Abstract
Reducing carbon dioxide (CO2) emissions through a reliance on natural gas can create a hidden commitment to methane (CH4) leakage mitigation. While the quantity of CH4 leakage from natural gas has been studied extensively, the magnitude and timing of the CH4 mitigation required to meet climate policy goals is less well understood. Here we address this topic by examining the case of US electricity under a range of baseline natural gas leakage rate estimates and emissions equivalency metrics for converting CH4 to CO2-equivalent emissions. We find that CH4 emissions from the power sector would need to be reduced by 30%–90% from today's levels by 2030 in order to meet a CO2-equivalent climate policy target while continuing to rely on natural gas. These CH4 emissions reductions are greater than the required CO2 reductions under the same policy. Alternatively, expanding carbon-free sources more rapidly could meet the 2030 target without reductions in natural gas leakage rates. The results provide insight on an important policy choice in regions and sectors using natural gas, between emphasizing a natural gas supply chain clean-up effort or an accelerated transition toward carbon-free energy sources.
Export citation and abstract BibTeX RIS

Original content from this work may be used under the terms of the Creative Commons Attribution 3.0 licence. Any further distribution of this work must maintain attribution to the author(s) and the title of the work, journal citation and DOI.
1. Introduction
Natural gas is less CO2-intensive than coal and is contributing to the decarbonization of energy systems in various locations [1, 2]. Reductions in CO2 emissions from the US power sector since 2005, for instance, have been caused largely by the displacement of coal by natural gas [3, 4]. However, this transition can come with unintended consequences in the form of CH4 leakage from the natural gas supply chain [5–16]. The warming impacts of various levels of CH4 leakage have been studied [17–23], but the scale and timing of CH4 mitigation required to meet CO2-equivalent climate policy targets, and the technological pathways for doing so, remain largely unexplored.
Here we use the US power sector as a sample case to examine a set of strategies for reducing CO2 and CH4 emissions to meet policy targets. We identify different combinations of fossil and very low carbon (hereafter called 'carbon-free') generation to meet electricity demand while achieving a cut in CO2-equivalent emissions by 2030 of 32% below 2005 levels. This target is meant to reflect one scenario for the power sector's contribution to meeting economy-wide US climate policy commitments under the Paris Agreement [24, 25], and we consider other targets as well. We study the effects of applying various emissions equivalency metrics to convert CH4 to CO2-equivalent units. Finally, we compare the warming impacts of different mitigation pathways that achieve the same percent reduction in CO2-equivalent emissions over the 2005–2030 period, and we discuss the feasibility of natural gas leakage rate reductions in the context of historical data.
Our results show the degree to which a reliance on natural gas over the next two decades would need to be accompanied by a CH4 mitigation effort in order to meet climate policy targets. We find that relying substantially on natural gas would require CH4 reductions that are equal to or greater than the percent reductions in CO2. Alternatively, accelerating a shift to carbon-free sources could meet 2030 policy goals through deeper CO2 reductions and without natural gas leakage rate reductions. These two strategies bound a set of options available to policy makers for meeting climate targets while continuing to rely partially on natural gas.
The problem analyzed here is relevant not only to the US but also to other countries producing and using natural gas while pursuing emissions targets. Examples include Australia and the UK [26, 27], as well as developing countries such as China and India where natural gas production and demand are rising [1, 28, 29]. Policies emphasizing CO2 in these and other locations [30, 31] should be accompanied by a consideration of CH4.
2. Methods
Our analysis follows five steps. We (1) model CH4 emissions from the power sector for a scenario that reduces CO2 emissions but assumes no reductions in the natural gas leakage rate (scenario 1); (2) use emissions equivalency metrics to compute CO2-equivalent emissions from electricity; (3) compute reductions in the natural gas leakage rate, and in overall power sector CH4 emissions, to meet US climate policy goals; (4) consider alternative scenarios to meet these goals through faster expansion of carbon-free power, without natural gas leakage rate reductions (scenarios 2–5); and (5) compare the warming impacts of scenarios. Table 1 defines key terms used throughout the article.
Table 1. Definitions of terms used frequently throughout the article.
Term | Definition |
---|---|
Power sector CO2 emissions | Mass of CO2 emissions from coal and natural gas combustion |
for power generation | |
Power sector CH4 emissions | Mass of CH4 emissions from natural gas supply and coal mining, |
scaled to reflect the power sector's share in total consumption | |
Natural gas leakage rate | Mass fraction of emitted gas including unintentional leakage |
and intentional venting (from production, processing, | |
storage, transmission, and distribution) and produced natural gas | |
Emissions equivalency metric | Conversion factor to compute mass units of CO2-equivalent |
emissions from mass units of a non-CO2 greenhouse gas (GHG) |
2.1. Estimating baseline power sector
emissions
We first estimate the contributions of CH4 emissions from natural gas systems and coal mining to power sector CH4 emissions for all scenarios (1–5), which each assume different consumption levels of coal and natural gas. To estimate CH4 emissions from natural gas systems, we draw on review studies to define a baseline range of natural gas leakage rates. The low end is based on the EPA's mean estimate for CH4 emissions from natural gas systems published in the US greenhouse gas (GHG) inventory [3]. This estimate is a 'bottom-up estimate', where emissions are measured in the vicinity of individual pieces of equipment and statistical sampling methods and device counts are used to infer emissions rates for entire facilities, regions, or countries (e.g. [8, 32]). The top end of our range is from a review study [5] that covers both bottom-up measurements, as well as studies that measure total atmospheric CH4 enhancement in larger geographical areas ('top-down' studies, e.g. [10, 33]). The resulting range of leakage rates is 1.5%–4.9% (see supplementary material 1 available online at stacks.iop.org/ERL/14/124069/mmedia).
As indicated by this range, the results of bottom-up and top-down studies often disagree. Top-down estimates tend to exceed bottom-up estimates. Differences in measurement techniques partially explain the differences, but the reasons for the discrepancies are an open question and active area of research [16]. Another source of variation, whether in bottom-up or top-down estimates, is the choice of regions in which emissions are sampled. The low end of our range accounts for regional variability in the CH4 content of natural gas across production basins through a production-weighted national average [34]. However, other possible drivers of regional variability may not be captured, including local production levels, device operating practices and malfunctions, as well as regulations [16, 35]. For the high end of our range we therefore rely on a higher, regional top-down estimate from [5], with an understanding that this is not a national estimate but allows the estimated range to span both high and low observations in the literature, and recognizes that the national average rate is a subject of ongoing debate. In supplementary material 1 we discuss regional leakage rates that fall outside our baseline range, as well as CH4 emissions at power plants and attributional errors due to the co-production of natural gas and oil. These factors have a small effect relative to the range we already consider.
Estimated uncertainties in CH4 emissions from coal are smaller [3], and thus we consider only a single emission factor in our model (3 g CH4/kg coal, see supplementary material 1). We neglect the CH4 contribution from petroleum systems due to the only 1% contribution of petroleum to the final energy used to generate power in the US [36].
We assume that the contributions from natural gas and coal to power sector CH4 emissions (,
) change in proportion to the ratios of future to present power sector natural gas and coal consumption (
/
,
/PC(t0)), respectively:


Future coal and natural gas consumption in scenario 1 are based on the EPA's Clean Power Plan [37]. Retirements of older, less efficient coal and natural gas-fired power plants reflected in EPA's projection of primary energy use [37] contribute to CH4 emissions reductions per unit of electricity.
2.2. Computing
-equivalent emissions using equivalency metrics
In all scenarios we convert CH4 emissions from natural gas and coal use for electricity (,
) to CO2-equivalent emissions
:

where eK refers to power sector CO2 emissions and μ is an emissions equivalency metric. We use a set of metrics including the current default metric, the global warming potential with a 100 year time horizon (GWP(100)), and three alternative metrics proposed in the literature (see section 3.2 for metric values and a discussion of policy implications).
The GWP(100) is based on the ratio of the time-integrated radiative forcing per unit mass of CH4 and CO2:

where AM and AK are the radiative efficiencies of CH4 and CO2 [38], respectively, and and
are removal functions. Accounting for the CO2 contribution from the oxidation of fossil CH4 increases the metric value [38, 39].
The GWP(100)'s fixed time horizon leads to a constant impact value assigned to CH4 regardless of when emissions occur. In contrast, dynamic metrics place a higher weight per unit mass on CH4 emissions over time by reducing the time horizon over which impacts are evaluated as a threshold year is approached. This metric formulation can reduce overshoots of climate thresholds [40, 41]. For the instantaneous climate impact (ICI) metric (equation (5)), the threshold year is the intended radiative forcing stabilization year ts [40]. For the calculation of metric values, small increments of time are taken to approximate a continuous function [40] (see supplementary material 2).

The stabilization year 2050 was used for this analysis and falls within a 15 year range of ts defined by a set of possible emissions scenarios corresponding to a radiative forcing stabilization target of 3 W m−2 [40].
Removal functions take the general form [38]:

where gives the fraction of a gas emitted at time
remaining in the atmosphere at time t. For CH4, n = 1 and a0 = 0, thus removal follows an exponential decline. In the case of CO2, n = 3 and
and therefore the rate of removal changes with time. Parameter values represent a multi-model mean used in the Fifth Assessment Report (AR5) by the Intergovernmental Panel on Climate Change (IPCC) report (a1 = 0.224, a2 = 0.282, a3 = 0.282, τ1 = 394.4, τ2 = 36.54, τ3 = 4.304) [38, 42].
We also examine another dynamic metric, the time-dependent global temperature change potential (GTP), which is based on temperature change instead of radiative forcing. As shown in equation (7), the time-dependent GTP gives the ratio of temperature change in a future year te due to a pulse emission of gas in year t [43]:

The year te is a parameter to be chosen by policymakers and their constituents. We consider two evaluation years, 2050 and 2080. Details are given in supplementary material 2.
We calculate the fossil variants of the dynamic GTP using the same temperature model used to estimate temperature impacts of power sector GHG emissions scenarios. This approach produces metric values that match those in the IPCC AR5 report to within ± 10%–15% [38] for the GTP with a 20 and 50 year time horizon. See supplementary material 2 for a sensitivity analysis.
2.3. Modeling
reductions to meet
-equivalent targets
In scenarios 1–5, we model the CH4 emissions reductions needed to achieve a 32% reduction in power sector CO2-equivalent emissions over the 2005–2030 period. Target year CO2-equivalent emissions are set equal to a fraction 1-p(t, t') of base year emissions, with base year emissions e(t) (see equation (8)). We consider a target year of t' =2030, a base year of t = 2005, and an emissions reductions target of p = 0.32. The 32% goal considered in scenarios 1–5 is based on an expansion of the reduction target for CO2 emissions under the EPA's Clean Power Plan [37] to CO2-equivalent emissions, and is also chosen to be consistent with the US commitment in the Paris Agreement of a 26%–28% reduction in 2005 economy–wide CO2-equivalent emissions by 2025. The effect of applying other CO2-equivalent targets is discussed in supplementary material 6.
Base year CO2-equivalent emissions are defined by the EPA's CO2 and CH4 emissions estimate published in 2016 [3]. We apply a scaling factor to the natural gas contribution to CH4 emissions of approximately 3 (based on a review [5]), to capture leakage uncertainties, and use a metric value in the base year, μ(t), to convert CH4 emissions to CO2-equivalent emissions. The same approach is used for the year 2014, in which emissions reductions begin (see Methods 2.1 and supplementary material 1).

For each equivalency metric μ, the CH4 emissions allowed in year t' (, t' = 2030) were determined by subtracting target power sector CO2 emissions in that year (
) from
, and dividing the resulting CO2-equivalent emissions by the metric value
:

Natural gas leakage rates qNG are expressed as the mass fractions of dry natural gas production for the power sector that leaks along the supply chain:


We assume a CH4 density ρM (mass per unit volume) at temperature T = 15 °C and pressure p = 1 atm and employ the ideal gas law. We further assume a 85%–95%... volumetric CH4 content (fraction fM,NG) [44, 45]. The target level of CH4 emissions from natural gas eM,NG,allowed in the numerator of equation (10) is based on applying equal percent reductions to 2014 CH4 emissions from coal and natural gas. Natural gas leakage rate reductions can be interpreted as changes in the system–wide natural gas leakage rate (see supplementary material 1).
Note that although we consider a range of metrics, we do not include a version of the GWP with a shorter time horizon. Under any emissions target defined as a fraction of emissions in a historical year, constant value metrics will lead to the same percentage CH4 reductions as the GWP(100). A higher metric value increases the CO2-equivalent budget for CH4 in the target year but this effect will be offset by using the same value () to reconvert CO2-equivalent emissions to allowed CH4 emissions in 2030 (
, t' = 2030):
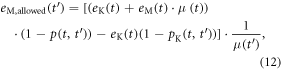
and

Using equations (12) and (13) we compute the fractional change in power sector CH4 emissions from the base year of the policy, :
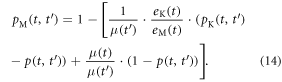
The metric that requires the largest emissions cut depends on the scenario. In scenario 1, where the target is met through natural gas leakage rate reductions, the ICI requires the largest reduction in power sector CH4 emissions because the metric values show the greatest change over time. In the scenarios 2–5, where the target is met through deeper CO2 reductions, the GTP(te = 2050) calls for the largest reduction in CO2 emissions because it places the largest weight on CH4 emissions in 2030 (see sections 3.3 and 3.4).
2.4. Modeling alternative scenarios to meet
-equivalent targets
We model alternative scenarios that also meet the 2030 CO2-equivalent emissions target, but without the reductions in the natural gas leakage rate assumed in scenario 1. Instead, CO2 emissions are reduced by more than 32% over the 2005–2030 period. Power sector CO2 emissions in 2030 therefore equal:

where is computed as in scenario 1 (equation (8)). In scenarios 2–5, CO2 emissions from coal electricity generation are reduced by replacing coal with other electricity sources, in order to meet the CO2-equivalent target. We label these sources 'carbon–free' but their lifecycle emissions are not zero [46] and are accounted for in the analysis (see supplementary material 3). Additional scenarios (6–9) instead replace natural gas with other electricity sources and are discussed in supplementary material 3. The effect of varying the CO2-equivalent target is discussed in supplementary material 6.
2.5. Temperature model
We model concentration and temperature responses to CO2 and CH4 emissions separately in all scenarios using exponential impulse response functions (IRFs) fitted to the results of atmosphere-ocean coupled general circulation models (see supplementary material 5). This simplified representation of the climate system has been shown to closely reproduce the results of more complex models such as fully coupled atmosphere-ocean general circulation models (AOGCMs) [47–49]. To isolate the warming impacts of US electricity sector emissions, we do not consider emissions by other sectors, and we compare warming impacts of US emissions to those under IPCC's Representative Concentration Pathway 2.6.
Equation (16) gives the linearized IRF for relating the change in global mean surface temperature (ΔT) to the change in radiative forcing (). This linearization is adequate for an RF range between 3 and 4 W m−2, which corresponds to a doubling of atmospheric CO2 concentration [47].

R(t) is represented as a sum of three exponentials (equation (17)) with different amplitudes and time constants:

where r0 is the height of the RF step function used to simulate the climate response to a doubling of CO2 concentration. This functional form has been shown to provide the best fit to simulation results for an ensemble of 20 AOGCMs that participated in phase 5 of the Coupled Model Intercomparison Project (CMIP5) [48].
The amplitudes Aj and time constants τj taken from CMIP5 are A1 = 0.60, τ1 = 0.655, A2 = 0.86, τ2 = 9.46, A3 = 1.04 and τ3 = 257.1 [48]. We use an equilibrium climate sensitivity (ECS) of 2.5 °C (i.e. the long-term equilibrium temperature response to a doubling of atmospheric CO2 concentration), which is within the ECS range considered as likely in IPCC's AR4 (2 °C–4.5 °C) and AR5 (1.5 °C–4.5 °C) and was supported by a study incorporating the full observational record from 1765 to 2011 [50]. We examine different ECS choices in supplementary material 5.
3. Results
3.1.
emissions impacts of a
-focused policy
In many modeled scenarios, natural gas use continues to displace coal in the US power sector over the next two decades [37, 51]. In one scenario where power sector CO2 emissions decline by 32% below 2005 levels by 2030, the Environmental Protection Agency (EPA) projects a 21% growth in natural gas electricity between 2014 and 2030, and a 28% decline in coal electricity (figure 1(a)) [37]. We first explore this example (scenario 1, see Methods 2.1), and then examine other scenarios. Scenario 1 is not a projection but rather represents one possible outcome under a policy that reduces CO2 emissions while continuing to rely on CH4-emitting fuels.
Figure 1. Coal and natural gas electricity generation and CO2 and CH4 emissions (eK, eM) from electricity in scenario 1 without natural gas leakage rate mitigation. Coal electricity generation (black) is projected to decrease and natural gas electricity (purple) to increase (a). Historical trends are based on EIA data [36]. The lower CO2 intensity of natural gas leads to (b) decreasing power sector CO2 emissions. The line in (b) reaches the 2030 target under the US EPA's Clean Power Plan, a 32% reduction from 2005, while also reflecting EPA projections for 2020 and 2025 CO2 emissions under this policy [88]. The decrease in CH4 emissions from coal is offset by rising CH4 emissions from natural gas, resulting in relatively flat trends to 2030 [5, 37] for (c) low (1.5%) and (d) high (4.9%) natural gas leakage rates.
Download figure:
Standard image High-resolution imagePower sector CH4 emissions in scenario 1 are shown in figures 1(c) and (d). The decline in CH4 emissions from coal production is not substantial enough to offset increasing CH4 emissions from natural gas. The resulting trend in power sector CH4 emissions is nearly flat. The low estimate of the contribution of natural gas (63%) to power sector CH4 emissions is shown in figure 1(c). It is based on estimates for CH4 emissions from natural gas systems and coal mining from the US GHG inventory, scaled in proportion to the share of coal and natural gas used for electricity [3]. For the high estimate (figure 1(d)) we multiply the natural gas contribution by an adjustment factor that reflects reviews of measurements [5, 52, 53], many of which exceed estimates in the US GHG inventory [5–8, 10–12, 35, 52, 53] (Methods 2.1).
3.2.
-equivalent emissions impacts of a
-focused policy
Having estimated the CH4 emissions associated with scenario 1, we ask how they can be counted toward a CO2-equivalent emissions target. Policy goals are often framed in terms of CO2-equivalent emissions, as this allows for a single-unit comparison of commitments across locations and sectors emitting different ratios of GHGs such as CO2 and CH4. Measuring all emissions on a single CO2-equivalent scale requires a metric for converting non-CO2 emissions to CO2-equivalent units. Selecting an emissions equivalency metric for this purpose requires the choice of a climate impact indicator (e.g. radiative forcing, temperature change, economic damages) and a time horizon [40, 54–57]. Many different metrics have been proposed in the literature [40, 43, 56, 58–64]. However, despite these many proposals, most policies use one metric, the global warming potential with a 100 year time horizon (GWP(100)) (see Methods 2.2, equation (4)). This metric was originally proposed as a placeholder [43, 65], but its use has nonetheless persisted over time. The GWP(100) was adopted in the Kyoto protocol, and it was used in most country pledges to the Paris Agreement [66, 67]. As shown in figure 2(a), the GWP(100)'s fixed time horizon leads to a constant impact value assigned to CH4 regardless of when emissions occur. This formulation has been criticized for underemphasizing the decadal scale radiative forcing impacts of CH4, which is shorter-lived but has a higher radiative efficiency than CO2 (e.g. [40, 41, 60, 68, 69]).
Figure 2. Metric values and CO2-equivalent emissions reductions between 2005 and 2030 under scenario 1 and without natural gas (NG) leakage rate mitigation. The metrics emphasize the climate impacts of CH4 over different timescales. This leads to different achieved CO2-equivalent emissions cuts unless the natural gas leakage rate is reduced (see figure 4(a)). (a) The ICI(ts = 2050) (red), GTP(te = 2050) (blue), and GTP(te = 2080) (green) assign a higher CO2-equivalent mass to CH4 as a climate target is neared. The GWP(100) (magenta) is constant. (b) Estimated CO2-equivalent cuts (below 2005 levels) in 2030 are further from the target (32%, dashed line) under the ICI(ts = 2050) and GTP(te = 2050), and closer under the GWP(100) and GTP(te = 2080). Light (dark) bars show results for low (high) natural gas leakage rates [3, 5].
Download figure:
Standard image High-resolution imageAnother approach to selecting an emissions equivalency metric is to evaluate its performance against the climate change mitigation goal of a policy [40, 41]. This approach can allow a decision-maker to weigh the benefits and drawbacks of choosing different indicators and time horizons. For example, a policy maker may choose to use a metric with a time horizon that shortens as a pre-determined threshold in radiative forcing or temperature is approached, thereby capturing short-term impacts as the threshold is approached [40, 41].
Although current climate policies predominantly use the GWP(100), it is relevant for policymakers and other decision makers to understand the impacts of choosing this over other metrics. This importance is reflected in an active debate about which metrics to use in policy [70, 71]. Because of this we consider in our analysis not just one but a set of metrics. This set covers the range in conversion factors resulting from different emissions equivalency metric formulations proposed in the literature (see Methods 2.2). As shown in figure 2(a), the values of the metrics in our set range from high to low and static to time-dependent (see equations (4)–(7) in Methods).
When we use this set of metrics to evaluate scenario 1, where only CO2 is mitigated, we find that CH4 emissions add substantially to CO2-equivalent emissions (figure 2(b)). While CO2 emissions decline by 32% over the 2005–2030 period, the CO2-equivalent changes are substantially less when applying the ICI and GTP metrics (figure 2(b)). The CO2-equivalent emissions cuts under the GWP(100) come closest to achieving the 32% target. However, as shown in the section on 'Temperature impacts', the near-term warming impacts are higher when applying the GWP(100) to regulate CO2-equivalent emissions than when using the other metrics. In the next section we explore the CH4 mitigation that would be needed to reach a 32% cut in CO2-equivalent emissions across a range of emissions equivalency metrics and technology transition pathways.
3.3.
reductions to meet
-equivalent targets
Having examined the CO2-equivalent emissions in scenario 1, where CO2 alone is regulated, we now simulate the effects of adding a policy that regulates CH4. We first examine scenario 1, and determine the CH4 cuts required to reach a reduction in CO2-equivalent emissions of 32% below 2005 levels by 2030. In scenario 1, CO2 is also reduced by 32% over this period [37]. We then consider other scenarios that achieve deeper CO2 cuts to reach the same 32% cut in CO2-equivalent emissions by 2030, without requiring a reduction in the natural gas leakage rate. Our modeling approach is described in Methods section 2.3. The results are presented both in terms of a reduction in CH4 from electricity, including contributions from natural gas and coal, and a reduction in the natural gas leakage rate from today's (2014) levels (equation (10) in Methods).
The emissions target examined, a 32% reduction in 2005 CO2-equivalent emissions by 2030, is meant to represent a power sector goal that would help enable the US to meet its economy-wide commitments to the Paris Agreement. In the US nationally determined contribution (NDC) to the Paris Agreement, the economy-wide CO2-equivalent emissions goal is a 26%–28% reduction by 2025, relative to 2005 levels, and the NDC also references a more ambitious target to cut emissions by 80% by 2050 [24, 72]. Meeting these goals would likely require a decarbonization of electricity at rates comparable to those analyzed here [73, 74]. In supplementary material 6, we discuss the impact on our results of adjusting the target.
We find that scenario 1 would require natural gas leakage rate reductions of roughly 40%–90% over the 2014–2030 period to meet the 2030 CO2-equivalent target (figure 6). The low end of this range is based on using the GWP(100), and the upper end is based on using the ICI. The target range for 2030 leakage rates is 0.2%–0.9% when assuming low natural gas leakage rates in 2005, which is the base year for the CO2-equivalent target. The 2030 target range is 0.5%–2.6% for high natural gas leakage rates. See figure 6 for results based on low leakage rates and supplementary table 3 for the full set of results for all metrics. As shown in figure 4, the required changes in overall power sector CH4 emissions range from 30% to 90% over the 2005–2030 period, up to three times greater than the 32% CO2 cut. The decline in total power sector CH4 emissions would also require a reduction in CH4 from coal mining relative to 2014, both in absolute terms and per unit of electricity generated (supplementary material 3).
Figure 3. 2030 electricity supply scenarios to meet the 2030 CO2-equivalent target (32% reduction, 2005–2030). Scenario 1 combines a 32% CO2 reduction from 2005 to 2030 with natural gas leakage rate (NG LR) mitigation. The amount of NG leakage rate mitigation depends on the metric. The results shown here are for the high NG leakage rate of 4.9% in 2014 (figure 1), and a 2030 target leakage rate range of 0.5% under the ICI and 2.6% under the GWP(100). For a low leakage rate (1.5% in 2014), the 2030 target ranges from 0.2% under the ICI to 0.9% under the GWP(100) (see figure 6 and supplementary table 3). In scenario 1, the electricity mix is based on a projection by the US EPA [37]. Scenarios 2–5 meet the 2030 CO2-equivalent target through deeper CO2 cuts (using less coal electricity as compared to scenario 1), and require no NG leakage rate changes relative to today's estimated levels. CO2 emissions reductions required by 2030 in scenarios 2–5 range from 33% to 48% relative to 2005 levels, and from 20% to 38% relative to 2014 levels, for the full range of low to high leakage rates considered (see supplementary table 2 for low leakage rate results). The amount of carbon-free energy depends on the metric and the leakage rate (high leakage rate shown here, see supplementary table 1 for low leakage rate results). Total estimated 2030 electricity supply is 4122 TWh in all scenarios, including a fixed contribution from hydroelectric power, nuclear fission, and other sources [37]. Increasing electricity generation from hydroelectric power or nuclear fission instead of renewables to achieve the deeper CO2 reductions in scenarios 2–5 would result in similar required amounts of carbon-free energy in 2030, as would shifting away from natural gas instead of coal (see supplementary material 3, figure 2).
Download figure:
Standard image High-resolution imageFigure 4. 2005–2030 electricity CH4 and CO2 changes to meet the 2030 CO2-equivalent target. Electricity CH4 (CO2) emissions represent the sum of CH4 (CO2) emissions from coal and natural gas. (a) In scenario 1, CH4 is reduced through natural gas (NG) leakage rate reductions and cuts in CH4 from declining coal electricity generation. Required CH4 emissions changes do not depend on the NG leakage rate (equal light and dark bars) because a higher leakage rate also increases the emissions budget for CH4 emissions. (b) CO2 changes are shown for scenarios 2–5, in which CO2 is reduced through a shift away from coal relative to scenario 1. CO2 cuts are adjusted to offset CO2-equivalent emissions from CH4 and therefore depend on the metric and the NG leakage rate. Shifting away from natural gas instead of coal generates similar results (see supplementary material 3).
Download figure:
Standard image High-resolution image3.4. Deeper
reductions to meet
-equivalent targets
An alternative to cleaning up the natural gas supply chain is to pursue CO2 cuts that are deeper than 32%, in order to meet the 2030 CO2-equivalent target despite CH4 emissions from natural gas and coal. We explore this approach in scenarios 2–5 (figure 3), which are designed to require no reduction in the natural gas leakage rate. Natural gas electricity generation and resulting CH4 emissions are held constant relative to scenario 1, and coal electricity is reduced until the CO2-equivalent target is met (see Methods section 2.4). Generation from non-hydro renewables is increased to supply carbon-free energy. Expanding nuclear or hydro would lead to similar results, with small differences due to the variation in non-zero life-cycle emissions intensities of 'carbon-free' sources (supplementary material 3). Results are given in units of energy (TWh) to indicate that these results represent outcomes of energy transitions needed to meet climate policy goals, rather than power capacity changes needed to reach these outcomes. Examining possible implementation strategies is beyond the scope of this paper, and would require more detailed regional analyses of supply mix changes. Instead our analysis can inform the end goal of these strategies.
As shown in figure 4(b), the required CO2 cuts can be substantially greater than the 32% assumed in scenario 1. The metric choice is the most important determinant of the amount of carbon-free energy needed to meet the 2030 CO2-equivalent target, followed by the natural gas leakage rate. Applying the GTP(te = 2050) and the ICI requires up to 50% more carbon-free power in 2030 than using the GWP(100) (figure 3), and roughly twice as much as in scenario 1.
A wider set of scenarios are discussed in supplementary material 3 and 6, spanning a range of conditions that may be present in other policy and market contexts. We consider the effects of prioritizing coal over natural gas, adopting different emissions targets, and realizing different electricity demand levels. In scenarios 1–5 above, the CO2 cuts are equal to or greater than the percent reduction in CO2-equivalent emissions. In the supplementary information we also consider scenarios where the CO2 cuts are less ambitious than the target reduction in CO2-equivalent emissions. A similar decision emerges across these scenarios, between a strategy that emphasizes a CH4 clean-up or deeper CO2 cuts through faster expansion of carbon-free power.
3.5. Temperature impacts
Here we evaluate the temperature impacts of scenarios that meet the 2030 CO2-equivalent target. We find that metric choices affect warming impacts under the policy considered here. Metrics that require the greatest CH4 or CO2 cuts—the ICI or the GTP(te = 2050) in the set of metrics examined—also achieve the greatest temperature reductions in 2030 relative to a no-policy scenario (figure 5). This no-policy scenario is based on EPA's projection of primary energy use without CO2 and CH4 regulations [37].
Figure 5. Comparison of the avoided warming achieved in 2030 through the use of different metrics to implement the CO2-equivalent target. Warming is shown relative to a no-policy scenario and normalized to the most emissions-mitigating metric. This metric achieves the largest warming reduction (100%, supplementary material 5) relative to the no-policy scenario. Panel (a) shows scenario 1 with natural gas (NG) leakage rate reductions and (b) shows scenarios 2–5, without reductions in the NG leakage rate. Light (dark) shaded bars show results for low (high) NG leakage rates in the year 2030. The difference increases for metrics allowing more CH4 emissions (e.g. the GWP(100)). Using the the ICI or the GTP(te = 2050) reduces temperature twice as much compared to the no-policy scenario as using the GWP(100) (red/blue bars versus magenta bars). Absolute warming impacts would be magnified for a global scenario. Results are similar for scenarios where deeper CO2 cuts are achieved through a shift away from natural gas instead of coal assumed in scenarios 2–5 (supplementary material 3). Temperature and radiative forcing profiles over time are shown in supplementary material 5.
Download figure:
Standard image High-resolution imageAs shown in figure 5, using the ICI or the GTP(te = 2050) to reach the 2030 CO2-equivalent target can reduce warming in 2030 by twice as much as the GWP(100), as compared to the no-policy scenario. The absolute effects on global mean temperature are limited, since we are considering a near term (2030) emissions target for one country's electricity sector (see supplementary material 5). But relative to the impact of the policy, the choice of metric makes a significant difference.
Both strategies for achieving the 32% CO2-equivalent emissions cut—emphasizing CH4 or CO2 reductions—result in similar temperature profiles between now and 2030, but diverge in later years. Due to the much longer atmospheric lifetimes of CO2, the strategy that emphasizes reducing CO2 is much more effective at mitigating temperature increases in the longer term. This effect is studied in supplementary material 5 by examining the temperature profiles resulting from the two strategies if all emissions cease in the year 2030.
Finally, we find that the uncertainties in CH4 leakage estimates and metric choice have a similar magnitude of impact on the 2030 temperature change. In other words, changing the metric can have a similar impact on the estimated temperature profile to changing the CH4 leakage rate from the high to the low values considered in this analysis.
3.6. Feasibility of
mitigation
How difficult will it be to achieve CH4 cuts at the scale suggested by this analysis? Here we compare natural gas leakage rates that are consistent with the 2030 CO2-equivalent target to past natural gas leakage rate estimates in order to gain historical perspective. We also briefly discuss different mitigation strategies and past examples in the transportation sector.
As shown in figure 6, the range of historical estimates is significantly above the natural gas leakage rates required to meet the 2030 CO2-equivalent target. The target leakage rates are lowest for the dynamic metrics considered. The substantial scatter in historical estimates point to large and persistent measurement uncertainties, even when considering one data source alone [3]. Multiple data points are sometimes shown for the same year, indicating adjustments of estimates in the US EPA's GHG inventory as protocols changed.
Figure 6. Historical estimates of natural gas (NG) leakage rates qNG (circles) and leakage rates that meet the 2030 CO2-equivalent target under scenario 1 (squares). Target leakage rates are lower than historical estimates, which are based on the EPA's GHG inventories published since 1998 (e.g. [3]), and a 95% CH4 content of natural gas. Most target leakage rates are also lower than estimated leakage rates under CH4 regulations proposed in the US, which target a 40%–45% reduction from 2012 levels by 2025. Other estimates of current leakage rates are higher than those shown here [5], and have informed the high end (4.9%) of the range used in figures 1–5. Target leakage rates for all scenarios and parameters are given in supplementary table 3.
Download figure:
Standard image High-resolution imageWhether these reductions in the natural gas leakage rate are realistic will depend on the costs of monitoring, mitigating, and preventing leaks [75, 76], particularly if aiming for the lowest leakage rates suggested by the dynamic metrics (figure 6). Recent literature suggests that targeting disproportionately high-emitting equipment, point sources, or regions, often referred to as 'super-emitters', may be an effective mitigation strategy [5, 8, 10, 32, 52, 75–80]. However, knowledge of why these disproportionate emissions rates occur is still limited. Studies of individual regions point to equipment malfunctions and routine high-emissions operations as causes of disproportionate emissions, but these studies have also suggested that major gaps remain in our understanding of both spatial and temporal variation in emissions rates from point sources [16, 77, 78, 81, 82]. These gaps add uncertainty as to whether the same CH4 mitigation strategies will work across different sites and production regions, which will likely impact the cost reductions achievable.
One past example of success in monitoring and mitigating point source emissions, including from older, high-emitting equipment, is the reduction in carbon monoxide emissions from vehicles [83]. However, vehicles are mobile and thus eventually pass by even sparsely distributed sensors. In contrast, sensors in the stationary natural gas supply chain would need to travel to the source. To lower the costs of comprehensive monitoring, innovations will likely be needed both in the 'soft' technologies to enable effective CH4 mitigation (e.g. knowledge embodied in firms and institutions, skills of technology inspectors, web-tools for sharing emissions data) and in hardware (e.g. sensors, cameras).
4. Conclusions and discussion
This research examines the scale of CH4 mitigation required when natural gas is used as part of an energy supply portfolio to achieve climate policy goals. We consider a scenario where CO2 is reduced by 32% over the 2005–2030 period (scenario 1), and find that achieving the same 32% percent reduction in CO2-equivalent emissions would require substantial reductions in the natural gas leakage rate from today's levels. We then explore a set of alternative scenarios and candidate technology pathways (scenarios 2–5) to ask how climate policy goals could be met without reductions in the natural gas leakage rate, by reducing CO2 faster than in the first scenario.
The first scenario calls for power sector CH4 emissions percent reductions that are substantially larger than the required CO2 reductions. In this scenario, CO2 emissions would need to be reduced by roughly 20% relative to today's levels by 2030, while the required CH4 reductions would range from 30–90%. Alternatively, in scenarios 2–5, this CH4 mitigation effort could be avoided through deeper CO2 reductions by 2030, of 33–48% from 2005 levels and 20–38% from 2014 levels, and a more rapid growth of carbon-free power. In this case, natural gas leakage rate reductions would not be required despite a 20%–30% share of natural gas in the 2030 electricity mix. These results reveal the features of two distinct strategies that emphasize either a CH4 clean-up effort or deeper CO2 cuts.
In each scenario we use a set of emissions equivalency metrics from the literature to account for CO2 and CH4 emissions on a single CO2-equivalent scale. Across the scenarios considered, we find that the emissions equivalency metric choice is an important determinant of the amount of CH4 mitigation or carbon-free power needed to meet the 2030 target. Dynamic metrics call for much more aggressive CH4 reductions or faster transitions to carbon-free electricity, and can avoid up to twice as much warming in 2030 under the same CO2-equivalent target. These estimated differences in avoided warming are small in absolute terms but are significant relative to the impact on the near-term rate of warming of the one-sector policy considered here.
Although most commitments under the Paris Agreement use the GWP(100) to compare CO2 and non-CO2 GHGs in CO2-equivalent terms, alternative metrics are a subject of active debate in policy and environmental impact research [60, 70, 84]. For example, recent research has called for reporting CO2-equivalent emissions using multiple metrics to better represent the effects of different time horizons, physical and economic impact indicators, and modeling uncertainties [70, 71]. Here we show how multiple metrics can be used to evaluate the benefits and drawbacks of policies that assign differing levels of importance to mitigating CH4.
Longer-term mitigation plans are also important to consider in evaluating the mitigation options identified in this work. Pursuing deeper CO2 reductions instead of a CH4 clean-up effort would not only achieve the 2030 CO2-equivalent target but would also allow the US to move closer to the reduction in fossil fuel use needed to reach 2050 targets. For example, all scenarios presented in the US Mid-Century Energy Strategy reach a natural gas share lower than 10% of electricity in 2050 [74], and many feature over three quarters of electricity supplied by carbon-free sources. Under these scenarios, investments in improving natural gas infrastructure, for example by learning how to detect, repair, and avoid CH4 leaks, might see limited use beyond the next couple of decades, since natural gas would be phased out to meet more stringent climate goals.
Our quantitative results apply to the US power sector, but other sectors and regions face similar choices between improving existing, leaky technology supply chains, and phasing these technologies out more rapidly. One example is hydrofluorocarbons (HFCs), where there is currently a debate about preventing leaks through better designs versus transitioning to other coolant technologies that do not use HFCs (e.g. [85–87]). The conceptual approach developed here could be extended to analyze such technology choice problems under emissions targets covering multiple GHGs.
Acknowledgments
We thank Victor Ocaña for his contributions to modeling temperature impacts; Morgan Edwards for ongoing discussions of natural gas infrastructure and emissions metrics; Marco Miotti for input on graphics; Susan Solomon for discussions regarding the Clean Air Act and vehicle pollutant emissions; and Larry Birenbaum for discussions of the EPA Clean Power Plan and CH4 regulation. We thank the MIT Environmental Solutions Initiative for funding this research. We also gratefully acknowledge support from MIT's Policy Lab at the Center for International Studies.