Abstract
Graphene oxide has been functionalized with 1-aza-15-crown-5 ether via chemical route synthesis. Modification of graphene oxide was achieved via nucleophilic attack where the amine groups of an aza-crown ether molecule can easily react with the epoxy sites of graphene oxide basal plane. Owing to the inherent two-dimensional character of graphene oxide, it resulted in large specific-surface material with strong affinity for charged chemical species. Such property was exploited for reversible and controlled interaction of adsorbed species, envisaging two possible applications of the functionalized graphene oxide. Thus, an easy-to-fabricate and high-sensitivity functionalized graphene oxide-based gas sensor was achieved. The sensing material proved to be highly stable and capable of selectively detecting humidity at room temperature over a wide range of concentrations. Moreover, the porous scaffold built by the functionalization, together with the well-known affinity of crown ethers to metal ions, allow the use of aza-crown ether functionalized graphene oxide for cation trapping application, e.g. pre-concentration of trace amount of metals or filter for water. Remarkable results in this field have been obtained with respect to some heavy-metal cations of environmental interest. We also demonstrated significant enhancement in performance versus pure graphene oxide in both tested applications. More generally, the functionalization approach we pursued appears to be quite flexible in the tested applications. In fact, with an appropriate selection of crown ethers with specific cage-like structure, functionalized graphene oxide allows the capture of any desired guest in order to prepare a wide range of other crown-ether-GO nanocomposites for different applications.
Export citation and abstract BibTeX RIS
1. Introduction
In the wide palette of multifunctional materials, graphene nanocomposites have quickly gained a prominent position. So far, the research focused on graphene and graphene-based materials has led to an extensive assortment of highly performing composites. Graphene Oxide features diverse functional groups that make its chemical and physical properties largely different from those of pure graphene. These properties, such as high-carrier mobility, tunable band gap, high mechanical strength and thermal conductivity, have naturally attracted the interest of the researchers, allowing extensive application in the field of nanoelectronics, supercapacitors, sensors, H2 storage, drug delivery, fuel cells, transistors, and polymer nanocomposites [1–8]. Several studies have been focused on the functionalization of GO surface [9–11]. Indeed, such approach allows enhancing the functional groups already present on the basal plane of GO, making it a versatile material for a wide range of applications.
As proposed by the Lerf-Klinowski model [9], GO nanosheets have chemically reactive oxygen-based functionality groups, such as carboxylic acid on the edge, hydroxyl and epoxy groups on the surface. An ideal approach to the chemical modification of graphene oxide would utilize reactions of these groups to selectively functionalize one site over another [12, 13]. In particular, the GO platelets feature chemically reactive epoxy groups on their basal planes. The amount of these groups depends on the GO oxidation degree. They can easily be modified under various conditions through ring-opening reactions [14], an effective mechanism, which involves the nucleophilic attack at the α-carbon by the amine group, i.e. aza-crown ether composites amine site [15].
Cyclic ether functionalities provide a potential acceptor site for chemical species due to charge distribution and to their consequent affinity to some chemical species (e.g. polar molecules and cations). Thus, being interested in straightening the properties of reversible and controlled interaction of the surface with different chemical species, we functionalized GO with 1-aza-15-crown-5 ether via chemical route synthesis and explored two possible applications of the functionalized graphene oxide (FGO) We probed the possible use of FGO for gas sensing, especially for selectively sensing humidity under room temperature conditions, and as cation membrane. In order to achieve these goals, we prepared FGO by tuning different synthesis parameters, then we compared the functionalized material to pristine GO from a morphological, structural and chemical point of view. FGO and GO films were deposited and comparatively probed versus detection of gaseous molecules. Moreover, we tested the absorbent capabilities of FGO and pristine GO with some cations of environmental interest, such as Ni(II), Cu(II) and Cr(III) by the means of Total Reflection x-Ray Fluorescence (TXRF) analysis, measuring the cation concentration before and after interaction with the FGO membranes in aqueous solution.
2. Methods
2.1. Material synthesis
The synthetic procedure adopted for the preparation of aza-crown-functionalized graphene oxide composite is shown in figure 1.
Figure 1. Schematic representation of the synthesis process of aza-crown-functionalized graphene oxide.
Download figure:
Standard image High-resolution imageAza-crown-functionalized GO was obtained starting from commercial GO (4 mg/mL) (Sigma-Aldrich), 1 ml of GO solution was diluted to 10 ml with deionized water in a beaker, thus stirred to obtain a clear homogeneous brown dispersion (0.4 mg/mL). 1-Aza-15-crown-5 (TCI Europe) and tetrabutylammonium hydroxide (TBAOH) (Sigma-Aldrich) were added to the GO solution at room temperature. The role of the latter in the reaction mechanism is to deprotonate the nucleophile in order to increase its nucleophilicity and hence the rate with which the nucleophilic substitution takes place. The mixture was stirred and heated gradually up to 80 ◦C. Then, the temperature was kept constant for 20 h and thermalized to room temperature (figures 2(a), (b), (c)).
Figure 2. XRD pattern of (a) pristine GO, (b) FGO 1:1, (c) GO mixed with TBAOH and (d) GO with 1-Aza-15-crown-5. From the bottom of every sample plot: single fitted gaussian peaks, XRD data and fitted results and residual plots between experimental and fitted data.
Download figure:
Standard image High-resolution image2.2. Material characterization
After purification with several aqueous washings synthetized FGO and pristine GO films were characterized by means of several techniques. The morphology of the samples was investigated by Scanning Electron Microscopy (SEM), using a Zeiss EVO 40 microscope scope with an acceleration voltage of 20 kV, and by Transmission Electron Microscopy (TEM), using a FEI Tecnai F20 ST microscope with an acceleration voltage of 120 kV.
Atomic force microscopy (AFM) images were acquired with a Solver Px Scanning Probe Microscope from NT-MDT.
Raman measurements were performed by LabRam HR800 spectrometer (Horiba Jobin Yvon, France), coupled with an Olympus BXFM optical microscope (Olympus, Tokyo, Japan). The spectrometer was equipped with air-cooled CCD detector (1024 × 256 pixels) set at −70 °C, and with 600 and 1800grooves/mm gratings. The laser beam was concentrated in a spot with 1 mm diameter and the spectral resolution was approximately 4 cm−1. The He–Ne laser line at 632.82 nm was used as excitation source and was filtered to keep the laser power varying from 0.2 to 10 mW. Exposure time, beam power and accumulations were optimized for each sample in order to obtain sufficiently informative spectra but ensuring to avoid alteration of the sample. X-ray Diffraction (XRD) pattern was used to characterize the structure of GO, FGO, GO-TBAOH and GO-1-aza-15-crown-5 ether mixture, those last two were only produced for XRD characterization. XRD patterns were collected at room temperature on a Bruker D8 Advance DaVinci diffractometer with theta-theta geometry, equipped with an Cu x-ray tube operating at 40 kV and 40 mA and with a LYNXEYE XE solid-state detector set to discriminate the Cu Kα 1,2 radiation (about 2% Kβ). Fourier Transform Infrared Spectroscopy (FTIR) analyses were carried out with a Nicolet Avatar 330 Thermo Electron. X-ray Photoelectron Spectroscopy (XPS) measurements were performed using a Kratos AXIS UltraDLD instrument equipped with a monochromatic Al Kα (1 486.6 eV) x-ray source. The emission angle between the axis of the analyzer and the normal to the sample surface was 0°. For each sample, surveys and high-resolution scans of the O 1s, N 1s and C 1s core levels were collected. XPS quantification was performed using the atomic sensitivity factors and the high-resolution scans. Charge compensation was achieved using a charge neutralizer located at the bottom of the electrostatic input lens system and all core levels were referenced to the C-C/C-H component of C 1s at 284.5 eV.
2.3. Gas sensing measurements
In order to evaluate the sensing properties by electrical characterization, the material was deposited by drop-casting technique onto alumina substrates with interdigitated gold electrodes, thus packaged with a proper support (TO-39) by gold wire. The sensing properties of the drop-casted films, about 3 μm thick measured with a surface profilometer, were tested out at room temperature (25 °C) by a dedicated apparatus, which consists of an isolate gas-flow chamber and a data acquisition system (figure S1 available online at stacks.iop.org/MRX/6/075603/mmedia) [16]. Gaseous molecules are fed by certified bottles into a proper pneumatic system based on mass-flow meters. A dedicated test chamber (volume of 500 cm3) was controlled by a circuit based on an operational amplifier (OA). The voltage values Vin and Vout are connected at the ends of sensor resistor RS and applied load resistor Rf, respectively. Then, the gain is given by Vout/Vin = −Rf/RS. The expression for sensor conductance GS results equal to:
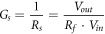
Sensor response is defined as:
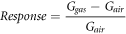
where Ggas and Gair are the steady-state conductances in gas and in air, respectively [17]. The acquisition system must manage all phases regarding the sensor electronics and is composed of a Keitheley K2000 Multimeter, to convert output voltages from analog to digital, and a management/acquisition software consisting of an acquiring software, at regular intervals, the input channel of the multimeter (gas sensor, temperature sensor and humidity sensor). The sensing measures were performed in either dry or wet air, gas from certified synthetic air bottles (20% O2 and 80% N2) were fluxed through mass-flow controllers with a fixed flow of 500 cc/min. A fixed fraction of the total flux came from the gas bubbler filled with distilled water to simulate humidity condition, the relative humidity in the test chamber has been controlled with a commercial Honeywell HIH-4000 humidity sensor. In order to assess any possible employment of this material as a sensing device, we exposed the film to several analytes, i.e. methane, acetaldehyde, ammonia, acetone, toluene, ethanol, benzene and humidity. Then, to investigate the possible role of the functionalization on the sensing perfomance two synthesis parameters, GO:Aza-crown rate and TBAOH concentration, were modified to achieve diverse functionalization levels. (table S1). The FGO 2:1, 3:1, GO and partially reduced GO (rGO) sensing performance were compared to those of the first sample synthetized, FGO 1:1, by means of electrical measurements.
2.4. Cation trapping measurements
For cation trapping measurements 10 mg of GO and 10 mg of FGO were placed in different test tubes, each one containing a 10 ml solution of one of the following cations: Cu(II), Ni(II) and Cr(III), all at the nominal concentration of 40 ppm. Then, through a centrifugation process of 30 min at 5000rpm, GO and FGO materials were separated from the suspension. 0.01 ml of an internal standard solution (Gallium 1000ppm) were added to 0.99 ml of the supernatant solutions for each samples. TXRF was used to quantify the amount of cations before and after the interaction with GO and FGO at Micro Nano Facility of Bruno Kessler Foundation. TRFX measurements were performed by an in-house build spectrometer based on a Phoenix-TNX theta-theta diffractometer modified by DFP (Italy) and equipped with a Silicon Drift Detector (SDD) having an active area of 50 mm2 (Ketek, Germany), was used to acquire the x-ray fluorescence photons emitted by the sample at grazing incidence. The SDD was mounted at 90 degrees with respect to the 0 degree incidence angle (theta = 90). The instrument is equipped with a Mo tube, which was operated at 40 kV and 30 mA and a parabolic graded multilayer monochromator (AXO, Germany) aligned to select the Mo Kα radiation. Aliquots of 5 μl of the solutions were deposited on a Si substrate, dried in vacuum and analyzed at 0.08 degrees incidence. The quantification was performed by ab initio simulation of the fluorescence spectrum through the GIMPy code—a software for the simulation of x-ray fluorescence and reflectivity of layered materials [18].
3. Results and discussion
3.1. Morphological and structural characterizations
SEM, TEM images and the Selected Area Electron Diffraction (SAED) of GO and synthetized FGO 1:1 films presents similar smooth featureless surface patterns (figure S2).
AFM was used to determine the thickness and size of GO and FGO nanosheets (figure S3), which resulted to be in the range of 1 to 8 μm wide and ≈1.7 nm thick for the GO. The lateral dimensions of FGO were of the order of 1–4 μm in widness and ≈1.8 nm in thickness and present elongated shape. Multi-layer stracking in FGO prevail over single sheet, which is presumably caused by the basal plane functionalization.
Figure 2 shows the XRD pattern of pristine GO, FGO 1:1 composites, GO with only TBAOH and GO in solution with 1-aza-15 crown-5. The last two samples were produced while maintaining all the reaction conditions (i.e. reagents concentration and temperature) used for FGO 1:1 sample, in order to study the interaction of GO with every single reagents in the process, to allow a more straightforward interpretation of the reaction. Figure 2(a) shows the typical peak of the GO placed at about 12.0° 2-theta, due to 0001 reflection [19]. FGO sample (figure 2(b)) shows a diffraction peak at 12.0° 2-theta that is along with previously cited GO peak. In addition, there are two new peaks at 7.6° and at 22.5° 2-theta. The peak at lower angle is ascribed to an increase in interlayer separation from 7.9 to 11.5 Å due to the functionalization of GO with bulky aza-crown ligand, whereas, the higher-angle peak is assigned to disordered-graphitic system [20]. GO-TBAOH (figure 2(c)) shows, other than the previously cited peaks of FGO at 12,0° and 22,5° 2-theta, a peak at 5.4° 2-theta, which is to be ascribed to the presence of TBAOH in GO interlayers. GO-1-aza-15-crown-5 ether mixture (figure 2(d)) presents peaks at 25.5° and at 38.0° 2-theta not overlapping with the previous patterns. In conclusion, the pristine GO, GO-TBAOH, GO-1-aza-15-crown-5 ether mixture and FGO show well defined XRD fingerprint peaks, which could be used to discriminate reaction products.
Figure 3 shows the FTIR spectra for GO and FGO films. According to previous FTIR studies of pure GO [21], various peaks were observed at ≈3385, 1720, 1600, 1220 and 1052 cm−1, which correspond to the hydrogen-bonded O–H stretching, carbonyl (C=O) stretching, C=C stretching, O–H deformation and the C–O stretching of the epoxides (C–O–C), respectively. In the FGO composite, the peak at 3200 cm−1 is due to the presence of the –OH groups. Skeletal C=C vibrations are observed at 1570 cm−1. It is also observed that some new peaks at 1230 and 1030 cm−1, corresponding to C–N stretching (tertiary amine) and C–O stretching vibrations, have appeared. These results confirm that aza-crown molecules in FGO react with the oxygen-containing functional groups of GO, generating C–N covalent bonds through the nucleophilic addition reaction of amine on the epoxy group [22].
Figure 3. FTIR spectra of GO (left) and FGO 1:1 (right).
Download figure:
Standard image High-resolution imageMoreover, the FTIR results are helpful to estimate the bond length, thus, the force constant of 5.8 N/cm for the C–N linkage, calculated from FTIR stretching frequency, can be correlated with the average C–N bond length by the 'Badger's Rule', resulting in ≈1.4 Å[23]. Details of the full calculations are provided in the Supporting Information.
In order to retrieve useful information about the composite structures, Micro-Raman analysis have been performed on GO and FGO samples (figure 4). GO exhibits two main bands: G band at ≈1597 cm−1 corresponding to the first-order scattering of the E2g phonon of in-plane vibration mode of sp2 carbon atoms and the D band ≈1335 cm−1 assigned to the vibrations of sp3 hybridized carbon atoms of disordered graphene such as partially disordered structures of the sp2 network and structural defects. In aza-crown functionalized GO, the G band is red-shifted to 1590 cm−1. Such shift of the binding energy of G band has also been reported by Banerjee et al [22].
Figure 4. Raman spectra of GO and FGO 1:1.
Download figure:
Standard image High-resolution imageXPS spectra of GO (figure 5, bottom) show clearly C 1s and O 1s core levels (left and centre panels) and a small amount of nitrogen, probably due to impurities (right panel). The carbon spectrum (figure 5, left) exhibits three peaks, the C–C/C–H component at ≈284.5 eV, C–O at ≈287 eV, and carboxylic group at ≈288 eV [21]. The oxygen spectrum (figure 5, centre) shows a single peak at ≈532.4eV. XPS spectra of FGO (figure 5, top) show C 1s, O 1s and N 1s core levels. The carbon spectrum shows the C–C/C–H peak at ≈284.5 eV and COO at ≈288 eV and a shoulder at 285.8 eV originated from a combination of C–N and C–O bonds. One can observe that the previous C–O peak of GO (287 eV) is diminished as a result of the reduction of GO during the functionalization reaction [24]. The oxygen spectrum of FGO shows two peaks at 532eV and 529 eV, originated from the C–OH and C=O bonds, respectively. The nitrogen spectrum of FGO shows two peaks at 401.5 and 398 eV, assignable to tertiary amine N atoms in the aza-crown ether accompanied by some imine N atoms. As previously suggested by Ballesteros et al the imines peak at 398 eV could be formed by the condensation of a 1-aza-15-crown-5-ether with GO C=O groups [15].
Figure 5. High resolution XPS spectra of GO and FGO 1:1 materials for carbon (C 1s-left), oxygen (O 1s-centre) and nitrogen (N 1s-right), showing the fit and the resulting components.
Download figure:
Standard image High-resolution imageMultiple XPS analyses were performed on different spot and on different produced samples also to quantify the elemental composition of GO and FGO. The percentage amounts of C, O and N are reported in table 1 as the results of the elaboration of elemental survey XPS spectra. The analysis shows that the amount of oxygen decreases during the functionalization reaction, providing a reduction of GO. FGO carbon to crown-ether ratio can be determined by using the atomic percentage difference between nitrogen atoms in GO and FGO [15]. This difference depends on the added functional groups of the aza-crown moiety, which amounts to 3.6% (C10H21NO4) equivalent to an average CGO:aza-crown-ether ratio of 12.5.
Table 1. Elemental percentages in GO and FGO samples.
Sample | %C | %O | %N |
---|---|---|---|
GO | 68.4 | 30.6 | 1.0 |
FGO 1:1 | 81.0 | 14.4 | 4.6 |
The characterizations results reported gave a sufficient insight to model the FGO structure through simulation. Indeed, performing MMFF94 energy minimization with a modified MM2 with Truncated-Newton-Raphson method force field, we propose a simulated FGO structure starting from GO layers (figure 6), taking into account the formation of the C–N bond with the consequent increase in interlayer separation, as obtained from XRD pattern, and the previously calculated CGO:aza-crown-ether ratio. Structural characteristics of simulated nanocomposite are comparable to experimental characterizations confirming the coherence between experimental and simulated data. Indeed, energy minimization distances from the simulation are consistent with experimental data, i.e. 8 Å and 12 Å for interlayer separation of GO and FGO, respectively, 1.4 Å for C–N bond for FGO.
Figure 6. Simulation of stacked GO (left) and FGO (right) layers: carbon atoms in grey, oxygen atoms in blue, hydrogen atoms in white and nitrogen atoms in red.
Download figure:
Standard image High-resolution image3.2. Gas sensing performance
3.2.1. Electrical characterization
GO-based gas sensors have been widely investigated by researchers and the number of publications has increased considerably over the last years. Indeed, its large specific surface area makes GO a promising material for applications where functionality is magnified by the extension of the surface exposed for interaction with the environment. At the same time, the high charge-carrier mobility enhances the transduction of the chemico-physical conditions variation, e.g. as occurs in chemoresistive gas sensors. Contrary to graphene and reduced graphene oxide, widely used for gas sensing applications both as pristine material and in functionalized arrangements [25], GO is proton conductive and hydrophilic, which makes it a promising material for moisture sensing [26, 27]. The interaction with certain gases, e.g. water vapour, NO2 and H2 [28], can be controlled by tuning the physical properties of GO sheets by reduction and/or chemical functionalization approaches [29]. As for any new sensing material, the performance of produced FGO and GO films were probed through the so-called 3S-rule [30]:
- Sensitivity is the slope of the calibration curve, that is, how large is the change in the sensor signal upon a certain change in the analyte concentration,
- Selectivity is the ability of a sensor to respond primarily to only one chemical species (specific sensor response) in the presence of other species (interferants),
- Stability is the ability of a sensor to provide reproducible results over a certain period of time; it depends on drift of the baseline signal, drift of response, and regeneration after interaction with analyte. In particular, long-term stability is related to aging of the sensor materials.
Among fifteen diverse gases tested at room temperature (methane, acetaldehyde, ammonia, acetone, toluene, ethanol, butanol, benzene, nitrogen oxide, nitrogen dioxide, carbon monoxide, carbon dioxide, hydrogen sulfide, sulfur dioxide and humidity), prepared FGO films resulted selective only to water vapors. Figure 7(a) shows the response of FGO 1:1 film at 25% RH in comparison to pristine GO and rGO. Unlike GO and rGO, FGO 1:1 film appears to be selective toward humidity and demonstrated good stability during repeated measurements (figure 7(b)).
Figure 7. Electrical characterization at room temperature of (a) GO, rGO and different GO:1-Aza-15-crown-5 rate devices versus 25% RH. (b) Stability and complete reversibility of the sensing reaction, (c) Dry-wet cycles from 10% RH to 80% RH. FGO 1:1 based sensor present better stability and sensitivity and (d) humidity calibration curve of FGO sensors.
Download figure:
Standard image High-resolution imageThe response and recovery times of the tested films, calculated as the time necessary to attain 90% of steady-state sensor response and as the e-folding response, respectively, ranged from 500 to 900 s. The response time exhibited by FGO sensors are comparable with the response time of the commercial HIH-4000 Honeywell capacitive humidity sensor used to control Relative Humidity (%RH) in the test chamber.
In order to evaluate the role of the 1-aza-15-crown-5 in humidity detection, different sensing films with different GO:1-aza-15-crown-5 weight rate were produced (table S1). Figure 7(c) shows the dependence of the sensing performance on graphene:aza-crown-ether rate.
Produced FGO devices showed different responses while exposed to a fixed humidity of 25% RH. Among the FGOs synthetized, best performing devices were provided by 1:1 GO:aza-crown-ether concentration rate, confirming that cyclic ether acts as receptor in the sensing mechanism. Functionalization plays a crucial role in improving the sensing performance of the device. Indeed, as it can be seen in figure 7(c), at low humidity concentrations all the FGO films exhibit the same sensing trends. On the contrary, by increasing the concentration of water molecules, the sensing films response increases for highest functionalized film (FGO 1:1), since all the active sites of the 1-aza-15-crown-5-ethers tend to become saturated. The stability of the sensing film and the repeatability of its response versus humidity (figure 7(b)) were not affected by any memory effects in the material during the dry-wet cycles. The normalized responses of sensors prepared under the same procedure were investigated over a wide RH% range (figure 7(c)).
The demonstrated high selectivity of the sensing film alongside with room temperature operation without employing any thermo- or photo-activation makes FGO a promising graphene oxide-based gas sensor, even in comparison to those obtained with other functional GO, pure GO or defected graphene films prepared by various methods, not only in the chemoresistive domain [31–33].
3.2.2. Proposed sensing mechanism
Figure 8 illustrates the proposed sensing mechanism of FGO. In our assumption, the interaction between the functionalization and water molecules causes a change in conductance, which is due to hydrogen-bonding interaction via proton-electron exchange between FGO and the adsorbed molecules. Previous deepened study by Carnegie et al [34] demonstrated that the crown-ether structure presents two primary binding sites, both exposed in a Cs symmetry. Theoretically, even an individual water molecule can change the conformational distribution of aza-crown structure. Water molecule(s) take advantage of the oxygen-rich character of the aza-crown ether, donating its OH groups to H-bonds to the crown oxygens. The formation of hydrogen-bonded networks facilitates the rapid transport of protons, in this way, hopping between adjacent water molecules occurs easily employing the Grotthuss mechanism, which can cause an increase of conductance [35, 36]. Furthermore, the fact that the aza-crown possesses multiple oxygen sites in proximity to one another raises the possibility that bound water molecules could interact with more than one site simultaneously. Water bonded molecules can interact via H-bond with other water molecules, forming clusters, then affecting still more the film conductance. In addition, with further increase in humidity, the large number of existing functional groups in FGO could aid proton migration between layers. All of these factors would lead to a sudden increase in conductance, leading to a quite high sensitivity humidity sensor.
Figure 8. Scheme for the humidity sensing mechanism proposed for FGO.
Download figure:
Standard image High-resolution imageAs dry condition has been restored in the test chamber, decrease in humidity will cause water molecule desorption from the surface. Indeed, Water groups have mostly electrostatic interaction with the FGO sheet, and then they can be easily removed from it. Moreover, sensing performance is not only ascribed to the abundant hydrophilic functional groups on FGO sheets but also to the large interlayer space in the FGO sheets, which facilitates water molecule adsorption and desorption. Both processes at tested humidity concentrations highlighting complete reversibility of surface reaction.
3.3. Cation trapping measurements
The use of graphene oxide as a ionic membrane was developed by Nair et al [37]. Indeed, graphene-based materials have recently emerged as potential candidates for heavy metal absorbent [38] and desalinator with excellent characteristics [24, 39, 40]. FGO synthetized in this work combines the high affinity for cations of the aza-crown-ether, that could not be used as is owing to its solubility in water, with the mechanical properties of graphene oxide. Indeed, it is well known that crown ethers form strong bonds with certain cations, generating chemical complexes [41–43]. The oxygen atoms are well situated to coordinate with a cation located inside the ring thus the denticity of the polyether influences the affinity of the crown ether versus various cations, as schematized in figure 9.
Figure 9. Schematic rapresentation of FGO sorbent capabilities.
Download figure:
Standard image High-resolution imageTXRF analyses were carried out to quantify the amount of three selected cations via internal standard method, using Gallium as reference, on initial sample and after its interaction with GO and FGO 1:1.
The amount of cations for every sample solution was determined by:

where ci is the cation concentration (ppm), Ii is the count number of the cation of interest, cs is the concentration of the internal standard (10 ppm), Is is the count number of the internal standard and Si is the relative sensitivity.
Table 2 reports TRXF analysis results, i.e. the cation concentrations before and after the interaction with GO and FGO 1:1 materials. The nominal cation concentration was 40 ppm for each solution, whereas the real values were slightly different. The percentage recovery for GO and FGO 1:1 were 45.0% and 99.4% for Cu(II) solution, 32.4% and 96.0% for Ni(II) solution, 22.8% and 86.5% for Cr(II) sample. In each sample GO layer plays a role in cation adsorption [38], but a comparison between GO and FGO 1:1 highlights an outstanding activity of the aza-crown-ether moieties. Indeed, the absorption capability of the adduct is more than the double with respect to our tested pure GO.
Table 2. Concentration (ppm) of the initial experimental cation solutions, pristine GO and FGO 1:1 after treatment.
Sample | Cu (ppm) | Ni (ppm) | Cr (ppm) |
---|---|---|---|
Initial solution | 43.05 ± 0.01 | 37.52 ± 0.20 | 41.74 ± 0.04 |
GO | 23.67 ± 0.01 | 25.36 ± 0.19 | 32.22 ± 0.05 |
FGO 1:1 | 0.24 ± 0.01 | 1.51 ± 0.19 | 5.62 ± 0.05 |
The remarkable stability of crown ether complexes with cations is enthalpic in origin [44]. However, such a complex system depends on various factors. Stability decreases in the same order as electronegativity of heteroatoms included in the cycle. Moreover, cation affinity of aza-crown ethers depends on the type of substituent attached to the nitrogen, GO in our case of study. In order to explain the different behaviour of FGO 1:1 in sorbtion capability, it is useful to apply to the utilized transition metals the 'hard and soft acids and bases' (HSAB) theory [45] and taking into account the cation valence. Within the same category of cations, the most stable complexes are formed when the ratio of cation size and cavity diameters is close to one. Any deviation from the perfect fit results in a loss of stability and, consequently, in a loss in binding capacity [41]. For Ni(II) (0.166nm of ionic diameter) and Cu(II) (0.174 nm of ionic diameter), the ratio of cation diameter over aza-crown cavity (whose size is ≈0.175 nm), corresponds to 0.948 and 0.994, respectively. In this way, the better affinity of the aza-crown moieties for Cu(II) with respect to the Ni(II) cations is well justified. The behavior of FGO with an 'hard' cation like Cr(III) is different from that with 'intermediate' cations, as Ni(II) and Cu(II). This evidence demonstrates the better affinity of the aza-crown site of FGO for relatively easy polarizability of transition metal cations, since cation-dipole interactions are thought to provide the binding energy of crown-ether complexes with 'hard' cations. Prepared FGO material showed excellent results in cation adsorption capabilities, as can be seen in table 3 FGO sorbing performance are comparable with other carbon-based nanomaterials for determination/preconcentration of metal ions. Moreover, the direct interaction between the cations and the sorbent material allows one to reduce the time of sample preparation in comparison with a classical Solid Phase Extraction (SPE) method [46].
Table 3. Comparison of the presented method with published procedures based on solid-phase extraction and determination of Cr, Cu and Ni ions.
Sorbent | Method | Element | Recovery, % | Reference |
---|---|---|---|---|
GO | EDXRF | Ni(II) | 94 | [47] |
Cu(II) | 97 | |||
GO-EDA | EDXRF | Ni(II) | 93 | [48] |
Cu(II) | 96 | |||
GO-Gly | EDXRF | Cr (III) | 98 | [49] |
Cu(II) | 99 | |||
FGO 1:1 | TRXF | Cu(II) | 99.4 | This work |
Ni (II) | 96.0 | |||
Cr (III) | 86.5 |
4. Conclusions
In this work, we have presented the synthesis procedure, characterizations and structure simulation of 1-aza-15-crown-5 ether FGO with a high coverage of crown-ether functional molecules, i.e. one crown-ether unit per approximately 12.5 carbon atoms in the graphene oxide plane. Extensive characterization via several technique (SEM, TEM, AFM, XRD, IR, RAMAN and XPS) allowed one to determine the structure of the functionalized graphene oxide nanocomposite. With the aim of exploring the physical and chemical properties of the material, in particular the reversible and controlled interaction of the surface with diverse chemical species, we employed FGO in two different fields of application that both exploit the highly specific properties of the employed functionalization. We produced a gas sensing device by depositing FGO onto alumina substrates via drop casting technique. The films showed chemoresistive behaviour with relevant selectivity to humidity and fast response time. We proposed a humidity sensing mechanism based on H-bond interaction between the film and water molecules. The sensing unit holds all the fundamental properties for a good-quality gas sensor, and makes them competitive with respect to the traditional Metal-OXide semiconducting (MOX) sensors. Furthermore, the FGO sensors accomplish sensing performance at room temperature without employing any thermo- or photo-activation stimulation to attain full reversibility of the sensing process occurring at surface. Finally, we tested FGO as cation sorbent material with a simple, time-efficient and reagent-saving method. The results highlighted possible usage of FGO as a filter for heavy metals polluted water or preconcentrator of trace amount of metals. In this way, any additional treatment, such as the elution of analytes, is unnecessary, thereby reducing the sources of contamination as well as the sample preparation time. Using FGO may be an efficent alternative to the classical sorbent such as active carbon. Indeed, conducted studies reveal the great potential of FGO towards Ni(II), Cu(II), Cr(III) as excellent sorbent material in analytical chemistry.
More generally, the method proposed in this paper is quite flexible. Indeed, with an appropriate selection of specific crown ethers, it is possible to tailor molecules with cavities whose size, shape and donor properties would allow the capture of any desired guest in order to prepare a wide range of other crown-ether-GO nanocomposites for gas sensing as well as sorbent materials.
Acknowledgments
The authors would like to thank Professor A Quaranta from University of Trento, Professor S Caramori and Dr S Pepi from University of Ferrara, D Palmieri of the Ferrara Centre of Microscopy, V Morandi and L Ortolani of CNR-IMM Bologna. The whole project was supported by 'Progetto Agroalimentare Idrointelligente' POR-FESR 2014-2020 Regione Emilia-Romagna, Progetti di ricerca industriale strategica, Asse1, Azione 1.2.2.