Abstract
The most common reasons for hard-tissue implant failure are structural loosening and prosthetic infections. Hence, in this study, to overcome the first problem, different bioinspired coatings, including dual acid-etched, anodic TiO2 nanotubes array, anodic hierarchical titanium oxide (HO), micro- and nanostructured hydroxyapatite (HA) layers, and HA/chitosan (HA/CS) nanocomposite, were applied to the titanium alloy surfaces. X-ray diffraction and FTIR analysis demonstrated that the in situ HA/CS nanocomposite formed successfully. The MTT assay showed that all samples had excellent cell viability, with cell proliferation rates ranging from 120% to 150% after 10 days. The HO coating demonstrated superhydrophilicity (θ ≈ 0°) and increased the wettability of the metallic Ti surface by more than 120%. The friction coefficient of all fabricated surfaces was within the range of natural bone's mechanical behavior. The intermediate HO layer increased the adhesion strength of the HA/CS coating by more than 60%. The HO layer caused the mechanical stability of HA/CS during the 1000 m of friction test. The microhardness of HA/CS (22.5 HV) and micro-HA (25.5 HV) coatings was comparable to that of human bone. A mechanism for improved adhesion strength of HA/CS coatings by intermediate oxide layer was proposed.
Export citation and abstract BibTeX RIS
1. Introduction
Titanium (Ti) and its alloys are forefront materials for biomedical applications because of their decent biocompatibility, excellent mechanical properties, and corrosion resistance [1–5]. These characteristics make titanium a promising candidate for orthopedic implants. However, the interactions of metallic titanium surface with the living tissue during the initial stages of implantation should be considered [6–8]. Inadequate bioactivity of titanium, which is followed by accumulation of fibrous tissue on the implant's surface, could result in isolation of implant from its adjacent living tissue and consequent patient suffering of precocious loosing. Accordingly, Ti implants need surface modification for improving their biological responses and subsequent osseointegration [9, 10].
Many surface treatment methods, including chemical, physical, mechanical, and biological modifications, have been studied to improve Ti surface bioactivity and osteoconductivity [11, 12]. The purpose of most modification approaches is to alter the surface chemistry and morphology, which are influential factors in the biological performance of the implant [13, 14]. Among various proposed modification treatments, simple, rapid, and low-cost procedures such as anodic oxidation (anodization) and sol–gel could be noteworthy processes to enhance surface properties. Anodization is an electrochemical approach mainly used to fabricate thick oxide layers on valve metals. Various anodic oxide morphologies can be produced on Ti substrates through controlling the process parameters such as different electrolyte solutions, voltages, and time [15–18]. The anodic films fabricated by anodization could be thick, adhesive, and benefited from engineering porosities for interlocking with surface coatings. The sol–gel process, on the other hand, is a simple but effective approach for depositing a wide range of compounds on a variety of surfaces. Process parameters such as the number of dipping stages and substrate immersion velocity can regulate film qualities like thickness and layer uniformity [19, 20].
Hydroxyapatite (HA) is the main inorganic component of the natural bone. Therefore, the presence of HA in biomedical coatings can encourage the formation of living tissue [21–23]. On the other hand, the natural bone's HA has been distributed in a polymeric matrix of collagen [24]. Accordingly, a polymeric biomaterials such as chitosan (CS) can modify the properties of the implant ceramic coatings. Hence, a composite made of HA as the same bone's inorganic component, and CS as a bioactive polymer, can biomimetically enhance the implant coating's performance.
Nevertheless, the interfacial adhesion for this kind of nanocomposite coatings, which are typically deposited through the dip-coating sol–gel method, is relatively weak [25, 26]. To tackle this challenge, different strategies for increasing adhesion strength at the substrate|coating interface have been proposed [27–30]. Most prior works have primarily focused on developing new coatings or altering the chemistry of suitable materials to improve the physical and mechanical properties of the coating. However, no comprehensive study on the adhesion improvement of biomedical coatings on Ti implants using intermediate layers has been reported.
This study focuses on the synthesis and evaluation of different conventional and straightforward multi-layer coatings with the goal of improving their mechanical behavior by regulating the properties of the intermediate layers at the substrate/coating interface. To achieve this purpose, different bioinspired coatings, including dual acid-etched, anodic TiO2 nanotubes array (TNTs), anodic hierarchical titanium oxide (HO), micro-and nanostructured HA layers, and HA/chitosan (HA/CS) nanocomposite coatings were fabricated on the Ti alloy implants. In addition, HA-based coatings were formed on the anodized surfaces comprised of TNTs and HO intermediate layers. Based on the in situ precipitation of the coatings on intermediate layers using the sol–gel process, we anticipate that the intermediate layers will affect the physical and mechanical characteristics of the coatings, potentially improving the biomedical performance of the implants.
2. Materials and methods
2.1. Sample pretreatment
Ti6Al4V plates were prepared at 10 mm × 10 mm × 1 mm. The Ti6Al4V samples were polished before surface coating processes using different SiC emery papers and Al2O3 nanoparticles (200 nm) suspension. The shiny sheets were ultrasonically washed in deionized (DI) water and subsequently cleaned with acetone to remove organic components and improve surface purity. Eventually, the samples were rinsed in DI water and dried with a flowing air stream.
2.2. Dual acid-etched surfaces
Ti6Al4V samples were immersed in a 24% V/V hydrofluoric acid (HF) solution for 120 s. Subsequently, the specimens were etched by a combination solution of 70% hydrochloric acid (HCl)/sulfuric acid (H2SO4) with a ratio of 4:1 at 80 °C for 5 min. Finally, the samples were washed with DI water.
2.3. Titanium oxide coatings
TNTs array and HO surfaces were synthesized using an anodic oxidation (anodizing) technique. Before electrochemical treatments, the shiny Ti6Al4V sheets were etched in HF solution for 10 s to surface activation and then rinsed with DI water. The electrochemical cell had a two-electrode configuration, including a platinum sheet as the cathode and a prepared Ti6Al4V sheet as the anode. Anodizing was accomplished by using a 0.5 wt.% HF aqueous solution at ambient temperature to obtain the TNTs coating layer. The process involved a potential of 20 V for 180 min at ambient temperature. For HO coating fabrication, a 20 V anodizing route was conducted for 60 min using an H2SO4 electrolyte solution at ambient temperature. After anodizing procedures, the samples were rinsed with DI water and dried with a stream of flowing air. Finally, the samples were annealed at 500 °C for 2 h.
2.4. HA coatings
In this section, two types of HA coatings, including micro-HA and nano-HA, were deposited on Ti6Al4V substrates. To fabricate the micro-HA coating layer, a suspension of HA powder (Merck Co., Ltd) was prepared. The dip-coating method was applied to precipitate different thicknesses of HA layers by controlling process parameters. After deposition, the coated samples were dried, annealed at 600 °C for 15 min, and gently cooled to room temperature. A sol–gel method including the following steps was conducted for the synthesis of the nano-HA coating: 0.43 g of P2O5 was dissolved in 5 ml absolute ethanol, and then 2.36 g of Ca(NO3)2.4H2O (Merck Co., Ltd) was also dissolved in 5 ml ethanol to form a 1.67 M solution. The solutions were mixed to obtain a calcium phosphate (CaP) sol with a 1.67 molar ratio of Ca/P. The pH value was kept close to nine for the sol. The prepared sol was aged for 12 h. Then, the aged sol was heat-treated at 700 °C for 4 h to obtain pure HA powder. For the coating process, a suspension of synthesized HA powder in DI water was made. The prepared suspension was dispersed using ultrasonic microwaves with stirring for 2 h to achieve a homogeneous suspension. The nanostructured HA layers were deposited on the polished and anodized Ti6Al4V (TNTs) substrates to fabricate Nano-HA and TNT/HA coating samples, respectively. The substrates were immersed in the prepared HA suspension by a dip-coating system consisting of a two-way electronic switch to descend and ascend with a constant speed of 5 cm min−1. After deposition, the coated specimens were annealed at 600 °C for 15 min and subsequently cooled to ambient temperature.
2.5. HA/CS nanocomposite coating
An in situ synthesis process was utilized to synthesis of the HA/CS nanocomposite coating. The CS powder (Sigma-Aldrich) with >85% deacetylation degree and a viscosity average relative molecular weight of 1.8 × 105 Da. was supplied. A solution containing 1.5 Vol.% acetic acid was prepared. To make a CS aqueous-based solution, 1 gram of CS powder was dissolved in acetic acid. Following that, the CS solution was gradually added to the 5.8 wt.% H3PO4 solution while being agitated. For the final HA/CS composition, the ratio of H3PO4 to CS range was adjusted from 100/15 to 50/50 by weight. The CS/H3PO4 solution was gradually dropped into a 7.4 wt% Ca(OH)2 suspension upon stirring until a pH value of 9 ± 0.2. The molar amounts of calcium and phosphor were adjusted by 0.1 and 0.06, respectively, to obtain Ca/P atomic ratio of 1.67. In this situation, the CS became insoluble in Ca(OH)2 suspension and precipitated accompanying with small HA crystals, forming an in situ HA/CS nanocomposite. The reaction temperature was kept at 25 °C, and the dropping speed was adjusted at 3 ml min−1 by a tube pump. In the next stage, the obtained slurry was aged for 24 h upon continuous stirring. Ultimately, the precipitate was filtered and washed several times with DI water, and dried at 125 °C for 1 h. For the coating process, a suspension of the prepared HA/CS nanocomposite powder in DI water was prepared. The HA/CS suspension was dispersed under stirring and sonication for 2 h to achieve a homogeneous suspension. A dip coating system was used for the deposition of the HA/CS nanocomposite coating on the prepared Ti-based substrates. The dip coating system consisted of a two-way electronic switch to descend and ascend. The substrates were immersed in the suspension at a constant speed of 5 cm min−1 at ambient temperature. The coated specimens were annealed at 125 °C for 60 min after deposition.
2.6. Morphology and phase structure characterizations
Field emission scanning electron microscopy (FESEM) (MIRA3 T-Scan) was utilized to study the surface morphologies of prepared samples. The samples were coated with Au before FESEM analysis. X-ray diffraction (XRD) (Philips X-Pert diffractometer) was used to analyze the crystal structures and phases of synthesized powder and deposited coatings using Cu Kα radiation of λ = 0.154 nm, 2θ of 3–120°, scanning rate of 0.02° s−1, time step of 4 s. For data analysis, the X'Pert Highscore plus software was employed.
2.7. Wettability analysis
The surfaces' wettability was investigated using the sessile drop method in connection with a contact angle setup supplied with a microscope equipped with charged-coupled device (CCD) camera. The experiment was carried out in the air with a 2 μl droplet of distilled water floating from the tip of a microliter syringe. Considering the high organic pollutant absorption capacity of the surfaces, the contact angle tests were performed two weeks after the coating procedure to assess the wettability in work circumstances.
2.8. Adhesion strength and hardness measurements
The pin-on-disc test (ASTM G99) determined the adhesive bond strength of the coatings to substrates and the substrate effects on coating adhesion. A load of 0.15 N and a slow speed of 0.08 m s−1 were applied to the pin to prevent sudden high forces. Five replicates were tested, and an average was reported for each specimen. Friction coefficients (ξ) were calculated for coatings by evaluating the movement behavior of the pin on the surface. Vickers microhardness measurements (ASTM B578) were analyzed on the coatings using a 25 g load for 15 s, and an average value of five measures was reported for each sample.
2.9. In vitro biological studies
2.9.1. Cell culture
The osteoblast cells (MG63, National Cell Bank of Iran) were cultured in an Roswell Park Memorial Institute (RPMI) medium. The culture medium was enriched with 10% (v/v) fetal bovine serum (FBS, Gibco) and 1% (v/v) penicillin-streptomycin solution (Gibco) before being incubated at 37 °C in a humidified atmosphere with 5% CO2 gas flow. The cells were quantified using Trypan blue dye following the passage with 0.05% Trypsin-Ethylenediaminetetraacetic acid (EDTA) (Gibco).
2.9.2. Biocompatibility and cell proliferation
The direct contact method was employed to assess the biocompatibility and cell proliferation on the samples. Prior to the in-vitro assay, all the samples were cut to pieces with dimensions of 1 cm × 0.5 cm × 0.2 cm. After sterilizing under UV exposure for 24 h, three pieces of each sample were placed in a 24-well plate, and 2 × 103 cells with 100 μl of culture medium (RPMI + 10% FBS) were seeded on the samples. Following incubating the plate containing samples at 37 °C for 4 h, 1 ml of culture medium was poured into each well. The cell viability was evaluated by 3-(4,5-dimethylthiazol-2-yl)-2,5-diphenyltetrazolium bromide (MTT) assay. Accordingly, after five and ten incubation days, the culture medium was removed from the wells. Then 300 μl of the MTT at a concentration of 0.5 mg ml−1 was poured into each well and incubated for 4 h. The mixture was taken from the samples after 4 h, and 300 μl of isopropanol was added to dissolve the purple crystals. The plate was shaken for 15 min to help dissolve the MTT solution. Subsequently, the optical density (OD) at 545 nm was quantified using an Elisa Plate Reader (Molecular Devices, Model Maxline, USA). The wells with more live cells showed higher absorbance intensity than wells with fewer ones. The assay was conducted in triplicate. Cell viability of the samples was calculated using the following equations:
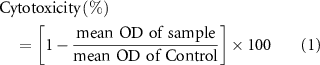

2.9.3. Cell attachment and morphology
The samples were cut into 1 cm × 0.5 cm × 0.2 cm pieces. The sterilized specimens were inserted in wells of 24-well plates containing 100 μl RPMI culture media. Following that, 1 × 104 MG63 cells were seeded onto each sample, and the plate was incubated for 4 h at 37 °C. Following primary cell attachment, 100 μl RPMI supplemented with 10% FBS was poured into each well. The plate was incubated at 37 °C for a further 48 h. Subsequently, the samples were rinsed by PBS and fixed for 4 h in 2.5% glutaraldehyde. The fixative was then discarded, and the cells were dehydrated with 50, 60, 70, 80, 90, 95, and 100% alcohol, respectively, for 5 min. Then cell attachment to the surface was examined by scanning electron microscopy. Figure 1 illustrates a flow chart of the applied procedure steps to prepare the samples for characterization.
Figure 1. A representation of the sample preparation flow chart and characterization stages.
Download figure:
Standard image High-resolution image2.10. Statistical analysis
The data were examined by one-way analysis of variance. To determine a statistical significance between groups, Tukey post-hoc test was performed by Jamovi software. The experiments were repeated at least three times for each sample. The results were expressed as the average ± standard deviation (SD) for the data obtained from MTT assay, microhardness tests, scratch analysis, and wettability measurements. Differences between groups were considered significant at p < 0.05.
3. Results and discussion
3.1. XRD and FTIR analysis
XRD analysis of the synthesized nano-HA and HA/CS nanocomposite powders have been shown in figure 2(a). As shown in figure 2(a), the pure HA (Ca10(PO4)6(OH)2 phase was successfully synthesized. Other CaP compounds, such as tricalcium phosphate (TCP) and octacalcium phosphate, have lower stability, a faster dissolving rate, and weak biological responses than HA [31], were not formed. The following reaction could indicate HA formation during the applied process:

Figure 2. (a) XRD analysis of the synthesized nano-HA and in situ HA/CS nanocomposite powders. (b) FTIR spectra for the synthesized nano-HA and in situ HA/CS nanocomposite powders (inset images show FESEM micrographs of the nano-HA and HA/CS nanocomposite powders).
Download figure:
Standard image High-resolution imageIt has been demonstrated that improper heat-treatment of the products can result in the different phases formation, including β-TCP or calcium oxide (CaO), when obtaining pure HA from Ca(NO3)2.4H2O and P2O5 using the sol–gel method [32, 33]. While the obtained XRD analysis suggests that pure crystalline HA can be synthesized at 700 °C. The XRD pattern of HA/CS nanocomposite coating demonstrates the successful formation of HA nanoparticles through an in situ procedure. The peak at 2θ = 20.8° corresponding to the crystalline phase of CS [34, 35], has become broad in the nanocomposite XRD pattern accompanying with the peak splitting into multiple separate ones. In addition, several possible CS's peaks have been obtained from the HA/CS nanocomposite. It should be noted that the presence of several peaks near the XRD characteristic peaks of the CS that indicate irregular deviation from HA peaks increases the possibility of the formation of CS. An FTIR study was performed to achieve a more accurate assessment of the chemical properties of the synthesized powders.
Figure 2(b) shows FTIR spectra for the prepared nanostructured HA and in situ synthesized HA/CS nanocomposite powders. As is seen, the phosphate absorption bands within 1000–1100 cm−1 and phosphate bending vibrations within 500–600 cm−1 of HA appeared in the nano-HA sample. The bands at 1418–1485 cm−1 and near 875 cm−1 are due to carbonate ions in apatite. However, the most significant CO3 band was revealed at 1418–1485 cm−1. The 1550–1700 cm−1 vibration bands are related to OH ions of HA that could be related to carbonate species. The HA-based absorption bands of the HA/CS nanocomposite have been extended significantly compared to the corresponding nano-HA vibrations. This could be attributed to the formation of nanocomposite powder by adding the CS, as shown in figure 2(b). It should be noted that the broadband at 1036 cm−1 demonstrates the presence of polymeric CS and its reaction with phosphate species [36]. The bands within 1550–1700 cm−1 could be related to the hydroxyl ions of HA and the 1,2-amid CS groups. The 3600–3700 cm−1 and 2800–2950 cm−1 wavenumbers are attributed to the presence of hydroxyl groups in CS. At the same time, the appearance of 2849 cm−1 and 2918 cm−1 absorption bands could be due to the C–H bonds of CH2 groups present in the CS.
Also, FTIR analysis of the HA/CS nanocomposite indicated 1467 cm−1, 1552 cm−1, and 1615 cm−1 vibrations attributed to the NH2, –NH, and C=O bonds of CS, respectively. The 565 cm−1, 839 cm−1, 952 cm−1, and 1036 cm−1 indicate phosphate groups of the HA. While the appearance of absorption bands within 2900–3700 cm−1 is assigned to the OH groups of HA. The vibration near 1412 cm−1 wavenumber is the asymmetric C–H bending band of the carbonate group in the nanocomposite. The other carbonate absorbance bands appeared at 840 cm−1 and 1433 cm−1. The vibration at 3418 cm−1 is attributed to the O–H and N–H absorption bands overlapping the HA/CS nanocomposite. Accordingly, the presence of HA nanoparticles in nanocomposite powder synthesized via an applied in situ fabrication process could be found. The vibrations for [HPO4]2− were specified at 528 cm−1, 576 cm−1, 876 cm−1, 988 cm−1, 1062 cm−1, 1131 cm−1 wavenumbers, and their intensities were reduced during the nanocomposite production process and disappeared via in situ hybridization. However, the vibration bands of [PO4]3− appeared at 963 cm−1 [37].
According to figure 2(b), specific vibrations related to phosphate bands appeared near 600 cm−1 wavenumbers. It should be noted that the crystallinity of HA could be evaluated based on the vibrations at the phosphate band of 600 cm−1 as follows: (a) three significant separate vibrations indicate high crystallinity of more than 70%, (b) two significant separate vibrations, and one small vibration result in a medium crystallinity of approximately 50%, (c) three small vibrations designate crystallinity values ranging from 30% to 50%, and (d) only one vibration shows low crystallinity of less than 30% for HA structure. Accordingly, the specified vibrations (two separate and one small vibration) near 600 cm−1 in both FTIR spectra revealed medium crystallinity values of approximately 50% for the formed HA in the prepared powder. It is also worth noting that there are no other CaP compounds except HA detected in the synthesized nano-HA and HA/CS nanocomposite powders, which confirms the XRD results. In addition, according to the XRD results (see figure 2(a)) and FTIR analysis, it can be understood that the in situ fabrication of the HA/CS nanocomposite by the applied sol–gel method was successful.
XRD on of prepared coatings is illustrated in figure 3. In the dual acid-etched surface, XRD analysis indicated a titanium hydride (TiH2) crystalline phase along with the Ti substrate peaks (figure 3(a)). While, there is no hydride phase of titanium in the XRD patterns of TNTs and HO samples anodized in HF and H2SO4 electrolytes, as illustrated in figure 3(b). As mentioned, the dual acid-etch process of Ti6Al4V alloy was conducted by HF for the first step and a combination of HCl/H2SO4 electrolyte for the second one. Hence, it could be understood that the presence of the TiH2 phase in the acid-etched surface structure is probably due to the second step of the process that was done using the cooperation of HCl. It should be noted that the XRD analysis revealed anatase crystalline phases for both TNTs and hierarchical nanostructured coatings. The high crystallinity of the anodic coatings could be due to annealing of the samples at 500 °C that results in an amorphous to anatase phase transformation, accompanying with increasing their crystallinity [38, 39]. The appearance of substrate peaks could be attributed to the formation of thin anodic films using applied procedures.
Figure 3. X-ray diffraction patterns for (a) dual acid-etched surface, (b) TiO2 nanotubes (black line) and hierarchical titanium oxide (blue line) coatings, (c) synthesized nano-HA layer, and (d) HA/CS nanocomposite coating fabricated on Ti6Al4V alloy.
Download figure:
Standard image High-resolution imageFigure 3(c) shows the HA phase layer fabricated on a Ti6Al4V alloy substrate. As expected, the coating offers a pure and crystalline HA phase. However, some intensities have changed compared to those of the synthesized HA powder (figure 2(a)). It may be related to the directional crystallization of the HA coating on the Ti substrate. Figure 3(d) shows XRD analysis of the HA/CS nanocomposite coating fabricated on a Ti6Al4V substrate. As is seen, the layer consists of the crystalline phase of HA. However, it is understood that the CS is formed in an amorphous phase. This behavior of CS could be attributed to the dissolution stage that was continued by the rapid or non-homologous formation of the HA/CS nanocomposite layer. This formation could have resulted from the fast dropping of CS/H3PO4 solution and/or high-speed stirring during the aging process. However, semi-broad divided peaks near the characteristic 2θ positions of CS, similar to the XRD pattern of the synthesized HA/CS powder (figure 2(a)), can be detected.
3.2. FESEM studies
Figure 4 illustrates FESEM images of the prepared coatings. As is seen, the dual acid-etched sample indicates a rough surface morphology consisting of micrometer structures (see figure 4(a)). The non-uniform roughness variations and orientations might be due to different chemical compositions and the α + β phase structures of the Ti alloy substrate being etched at various rates. Hence, it can be a promising capability to obtain controllable various surface morphologies via multi-step etching methods using other acid solutions. Figure 4(b) illustrates the HO coating synthesized using anodization. The prepared anodic layer indicates a distinct micrometer conical-shaped titanium oxide, which could be due to selective anodizing in the presence of H2SO4 electrolyte solution. The nanoscale structures are observable on the conical-shaped microstructures' surfaces, indicating outstanding hierarchical morphologies. On the other hand, the cones indicated a diameter of approximately 5 μm formed by nanostructured layers. Kunrath et al [40] fabricated different surface morphologies on titanium alloys and indicated that osteoblast cells present better adhesion and behavior in nanostructured morphologies. Furthermore, it has been demonstrated that the nanostructured surfaces, which is on the scale of various biological species, improve protein adsorption, cell mobility, cell differentiation, cell proliferation, and surface bioactivity as well as stimulate outside-in signals through the formation of focal adhesion complex [41]. Hence, these hierarchical conical-shaped nanostructured coatings could be fittingly function as biomimetic surfaces because of their multi-dimensional properties and similarity to human bone structures.
Figure 4. FESEM images of (a) dual acid-etched, (b) hierarchical titanium oxide structure, (c) titania nanotube array, (d) micro-HA, (e) nano-HA, and (f) HA/CS nanocomposite coatings fabricated on Ti alloy implants.
Download figure:
Standard image High-resolution imageFigure 4(c) shows the anodic TNTs array synthesized on a Ti alloy substrate using the anodization technique. The uniform porous morphology demonstrates that the fabrication process benefits from suitable electrolyte solution and enough proper anodization time and voltage to complete the pore formation stages. It has been reported that the anodic oxide nanotubes are formed using fluoride-containing electrolytes during three steps of anodization, including (a) a plunge in the current density because of the formation of a titanium oxide compact layer, (b) an increase in the current density attributed to the pore formation process in the preformed oxide layer, and (c) constant current density towards equalization of redox reactions, resulting in the synthesis of tubular pores on the Ti alloy surface [42]. The average pore diameter of approximately 50 nm introduces the prepared coating as an excellent template for bone cell growth and proper pathways to store nutrients and body fluids, which could enhance biological responses.
Figures 4(d) and (e) depict micro- and nano-HA coatings dip-coated on Ti alloy substrates, respectively. As can be observed, the micro-HA coating consists of deposited micrometer laminar structures with a disordered aggregation of nanoparticles. At the same time, the nano-HA deposit shows a uniform nanosized porous structure. The subsequent sintering resulted in the formation of a consistent HA lattice, including pores that are appropriate templates for biological responses and proper pathways to body fluids and nutrient storage. It should be noted that the inadequate bioactivity of the implant surface, which results in the accumulation of fibrous tissue around the implant surface, could lead to isolation and precocious loss of the implant in the patient. Hence, the high porosity and large specific surface area of the bioactive nano-HA coating could be excellent properties to improve cell anchoring and osteointegration, which would increase implant stabilization at the initial stage of implantation and reduce the patient's healing duration.
Figure 4(f) shows the in situ HA/CS nanocomposite coating synthesized on a Ti implant. It is worth noting that, although polymeric compounds deposit as a compact coating due to their high molecular structures and weights, the nanocomposite layer indicates a uniform and cohesive design consisting of wormholes. The present pores could be caused by the exhaust water of suspension that has been evaporated by subsequent heat treatment at 125 °C. It means the water in suspension has acted as a pore-forming agent to make open holes. Therefore, the applied coating process could be a promising candidate for in situ syntheses of porous layers appropriated for biological applications.
3.3. Wettability
The contact angles between DI water droplets and manufactured implant surfaces are depicted in figure 5. As observed, the measured wettability values for the prepared implants have changed due to applied coatings and surface modifications. It means hydrophilicity and surface properties such as free energy could be different for each implant based on the chemistry and topography of the fabricated surface. It is worth noting that all prepared samples benefit from appropriate hydrophilic surfaces, which are essential to maximizing the implant's ability to react with bodily fluids. However, the bare Ti6Al4V exhibited a hydrophobic surface with a contact angle of 127°, which showed the lowest hydrophilicity compared to the other samples. In contrast, the anodized surface with hierarchical morphologies indicated excellent hydrophilicity with the measured contact angle of approximately θ ≈ 0° that could be caused by the nanostructures formed on the micro-conical surface topographies. Furthermore, the surface chemistry of anodic titanium oxide could enhance wettability through a potential hydrogen bond-driven process. The previous investigations demonstrated that surface modification, which leads to increased hydrophilicity, can significantly improve cellular responses [43–45]. Kunrath et al [46] performed physico-chemical surface modifications on Ti alloys to generate and maintain high hydrophilicity accompanied with proper biocompatibility for biomedical implant applications. They indicated that the anodized surfaces modified by reactive plasma suggest better hydrophilicity stability, which leads to improved biocompatibility performances [46]. Sarraf et al [47] applied heat treatment to increase the surface wettability of the anodized samples. They controlled the contact angle of the anodic TiO2 surface in the range of 7.5°–13.3° via annealing of the sample at 500 °C–700 °C. However, it should be noted that the hierarchical TiO2 fabricated in this work showed superhydrophilicity without any post-treatment. It occurred due to the high surface energy as well as the semi-conductive nature of the hydroxylated TiO2 surfaces. Furthermore, the penetration force of the pin-like nanostructures of the HO into the water droplets can overcome surface energy at the solid/liquid interface. Hence, the combination of high surface energy of the semiconductor HO, as well as its unique morphology, results in the obtained surface superhydrophilicity.
Figure 5. Wettability analysis of the fabricated surfaces including the machined (polished metallic Ti) surface, dual acid-etched, anodic hierarchical titanium oxide, TNTs, micro-HA, nanostructured HA, and HA/CS nanocomposite coatings synthesized on Ti implants.
Download figure:
Standard image High-resolution imageThe TNTs array as the another anodic layer showed a low contact angle of approximately 16.7°, which demonstrates the high hydrophilicity of its porous surface. It has been demonstrated that the UV-exposed TiO2 surfaces release charge carriers (electron–hole pairs), which results in surface polarization [48]. Hence, the electron–hole pairs produced on the TNTs result in the reaction of Ti4+ with oxygen and the absorption of O2− on the surface of the titanium oxide film. The O2− was oxidized by the produced holes and released as O2 molecules. The leftover free spots from the released O2 are favorable sites for H2O molecules to occupy. This can increase the wettability of anodic titanium oxides, especially their porous forms such as the TNTs. Accordingly, it could be found that the nanostructured titanium oxide coatings provide high hydrophilicity due to their surface chemistry, roughness, and porosity.
As is seen in figure 5, the HA coatings have higher wettability compared to the bare Ti surfaces. This hydrophilic behavior might be related to oxygen functional groups of HA that can make hydrogen bonds with water molecules and increase wettability. It should be mentioned that the nanostructured HA coating is more hydrophilic than the micro-HA layer. Actually, the high specific surface area of the nano-HA coating reduces the contact angle from 39.7° to 20.5°, showing approximately 50% improvement in hydrophilicity. However, the CaP-based compounds could be mixed with other biomaterials, especially natural polymers such as CS, to mimic human bone properties. The high contact angle value of ∼96° has been reported for pure CS films [49]. Mokhtari et al [50] fabricated anodic titania nanotube array coating as the substrate for CS/bioactive glass nanocomposite coatings. Their results showed a contact angle of ∼91° for TNT/CS. While the HA/CS nanocomposite coating fabricated in this study showed a low contact angle of ∼30° that demonstrates more than 60% improvement in hydrophilicity of the CS by adding HA nanoparticles. It seems the presence of HA nanoparticles in the HA/CS structure has modified the wettability responses of the hydrophobic backbone of the CS chains [51]. Furthermore, the HA/CS nanocomposite demonstrated more excellent hydrophilicity than the pure micro-HA coating. Although the CS has weak wettability compared to the pure HA layer, the HA/CS nanocomposite indicated a higher hydrophilicity than the pure HA. This could be attributed to the modification of CS's chemical composition and physical specifications by addition of the HA nanoparticles. Moreover, it has been demonstrated that the porosity and roughness of the coating increase hydrophilicity, as demonstrated for the anodic titania layers. Accordingly, it can be understood that morphology, especially nanosized features, can play an essential role in enhancing surface wettability when a particular coating material is considered.
3.4. In vitro biological responses
Cytotoxicity of the samples was assessed via the MTT assay using MG63 osteoblast cells. The MTT assay shows the mitochondrial activities of the cells and introduces a considerable parameter for evaluating cell metabolic activities. The cells were cultured for five and ten days to study the biological responses of the sample surface at the initial and middle stages of implantation. The MTT assay results, including mean values and SDs of the cell viability and proliferation rate measurements (P < 0.05) on the prepared samples have been indicated in figure 6. As is observed, all prepared samples indicated excellent cell viability values, which were in the acceptable biomedical range.
Figure 6. (a) Cell viability and (b) normalized cell proliferation rate measurements on the prepared sample surfaces at 5 and 10 days.
Download figure:
Standard image High-resolution imageThe MTT measurements indicated similar cell viability values for the samples after five days, as shown in figure 6(a). While, the results demonstrated significant cell proliferation rates after ten days, which is relevant to the proper biological responses of the fabricated sample surfaces. It is worth noting that the bare Ti surface shows the highest cell viability for both measurements after five and ten days, with more than 157% improvement after ten days. FESEM image of the cell attachment on the bare Ti surface has been illustrated in figure 7(a). According to the FESEM micrograph (figure 7(a)), the cells attached to the Ti surface present the osteoblast cells with spindle-like morphologies. Also, there were no obvious long filopodia for the cells on the metallic Ti surface. Although the high cell viability of the attached cells with short filopodia might be considered as the appropriate biocompatibility of implant, it does not demonstrate that the implant's surface is bioactive enough to encourage cell proliferation, growth, and bone regeneration.
Figure 7. FESEM images of the cell attachment and morphology on (a) polished Ti alloy, (b) dual acid-etched surface, (c) anodic TiO2 nanotubes array, (d) micro-HA, (e) nanostructured hydroxyapatite, and (f) HA/CS nanocomposite coating synthesized on Ti alloy surfaces.
Download figure:
Standard image High-resolution imageThe other samples show convenient behavior with increased cell proliferation rates after ten days compared to the first analysis (five days). The Ti-based surfaces of the acid-etched and HO indicated 150% and 118% cell proliferation rates, respectively, during two OD measurements (see figure S1 in the supplementary material available online at stacks.iop.org/BMM/17/035007/mmedia). While the anodic TNT arrays indicated a 123% improvement in cell proliferation after ten days. FESEM images of the cell attachments on the acid-etched surface and TNTs have been shown in figures 7(b) and (c), respectively. According to FESEM studies, the proper cell attachments on the acid-etched and TNTs surfaces might be appertaining to the samples' chemical composition and surface roughness, which enhances biological responses at the bone|implant interface [52, 53]. It has been indicated that the higher surface area, hydrophilicity, and nanoscale roughness of the prepared surfaces could strongly improve the adhesion and proliferation of osteoblast cells [54]. In other words, similar to the natural bone microstructure, the surface roughness provides convenient biomimetic regions for cell anchoring and growth [12]. On the other hand, the positive effect of using TNT substrate for natural polymer coating on improved bioactivity of implant has been demonstrated [50]. Moreover, it has been indicated that the porous nanoscale TiO2 coating can convert the titanium surface from bioinert to bioactive, which leads to promoting human marrow mesenchymal stem cells toward the osteogenic differentiation [41]. As is observed, the nanotubular pores provide proper templates for filopodia penetration into the anodic oxide layer and enhance cell attachment (figure 7(c), inset: high magnification of cell attachment with long filopodia on the TNTs). However, the cell attached on the acid-etched and HO surfaces indicated similar biological behavior of the bare Ti surface, i.e. spindle-like cell morphologies with short filopodia. Hence, although the Ti-based surfaces are biocompatible, their weak bioactivity performances can result in temporary implant stabilization or delayed bone regeneration [55].
Figures 7(d)–(f) illustrate FESEM micrographs of the cell attachment on the HA-based surface coatings. As demonstrated in figure 7(d), the cells attached to the micro-HA coating show expanded morphologies with long filopodia that penetrated the surface porosities. The MTT assay revealed high cell viability values of ∼78% and ∼85% after five days for the micro-HA and nano-HA coatings, respectively. While cell proliferation rates of 136% for the micro-HA and 134% for the nano-HA coatings were obtained after ten days. This biological aptitude behavior arises from the CaP chemical composition of the fabricated HA coating that mimics natural bone properties. At the same time, the nanostructured HA coating presents a similar proper biological behavior, including proliferated osteoblast cells with expanded cell morphologies. It is worth noting that the nanostructured HA layer is associated with biomimetic bone-like nanoscale characteristics that improve the biological performance of the implant. The enhanced cell viability of nano-HA compared to the micro-HA has been demonstrated by MTT results, as shown in figure 6. According to similar chemical composition of HA coatings (micro- and nano-HA layers), the higher cell viability values for the nano-HA compared to the micro-HA demonstrate nanostructured materials' superior biological performance and size sensitive biological interactions at the bone|implant interface.
FESEM micrograph of the cell attachment and morphologies on the HA/CS nanocomposite coating has been shown in figure 7(f). As is observed, the cells on the HA/CS surface showed expanded morphologies accompanied by long filopodia that demonstrated good biological behavior of the nanocomposite coating. The MTT results showed a significant ∼154% cell proliferation rate for the HA/CS nanocomposite from day 5 to day 10. It should be noted that CS is a biopolymer that can provide suitable nanomechanical responses to bone cells. In other words, pure HA exhibits rigid and brittle mechanical behavior facing the biological tissue. At the same time, a polymeric material like CS can serve as a soft material to moderate of mechanical properties of the implant surface. On the other hand, the natural bone consists of CaP phases distributed in a collagen matrix that present ceramic and polymeric properties, respectively. Therefore, a composite made of HA as the major inorganic component of natural bone, and CS as a bioactive polymer, can biomimetically enhance the performance of implant coating. Figure 8 illustrates how the fabricated multi-layer HO–HA/CS nanocomposite mimics the human bone's composition and microstructure.
Figure 8. An overview of human bone anatomy and microstructure and the fabricated multi-layer HA/CS nanocomposite coating on the anodized Ti implant. The human bone is formed hydroxyapatite and collagen compounds (HA/Collagen nanocomposite) with spongy structure consisting micro- and nano porosities [56]. The implant was modified with multi-layer coatings, indicating the anodic TiO2 film with hierarchical morphology as an intermediate layer and HA/CS nanocomposite surface coating. The intermediate hierarchical titanium oxide (HO) with conical-shaped nanostructures improves mechanical adhesion strength of the coating, and the surface HA/CS nanocomposite coating mimics the physical, chemical, and mechanical properties of the human bone. Reprinted from [56], Copyright (2012), with permission from Elsevier.
Download figure:
Standard image High-resolution image3.5. Mechanical behavior
3.5.1. Abrasion resistance
Figures 9(a)–(d) represent friction coefficient (ξ) curves resulting from the pin on disc tests for different coatings fabricated on Ti substrates. As shown in figure 9(a), the nano-HA layer coated on the anodized surface (HA/TNTs) shows a steady-state in the range of 0.6–0.7 friction coefficient. The ξ of HA/CS nanocomposite on TNTs array (HC/TNTs) was obtained in a wide range of approximately 0.5–0.7, as indicated in figure 9(b). It should be remarked that the friction coefficient of HC/TNTs shows a plunge at the first 100 m of the scratch distance, followed by an increase in the friction coefficient value over longer distances. In contrast, the HA/TNTs sample indicates an ascending friction coefficient graph at the initial stage followed by a steady-state. These changes in abrasion resistance for HA/TNTs and HC/TNTs samples might have originated from shifting the layer under a load of HA and HA/CS, respectively, to an intermediate layer of titanium oxide nanotubes. In other words, the top surface coatings of HA and HA/CS were discovered to be removed within the first ∼100 m, and the next friction coefficient values were determined based on the anodic oxide layer. As is clear, the HA/TNTs and HC/TNTs samples illustrate similar abrasion behaviors for distances longer than 100 m, which could be attributed to the same coating material (intermediate TNTs layer), as shown in figures 9(a) and (b). The HA-based coatings may have been removed during scratch tests due to weak adhesion strength to the intermediate nanotubular oxide film.
Figure 9. Friction coefficient measurements of (a) hydroxyapatite coating on intermediate TNTs layer, HA/CS nanocomposite coating synthesized on (b) TNTs layer, (c) dual acid-etched surface, and (d) hierarchical titanium oxide film fabricated on Ti implants.
Download figure:
Standard image High-resolution imageFriction coefficient curves of the HA/CS nanocomposites deposited on the dual acid-etched surface (HC/DE) and hierarchical titanium oxide layer (HC/HO) have been illustrated in figures 9(c) and (d), respectively. As observed, the abrasion resistance of these coatings has been enhanced using double acid-etched and HO intermediate layers compared to the HC/TNTs sample. However, the friction coefficient of HC/DE shows an ascending behavior with a constant slope angle up to approximately 500 m of scratch distance, which could be attributed to the top surface coating of the HA/CS layer, as shown in figure 9(c). According to figure 9(c), the curve changes slope angle after 500 m of scratch distance followed by a lower slope ascending close to approximately 0.5–0.6 value of friction coefficient. Interestingly, the second part related to the friction coefficient curve of HC/DE is similar to the abrasion resistance properties of intermediate TNTs, as shown in figures 9(a) and (b). Hence, it could be found that the HA/CS nanocomposite coating may have been separated from the HC/DE sample after ∼500 m of scratch distance. The HA/CS coating, on the other hand, exhibited higher adhesion strength with the double etched intermediate layer in the HC/DE sample than with the TNTs intermediate layer in the HC/TNTs sample.
Among all coating samples, the HC/HO showed the lowest ξ, which demonstrated the best abrasion resistance. However, its surface nanocomposite layer (HA/CS) was similar to the surface layers of the HC/DE and HC/TNTs samples. Accordingly, the proper abrasive properties of the HC/HO sample could be attributed to the intermediate layer of hierarchical nanostructured titanium oxide. It is interesting to point out that the obtained ξ for the HC/HO demonstrated comparable or better mechanical performance than the HA-based composite coatings reinforced by carbon nanotubes and nanodiamond [57, 58]. Table 1 shows the friction coefficient values calculated for the synthesized surface coatings in comparison with human bone.
Table 1. Friction coefficient values calculated for the synthesized multi-layer surface coatings in comparison with human bone.
Sample name | Friction coefficient (ξ) | Friction gradient (Δξ)% during 1000 m |
---|---|---|
Ti6Al4V | 0.42 ± 0.02 | Low |
TNTs/HA | 0.60–0.68 ± 0.025 | 8 |
TNTs/HC | 0.46–0.68 ± 0.04 | 12 |
DE/HC | 0.19–0.59 ± 0.025 | 40 |
HO/HC | 0.18–0.35 ± 0.05 | 17 |
Human bone | 0.372–0.582 [59, 60] | Different |
Figure 10 illustrates schematic representation and FESEM images of the Ti implant and its multi-layers coating consisting of the intermediate HO layer and HA/CS nanocomposite surface coating. The implant is exposed to severe shear stress during the implantation procedure. This stress could damage the coating and separate it from the implant surface (see figures 9(a)–(c)), resulting in undesired interactions at the implant/bone interface like possible corrosion, ion release, and subsequent infections. Therefore, in addition to the proper biologicalproperties of the implant coating, its mechanical stabilization must also be considered. Figure 10(b) shows how an intermediate hierarchical nanostructured film improves scratch resistance and bonding strength of the HA/CS coating to the substrate. As is seen, the HO layer makes a conical screw-shaped interlocking with the HC coating that results in strengthening forces in contrast to implantation stress. The strengthening forces are (a) shear strength in the vicinity of HC/HO interfaces, which are parallel with implantation stress, (b) compressive strength by the faces of the hierarchical layer, which have a perpendicular component factor versus the implantation shear stress plan, and (c) bearing strength consisting of shear and compressive strengthening forces. In addition, the proper scratch resistance of HC/HO could also be attributed to the mechanical interlocking of CS chains with porous conical-shaped oxide microstructures. It is worth noting that the intermediate anodic layer with nanostructured hierarchical morphology provides new preferential sites for nucleation and growth of HA/CS nanocomposite crystals. This improvement could increase nucleation and grain boundaries that result in a poly-crystalline nanostructured coating and affect mechanical properties.
Figure 10. (a) Implant fixture designed for coating process, (b) schematic representation of the multi-layer coatings fabricated on the Ti implant showing shear forces at the bone/implant interface during implantation and different strengthening forces by intermediate hierarchical structures. FESEM images of (c) coated Ti implant fixture manufactured by CNC machine, (d) intermediate hierarchical titanium oxide (HO) layer prepared using anodization, and (e) in situ HA/CS (HC) nanocomposite coating synthesized on the HO-coated implant.
Download figure:
Standard image High-resolution image3.5.2. Hardness
Table 2 presents the microhardness values of the prepared coatings on Ti alloy surfaces. According to table 2, the microhardness of the Ti6Al4V surface shows an extreme value of 280 HV that is 7–9 times greater than the microhardness values of human bone. Due to the lack of periodontal ligament in dental implants, chewing stresses are applied directly to the surrounding bones near the implant [61]. Moreover, different mechanical properties of the metallic implant in comparison with bone could result in stress shielding and change bone density. Although acid-etching decreased surface hardness to 160 HV, the obtained value is still high compared to the bone one. In contrast, the anodic oxide layers decreased microhardness values. The obtained hardness values of the HO and TNTs layers are approximately 71 HV and 68 HV, respectively. These comparable hardness values for these coatings are most likely due to anodic titanium oxides' similar chemical composition and microstructure. However, a slight difference between the microhardness values of the hierarchical layer and TNTs was predictable due to their different surface morphologies. Microhardness values for micro- and nanostructured HA coatings were approximately 25 and 16 HV, respectively. Even though micro- and nanostructured HA coatings have the same chemical composition, different microhardness measurements may be due to their different morphologies. As shown in figures 4(d) and (e), the micro-HA coating consists of large cohesive leaf-like microstructures, while the nanostructured layer has been formed of HA nanoparticles that have been agglomerated with each other and have created a nanoporous configuration. This nanoporous structure allows deeper indenter penetration due to its porosities and weak cell walls and shows lower microhardness than the micro-HA layer. The HA/CS coated sample showed a microhardness value of ∼22.5 HV. In previous studies, the microhardness of CS coating was reported to be 0.12 GPa (12.24 HV) [63]. This means the microhardness of CS layer has been increased by 45% through the formation of HA nanoparticles during in situ synthesis of the nanocomposite.
Table 2. Microhardness measurements of bone material and prepared coatings on Ti alloy implants.
Sample code | Sample name | Microhardness (HV) |
---|---|---|
PT | Polished Ti6Al4V alloy | 279.5 ± 2.2 |
DE | Double etched surface | 160 ± 5.8 |
HO | Hierarchical titanium oxide | 71.1 ± 3.6 |
TN | TiO2 nanotube (TNTs) | 68 ± 4.2 |
MH | Micro-Hydroxyapatite | 25.5 ± 1.2 |
NH | Nanostructured HA | 16.2 ± 1.3 |
HC | HA/CS nanocomposite | 22.5 ± 1.8 |
— | Human bone | 30–36 [62] |
4. Conclusions
In this work, different biomedical coatings, including dual acid-etched, TNTs array, anodic hierarchical titanium oxide, micro- and nanostructured HA, and HA/CS nanocomposite coatings, were successfully synthesized on Ti alloy surface. In vitro biological measurements revealed high biocompatibility values and appropriate cell proliferation for the synthesized surface coatings. The results indicated that surface roughness affects cell attachment, and surface chemistry encourages cell proliferation and growth. Wettability tests revealed that the surface topography could be an effective factor along with surface chemistry in improving the hydrophilicity of the coatings. Friction tests demonstrated that all fabricated surface samples have friction coefficient values in the range of mechanical properties of the natural bone. The scratch analysis indicated that the adhesion strength of the HA/CS coating is improved by more than 60% using pin-like hierarchical nanostructures of the intermediate oxide layer compared to the tubular porosities of the TNTs array. The novel pin-like hierarchical oxide layer, which has been introduced in this work, can be used as an intermediate layer for mechanical improvement of the surface coatings to overcome the stresses during implantation. A mechanism for the enhancement of adhesion strength of the HA/CS nanocomposite coating using intermediate hierarchical oxide was proposed. The results showed that the fabricated surface coatings can mimic the human bone's physical, chemical and mechanical properties, resulting in proper biological performance to achieve a long-term stabilization.
Acknowledgment
The Iran National Science Foundation (INSF) is thanked for the general support of the research.
Data availability statement
The data that support the findings of this study are available upon reasonable request from the authors.
Conflict of interest
On behalf of all authors, the corresponding author states that there is no conflict of interest.