- 1Agri-Biotechnology Division, National Agri-Food Biotechnology Institute, Mohali, India
- 2Department of Biotechnology Engineering, University Institute of Engineering and Technology, Panjab University, Chandigarh, India
- 3Department of Immunopathology, Post Graduate Institute of Medical and Education and Research, Chandigarh, India
- 4School of Agricultural Biotechnology, Punjab Agricultural University, Ludhiana, India
Recent weather fluctuations imposing heat stress at the time of wheat grain filling cause frequent losses in grain yield and quality. Field-based studies for understanding the effect of terminal heat stress on wheat are complicated by the effect of multiple confounding variables. In the present study, the effect of day and day–night combined heat stresses during the grain-filling stage was studied using gene expression and proteomics approaches. The gene expression analysis was performed by using real-time quantitative PCR (RT-qPCR). The expression of genes related to the starch biosynthetic pathway, starch transporters, transcription factors, and stress-responsive and storage proteins, at four different grain developmental stages, indicated the involvement of multiple pathways. Under the controlled conditions, their expression was observed until 28 days after anthesis (DAA). However, under the day stress and day–night stress, the expression of genes was initiated earlier and was observed until 14 DAA and 7 DAA, respectively. The protein profiles generated using two-dimensional polyacrylamide gel electrophoresis (2D-PAGE) and matrix-assisted laser desorption/ionization time-of-flight mass spectroscopy (MALDI-TOF MS/MS) showed a differential expression of the proteins belonging to multiple pathways that included the upregulation of proteins related to the translation, gliadins, and low-molecular-weight (LMW) glutenins and the downregulation of proteins related to the glycolysis, photosynthesis, defense, and high-molecular-weight (HMW) glutenins. Overall, the defense response to the day heat stress caused early gene expression and day–night heat stress caused suppression of gene expression by activating multiple pathways, which ultimately led to the reduction in grain-filling duration, grain weight, yield, and processing quality.
Introduction
World population by enlarging depends on wheat as a cereal food. Despite significant improvement in wheat production achieved over the last couple of decades, wheat production is always threatened due to biotic and abiotic stress. Recent climate change has experienced frequent episodes of unexpected fluctuations in temperature. Such adverse temperatures cause severe losses, particularly during the grain-filling stage in wheat. Despite being a high-yielding cereal crop, wheat is sensitive to heat stress (Porter and Gawith, 1999). The optimum temperature at the wheat reproductive period is 18–24°C (Stone et al., 1997). The high temperatures during the plant growth and development might cause 2.5–10% of yield losses in field crops (Hatfield et al., 2011). In the case of wheat, every 1°C increase above the optimum temperature reduces the yield by 4–6% (Bennett et al., 2012; Liu et al., 2016). Many climate models on global warming had predicted that by the end of the Twenty-first century, the optimum temperature of the globe would increase up to 6°C (De Costa, 2011).
In many countries, heat stress is mainly experienced during seed development. Although all the growth stages of wheat are sensitive to high-temperature stresses, the reproductive phase is the most critical. A slight elevation in temperature during the pollination affects pollen sterility, floret abortion, damage to stigma, and fertilization (Wollenweber et al., 2003; Kumar et al., 2015; Rieu et al., 2017). The terminal heat stress reduces photosynthesis due to thylakoid membrane damage (Ristic et al., 2007) and inhibition of photosystem II (PSII) (Wollenweber et al., 2003). Heat stress during the reproductive stage also increases the senescence rate, resulting in the decrease of grain-filling duration and the subsequent decrease in the grain weight, defragmented starch granules, and seed quality (Hays et al., 2007; Kumar et al., 2013). Heat stress also causes downregulation of several genes related to various pathways. High postanthesis temperature downregulated the genes involved in the pericarp cell wall expansion leading to reduction in matured grain weight (Kino et al., 2020). Rangan et al. (2020) identified a cluster of genes that are upregulated in the heat-tolerant cultivar during early and late grain-filling heat stress as compared with the susceptible cultivar. Recent genome-wide studies have identified various heat shock protein (HSP) genes and transcription factors under heat and drought stresses (Liu et al., 2015; Kumar et al., 2020).
Most of the previous studies had addressed the effects of heat stress imposed only either during daytime (Wang et al., 2015) or nighttime (García et al., 2015; Impa et al., 2019). Besides, limited efforts are employed to reveal the molecular mechanisms explaining yield reduction in heat stress. Some studies report single time-point stress (Impa et al., 2020), while others are limited to a few hours of heat stress (Kumar et al., 2014). Only a few studies had observed the gene expression throughout the grain-filling period, but they were restricted only to the genes involved in the starch biosynthesis pathway (Hurkman et al., 2003). Although gene expression is important to investigate the heat stress response in developing an endosperm, ultimately, the protein content and composition of matured grains determine the processing quality of wheat. Recently, high-throughput proteomics approaches, such as two-dimensional gel electrophoresis/mass spectroscopy (2DE/MS) and iTRAQ, have become powerful tools for identifying and quantifying large number of differentially expressed proteins (Finnie et al., 2011; Zhang et al., 2017). In this study, the effects of day stress and day–night combined stress on gene expression at different grain-filling stages were investigated. Efforts were also being made to understand the protein profiles of the matured grains by 2D polyacrylamide gel electrophoresis (2D-PAGE) combined with the matrix-assisted laser desorption/ionization time-of-flight mass spectroscopy (MALDI-TOF MS/MS) analysis.
Materials and Methods
Plant Material and Growth Conditions
The current study used BWL4444 (HD2967+ Yr10), a promising heat-tolerant wheat germplasm to understand the heat stress response during the grain-filling period. The germplasm was obtained from Punjab Agricultural University, Ludhiana, Punjab, India. A total of 120 seeds were sown in 400 ml pots filled with vermiculite. Osgrel and Somerville soil-less planting media were supplied to each pot. The plants were grown under controlled conditions of 24/17°C and 16 h of light (300 μM m−2 s−2) until anthesis. Two days after anthesis (DAA), plants were divided into three sets of 40 plants each. These plants were then subjected to three temperature regimes—(1) control (24/17°C), (2) day heat stress (35/17°C), and (3) day–night heat stress (35/24°C). The heat treatment was continued until maturity. To simulate the field conditions, the temperature in the growth chambers was gradually increased at the rate of 2–3°C/h, starting at 6.00 A.M., and the highest temperature of 35°C was achieved at 11.00 A.M. The highest temperature was provided for 6 h from 11.00 A.M. to 5.00 P.M. From 5.00 P.M. to 9.00 P.M., the temperature was then gradually reduced at the rate of 2–3°C/h to 17°C or 24°C for day stress and day–night stress, respectively. The night heat treatment in the third set of plants was provided for 10 h from 9.00 P.M. to 7.00 A.M. (Supplementary Table 1).
Biochemical Parameters
Flag leaves from more than three biological replicates were collected at 7 DAA at 5 P.M. They were immediately frozen in liquid nitrogen and stored at −80°C for biochemical analysis. Proline was quantified according to Bates et al. (1973), with slight modification. Of note, 0.2 g of fresh leaves were homogenized with 2 ml of 3% aqueous sulfosalicylic acid. Furthermore, 1 ml of acid ninhydrin solution and 1 ml of glacial acetic acid were added to 1 ml of the supernatant and incubated in a boiling water bath for 60 min. After cooling down to the room temperature, 4 ml of toluene was added to the reaction mixture and vortexed thoroughly. The absorbance of the toluene layer was measured at 520 nm using toluene as a blank.
For estimating hydrogen peroxide (H2O2), 0.2 g of fresh leaves were homogenized in 2 ml of 0.1% trichloroacetic acid (TCA) (Alexieva et al., 2001). Furthermore, 1 ml of the supernatant was mixed with 2 ml of assay mixture containing 4 mM of potassium iodide and 0.1 mM of potassium phosphate buffer (pH 7.0) and incubated at the room temperature in the dark for 1 h. The absorbance was read at 390 nm, and the concentration was quantified using the H2O2 standard curve.
Extraction of the antioxidant enzyme was carried out according to Yang et al. (2008). Of note, 200 mg of leaf tissue was homogenized in 2 ml of buffer (50 mM of potassium phosphate buffer [pH 7.0], 1 mM of EDTA, 2% polyvinyl pyrrolidone (PVP), and 0.05% Triton-X-100). The homogenate was then centrifuged at 10,000 × g at 4°C for 15 min. Guaiacol peroxidase (GPOX) was assayed (Simova-Stoilova et al., 2008) by mixing 50 μl of enzyme extract with 2.5 ml of buffer containing 100 mM of potassium phosphate buffer (pH 6.5), 50 mM of guaiacol, and 32 mM of H2O2. A change in absorbance at 470 nm was recorded at 25°C. A change in mM of tetraguaiacol per minute was calculated using the molar extinction coefficient of tetraguaiacol (26.6/mM/cm).
Yield-Related Parameters
At physiological maturity, the primary tillers from each plant were harvested, and yield-related components were recorded. The kernels from each spikelet were harvested separately, and kernel length and width were measured using a vernier caliper. On an average, we took two kernels/spikelet from four different spikes for measuring kernel dimensions. Kernel weight per spike and thousand kernel weight (TKW) were also recorded.
Differential Gene Expression
For differential gene expression, the developing grains were collected at 7, 14, 21, and 28 DAA at 5.00 P.M. and immediately frozen in liquid nitrogen and stored at −80°C. Total RNA was isolated from the developing grains using the TRIZOL® reagent (Ambion, Austin, Texas, USA). Two micrograms of DNA-free RNA were used for the first-strand cDNA synthesis using the first strand cDNA synthesis kit (ThermoFisher, Massachusetts, USA) with random hexamer primers as per the guidelines of the manufacturer. The real-time quantitative PCR (RT-qPCR) was performed for selected candidate genes involved in starch biosynthesis and transport, storage protein synthesis, stress response, and transcription factors (Supplementary Table 2) using SYBR Green Real-Time-PCR Master Mix (Ambion) on ABI 7700 sequence detector (Applied Biosystems, Foster City, CA, USA). Each reaction was carried out in three biological replicates and with three technical replicates each. Amplification was performed according to the default cycle, and fold change values were calculated based on average 2−ΔΔCT values with ADP-ribosylation factor (ADPRF) as an internal control (Livak and Schmittgen, 2001; Kumar et al., 2020). MeV (multiexperiment viewer) software was used for the data analysis.
Proteomic Analysis
Protein Extraction and Quantification
For proteome analysis, mature grains of more than three biological replicates were collected. Protein extraction was performed sequentially, and separate fractions of albumins–globulins (0.1 M sodium chloride in 25 mM sodium phosphate buffer of pH 7.0), gliadins (1.5 M dimethylformamide), and glutenins [50 mM Tris-HCL dissolved in 50% (v/v) isopropanol] (Garg et al., 2014) were collected. The supernatants from different fractions were precipitated with four volumes of acetone containing 10% trichloroacetic acid (TCA) and 20 mM dithiothreitol (DTT). The pellets were washed with 20 mM DTT in 100% acetone, air-dried, and solubilized in 1 ml of rehydration buffer containing 7 M urea, 2 M thiourea, 4% CHAPS, 30 mM Tris, and 40 mM DTT. The protein concentration was measured using the 2D-quant kit (GE Healthcare, Chicago, Illinois, USA). The samples were stored at −20°C for further analysis.
Two-Dimensional Gel Electrophoresis
For the first dimension, the IPG strips (linear pH 3–10, 13 cm, GE Healthcare) were rehydrated in 250 μl of rehydration buffer containing 150 μg of protein sample and 1% ampholyte with pH 3–10 (GE Healthcare). The isoelectric focusing (IEF) was performed using Etton IPGphor 3 (GE Healthcare). After IEF, the strips were subsequently equilibrated in 5 ml of equilibration buffer [50 mM Tris-HCl pH 8.8, 6M urea, 30% (v/v) glycerol, 2% (w/v) sodium dodecyl sulfate (SDS)] containing 1% (w/v) DTT for 15 min followed by 15 min incubation in 5 ml equilibration buffer with 2.5% (w/v) iodoacetamide. The second dimension was performed using Ettan™ Daltsix electrophoresis unit (GE Healthcare). Strips were placed on the gels and overlaid with 0.4% molten agarose. Protein separation was carried out using an electric current of 2 W per gel for 45 min, followed by 12 W per gel until the dye front reached the gel bottom. Gels were stained by colloidal Coomassie Brilliant Blue G-250.
Image Analysis
The gels were scanned with an Amersham imager, and the images were analyzed using the ImageMaster 2D Platinum v7 software (GE). ANOVA was carried out at a threshold of p ≤ 0.05, power ≥0.8, and ≥1.5-fold change in average spot volume between control and heat treatments for further MS analysis.
In-gel Digestion and Protein Identification
According to Wang et al. (2015), the differentially expressed protein spots between control and heat stress were subjected to the in-gel trypsin digestion. The digested spots were loaded on the MALDI plate, and the samples were analyzed using the AB SCIEX TOF/TOF 5800 (SCIEX) mass spectrometer using an accelerating voltage of 25 kV and 200 spectra/s speed for the peptide mass fingerprinting. Laser power was modulated to have the best signal-to-noise ratio. The Protein Pilot software (SCIEX) was used to extract and process the peptide mass peaks from the spectrum. Protein identification was performed by searching the SWISSPROT database using an in-house Mascot server (http://www.matrixscience.com) integrated with MaxQuant software v1.5.3.8. Carbamidomethyl cysteine was chosen for global modification; oxidation of methionine was chosen for variable modification; one missed cleavage was allowed; and minimum peptide length was set to seven peptides.
Statistical Analysis
Statistical significance among all the biochemical assays was performed by using one-way ANOVA followed by Tukey's test using SPSS25.0. However, all graphical and statistical significance representations were performed by MS-Excel 2019, showed as mean ± SEM.
Results
Effect of Heat Stress on Antioxidants and Related Metabolites
The antioxidant measurements in the flag leaves indicated that proline and H2O2 increased significantly under heat stress when compared with control plants at 7 DAA. Under day stress, proline and H2O2 content were increased by 238 and 29%, respectively (Figures 1A,B). The increase in concentration was higher under day–night stress, i.e., 780% in case of proline and 61% for H2O2 (Figures 1A,B).
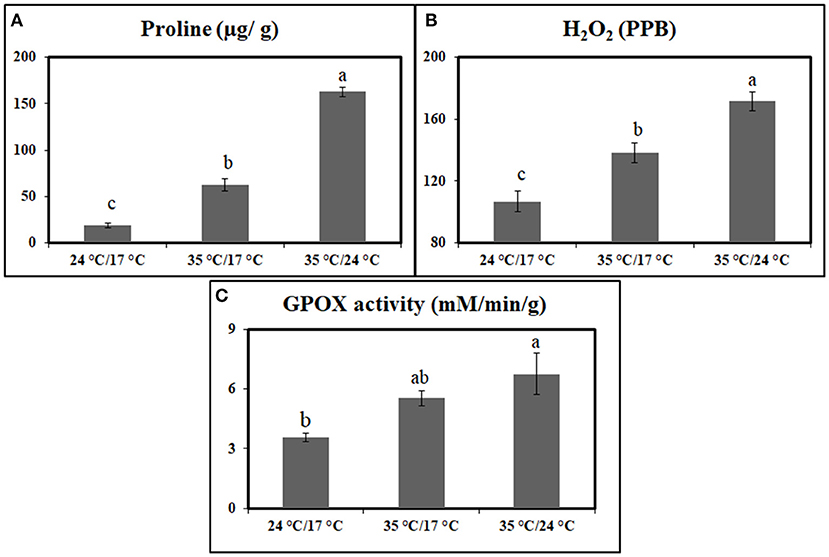
Figure 1. Graph representing the effect of heat stress during the grain-filling period on antioxidants and antioxidant enzymes between control, day heat stress, and day–night combined heat stress. (A) Proline (μg/g); (B) H2O2 (ppb). (C) Glutathione peroxidase (mM/min/g). Values were given in Mean ± SE. Variables with the same letter are not significantly different from each other at P ≤ 0.05.
GPOX activity also showed a significant increase under heat stress when compared with control. Under day stress and day–night stress, GPOX activity showed 1.5- and 1.8-fold increase, respectively, as compared with control (Figure 1C).
Effect of Heat Stress on Grain-Filling Duration and Yield-Related Parameters
Heat stress significantly affected the grain-filling period. Under the controlled conditions, plants took 35 days to complete the grain-filling period. It was reduced to 28 days under day stress and 25 days under day–night stress. The mean reductions in kernel weight per spike were 32 and 41% under day stress and day–night stress, respectively. Also, the reductions in the TKW were 11 and 18% under day stress and day–night stress, respectively (Figure 2A). Heat stress affected the grain seed length and width as well. Under day stress, the reductions in seed length and width from the top spikelets were higher than middle spikelets compared with control (Figures 2B,C). Under day–night stress, the seed length and the width from both top and bottom spikelets observed higher reduction than middle spikelets as compared with control.
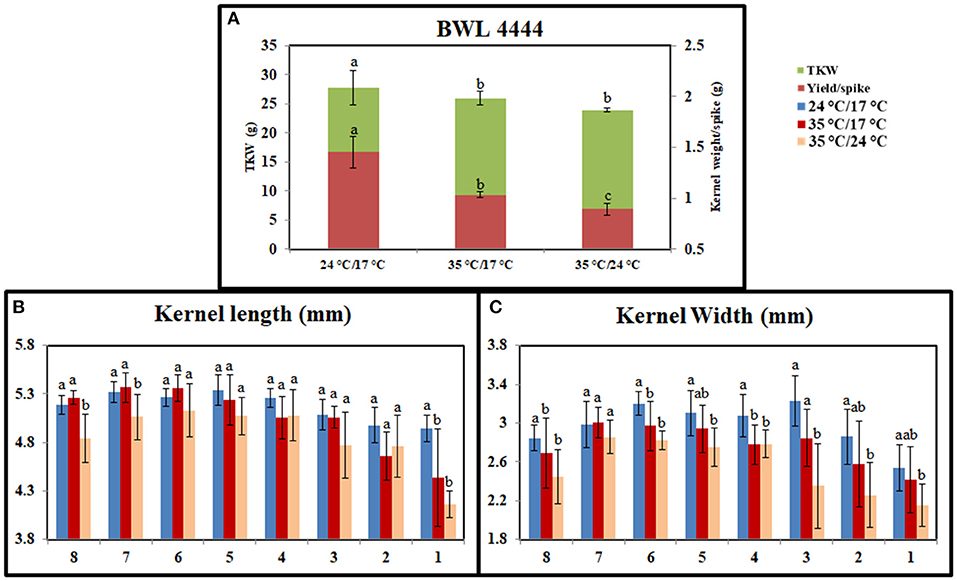
Figure 2. Graph representing the effect of heat stress during grain-filling period on yield parameters between control, day heat stress, and day–night combined heat stress. (A) Kernel weight/spike in grams (g) and thousand kernel weight (TKW) in grams (g). (B) Kernel length (mm). (C) Kernel width (mm). The x-axis in (B,C) indicates bottom spikelet (8) to top spikelet (1). Values were given in Mean ± SE. Variables with the same letter are not significantly different from each other at P ≤ 0.05.
Effect of Heat Stress on Gene Expression
The expression profiles of different pathway genes from four developmental stages and three temperature regimes were monitored by RT-qPCR (Figure 3; Supplementary Table 3).
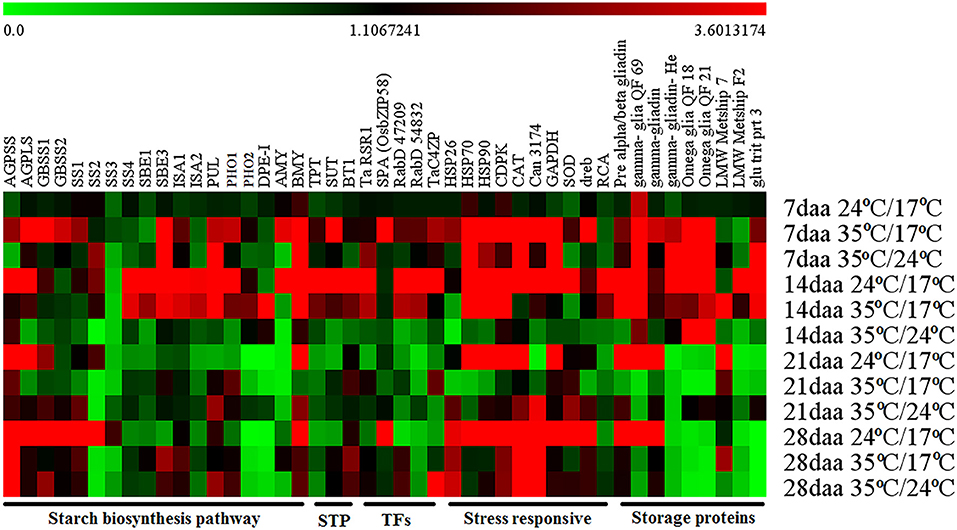
Figure 3. Heat map obtained from RT-qPCR representing the gene expression at different grain developmental stages from various temperature regimes.
Starch Biosynthesis Pathway Genes
The genes involved in the starch biosynthetic pathway were divided into four categories based on the expression pattern in the control group. The first category included genes with high expression at the early grain developmental stage, i.e., 14 DAA and relatively less expression in later stages, i.e., 21 DAA and 28 DAA. It included soluble starch synthase 4 (SS4), starch branching enzymes (SBE1 and SBE3), isoamylase (ISA1 and ISA2), pullulanase (PUL), starch phosphorylase (PHO1), and α-amylase (AMY). Under day stress, the expression of first category genes was higher at 14 DAA, with few genes started expressing at 7 DAA (SBE3, ISA1, PUL, and PHO1). The expression of these genes was reduced at later developmental stages with few showing minimal expression (SBE3, PUL, and PHO1) at 21 DAA and others (SBE1, SBE3, ISA, PUL, and PHO1) at 28 DAA. Under day–night stress, SBE3, PUL, and BMY showed relatively high expression at the early stage, i.e., 7 DAA, whereas the expression of other genes was low throughout the grain developmental stages (Figure 3).
The second category included genes with high expression toward the later stage of seed development (28 DAA) with minimal expression during early stages, i.e., 7 DAA, 14 DAA, and 21 DAA. They were granule-bound starch synthase (GBSSI and GBSSII) and soluble starch synthase (SS1, SS2, and SS3). Under day stress, the expression of GBSSI, GBSSII, and SS1 genes was observed at 7 DAA with a limited expression at 28 DAA. The expression of SS2 was observed only at 7 DAA and 14 DAA, and relatively lower expression of SS3 was observed at 7 DAA. Under day–night stress, very low expression of GBSSI, GBSSII, and SS1 was observed at 7 DAA and 14 DAA, but the relatively higher expression was observed at 21 DAA and 28 DAA. The expression of SS2 under day–night stress was only observed at 7 DAA, and the expression of SS3 was not observed throughout the development (Figure 3).
The third category included genes with high expression throughout the grain development, i.e., 14 to 28 DAA. It included ADP glucose pyrophosphorylase (AGP) large subunit (AGPL1), small subunit (AGPS1), and β-amylase (BMY). Under day stress, the expression of AGPS1 was high at 7 DAA and 28 DAA and low at 14 DAA and 21 DAA, while the expression of AGPL1 was high at 7 DAA and reduced after that with a limited expression at 28 DAA. The expression of BMY was observed at 7 DAA and 14 DAA, and subsequently, its expression was reduced. Under day–night stress, AGPS1 and AGPL1 had less expression from 7 to 21 DAA. At 28 DAA, they had a relatively higher expression. The expression of BMY was observed to be high at 7 DAA and low at 14–28 DAA (Figure 3).
The fourth category included genes with low to negligible expression throughout the development, i.e., starch phosphorylase 2 (PHO2) and disproportionating enzyme (DPE-1). Under day stress, these genes showed limited expression at 7 DAA, relatively higher expression at 14 DAA, and negligible expression at 21 and 28 DAA. Under day–night stress, these genes had limited expression at 14 and 21 DAA and negligible expression at 7 and 28 DAA (Figure 3).
Starch Transporter Genes
Under control conditions, the expression of starch transporter genes, i.e., triose phosphate translocator (TPT), sucrose transporter (SUT), and brittle-1 transporter (BT-1), was high at 14 DAA. Under day stress, these genes showed high expression during 7 and 14 DAA and minimal expression at 28 DAA, but at 14 DAA their expression was relatively lower than control. Under day–night stress, these genes had low to negligible expression throughout the development (Figure 3).
Stress-Responsive Genes
Under control conditions, most of the stress response genes, i.e., HSP (HSP26, HSP70, and HSP90), calcium-dependent protein kinase–signaling molecule (CDPK), catalase (CAT), hypothetical protein candidate (Can 3174), glyceraldehyde-3-phosphate dehydrogenase (GAPDH), superoxide dismutase (SOD), and dehydration-responsive element–binding proteins (dreb) had a high expression from 14 DAA to 28 DAA except for Can3174 that had a negligible expression at 21 DAA. Under day stress, the peak expression of these genes was observed at 7 DAA. HSP70, HSP90, and CDPK showed high expression at 14 DAA, and CDPK, CAT, and Can3174 showed high expression at 28 DAA. Under day–night stress, the expression of HSP70, HSP90, CDPK, CAT, Can3174, and GAPDH was high at 7 DAA. The expression of HSP26, CAT, Can-3174, and SOD was high at 21 DAA and 28 DAA. The expression of rubisco activase (RCA) was highest at 14 DAA under control and day stress conditions, but it had reduced expression during other stages. Under day–night stress, the expression of RCA was very less throughout the grain development (Figure 3).
Storage Proteins
Under control conditions, the γ-gliadin QF 69 had shown its expression throughout the development, but under day and day–night stress its expression was limited to 7 and 14 DAA. Pre-α/β gliadin and γ-gliadin had high expression during 14 and 21 DAA under control conditions. However, under day stress, the expression of these genes was observed at 7 and 14 DAA with a limited expression at 28 DAA. Under day–night stress, the expression of pre-α/β gliadin and γ-gliadin was observed at 7, 21, and 28 DAA. The expression levels of γ-gliadin He, ω-gliadin QF-18, ω-gliadin QF21, and glu trit prt 3 were high at 14 DAA under control conditions, but under day stress their expression was high during 7 and 14 DAA. At day–night stress, their expression was high at 7 DAA, and only γ-gliadin He, ω-gliadin QF-18, and ω-gliadin QF21 showed expression at 14 DAA. Under control conditions, LMW metship 7 had a high expression at 21 DAA, but under day stress it had high expression at 14–28 DAA. Under day–night stress, the expression of LMW metship 7 was low throughout the developmental stages with relatively high expression at 21 DAA. The expression of LMW metship F2 was high at 14 DAA under control and day stress conditions, but its expression was low in day–night stress throughout the development (Figure 3).
Transcription Factors
The expression of rice starch regulator (TaRSR1), Rab GTPases (RabD54832 and RabD47209), and TaC4ZFP (C4-type zinc finger transcription factor) was high at 14 DAA under control conditions. Under day stress, these genes had high expression during 7 and 14 DAA; however, at 14 DAA, their expression was relatively lower than that compared with control. Under day–night stress, these genes had minimal to low expression throughout the development except for TaC4ZFP, which showed high expression at 28 DAA. The expression of basic leucine zipper SPA (Os bZIP 58) was high at 28 DAA under control condition, but under day stress its expression was shifted to 7 DAA. The expression of Os bZIP 58 was very low throughout the grain development under day–night stress (Figure 3).
Protein Content and Profiles
The concentration of albumins–globulins and gliadins increased by 42% and 51% (under day stress) and 16 and 21% (under day–night stress), respectively. But the concentration of glutenins decreased by 7 and 29% under day stress and day–night stress, respectively. Overall, the total protein content increased under day stress; however, it remained unchanged under day–night stress as compared with control (Supplementary Table 4).
A total of 153 differentially expressed protein spots with a fold change >1.5 were detected under day stress compared with control. Out of 153, 32 spots (13 up- and 19 downregulated) in albumin–globulin fraction, 77 spots (36 up- and 41 downregulated) in gliadins, and 44 spots (29 up- and 15 downregulated) in glutenins were detected (Figures 4A–C; Table 1). Only 73 out of 153 spots (39 up- and 34 downregulated) were identified by mass spectrometry (MS). Under day–night stress, 95 spots were detected to have a fold change of >1.5. About 28 spots (3 up- and 25 downregulated) in albumin–globulin fraction, 38 spots (16 up- and 22 downregulated) in gliadins, and 29 spots (18 up- and 11 downregulated) in glutenins were observed (Figures 4D–F; Table 2). Out of 95 spots, only 32 (15 up- and 17 downregulated) were identified by MS. According to Bevan et al. (1998), these protein spots were classified into different groups: metabolism, energy, cell growth/division, transcription, protein synthesis, protein destination and storage, intracellular traffic, cell structure, signal transduction, defense, and unknown functions (Figure 5).
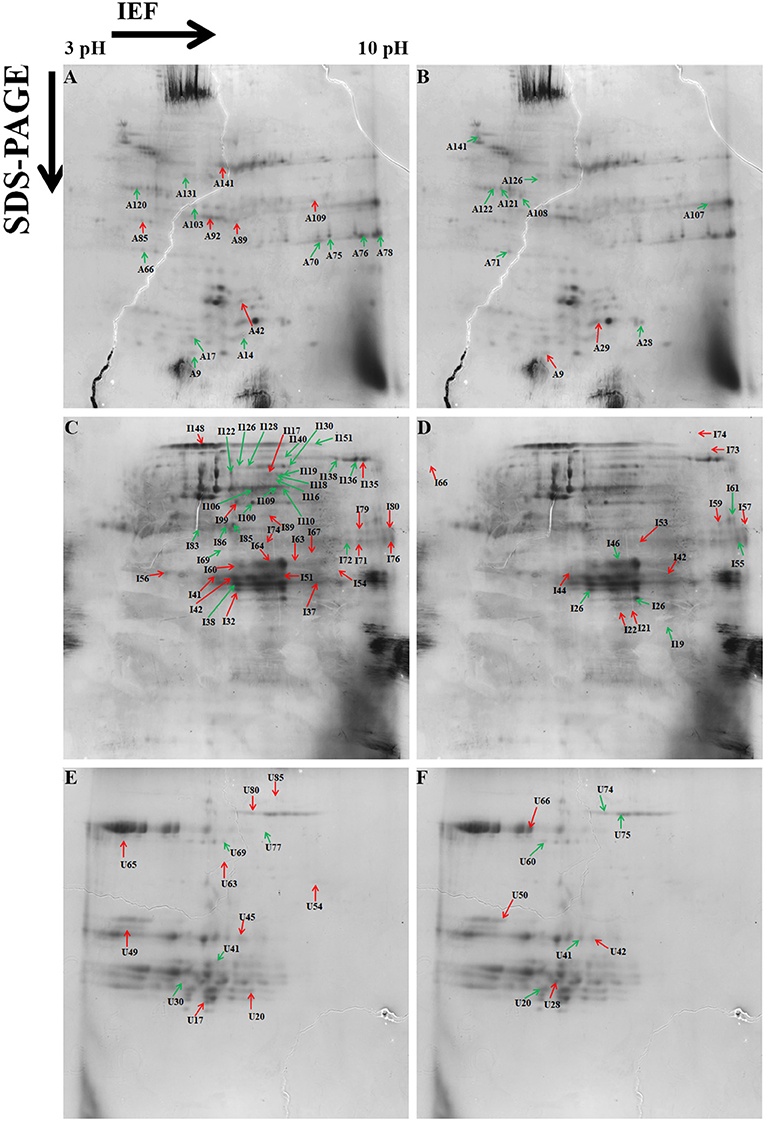
Figure 4. Representative two-dimensional electrophoresis (2-DE) gels of the wheat grain proteins analyzed by Protein Pilot software from different protein fractions. (A) Albumins–globulins day stress, (B) gliadins day stress, (C) glutenins day stress, (D) albumins–globulins day–night stress, (E) gliadins day–night stress, and (F) glutenins day–night stress. Red-colored arrows indicate upregulated protein spots between control and treatment. Green-colored arrows indicate downregulated protein spots between control and treatment.
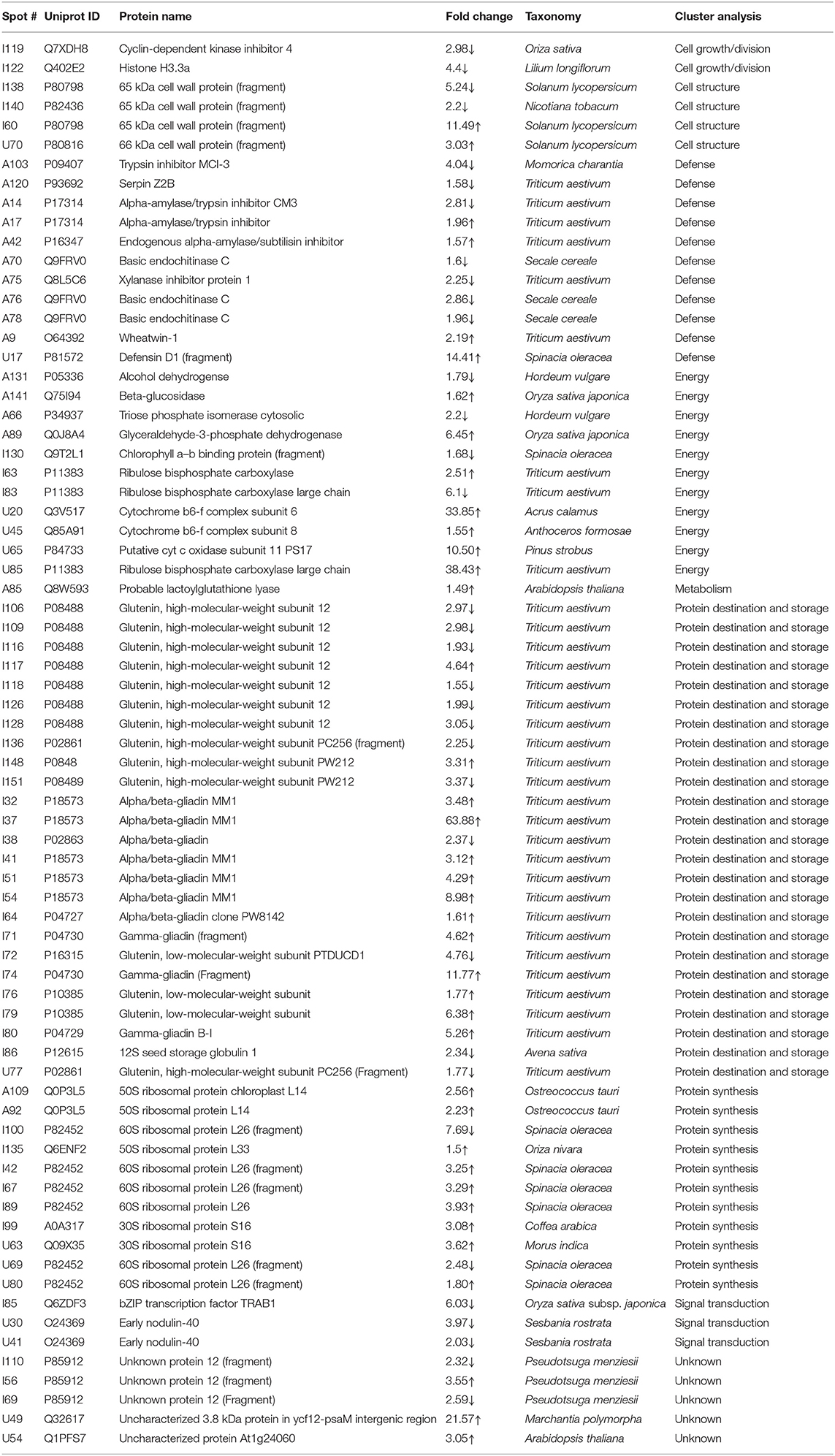
Table 1. List of differentially expressed proteins in mature grains under day heat stress (35 /17°C vs. 24/17°C) as analyzed by matrix-assisted laser desorption/ionization time-of-flight mass spectroscopy.
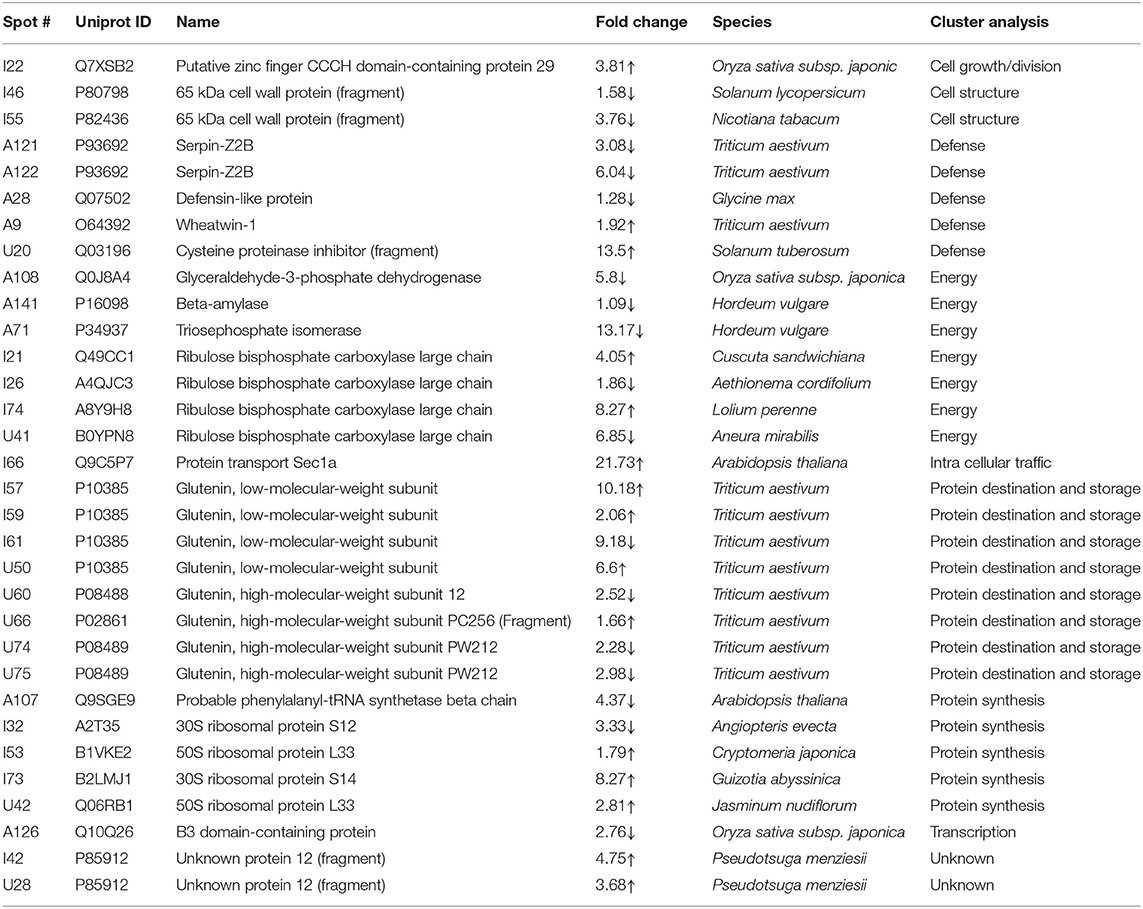
Table 2. List of differentially expressed proteins in mature grains under day-night combined heat stress (35/24°C vs. 24/17°C) as analyzed by matrix-assisted laser desorption/ionization time-of-flight mass spectroscopy.
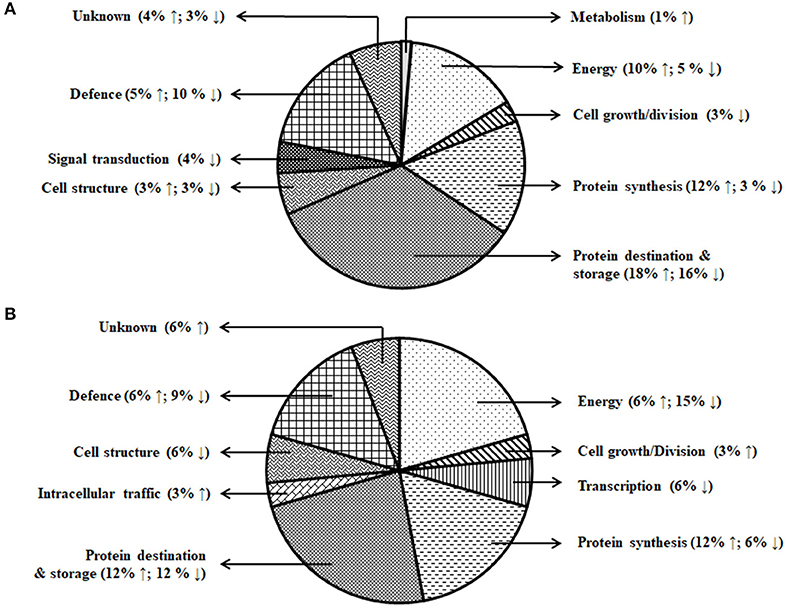
Figure 5. Functional classification of the differentially expressed proteins. (A) Day stress and (B) day–night stress. Proteins are classified according to Bevan et al. (1998).
The energy group was represented by 15 and 20% of protein spots in the day stress and day–night stress, respectively. In day stress, seven protein spots belonging to energy group showed upregulation and four protein spots showed downregulation. The upregulated protein spots belonged to ribulose bisphosphate carboxylase large chain (RCL) (spot no: I63, U85), GAPDH (spot no. A89), beta-glucosidase (spot no. A141), cytochrome b6-f complex (C b6-f C) subunit-6 (spot no. U20), C b6-f C subunit 8 (spot no. U45), and putative Cyt c oxidase subunit 11 PS17 (spot no. U65). The identified downregulated protein spots were triose phosphate isomerase (TPI) (spot no. A66), alcohol dehydrogenase (spot no. A131), RCL (spot no. I83), and chlorophyll a–b binding protein (spot no. I130). In day–night stress, two protein spots that belonged to RCL (spot no. I21 and I74) were upregulated, and five protein spots, i.e., triosephosphate isomerase (A71), GAPDH (A108), and RCL (I21, I74, and U41) were downregulated.
The cell growth/division group was represented by 3% protein spots in both day stress and day–night stress conditions. The two downregulated protein spots under day stress belonged to cyclin-dependent kinase inhibitor 4 (CDKI) (I119) and histone H3.3a (I122). In day–night stress, a protein spot putative zinc finger CCCH domain-containing protein 29 (I22) showed upregulation.
The protein synthesis group was represented by 15% and 18% protein spots in day stress and day–night stress, respectively. Under day stress, 11 protein spots showed differential expression. Two protein spots showed downregulation and belonged to 60S ribosomal protein L26 (I100 and U69). The remaining nine upregulated protein spots were 50S ribosomal protein L14 (A92 and A109), 60S ribosomal protein L26 (I42, I67, I89, and U80), 30S ribosomal protein S16 (I99 and U63), and 50S ribosomal protein L33 (I135). In day–night stress, two out of identified six spots were downregulated. These were probably phenylalanyl-tRNA synthetase beta chain (A107) and 30S ribosomal protein S12 (I32). The remaining four upregulated spots were 50S ribosomal protein L33 (I53 and U42), 30S ribosomal protein S7 (I66), and 30S ribosomal protein S14 (I73).
The protein destination and storage group were represented by 35 and 24% of the protein spots in day stress and day–night stress, respectively. In day stress, 14 protein spots out of 25 identified were upregulated and 12 were downregulated. The upregulated protein spots were glutenin—LMW subunit (I76 and I79), glutenin—HMW subunit 12 (I117), glutenin—HMW subunit PW212 (I148), alpha-/beta-gliadin MM1 (I32, I37, I41, I51, and I54), alpha-/beta-gliadin clone PW8142 (I64), gamma-gliadin (I71, I74, and I80), and gamma-gliadin B-I (I80). The downregulated protein spots were alpha-/beta-gliadin (I38), 12S seed storage globulin 1 (I86), glutenin—LMW subunit PTDUCD1 (I72), glutenin—HMW subunit 12 (I106, I109, I116, I118, I126, and I128), glutenin—HMW subunit PC256 (I136 and U77), and glutenin—HMW subunit PW212 (I151). In day–night stress, four protein spots out of eight showed downregulation, and they were glutenin—LMW subunit (I61), glutenin—HMW subunit 12 (U60), and glutenin—HMW subunit PW212 (U74 and U75). The remaining four upregulated protein spots were glutenin—LMW subunit (I57, I59, and U50) and glutenin—HMW subunit PC256 (fragment) (U66).
The cell structure group was represented by 5 and 6% of the protein spots in day stress and day–night stress, respectively. The differentially expressed proteins spots belonged to 65 kDa cell wall protein (I138, I140, I60, U70, I46, and I55).
The defense group was represented by 15% of the protein spots in day stress and day–night stress. In day stress, 4 protein spots out of 11 were upregulated, and they were wheatwin-1 (A9), defensin D1 (U17), endogenous alpha-amylase/subtilisin inhibitor (A42), and alpha-amylase/trypsin inhibitor (A17). The other seven downregulated protein spots were alpha-amylase/trypsin inhibitor CM3 (A14), trypsin inhibitor MCI-3 (A103), serpin Z2B (A120), basic endochitinase C (A70, A76, and A78), and xylanase inhibitor protein 1(A75). In day–night stress, two out of five upregulated protein spots were wheatwin-1(A9) and cysteine proteinase inhibitor (U20). The other downregulated protein spots were serpin-Z2B (A121 and A122) and defensin-like protein (A28).
Under day stress, one protein spot (1%), probably lactoyl glutathione lyase (spot no. A85), belonging to metabolism group showed upregulation and three protein spots (4%) belonging to signal transduction group, bZIP transcription factor TRAB1 (I85) and early nodulin-40 (U30 and U41), showed downregulation. Under day–night stress, one protein spot (3%), protein transport Sec1a (I66), belonging to the intracellular transport group showed upregulation. The transcription group was represented by 6% of the protein spots under day–night stress. The two downregulated protein spots were B3 domain-containing protein (A126) and pentatricopeptide repeat-containing protein (I32).
The differentially expressed proteins detected under both day and day–night heat stress included proteins belonging to starch biosynthesis, cell wall synthesis, defense, and storage proteins. Cell wall and starch synthesis proteins were downregulated under both types of stresses; these included 65 kDA cell wall protein, TPI, RCL, and GAPDH. While protein synthesis-related proteins, i.e., 50s ribosomal proteins were mainly upregulated under both types of stress. Contrasting protein expression was observed for storage proteins and defense-related proteins as wheatwin-1, LMW glutenins, and gliadins were upregulated, and serpin Z2B and HMW-GS were downregulated in both the conditions (Table 3).
Discussion
Wheat grain-filling period is the most susceptible phase for high-temperature stress (Farooq et al., 2011). Previous agronomic trait-based studies had observed that heat stress retards seed growth during grain-filling period by affecting the biochemical events such as the ability of the grains to accumulate water (Kino et al., 2020) causing losses in grain yield and number (Prasad and Djanaguiraman, 2014; Bheemanahalli et al., 2019). Most of the studies had only provided periodic heat stresses (Chauhan et al., 2011; Yang et al., 2011; Liu et al., 2015; Rangan et al., 2020; Tomás et al., 2020) during the reproductive stages for studying their effect on morphological and physiological parameters, but the molecular mechanism behind the effect of heat stress on grain-filling period is less understood (Liu et al., 2015; Kino et al., 2020; Rangan et al., 2020). Only few studies are available that have used heat stress throughout the grain-filling period and studied either protein or gene profiles (Zhang et al., 2017). In this study, the effect of postanthesis day heat stress and day–night combined heat stress was observed throughout the grain-filling period, and the molecular mechanism involved in the plant heat stress response through both gene and protein profiles have been attempted for better understanding.
Effect of Heat Stress on Metabolism and Yield
Reduction in yield is associated with lower photosynthesis and reduced starch accumulation (Wang et al., 2012). In the present study, the protein profiles showed the downregulation of RCL subunits and Cyt b6-f complex under day stress conditions, indicating reduced carbon fixation and photosynthesis. The downregulation of Cyt b6-f protein under heat stress condition had also been observed earlier in the case of wheat seedling (Luo et al., 2018). Similarly, the authors have observed that heat stress affected the expression of various starch biosynthesis pathway–related genes. The limited expression of AGPL1, AGPS1, GBSSI, GBSSII, SS (1, 2, and 3), and ISA under heat stress conditions caused a reduction in the synthesis of ADP-glucose and conversion to amylose and amylopectin that led to a reduced starch accumulation. Heat stress-associated reduction in the expression of genes involved in the starch biosynthetic pathway such as AGPL, AGPS (Impa et al., 2019), and SS (Hurkman et al., 2003) has also been reported earlier. Similarly, the expression of starch transporter genes (SUT, BT-1, and TPT) under heat stress was initiated earlier at 7 DAA under day stress conditions in contrast to the control condition where their expression was observed at 14 DAA, indicating that the starch synthesis occurred during early developmental stages. However, under the day–night stress, the expression of these genes was minimum throughout the grain development, indicating the reduced transportation of starch precursors that has led to decreased starch levels and grain yield. Reduction in expression levels of SUS and SUT genes was also observed in rice under heat stress leading to reduced kernel weight (Zhang C. X. et al., 2018). The present study also observed the limited expression of BMY, an enzyme that converts starch to glucose under heat stress conditions, indicating reduced supplementation of energy for metabolic pathways. The present study also observed lower expression of the transcription factors (TaRSR1 and OsbZIP58) that regulate the starch biosynthesis (Kang et al., 2013; Wang et al., 2013; Singh et al., 2015). It can be postulated that defense response activated by heat stress has reduced the expression of the starch synthesis related to the regulatory transcription factors. This has reduced the expression of biosynthetic, processing, and transport associated genes, ultimately leading to the lower starch deposition.
The protein profiles have also indicated upregulation of GAPDH, a rate-limiting enzyme in the glycolysis pathway under day stress, and downregulation under day–night stress indicating that its activity increases under day stress but reduces on higher stress. Previous studies had also observed an increase in GAPDH levels under heat stress in rice seedling (Han et al., 2009) and wheat seedling (Wang et al., 2015). TPI, another important glycolytic pathway enzyme that catalyzes the reversible interconversion of dihyroxyacetone phosphate to glyceraldehyde 3-phosphate, was downregulated in both day and day–night stresses. The decreased abundance of GAPDH and TPI in glycolysis leads to the oxidation of glucose-6-phosphate, which further enters the pentose phosphate pathway for the generation of NADPH. Studies also reported the decreased abundance of TPI under heat stress conditions (Yang et al., 2011). It has been reported that several glycolytic enzymes including TPI were increased at 10 DAA under heat stress condition (Hurkman et al., 2009). This is in contrast to the present results, which might be due to the duration of stress exposure or being cultivar-specific.
Increase in grain protein content had been observed under heat stress (Ashraf, 2014). From our data, we observed that the genes related to gliadins (α/β, γ-gliadins) showed high expression during early developmental stages, i.e., 7 and 14 DAA under heat stress conditions, but under control conditions, their expression was observed throughout the grain development. ω-gliadin showed high expression only at 14 DAA under control conditions, but its expression under stress conditions was observed at 7 and 14 DAA (day alone) and 7, 14, and 21 DAA (day–night stress) that indicated higher expression of ω-gliadins under stress conditions. LMW metship 7, a precursor gene for LMW glutenins, had a high expression at 21 DAA under control conditions, but its expression was observed at 14, 21, and 28 DAA under day stress conditions that indicated higher LMW-GS synthesis under stress conditions. Glu trip prt 3, a precursor for HMW glutenins (Zeltner et al., 2009), had high expression at 14 DAA under control conditions. An increased expression was observed at 7 DAA under heat stress with very low expression in later stages (Figure 3). Overall, HMW-GS proteins were downregulated, and LMW-GS, α/β, and γ-gliadin proteins were found to be upregulated under both stress conditions. Previous studies had also observed continuous synthesis of both gliadin and glutenins throughout the grain-filling period under heat stress (Bernardin et al., 1995). The study of Altenbach et al. (2002) indicated that under high-temperature regimens, transcripts from all seed storage protein gene families, appeared slightly earlier, but the timeframe of accumulation was shorter. Majoul et al. (2003) also observed decreased synthesis of glutenins and stable or increased synthesis of gliadins under heat stress. This might be due to the earlier synthesis of gliadins and LMW-GS compared with HMW-GS during grain development (Mazzeo et al., 2017). Heat stress reduces grain-filling period and advances the expression of biosynthetic genes, and thus gliadins and LMW-GS might be less affected or have increased as compared with HMW-GS. Our results also observed a reduction in glutenin-to-gliadin ratio of the matured grain under heat stress by 35–40% (Supplementary Table 4). Previous studies had reported a reduction in glutenin/gliadin ratio (Blumenthal et al., 1993) and HMW/LMW ratio (Don et al., 2005) under heat stress. Decreased glutenin/gliadin and HMW/LMW ratio reduces the baking quality (Balla et al., 2011; Garg et al., 2014).
Our protein profiles also found a higher abundance of ribosome-binding proteins that belong to the protein synthesis group under both heat stress conditions. Similar observations for ribosome-binding proteins had also been reported earlier in the matured grains after 2-h stress (Zhang Y. et al., 2018).
The effect of heat stress on various metabolic pathways has been graphically represented in Figure 6A. It indicates that a reduction in photosynthesis affects starch and protein biosynthesis. Combined stress induces a higher effect as compared with a single stress. It involves the interplay of several genes involved in multiple pathways including photosynthesis, transport of photosynthates to cellular organelles including amyloplasts, starch biosynthesis, glycolysis, protein precursors, and protein synthesis.
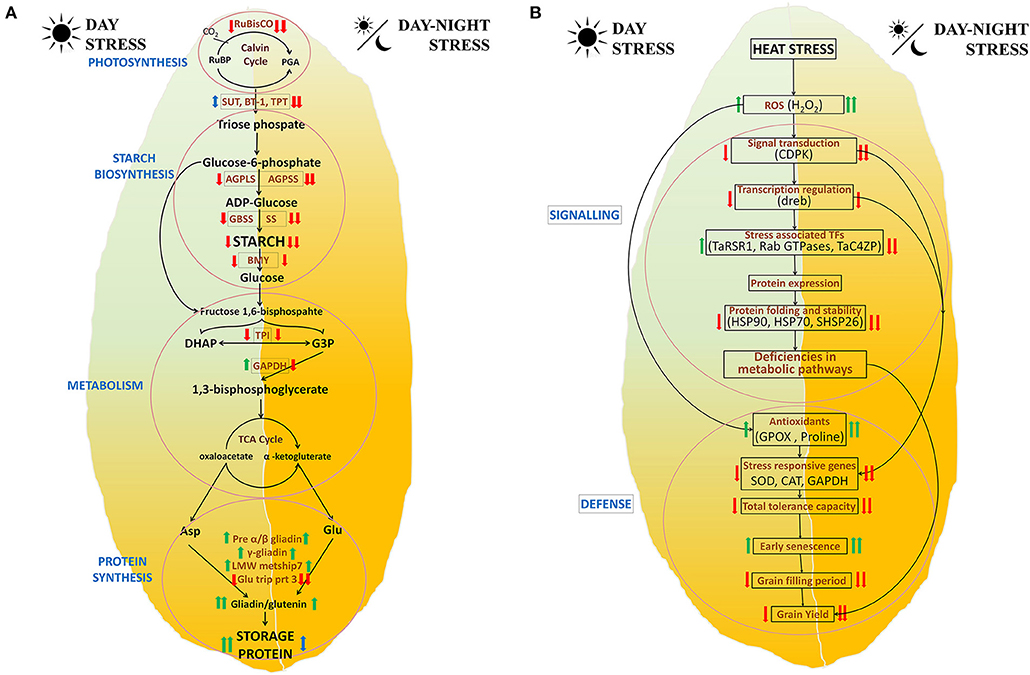
Figure 6. Proposed mechanism of the postanthesis day and day–night heat stress responses in BWL4444. (A) Biosynthesis pathway and (B) stress response.
Effect of Heat Stress on the Stress Response
Plants have adapted to various mechanisms to handle abiotic stresses at the morphological, physiological, biochemical, and genetic levels, for allowing them to survive under adverse conditions (Huber and Bauerle, 2016). In the present study, the increase in H2O2 concentration under stress conditions increased reactive oxygen species, which further triggers several signaling cascades (Lamaoui et al., 2018). Increased H2O2 content led to the activation of GPOX and an increase in proline content. It is a defense mechanism by plants that reduces the H2O2 concentration inside the cell. Our gene expression data showed high expression of CDPK until 14 DAA under day stress and 7 DAA under day–night stress in contrast to control where its expression was observed throughout the development. CDPK is a stress response gene that modulates the expression of several genes and proteins throughout plant growth and development (Asano et al., 2012). The reduced duration of CDPK expression indicated that under prolonged heat stress, the plant lost its ability to trigger other genes for the proper functioning of the cell. The increase in expression of CDPK after 2 h heat stress had also been reported earlier (Kumar et al., 2014). Similarly, the limited expression of transcription factor TaC4ZFP under heat stress indicated the loss of abiotic stress response as TaC4ZFP is the major heat stress response gene known to be a regulator for ABA-responsive signaling under heat stress (Li et al., 2013). The previous study observed an upregulation of 60-fold after 1.5 h heat stress, but its expression reduced after 5 h of treatment. The present study has also observed very low expression of other regulatory genes related to protein trafficking throughout the seed development (Rab GTPases), indicating their improper regulation under stress conditions. Tyler et al. (2015) also showed decreased expression of Rab GTPases under heat stress, thus stopping the regulation of vesicles carrying seed storage proteins from endoplasmic reticulum to Golgi complex. To sum up, heat stress has enhanced various stress-responsive genes and proteins but cannot maintain their expression under prolonged stress duration.
Our gene expression data have also shown limited expression of HSP90 and HSP70 under heat stress conditions (until 14 DAA), indicating reduced processing and folding of proteins during late developmental stages. Kumar et al. (2014) had observed an increase in expression of HSP70 and HSP90 after 2 h of heat stress post anthesis. Chloroplast-specific sHSP26, which helps in protecting the PS-II under heat stress (Heckathorn et al., 2002), had also shown reduced expression under continuous day and day–night stresses in later developmental stages indicating damage to the PS-II and reduction in the photosynthesis. RCA, a key chaperon that helps in the proper functioning of RUBISCO enzyme (Perdomo et al., 2017), was also downregulated under both day and day–night stress, which indicates decreased photosynthetic capacity (Supplementary Table 3). Kumar R. et al. (2016); Kumar R. R. et al. (2016) had observed an increase in RCA expression under 2 h of high-temperature stress; however, in the present study, the expression was observed throughout the grain development. The other heat response genes such as CAT, Can3174, SOD, and GAPDH, which are helpful in signaling and reduction of reactive oxygen species produced due to heat stress, had also shown increased expression during early developmental stages but were unable to maintain throughout the grain development. Overall, the continuous exposure to heat stress reduced the expression of proteins involved in folding and processing and increased the ROS, thereby decreasing the photosynthesis and metabolic processes.
The protein profiles also indicated downregulation of tumor suppressor protein, CDKI, under heat stress indicating enhanced cell division. Kamal et al. (2010) had observed enhanced expression of CDKI under abiotic stress. While reports on the reduction of the expression of cyclins by enhancing the inhibitory phosphorylation of CDKs are also available (Rowley et al., 1993), histone H3 3a also got downregulated under heat stress, which indicates the instability of DNA structure and defects in transcription regulation. The downregulation of histone H3 3a leads to irregularities in histone acetylation and methylation, which further affects the transcription regulation (Kong et al., 2020). Proteins with antifungal activity like wheatwin-1 (Caporale et al., 2004) and defensin D1 had shown upregulation under day stress. However, defensin D1 showed downregulation under day–night stress. Previous studies also observed an increase in transcript levels of wheatwin under heat stress (Altenbach et al., 2007). However, basic endochitinase c and xyalanase inhibitors with antifungal properties (Ohnuma et al., 2002) showed downregulation. Alpha-amylase inhibitor was highly expressed under heat stress. Studies had also reported an increased abundance of alpha-amylase inhibitors under heat stress (Laino et al., 2010). Serpins that have inhibitor function for chymotrypsin and trypsin may also act as allergens. Our studies have shown downregulation of serpins under heat stress. Laino et al. (2010) had observed upregulation of serpins under heat stress. The proteins related to the cell wall (65 kDa cell wall protein), which help in binding to tubulins, had shown downregulation under heat stress which indicates the loss of cell structure.
The effect of heat stress on various stress-related pathways has been graphically represented in Figure 6B. It indicates that long-term heat stress reduces the expression of genes related to various signaling pathways, transcription factors, protein folding machinery, and abiotic stress-related genes, thus leading to reduced tolerance capacity that further affects the other metabolic processes, yield-related parameters, and quality.
Overall, continuous day stress has reduced grain fill duration, seed dimensions, yield, and TKW and increased LMW proteins (gliadins and LMW-GS). Further reduction in these parameters was observed in day–night stress which might be due to suppression of most genes and reduced metabolism, thus leading to a reduction in starch deposition and early senescence (Figures 6A,B).
Conclusion
The present study observed the high expression of the genes related to starch biosynthesis pathway, starch transporters, transcription factors, stress response, and storage protein synthesis throughout the development under normal conditions. The expression of heat response genes increased under heat stress, which triggered regulatory, biosynthetic, and transport pathway genes to express earlier. The expression of these genes was high until 14 DAA under day stress and 7 DAA under day–night stress, which indicated that the plants tried to speed up the starch and protein synthesis and accumulation process. However, the continuous heat stress caused early senescence and maturation of the plant because of a reduction in gene expression related to antioxidant, defense, cell division, and biosynthetic machinery. The reduction in gene expression occurred because of an interplay of multiple pathways that led to a reduction in photosynthesis, glycolysis, other metabolite biosynthesis, and improper protein folding. Overall, the defense response to the day heat stress caused early gene expression, and day–night heat stress caused suppression of gene expression by activating multiple pathways, which ultimately led to the reduction in grain-filling duration, grain weight, yield, and processing quality.
Data Availability Statement
The original contributions presented in the study are included in the article/Supplementary Material, further inquiries can be directed to the corresponding author/s.
Author Contributions
VC and MG planned and designed the study. VC wrote the manuscript. VC, AK, and ShK performed the experiments. AmK, SS, PS, and PK helped in experiments. SS, NS, SaK, AnK, JK, and JR helped in editing. MG supervised the experiments and edited the manuscript. All authors contributed to the article and approved the submitted version.
Funding
This work was supported by Department of Biotechnology, India (Grant No. BT/IN/Indo-UK/CGAT/16/SK/2014-15).
Conflict of Interest
The authors declare that the research was conducted in the absence of any commercial or financial relationships that could be construed as a potential conflict of interest.
Acknowledgments
The authors are thankful to Mainpal Singh for his help in performing the experiments.
Supplementary Material
The Supplementary Material for this article can be found online at: https://www.frontiersin.org/articles/10.3389/fpls.2021.660446/full#supplementary-material
References
Alexieva, V., Sergiev, I., Mapelli, S., and Karanove, E. (2001). The effects of drought and ultraviolet radiations on growth and stress markers in pea and wheat. Plant Cell Environ. 24, 1337–1344. doi: 10.1046/j.1365-3040.2001.00778.x
Altenbach, S. B., Kothari, K. M., and Lieu, D. (2002). Environmental conditions during wheat grain development alter temporal regulation of major gluten protein genes. Cereal Chem. 79, 279–285. doi: 10.1094/CCHEM.2002.79.2.279
Altenbach, S. B., Kothari, K. M., Tanaka, C. K., and Hurkman, W. J. (2007). Genes encoding the PR-4 protein wheatwin are developmentally regulated in wheat grains and respond to high temperatures during grainfill. Plant Sci. 173, 135–143. doi: 10.1016/j.plantsci.2007.04.007
Asano, T., Hayashi, N., Kikuchi, S., and Ohsugi, R. (2012). CDPK-mediated abiotic stress signaling. Plant Signal Behav. 7, 817–821. doi: 10.4161/psb.20351
Ashraf, M. (2014), Stress-induced changes in wheat grain composition and quality. Crit. Rev. Food Sci. Nutr. 54, 1576–1583. doi: 10.1080/10408398.2011.644354.
Balla, K., Rakszegi, M., Li, Z., Bekes, F., Bencze, S., and Veisz, O. (2011). Quality of winter wheat in relation to heat and drought shock after anthesis. Czech J. Food Sci. 29, 117–128. doi: 10.17221/227/2010-CJFS
Bates, L. S., Waldren, R. P., and Teare, I. D. (1973). Rapid determination of free proline for water stress studies. Plant Soil 39, 205–207. doi: 10.1007/BF00018060
Bennett, D., Reynolds, M., Mullan, D., Izanloo, A., Kuchel, H., Langridge, P., et al. (2012). Detection of two major grain yield QTL in bread wheat (Triticum aestivum L.) under heat, drought and high yield potential environments. Theor. Appl. Genet. 125, 1473–1485. doi: 10.1007/s00122-012-1927-2
Bernardin, J. E., Witt, S. C., and Milenic, J. (1995). Storage protein synthesis in the wheat endosperm at elevated temperature. Chem. Aust. 61, 499–500.
Bevan, M., Bancroft, I., Bent, E., Love, K., Goodman, H., Dean, C., et al. (1998). Analysis of 1.9 Mb of contiguous sequence from chromosome 4 of Arabidopsis thaliana. Nature 391, 485–488. doi: 10.1038/35140
Bheemanahalli, R., Sunoj, V. J., Saripalli, G., Prasad, P. V., Balyan, H. S., Gupta, P. K., et al. (2019). Quantifying the impact of heat stress on pollen germination, seed set, and grain filling in spring wheat. Crop Sci. 59, 684–696. doi: 10.2135/cropsci2018.05.0292
Blumenthal, C. S., Barlow, E. W. R., and Wrigley, C. W. (1993). Growth environment and wheat quality: the effect of heat stress on dough properties and gluten proteins. J. Cereal Sci. 18, 3–21. doi: 10.1006/jcrs.1993.1030
Caporale, C., Di Berardino, I., Leonardi, L., Bertini, L., Cascone, A., Buonocore, V., et al. (2004). Wheat pathogenesis-related proteins of class 4 have ribonuclease activity. Febs Lett. 575, 71–76. doi: 10.1016/j.febslet.2004.07.091
Chauhan, H., Khurana, N., Tyagi, A. K., Khurana, J. P., and Khurana, P. (2011). Identification and characterization of high temperature stress responsive genes in bread wheat (Triticum aestivum L.) and their regulation at various stages of development. Plant Mol. Biol. 75, 35–51. doi: 10.1007/s11103-010-9702-8
De Costa, W. A. J. M. (2011). A review of the possible impacts of climate change on forests in the humid tropics. J. Natl. Sci. Found. Sri. 39, 281–302. doi: 10.4038/jnsfsr.v39i4.3879
Don, C., Lookhart, G., Naeem, H., MacRitchie, F., and Hamer, R. J. (2005). Heat stress and genotype affect the glutenin particles of the glutenin macropolymer-gel fraction. J. Cereal Sci. 42, 69–80. doi: 10.1016/j.jcs.2005.01.005
Farooq, M., Bramley, H., Palta, J. A., and Siddique, K. H. (2011). Heat stress in wheat during reproductive and grain-filling phases. Crit. Rev. Plant Sci. 30, 491–507. doi: 10.1080/07352689.2011.615687
Finnie, C., Sultan, A., and Grasser, K. D. (2011). From protein catalogues towards targeted proteomics approaches in cereal grains. Phytochemistry 72, 1145–1153. doi: 10.1016/j.phytochem.2010.11.014
García, G. A., Dreccer, M. F., Miralles, D. J., and Serrago, R. A. (2015). High night temperatures during grain number determination reduce wheat and barley grain yield: a field study. Glob. Chang. Biol. 21, 4153–4164. doi: 10.1111/gcb.13009
Garg, M., Kumar, R., Singh, R. P., and Tsujimoto, H. (2014). Development of an Aegilops longissima substitution line with improved bread-making quality. J. Cereal Sci. 60, 389–396. doi: 10.1016/j.jcs.2014.05.006
Han, F., Chen, H., Li, X. J., Yang, M. F., Liu, G. S., and Shen, S. H. (2009). A comparative proteomic analysis of rice seedlings under various high-temperature stresses. Biochem. Biophys. Acta Proteins Proteom. 1794, 1625–1634. doi: 10.1016/j.bbapap.2009.07.013
Hatfield, J. L., Boote, K. J., Kimball, B. A., Ziska, L. H., Izaurralde, R. C., Ort, D., et al. (2011). Climate impacts on agriculture: implications for crop production. Agron. J. 103, 351–370. doi: 10.2134/agronj2010.0303
Hays, D. B., Do, J. H., Mason, R. E., Morgan, G., and Finlayson, S. A. (2007). Heat stress induced ethylene production in developing wheat grains induces kernel abortion and increased maturation in a susceptible cultivar. Plant Sci. 172, 1113–1123. doi: 10.1016/j.plantsci.2007.03.004
Heckathorn, S. A., Ryan, S. L., Baylis, J. A., Wang, D., Hamilton, E. W., Cundiff, L., et al. (2002). In vitro evidence from an Agrostis stolonefera selection genotype that chloroplast small heat shock protein can protect photosystem II during heat stress. Funct. Plant Biol. 29, 933–944. doi: 10.1071/PP01191
Huber, A. E., and Bauerle, T. L. (2016), Long-distance plant signaling pathways in response to multiple stressors: the gap in knowledge. J. Exp. Bot. 67, 2063–2079. doi: 10.1093/jxb/erw099
Hurkman, W. J., McCue, K. F., Altenbach, S. B., Korn, A., Tanaka, C. K., Kothari, K. M., et al. (2003). Effect of temperature on expression of genes encoding enzymes for starch biosynthesis in developing wheat endosperm. Plant Sci. 164, 873–881. doi: 10.1016/S0168-9452(03)00076-1
Hurkman, W. J., Vensel, W. H., Tanaka, C. K., Whitehand, L., and Altenbach, S. B. (2009). Effect of high temperature on albumin and globulin accumulation in the endosperm proteome of the developing wheat grain. J Cereal Sci. 49, 12–23. doi: 10.1016/j.jcs.2008.06.014
Impa, S. M., Sunoj, V. J., Krassovskaya, I., Bheemanahalli, R., Obata, T., and Jagadish, S. K. (2019). Carbon balance and source-sink metabolic changes in winter wheat exposed to high night-time temperature. Plant Cell Environ. 42, 1233–1246. doi: 10.1111/pce.13488
Impa, S. M., Vennapusa, A. R., Bheemanahalli, R., Sabela, D., Boyle, D., Walia, H., et al. (2020). High night temperature induced changes in grain starch metabolism alters starch, protein, and lipid accumulation in winter wheat. Plant Cell Environ. 43, 431–447. doi: 10.1111/pce.13671
Kamal, A. H. M., Kim, K. H., Shin, K. H., Choi, J. S., Baik, B. K., Tsujimoto, H., et al. (2010). Abiotic stress responsive proteins of wheat grain determined using proteomics technique. Aust. J. Crop Sci. 4, 196–208.
Kang, G., Xu, W., Liu, G., Peng, X., and Guo, T. (2013). Comprehensive analysis on the transcript levels of starch synthesis genes and RSR1 transcript factor in endosperm of wheat. Genome 56, 115–122. doi: 10.1139/gen-2012-0146
Kino, R. I., Pellny, T. K., Mitchell, R. A., Gonzalez-Uriarte, A., and Tosi, P. (2020). High post-anthesis temperature effects on bread wheat (Triticum aestivum L.) grain transcriptome during early grain-filling. BMC Plant Biol. 20:170. doi: 10.1186/s12870-020-02375-7
Kong, L., Liu, Y., Wang, X., and Chang, C. (2020). Insight into the role of epigenetic processes in abiotic and biotic stress response in wheat and barley. Int. J. Mol. Sci. 21:1480. doi: 10.3390/ijms21041480
Kumar, A., Sharma, S., Chunduri, V., Kaur, A., Kaur, S., Malhotra, N., et al. (2020). Genome-wide identification and characterization of heat shock protein family reveals role in development and stress conditions in Triticum aestivum L. Sci. Rep. 10:7858. doi: 10.1038/s41598-020-64746-2
Kumar, R., Kumar, A., Sharma, N. K., Kaur, N., Chunduri, V., Chawla, M., et al. (2016). Soft and hard textured wheat differ in starch properties as indicated by trimodal distribution, morphology, thermal and crystalline properties. PloS ONE 11:e0147622. doi: 10.1371/journal.pone.0147622
Kumar, R. R., Goswami, S., Singh, K., Dubey, K., Singh, S., Sharma, R., et al. (2016). Identification of putative RuBisCoActivase (TaRca1)—the catalytic chaperone regulating carbon assimilatory pathway in wheat (Triticum aestivum) under the heat stress. Front. Plant Sci. 7:986. doi: 10.3389/fpls.2016.00986
Kumar, R. R., Sharma, S. K., Goswami, S., Singh, G. P., Singh, R., Singh, K., et al. (2013). Characterization of differentially expressed stress-associated proteins in starch granule development under heat stress in wheat (Triticum aestivum L.). IJBB 50, 126–38.
Kumar, R. R., Sharma, S. K., Goswami, S., Verma, P., Singh, K., Dixit, N., et al. (2015). Salicylic acid alleviates the heat stress-induced oxidative damage of starch biosynthesis pathway by modulating the expression of heat-stable genes and proteins in wheat (Triticum aestivum). Acta Physiol. Plant 37:143. doi: 10.1007/s11738-015-1899-3
Kumar, R. R., Singh, G. P., Goswami, S., Pathak, H., and Rai, R. D. (2014). Proteome analysis of wheat ('Triticum aestivum') for the identification of differentially expressed heat-responsive proteins. Aust. J. Crop Sci. 8, 973–986.
Laino, P., Shelton, D., Finnie, C., De Leonardis, A. M., Mastrangelo, A. M., Svensson, B., et al. (2010). Comparative proteome analysis of metabolic proteins from seeds of durum wheat (cv. Svevo) subjected to heat stress. Proteomics 10, 2359–2368. doi: 10.1002/pmic.200900803
Lamaoui, M., Jemo, M., Datla, R., and Bekkaoui, F. (2018). Heat and drought stresses in crops and approaches for their mitigation. Front. Chem. 6:26. doi: 10.3389/fchem.2018.00026
Li, J., Baroja-Fernández, E., Bahaji, A., Muñoz, F. J., Ovecka, M., Montero, M., et al. (2013). Enhancing sucrose synthase activity results in increased levels of starch and ADP-glucose in maize (Zea mays L.) seed endosperms. Plant Cell Physiol. 54, 282–294. doi: 10.1093/pcp/pcs180
Liu, B., Asseng, S., Liu, L., Tang, L., Cao, W., and Zhu, Y. (2016). Testing the responses of four wheat crop models to heat stress at anthesis and grain filling. Glob. Chang. Biol. 22, 1890–1903. doi: 10.1111/gcb.13212
Liu, Z., Xin, M., Qin, J., Peng, H., Ni, Z., Yao, Y., et al. (2015). Temporal transcriptome profiling reveals expression partitioning of homeologous genes contributing to heat and drought acclimation in wheat (Triticum aestivum L.). BMC Plant Biol. 15:152. doi: 10.1186/s12870-015-0511-8
Livak, K. J., and Schmittgen, T. D. (2001). Analysis of relative gene expression data using real-time quantitative PCR and the 2−ΔΔCT method. Methods 25, 402–408. doi: 10.1006/meth.2001.1262
Luo, Y., Liu, H. Y., Fan, Y. Z., Wang, W., and Zhao, Y. Y. (2018). Comparative chloroplast proteome analysis of exogenously supplied trehalose to wheat seedlings under heat stress. Photosynthetica 56, 1123–1133. doi: 10.1007/s11099-018-0808-6
Majoul, T., Bancel, E., Tribo,ï, E., Ben Hamida, J., and Branlard, G. (2003). Proteomic analysis of the effect of heat stress on hexaploid wheat grain: characterization of heat-responsive proteins from total endosperm. Proteomics 3, 175–183. doi: 10.1002/pmic.200390026
Mazzeo, M. F., Di Stasio, L., D'Ambrosio, C., Arena, S., Scaloni, A., Corneti, S., et al. (2017). Identification of early represented gluten proteins during durum wheat grain development. J. Agric. Food Chem. 65, 3242–3250. doi: 10.1021/acs.jafc.7b00571
Ohnuma, T., Yagi, M., Yamagami, T., Taira, T., Aso, Y., and Ishiguro, M. (2002). Molecular cloning, functional expression, and mutagenesis of cDNA encoding rye (Secale cereale) seed chitinase-c. Biosci. Biotechnol. Biochem. 66, 277–284. doi: 10.1271/bbb.66.277
Perdomo, J. A., Capó-Bau,çà, S., Carmo-Silva, E., and Galmés, J. (2017). Rubisco and rubiscoactivase play an important role in the biochemical limitations of photosynthesis in rice, wheat, and maize under high temperature and water deficit. Front. Plant Sci. 8:490. doi: 10.3389/fpls.2017.00490
Porter, J. R., and Gawith, M. (1999). Temperatures and the growth and development of wheat: a review. Eur. J. Agron. 10, 23–36. doi: 10.1016/S1161-0301(98)00047-1
Prasad, P. V., and Djanaguiraman, M. (2014). Response of floret fertility and individual grain weight of wheat to high temperature stress: sensitive stages and thresholds for temperature and duration. Funct. Plant Biol. 41, 1261–1269. doi: 10.1071/FP14061
Rangan, P., Furtado, A., and Henry, R. (2020). Transcriptome profiling of wheat genotypes under heat stress during grain-filling. J. Cereal Sci. 91:102895. doi: 10.1016/j.jcs.2019.102895
Rieu, I., Twell, D., and Firon, N. (2017). Pollen development at high temperature: from acclimation to collapse. Plant Physiol. 173, 1967–1976. doi: 10.1104/pp.16.01644
Ristic, Z., Bukovnik, U., and Prasad, P. V. (2007). Correlation between heat stability of thylakoid membranes and loss of chlorophyll in winter wheat under heat stress. Crop Sci. 47, 2067–2073. doi: 10.2135/cropsci2006.10.0674
Rowley, A., Johnston, G. C., Butler, B., Werner-Washburne, M., and Singer, R. A. (1993). Heat shock-mediated cell cycle blockage and G1 cyclin expression in the yeast Saccharomyces cerevisiae. Mol. Cell Biol. 13, 1034–1041. doi: 10.1128/MCB.13.2.1034
Simova-Stoilova, L., Demirevska, K., Petrova, T., Tsenov, N., and Feller, U. (2008). Antioxidative protection in wheat varieties under sever recoverable drought at seedling stage. Plant Soil Environ. 54, 529–536. doi: 10.17221/427-PSE
Singh, A., Kumar, P., Sharma, M., Tuli, R., Dhaliwal, H. S., Chaudhury, A., et al. (2015). Expression patterns of genes involved in starch biosynthesis during seed development in bread wheat (Triticum aestivum). Mol. Breed. 35:184. doi: 10.1007/s11032-015-0371-9
Stone, P. J., Gras, P. W., and Nicolas, M. E. (1997). The influence of recovery temperature on the effects of a brief heat shock on wheat. III. Grain protein composition and dough properties. J. Cereal Sci. 25, 129–141. doi: 10.1006/jcrs.1996.0080
Tomás, D., Viegas, W., and Silva, M. (2020). Effects of post-anthesis heat waves on the grain quality of seven european wheat varieties. Agronomy 10:268. doi: 10.3390/agronomy10020268
Tyler, A. M., Bhandari, D. G., Poole, M., Napier, J. A., Jones, H. D., Lu, C., et al. (2015). Gluten quality of bread wheat is associated with activity of RabD GTP ases. Plant Biotechnol. J. 13, 163–176. doi: 10.1111/pbi.12231
Wang, J. C., Xu, H., Zhu, Y., Liu, Q. Q., and Cai, X. L. (2013). OsbZIP58, a basic leucine zipper transcription factor, regulates starch biosynthesis in rice endosperm. J. Exp. Bot. 64, 3453–3466. doi: 10.1093/jxb/ert187
Wang, X., Cai, J., Liu, F., Jin, M., Yu, H., Jiang, D., et al. (2012). Pre-anthesis high temperature acclimation alleviates the negative effects of post-anthesis heat stress on stem stored carbohydrates remobilization and grain starch accumulation in wheat. J. Cereal Sci. 55, 331–336. doi: 10.1016/j.jcs.2012.01.004
Wang, X., Dinler, B. S., Vignjevic, M., Jacobsen, S., and Wollenweber, B. (2015). Physiological and proteome studies of responses to heat stress during grain filling in contrasting wheat cultivars. Plant Sci. 230, 33–50. doi: 10.1016/j.plantsci.2014.10.009
Wollenweber, B., Porter, J. R., and Schellberg, J. (2003). Lack of interaction between extreme high-temperature events at vegetative and reproductive growth stages in wheat. J. Agron. Crop Sci. 189, 142–150. doi: 10.1046/j.1439-037X.2003.00025.x
Yang, F., Jørgensen, A. D., Li, H., Søndergaard, I., Finnie, C., Svensson, B., et al. (2011). Implications of high-temperature events and water deficits on protein profiles in wheat (Triticum aestivum L. cv. Vinjett) grain. Proteomics 11, 1684–1695. doi: 10.1002/pmic.201000654
Yang, Y., Han, C., Liu, Q., Lin, B., and Wang, J. (2008). Effect of drought and low light on growth and enzymatic antioxidant system of Picea asperata seedlings. Acta Physiol. Plant. 30, 433–440. doi: 10.1007/s11738-008-0140-z
Zeltner, D., Glomb, M. A., and Maede, D. (2009). Real-time PCR systems for the detection of the gluten-containing cereals wheat, spelt, kamut, rye, barley and oat. Eur. Food Res. Technol. 228, 321–330. doi: 10.1007/s00217-008-0937-4
Zhang, C. X., Feng, B. H., Chen, T. T., Fu, W. M., Li, H. B., Li, G. Y., et al. (2018). Heat stress-reduced kernel weight in rice at anthesis is associated with impaired source-sink relationship and sugars allocation. Environ. Exp. Bot. 155, 718–733. doi: 10.1016/j.envexpbot.2018.08.021
Zhang, Y., Lou, H., Guo, D., Zhang, R., Su, M., Hou, Z., et al. (2018). Identifying changes in the wheat kernel proteome under heat stress using iTRAQ. Crop J. 6, 600–610. doi: 10.1016/j.cj.2018.04.003
Keywords: heat stress, wheat grain, gene expression, 2D-PAGE, MALDI–TOF
Citation: Chunduri V, Kaur A, Kaur S, Kumar A, Sharma S, Sharma N, Singh P, Kapoor P, Kaur S, Kumari A, Roy J, Kaur J and Garg M (2021) Gene Expression and Proteomics Studies Suggest an Involvement of Multiple Pathways Under Day and Day–Night Combined Heat Stresses During Grain Filling in Wheat. Front. Plant Sci. 12:660446. doi: 10.3389/fpls.2021.660446
Received: 29 January 2021; Accepted: 29 April 2021;
Published: 31 May 2021.
Edited by:
Raul Antonio Sperotto, Universidade do Vale do Taquari–Univates, BrazilReviewed by:
Xiaohui Li, Capital Normal University, ChinaManuela Silva, University of Lisbon, Portugal
Copyright © 2021 Chunduri, Kaur, Kaur, Kumar, Sharma, Sharma, Singh, Kapoor, Kaur, Kumari, Roy, Kaur and Garg. This is an open-access article distributed under the terms of the Creative Commons Attribution License (CC BY). The use, distribution or reproduction in other forums is permitted, provided the original author(s) and the copyright owner(s) are credited and that the original publication in this journal is cited, in accordance with accepted academic practice. No use, distribution or reproduction is permitted which does not comply with these terms.
*Correspondence: Monika Garg, mkajgarg@gmail.com; monikagarg@nabi.res.in