Abstract
High-contrast optically detected magnetic resonance is a valuable property for reading out the spin of isolated defect colour centres at room temperature. Spin-active single defect centres have been studied in wide bandgap materials including diamond, SiC and hexagonal boron nitride, each with associated advantages for applications. We report the discovery of optically detected magnetic resonance in two distinct species of bright, isolated defect centres hosted in GaN. In one group, we find negative optically detected magnetic resonance of a few percent associated with a metastable electronic state, whereas in the other, we find positive optically detected magnetic resonance of up to 30% associated with the ground and optically excited electronic states. We examine the spin symmetry axis of each defect species and establish coherent control over a single defect’s ground-state spin. Given the maturity of the semiconductor host, these results are promising for scalable and integrated quantum sensing applications.
This is a preview of subscription content, access via your institution
Access options
Access Nature and 54 other Nature Portfolio journals
Get Nature+, our best-value online-access subscription
$29.99 / 30 days
cancel any time
Subscribe to this journal
Receive 12 print issues and online access
$259.00 per year
only $21.58 per issue
Buy this article
- Purchase on Springer Link
- Instant access to full article PDF
Prices may be subject to local taxes which are calculated during checkout





Similar content being viewed by others
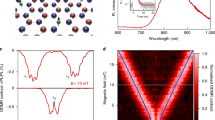
Data availability
All data are available on eCommons: Digital Repository at Cornell (https://doi.org/10.7298/azf0-hc03). Source data are provided with this paper.
Code availability
All codes for analysing data in this study are available on eCommons: Digital Repository at Cornell (https://doi.org/10.7298/azf0-hc03).
References
Köhler, J. et al. Magnetic resonance of a single molecular spin. Nature 363, 242–244 (1993).
Wrachtrup, J., von Borczyskowski, C., Bernard, J., Orrit, M. & Brown, R. Optical detection of magnetic resonance in a single molecule. Nature 363, 244–245 (1993).
Degen, C. L. Scanning magnetic field microscope with a diamond single-spin sensor. Appl. Phys. Lett. 92, 243111 (2008).
Taylor, J. M. et al. High-sensitivity diamond magnetometer with nanoscale resolution. Nat. Phys. 4, 810–816 (2008).
Rondin, L. et al. Magnetometry with nitrogen-vacancy defects in diamond. Rep. Prog. Phys. 77, 56503 (2014).
Gottscholl, A. et al. Spin defects in hBN as promising temperature, pressure and magnetic field quantum sensors. Nat. Commun. 12, 4480 (2021).
Dolde, F. et al. Electric-field sensing using single diamond spins. Nat. Phys. 7, 459–463 (2011).
Acosta, V. M. et al. Temperature dependence of the nitrogen-vacancy magnetic resonance in diamond. Phys. Rev. Lett. 104, 070801 (2010).
Toyli, D. M. et al. Measurement and control of single nitrogen-vacancy center spins above 600 K. Phys. Rev. X 2, 031001 (2012).
Doherty, M. W. et al. The nitrogen-vacancy colour centre in diamond. Phys. Rep. 528, 1–45 (2013).
Jelezko, F. & Wrachtrup, J. Single defect centres in diamond: a review. Phys. Status Solidi A 203, 3207–3225 (2006).
Widmann, M. et al. Coherent control of single spins in silicon carbide at room temperature. Nat. Mater. 14, 164–168 (2015).
Koehl, W. F., Buckley, B. B., Heremans, F. J., Calusine, G. & Awschalom, D. D. Room temperature coherent control of defect spin qubits in silicon carbide. Nature 479, 84–87 (2011).
Gottscholl, A. et al. Initialization and read-out of intrinsic spin defects in a van der Waals crystal at room temperature. Nat. Mater. 19, 540–545 (2020).
Gao, X. et al. High-contrast plasmonic-enhanced shallow spin defects in hexagonal boron nitride for quantum sensing. Nano Lett. 21, 7708–7714 (2021).
Chejanovsky, N. et al. Single-spin resonance in a van der Waals embedded paramagnetic defect. Nat. Mater. 20, 1079–1084 (2021).
Stern, H. L. et al. Room-temperature optically detected magnetic resonance of single defects in hexagonal boron nitride. Nat. Commun. 13, 618 (2022).
Rondin, L. et al. Magnetometry with nitrogen-vacancy defects in diamond. Rep. Prog. Phys. 77, 056503 (2014).
Mathur, N. et al. Excited-state spin-resonance spectroscopy of \({{\mathrm{V}}}_{{\mathrm{B}}}^{-}\) defect centers in hexagonal boron nitride. Nat. Commun. 13, 3233 (2022).
Mu, Z. et al. Excited-state optically detected magnetic resonance of spin defects in hexagonal boron nitride. Phys. Rev. Lett. 128, 216402 (2022).
Reimers, J. R. et al. Photoluminescence, photophysics, and photochemistry of the VB– defect in hexagonal boron nitride. Phys. Rev. B 102, 144105 (2020).
Xu, X. et al. Greatly enhanced emission from spin defects in hexagonal boron nitride enabled by a low-loss plasmonic nanocavity. Nano Lett. 23, 25–33 (2023).
Qian, C. et al. Unveiling the zero-phonon line of the boron vacancy center by cavity-enhanced emission. Nano Lett. 22, 5137–5142 (2022).
Lukin, D. M. et al. 4H-silicon-carbide-on-insulator for integrated quantum and nonlinear photonics. Nat. Photon. 14, 330–334 (2020).
Li, Q. et al. Room-temperature coherent manipulation of single-spin qubits in silicon carbide with a high readout contrast. Natl Sci. Rev. 9, nwab122 (2022).
Wang, J. et al. Efficient generation of an array of single silicon-vacancy defects in silicon carbide. Phys. Rev. Appl. 7, 064021 (2017).
Wang, J.-F. et al. Coherent control of nitrogen-vacancy center spins in silicon carbide at room temperature. Phys. Rev. Lett. 124, 223601 (2020).
Burk, A. A. Jr et al. SiC and GaN wide bandgap semiconductor materials and devices. Solid-State Electron. 43, 1459–1464 (1999).
Milligan, J. W. et al. SiC and GaN wide bandgap device technology overview. In Proc. 2007 IEEE Radar Conference 960–964 (IEEE, 2007).
Chen, K. J. et al. GaN-on-Si power technology: devices and applications. IEEE Trans. Electron Devices 64, 779–795 (2017).
Mishra, U., Parikh, P. & Wu, Y.-F. AlGaN/GaN HEMTs-an overview of device operation and applications. Proc. IEEE 90, 1022–1031 (2002).
Berhane, A. M. et al. Bright room-temperature single-photon emission from defects in gallium nitride. Adv. Mater. 29, 1605092 (2017).
Berhane, A. M. et al. Photophysics of GaN single-photon emitters in the visible spectral range. Phys. Rev. B 97, 165202 (2018).
Geng, Y. et al. Dephasing by optical phonons in GaN defect single-photon emitters. Sci. Rep. 13, 8678 (2023).
Geng, Y., Jena, D., Fuchs, G. D., Zipfel, W. R. & Rana, F. Optical dipole structure and orientation of GaN defect single-photon emitters. ACS Photon. 10, 3723–3729 (2023).
Geng, Y. & Nomoto, K. Ultrafast spectral diffusion of GaN defect single photon emitters. Appl. Phys. Lett. 123, 174002 (2023).
Epstein, R. J., Mendoza, F. M., Kato, Y. K. & Awschalom, D. D. Anisotropic interactions of a single spin and dark-spin spectroscopy in diamond. Nat. Phys. 1, 94–98 (2005).
Exarhos, A. L., Hopper, D. A., Patel, R. N., Doherty, M. W. & Bassett, L. C. Magnetic-field-dependent quantum emission in hexagonal boron nitride at room temperature. Nat. Commun. 10, 222 (2019).
Lee, S.-Y. et al. Readout and control of a single nuclear spin with a metastable electron spin ancilla. Nat. Nanotechnol. 8, 487–492 (2013).
Guo, N.-J. et al. Coherent control of an ultrabright single spin in hexagonal boron nitride at room temperature. Nat. Commun. 14, 2893 (2023).
Acknowledgements
We thank L. van Deurzen, D. Jena and H. G. Xing for useful discussions and for supplying the GaN substrates. We thank B. McCullian, N. Mathur, A. D’Addario and J. Kuan for helpful discussions on the physics and microwave experiments. This work was supported by the Cornell Center for Materials Research (CCMR), a National Science Foundation (NSF) Materials Research Science and Engineering Center (DMR-1719875; J.L., Y.G., F.R. and G.D.F.). We also acknowledge support through the Cornell Engineering Sprout programme (J.L.). Preliminary work was supported by the NSF TAQS programme (ECCS-1839196; J.L., Y.G., F.R. and G.D.F.). This work was performed in part at the Cornell NanoScale Science & Technology Facility (CNF), a member of the National Nanotechnology Coordinated Infrastructure (NNCI), which is supported by the NSF (grant NNCI-2025233).
Author information
Authors and Affiliations
Contributions
All authors conceived the experiment. J.L. and G.D.F. developed the experimental approach. J.L. and Y.G. prepared samples. J.L. made measurements. J.L. and G.D.F. analysed the experiment. J.L. and G.D.F. wrote the paper. All authors reviewed the paper.
Corresponding author
Ethics declarations
Competing interests
The authors declare no competing interests.
Peer review
Peer review information
Nature Materials thanks Fedor Jelezko and the other, anonymous, reviewer(s) for their contribution to the peer review of this work.
Additional information
Publisher’s note Springer Nature remains neutral with regard to jurisdictional claims in published maps and institutional affiliations.
Supplementary information
Supplementary Information
Supplementary Figs. 1–9, Discussion and Tables 1 and 2.
Source data
Source Data Fig. 3
Source data for Fig. 3a,b (cw-ODMR as a function of microwave drive frequency and magnetic field).
Source Data Fig. 5
Unnormalized source data for Fig. 5b–d (Rabi oscillations for the lower-frequency, intermediate-frequency and higher-frequency spin resonances of defect no. 6).
Rights and permissions
Springer Nature or its licensor (e.g. a society or other partner) holds exclusive rights to this article under a publishing agreement with the author(s) or other rightsholder(s); author self-archiving of the accepted manuscript version of this article is solely governed by the terms of such publishing agreement and applicable law.
About this article
Cite this article
Luo, J., Geng, Y., Rana, F. et al. Room temperature optically detected magnetic resonance of single spins in GaN. Nat. Mater. 23, 512–518 (2024). https://doi.org/10.1038/s41563-024-01803-5
Received:
Accepted:
Published:
Issue Date:
DOI: https://doi.org/10.1038/s41563-024-01803-5
This article is cited by
-
Optical manipulation of spin resonance in gallium nitride
Nature Photonics (2024)
-
Identifying single spin defects in gallium nitride
Nature Materials (2024)
-
Spectral stability of V2 centres in sub-micron 4H-SiC membranes
npj Quantum Materials (2024)