- 1Beijing Key Laboratory of Growth and Developmental Regulation for Protected Vegetable Crops, College of Horticulture, China Agricultural University, Beijing, China
- 2School of Environmental and Life Sciences and Australia-China Research Centre for Crop Improvement, The University of Newcastle, Newcastle, NSW, Australia
Root-knot nematodes (Meloidogyne incognita) induce specific feeding sites in cucumber roots where they absorb carbon nutrients from the host, thereby turning the feeding sites into a strong sink for assimilates. Nematode infection may alter host sugar metabolism in the roots of sucrose-transporting species. However, much less is known about the species translocating raffinose family oligosaccharides (RFOs), such as cucumber. To address this knowledge gap, the dynamics of RFOs and sucrose metabolisms, two major sugar-metabolism processes, in cucumber roots during nematode infection at transcription and protein levels were analyzed. In the nematode-infected root, the expressions of RFO-synthesis genes, CsRS (Raffinose Synthase) and CsGolS1 (Galactinol Synthase 1), were upregulated at early stage, but were significantly decreased, along with CsSTS (Stachyose Synthase), at the late stage during nematode infection. By contrast, α-galactosidase hydrolyzed RFOs into sucrose and galactose, whose encoding genes was suppressed (CsaGA2) at early stage and then elevated (CsaGA2, 4, and CsAGA1) at the late stage of nematode infection. Consistently, stachyose level was significantly increased by ∼2.5 times at the early stage but reduced at the late stage of infection in comparison with the uninfected roots, with a similar trend found for raffinose and galactinol. Moreover, the genes encoding sucrose synthase and cell wall invertase, which are responsible for sucrose degrading, were differentially expressed. In addition, sugar transporter, CsSUT4, was enhanced significantly after nematode infection at early stage but was suppressed at the late stage. Based on the observation and in connection with the information from literature, the RFOs play a role in the protection of roots during the initial stage of infection but could be used by nematode as C nutrients at the late stage.
Introduction
The plant-parasitic nematodes are devastating pests causing billions of dollars in crop losses annually (Bozbuga et al., 2018). Among these nematodes, root-knot nematode (RKN) is a major sedentary endoparasitism, which is capable of inducing highly specific feeding cell systems in its host roots and damaging root development and function. This nematode could infect more than 5,000 plant species, causing approximately 30% yield loss by direct infestation and various degrees of indirect losses due to predisposition or breakdown of resistance to other root diseases (Castagnone-Sereno et al., 2013). So far, four kinds of RKNs comprising of Meloidogyne incognita (M. incognita), Meloidogyne javanica (M. javanica), Meloidogyne arenaria (M. arenaria), and Meloidogyne hapla (M. hapla) are considered as serious threats to crop production (Brinkman et al., 2015). Thus, understanding and preventing nematode parasitism are of importance in crop production.
After invading the host plant, the RKNs grow inside root and develop from juvenile to adult stages, the process of which remains for several weeks (Abad et al., 2008). Specifically, after egg hatching, the infective second-stage juvenile (J2) nematodes enter the vascular cylinder of root tips and move upward until near the elongation zone to form a feeding site (Perry and Moens, 2011). The vascular cells selected by J2 form several binucleate cells; these nuclei are further divided and lead to the formation of multinucleated giant cells (GCs). As the GCs develop, the neighboring cells proliferate and enlarge to form a characteristic hyperplastic structure called a root-knot or gall. After then, the RKNs become sedentary within the gall and feed on the nutrient channeled from GCs until life cycle completion. The GCs possess high metabolic activity and withdraw significant amounts of sugars and protein from the host to support the growth and reproduction of RKNs (Gommers and Dropkin, 1977; Bartlem et al., 2014; Escobar et al., 2015). The continuous withdrawal of nutrients by RKNs turns the GCs into strong nutrient sinks in the host roots. The nematode-induced galls in vascular cylinder and cortex cells of roots could impair water and mineral transport, inhibit shoot growth, and ultimately lead to irreversible yield loss.
The nematodes at feeding sites could use specific strategies to snatch host carbohydrates to fuel their growth (Slewinski, 2011). For most flowering plants, sucrose is the major end product of photosynthesis, sucrose metabolic enzymes, such as sucrose synthase (SUS), cell wall invertase (CWIN), and sugar transporters that may include hexose transporters (HTs) and sucrose transporters (H+-coupled sucrose transporter, SUT) were suggested to involved in the processes of phloem loading or unloading (Braun et al., 2014). In Arabidopsis, the genes encoding sucrose transporter (e.g., AtSUC1 and AtSUC2), SUS, and CWINs were differently expressed in response to the infections of Heterodera schaschtii (H. schaschtii; cyst nematode) and M. javanica (Hofmann et al., 2007b,2010). In syncytia, the feeding site caused by H. schaschtii, no plasmodesmata were found between syncytia and surrounding cells at early stages, and sucrose was supplied to syncytia through H+-coupled sucrose transporter AtSUC4 in Arabidopsis roots (Hofmann and Grundler, 2007; Hofmann et al., 2007b). After infecting M. incognita, the significant upregulation of AtSUC4 suggested its role in the sucrose supply of GCs embedded in Arabidopsis root galls (Hofmann et al., 2009). Hammes et al. (2005) analyzed the expression of transporter genes in response to RKN infection and found significant changes in expression levels of at least 50 transporter genes from 18 different gene families, particularly sugar transporter genes including AtSUC1, AtSTPs, and AtSFPs. Differently, in rice root with an infection of Meloidogyne graminicola, the plasmodesmata-mediated, instead of sugar transporters-facilitated, sucrose transport plays key role in sugar supply to the GCs (Xu et al., 2021). Although the cyst nematodes and RKNs differ in terms of host selection and invasion mechanisms, they both withdraw nutrients from the host roots (Slewinski, 2011).
For some plant species, raffinose family oligosaccharides (RFOs) are the main sugars for phloem loading and translocation to sink organs. The RFOs involve in many growth and development processes including phloem translocation, storage of carbon in vegetative and reproductive tissues, and response to abiotic and biotic stresses (Taji et al., 2002; Pennycooke et al., 2003). In Cucurbitaceae, raffinose and stachyose are synthesized by raffinose synthase (RS) and stachyose synthase (STS) in the leaves and transported to sink organs via the phloem (Ma et al., 2019). During unloading, the RFOs are hydrolyzed into sucrose and galactose by α-galactosidase (α-Gal) and then undergo sucrose metabolic processes. As stated above, most of the previous studies focused on the sucrose-transport species (Hofmann et al., 2007a,b, 2009; Xu et al., 2021), however, in RFOs-transport species, there is still limited information regarding the response of sugar metabolism to nematode infection.
Cucumber (Cucumis sativus L.) is widely cultivated as an economical vegetable crop worldwide but could suffer from serious nematode infection, especially from a RKN, M. incognita (Kayani et al., 2017). Currently, cultivars or commercial rootstocks that are resistant to M. incognita are restricted to solanaceous crops such as tomato and pepper (Jacquet et al., 2005; Ros-Ibáñez et al., 2014) but barely reported in cucumber (Li et al., 2021). A major limitation to breed nematode-resistant cucumber varieties is insufficient knowledge on genes responding to, or mechanisms underlying, M. incognita infection in cucumber. Although sugar and its metabolism and transport in cucumber have been well-understood (Sui et al., 2018), their roles in and responses to nematode infection in cucumber are obscure. Considering they are the most important nutrient sources for nematode development (Escobar et al., 2015), they deserve more attention. There are large numbers of genes involved in sugar metabolism and transport in cucumber, including four Galactinol Synthase (GolSs), one RS, one STS (Ma et al., 2019), eight α-Gal genes (four acid α-gal genes, CsaGAs, and four alkaline α-gal genes, CsAGAs) (Sui et al., 2018), four SUSs (Wang et al., 2014), five CWINs (Hu et al., 2011), and three sugar transporters (SUTs) (Ma et al., 2019). However, there is still a lack of holistic understanding on whether any of these genes are regulated in response to nematode infection. To address this issue, in this study, the dynamics in sugar contents and gene expressions for RFO and sucrose metabolism and sugar transport were investigated in cucumber root with or without nematode infection. These findings provide theoretical basis for breeding for nematode-resistance cultivars of cucumber through manipulation of sugar withdrawal process.
Materials and Methods
Plant Materials and Growth Conditions
The cucumber (Xintaimici) seeds were sown in plastic pots containing sterilized sand:vermiculite [1:1, (v/v)]. The seedlings were grown in a growth chamber under day/night temperature of 28°C/18°C and during 16 h/8 h cycle. The stocks of M. incognita were maintained on the roots of soil-grown water spinach (lpomoea aquatica Forsk cv Liuye). The egg masses were collected from spinach root galls and placed in water at 28°C for 3 days. The preparasitic J2s (pre-J2s) were collected for cucumber root infection (Ding et al., 1998).
Nematode Culture and Infection Assays
For infection assays, about 300 fresh pre-J2s were inoculated to invade the root of each cucumber seedling with two leaves. The samples were collected at 7–35 days postinoculation (dpi). At 7 dpi, the parasitic second juvenile stage nematode (J2s) are found in host plants. At 14 dpi, J2s and J3s are found and feeding sits initiate expansion. At 21 dpi, nematodes are in J3 stage and sexually differentiating, the female/male nematodes can be discerned. At 28 dpi, fourth-stage juveniles (J4) become adult nematodes. At 35 dpi, egg masses can be easily recognized. Full cucumber roots were sampled because the galls could not be easily discerned at 7 dpi, but the galls were sampled in the late stage of nematode infection (14, 21, 28, 35). The roots were speedily washed in 0.1% diethyl pyrocarbonate (DEPC) water, then the infected galls and corresponding uninfected lateral roots were immediately frozen in liquid nitrogen, and stored in –80°C until use. For RNA extraction and enzyme activity analysis, 100 mg of fresh roots or galls were collected in each sample, and 500 mg of fresh roots or galls were collected for detecting sugar content.
Nematode Staining Tests
For observing the developmental stages of nematode, the cucumber roots were infected by pre-J2s M. incognita and then stained by using acid fuchsin according to Bybd et al. (1983) with some modifications. First, the cucumber roots were soaked in 1.5% NaClO4 for 4 min and rinsed with tap water for 45 s. Then, they were soaked for 15 min with distilled water to remove residual NaClO4 and dried. The roots were placed in 30 ml distilled water and 1 ml of acid-fuchsin solution was added and boiled for 30 s. Subsequently, the stained roots were washed with running water and transferred to acidic glycerin with heating to fade. Finally, the roots were stored overnight in acidic glycerin for detection of nematodes.
Carbohydrate Extraction
The extraction of soluble sugar was based on the method of Ma et al. (2019). The roots or galls frozen in liquid nitrogen were grounded into powder in a mortar with 5 ml of 80% (v/v) ethanol and 80°C water bath for 30 min, centrifuged at 4,000 × g for 10 min (H2050R, Xiangyi Centrifuge Instrument Co., Ltd., China), and then the supernatant was collected. The precipitate after centrifugation was repeated three times for extraction. All the supernatants collected from the three extractions were vacuum dried. After resuspending the dried sample in 1 ml deionized water, the sample was filtered using the filter membrane with a diameter of 0.45 μm and detected by high performance liquid chromatography.
RNA Isolation and Quantitative Real-Time PCR
The total RNA was extracted from the lateral roots and galls using RNeasy Plant kit (Huayueyang, Beijing, China) according to the manufacturer’s protocol. For each case, 1 μg RNA was used for cDNA synthesis using the Faster Quant RT kit (Qiagen, Beijing, China). The cDNA samples were used as templates for RT-qPCR analysis. Quantitative RT-PCR was performed in 384 well plates with a 7,500 real-time (RT) PCR System from Applied Biosystems using SYBR Green according to the manufacturer’s protocol (Takara, Japan). All of the samples were tested in five biological replicates with two technical replicates for each reaction. CsUBQ (Csa4G089780) and CsEF1α (Csa2G139820) were employed as internal reference genes. The results were determined by using the 2–△△CT method (Schmittgen and Livak, 2008). The primers and gene accession data are listed in Supplementary Tables 1, 2, respectively.
Enzyme Activity Analysis
The samples (0.5 g) of lateral roots and galls were grounded in a mortar containing liquid nitrogen. The SUS was measured using Sucrose Synthase (degradative direction; SUS) Activity Assay Kit (Solarbio, BC4315, Beijing, China). The SUS catalyzes sucrose degradation in the presence of uracil diphosphate (UDP) to produce fructose and UDP-glucose at the pH 5.5. The reactions were proceeded for 20 min at 30°C and were terminated by 95°C for 5 min. The fructose reacts with 3, 5-dinitrosalicylic acid to produce brown-red substance with characteristic absorption peak at 540 nm. The SUS activity can be calculated by measuring the change of absorption value at 540 nm. Briefly, the evenly ground samples were added with 1 ml extraction buffer and centrifuged at 8,000 × g for 10 min at 4°C. The supernatant was collected and placed on ice for enzyme activity measurement according to the instruction of kit and Wang et al. (2014) with some changes.
The CWIN was extracted and determined using Cell Wall-Bound Acid Invertase Activity Assay Kit (Solarbio, BC4325, Beijing, China) according to the instruction of kit and Palmer et al. (2015) with some modifications. The CWIN catalyzes sucrose to produce glucose and fructose, which react with 3, 5-dinitrosalicylic acid to produce brown-red substance with characteristic absorption peak at 540 nm (Thermo Fisher Scientific Oy, Ratastie 2, FI-01620 Vantaa, Finland). Mainly, the grounded samples were added with 1 ml extraction buffer I and centrifuged at 12,000 × g for 10 min at 4°C (H2050R, Xiangyi Centrifuge Instrument Co., Ltd., China). The supernatant was discarded and the sediment was collected and added with 1 ml distilled water before fully mixed. Once again the sediment was retained by centrifugation at 12,000 × g for 10 min. Then, the precipitate was added with l ml extraction buffer II and fully blended. The sample was incubated at 4°C for 15 h and centrifuged at 12,000 × g for 10 min at 4°C. The supernatant was collected and placed on ice for enzyme activity measurement with Thermo Scientific Microplate Reader.
The α-galactosidase activity was determined by using the α-Galactosidase Activity Assay Kit (Solarbio, BC2575, Beijing, China) following the instruction of kit. The α-galactosidase decomposes p-nitrobenzene-α-D-galactoside to p-nitrophenol, the latter has the maximum absorption peak at 400 nm. The activity of α-galactosidase was calculated by measuring the increasing rate of the absorption value. Mainly, the homogenous sample was added with 1 ml extraction buffer by centrifuging at 15,000 × g for 20 min at 4°C. The supernatant was collected for enzyme activity assay using Thermo Scientific Microplate Reader.
In all the enzyme activity assays, the protein concentration was measured using the Bradford Method Protein Quantitative Kit (Huayueyang, PP0202, Beijing, China).
Statistical Analysis
For RNA extraction and enzyme activity analysis, six plants were pooled as one biological replicate with 5 replicates and three technique repeats each replicate used in each experiment. For detecting the sugar content, six plants were pooled as one biological replicate with 3 replicates. The experimental data were expressed as mean ± SE. The experimental data were analyzed using statistical product and service solutions (SPSS) v. 30.0 (SPSS Inc.). The data significant differences were analyzed using a two-tailed Student’s t-test, values with p < 0.05, p < 0.01, or p < 0.001 were considered statistically significant.
Results
Development of M. incognita in Cucumber Roots
To understand the RKN life cycle inside cucumber roots for the selection of the right stage of nematode development for analyses, M. incognita-induced galls were observed in Cucumis sativus (Figure 1). Visible galls were observed in cucumber root systems at 7–35 days postinoculation (dpi) by M. incognita (Figures 1A–D). To investigate the intercellular states of nematodes along different timepoints in infected cucumber roots, histological experiments were conducted. At 7 dpi, the feeding sites (GCs) were formed in vascular cylinder, with later continuous expanding at 14 dpi (Figure 1E), and a high increase of nematodes (juveniles, adults, and eggs) was observed along different stages of infected roots at 21, 28, and 35 dpi (Figures 1F1–F5). Based on the stage of nematode development, the galls at typical timepoints (0, 7, 14, 21, 28, and 35) were sampled for further study.
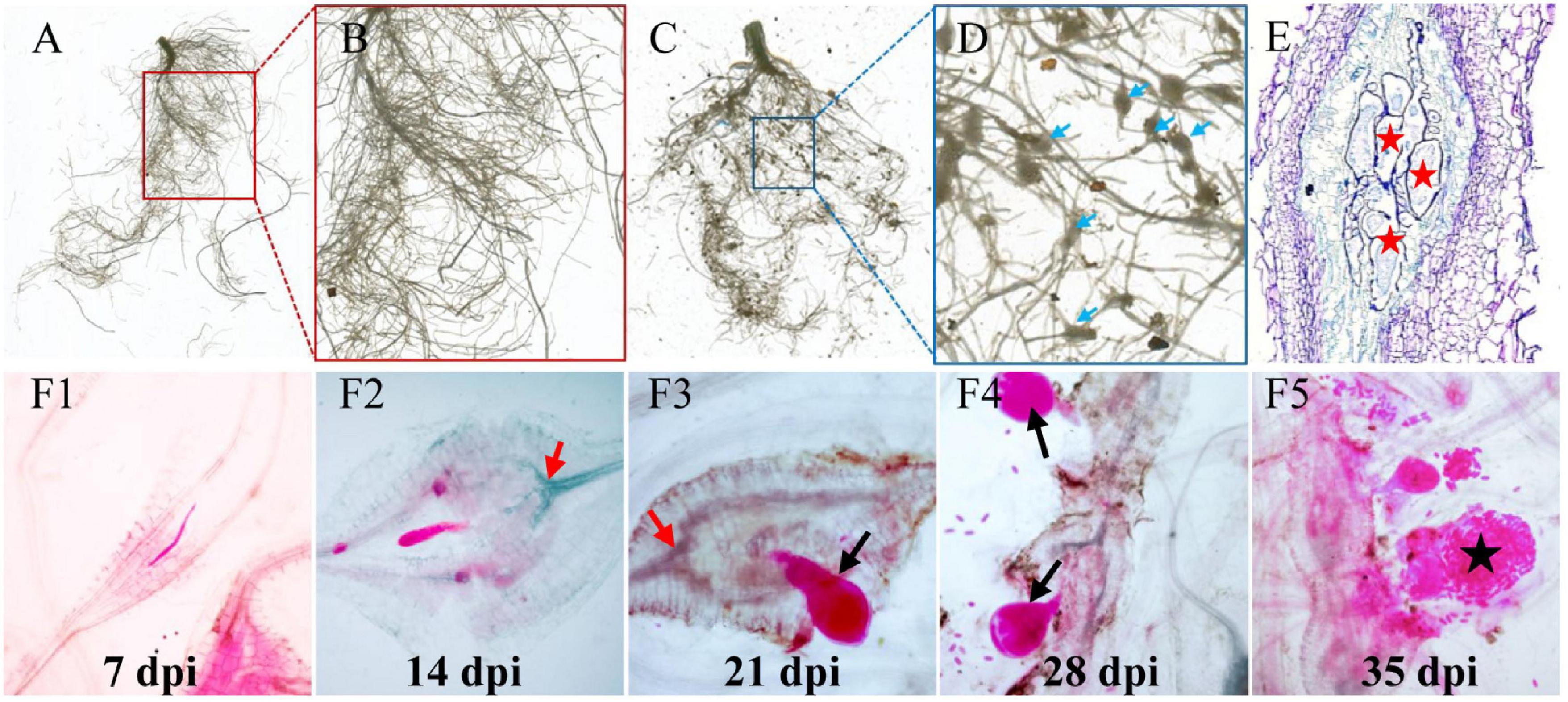
Figure 1. Infection of M. incognita to cucumber roots. (A) Control cucumber roots without nematode infection. (C) Galls (blue arrows) are induced in roots by M. incognita. (B,D) Are the close-up views of sections marked in (A) (red) and (C) (blue) regions. Blue arrows in (D) indicate root knots or galls induced by nematodes. (E) The anatomic structure of a gall. Red pentagrams indicate the giant cells (GCs). (F1–F5) RKN life cycle inside cucumber roots, J2 (F1)-J3 (F2)-J4 (F3,F4)-eggs (F5). Black arrows indicate the female nematodes with a pear-shaped body. Black pentagram indicates the eggs produced by adult nematodes.
Soluble Sugar Dynamics in Nematode-Infected Cucumber Roots
To understand how nematode infection may affect sugar status in the root, the soluble sugars were measured in the infected cucumber roots (Figure 2). Five timepoints (7, 14, 21, 28, and 35 days) selected from infected and non-infected roots were chosen. Compared with non-infected roots, the stachyose level was induced and sucrose was decreased at 7 dpi (Figures 2D,F). In the late stage of nematode infection, glucose, but not fructose, was significantly induced at 21 dpi, however, galactinol was decreased at 35 dpi, raffinose was decreased at 28 and 35 dpi, and stachyose was decreased at 21 dpi in infected roots compared with non-infected roots (Figures 2B–F).
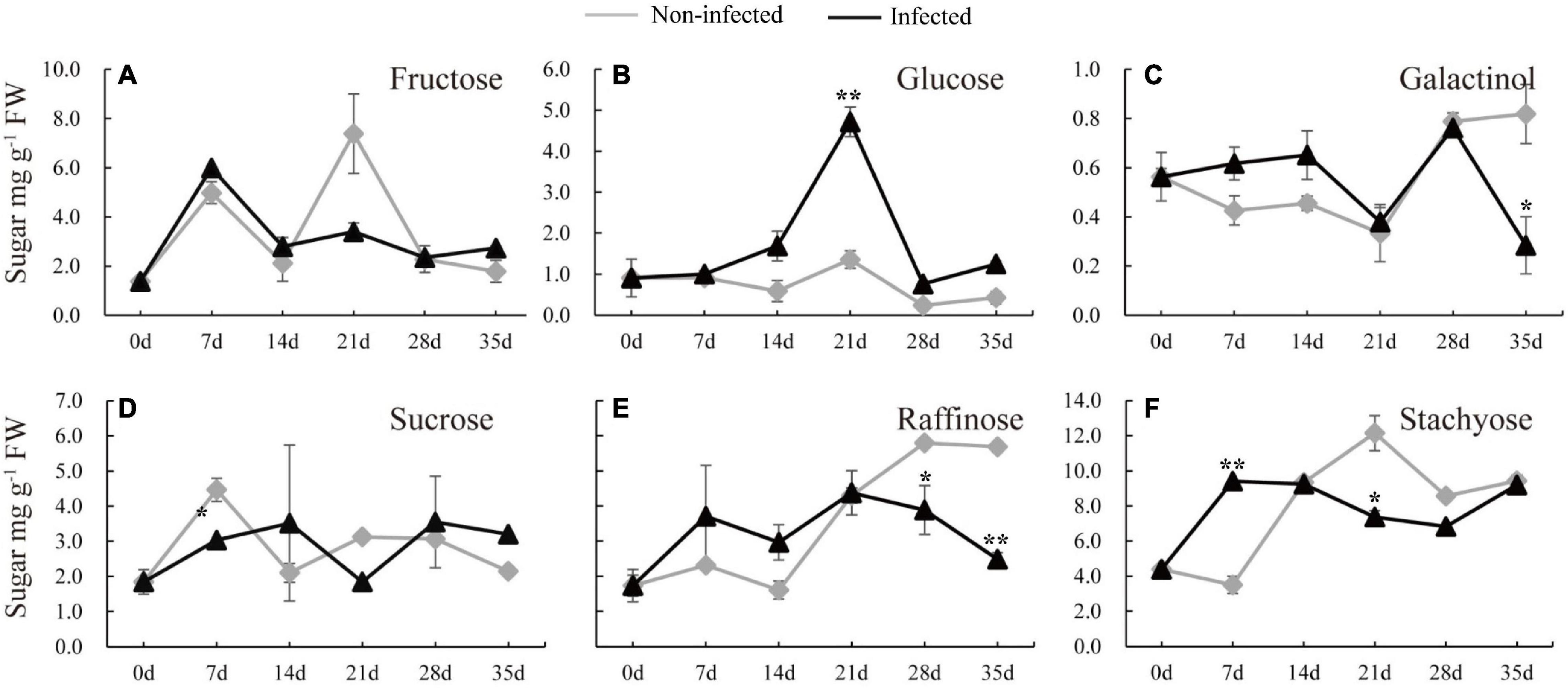
Figure 2. Changes of carbohydrate content in infected and non-infected cucumber roots by M. incognita. (A–F) Are the content of fructose, glucose, galactinol, sucrose, raffinose and stachyose in cucumber roots, respectively. Error bars represent ± SE (n = 6). Student’s t-test is analyzed between infected and non-infected roots, *P < 0.05; **P < 0.01.
Responses of CsRS, CsSTS, and CsGolS1 in Nematode-Infected Roots
To examine whether RFOs metabolism responded to nematode infection, qRT-PCR was performed to determine the expressions of CsRS, CsSTS, and CsGolSs. Compared with the non-infected roots, the transcript levels of CsRS and CsGolS1 were enhanced at 7 dpi (Figure 3). However, their mRNA levels, together with that of CsSTS, were suppressed at 28 dpi in infected cucumber roots, with no difference detected at 14 dpi (Figure 3). This result was consistent with the finding that RFOs (galactinol, raffinose, and stachyose) were decreased at the late stage of nematode infection (Figure 2). The expression of CsGolS4 was increased at 21 dpi, but then decreased at 28 and 35 dpi, in the infected roots, however, its expression was weak (qPCR Ct value was ∼32). By comparison, the expressions of CsGolS2 and CsGolS3 were not affected by nematode infection (Figure 3). Thus, CsGolS1 dominated most of the GolS transcripts in cucumber roots under nematode infection.
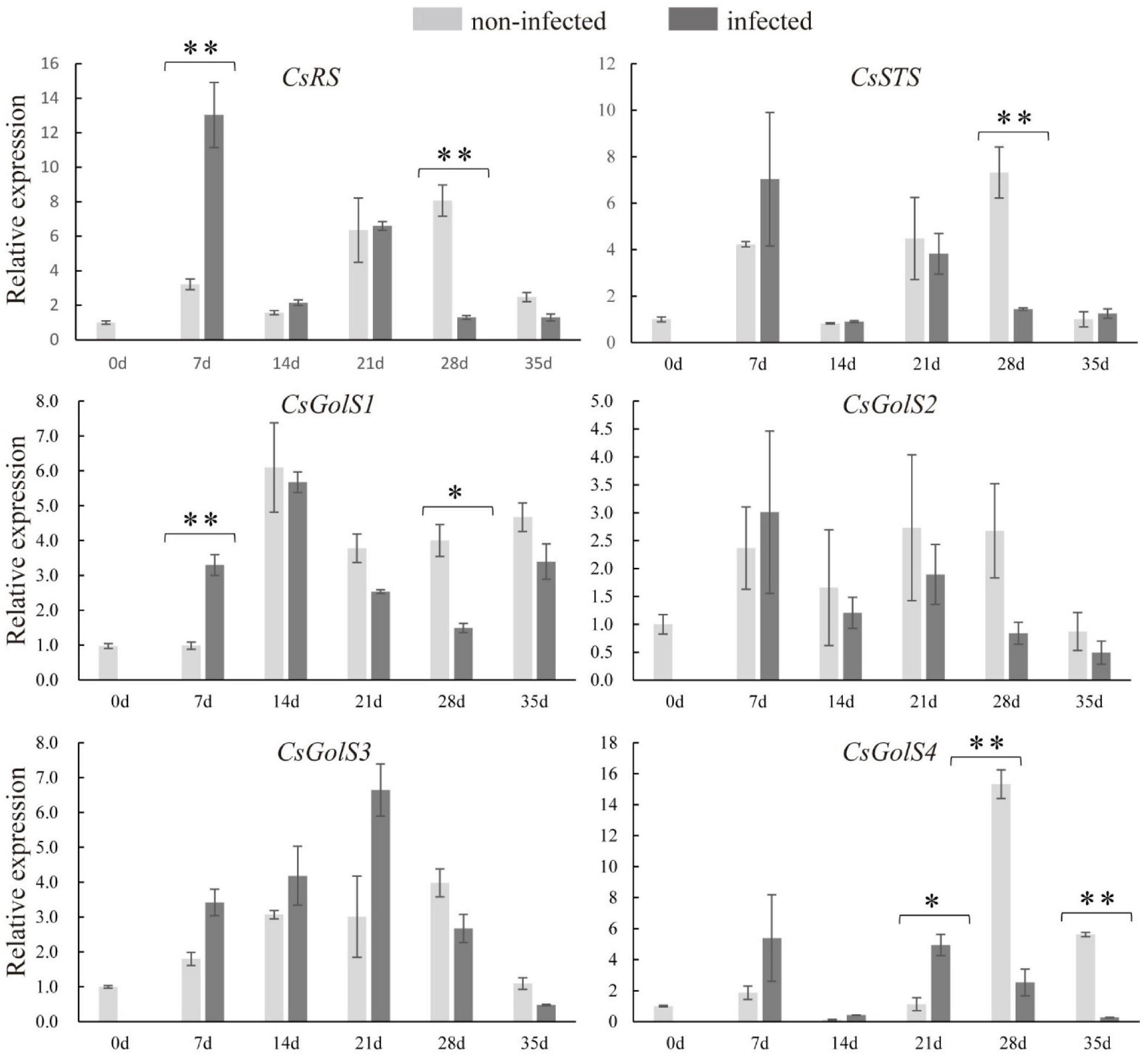
Figure 3. Expression levels of CsRS, CsSTS, CsGolSs in infected and non-infected cucumber roots by M. incognita. CsRS, raffinose synthase gene; CsSTS, stachyose synthase gene; CsGolS1–4, galactinol synthase genes, respectively. Error bars represent ± SE (n = 5). Student’s t-test is analyzed between infected and non-infected roots, *P < 0.05; **P < 0.01.
The expression levels of RFOs degradation-related genes (CsaGAs and CsAGAs) encoding α-galactosidase (α-gal) were also examined. The expression of CsAGA1 was stimulated at 7, 14, 28, and 35 dpi, the expression of CsaGA4 was enhanced at 21 and 28 dpi, whereas the expression of CsaGA2 was suppressed at 7 dpi but then induced at 35 dpi in the infected roots compared with the non-infected roots (Figures 4A–D). However, CsaGA1, 3, and CsAGA2–4 did not respond to nematode infection (Figures 4E–H). The enzyme assay revealed that α-gal activity was significantly suppressed at 7 dpi but enhanced at 21 dpi (Figure 4I). Overall, these results showed that RFO metabolism seemed to be associated with the infection by nematodes.
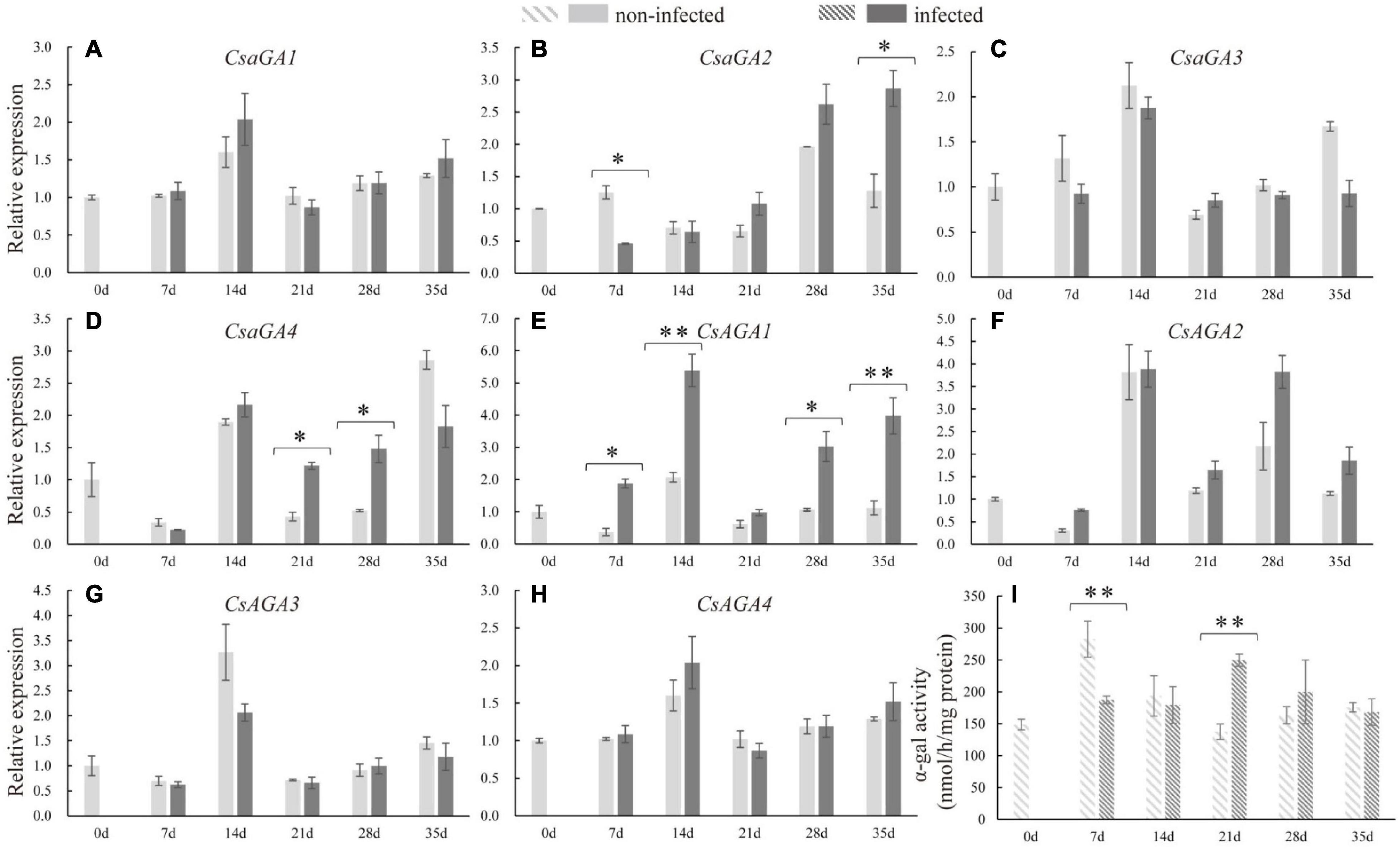
Figure 4. Expression levels of α-galactosidase genes and α-galactosidase activity analysis in infected and non-infected cucumber roots by M. incognita. (A–D) Are acid α-galactosidase genes, CsaGA1 (A), CsaGA2 (B), CsaGA3 (C) and CsaGA4 (D). (E–H) Are alkline α-galactosidase genes, CsAGA1 (E), CsAGA2 (F), CsAGA3 (G) and CsAGA4 (H). (I) Is α-galactosidase activity analysis. Error bars represent ± SE (n = 5). Student’s t-test is analyzed between infected and non-infected roots, *P < 0.05; **P < 0.01.
Sucrose Synthase and Cell Wall Invertase Gene Expressions During Nematode Infection
To explore the molecular basis underlying sucrose metabolism and transport upon infection, related gene expression levels and enzyme activities in infected cucumber roots were measured. At 7 dpi, the expression of CsSUS3 was stimulated. At the late stage of nematode infection, the expressions of CsSUS3 at 28 dpi and CsSUS4 at 21 dpi were enhanced in infected roots (Figures 5C,D). Regarding the expression of CsSPSs in infected roots, CsSPS1 and CsSPS2 were suppressed in infected roots compared with non-infected roots at 7 and 35 dpi, respectively (Supplementary Figure 1). Moreover, at the early stage of nematode infection (at 7 dpi), the expression of CsCWIN5 was increased in infected roots. However, the expression of CsCWIN1 at 21 and 35 dpi, CsCWIN2 and 3 at 28 and 35 dpi, and CsCWIN5 at 14 dpi were suppressed in infected roots (Figures 5F–J). By comparison, nematode infection did not affect the expression of CsSUS1, CsSUS2, CsSPS4, and CsCWIN4 (Figures 5A,B,H and Supplementary Figure 1). The enzyme activity assay revealed that CsSUS was enhanced at 7, 14, and 21 dpi, but decreased at 28 dpi, while CsCWIN was increased at 7 dpi (Figures 5E,K).
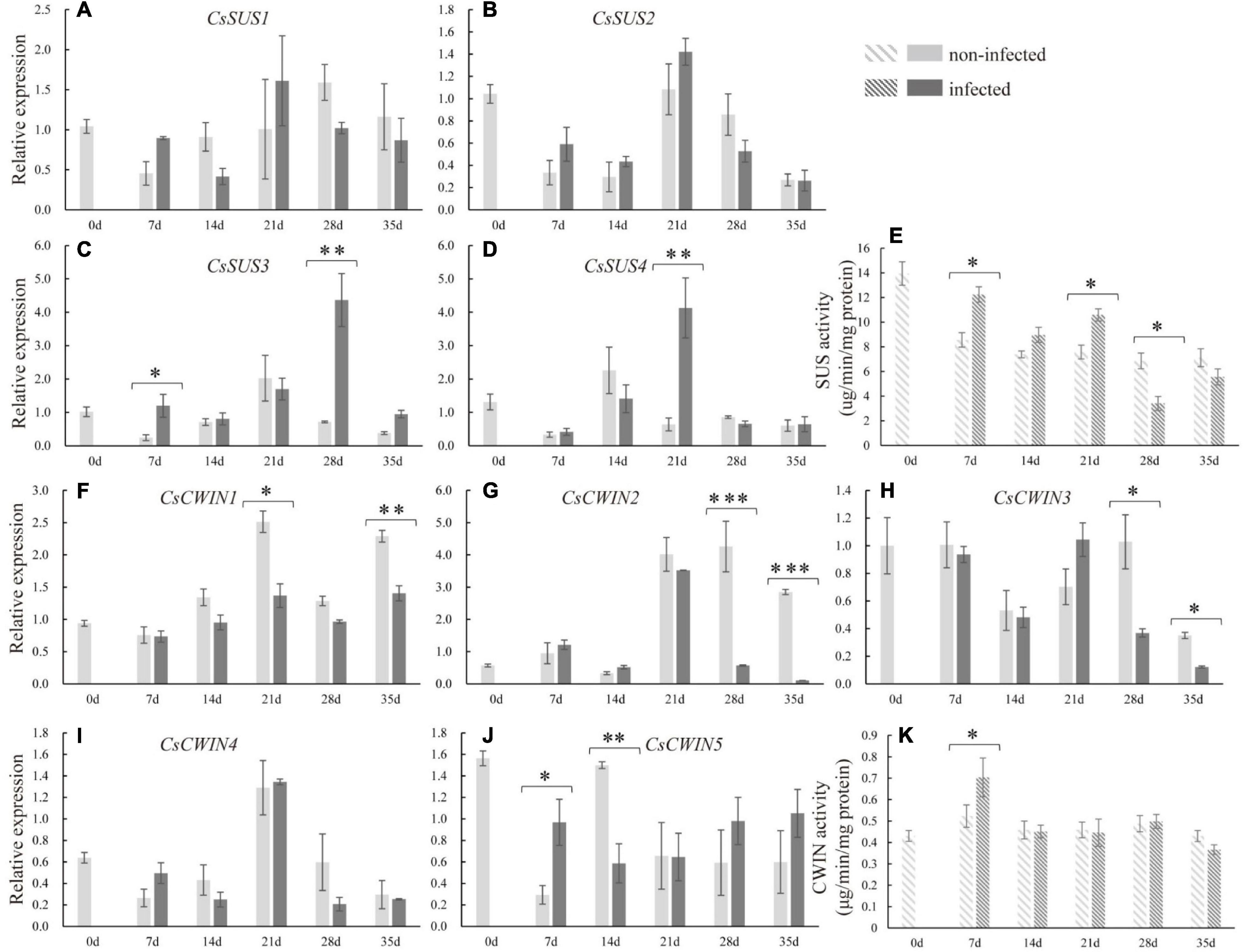
Figure 5. Expression levels of sucrose synthase genes (CsSUS1–4) (A–D), cell wall invertase genes (CsCWIN1–5) (F–J), and the enzyme activity analysis of CsSUS (E) and CWIN (K) in infected and non-infected cucumber roots by M. incognita. Error bars represent ± SE (n = 5). Student’s t-test is analyzed between infected and non-infected roots, *P < 0.05; **P < 0.01; ***P < 0.001.
Responses of Sugar Transporter Genes in Nematode-Infected Roots
In order to identify whether sugar transporter genes respond to nematode infection in cucumber, the expression of CsSUTs and CsHTs were examined. The qRT-PCR analysis revealed that the expression of CsSUT4 was induced at 7 dpi and suppressed at 28 dpi in infected roots (Figure 6), and CsSUT2 expression was increased at 14 dpi in infected roots; however, the expression of CsSUT1 did not respond to nematode infection. The HTs were also involved in sugar partitioning processes. Here, the expression of CsHT1 and CsHT4 was downregulated at 21 and 14 dpi in infected roots, respectively (Supplementary Figure 2). By comparison, the expression of CsSUT1 and CsHT3 showed no change in infected roots by nematode infection (Figure 6 and Supplementary Figure 2).
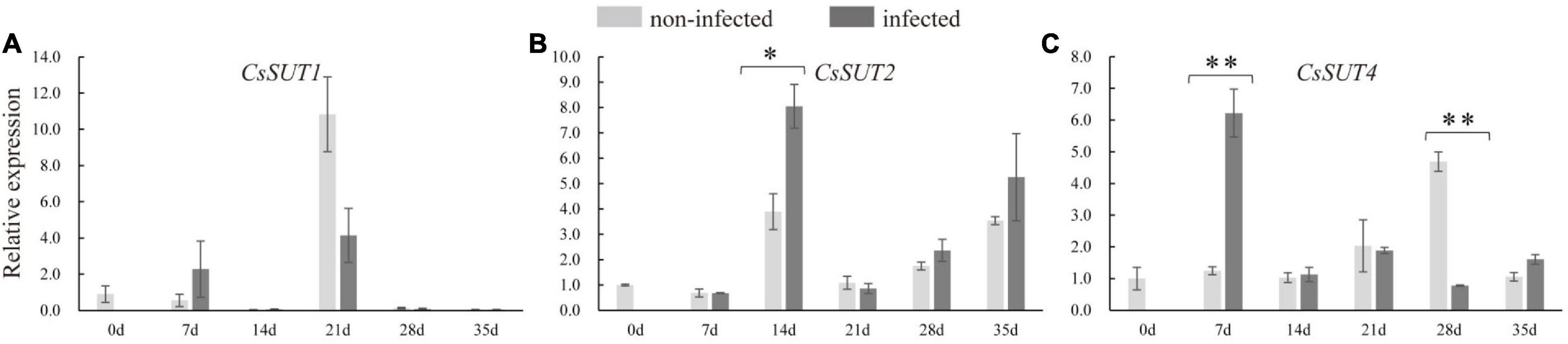
Figure 6. Expression levels of sucrose transporter genes (CsSUTs) (A–C) in infected and non-infected cucumber roots by M. incognita. Error bars represent ± SE (n = 5). Student’s t-test is analyzed between infected and non-infected roots, *P < 0.05; **P < 0.01.
Discussion
The biotrophic parasites could alter carbohydrate metabolism in infected host plants to acquire sugars to fuel their growth (Hofmann et al., 2007a). Previous studies have described the changes of sugar metabolism and import into syncytia of sucrose-transporting species in response to H. schachtii infection (Hammes et al., 2005; Hofmann et al., 2007a,b; Cabello et al., 2014). However, it remains elusive how sucrose or RFOs are imported into and utilized within the M. incognita-induced GCs in RFOs-transported species.
Dual Roles of Raffinose Family Oligosaccharides in Nematode Resistance and Feeding
The first step of nematode infection in host is to break the physical barrier, cell wall, for entering the host’s vascular cylinder. Plethora studies suggested that the intracellular accumulation of RFOs metabolic enzymes are closely associated with the response to environmental stresses no matter in sucrose- or RFOs-transported species (Klotke et al., 2004; Peters and Keller, 2009; Ma et al., 2021). Previous studies have supported that RFOs could be inserted into lipid headgroups of membrane bilayer and stabilize membrane when under stress, and RFOs could interact with ROS signaling pathway and act as a free radical scavenger (Hincha et al., 2003; Nishizawa et al., 2008). In Arabidopsis, a sucrose-transported species, the sugar was transported into feeding sites induced by RKNs/cyst nematodes and stored as starch for carbohydrate need of nematodes (Hofmann et al., 2007a). In addition, previous studies have revealed that plants produce osmotic substances, such as sucrose and RFOs, to resist stress (Zulfiqar et al., 2020). In previous study, after being infected by cyst nematode, raffinose and galactinol were increased, whereas stachyose was not detected in Arabidopsis roots over the course of development (from 5 to 15 dpi) (Hofmann et al., 2010). In infected cucumber, stachyose content was increased in roots at early stage (7 dpi), with a similar pattern observed in raffinose and galactinol (Figure 2), and the expression of CsRS was significantly induced at 7 dpi, it was possibly that synthesized raffinose and galactinol may be further converted to stachyose by STS in the infected cucumber roots. This result showed that stachyose plays a key role at early stage of nematode infection in cucumber.
In the current study, at the late stage of nematode infection, stachyose, raffinose, and galactinol contents were decreased, consistent with the downregulated transcript level of CsSTS, CsRS, and CsGolS1 and increased α-gal activity in infected cucumber roots (Figures 2, 3), whereas glucose was significantly accumulated in infected roots at 21 dpi (Figure 2). These results suggested that RFOs were degraded by α-galactosidase likely for providing sucrose and hexose at the late stage for nematode development, which was also supported by the induced α-gal enzyme activity at 21 dpi (Figure 4). The infected cucumber roots accumulated RFOs at the early stage of nematode infection (Figure 2). We speculated that the promoted RFOs might be relevant to resist the nematode infection at the early stage. However, the RFOs tended to be cleaved for supplying sugar for nematode spawning at the late stage of nematode infection. Thus, we proposed that the RFOs could play dual roles in the host-nematode interactions in cucumber, including resistance at the early stage and fueling nutrient at the late stage. This finding supports the possibility to breed nematode-resistant cultivars through manipulating RFO metabolism in Cucurbitaceae plants.
Different Responses of CsSUSs and CsCWINs After Nematode Infection
In this study, the glucose was increased significantly at the late stage of nematode infection, but the RFOs content was decreased (Figures 2B,E). This finding suggests that, in cucumber, the glucose was the major induced sugar in galls and thus may play important role in fueling nematode development. Consistently, the expressions of CsSUS3 and -4 were enhanced in cucumber roots after infecting nematode, and the CsSUS activity was also promoted at 7 and 21 dpi (Figures 5A–D). Interestingly, the genes encoding CWIN, CsCWIN5 was induced at the early stage, while CsCWIN1, -2, and -3 were decreased at the late stage, in infected root (Figures 5F–J). As CsSUS was the only promoted sucrose-degrading enzyme at the late stage, the increased glucose at 21 dpi was very likely generated from sucrose degradation by SUS. The CsCWIN activity was induced only at the early stage, but not at the late stage, of nematode infection (Figure 5), which was in accordance with the enhanced expression of CsCWIN5 (Figure 5J). The transcriptional level of CsCWINs and enzyme activity of CsCWIN showed different change trends at the late stage of nematode infection, and this was possibly caused by posttranscriptional regulation or posttranslational regulation (Jin et al., 2009). It had been proposed that invertase was posttranscriptionally regulated when plants suffered from biotic stresses (Bonfig et al., 2010). In this study, the result of increased CsCWIN and CsSUS activity in infected cucumber roots was similar with previous findings that SUS and CWIN activity was induced in syncytia (3 dpi) (Cabello et al., 2014). Together with the previous findings on SUS and CWIN mutants of Arabidopsis demonstrating less resistance to H. schaschtii (Cabello et al., 2014), our finding suggests that in cucumber, a RFO-transporting species, CsCWIN and CsSUS may relate to nematode resistance. In this study, glucose was promoted at the late stage (Figure 2), which may be contributed by (i) sucrose degradation by enzymes of SUS and CWIN and (ii) galactose metabolism after RFO being degraded into galactose by α-gal. This statement is supported by the promoted SUS and CWIN and decreased RFO contents at the later stage of nematode infection (Figures 2, 5). The roles of SUS and CWIN in nematode infection and resistance deserve further investigation, and evidence regarding functional identification, protein localization, and other methodologies may provide more insight into the parasitism of nematode in cucumber.
Collectively, we propose that the RFOs were largely synthesized by CsRS, CsSTS, and CsGolS1 at the early stage for resisting the infection of nematode. Afterward, upon the nematode has infected and proceed to develop, the RFOs were cleaved by α-gal encoded by CsAGA1 and CsaGA4 to sucrose and galactose. The sucrose was further degraded by CsSUS encoded by CsSUS3, -4 into hexoses, providing energy and carbon to supply nematode development (Figure 7). These findings identified the candidate genes involved in nematode infection for further studies and provided new insight into the sugar metabolic dynamics in cucumber roots infected by M. incognita.
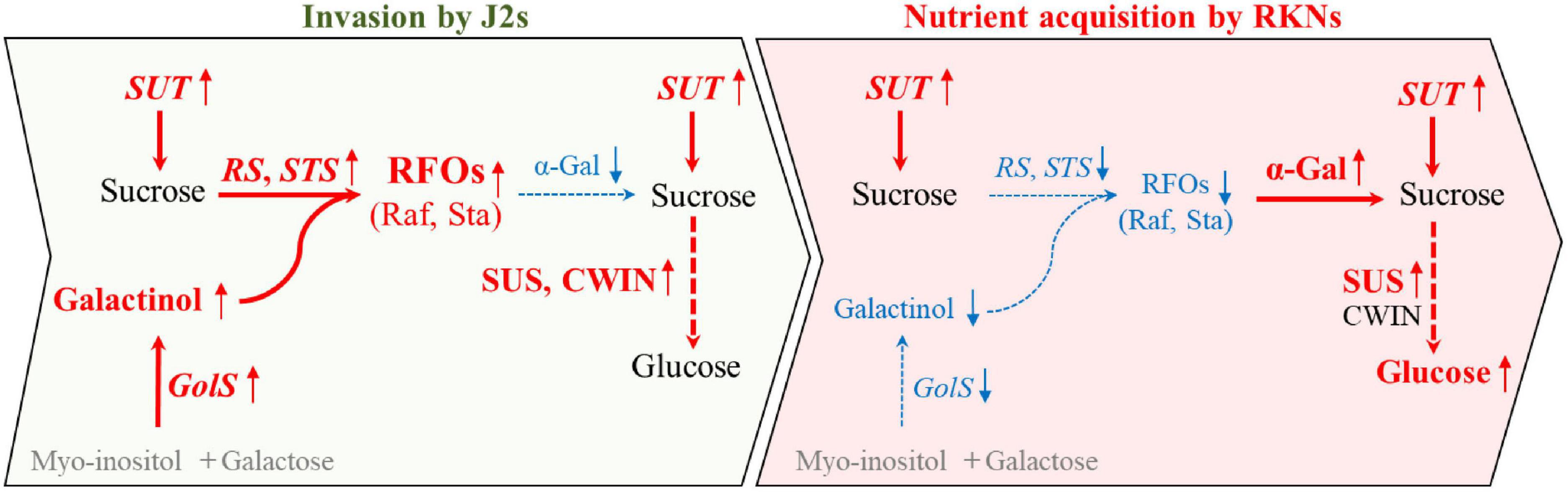
Figure 7. A model describing the distinct responses of sugar contents and the expression of metabolic genes at early (invasion by J2s) and later (nutrient acquisition by RKNs) stages during infection. During the invasion of nematode in cucumber roots, CsGolS was induced and galactinol was synthesized with myo-inositol and galactose. Further, RFOs may be largely synthesized by CsRS and CsSTS from galactinol and sucrose which was transported by SUT at early stage, possibly for resisting the infection of nematode. Afterward, upon nematode development, RFOs may be cleaved to sucrose and galactose by α-gal. Furthermore, sucrose was degraded by SUS encoded into hexoses at early and late stage of nematode infection, providing energy and carbon to supply nematode development. Red font and blue font indicate increase and decrease after nematode infection compare with non-infection, respectively. Black font imply no differences between infected and non-infected by nematode. Gray parts have not been detected in this study.
Data Availability Statement
The datasets presented in this study can be found in online repositories. The names of the repository/repositories and accession number(s) can be found in the article/Supplementary Material.
Author Contributions
SM and YT conceived the project and designed the experiments. XW, SL, and XZ conducted the experiments and collected the data. SM, XW, and SL analyzed the data and wrote the manuscript with inputs from Y-LR and LG. All authors contributed to the article and approved the submitted version.
Funding
This work was financially supported by the National Natural Science Foundation of China (Nos. 31972478 and 32002026), the National Key Research and Development Program of China (No. 2019YFD1001900), and the China Agriculture Research System (No. CARS-23).
Conflict of Interest
The authors declare that the research was conducted in the absence of any commercial or financial relationships that could be construed as a potential conflict of interest.
Publisher’s Note
All claims expressed in this article are solely those of the authors and do not necessarily represent those of their affiliated organizations, or those of the publisher, the editors and the reviewers. Any product that may be evaluated in this article, or claim that may be made by its manufacturer, is not guaranteed or endorsed by the publisher.
Acknowledgments
We would like to thank Si Shen (China Agricultural University) and Jun Li (Zhejiang University) for editing this manuscript.
Supplementary Material
The Supplementary Material for this article can be found online at: https://www.frontiersin.org/articles/10.3389/fpls.2022.823382/full#supplementary-material
References
Abad, P., Gouzy, J., Aury, J.-M., Castagnone-Sereno, P., Danchin, E. G. J., Deleury, E., et al. (2008). Genome sequence of the metazoan plant-parasitic nematode Meloidogyne incognita. Nat. Biotechnol. 26, 909–915. doi: 10.1038/nbt.1482
Bartlem, D. G., Jones, M. G., and Hammes, U. Z. (2014). Vascularization and nutrient delivery at root-knot nematode feeding sites in host roots. J. Exp. Bot. 65, 1789–1798. doi: 10.1093/jxb/ert415
Bonfig, K. B., Gabler, A., Simon, U. K., Luschin-Ebengreuth, N., Hatz, M., Berger, S., et al. (2010). Post-translational derepression of invertase activity in source leaves via down-regulation of invertase inhibitor expression is part of the plant defense response. Mol. Plant 3, 1037–1048. doi: 10.1093/mp/ssq053
Bozbuga, R., Lilley, C. J., Knox, J. P., and Urwin, P. E. (2018). Host-specific signatures of the cell wall changes induced by the plant parasitic nematode, Meloidogyne incognita. Sci. Rep. 8:17302. doi: 10.1038/s41598-018-35529-7
Braun, D. M., Wang, L., and Ruan, Y. L. (2014). Understanding and manipulating sucrose phloem loading, unloading, metabolism, and signalling to enhance crop yield and food security. J. Exp. Bot. 65, 1713–1735. doi: 10.1093/jxb/ert416
Brinkman, E. P., Duyts, H., Karssen, G., van der Stoel, C. D., and van der Putten, W. H. (2015). Plant-feeding nematodes in coastal sand dunes: occurrence, host specificity and effects on plant growth. Plant Soil 397, 17–30. doi: 10.1007/s11104-015-2447-z
Bybd, D. W., Kirkpatrick, T., and Barker, K. R. (1983). An improved technique for clearing and staining plant tissues for detection of nematodes. J. Nematol. 15, 142–143.
Cabello, S., Lorenz, C., Crespo, S., Cabrera, J., Ludwig, R., Escobar, C., et al. (2014). Altered sucrose synthase and invertase expression affects the local and systemic sugar metabolism of nematode-infected Arabidopsis thaliana plants. J. Exp. Bot. 65, 201–212. doi: 10.1093/jxb/ert359
Castagnone-Sereno, P., Danchin, E. G. J., Perfus-Barbeoch, L., and Abad, P. (2013). Diversity and evolution of root-knot nematodes, genus Meloidogyne: new insights from the genomic era. Annu. Rev. Phytopathol. 51, 203–220. doi: 10.1146/annurev-phyto-082712-102300
Ding, X., Shields, J., Allen, R., and Hussey, R. S. (1998). A secretory cellulose-binding protein cDNA cloned from the root-knot nematode (Meloidogyne incognita). Mol. Plant Microbe Interact. 11, 952–959. doi: 10.1094/mpmi.1998.11.10.952
Escobar, C., Barcala, M., Cabrera, J., and Fenoll, C. (2015). “Chapter One–Overview of root-knot nematodes and giant cells,” in Advances in Botanical Research, eds C. Escobar and C. Fenoll (Cambridge, MA: Academic Press), 1–32.
Gommers, F. J., and Dropkin, V. H. (1977). Quantitative histochemistry of nematode-induced transfer cells. Phytopathology 67, 869–873. doi: 10.1094/phyto-67-869
Hammes, U. Z., Schachtman, D. P., Berg, R. H., Nielsen, E., Koch, W., McIntyre, L. M., et al. (2005). Nematode-induced changes of transporter gene expression in Arabidopsis roots. Mol. Plant Microbe Interact. 18, 1247–1257. doi: 10.1094/mpmi-18-1247
Hincha, D. K., Zuther, E., and Heyer, A. G. (2003). The preservation of liposomes by raffinose family oligosaccharides during drying is mediated by effects on fusion and lipid phase transitions. Biochim. Biophys. Acta Biomembr. 1612, 172–177. doi: 10.1016/S0005-2736(03)00116-0
Hofmann, J., El Ashry, A. E. N., Anwar, S., Erban, A., Kopka, J., and Grundler, F. (2010). Metabolic profiling reveals local and systemic responses of host plants to nematode parasitism. Plant J. 62, 1058–1071. doi: 10.1111/j.1365-313X.2010.04217.x
Hofmann, J., and Grundler, F. (2007). How do nematodes get their sweets? Solute supply to sedentary plant-parasitic nematodes. Nematology 9, 451–458. doi: 10.1163/156854107781487305
Hofmann, J., Kolev, P., Kolev, N., Daxböck-Horvath, S., and Grundler, F. M. W. (2009). The Arabidopsis thaliana sucrose transporter gene AtSUC4 is expressed in Meloidogyne incognita-induced root galls. J. Phytopathol. 157, 256–261. doi: 10.1111/j.1439-0434.2008.01468.x
Hofmann, J., Wieczorek, K., Blöchl, A., and Grundler, F. M. W. (2007b). Sucrose supply to nematode-induced syncytia depends on the apoplasmic and symplasmic pathways. J. Exp. Bot. 58, 1591–1601. doi: 10.1093/jxb/erl285
Hofmann, J., Szakasits, D., Blöchl, A., Sobczak, M., Daxböck-Horvath, S., Golinowski, W., et al. (2007a). Starch serves as carbohydrate storage in nematode-induced syncytia. Plant Physiol. 146, 228–235. doi: 10.1104/pp.107.107367
Hu, L., Sun, H., Li, R., Zhang, L., Wang, S., Sui, X., et al. (2011). Phloem unloading follows an extensive apoplasmic pathway in cucumber (Cucumis sativus L.) fruit from anthesis to marketable maturing stage. Plant Cell Environ. 34, 1835–1848. doi: 10.1111/j.1365-3040.2011.02380.x
Jacquet, M., Bongiovanni, M., Martinez, M., Verschave, P., Wajnberg, E., and Castagnone-Sereno, P. (2005). Variation in resistance to the root-knot nematode Meloidogyne incognita in tomato genotypes bearing the Mi gene. Plant Pathol. 54, 93–99. doi: 10.1111/j.1365-3059.2005.01143.x
Jin, Y., Ni, D.-A., and Ruan, Y.-L. (2009). Posttranslational elevation of cell wall invertase activity by silencing its inhibitor in tomato delays leaf senescence and increases seed weight and fruit hexose level. Plant Cell 21, 2072–2089. doi: 10.1105/tpc.108.063719
Kayani, M. Z., Mukhtar, T., and Hussain, M. A. (2017). Effects of southern root knot nematode population densities and plant age on growth and yield parameters of cucumber. Crop Prot. 92, 207–212. doi: 10.1016/j.cropro.2016.09.007
Klotke, J., Kopka, J., Gatzke, N., and Heyer, A. G. (2004). Impact of soluble sugar concentrations on the acquisition of freezing tolerance in accessions of Arabidopsis thaliana with contrasting cold adaptation-evidence for a role of raffinose in cold acclimation. Plant Cell Environ. 27, 1395–1404. doi: 10.1111/j.1365-3040.2004.01242.x
Li, X., Sun, Y., Yang, Y., Yang, X., Xue, W., Wu, M., et al. (2021). Transcriptomic and histological analysis of the response of susceptible and resistant cucumber to Meloidogyne incognita infection revealing complex resistance via multiple signaling pathways. Front. Plant Sci. 12:675429. doi: 10.3389/fpls.2021.675429
Ma, S., Lv, J., Li, X., Ji, T., Zhang, Z., and Gao, L. (2021). Galactinol synthase gene 4 (CsGolS4) increases cold and drought tolerance in Cucumis sativus L. by inducing RFO accumulation and ROS scavenging. Environ. Exp. Bot. 185:104406. doi: 10.1016/j.envexpbot.2021.104406
Ma, S., Sun, L., Sui, X., Li, Y., Chang, Y., Fan, J., et al. (2019). Phloem loading in cucumber: combined symplastic and apoplastic strategies. Plant J. 98, 391–404. doi: 10.1111/tpj.14224
Nishizawa, A., Yabuta, Y., and Shigeoka, S. (2008). Galactinol and raffinose constitute a novel function to protect plants from oxidative damage. Plant Physiol. 147, 1251–1263. doi: 10.1104/pp.108.122465
Palmer, W. M., Ru, L., Jin, Y., Patrick, J. W., and Ruan, Y. L. (2015). Tomato ovary-to-fruit transition is characterized by a spatial shift of mRNAs for cell wall invertase and its inhibitor with the encoded proteins localized to sieve elements. Mol. Plant 8, 315–328. doi: 10.1016/j.molp.2014.12.019
Pennycooke, J. C., Jones, M. L., and Stushnoff, C. (2003). Down-regulating α-galactosidase enhances freezing tolerance in transgenic Petunia. Plant Physiol. 133, 901–909. doi: 10.1104/pp.103.024554
Perry, R. N., and Moens, M. (2011). “Introduction to plant-parasitic nematodes; modes of parasitism,” in Genomics and Molecular Genetics of Plant-Nematode Interactions, eds J. Jones, G. Gheysen, and C. Fenoll (Dordrecht: Springer Netherlands), 3–20.
Peters, S., and Keller, F. (2009). Frost tolerance in excised leaves of the common bugle (Ajuga reptans L.) correlates positively with the concentrations of raffinose family oligosaccharides (RFOs). Plant Cell Environ. 32, 1099–1107. doi: 10.1111/j.1365-3040.2009.01991.x
Ros-Ibáñez, C., Robertson, L., Martínez-Lluch, M., Cano-García, A., and Lacasa-Plasencia, A. (2014). Development of virulence to Meloidogyne incognita on resistant pepper rootstocks. Span. J. Agric. Res. 12, 225–232.
Schmittgen, T. D., and Livak, K. J. (2008). Analyzing real-time PCR data by the comparative CT method. Nat. Protoc. 3, 1101–1108. doi: 10.1038/nprot.2008.73
Slewinski, T. L. (2011). Diverse functional roles of monosaccharide transporters and their homologs in vascular plants: a physiological perspective. Mol. Plant 4, 641–642. doi: 10.1093/mp/ssr051
Sui, X., Nie, J., Li, X., Scanlon, M. J., Zhang, C., Zheng, Y., et al. (2018). Transcriptomic and functional analysis of cucumber (Cucumis sativus L.) fruit phloem during early development. Plant J. 96, 982–996. doi: 10.1111/tpj.14084
Taji, T., Ohsumi, C., Iuchi, S., Seki, M., Kasuga, M., Kobayashi, M., et al. (2002). Important roles of drought- and cold-inducible genes for galactinol synthase in stress tolerance in Arabidopsis thaliana. Plant J. 29, 417–426. doi: 10.1046/j.0960-7412.2001.01227.x
Wang, H., Sui, X., Guo, J., Wang, Z., Cheng, J., Ma, S., et al. (2014). Antisense suppression of cucumber (Cucumis sativus L.) sucrose synthase 3 (CsSUS3) reduces hypoxic stress tolerance. Plant Cell Environ. 37, 795–810. doi: 10.1111/pce.12200
Xu, L., Xiao, L., Xiao, Y., Peng, D., Xiao, X., Huang, W., et al. (2021). Plasmodesmata play pivotal role in sucrose supply to Meloidogyne graminicola-caused giant cells in rice. Mol. Plant Pathol. 22, 539–550. doi: 10.1111/mpp.13042
Keywords: Cucumis sativus, Meloidogyne incognita, sugar metabolism, RFOs, biotic stress
Citation: Wang X, Li S, Zhang X, Gao L, Ruan Y-L, Tian Y and Ma S (2022) From Raffinose Family Oligosaccharides to Sucrose and Hexoses: Gene Expression Profiles Underlying Host-to-Nematode Carbon Delivery in Cucumis sativus Roots. Front. Plant Sci. 13:823382. doi: 10.3389/fpls.2022.823382
Received: 27 November 2021; Accepted: 21 January 2022;
Published: 17 February 2022.
Edited by:
Essaid Ait Barka, Université de Reims Champagne-Ardenne, FranceReviewed by:
Tariq Mukhtar, Pir Mehr Ali Shah Arid Agriculture University, PakistanKamal Aberkani, Mohamed Premier University, Morocco
Copyright © 2022 Wang, Li, Zhang, Gao, Ruan, Tian and Ma. This is an open-access article distributed under the terms of the Creative Commons Attribution License (CC BY). The use, distribution or reproduction in other forums is permitted, provided the original author(s) and the copyright owner(s) are credited and that the original publication in this journal is cited, in accordance with accepted academic practice. No use, distribution or reproduction is permitted which does not comply with these terms.
*Correspondence: Yongqiang Tian, tianyq1984@cau.edu.cn; Si Ma, masi@cau.edu.cn
†These authors have contributed equally to this work