SSDLND,w,Q0 and the CrossND,w,Q0 calibration factors. The error bars represent the uncertainty of the absorbed dose determinations for each chamber and each calibration used.
Download figure:
Standard image High-resolution imageFigure 2 also shows that the Dw obtained with the PTW30011 chamber is systematically lower than the Dw for the PTW30012 chamber in each MV photon beam examined. The difference is between 0.5% and 0.7%. When using the CrossND,w,Q0 calibration factor for the Dw determination this difference ranges from 0.2% to 0.4%. The lower the photon beam quality index (TPR20/10) the higher the difference between the Dw's for these two chambers. This relation or ratio CDw/AlDw is presented in figure 3 as a function of photon beam quality (TPR20/10). The error bars included represent the uncertainty of the ratio CDw/AlDw. It is assumed that the uncertainty of the products CMQ CND,w,Q0 and AlMQ AlND,w,Q0 in the absorbed dose determinations (cf equation (3)) with the PTW30011 and PTW30012 chambers is the same. Thus, the uncertainty of the ratio CDw/AlDw is given by the uncertainty of the Alpcel,MV/Alpcel,Co ratio. As can be seen in figure 3, the ratio CDw/AlDw has a linear dependency on the TPR20/10 (the linear correlation coefficient (R) is 0.990), (cf figure 4), and its values are lower than 1. This dependency or difference in the CDw and AlDw determination may be explained to some extent by inaccuracy or uncertainty in the Alpcel,MV, Alpcel,Co, Cpcel,MV and Cpcel,Co correction factors. Note, the beam quality correction factor, kQ,Q0, for the PTW chambers differs by 0.2%–0.3% in the photon beams used (table 4). Also a lower value of Dw is obtained using the PTW30011 chamber compared with the Dw for the PTW30012 chamber in the 60Co γ-beam (figure 1). This difference is 0.25%, thus comparable with the differences obtained in the MV photon beams. It should be remembered, that the Dw determination in the 60Co γ-beam was performed using the SSDLND,w,Q0 calibration factor.
Figure 3. Ratio of CDw/AlDw shown as a function of the photon beam quality (TPR20/10) of the MV photon beams examined using the PTW chambers with the CrossND,w,Q0 calibration factors. The linear correlation coefficient (R) is included.
Download figure:
Standard image High-resolution imageFigure 4. Ratios of Alpcel,MV/Alpcel,Co computed using the SSDLND,w,Q0 and the CrossND,w,Q0 calibration factors for the PTW chambers together with the data given by Andreo et al (2000). The dashed and the dotted lines represent the relation for the FFF and the conventional beams, respectively.
Download figure:
Standard image High-resolution imageThe comparison of the ratio Alpcel,MV/Alpcel,Co, calculated using equation (3) with the SSDLND,w,Q0 and the CrossND,w,Q0 calibration factors for the PTW chambers as well as the data given by Andreo et al (2000), is presented in figure 4. The error bars correspond to the uncertainty of the ratio Alpcel,MV/Alpcel,Co presented by Andreo et al (2000). The results (Alpcel,MV/Alpcel,Co) are given as a function of TPR20/10 representing the different MV photon beams used. This ratio computed by using the CrossND,w,Q0 calibration factors is systematically about 0.25% lower for the MV photon beams employed than the data given by Andreo et al (2000). The corresponding difference for the SSDLND,w,Q0 calculations is about 0.5%. As can be seen from figure 4, the difference in the Alpcel,MV/Alpcel,Co ratio for the conventional beams as well as for the FFF beams is about 0.1% in both the present investigation and in the data presented by Andreo et al (2000). The ratio Alpcel,MV/Alpcel,Co shows a linear dependency on the photon beam quality (TPR20/10), as described above.
The coefficient of variation for the ten consecutive measurements in each measurement series using the Farmer-type chambers as well as the external monitor chamber in the photon beams utilized was less than 0.14%. When using the external monitor chamber, the difference between the mean values of charge obtained in the measurement series was less than 0.06% in each MV photon beam showing excellent stability in accelerator output. The long-term stability (during two years), determined via constancy checks using the 60Co γ-beam, of the Farmer-type chambers used was less than 0.2%, expressed as the CV. The corresponding long-term stability (during one year) of the external monitor chamber was less than 0.1%. In other words, the long-term stability was almost perfect for all of the ionization chambers employed in the present investigation. The uncertainty associated with the determination of the absorbed dose to water in the 60Co γ-beam using the Farmer-type chambers with the SSDLND,w,Q0 calibration factor is estimated to be between 1.3% and 2.6% (figure 1). In the MV photon beams this uncertainty is estimated to be 1.7%–2.9% and 1.9% when using the SSDLND,w,Q0 and the CrossND,w,Q0 calibration factor, respectively (figure 2). The uncertainty regarding the MV photon beam determinations, with the CrossND,w,Q0 calibration factor, includes the uncertainty of the cross-calibration procedure of the Farmer-type chambers against the reference chamber RefNE2571 (Andreo et al 2000).
4. Discussion
Ionization chamber dosimetry is used for calibration of radiotherapy beams. In the present investigation the relation between the Dw found using various Farmer-type air-filled cylindrical ionization chambers in 60Co and high-energy radiotherapy photon beams (including conventional as well as FFF beams) was studied. The purpose of this investigation was to experimentally examine the responses of five different Farmer-type ionization chambers, with regard to absorbed dose determination in reference conditions and compare the outcomes in an inter-chamber comparison when using the dosimetry protocol TRS-483/TRS-398.
Relatively good agreement was found between the SSDLND,w,Q0 and the CrossND,w,Q0 calibration factors. The difference ranged between 0% and 0.5% (table 3). Good consistency was also found regarding the determination of the ion recombination correction (table 2). The correction factors obtained for the different chambers show the dependency of ion recombination on the dose per pulse in the pulsed radiation, as expected. The spread or variation in the individual measurement series of the charge in water and also in the data from the constancy checks (long-term stability determinations) of the ionization chambers used were relatively low, less than 0.2% expressed as CV. The difference between the two cross-calibration sequences (determination of the CrossND,w,Q0 factor) was less than 0.2% for all the chambers employed. Thus, these relatively low uncertainties and good agreements/consistencies associated with the ionization chambers utilized strengthen the accuracy of the outcomes found in the present investigation.
For the 60Co γ-beam the difference found between the results obtained employing the ionization chambers with the SSDLND,w,Q0 factors is 0.5% at maximum. Among the chambers used in the 60Co γ-beam, the closest values to each other concerning the absorbed dose determination (with the SSDLND,w,Q0 factor) were found for the ExradinA12, PTW30012 and the two NE2571 chambers. The spread in the data for these chambers is within 0.15%. The mean value of the absorbed dose obtained using the ExradinA12, PTW30012 and the two NE2571 chambers in the 60Co γ-beam compared with the results found with the other two chambers (i.e. IBA FC65-G and PTW30011) is on average 0.4% higher. As shown in table 3, the ratio between the SSDLND,w,Q0 and CrossND,w,Q0 factors for the IBA FC65-G and PTW30011 chambers is on average 0.996. Thus, there is a 0.4% difference between these calibration factors and this difference is the same as the average difference of 0.4% found in the absorbed dose in comparison with the ExradinA12, PTW30012 and the two NE2571 measurements. Consequently, it may indicate that the SSDLND,w,Q0 calibration factor for the IBA FC65-G and PTW30011 chambers are associated with an inaccuracy of about 0.4%. Accordingly, this inaccuracy will influence the absorbed dose determinations in the MV photon beams. For the PTW30011 chamber this 0.4% will also influence the comparisons with the PTW30012 chamber when using the SSDLND,w,Q0 calibration factor.
Regarding the MV photon beams examined, the results found in the absorbed dose determinations, computed using the SSDLND,w,Q0 and CrossND,w,Q0 calibration factors, show that the variation or spread in the data is within 1% in each MV photon beam. The closest values to each other in the absorbed dose determination are obtained for the PTW30012 and the NE2571 chamber. The difference found using these chambers is within 0.1% in each MV photon beam examined. The relatively good agreement seen in the 60Co γ-beam between the ExradinA12 and the PTW30012/NE2571 chambers is not present in any of the MV photon beams used. In other words, in contrast to the 60Co γ-beam determination in the MV photon beams the absorbed dose values obtained with the ExradinA12 chamber are lower than those found with the PTW30012/NE2571 chambers. On average, this difference is 0.6%. Note, the ExradinA12 chamber differs mostly compared with the other chamber used in this study with reference to the chamber construction and materials, i.e. chamber wall and central electrode made of plastic (pcel = 1). The difference in the kQ,Q0 corrections for the ExradinA12, PTW30012 and the NE2571 chamber is 0.1%–0.2% (table 4). Thus, the differences found in the absorbed dose determination in the MV beams, 0.6% (ranges between 0.4% and 0.8%), using the ExradinA12 and the PTW30012/NE2571 chambers suggest that the kQ,Q0 corrections for either the ExradinA12 or the PTW30012/NE2571 chambers may be associated with inaccuracy. Note, for the MV photon beams the uncertainty in the kQ correction presented by Andreo et al (2000) and Palmans et al (2017) is 1%. It should be remembered, that the SSDLND,w,Q0 calibration factors were used in the 60Co γ-beam determinations. However, the difference between the SSDLND,w,Q0 and CrossND,w,Q0 factor for the ExradinA12 chamber as well as for the PTW30012 and the NE2571 chamber is very small (0.1% at maximum) (table 3). It is assumed that the uncertainties associated with the absorbed dose determinations, including the cross-calibration procedure of the chambers, etc, are equal for all the chambers studied in the present investigation. The IBA FC65-G chamber has similar construction and materials to the PTW30012 and the NE2571 chamber, i.e. the chamber wall made of graphite and the central electrode made of aluminium (table 1). Note, the IBA FC65-G chamber is waterproof. When using the CrossND,w,Q0 calibration factor for the determination of the absorbed dose to water in the 6 MV, 15 MV, 6 MVFFF and 10 MVFFF beams the differences found between the absorbed dose values obtained with the IBA FC65-G and the PTW30012/NE2571 chambers are about 0.4%, 0.1%, 0.2% and 0.2%, respectively. The corresponding differences in the kQ,Q0 correction are 0.25%, 0.15%, 0.05% and 0%, respectively (table 4). As shown in figure 2, in each MV photon beam the highest and the lowest Dw values, computed using the CrossND,w,Q0 calibration factor, are obtained with the IBA FC65-G and the ExradinA12 chamber, respectively. The kQ,Q0 corrections for the IBA FC65-G and the ExradinA12 chamber are very close to each other, on average the difference is less than 0.1% (table 4). In contrast to this, the difference found in the Dw determination in the MV photon beams using these two chambers ranges between 0.7% and 0.9%. Thus, the possible inaccuracy in the kQ,Q0 corrections, described above, for the Farmer-type chambers used in this investigation is emphasized. In addition, in the relatively new TRS-483 dosimetry protocol (Palmans et al 2017) 'old' correction factors are adopted from the TRS-398 dosimetry protocol (Andreo et al 2000). The results obtained in the present experimental investigation imply the need for a revision of these correction factors.
As also shown in figure 2, the differences found in absorbed dose determination using the various Farmer-type chambers were similar in the conventional as well as in the FFF photon beams. Thus, the type of photon beam did not significantly influence the distribution of the absorbed dose values obtained with the different chambers. Note, the calculation of the absorbed dose values for the FFF beams was performed using the TRS-483 dosimetry protocol.
The PTW30011 and PTW30012 chambers had identical construction but had different central electrodes, graphite and aluminium, respectively. The beam quality correction factor (kQ,Q0) for these chambers differs by 0.2%–0.3% in the photon beams examined, i.e. larger corrections for the PTW30011 chamber, (table 4). In the kQ,Q0 correction the central electrode correction factor (pcel) is included (equation (2)). Thus, for these chambers the difference of 0.2%–0.3% in the kQ,Q0 correction is due to the difference in the pcel correction factor. Note, the uncertainty of the pcel correction given by Andreo et al (2000) is 0.1%. Assuming that the uncertainties in the influence quantities, i.e. leakage current, ion recombination, etc, are the same for these two chambers, the measurements of the charge should result in the same absorbed dose determination. However, the results found with the PTW30011 chamber are lower than those using the PTW30012 chamber in each photon beam examined. Regarding the MV photon beam measurements using the CrossND,w,Q0 calibration factor, this difference ranges between about 0.2% and 0.4% and shows a linear dependency on the photon beam quality (TPR20/10), (figure 3). Accordingly, this difference and dependency imply that the correction factors, pcel,Co and pcel,MV, for the PTW30011 and/or the PTW30012 chamber are associated with inaccuracy. In each MV photon beam, lower Dw determinations (with the CrossND,w,Q0 factor) are found using the PTW30011 and the ExradinA12 chamber compared to the Dw obtained with the other chambers employed in the present study (figure 2). For these other chambers the central electrode material is aluminium whereas for the PTW30011 and the ExradinA12 chamber the central electrode is made of graphite and plastic, respectively. The central electrode correction factor for the PTW30011 and the ExradinA12 chamber is unity (pcel = 1) in both 60Co γ-beam and MV photon beams (Andreo et al 2000).
When using ionization chamber dosimetry for reference dose determinations in megavoltage photon beams the kQ,Q0 correction is implemented in the dosimetry formalism. In the kQ,Q0 correction, the mass stopping power ratio (s/ρ)w,air is included. The spectral distribution of the photon and electron fluence in FFF photon beams at the point of interest may differ compared with conventional photon beams with the same beam quality index (TPR20/10 or %dd(10)x) and consequently the mass stopping power ratio, (s/ρ)w,air, may differ between these beams. Also, for FFF beams the lateral absorbed dose profile on the central axis of the beam at the depth of interest is peaked. Thus, when using Farmer-type chambers for absorbed dose determination there is an incomplete measurement of the charge. In the dosimetry protocol TRS-483 regarding FFF beams correction for volume averaging effect as well as correction for difference in (s/ρ)w,air with reference to conventional beams are included in the chamber specific beam quality correction factor (Palmans et al 2017). The correction factors for volume averaging effect in FFF beams, presented by Palmans et al (2017), are the same for all of the Farmer-type chambers utilized in the present investigation. As can be seen in table 1, the length of the air cavity of the chamber for the Farmer-type chambers differs, 1.1 mm at maximum, and this introduces an uncertainty less than about 0.05% in absorbed dose determination for the different chambers used.
The Farmer-type cylindrical ionization chambers studied had different constructions and materials. Both the factory or SSDL provided calibration factors (SSDLND,w,Q0) and the cross-calibrated (CrossND,w,Q0) calibration factors were utilized for the determination of the absorbed dose to water. These calibration factors are traceable to three different primary standards at three different PSDLs. As described above, the degree of equivalence between these primary standards at the various PSDLs may differ, up to about 0.4% (Kessler et al 2013). The determination of the true absorbed dose to water is difficult. When using ionization chamber dosimetry for absorbed dose determination the statistical uncertainty associated with the method employed is 0.9% for the 60Co γ-beam and 1.5% for the MV photon beams (Andreo et al 2000). In other words, for MV photon beams, from a statistical standpoint it is acceptable to have a difference of 3% in Dw (obtained with two different chambers), which is a relatively large difference in this context. Consequently, the true absorbed dose to water can be associated to be in a range of 6% (+1.5%+3%+1.5%) and this is, statistically, an adequate assessment. Though, from a clinical standpoint of view, in external beam radiotherapy delivery of an accurate radiation dose to the target volume in the patient is of prime interest, because, in addition to the uncertainties associated with the radiation dose, there are other uncertainties present (i.e. patient positioning errors, etc). Comparison of the individual Dw values found in the present experimental investigation using the ionization chambers in the 60Co γ-beam and the MV photon beams shows that each of the results obtained are within the statistical uncertainties of all of the other results. However, when assuming that the uncertainties in the absorbed dose determination are similar for all the chambers used, there are noticeable differences between the results obtained. In other words, the selection of the Farmer-type chamber for the determination of the reference dose can considerably influence the outcomes and this is most likely for megavoltage photon beams, conventional as well as FFF beams. It should be remembered that the present experimental investigation is an inter-chamber comparison. The main purpose of this study was to make relative comparisons of the Dw results obtained with the various Farmer-type ionization chambers in conventional as well as FFF-beams. An absolute comparison of the results was not possible since the true absorbed dose value was not known.
5. Conclusions
The spread in the data obtained using the various Farmer-type ionization chambers indicates that the selection of the ionization chamber for reference dose determination, in reference conditions, can considerably (up to about 1%) affect the outcomes in conventional as well as FFF photon beams. The differences found in absorbed dose determination employing the Farmer-type chambers were similar in both the conventional and the FFF photon beams. Comparison of the absorbed dose determination obtained using the PTW30011 and PTW30012 type ionization chambers shows that the correction for the central electrode (pcel) regarding these chambers is associated with an inaccuracy that is larger than the uncertainty specified for this quantity. Also, the outcomes found in the present study show possible inaccuracy in the employed beam quality correction factors (kQ) and imply the need for a revision of these factors.