Loss of foxo rescues stem cell aging in Drosophila germ line
Abstract
Aging stem cells lose the capacity to properly respond to injury and regenerate their residing tissues. Here, we utilized the ability of Drosophila melanogaster germline stem cells (GSCs) to survive exposure to low doses of ionizing radiation (IR) as a model of adult stem cell injury and identified a regeneration defect in aging GSCs: while aging GSCs survive exposure to IR, they fail to reenter the cell cycle and regenerate the germline in a timely manner. Mechanistically, we identify foxo and mTOR homologue, Tor as important regulators of GSC quiescence following exposure to ionizing radiation. foxo is required for entry in quiescence, while Tor is essential for cell cycle reentry. Importantly, we further show that the lack of regeneration in aging germ line stem cells after IR can be rescued by loss of foxo.
https://doi.org/10.7554/eLife.27842.001eLife digest
Stem cells are unspecialized cells that have the unique ability to replace dead cells and repair damaged tissues. To give rise to new cells, stem cells need to divide. This process, known as the cell cycle, includes several stages and is regulated by many different genes.
For example, in many organisms, a gene called foxo helps cells respond to stress and to regulate the cell cycle and cell death. Defects in this gene have been linked to age-related diseases, such as cancer and Alzheimer’s disease. Previous research has shown that foxo can also regulate Tor – a gene that helps cells to divide and grow.
As we age, stem cells become less efficient at regenerating tissues, especially after exposure to toxins and radiation. However, until now, it was not known how stem cells control their division after injury and during aging, and what role these two genes play in injured and aging stem cells.
Now, Artoni, Kreipke et al. used germline stem cells from fly ovaries to investigate how young and old stem cells respond to injury. In young flies, foxo paused the cell cycle of the damaged stem cells. After 24 hours, Tor was able to overcome the action of foxo, and the stem cells resumed dividing and regenerating the damaged tissue. However, in old stem cells, foxo and Tor were misregulated and the stem cells could not restart dividing or repairing tissue after injury. When the levels of foxo in old stem cells were experimentally reduced, their ability to regenerate the tissue was restored.
These discoveries provide new insights into how stem cells respond to injury and suggest that stem cell aging may be a reversible process. A next step will be to investigate why foxo and Tor are misregulated during aging and how these two genes interact with each another. In future, this could help develop new anti-aging therapies that can restore the body’s natural ability to repair itself following injury. Moreover, since cancer cells can become resistant to conventional cancer treatment by withdrawing from the cell cycle, developing new treatments that target foxo and Tor could help beat cancer and prevent its reoccurrence.
https://doi.org/10.7554/eLife.27842.002Introduction
In tissues with continuous cellular turnover, homeostasis is maintained by resident populations of adult stem cells. These cells both self-renew to maintain a constant pool of pluripotent cells and differentiate into a variety of cell types to replace cells that are lost to either natural wear and tear or to acute injury and insult (Fuchs et al., 2004). As tissues age, the ability of adult stem cells to replenish tissues is impaired (Schultz and Sinclair, 2016). As a result, tissue function declines, leading to a number of different age-related deficits: grey hair is a result of impaired melanocyte maintenance (Nishimura et al., 2005), decreased immunity results from reduced hematopoietic stem cell populations (Linton and Dorshkind, 2004), and decreases in neuron production has been implicated in the pathogenesis of a number of different neurodegenerative disorders, such as Alzheimer’s Disease (Donovan et al., 2006). However, the mechanisms that govern the regenerative competence of aging adult stem cells remain unclear. Of particular importance is the period when age-related declines first begin to manifest – when baseline stem cell function is preserved, yet, the ability to recover from injury may be impaired.
One of the most prevalent causes of injury in adult stem cells is genotoxic stress, such as that induced by exposure to ionizing radiation (IR). The fly is a particularly interesting model organism with which to examine stem cell survival post IR because recent work has demonstrated that there are several cell populations that display differing levels of resistance to ionizing radiation. Previous work in the young fly has shown a remarkable ability of Drosophila germline stem cells (GSCs) to survive IR, even when their progeny undergo rapid apoptosis. GSCs are resistant to the apoptotic effects of ionizing radiation (Xing et al., 2015): when flies are exposed to low doses of ionizing radiation GSCs survive, while their progeny, the transiently amplifying cells, do not. Dying GSC daughter cells secrete the ligand Pvf1, which signals via the Tie receptor and microRNA bantam to inhibit the apoptotic machinery in GSCs (Bilak et al., 2014; Xing et al., 2015). After a period of quiescence, the GSCs re-enter the cell cycle and, ultimately, regenerate the germline. Knockdown of Pvf1, a Tie ligand, in differentiating daughter cells rendered stem cells sensitive to IR, suggesting that differentiating daughter cells send survival signals to protect stem cells for future repopulation. Similar pools of IR-resistant cells have also been identified in other tissues. For example, in the larval imaginal disc, there is a population of IR-resistant cells that are able to generate viable adult tissues, even when exposed to high levels of radiation (Verghese and Su, 2016). Today, however, the ability of aging adult stem cells to maintain their resistance to ionizing radiation remains unexamined. Gaining a better understanding of stem cells' ability to recover from ionizing radiation will provide valuable insight into a wide range of physical phenomena, ranging from development of cancer therapeutics to improved aging remedies.
Stem cells in Drosophila melanogaster are a versatile system with which to study age related changes in regenerative potential (Lucchetta and Ohlstein, 2017; Fabian and Brill 2012; Resende et al., 2017; Resnik-Docampo et al., 2017). Defects in GSC function in aged flies have been identified and are in line with hypothesized defects in aging human stem cells: decreased proliferative capacity, accumulation of DNA damage, and eventual loss of stem cells (Zhao et al., 2008; Kao et al., 2015). However, the initiation of the aging process, and, particularly, how GSCs early in the aging process respond to injury, remains an open area of investigation. Furthermore, the ability of aging GSCs to regenerate their resident tissue following injury has not been fully elucidated. Since a hallmark of aging stem cells is the inability to properly regenerate tissue following injury and insult (Sharpless and DePinho, 2007), it is critical to understand the relationship between the initiation of aging and the ability of stem cells to recover from injury, such as following exposure to ionizing radiation.
Here, we identify and mechanistically dissect a regeneration defect in aging GSCs following exposure to ionizing radiation. Aging GSCs survive exposure to radiation, but exhibit a defect in cell cycle reentry upon completion of DNA repair. We further show that young GSCs enter a 24 hr period of quiescence following exposure to ionizing radiation before reentering the cell cycle and beginning to regenerate the germline. In our investigation of the mechanisms governing this process, we identify the foxo-encoded transcription factor and the human mTOR ortholog, Tor as important regulators for GSC entry and exit of quiescence following exposure to ionizing radiation, respectively. Lastly, we show that the regeneration defect of aging GSCs can be rescued by knockdown of foxo, suggesting that misregulation of foxo may underlie the regenerative decline with age.
Results
Aging germline stem cells survive exposure to ionizing radiation, but fail to re-enter the cell cycle in a timely fashion
In the Drosophila ovary, at the apical tip of each germarium are two to three germline stem cells (GSCs) in direct contact with their somatic niche (Spradling 1993). These GSCs undergo asymmetric rounds of self-renewing divisions to give rise to a new stem cell and to a transiently amplifying cell (cystoblast) that undergoes four incomplete divisions generating an interconnected 16 cell cyst, of which one cell will eventually become an oocyte. Intricate interactions between GSCs and somatic cells allow for GSC maintenance in the niche (Fuchs et al., 2004; Ward et al., 2006). Germline stem cells can be identified by their proximity to the cap cells in the niche and the prominent foci of adducin staining, labeling the subcellular structures called spectrosomes, while progeny can be identified by a branched focus of adducin, known as the fusome, in cells that do not reside within the niche (Figure 1A.). As GSCs progress through the cell cycle, they alternately have an elongated or a round spectrosome, the morphology of which can be used to identify dividing GSCs (Figure 1A, (de Cuevas and Spradling, 1998). GSCs in the female Drosophila ovariole lose replicative capacity with age (Pan et al., 2007; Zhao et al., 2008; Kao et al., 2015), however, the early steps in aging and GSC ability to survive exposure to ionizing radiation during the early aging process has not been probed.
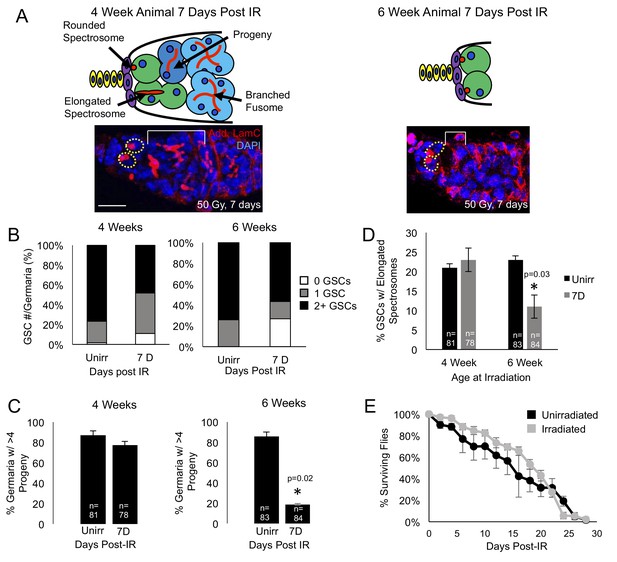
Aging GSCs survive exposure to IR, but do not regenerate the germarium.
(A) Top: Schematic diagrams of 4 week old vs. 6 week old germaria one week following IR. Terminal filament cells, yellow; cap cells, purple; germline stem cells, green, cystoblasts, dark blue; cysts, light blue. Bottom: representative images of 4 week old (left) and 6 week old (right) germaria stained for adducin and laminC (red) and DAPI. Germline stem cells (dotted yellow line) are visible in both 4 week old and 6 week old germaria, however, branched fusomes are only seen in the 4 week old germaria (white bracket). Scale bar = 10 μm. (B) Quantification of the number of GSCs/germaria in 4 week old (left) and 6 week old (right) germaria, before and one week following IR. White, 0 GSCs, grey, 1 GSC, black, 2 + GSCs. (C) Quantification of the percent of germaria with 4 or more progeny in 4 week old (left) and 6 week old (right) flies before and after IR. In 4 week old animals, there is no significant difference in the percent of germaria with 4 or more progeny, while in 6 week old animals, there is a decline in the percentage of germaria with 4 or more progeny after IR. (D) Quantification of the percentage of GSCs with elongated spectrosomes in 4 week old (left) and 6 week old (right) animals. While there is not a significant difference in the percentage of elongated spectrosomes before and one week following IR in 4 week old animals, there is a decrease in the percentage of GSCs with elongated spectrosomes one week following IR in 6 week old animals. (E) Survival curve of animals after 6 weeks, comparing unirradiated (black) and irradiated (grey) flies. There was no significant difference in the survival time of animals between irradiated and unirradiated flies.
We first asked whether aging GSCs survive radiation exposure. Since it has been shown that young GSCs survive exposure to IR and are able to regenerate the germline by one week following exposure to IR (Xing et al., 2015), we probed the system to identify the earliest time points where we could observe a defect in the aging GSCs’ ability to recover from exposure to IR. We found that at 4 weeks, recovery from IR and regeneration was normal, while a defect could be observed when 6 week old animals were irradiated. We exposed 4- and 6 week old wild type flies to 50 Grays of radiation and quantified the number of GSCs in unirradiated flies and compared them to the number of GSCs in germaria of flies one week following irradiation. We found that, although 4 and 6 week old germaria lose a small number of GSCs one week post IR the majority of 4 and 6 week old germaria still had 1 to 2 GSCs one week following irradiation (Figure 1B), indicating that the Tie-mediated protective mechanism remains mainly intact in aging germaria. Next, we assayed the level to which the GSCs were able to regenerate the germarium following exposure to ionizing radiation. We visualized GSC progeny with adducin staining and compared the number of germaria that had four or more progeny to those that had fewer than four progeny in unirradiated flies and in germaria of flies one week following exposure to irradiation. We found that, while at 4 weeks, the number of germaria with progeny was not significantly different before and after exposure to irradiation, at 6 weeks, the number of germaria with progeny one week following irradiation was significantly lower than in the germaria of unirradiated flies (Figure 1C). This suggests that, while aging GSCs are able to survive exposure to irradiation, they are unable to re-enter the cell cycle and regenerate the germarium in a timely manner. We confirmed that regeneration was impaired by assaying the percentage of GSCs with elongated spectrosomes, which is an indication of GSC division. We found that levels of spectrosome elongation were similar before and after irradiation in the 4 week old animals, however, in the 6 week old animals, there was a significant decrease in the percentage of GSCs with elongated spectrosomes one week after irradiation (Figure 1D). We further confirmed that regeneration was impaired in 6 week old animals by comparing the number of adults produced by 4 and 6 week old irradiated animals. We found that while irradiated 4 week old animals produced less adults than unirradiated animals of the same age , this defect was much more pronounced in irradiated 6 week old animals (Figure 1—figure supplement 1A,B). Taken together, our data suggest that aging GSCs are able to survive exposure to low IR, but are unable to reenter the cell cycle and regenerate the germline.
To assay the general fitness of the 6 week old flies, we compared the survival rates of 6 week old flies, both irradiated and unirradiated. We found no significant difference in the life span of the irradiated and unirradiated flies (Figure 1E). This indicates that we have found a time when GSCs have begun to age and show deficits in regenerative capacity, but dramatic aging phenotypes are not yet detectable at the organismal level. Hence, our analysis will allow us to understand the earliest processes in stem cell aging.
DNA damage is repaired within 24 hr following exposure to IR in aging animals
DNA damage can inhibit cell cycle progression (Bunz et al., 1998; Reinhardt and Schumacher, 2012), and increases in levels of DNA damage have been reported in aged GSCs (Kao et al., 2015). To assay whether the observed delay of cell cycle reentry in aging GSCs following irradiation was due to delays in DNA damage repair, we exposed 6 week old flies to 50 Gys of ionizing radiation. We then dissected ovaries from flies 30 min, 24 hr, and 7 days following irradiation and compared levels of DNA damage in GSCs to those of unirradiated flies, as visualized by γH2AV staining (Figure 2A–C). We compared the number of GSCs with high, moderate, or minimal levels of DNA damage at these time points. We found that DNA damage peaked 30 min following IR, with a majority of GSCs showing high levels of γH2AV staining (Figure 2B,D). However, by 24 hr following IR, levels of DNA damage had returned to baseline levels, similar to those in unirradiated flies (Figure 2C–E). Additionally, by 7 days post-irradiation, there was no significant difference in the level of DNA damage compared to unirradiated flies (Figure 2D). This indicates that DNA damage repair has concluded, even though the aging GSCs remain unable to regenerate the germline, suggesting that additional mechanisms must be responsible for the aging defect we identified in 6 week old GSCs.
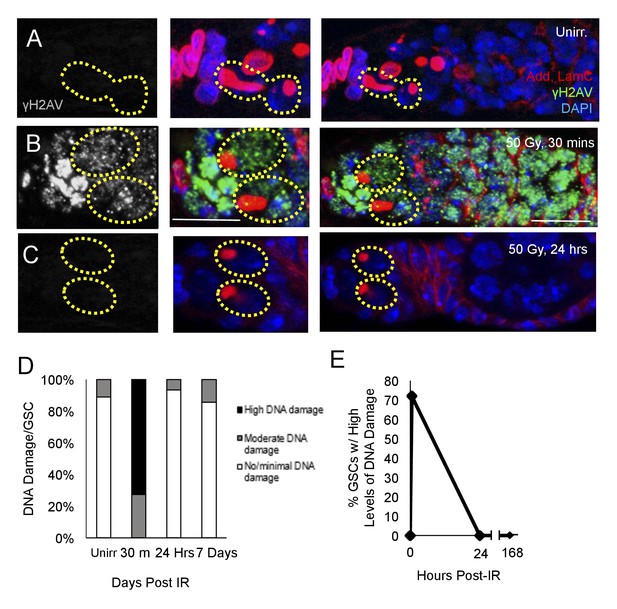
DNA damage repair concludes within 24 hr in aging animals.
(A-C) Representative images of a 6 week old germaria stained for adducin and lamC (red), γH2AV (green), and DAPI. (A) Unirradiated 6 week old germaria showing examples of GSCs with no γH2AV staining. (B) 6 week old germaria, 30 min post-IR. Scale bar = 10 μm. (C) 6 week old germaria, 24 hr post- IR. (D) Stacked bar plot showing percentage of GSCs with low (white), medium (grey), or high (black) levels of γH2AV staining following IR. High levels of DNA damage peak 30 min following IR and return to baseline by 24 hr. (E) Line graph showing percentage of GSCs with high levels of γH2AV staining over time.
Young germline stem cells enter a brief period of quiescence following exposure to IR
Having identified a regeneration defect in aging GSCs, we next investigated the timing of IR induced cell cycle exit and reentry in young, healthy flies. We exposed 2–7 day old flies to 50 Gys of ionizing radiation and compared levels of GSC division and regeneration to unirradiated flies at 24 hr intervals. We visualized branched fusomes and spectrosomes via adducin staining (Figure 3B–E). In order to assay the rates of GSC division, we compared the morphology of the spectrosomes in GSCs from flies that had been irradiated to unirradiated flies. We quantified the percentage of GSCs with elongated spectrosomes, as an indicator of GSC cellular division (Figure 3B,D, yellow arrow). We observed a significant decrease in the percentage of GSCs with elongated spectrosomes one day post-IR (Figure 3F). By two days following irradiation, the percentage of GSCs with elongated spectrosomes had returned to baseline (Figure 3F). This suggests that when well fed, young animals are exposed to low doses of irradiation, GSCs enter a brief, approximately 24 hr period of quiescence.
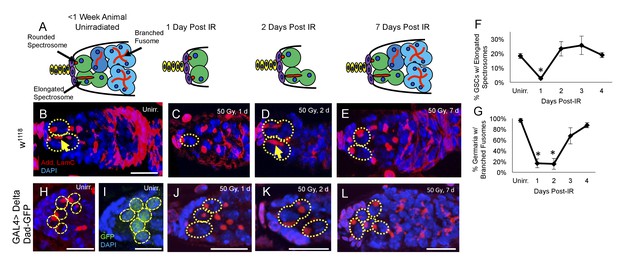
Young GSCs enter a brief period of IR-induced quiescence before returning to the cell cycle.
(A) Schematic of the progression of cell loss and recovery following exposure to IR in young animals. Terminal filament cells, yellow; cap cells, purple; germline stem cells, green, cystoblasts, dark blue; cysts, light blue. (B-E) Representative images of young w1118 germaria stained for adducin and lamC (red) and DAPI (blue). GSCs are indicated by the presence of a spectrosome and DAPI staining (dotted yellow line); elongated spectrosomes indicated with yellow arrow. Scale bar = 10 µm. B. Unirradiated germarium. (C) Germarium 1 day post-IR (50 Gγs). (D) Germarium 2 days post-IR. (E) Germarium 7 days post-IR. (F) Line graph of percentage of GSCs with elongated spectrosomes for days 1–4 post-IR. There is a significant decrease of percentage of GSCs with elongated spectrosomes at 1 day post-IR (3 biological experiments, mean ± s.e.m., *p<0.05, ANOVA). (G) Line graph of the percentage of germaria with branched fusome for days 1-4 post IR. (H) Representative unirradiated germaria of nos-Gal4 > Delta flies, showing increased niche size and supernumerary GSCs. (I) Unirradiated nos-Gal4 > Delta; Dad-GFP germaria. (J) nos-Gal4 > Delta germaria 1 day post-IR. (K) nos-Gal4 > Delta germaria 2 days post-IR. (L) nos-Gal4 > Delta germaria one week post-IR showing a fully regenerated germarium.
Similarly, we quantified the number of regenerated germaria by quantifying the number of germaria with germ line cysts containing branched fusomes (Figure 3G). Unlike GSCs, transiently amplifying cells do not survive exposure to ionizing radiation and the number of new daughter cells can, therefore, be used as an indirect measure of GSCs’ regeneration capacity following irradiation damage (Xing et al., 2015). There was a significant decline in the percentage of germaria with GSC daughters containing branched fusomes one and two days post-IR (Figure 3G). By 3 days, post-IR, the percentage of regenerated germaria had dramatically increased, with complete recovery achieved by 4 days post-IR. This suggests that GSCs give rise to progeny by 3 days post-IR, which is in line with our observation that GSCs begin dividing around two days post-IR. Taken together, our data indicate that GSCs enter an approximately 24 hr period of quiescence after exposure to ionizing radiation before returning to the cell cycle and regenerating the germline.
Notch signaling also plays an essential role in the development and maintenance of the Drosophila germline stem cell niche. Niche cells and GSCs communicate with one another via the Delta and Serrate Notch ligands to regulate various niche features, including niche size and GSC number (Ward et al., 2006; Song et al., 2007). Abrogation of Notch signaling by expressing a nos-Gal4-inducible RNAi construct against neuralized (neur), a ubiquitin ligase which mediates the internalization and subsequent activation of the Delta and Serrate Notch ligands in the germline, resulted in a complete loss of GSCs, even before exposure to ionizing radiation (data not shown), confirming the essential role of Notch signaling in GSC maintenance (Ward et al., 2006; Song et al., 2007). To study if supernumerary GSCs follow the wild type GSC kinetics of post-IR quiescence, we drove overexpression of Delta in the germline using the Gal4 system. Nos-Gal4 > Delta germaria showed an increased number of spectrosome marked cells, which we confirmed were GSCs via expression of the TGFβ target, Dad (Figure 3H–I). This indicates that the expanded TGFβ signaling from niche induced extranumerary GSCs, as seen previously (Ward et al., 2006; Song et al., 2007). We exposed Delta overexpression flies to ionizing radiation (50 Gys) and dissected their ovaries 1, 2 and 7 days post IR. GSCs in the expanded niche enter and exit quiescence in a timely manner (Figure 3H–L). However, while the somatic niche remained large, GSC number was reduced one day after IR (Figure 3—figure supplement 1), suggesting that the protective signal from daughter cells cannot penetrate to protect all the supernumerary GSCs after exposure to ionizing radiation.
Germline stem cell DNA damage is repaired within 24 hr of exposure to IR
To confirm that DNA damage repair kinetics are not substantially different in young flies and old flies, we assayed levels of DNA damage via γH2AV staining in the germaria of 2–7 day old flies exposed to ionizing radiation (Figure 4A–H). We quantified the percentage of GSCs with high, moderate, or no/minimal levels of γH2AV at 30 min, 12 hr, and 24 hr after exposure to ionizing radiation and compared this to levels of γH2AV in unirradiated germaria (Figure 4I). We found that there was a significant increase in the percentage of GSCs with high levels of DNA damage 30 min post-IR (Figure 4C,D,I). By 12 hr post-IR, a majority of the germaria had repaired DNA damage to a moderate amount: only 8% showed high levels of DNA damage, while 83% had moderate levels of DNA damage (Figure 4E–F,I–J). By 1 day following radiation exposure, only 34% of GSCs had moderate levels of yH2AV staining, with 66% of GSCs returned to baseline levels of DNA damage (Figure 4G,H,I,J). This suggests that DNA damage repair kinetics in young flies resemble those of the aging fly, supporting our previous findings that alterations in DNA damage repair kinetics alone cannot account for the regeneration defect in aging GSCs.
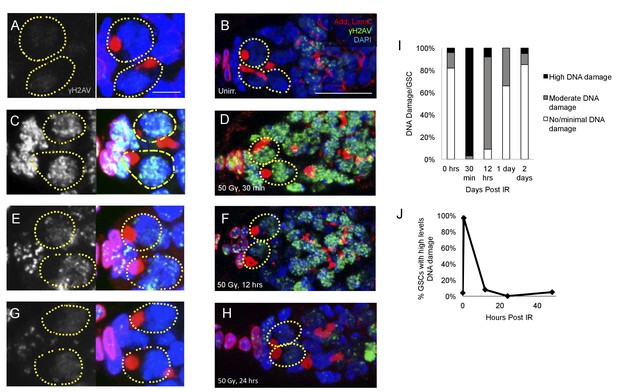
DNA damage repair concludes by 24 hr in young flies A-H.
Representative images of young germaria stained for γH2AV alone (grey) or adducin/lamC (red), γH2AV (green), and DAPI. (A) Unirradiated GSCs. γH2AV channel alone (left), color merge (right). Scale bar = 5 µm (B) Unirradiated germarium. Scale bar = 10 µm (C) GSCs 30 min post-IR. γH2AV channel alone (left); color merge (right) (D) Germarium 30 min post-IR. (E) GSCs 12 hr post-IR. γH2AV channel alone (left); color merge (right). (F) Germarium 12 hr post-IR. (G). GSCs 24 hr post-IR. γH2AV channel alone (left); color merge (right). (H) Germarium 24 hr post-IR. (I) Stacked bar plot showing percentage of GSCs with low (white), medium (grey), or high (black) levels of γH2AV staining following IR. High levels of DNA damage peak 30 min following IR and return to baseline by 24 hr. (J) Line graph showing percentage of GSCs with high levels of γH2AV staining over time.
We next asked what mechanisms are involved in regulating IR-induced quiescence in GSCs. We first probed the role of the G1 checkpoint in IR-induced quiescence by manipulating levels of the p21 ortholog, dacapo. We found that, while overexpression of dacapo was sufficient to prolong IR-induced quiescence (Figure 5—figure supplement 1B), there was no significant difference in the ability of GSCs to enter quiescence when dacapo levels were reduced (Figure 5—figure supplement 1E,F). This suggests that dacapo is not required for GSCs to enter quiescence after a radiation challenge, suggesting that the G1 checkpoint is not where GSCs arrest following exposure to IR. Additionally, we examined the role of the DNA damage sensing machinery in regulating IR-induced quiescence. We found that when the CHK2 ortholog, loki, was knocked down via RNAi, it impaired the ability of GSCs to enter quiescence (Figure 5—figure supplement 1G and H), consistent with recent work demonstrating the vital role of loki in regulating GSC survival following exposure to high levels of IR (Ma et al., 2016). Thus, we worked to identify the functional machinery downstream of CHK2 that regulates the stem cell quiescence.
foxo is required for GSC cell cycle arrest following exposure to IR
foxo is a key player in the cellular response to IR (Chung et al., 2012; Xing et al., 2015). To probe whether foxo was required for IR-induced GSC cell cycle exit and reentry, we knocked down foxo in the germline by crossing UAS-Dcr-2; nos-Gal4 flies to two independent UASp-foxo RNAi lines. We then assayed the morphology of GSCs' spectrosomes at 1 and 2 days post-IR in nos-GAL4 > foxo RNAi flies and compared them to unirradiated GSCs. We found that, while in UAS-Dcr-2; nos-Gal4 control flies, there is a dramatic decrease in the percentage of GSCs with elongated spectrosomes 1 day post-IR, foxo deficient GSCs in both RNAi lines kept dividing at a normal rate (Figure 5A,B). Additionally, the percentage of germaria with branched fusomes 1 day post-IR is increased in foxo RNAi flies (Figure 5C; Supplementary file 1A,B), further strengthening our finding that knockdown of foxo eliminates IR-induced quiescence.
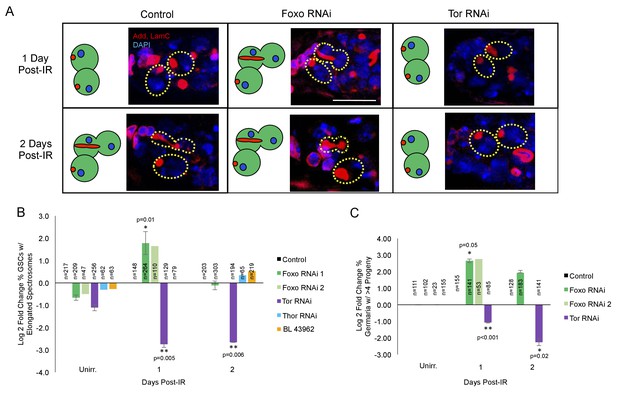
foxo and Tor regulate GSCs’ cell cycle exit and reentry.
(A) Representative images of germaria stained for adducin and lamC (red) and DAPI (blue). Left top: Control germaria, 1 day post-IR. Left bottom: Control germaria, 2 days post-IR. Middle top: foxo RNAi germaria, 1 day post-IR. Scale bar = 10 µm Middle bottom: foxo RNAi germaria, 2 days post-IR. Right top: Tor RNAi germaria, 1 day post-IR. Right bottom: Tor RNAi germaria, 2 days post-IR. (B) Bar plot of the percentage of GSCs with elongated spectrosomes up to two days post-IR for foxo, Tor, Thor and control RNAi lines, plotted as log2 fold change compared to control. foxo RNAi inhibits the ability of GSCs to exit the cell cycle. Tor RNAi inhibits the ability of GSCs to reenter the cell cycle. (C) Bar plot of the percentage of germaria with more than four progeny up to two days post IR for foxo, Tor, Thor and control RNAi lines, plotted as log2 fold change compared to control.
This suggests that foxo is required for GSCs to initiate IR-induced quiescence and withdraw from the cell cycle.
Tor is required for GSC cell cycle reentry post-IR
Foxo has been shown to regulate Tor in C.elegans, Drosophila, and mammalian systems (Puig et al., 2003; Jia et al., 2004; Chen et al., 2010). Since Tor signaling is known to modulate both Drosophila longevity and GSC division (Bjedov et al., 2010; LaFever et al., 2010) we analyzed its potential role in cell cycle regulation following exposure to IR. To probe whether Tor is required for IR-induced GSC cell cycle exit or reentry, we knocked down Tor in the germline using a nos-GAL4 driver to express a Tor RNAi construct under UAS control (nos-GAL4 > Tor RNAi). We then assayed Tor mutant GSC division capacity by analyzing the morphology of spectrosomes and the number of daughters produced at 1 and 2 days post-IR and compared them to control and unirradiated GSCs. We found that when Tor is knocked down, there is an even larger decrease in the percent of GSCs with elongated spectrosomes one day post-IR than in control animals, suggesting a higher penetrance in cell cycle exit (Figure 5A,B). Furthermore, the percentage of Tor RNAi GSCs with elongated spectrosomes and the number of GSC daughters remained decreased two days post-IR, when control GSCs have reentered the cell cycle (Figure 5C; Supplementary file 1A,B), suggesting a dramatic delay in the reentry to the self-renewing cell cycle and regenerative capacity in Tor mutant GSCs.
We also probed the role of Tor signaling pharmacologically with rapamycin. Rapamycin is a potent inhibitor of the TORC1 complex, preventing phosphorylation of Tor’s downstream targets (Sabatini et al., 1995). Following irradiation, wild type flies were fed grape juice with either rapamycin (200 µM) or vehicle for two days. There was a significant decrease in the percentage of GSCs with elongated spectrosomes 2 days post-IR with rapamycin treatment (Figure 6A–C). Taken together, these data suggest that Tor is required for GSC exit from quiescence and cell cycle reentry post-IR.
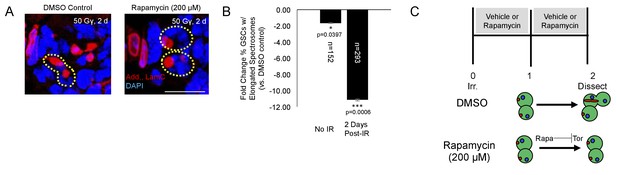
Tor activity is required for cell cycle reentry following IR.
(A) Representative images of GSCs from a fly fed vehicle control (left) or rapamycin (200 μM, right) stained for adducin/lamC (red) and DAPI (blue) two days post-IR. Scale bar = 10 µm (B) Bar plot showing percentage of GSCs with elongated spectrosomes two days post IR. Flies fed rapamycin had decreased rates of GSC division compared to flies fed vehicle control. The effect of rapamycin on GSC division was much more pronounced two days post IR. (C) Top:Experimental paradigm. Flies were irradiated at Day 0 and fed either vehicle control or Rapamycin for 48 hr post-IR. Ovaries were dissected 2 days post-IR and analyzed. Bottom: When rapamycin represses Tor activity, there is a decrease in the ability of GSCs to exit quiescence.
Finally, we probed the question of whether IR-induced quiescence is protective to GSCs. When nos-Gal4 > foxo RNAi flies were exposed to a secondary dose of ionizing radiation 24 hr following the initial dose (Figure 5—figure supplement 2A), we found that there was a decrease in the number of GSCs per germaria in foxo RNAi flies (Figure 5—figure supplement 2C). This difference cannot be attributed to foxo reduction alone, since unirradiated nos-Gal4 > foxo RNAi ovaries have a normal number of GSCs per germarium. This suggests that foxo-mediated IR-induced quiescence is important for GSC survival.
foxo represses Tor in GSC after IR
Since we identified opposing roles for foxo and Tor in regulating IR-induced quiescence, we next asked whether these two signaling components operated independently or in conjunction with each other. To visualize foxo activity, we stained for Foxo protein and to assay levels of Tor activity, we stained for phosphorylated ribosomal protein S6 (p-S6), a downstream effector of TORC1. We compared levels of Foxo and p-S6 staining in young, wild type files following exposure to ionizing radiation. We observed a dramatic increase in the level of Foxo in GSCs’ nuclei 1 day post-IR (Figure 7A). Levels of Foxo staining returned to baseline (Figure 7D) by 2 days post-IR. Phospho-S6 staining showed a complimentary pattern to Foxo staining: while foxo is highly expressed at the anterior tip of germaria and the GSCs, p-S6 levels are high in 8- and 16 cell cysts towards the posterior end of germaria, suggesting a possible regulatory role of Tor activity by foxo (Figure 7B). To test this, we reduced foxo levels and measured Tor activity by analyzing p-S6 patterns. When foxo is depleted via nos-Gal4-induced RNAi, the level of p-S6 staining increases and is observed closer to the anterior tip of the germaria and GSCs, which is not observed in wild type animals (Figure 7C). p-S6 staining was completely absent in germaria of nos-Gal4 > Tor RNAi flies (Figure 7F), confirming that p-S6 is a reliable measure of Tor activity. Together, this suggests that Tor and Foxo activity are spatially segregated due to an antagonistic relationship between the activity of these two proteins (Figure 7G). In particular, these data show that foxo can repress the TORC1 target, p-S6 in the Drosophila ovary.
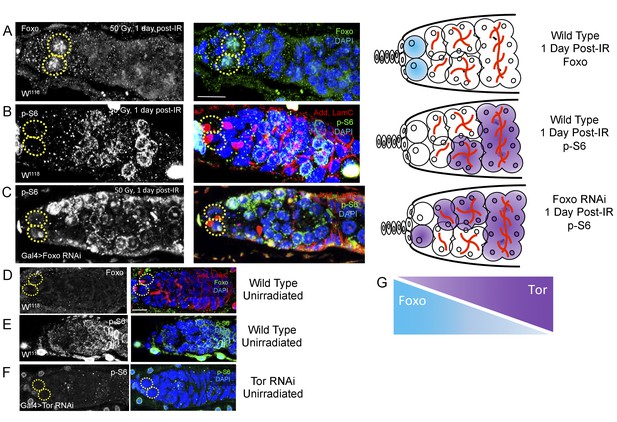
Foxo and Tor activity are spatially segregated.
(A) Foxo levels increase in GSCs one day post-IR. Scale bar = 10 µm (B) Phosphorylated S6 (p–S6) and Foxo signals have opposite gradients throughout the germarium. Levels of p-S6 increase in the anterior region of the germarium 1 day post-IR, and signaling returns to baseline at 2 days post-IR. (C) P-S6 staining moves closer to the anterior tip of the germaria in Foxo deficient flies 1 day post-IR. (D) Levels of Foxo are low in wild type, unirradiated flies. Scale bar = 10 µm (E) p-S6 staining is localized to the posterior end of the germarium in wild type, unirradiated flies. (F) In Tor RNAi flies, there was no detectable germline p-S6 staining. (G) Diagrammatic representation of Foxo and Tor gradients throughout the germarium. Foxo and Tor levels are elevated, respectively, in the anterior and posterior region of the germarium.
Knockdown of foxo levels rescues the GSC regeneration defect in aging animals
Since we identified foxo as a critical regulator of IR- quiescence, we next asked whether knockdown of foxo in the aging GSC could rescue the observed aging regeneration defect. We aged nos-Gal4 > foxo RNAi flies to 6 weeks and exposed them to 50 Gys of ionizing radiation. We quantified the number of GSCs per germaria, as well as the number of germaria with four or greater progeny in unirradiated and one week post-IR flies. We found that, compared to unirradiated flies, there was no significant difference in the number of GSCs/germaria in 6 week old nos-Gal4 > foxo RNAi flies one week following irradiation (Figure 8A,C). Strikingly, we also found that one week following exposure to IR, nos-Gal4 > foxo RNAi flies showed evidence of germline regeneration, with equal numbers of germaria with greater than four progeny when compared to their unirradiated counterparts (Figure 8B,D). We also observed large 8 cell cysts one week post-IR in 6 week old nos-Gal4 > foxo RNAi flies (Figure 8B) indicating robust and extensive regeneration of the germline. This developmental stage is never observed in 6 week-old wild type flies one week post-IR. These findings suggest that knockdown of foxo is sufficient to relieve the aging regeneration defect: aging flies with reduced levels of foxo are able to regenerate the germline within a week, while wild type flies cannot (Figure 8F).
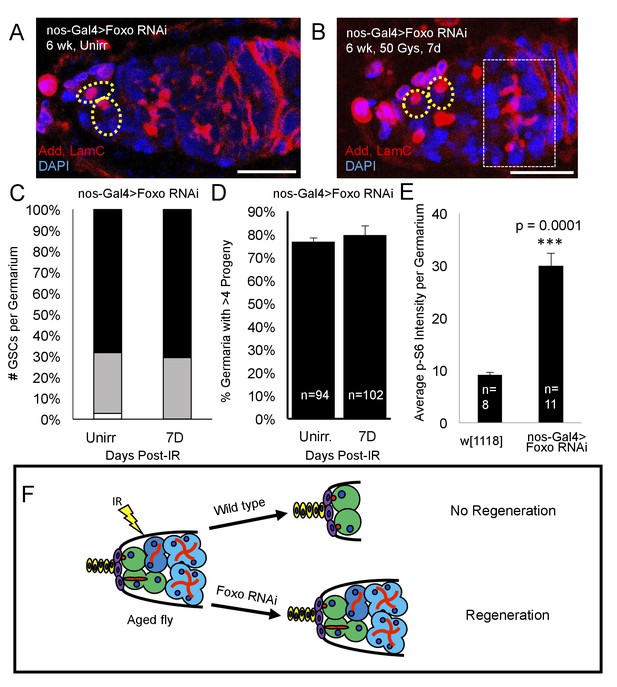
Loss of foxo rescues age-related regeneration defect.
(A) Representative image of a 6 week old nos-Gal4 > foxo RNAi germarium. (B) Representative image of a 6 week old nos-Gal4 > foxo RNAi germarium one week post-IR, showing a fully regenerated germline with a large 8 cell cyst (dotted white rectangle). (C) Bar graph quantifying the number of GSCs per germaria before and one week following irradiation, showing there is no difference in the number of GSCs per germaria. (D) Bar graph quantifying the percentage of germaria with four or more progeny, indicating a fully regenerated germline. (E) Bar plot showing average p-S6 intensity values for the germaria of 6 week-old w1118 and nos-Gal4 > foxo RNAi flies, showing an increase of p-S6 levels in foxo RNAi flies. (F) Schematic demonstrating the difference between a wild type aging fly one week following IR and a foxo RNAi fly one week following IR. While the wild type fly in incapable of regenerating the germaria, knockdown of foxo rescues this defect.
To study foxo’s mode of function in the context of aging, we probed Tor signaling, a Foxo target repressed post-injury in young animals. Aging flies expressing a UASp RNAi construct against foxo showed a dramatic increase in germline Tor activity, as measured by p-S6 antibody staining (Figure 8E). This suggests that Foxo represses Tor activity during aging and that overactivation of Foxo may account for the inability of aging GSCs to regenerate following exposure to IR (Figure 9), as evidenced by the ability of the aging germline to regenerate with decreased levels of Foxo.
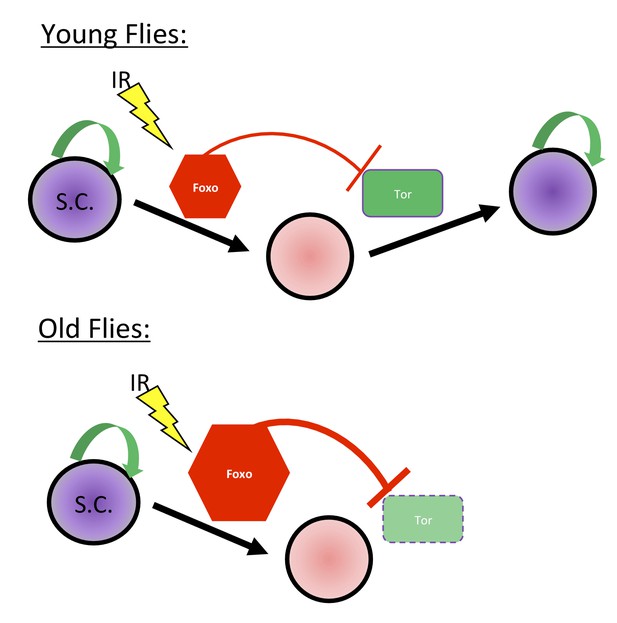
Proposed Mechanism.
In young flies, injury, such as exposure to ionizing radiation, leads to Foxo activation, which represses Tor activity, pushing the GSCs into a state of protective quiescence. Following deactivation of Foxo post-IR, Tor activity allows GSCs to reenter the cell cycle and regenerate the germline. In aging flies, increased Foxo activity prevents Tor activation and GSCs reentry in the cell cycle.
Discussion
Adult stem cells experience a decrease in regenerative potential with age that results in a decrease in the ability of adult tissues to repair themselves following injury or insult. We have now identified the earliest time at which aging Drosophila germline stem cells lose the ability to appropriately recover from exposure to sublethal doses of ionizing radiation (IR) and dissect the mechanism for this process. Following exposure to IR, most aging GSCs survive, but fail to reenter the cell cycle and regenerate the germline, a process that is activated in young flies post IR. This is not due to a defect in DNA damage repair, as DNA damage repair concludes in a timely manner, even though the aging GSCs fail to return to the cell cycle. We have now identified two key regulators for IR induced quiescence: foxo and Tor. These two genes have opposing roles in regulating GSC cell cycle, exit and reentry after IR, respectively. Furthermore, Tor inactivation by RNAi or Rapamycin treatment induces a premature GSC aging phenotype, impairing Tor-dependent regeneration post injury. Conversely, knocking down foxo in aging animals rescues the aging phenotype, allowing GSCs to regenerate the germline, as observed in young flies. Finally, we show that foxo and Tor have opposing patterns of expression in the germarium and depletion of foxo leads to increases in Tor activity. This suggests that foxo regulates post-IR quiescence and cell cycle reentry by regulating Tor activity. Importantly, we show that loss of foxo rescues the GSC age-related regeneration phenotype due to IR. Overall, this study shows that IR induced quiescence is regulated by foxo and the mTOR ortholog, Tor, and suggests that upregulation of foxo and misregulation of Tor signaling in aging adult stem cells may be responsible for the decline in regenerative capacity following injury or insult (Figure 9).
Aging adult stem cells are unable to regenerate injured tissue as effectively and efficiently as young stem cells (Schultz and Sinclair, 2016). However, it has remained an open area of investigation as to whether this is due to a loss of adult stem cells with age or whether this is due to a decrease in the ability of adult stem cells to regenerate appropriately. Our work shows that the anti-apoptotic protective mechanisms (Xing et al., 2015) that shield adult stem cells from death remain mainly intact, but the aging GSCs are unable to reenter the cell cycle following IR-induced quiescence.
Aging is a complex process, involving the cumulative decline of multiple cell types. Defects in the replicative potential of old GSCs have been reported by other groups (Zhao et al., 2008; Tseng et al., 2014; Kao et al., 2015; Rauschenbach et al., 2015). However, our work expands our understanding of the onset of aging in a unique way. Here, we identify the earliest time point at which defects can be detected in GSC proliferation in an injury model. Before the induction of IR-mediated quiescence in our aging flies, rates of GSC division, as well as the number of GSCs per germaria were similar to that seen in young, healthy flies. Defects were only readily observed following exposure to IR. This suggests that baseline levels of GSC function remain unperturbed, however, the GSCs are unable to recover successfully from insult. This leads us to believe that we have identified a defect early in the initiation of the aging process. Therapeutically, this is a very important window, as it allows us to identify times when an intervention may be useful in helping to slow the progression of aging, or prevent it from initiating in the first place, rather than attempting to reverse it late in the process.
High doses of irradiation have been shown to lead to GSC loss (Ma et al., 2016). We specifically utilized a relatively low dose of ionizing radiation, in order to induce damage, but not lead to GSC loss (Xing et al., 2015) and to probe the ability of aging stem cells to recover from an injury that should be surmountable were the cells functioning properly. We were able to identify critical roles for two known proteins involved in tissue homeostasis: Foxo for cell cycle withdrawal and Tor for cell cycle reentry. foxo has been well documented as a regulator of stem cell self-renewal and quiescence (Demontis and Perrimon, 2010; Xing et al., 2012; Eijkelenboom and Burgering, 2013; Gopinath et al., 2014; Xing et al., 2015). Notably, foxo tends not to be active during normal physiology, but rather during stressful conditions, when it responds to and counteracts a stressor in order to maintain homeostasis (Kenyon, 2010; Eijkelenboom and Burgering, 2013).
Here, we show that foxo activity is required in Drosophila GSCs in order for them to withdraw from the cell cycle following exposure to ionizing radiation. There are multiple ways that Foxo may be able to sense the damage caused to the cell by irradiation. In response to the presence of reactive oxygen species, JNK-mediated phosphorylation of Foxo can cause its translocation to the nucleus(van den Berg and Burgering, 2011). Foxo can also be the target of multiple pathways that are responsive to DNA damage: Foxo is a target of phosphorylation by ATM (Matsuoka et al., 2007) and the MAPK pathway (Kress et al., 2011) both of which have been shown to be activated by DNA damage. Lastly, Foxo is capable of directly sensing cellular redox status via oxidation and reduction of amino acids, particularly cysteine (Dansen et al., 2009).
CHK2, a highly conserved checkpoint kinase, controls DNA repair, cell cycle arrest and apoptosis following DNA damage. The fly CHK2 ortholog, loki, has been shown to mediate GSCs’ self-renewal and differentiation following high doses of ionizing radiation (Ma et al., 2016). Here we show that depletion of loki in the germline prevents GSCs from entering quiescence following exposure to low doses of ionizing radiation. Loki’s ability to sense DNA damage and interact with Foxo via the ATM-CHK2-p53 complex (Chung et al., 2012) could explain how GSCs know to activate Foxo and withdraw from the cell cycle following IR-induced double stranded breaks. Notably, p53, another component of the ATM-CHK2-p53 complex, has also been shown to regulate GSC irradiation-induced quiescence (Wylie et al., 2014) although how p53 interacts with Foxo in this context remains unclear. It is possible that any of these, or the combination of multiple of these systems sense the damage to the GSCs caused by the ionizing radiation and translocate Foxo to the nucleus, initiating IR-induced quiescence.
Mechanistic target of rapamycin (mTOR) signaling has been implicated in a number of different age-related functions, from extension of lifespan (Vellai et al., 2003; Harrison et al., 2009; Bjedov et al., 2010; Laplante and Sabatini, 2012; Bitto et al., 2016) to germline stem cell self-renewal (LaFever et al., 2010; Sun et al., 2010), induction of a diapause like quiescent state (Bulut-Karslioglu et al., 2016) and muscle satellite cell activation following injury (Rodgers et al., 2014). We found that Tor signaling was required in order for GSCs to reenter the cell cycle and regenerate the germline following exposure to IR. The sensitivity of wild type GSC proliferation to treatment with rapamycin after IR indicates that this could be mediated via the Tor complex 1 (TORC1) since rapamycin preferentially targets TORC1. We cannot completely rule out a role for Tor complex 2 (TORC2) in GSCs’ quiescence since rapamycin treatment has been shown to affect TORC2 activity by keeping Tor associated with TORC1 (Sarbassov et al., 2006; Lamming et al., 2012). Further studies will focus on investigating the roles of both TORC1 and TORC2 downstream effectors in GSC quiescence.
GSCs with decreased levels of Tor activity are unable to reenter the cell cycle post-IR, which is unlikely to be a general consequence of Tor inhibition inhibiting GSC division. In a number of different experiments, we observed a more pronounced defect in GSC proliferation in the context of recovery from injury post-IR than at baseline. This indicates that, while Tor might play a role in regulating stem cell division and self-renewal under normal physiological conditions, it likely has an additional injury-specific role in helping to replenish adult tissues that have been damaged, either by natural wear and tear or due to disease or injury. Given Tor’s ability to regulate translation, nucleotide synthesis, autophagy, lipid synthesis, and proteasome assembly, (Laplante and Sabatini, 2012) it will be important to dissect which of these or other cellular processes are required for GSCs’ exit from quiescence. It is also quite striking that inhibition of Tor resembles the defect observed in aging GSCs, while at an organismal level, inhibition of Tor increases lifespan, suggesting a slowing of the aging process. This would indicate that Tor inhibition, albeit beneficial at an organismal level, may damage stem cells’ capacity to regenerate tissue after injury. This is a particularly important implication of our findings, given the increasing number of anti-aging studies involving rapamycin (Fan et al., 2015; Bitto et al., 2016).
Mutations in insulin receptor (InR) in Drosophila and insulin-like growth factor (IGF1) in mice, result in Foxo activation and significant lifespan extension (Clancy et al., 2001; Tatar et al., 2001; Bluher et al., 2003; Holzenberger et al., 2003; Webb et al., 2016). In humans, single-nucleotide polymorphisms (SNPs) in the FOXO3 locus have been associated with extraordinarily long lifespans (Morris, 2005), though the mechanism for this remains elusive. Our study identifies a novel foxo-dependent stem cell defect in aged animals in which elevated foxo activity prevents GSCs from re-entering the cell cycle and regenerating the germline after a challenge. In contrast to other studies showing the benefits of high levels of foxo activity, we show, for the first time, that elevated levels of foxo activity, albeit beneficial in terms of lifespan extension, are detrimental to stem cell function in the context of tissue regeneration during aging. There are several reasons why pathologically high levels of foxo might prevent tissue regeneration in old animals. A meta-analysis of mouse Foxo targets that change with age has revealed that several cell cycle genes, such as the evolutionarily conserved cyclin-dependent kinase 4 (Cdk4), which controls the G1 to S transition, and several ribosomal proteins, which are directly involved in protein translation, are misregulated in aging (Webb et al., 2016). In our study, we show how, after IR exposure, foxo and Tor have opposing patterns of expression in young animals. We also demonstrate how reducing foxo levels via RNAi increases p-S6 levels in young and aging animals. This strengthens the idea that foxo and Tor signaling interact with one another to regulate GSC division following injury and that misregulatin of this crosstalk might contribute to stem cell aging.
Our study shows how Foxo misregulation may impair aging GSCs’ regeneration potential. foxo’s ability to repress Tor could shed light on aging GSCs’ inability to resume division following insult. Though the mechanism with which foxo and Tor interact in the context of aging remains elusive, previous studies have already probed the relationship between these signaling pathways. Foxo has been shown to repress Tor signaling by allowing TSC (Tuberous Sclerosis Complex) to localize to the lysosome (Menon et al., 2014). At the lysosomal membrane, TSC is then able to inhibit Rheb, an essential activator of mTORC1. Other studies have shown that Foxo is able to inhibit mTORC1 by reducing Raptor levels (Jia et al., 2004) or by promoting the transcription of Sestrin 3 and Rictor (Chen et al., 2010). Notably, Tor signaling can also inhibit Foxo activity by upregulating SGK (Saxton and Sabatini, 2017), an AGC-kinase shown to inhibit Foxo. This suggests the possibility of a negative feedback loop between these signaling pathways. In the future, it will be of vital importance to dissect the crosstalk between foxo and Tor signaling to understand why GSCs lose their regeneration potential with age.
Materials and methods
Fly stocks and culture conditions
Request a detailed protocolThe following stocks were obtained from the Bloomington Drosophila Stock Center at Indiana University: w[1118] (RRID:BDSC_3605), P[UAS-Dcr-2.D]1, w1118; P[GAL4-nos.NGT]40 (RRID:BDSC_25751), UASp-foxoRNAi (RRID:BDSC_32427 and RRID:BDSC_32993), UASp-TorRNAi (RRID:BDSC_35578), UASp-ThorRNAi (RRID:BDSC_36815), UASp-dmRNAi (RRID:BDSC_43962), UASp-dapRNAi (RRID:BDSC_36720), UASp-LokiRNAi (RRID:BDSC_64482). The following stocks were previously generated for and described in Ward et al., 2006: UASp-Delta/CyO, UASp-Delta/CyO; Dad-GFP/TM3, UASp-Delta/CyO; Ly/TM3. The following stocks were previously generated for and described in Yu et al., 2009: pin/CyO;hs-dap-7-7, hsFLP; FRT42B GFP/CyO, FRT42B/CyO, FRT42B dap4
w1118 flies were used as a control, unless noted otherwise. Flies were cultured at 25° C on standard cornmeal-yeast-agar medium, augmented with wet yeast. In aging experiments, flies were transferred to fresh vials without wet yeast every 2–3 days. Young and old flies were given wet yeast two days prior to irradiation.
Gamma-irradiation treatment
Request a detailed protocolAfter feeding on standard cornmeal-yeast-agar medium augmented with wet yeast paste for two days, young and old flies were transferred to empty vials and treated with 50 Gγs of gamma-irradiation. A Cs-137 Mark I Irradiator was used to administer the proper irradiation dosage, according to instructed dosage chart. Post-treatment animals were transferred back to fresh food with wet yeast and maintained at 25° C until dissection.
Rapamycin treatment
Request a detailed protocolFollowing irradiation, flies were place in an empty vial with filter paper soaked in grape juice with either 200 µM rapamycin or DMSO dissolved in it.
Fertility assay
Request a detailed protocolFollowing irradiation, 10 females were placed in a new vial with 5 young, unirradiated wild type male flies. Flies were transferred to new vials every 2–3 days and the death of any flies was noted. Vials from flies 5–7 days post-IR were collected and the number of progeny hatched per female was calculated.
Generation of clones
Request a detailed protocolGSCs clones were induced via the heat shock FLP-FRT system. Young flies (2–3 days old) of the following genotypes hsFLP; FRT42B GFP/FRT42B, hsFLP; FRT42B GFP/FRT42B dap4, were heat shocked in a 37° C water bath for 45 minutes hour once a day for two consecutive days Heat shocked flies were given fresh food and yeast paste every other day until dissection and stored at 25° C for the duration of the experiment.
Immunocytochemistry
Request a detailed protocolOvaries were fixed in 4% paraformaldehyde for 15 min, rinsed in PBT (PBS containing 0.2% Triton X-100), and blocked in PBTB (PBT containing 0.2% BSA, 5% normal goat serum) for at least one hour at room temperature. Samples were stored up to 72 hr at 4° in PBTB. The following primary antibodies were used: mouse anti-adducin (RRID:AB_528070 1:30), mouse anti-Lamin C (RRID:AB_528339 1:30) rabbit anti-γH2AV (RRID:AB_828383 1:200), rabbit anti-p-S6 (RRID:AB_916156 1:200), rabbit anti-foxo (generous gift from Pierre Léopold 1:200). Ovaries were incubated with primary antibodies for either 1.5 hr at room temperature or overnight at 4°. After washes with PBT, secondary fluorescence antibodies were utilized including anti-rabbit Alexa 488 (RRID:AB_221544 1:250) and anti-mouse 568 (RRID:AB_2535773 1:250) for 1.5–2 hr at room temperatures in the dark. DAPI was added to one of the final washes to visualize cells’ nuclei. The samples were mounted in glycerol and analyzed on a Leica SPE5 confocal laser-scanning microscope.
Statistical analysis
Request a detailed protocolAll data are presented as the mean of at least three independent experiments (n ≥ 3) with the standard error of the mean (SEM) indicated by error bars, unless otherwise indicated. Statistical significance was determined using Student's t test (for two groups) or ANOVA with the appropriate post hoc test (for more than two groups). Data were compiled using Excel 2013 software and analyzed using Excel (version 2013 for Windows; Microsoft, Seattle, WA, USA) or the Astatsa Online Web Statistical Calculator (astatsa.com, Philadelpha, PA, USA).
References
-
FOXO3 signalling links ATM to the p53 apoptotic pathway following DNA damageNature Communications 3:1000.https://doi.org/10.1038/ncomms2008
-
Redox-sensitive cysteines bridge p300/CBP-mediated acetylation and FoxO4 activityNature Chemical Biology 5:664–672.https://doi.org/10.1038/nchembio.194
-
Morphogenesis of the Drosophila fusome and its implications for oocyte specificationDevelopment 125:2781–2789.
-
Decreased adult hippocampal neurogenesis in the PDAPP mouse model of alzheimer's diseaseThe Journal of Comparative Neurology 495:70–83.https://doi.org/10.1002/cne.20840
-
FOXOs: signalling integrators for homeostasis maintenanceNature Reviews Molecular Cell Biology 14:83–97.https://doi.org/10.1038/nrm3507
-
Drosophila spermiogenesis: big things come from little packagesSpermatogenesis 2:197–212.https://doi.org/10.4161/spmg.21798
-
Age-related changes in lymphocyte development and functionNature Immunology 5:133–139.https://doi.org/10.1038/ni1033
-
A forkhead in the road to longevity: the molecular basis of lifespan becomes clearerJournal of Hypertension 23:1285–1309.https://doi.org/10.1097/01.hjh.0000173509.45363.dd
-
Interplay of insulin and dopamine signaling pathways in the control of Drosophila melanogaster fitnessDoklady Biochemistry and Biophysics 461:135–138.https://doi.org/10.1134/S1607672915020179
-
The rapamycin and FKBP12 target (RAFT) displays phosphatidylinositol 4-kinase activityJournal of Biological Chemistry 270:20875–20878.https://doi.org/10.1074/jbc.270.36.20875
-
How stem cells age and why this makes us grow oldNature Reviews Molecular Cell Biology 8:703–713.https://doi.org/10.1038/nrm2241
-
Integrating opposing signals toward Forkhead box OAntioxidants & Redox Signaling 14:607–621.https://doi.org/10.1089/ars.2010.3415
Article and author information
Author details
Funding
National Institute on Aging (Genetic Approaches to Aging Training Grant postdoctoral fellowship)
- Rebecca E Kreipke
Coordenação de Aperfeiçoamento de Pessoal de Nível Superior (Science without Borders)
- Ondina Palmeira
National Institute of General Medical Sciences (R01-GM084947)
- Hannele Ruohola-Baker
Hahn Family
- Hannele Ruohola-Baker
National Institutes of Health (UO1HL099993)
- Hannele Ruohola-Baker
National Institutes of Health (R01GM097372)
- Hannele Ruohola-Baker
National Institutes of Health (R01GM083867)
- Hannele Ruohola-Baker
National Institutes of Health (1P01GM081619)
- Hannele Ruohola-Baker
National Institutes of Health (U01HL099997)
- Hannele Ruohola-Baker
The funders had no role in study design, data collection and interpretation, or the decision to submit the work for publication.
Acknowledgements
We thank the TRiP at Harvard Medical School (NIH/NIGMS R01-GM084947) for providing transgenic RNAi fly stocks used in this study. We would like to thank the members of the Ruohola-Baker lab for their stimulating discussion and valuable comments. We thank Sonthaya Artphakdi for help with the nos-Gal4 > Delta studies. This work is supported by a Genetic Approaches to Aging Training Grant postdoctoral fellowship from the National Institute of Aging to REK, gift from Hahn Family and partly by grants from the National Institutes of Health R01GM097372, R01GM083867, 1P01GM081619, U01HL099997; UO1HL099993 for HRB.
Version history
- Received: April 18, 2017
- Accepted: August 28, 2017
- Accepted Manuscript published: September 19, 2017 (version 1)
- Version of Record published: October 17, 2017 (version 2)
Copyright
© 2017, Artoni et al.
This article is distributed under the terms of the Creative Commons Attribution License, which permits unrestricted use and redistribution provided that the original author and source are credited.
Metrics
-
- 2,541
- views
-
- 496
- downloads
-
- 21
- citations
Views, downloads and citations are aggregated across all versions of this paper published by eLife.
Download links
Downloads (link to download the article as PDF)
Open citations (links to open the citations from this article in various online reference manager services)
Cite this article (links to download the citations from this article in formats compatible with various reference manager tools)
Further reading
-
- Cell Biology
- Neuroscience
Alternative RNA splicing is an essential and dynamic process in neuronal differentiation and synapse maturation, and dysregulation of this process has been associated with neurodegenerative diseases. Recent studies have revealed the importance of RNA-binding proteins in the regulation of neuronal splicing programs. However, the molecular mechanisms involved in the control of these splicing regulators are still unclear. Here, we show that KIS, a kinase upregulated in the developmental brain, imposes a genome-wide alteration in exon usage during neuronal differentiation in mice. KIS contains a protein-recognition domain common to spliceosomal components and phosphorylates PTBP2, counteracting the role of this splicing factor in exon exclusion. At the molecular level, phosphorylation of unstructured domains within PTBP2 causes its dissociation from two co-regulators, Matrin3 and hnRNPM, and hinders the RNA-binding capability of the complex. Furthermore, KIS and PTBP2 display strong and opposing functional interactions in synaptic spine emergence and maturation. Taken together, our data uncover a post-translational control of splicing regulators that link transcriptional and alternative exon usage programs in neuronal development.
-
- Cell Biology
Amyotrophic lateral sclerosis (ALS) is a fatal neuromuscular disorder characterized by progressive weakness of almost all skeletal muscles, whereas extraocular muscles (EOMs) are comparatively spared. While hindlimb and diaphragm muscles of end-stage SOD1G93A (G93A) mice (a familial ALS mouse model) exhibit severe denervation and depletion of Pax7+satellite cells (SCs), we found that the pool of SCs and the integrity of neuromuscular junctions (NMJs) are maintained in EOMs. In cell sorting profiles, SCs derived from hindlimb and diaphragm muscles of G93A mice exhibit denervation-related activation, whereas SCs from EOMs of G93A mice display spontaneous (non-denervation-related) activation, similar to SCs from wild-type mice. Specifically, cultured EOM SCs contain more abundant transcripts of axon guidance molecules, including Cxcl12, along with more sustainable renewability than the diaphragm and hindlimb counterparts under differentiation pressure. In neuromuscular co-culture assays, AAV-delivery of Cxcl12 to G93A-hindlimb SC-derived myotubes enhances motor neuron axon extension and innervation, recapitulating the innervation capacity of EOM SC-derived myotubes. G93A mice fed with sodium butyrate (NaBu) supplementation exhibited less NMJ loss in hindlimb and diaphragm muscles. Additionally, SCs derived from G93A hindlimb and diaphragm muscles displayed elevated expression of Cxcl12 and improved renewability following NaBu treatment in vitro. Thus, the NaBu-induced transcriptomic changes resembling the patterns of EOM SCs may contribute to the beneficial effects observed in G93A mice. More broadly, the distinct transcriptomic profile of EOM SCs may offer novel therapeutic targets to slow progressive neuromuscular functional decay in ALS and provide possible ‘response biomarkers’ in pre-clinical and clinical studies.