- 1College of Agriculture, Yangtze University, Hubei Collaborative Innovation Center for Grain Industry, Engineering Research Center of Ecology and Agricultural Use of Wetland, Ministry of Education, Jingzhou, China
- 2Institute of Crop Science, Chinese Academy of Agricultural Sciences(CAAS)/National Key Facility for Crop Gene Resources and Genetic Improvement, Key Laboratory of Biology and Genetic Improvement of Triticeae Crops, Ministry of Agriculture, Beijing, China
- 3College of Agronomy, Anhui Science and Technology University, Fengyang, China
Abiotic stresses, such as drought and salinity, severely affects the growth, development and productivity of the plants. The Catharanthus roseus RLK1-like (CrRLK1L) protein kinase family is involved in several processes in the plant life cycle. However, there have been few studies addressing the functions of CrRLK1L proteins in soybean. In this study, 38 CrRLK1L genes were identified in the soybean genome (Glycine max Wm82.a2.v1). Phylogenetic analysis demonstrated that soybean CrRLK1L genes were grouped into clusters, cluster I, II, III. The chromosomal mapping demonstrated that 38 CrRLK1L genes were located in 14 of 20 soybean chromosomes. None were discovered on chromosomes 1, 4, 6, 7, 11, and 14. Gene structure analysis indicated that 73.6% soybean CrRLK1L genes were characterized by a lack of introns.15.7% soybean CrRLK1L genes only had one intron and 10.5% soybean CrRLK1L genes had more than one intron. Five genes were obtained from soybean drought- and salt-induced transcriptome databases and were found to be highly up-regulated. GmCrRLK1L20 was notably up-regulated under drought and salinity stresses, and was therefore studied further. Subcellular localization analysis revealed that the GmCrRLK1L20 protein was located in the cell membrane. The overexpression of the GmCrRLK1L20 gene in soybean hairy roots improved both drought tolerance and salt stresses and enhanced the expression of the stress-responsive genes GmMYB84, GmWRKY40, GmDREB-like, GmGST15, GmNAC29, and GmbZIP78. These results indicated that GmCrRLK1L20 could play a vital role in defending against drought and salinity stresses in soybean.
Introduction
Plants are sessile organisms that are subjected to both abiotic and biotic stressors during its life cycle. In order to adapt to adverse environmental factors, plants have evolved several complex signal transduction pathways and signaling mechanisms to defend against cellular damage (Ramachandra Reddy et al., 2004; Mittler, 2006). Some proteins can transmit stresses signals and regulate the expression of stresses-responsive genes, such as protein kinases, transcription factors, and protein phosphatases (Edwards et al., 2000; Shinozaki and Yamaguchi-Shinozaki, 2007; Hundertmark and Hincha, 2008). Of these proteins, protein kinases are a vital regulator that attaches to different ligands, resulting in downstream gene expression (Chinnusamy et al., 2004).
Receptor-like protein kinases (RLKs) were first identified in maize and are similar to animal receptor protein kinases in both their structure and function (Walker and Zhang, 1990). To date, approximately 600 and 1,100 RLKs have been discovered in Arabidopsis and rice, respectively (Shiu et al., 2004). RLKs consist of a protein kinase catalytic domain (PKC), a transmembrane domain (TM), and an extracellular ligand-binding domain (ECLB; Walker, 1994). The PKC is an intracellular domain and can promote or repress the expression of downstream genes by phosphorylation and dephosphorylation. The TM consists of 22–28 amino acids (aa) and transmits signals from extracellular to intracellular domains. The ECLBs identify and combine different signal molecules. However, most RLKs possess the N-terminal signal peptide (SP) in the ECLB, which is separate from epidermal growth factor-like repeats (EGFs). Based on differences in the ECLB, RLKs are mainly divided into six categories: LRR-RLKs, S-RLKs, WAK-RLKs, PR5-RLKs, CR4-RLKs, and Lectin-RLKs (Liu et al., 2017). Several studies have demonstrated that RLKs participate in almost all stages of a plant’s life cycle, including growth, development, and stresses response (Wang et al., 2008; Boisson-Dernier et al., 2011). For example, pea LecRLK (PsLecRLK) is a lectin receptor-like kinases (LecRLKs) located in the plasma membrane. Plants with PsLecRLK-overexpression displayed enhanced salt stresses tolerance (Vaid et al., 2015). FON1, a member of the LRR-RLK subfamily, can be induced by both drought and ABA treatment (Feng et al., 2014). CRLK1, a calcium-regulated RLK, responds to cold tolerance in plants (Yang et al., 2010).
Catharanthus roseus Receptor-Like Kinase 1 Like (CrRLK1L), a subfamily of RLKs, was first identified in Catharanthus roseus cell cultures (Schulze-Muth et al., 1996). CrRLK1L has one or two carbohydrate-binding malectin-like domains when compared to other RLKs in ECLBs (Boisson-Dernier et al., 2011; Lindner et al., 2012). Subsequently, CrRLK1L has been found in some plants. For example, 16, 17, and 40 members have been identified in rice, Arabidopsis, and cotton, respectively (Acharya et al., 2007; Nguyen et al., 2015; Niu et al., 2016). Several studies have demonstrated that CrRLK1L regulates protein kinase activity by intramolecular phosphorylation (Vaid et al., 2015). Some members of the CrRLK1L family play crucial roles in various kinds of cells (Lindner et al., 2012). For example, ANX1 (ANXUR1) and ANX2 (ANXUR2) are involved in pollen tube growth and development (Boisson-Dernier et al., 2009, 2013; Miyazaki et al., 2009). THE1 (THESEUS1) and HERK1 (HERCULES1) can regulate cell growth in hypocotyls and leaves (Hematy et al., 2007; Hematy and Hofte, 2008; Guo et al., 2009). Meanwhile, FERONIA (FER) is a member of the CrRLK1L subfamily and was first isolated from a pollen tube mutant (Huck et al., 2003). Previous studies demonstrated that FER responded to different hormone signals that contained auxin-mediated root hair growth in Arabidopsis (Duan et al., 2010), ethylene, and brassinosteroid (BR)-promoted hypocotyl elongation (Deslauriers and Larsen, 2010; Mao et al., 2015), and ABA-regulated abiotic stresses responses (Chen et al., 2016). Additionally, FER acts as a receptor for the Rapid Alkalization Factor (RALF) peptide ligand that causes a rapid increase in cytoplasmic calcium and inhibits cell elongation in plants (Haruta et al., 2014). Several studies demonstrated that the RALF-FER pathway leads to increases in NADPH oxidase-dependent ROS (reactive oxygen species), which regulates both cell expansion and stresses responses (Thynne et al., 2017). Based on prior research, we speculate that CrRLK1L family proteins are involved in signal transduction, and regulate cell wall integrity in different tissues.
Soybean (Glycine max L.) is a drought- and salt-tolerant dicotyledonous plant, although its growth and yield can both be severely affected by drought and salt stresses. There is little published information on the CrRLK1L gene family and how it relates to abiotic stresses mechanisms in soybeans. In this study, we identified 38 possible CrRLK1L genes in soybean and conducted analyses of their bioinformatics, including phylogenetic relationships, chromosomal location, intron–exon structure, tissue-specific expression patterns, and stresses-related cis-elements. Based on RNA-Seq and Quantitative Real-Time Polymerase Chain Reaction (qRT-PCR) methods, we further investigated GmCrRLK1L20 and whether it was significantly up-regulated when subjected to drought and salt stresses. Our results demonstrated that GmCrRLK1L20 overexpression enhanced tolerance to drought and salt stresses in soybeans. These findings provide an insight into the foundation of the GmCrRLK1L20 gene and how it functions in abiotic stresses responses.
Materials and Methods
Plant Materials, Growth Conditions, and Stress Treatments
The soybean Williams 82 was used for the experiments conducted in this study. Seedlings were grown in pots with a humus: vermiculite ratio of 1:1 in a growth chamber with about 60% relative humidity, 28°C day/20°C night temperatures, and 14 h light/10 h dark photoperiod 16-day-old seedling were used for drought and salinity treatments. For drought treatment, the soybean seedlings at the four-leaf stage were removed from the soil and placed on filter paper for drought treatment. For salinity treatment, the soybean seedlings roots at the four-leaf stage were immersed in 200 mM NaCl solution. The samples were collected at different times of post-stress exposure for further research.
Identification of CrRLK1L Gene Family in Soybean
The sequences of previously identified CrRLK1L genes in Arabidopsis and rice were acquired from the TAIR database1 and the RGAP database (http://rice.plantbiology.msu.edu), respectively. We utilized BLASTP searches (E-value ≤ 1E-5), using the Arabidopsis CrRLK1L proteins as queries, to find the soybean CrRLK1L gene in the phytozome database2. Each candidate GmCrRLK1L protein sequence was then submitted to the online SMART tool3 to corroborate the presence of the complete CrRLK1L domains. The physicochemical parameters of the soybean GmCrRLK1L gene were obtained using the online ExPASyProtParam program4.
Multiple Sequence Alignments and Phylogenetic Tree Construction
The full-length aa sequences of CrRLK1L genes obtained for rice, Arabidopsis, and the newly identified soybean GmCrRLK1L gene were aligned using the ClustalX software with default parameters. MEGA7.0 software was used to construct an unrooted phylogenetic tree using the neighbor-joining method according to the following parameters: pairwise deletion, Poisson model, and 1,000 bootstrap replications.
Chromosomal Localization Analysis and Structural Characterization
The locations of the CrRLK1L genes on soybean chromosomes were obtained from the chromosomal loci in the Phytozome database. The exon-intron organization of soybean CrRLK1L genes were analyzed via coding sequences with corresponding full-length sequences using the online program Gene Structure Display Server (GSDS)5. The conserved motifs of the identified soybean CrRLK1L protein sequences were determined using the MEME online program6.
Expression Pattern Analysis of GmCrRLK1L Genes in Different Tissues
Transcriptome data were retrieved from the Phytozome database to analyze the expression patterns of GmCrRLK1L genes in different tissues, including seeds, roots, root hairs, stem, leaves, flowers, and nodules. The heatmap was constructed using the HemI software.
Analysis of Cis-Acting Elements in GmCrRLK1L Gene Promoters
The 2.0 kb region of 5′ UTR upstream of the soybean CrRLK1L genes obtained from the Phytozome database were submitted to PlantCARE7 to identify ten cis-elements, including ABA-responsive elements (ABRE), anaerobic induction elements (ARE), low-temperature responsiveness elements (LTR), defense and stress responsiveness elements (TC-rich repeats), wound-responsive elements (WUN-motif), MYB binding sites involved in drought-inducibility (MBS), W-Box, MYB, MYC, and DRE.
RNA Extraction and qRT-PCR
The plants at the four-leaf stage were grown in a greenhouse (light period: 14 h light/10 h dark, temperatures: 25°C day/20°C night, relative humidity: 60%). They were then taken out of the pot and washed with water. For drought treatment, the soybean seedlings at the four-leaf stage were placed on filter paper, and samples were collected at 0, 1, 2, 4, 8, 12, and 24 h of post-stress exposure. For salt treatment, the soybean seedlings at the four-leaf stage were immersed in 200 mM of NaCl, and samples were also collected at 0, 1, 2, 4, 8, 12, and 24 h of post-stress exposure. The samples were immediately frozen at −80°C in liquid nitrogen. The total RNA was extracted using a Plant Total RNA Extraction Kit according to the manufacturer’s instructions (TIANGEN). The qRT-PCR was conducted according to the method provided by the Prime Script TM RT Kit (Takara, Shiga, Japan). The primers for qRT-PCR of the five soybean GmCrRLK1L genes from the de novo soybean transcriptome sequencing were designed by Primer Premier 5.0, while the soybean actin gene was used as a control. An ABI Prism 7500 real-time PCR system (Applied Biosystems, Foster City, CA, United States) was used to perform qRT-PCR (Choi et al., 2005). The data were analyzed by the 2–ΔΔCT method (Le et al., 2011).
Subcellular Localization of GmCrRLK1L20
The full-length cDNA sequence of GmCrRLK1L20 was cloned into the N-terminus hGFP protein, which was driven by the CaMV35S promoter. The 35S:GFP vector was used as a control. The recombinant plasmid of GmCrRLK1L20-GFP was transformed into Arabidopsis protoplasts using a PEG4000-mediated method (He et al., 2016). The fluorescence signal was observed using a confocal laser scanning microscope after incubating it in darkness at 22°C for 18–20 h (Zeiss LSM 700, Oberkochen, Germany) (Riechmann et al., 2000).
Drought and Salt Stresses Assays of Soybean Hairy Root Composite Plants
Transgenic hairy root composite soybean plants were constructed using the method described by Shi et al. (2018) (Shi et al., 2018). Twelve Williams 82 soybeans were placed in each pot. Three pots formed a group. Drought treatment was conducted for 2 weeks. Under salt stresses, transgenic soybeans and EV-Control seedlings were grown in 200 mM of NaCl for four days. Both the drought and salt treatment experiments were conducted a minimum of three times. Both the treated and the untreated soybean hairy roots were washed with water for RNA isolation and physiological and biochemical experiments. The contents of Catalase (CAT), chlorophyll, Peroxidase (POD), Superoxide dismutase (SOD), Proline (Pro), relative electrical conductivity, and Malondialdehyde (MDA) were measured with the corresponding kit produced by Suzhou Comin Biotechnology Co., Ltd. (Suzhou, China) based on the manufacturer’s instructions; all data used were the average of three biological replicates.
Trypan Blue and NBT Staining
The Williams 82 seedlings with hairy roots approximately 2–5 cm in size were placed in a pot for 5 days, after which they were subjected to either 2 weeks of drought or 200 mM of NaCl for 4 days in a growth chamber. For trypan blue staining, the samples were immersed in a 0.5% Trypan blue (BioDee, China) solution for 12 h and then in 75% ethanol for decoloring until the leaves become white. For NBT staining, the samples were immersed in an NBT staining solution for 12 h and then in 75% ethanol (Wang et al., 2017) until the leaves became white. The pictures were taken with a Canon 50D (Canon, Japan) camera.
Fresh Weight of Roots
The fresh weight of GmCrRLK1L20-RNAi, GmCrRLK1L20-OE, and the EV-Control were measured under normal conditions as well as drought and salt conditions. All data used are the average of three biological replicates.
Statistical Analyses
All experiments were performed twice with at least three independent replicates. The data were assessed with the Student’s t-test using functions in Excel 2007. Vertical bars demonstrate ± SE of three biological replicates. An ANOVA test indicated that there were significant differences (∗p < 0.05, ∗∗p < 0.01).
Primers
The primers and sequences used in this study are listed in Supplementary Table 1.
Results
Identification of RLK Subfamily CrRLK1L Genes in the Soybean Genome
Whole-length proteins and conserved domains of 17 Arabidopsis CrRLK1L genes were used as queries to search the soybean genome database. We identified 38 soybean CrRLK1L genes in the soybean genome, which were named GmCrRLK1L01-GmCrRLK1L38 based on their chromosomal locations (Table 1). Gene features, including the length of the aa, the protein molecular weight (MW), isoelectric point (pI), and the subcellular location were analyzed (Table 1). Of the 38 soybean CrRLK1L proteins, GmCrRLK1L02 was the smallest protein with 647 aa. The largest one was 1186 aa GmCrRLK1L36. The molecular weights (MW) of the proteins varied from 72.72 kDa GmCrRLK1L02 to 133.95 kDa GmCrRLK1L36, and the pI ranged from 5.24 GmCrRLK1L03 to 8.81 GmCrRLK1L25. Subcellular localization of each soybean CrRLK1L protein were predicted by the TargetP software of the CBS database (Yu et al., 2004). The predicted subcellular localization results indicated that 38 soybean CrRLK1L proteins were all located in the plasma membrane (PM).
Phylogenetic Analysis of the CrRLK1L Family in Arabidopsis, Rice, and Soybean
To investigate the evolutionary relationship among the CrRLK1L genes in soybean, Arabidopsis, and rice, an unrooted phylogenetic tree was constructed by aligning the full-length aa sequences from 38 soybean members, 17 Arabidopsis members, and 16 rice members. As shown in Figure 1, the 71 CrRLK1L genes were divided into three main groups. The soybean CrRLK1L family had 24 members in cluster II and 14 members in cluster III. The Arabidopsis CrRLK1L had 4 members in cluster I, 3 in cluster II, and 10 in cluster III. The rice CrRLK1L had 6 members in cluster II and 10 members in cluster III.
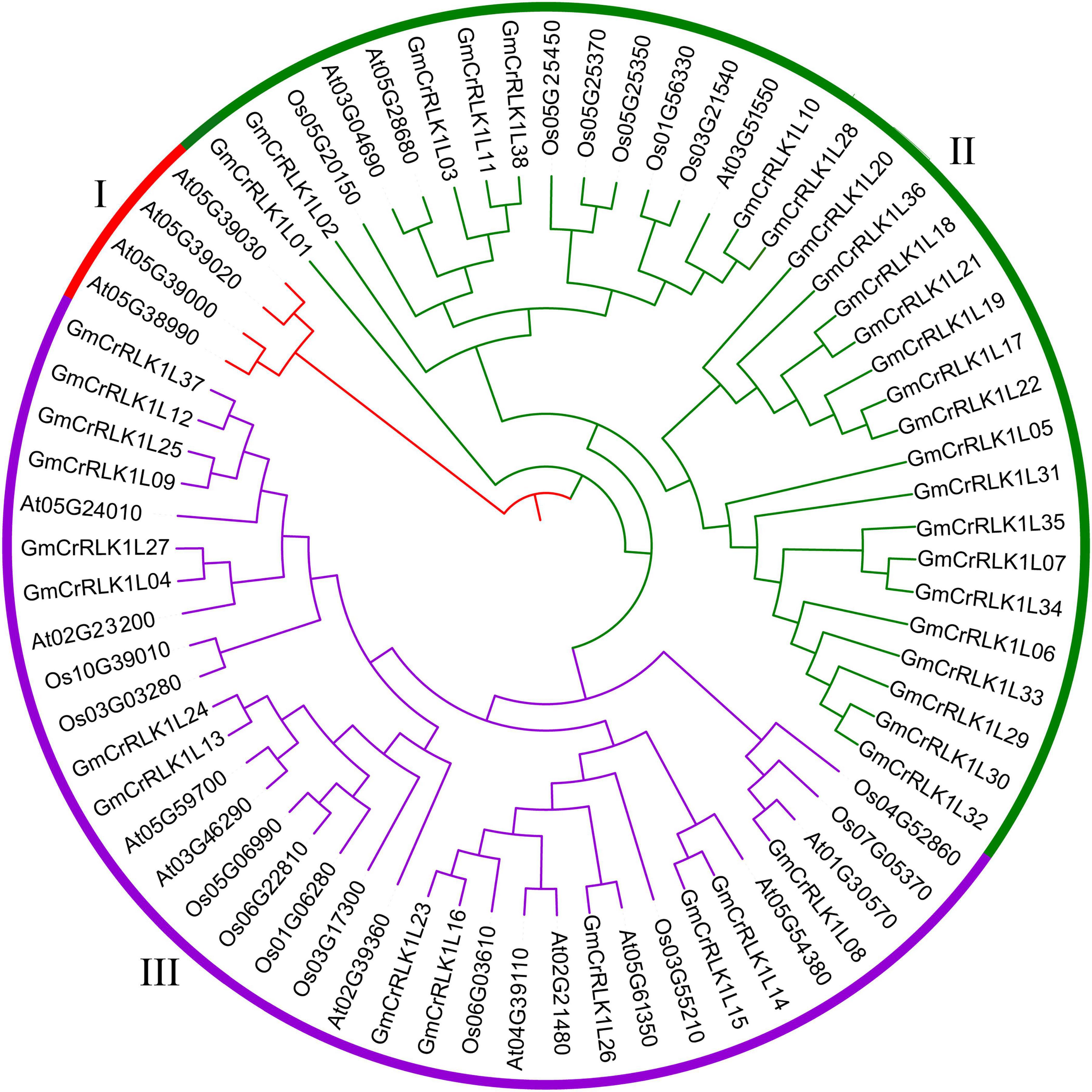
Figure 1. Phylogenetic tree analysis of CrRLK1L genes. The full-length amino acid sequence of the CrRLK1L protein from Arabidopsis, rice, and soybean were aligned by Crustal W and constructed by the NJ (Neighbor-joining) method with 1,000 bootstrap replicates. Distinct subfamilies are marked by different colors.
GmCrRLK1L Gene Distribution on Soybean Chromosome
Detailed information on the location of the 38 soybean CrRLK1L genes used in this study was obtained from Phytozome (Table 1). As shown in Figure 2, 38 CrRLK1L family members are distributed on 14 of 20 soybean chromosomes, with none found on chromosomes 1, 4, 6, 7, 11, and 14. The highest number of soybean CrRLK1L genes are found on chromosome 18, while the fewest number of GmCrRLK1L genes are found on chromosomes 3, 5, 8, 15, 16, and 19. Eight soybean CrRLK1L members were located on chromosome 18, seven soybean CrRLK1L members were located on chromosome 13, four soybean CrRLK1L members were located on chromosome 12, two soybean CrRLK1L members were located on chromosomes 2, 9, 10, 17, and 20, and one soybean CrRLK1L member was located on chromosomes 3, 5, 8, 15, 16, and 19.
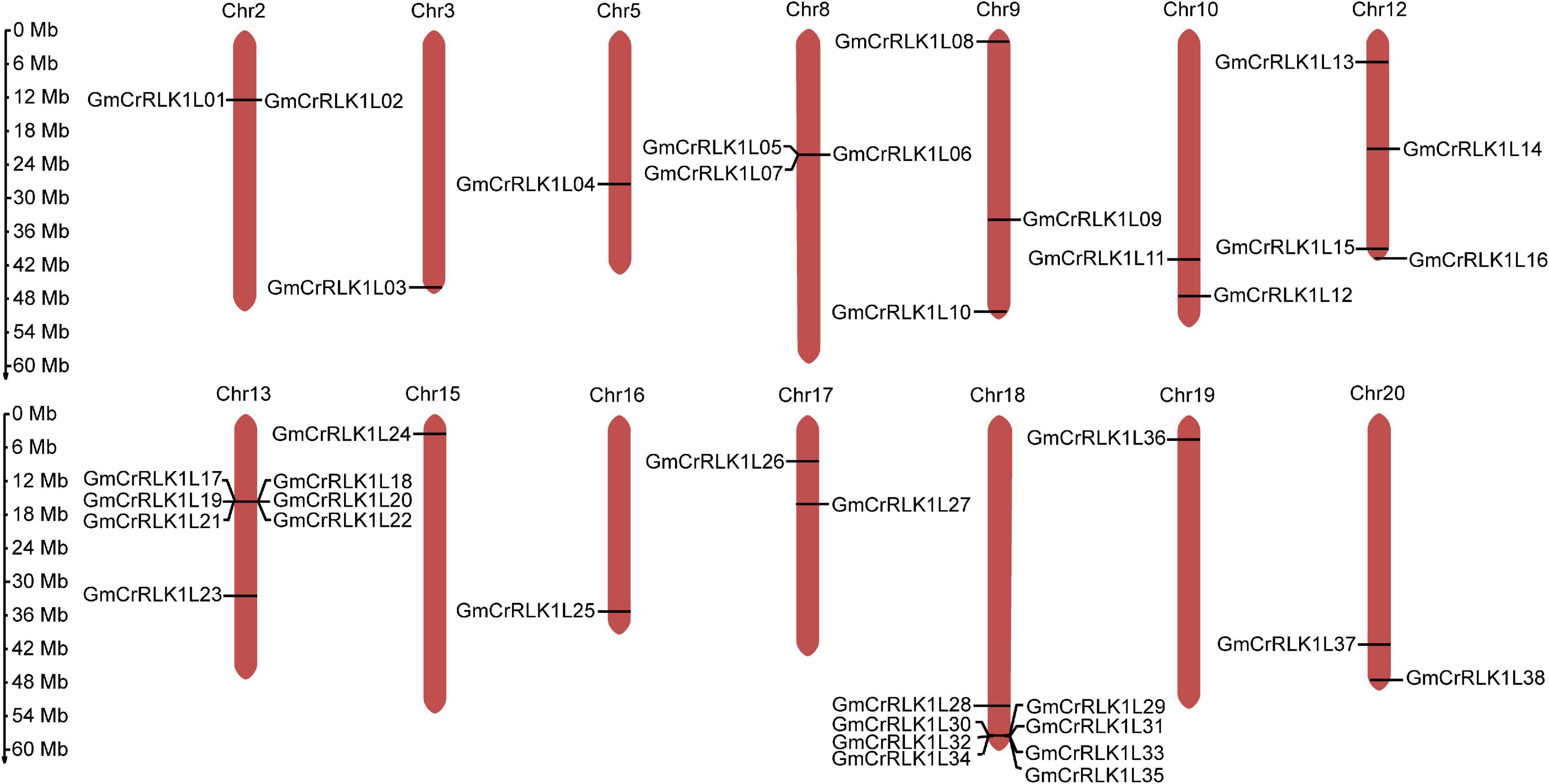
Figure 2. Chromosome distribution of the 38 GmCrRLK1L genes. Chromosome location map drawn with MG2C software. GmCrRLK1L genes distribution on 14 soybean chromosomes.
Gene Structure and Motif Composition of Soybean CrRLK1L Genes
We examined the exon–intron organization of all identified soybean CrRLK1L genes to better understand the evolution of the soybean CrRLK1L family. As shown in Figure 3B, this included very few introns, which was similar to the previous results conducted by Li et al. (2015). However, we found that several genes contained introns: among soybean CrRLK1L genes, more than half (28 soybean CrRLK1L genes, 73.6%) were free introns. Six soybean CrRLK1L genes (15.7%) had one intron and only four soybean CrRLK1L genes (10.5%) had more than one intron: GmCrRLK1L04 (seven introns), GmCrRLK1L36 (five introns), and GmCrRLK1L31 (three introns). The MEME website was used to identify the conserved motifs of soybean CrRLK1L genes, and 10 motifs were identified (Figure 3C). The lengths of these conserved motifs ranged from 29 to 50 aa. The 10 putative motifs are displayed in Table 2. Thirty-eight soybean CrRLK1L genes contained Motif 1 and Motif 5. Motif 2 and Motif 8 were found in 37 soybean CrRLK1L genes, with the exception of GmCrRLK1L04 and GmCrRLK1L02. The majority of soybean CrRLK1L proteins (94.7%) contained Motif 3, Motif 7, and Motif 9. Meanwhile, Motif 4 and Motif 5 were found in 35 soybean CrRLK1L genes and Motif 10 was only found in 22 soybean CrRLK1L genes. Soybean CrRLK1L genes in the same groups were generally found to possess a close motif element. However, most functions of these conserved motifs have yet to be explained.
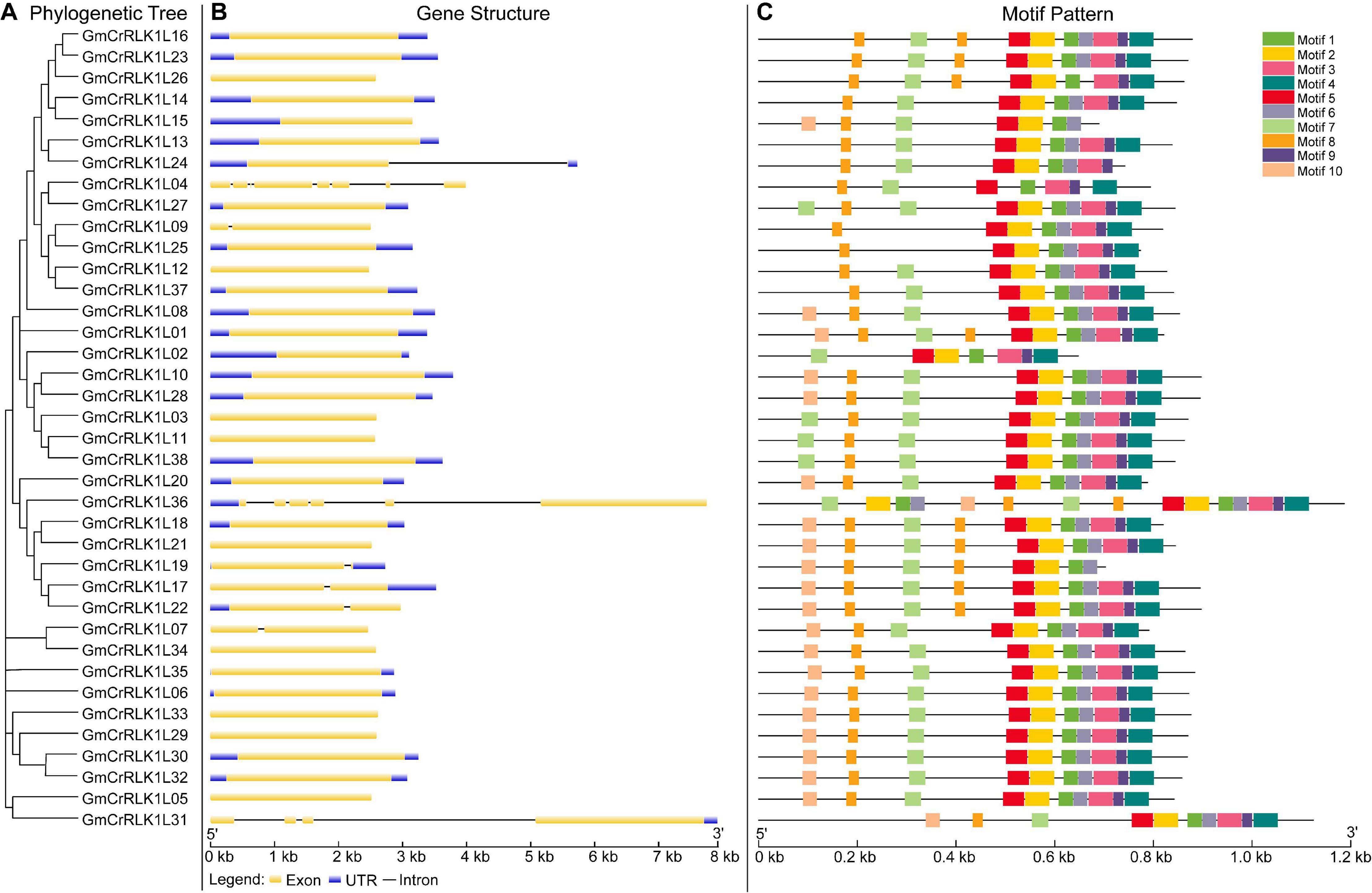
Figure 3. Phylogenetic relationship, gene structure, and architecture of conserved protein motif in CrRLK1L genes from soybean. (A) The phylogenetic tree analysis of CrRLK1L genes from soybean. (B) Exon/intron organization of GmCrRLK1L genes. Yellow boxes represent exons and black lines represent introns. (C) The motif composition of soybean CrRLK1L proteins. The motif, numbers 1–10, are displayed in different color boxes. For details of motifs refer to Table 2.
Expression Pattern Analysis of GmCrRLK1L Genes in Different Tissues
Different genes possess different expression abundances in different tissues or organs. This helps them adjust their physiological process. Our analyses of 38 soybean CrRLK1L gene expression patterns in seven tissues, including seeds, roots, root hairs, stems, leaves, flowers, and nodules, were conducted using known RNA-seq data from soybean genome databases. A heatmap of 38 soybean CrRLK1L genes was constructed using the HemI software. As shown in Figure 4, 32 GmCrRLK1L genes were expressed in at least one tissue, with the exception of GmCrRLK1L26, GmCrRLK1L04, GmCrRLK1L07, GmCrRLK1L20, GmCrRLK1L01, and GmCrRLK1L36. GmCrRLK1L38, GmCrRLK1L03, and GmCrRLK1L11 were only highly expressed in the flower. Approximately 11 genes (28.9%) were highly expressed in roots and root hairs, demonstrating that the soybean CrRLK1L genes could play vital roles in responding to adverse environmental factors.
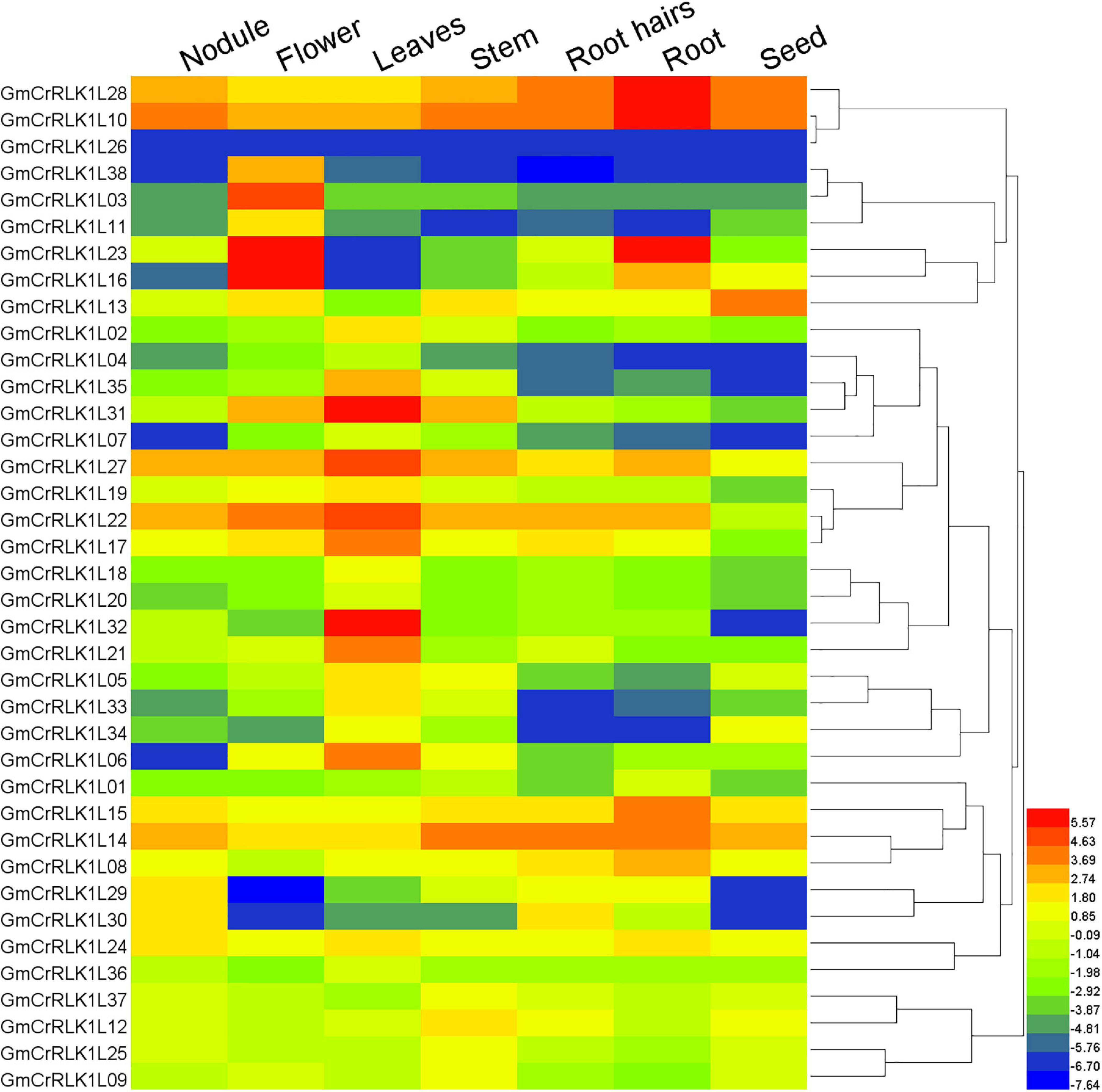
Figure 4. Expression profiles of soybean CrRLK1L genes from seven soybean tissues (seeds, roots, root hairs, stem, leaves, flowers, and nodules). Gene expression values are represented by different colors. The color scale is displayed at right of figure.
Stress-Related cis-Elements in Soybean CrRLK1L Genes Promoters
To further analyze possible regulatory mechanisms of soybean CrRLK1L genes among abiotic and biotic stress responses, the 2.0 kb upstream sequences from the start codon ATG of CrRLK1L genes were submitted to PlantCARE (see text footnote 7) to detect stresses-related cis-elements. Ten stresses response elements, including ABRE, MYB, MYC, ARE, LTR, W-box, WUN-motif, MBS, DRE, and TC-rich repeats were identified in these 38 soybean CrRLK1L gene promoters (Figure 5). Our results demonstrated that 38 soybean CrRLK1L genes have at least one cis-element related to stresses response, which demonstrated that soybean CrRLK1L gene expression could be associated with stresses response. For example, 36 and 29 soybean CrRLK1L genes (94.7%) have one or more MYB and MYC, respectively, and 24 soybean CrRLK1L genes possessed the ABA-responsive element. W-box, MBS, and LTR-responsive elements were found in 17, 12, and 11 soybean CrRLK1L genes, respectively. WUN-motif and TC-rich repeats were found on 13 soybean CrRLK1L genes. Seven DRE and one ARE were found on soybean CrRLK1L genes, respectively. The cis-elements analysis indicated that soybean CrRLK1L genes may be involved in different stresses responses.
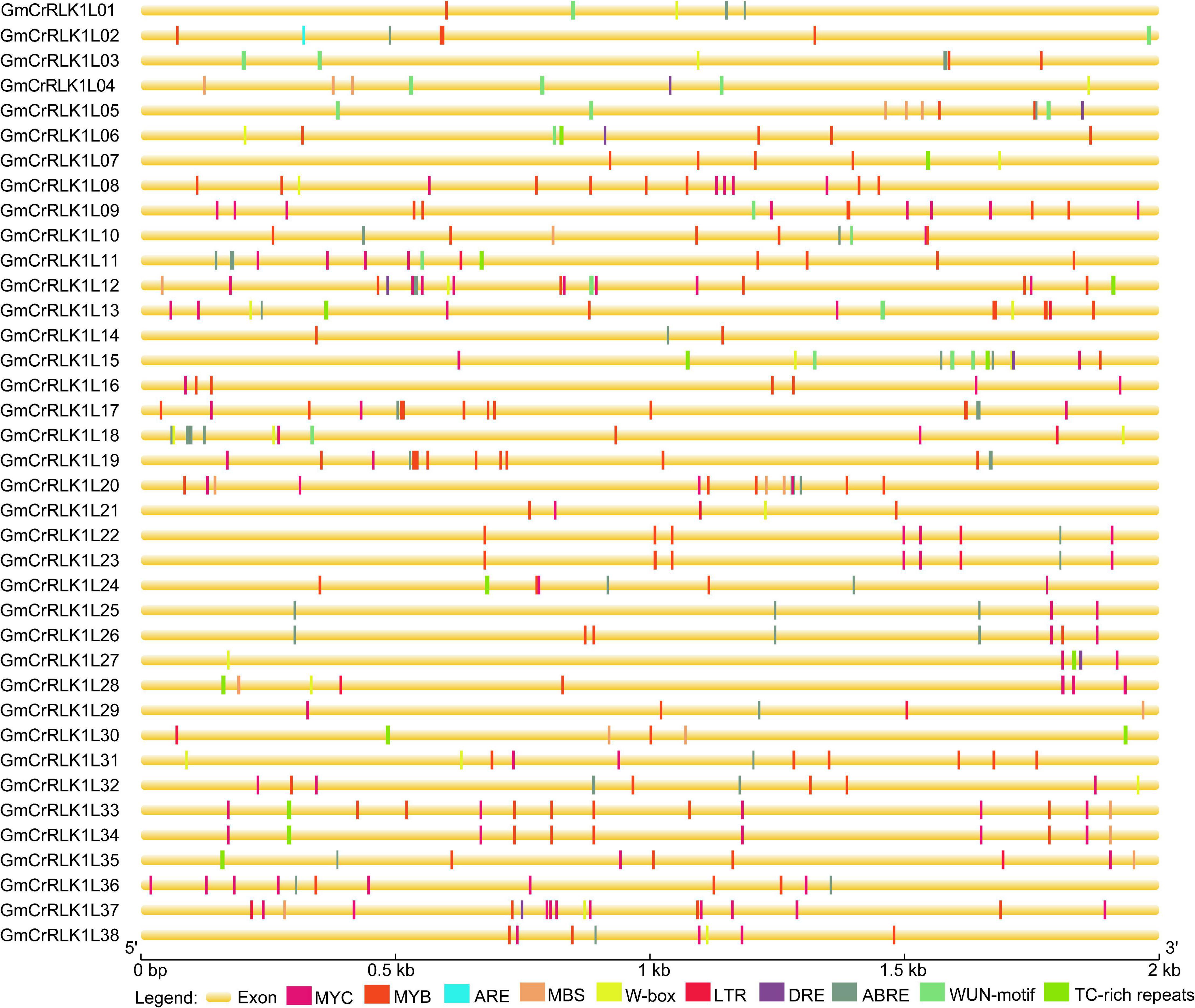
Figure 5. Predicted cis-elements in soybean CrRLK1L gene promoter’s region. Different cis-elements are marked by distinct color blocks and are located in relative positions on the promoter. The ABA-responsive element (ABRE), anaerobic induction element (ARE), low-temperature responsiveness element (LTR), defense and stresses responsiveness element (TC-rich repeats), wound-responsive element (WUN-motif), MYB binding site involved in drought-inducibility (MBS), W-Box, MYB, MYC, and DRE were analyzed. The upstream length to the translation starts site can be obtained using the scale at the bottom.
Expression Pattern Analysis of Five Soybean CrRLK1L Genes Under Drought and Salt Stresses Conditions
To thoroughly analyze the transcript levels of CrRLK1L genes in soybean under drought and salt stresses, we selected five CrRLK1L family members from the de novo transcriptome sequencing of soybean: GmCrRLK1L19, GmCrRLK1L20, GmCrRLK1L22, GmCrRLK1L24, and GmCrRLK1L31, which was based on the value of log2 (GH_treat/CK1_treat) and log2 (NaCl_treat/CK2_treat) > 1 (Figure 6). We also performed an expression pattern analysis of 5 CrRLK1L genes via qRT-PCR (Figure 7). These five GmCrRlLK1L genes were all upregulated under drought and salt stresses conditions (Figure 7), which was consistent with the hierarchical clustering found in expression profiles from drought and NaCl RNA-seq (Figure 6). Under drought treatment, 4 soybean CrRLK1L genes, GmCrRLK1L20, GmCrRLK1L22, GmCrRLK1L24, and GmCrRLK1L31, all peaked at 8 h (peaks of ∼ 20-, 5-, 2-, and 3-fold, observed at same time points, respectively). However, GmCrRLK1L19 peaked at 2 h (∼8-fold). Meanwhile, under NaCl condition, GmCrRLK1L22, GmCrRLK1L24, and GmCrRLK1L31 peaked at 1 h (∼6-fold), at 4 h (∼6-fold), and at 8 h (∼3-fold), respectively. GmCrRLK1L19 and GmCrRLK1L20 were all significantly upregulated (approximately 8- and 12-fold) at 12 h, respectively. By analyzing the levels of the expression pattern of GmCrRLK1L genes, we discovered that GmCrRLK1L20 had the highest expression level after 8 h of drought treatment (∼18-fold) and 12 h of high-salt conditions (∼13-fold). The qRT-PCR results showed that GmCrRLK1L20 had the highest expression level under drought and salt treatment. As such, GmCrRLK1L20 was used for further study. The expression data of the five genes were placed in the attachment Supplementary Table 3.
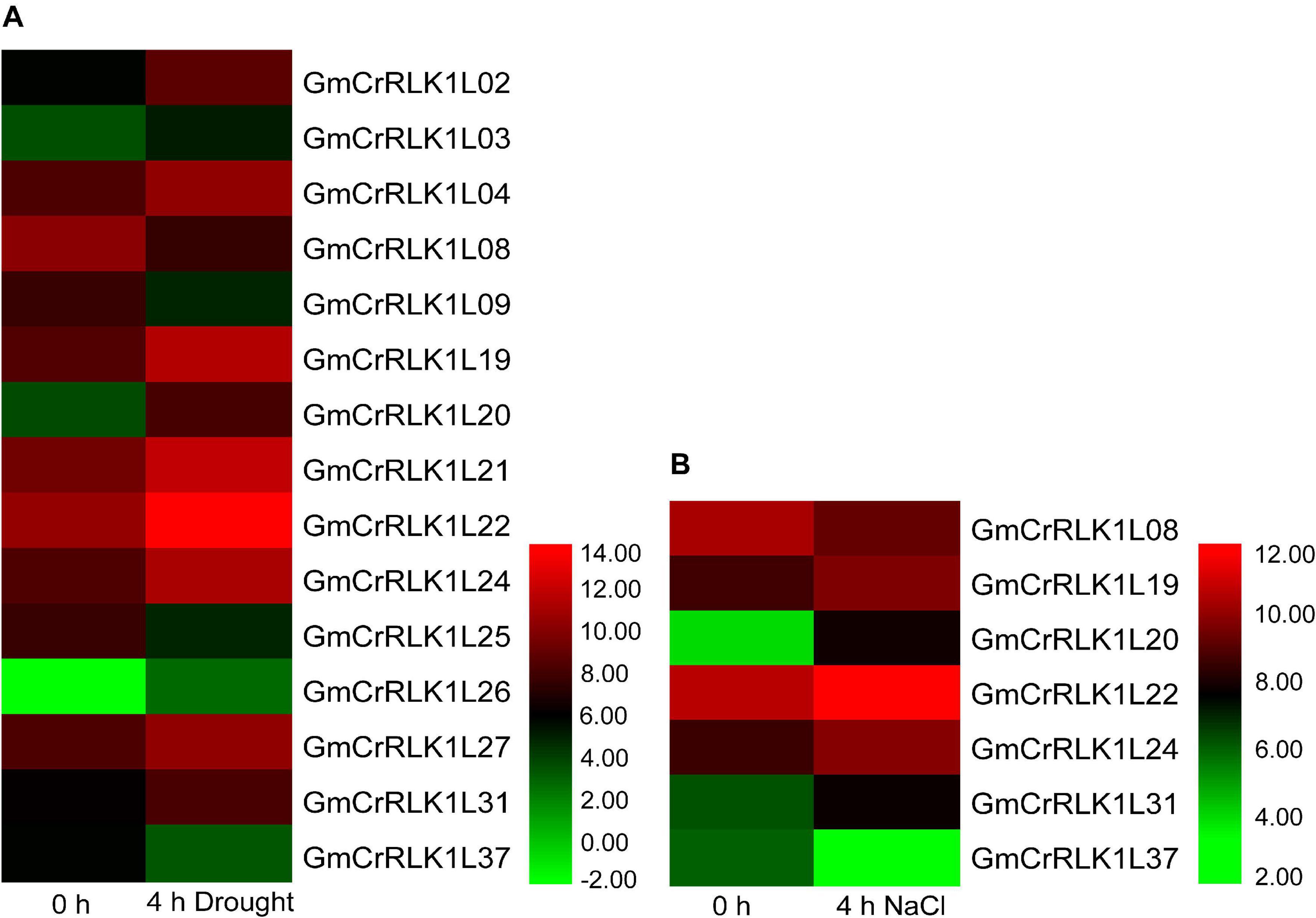
Figure 6. Hierarchical clustering of expression profiles of 15 and 7 drought- and NaCl-responsive genes, respectively. (A) The expression level of 15 drought responsive genes. (B) The expression level of 7 NaCl responsive genes. The color scale is shown at right of figure.
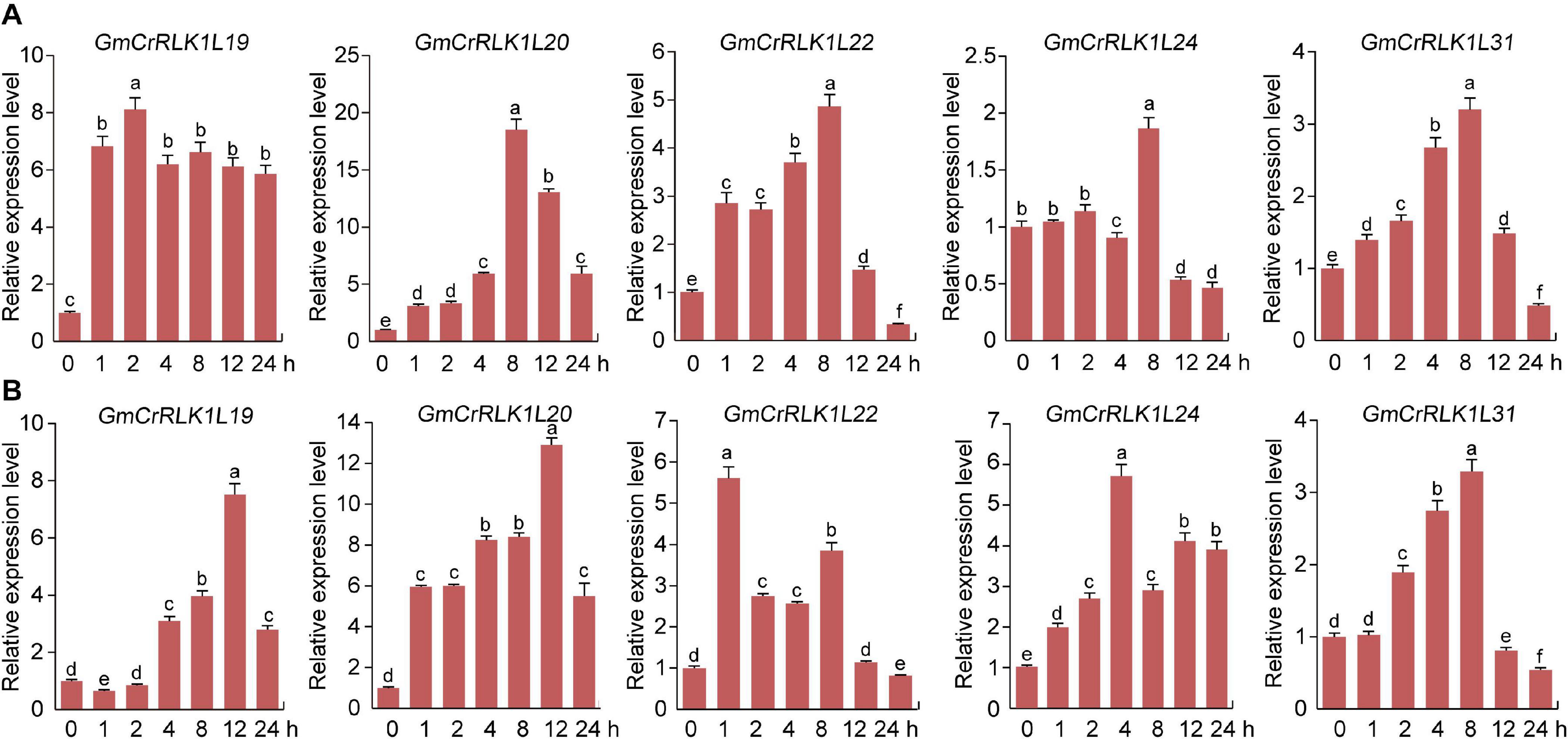
Figure 7. Analysis of expression patterns of five selected CrRLK1L genes under drought and salt treatment by qRT-PCR. (A) qRT-PCR of five GmCrRLK1L genes under drought treatment. (B) qRT-PCR of five GmCrRLK1L genes under salt treatment. The soybean Actin (U60506) was used as an internal control. The X-axes and Y-axes indicate time and relative expression levels, respectively. The data represent means ± SD of three biological replications.
Subcellular Localization of GmCrRLK1L20
To analyze the subcellular localization of GmCrRLK1L20, the open reading frame (ORF) sequence (without the stop codon of the GmCrRLK1L20 gene) was fused to the N-terminal of the humanized green fluorescent protein (hGFP) reporter protein and co-transformed into Arabidopsis protoplasts to identify the localization of the GFP fluorescence signal. The 35S:GFP vector was used as a control. The fluorescence signal of GmCrRLK1L20 was specifically detected in the cell membrane using a confocal laser scanning microscope, while the GFP fluorescence signal was identified throughout the whole cell (Figure 8).

Figure 8. Subcellular Localization analysis of the GmCrRLK1L20 Protein within the cell by GFP assays. 35S:GFP, control with empty vector; GmCrRLK1L20-GFP, GmCrRLK1L20-GFP fusion. Bars = 5 μm.
GmCrRLK1L20 Improved Drought and Salt Tolerance in Transgenic Soybean Hairy Roots
Of these five genes, GmCrRLK1L20 was notably upregulated under drought and salt treatment and was therefore used for further research (Figure 7). To further analyze the relationship between GmCrRLK1L20 and stresses response in soybean, we conducted transgenic soybean hairy root composite plants to identify the plant stresses resistance and discovered that the overexpression of GmCrRLK1L20 improved resistance to drought and salt (Figures 9A–C). The fresh weight of the roots was measured, which demonstrated that plants with GmCrRLK1L20-overexpression had higher root fresh weights (GmCrRLK1L20-OE) and that the GmCrRLK1L20-RNAi plants had lower fresh weights than wild-type plants (empty vector) under drought and salt stresses conditions (Figures 9H–J,R). These stressors can affect the intracellular reactive oxygen species (ROS) pathway which can produce H2O2 and O2–. Thus, we stained soybean leaves with 0.5% trypan blue and nitroblue tetrazolium (NBT) to analyze the H2O2 and O2– contents of GmCrRLK1L20-RNAi, wild-type and GmCrRLK1L20-OE plants under normal or stresses conditions. The results demonstrated that there was no difference in trypan blue and NBT staining on the plant leaves under normal growth conditions (Supplementary Figure 1). However, the brightness of the leaf color in GmCrRLK1L20-OE plants was significantly dimmer than in wild-type plants, and the leaf brightness of GmCrRLK1L20-RNAi plants was significantly higher than that of wild-type plants under drought and salt stresses conditions (Figures 9D–G). We also measured several physiological and biochemical indexes related to stresses response, including CAT, POD, SOD, Pro, MDA, Chlorophyll, and relative electrical conductivity (Figures 9K–Q). These results demonstrated that the physiological and biochemical indexes of GmCrRLK1L20-OE and GmCrRLK1L20-RNAi plants did not differ from wild-type plants under normal conditions (Figures 9K–Q). However, the GmCrRLK1L20-OE plants experienced delayed leaf wilt (Figures 9B,C) and had longer roots (Figures 9I,J), higher CAT, POD, SOD, Chlorophyll, and Pro contents (Figures 9K–O), lower relative electrical conductivity, and lower MDA contents compared to wild-type plants under drought and salt stresses conditions (Figures 9P,Q). GmCrRLK1L20-RNAi plants experienced significantly increased leaf wilt (Figures 9B,C), had shorter roots (Figures 9I,J), lower CAT, POD, SOD, Chlorophyll, and Pro contents (Figures 9K–O), higher relative electrical conductivity, and a higher MDA content (Figures 9P,Q) compared to EV plants under drought and salt stresses conditions.
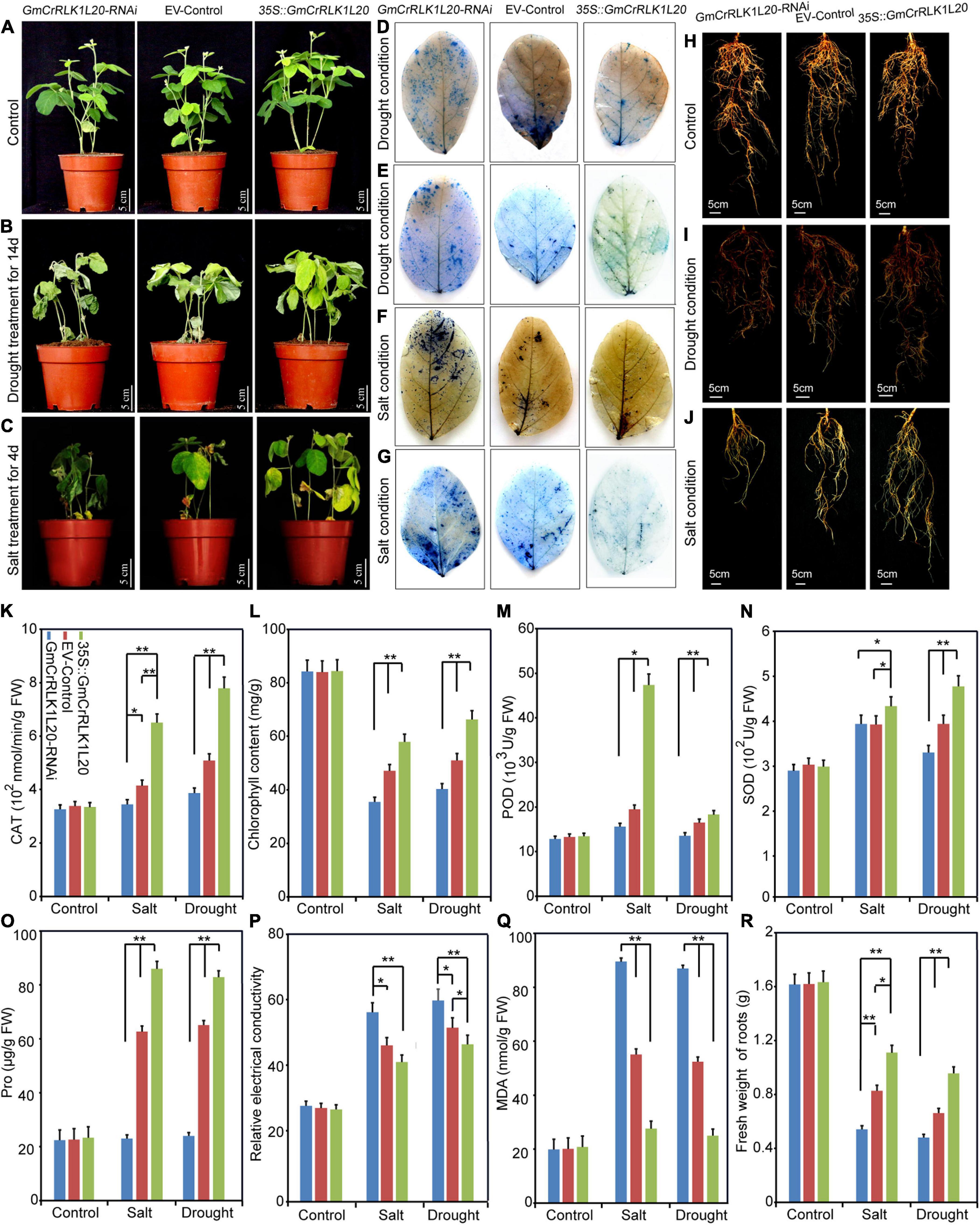
Figure 9. Phenotype and function analysis of soybean GmCrRLK1L20 in transgenic soybean hairy roots under drought and salt stresses conditions. (A–C) growth conditions of GmCrRLK1L20-RNAi, EV-Control (the empty plasmid of pCAMBIA3301), and GmCrRLK1L20-OE under normal, drought, and salt stresses conditions, respectively. NBT (D,F) staining of the leaves of GmCrRLK1L20-RNAi, EV-Control, and GmCrRLK1L20-OE under drought and salt conditions, respectively. The brightness of the leaf color indicates H2O2 and O2– contents (D,F). trypan blue (E,G) staining of the leaves of GmCrRLK1L20-RNAi, EV-Control, and GmCrRLK1L20-OE under drought and salt stresses conditions, respectively. Trypan blue staining demonstrates that dead cells can be stained, but living cells cannot (E,G). (H–J) The root phenotype of GmCrRLK1L20-RNAi, EV-Control, and GmCrRLK1L20-OE under normal, drought, and salt stresses conditions, respectively. (K) Catalase (CAT) content of transgenic soybean hairy root composite plants and EV-control plants under salt and drought conditions. (L) Chlorophyll content of transgenic soybean hairy root composite plants and EV-control plants under salt and drought conditions. (M) Peroxidase (POD) content of transgenic soybean hairy root composite plants and EV-control plants under salt and drought stresses conditions. (N) Superoxide dismutase (SOD) content of transgenic soybean hairy root composite plants and EV-control plants under salt and drought conditions. (O) Proline (Pro) content of transgenic soybean hairy root composite plants and EV-control plants under salt and drought conditions. (P) Relative electrical conductivity of transgenic soybean hairy root composite plants and EV-control plants under salt and drought conditions. (Q) Malondialdehyde (MDA) content of transgenic soybean hairy root composite plants and EV-control plants under salt and drought conditions. (R) Fresh weight of roots of transgenic soybean hairy root composite plants and EV-control plants under salt and drought conditions. Vertical bars demonstrate ± SE of three biological replicates. ANOVA test indicated that there were significant differences (*p < 0.05, **p < 0.01).
Analysis of Molecular Mechanism of GmCrRLK1L20 in Soybean
To further analyze the molecular mechanisms of GmCrRLK1L20 when subjected to abiotic stresses, we used qRT-PCR to assess the differential expressions of six stresses-responsive genes, GmWRKY40, GmMYB84, GmGST15, GmDREB-like, GmbZIP78, and GmNAC29 in the GmCrRLK1L20-RNAi, EV-Control, and GmCrRLK1L20-OE plants. Several studies have found that these six stresses-responsive genes were either directly or indirectly involved in abiotic stresses (Marè et al., 2004; Xiang et al., 2008; Shahnejat-Bushehri et al., 2016; Sarkar et al., 2019). Meanwhile, the six stresses-responsive genes were found to be significantly upregulated in our de novo transcriptomic soybean sequences, and the value of log2 fold change (GH_treat/CK1_treat) and (NaCl_treat/CK2_treat) of the six stresses-responsive genes varied from 3.6 to 9.5 (Supplementary Table 2). qRT-PCR assays of six stresses response-related genes were conducted after the transgenic soybean hairy root lines and EV-control plants were treated with 200 mM NaCl and 200 mM mannitol, using corresponding untreated lines. A two-fold change in expression was considered an induction of expression. These results demonstrating that expression levels of the six stresses-response genes in GmCrRLK1L20-OE plants were significantly upregulated compared to the EV-control plants under drought and salt conditions (Figure 10) and expression levels of these six stresses-response genes in the GmCrRLK1L20-RNAi were significantly down-regulated compared to the EV-control plants under drought and salt conditions (Figure 10).
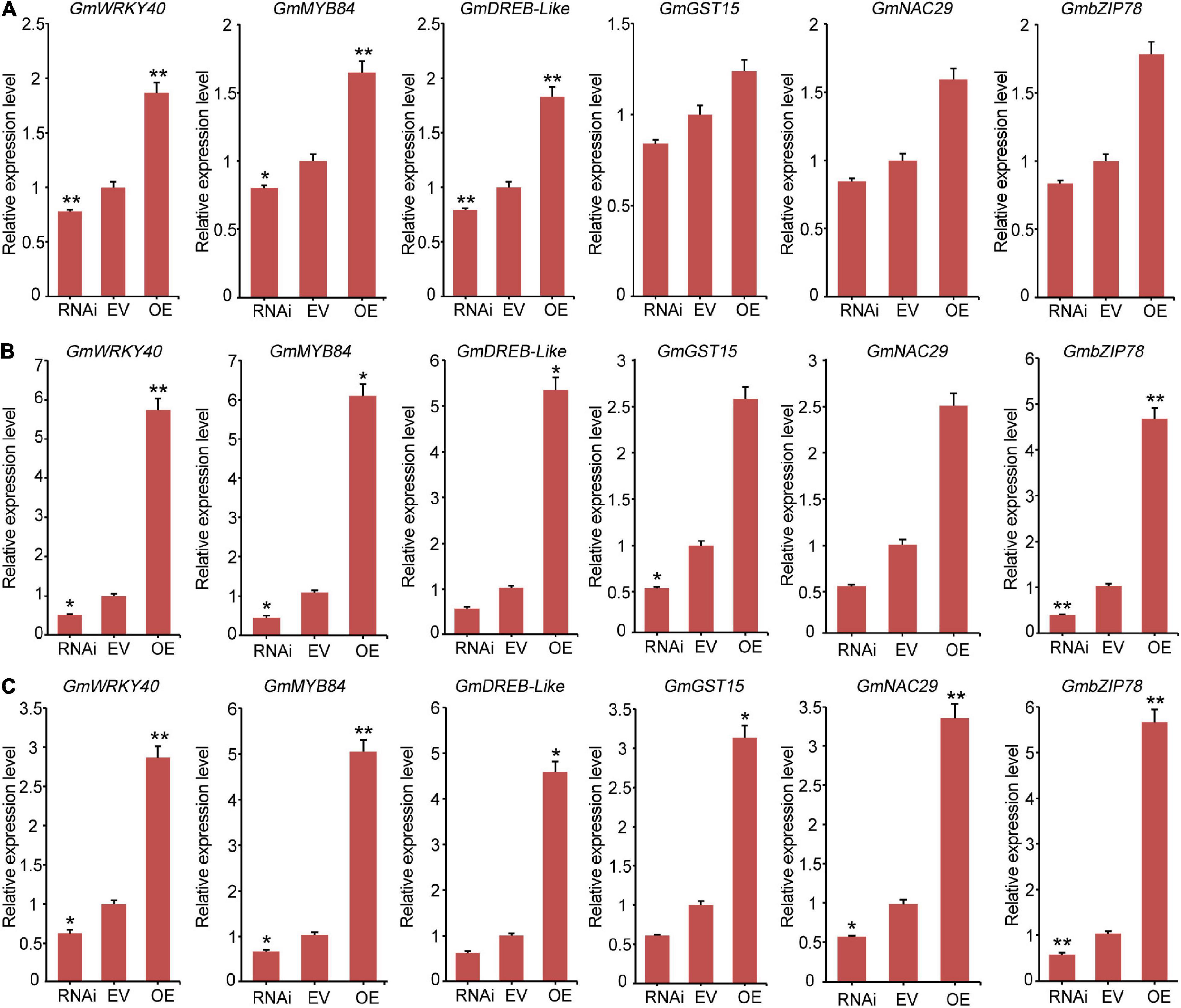
Figure 10. The expression levels of six stresses-responsive genes including GmWRKY40, GmMYB84, GmGST15, GmDREB-like, GmbZIP78, and GmNAC29. (A) The expression levels of six stresses-responsive genes were measured using qRT-PCR in transgenic GmCrRLK1L20 soybean hairy root plants under normal conditions. (B) The expression levels of six stresses-responsive genes were measured using qRT-PCR in transgenic GmCrRLK1L20 soybean hairy root plants under drought stresses conditions (200 mM mannitol). (C) The expression levels of six stresses-responsive genes were measured using qRT-PCR in transgenic GmCrRLK1L20 soybean hairy root plants under salt stress conditions (200 mM NaCl). RNAi, EV, and OE represent GmCrRLK1L20-RNAi, EV-Control (the empty plasmid of pCAMBIA3301), and GmCrRLK1L20-OE, respectively. Vertical bars indicate ± SE of three biological replicates. ANOVA test indicated that there were significant differences (*p < 0.05, **p < 0.01).
Discussion
Catharanthus roseus RLK1-likes are a special subfamily of RLKs that are found only in plants. Previous research has demonstrated that CrRLK1L has one or two more carbohydrate-binding malectin-like domains than that of the subfamily of RLKs in ECLB (Boisson-Dernier et al., 2011; Lindner et al., 2012). We believe that CrRLK1L could have evolved special new adaptations for their environment. CrRLK1L also plays an important role in growth, development, and stresses response in certain plants (Lindner et al., 2012). However, the biological and genetic roles of CrRLK1L in soybean are still unknown. By analyzing the structural characteristics of CrRLK1L genes in soybean, we found that the majority of soybean CrRLK1L genes lacked introns and that individual members have a short intron in the 5′UTR (5′untranslational region) or 3′UTR, but that no introns exist in both the 5′UTR and 3′ UTR (Figure 3). This indicates that these genes rarely exist in the form of alternative splicing (Li et al., 2015). The fact that most soybean CrRLK1L genes lack introns could be due to selection pressures during the evolutionary process. However, several soybean CrRLK1L genes have evolved a distinct exon-intron structure with 1–10 introns, and we discovered that the soybean CrRLK1L genes that contained similar gene structure and motif elements were divided into the same subfamily (Figure 3). We conducted an additional analysis of the phylogenetic tree of CrRLK1L genes in Arabidopsis, rice, and soybean according to their conserved amino acid sequence (Figure 1). The 71 CrRLK1L genes were classified into three clusters, while a majority of soybean CrRLK1L genes are distributed in cluster II. Five CrRLK1L family members from the de novo transcriptome soybean sequencing were classified into group II, with the exception of GmCrRLK1L24.
Analysis of the phylogenetic tree demonstrated that CrRLK1Ls shared high homology with Arabidopsis FER (Figure 1). The available evidence suggests that FER plays a vital role in hormone response (Hematy et al., 2007; Hematy and Hofte, 2008; Guo et al., 2009), cell growth, and abiotic stresses in plants (Huck et al., 2003). For example, FER participates in the identification between pollen tubes and its subsidiary cell (Boisson-Dernier et al., 2009), the elongation of pollen tubes and nutrient tissue cells, and the regulation of stomatal aperture (Rotman et al., 2003; Guo et al., 2009; Kessler et al., 2010). FER can regulate the production of ROS and calcium, which serve as vital second messengers in abiotic and biotic stresses responses (Luan et al., 2009; Gilroy et al., 2016). Based on the above analysis, we speculate that CrRLK1L family members could participate in hormone responses, and abiotic stresses. Further analysis of the cis-acting elements for the promoter of soybean CrRLK1L genes revealed that the 38 soybean CrRLK1L family members were highly correlated with stresses responses, such as ARE, ABRE, MYB, MYC, LTR, WUN-motif, MBS, DRE, TC-rich, and W-Box (Walker and Zhang, 1990; Walker, 1994; Chinnusamy et al., 2004; Shiu et al., 2004). This indicates that soybean CrRLK1L family members could play an important role in regulating stresses responses.
In our study, the method of the rapid drought that soybeans were placed on filter paper and the salt stress treatment that soybeans were immersed into 200 mM NaCl solution were used to preliminary screen the vital genes that rapidly respond to drought and salt stress, and this method of stress treatment can only be used in the laboratory and cannot be applied to agricultural environment or nature environment (Chen et al., 2015; Du et al., 2018). In addition, some studies have indicated that the methods for assaying plant resistance, on artificial media, filter paper and in hydroponic systems conditions, does not accurately mimic what is observed when plants are grown in soil (Rich and Watt, 2013; Robbins and Dinneny, 2015; Nelson and Oliver, 2017). Therefore, the method of the rapid drought and salt were used to initially screen the vital gene responding to drought and salt stress by qRT-PCR in our study. qRT-PCR analysis also found that drought and salt stresses could affect the expression levels of five CrRLK1L genes from de novo transcriptome sequencing of soybean. GmCrRLK1L20 had the highest transcription levels of the five genes under stresses conditions (Figure 7), which was consistent with the hierarchical clustering of expression profiles from drought and NaCl- RNA-seq (Figure 6). GmCrRLK1L20 was therefore selected for further analysis of its gene function under drought and salt stresses. Its molecular features indicated that the GmCrRLK1L20 protein is localized in the cell membrane. To further verify the interactions between the GmCrRLK1L20 gene and plant resistance, we generated transgenic soybean hairy root composite plants (RNAi, EV, and OE) via the Agrobacterium-mediated method and conducted nature drought and salt treatment for this composite plants, and we discovered that the GmCrRLK1L20-overexpression plants enhanced drought and salt tolerance compared with wide-type plants (Figures 9B,C). This suggests that the overexpressed plants had longer roots than the RNAi and EV plants (Figures 9H–J), had higher CAT, POD, SOD, and proline contents, and had lower relative electrical conductivity and a lower MDA content than RNAi and EV plants under drought and salt stresses (Figures 9K–Q). This demonstrates that GmCrRLK1L20 provides effective resistance against some abiotic stressors.
In order to analyze the molecular mechanism of the GmCrRLK1L20 gene, we selected the stresses-response genes GmWRKY40, GmMYB84, GmDREB-like, GmGST15, GmNAC29, and GmbZIP78 for additional study (Figure 10). GmWRKY40 is a WRKY transcription factor and can enhance the expression of downstream stresses-related target genes by specifically binding to the W-box. For example, researchers have demonstrated that WRKY transcription factor genes (OsWRKY11) can directly bind to the promoter of the stresses-responsive gene RAB21, and can enhance stresses tolerance in transgenic rice seedlings (Marè et al., 2004). GmMYB84, an R2R3-MYB transcription factor, can improve the expression levels of GmRBOHB-1&2 genes by binding to the MBS cis-elements in their promoter, enhancing stresses tolerance in soybean (Wang et al., 2017). GmDREB-like, a DREB-type transcription factor, can specifically recognize and bind to the DRE/CRT cis-acting element to regulate the expression of downstream stresses-responsive genes such as RD29A, which improves plant stresses resistance (Sarkar et al., 2019). GmNAC29, a NAC-type transcription factor, can recognize the CATGT and CACG elements of various downstream stresses-responsive gene promoters involved in plant stresses resistance (Tran et al., 2004). For example, the Arabidopsis NAC transcription factor JUB1 promotes stresses response by directly repressing the expression of GA3ox1 and DWF4 genes (Shahnejat-Bushehri et al., 2016). GmbZIP78, a bZIP-type transcription factor, can recognize ABRE responsive elements in stresses-responsive gene promoter regions to regulate the expression of ABA-related pathway genes. This improves sensitivity to ABA, which could enhance plant tolerance to drought and salt stresses (Xiang et al., 2008). GmGST15, a glutathione S-transferase protein, encodes a ROS-scavenging enzyme and regulates the homeostasis of ROS in cells. The ROS-scavenging enzyme was related to maintaining cell redox homeostasis and protecting cells from oxidative stresses under stressful conditions (Jha et al., 2011; Qi et al., 2018). Our results demonstrated that these stresses response genes were significantly upregulated in transgenic GmCrRLK1L20 soybean hairy root composite plants. This indicates that GmCrRLK1L20 improved soybean resistance to abiotic stresses by regulating the expression of several stresses response genes and playing a critical role in plant resistance to drought and salt stresses. These results indicate that the CrRLK1L gene is involved in plant stresses resistance and provides a theoretical basis for the additional study of plant production.
Conclusion
In this study, we identified 38 CrRLK1L genes in the soybean genome sequence. We conducted a comprehensive and systematic analysis of structural features and expression profiles and performed phylogenetic analyses. Finally, we identified a GmCrRLK1L20 gene that was significantly upregulated under drought and salt stresses conditions. These results provide insight into understanding the evolutionary mechanism of CrRLK1L genes and how they relate to stresses response in plants.
Data Availability Statement
The datasets presented in this study can be found in online repositories. The names of the repository/repositories and accession number(s) can be found below: NCBI SRA [accession: PRJNA694374].
Author Contributions
Z-SX coordinated the project, conceived and designed experiments, and edited the manuscript. Z-QW performed experiments and wrote the first draft of the manuscript. T-FY revised the manuscript. JC, Y-BZ, and MC contributed to data analysis and managed reagents. G-ZS, W-LW, and Y-ZM contributed with valuable discussions. All authors reviewed and approved the final manuscript.
Funding
This research was financially supported by the National Natural Science Foundation of China (31871624) and supported by China Postdoctoral Science Foundation (2019M660886) as well as Fundamental Research Funds for Central Non-Profit of Institute of Crop Sciences, Chinese Academy of Agricultural Sciences (Y2020PT12 and S2020ZC02).
Conflict of Interest
The authors declare that the research was conducted in the absence of any commercial or financial relationships that could be construed as a potential conflict of interest.
Acknowledgments
We are grateful to Drs. Lijuan Qiu and Tianfu Han of the Institute of Crop Science, Chinese Academy of Agricultural Sciences (CAAS) for providing soybean seeds. We would also like to thank Drs. Wensheng Hou and Hui Zhang of the Institute of Crop Science, CAAS, for providing vectors and the procedures for performing high-efficiency A. rhizogenes-mediated transformations, respectively.
Supplementary Material
The Supplementary Material for this article can be found online at: https://www.frontiersin.org/articles/10.3389/fpls.2021.614909/full#supplementary-material
Supplementary Figure 1 | The trypan blue and NBT staining of plant leaves under normal growth conditions.
Supplementary Table 1 | Primers and sequences used in this study.
Supplementary Table 2 | The value of log2 fold change of the six stresses-responsive genes from the de novo transcriptomic sequences of soybean.
Supplementary Table 3 | The expression data of the five soybean GmCrRLK1L genes under drought and salt treatment.
Abbreviations
RLKs, receptor-like protein kinases; PKC, protein kinase catalytic domain; ECLB, extracellular ligand-binding domain; TM, transmembrane domain; ABA, abscisic acid; ABRE, ABA-responsive elements; FW, fresh weight; GSDS, gene structure display server; LTR, low-temperature responsive; MBS, MYB binding site; NCBI, National Center for Biotechnology Information; qRT-PCR, real-time quantitative polymerase chain reaction.
Footnotes
- ^ http://arabidopsis.org
- ^ http://www.phytozome.net
- ^ http://smart.embl-heidelberg.de/
- ^ http://web.expasy.org/protparam/
- ^ http://gsds.cbi.pku.edu.cn
- ^ http://meme.nbcr.net/meme/intro.html
- ^ http://bioinformatics.psb.ugent.be/webtools/plantcare/html/
References
Acharya, B. R., Raina, S., Maqbool, S. B., Jagadeeswaran, G., Mosher, S. L., Appel, H. M., et al. (2007). Overexpression of CRK13, an Arabidopsis cysteine-rich receptor-like kinase, results in enhanced resistance to Pseudomonas syringae. Plant J. 50, 488–499. doi: 10.1111/j.1365-313X.2007.03064.x
Boisson-Dernier, A., Kessler, S. A., and Grossniklaus, U. (2011). The walls have ears: the role of plant CrRLK1Ls in sensing and transducing extracellular signals. J. Exp. Bot. 62, 1581–1591. doi: 10.1093/jxb/erq445
Boisson-Dernier, A., Lituiev, D. S., Nestorova, A., Franck, C. M., Thirugnanarajah, S., and Grossniklaus, U. (2013). ANXUR receptor-like kinases coordinate cell wall integrity with growth at the pollen tube tip via NADPH oxidases. PLoS Biol. 11:e1001719. doi: 10.1371/journal.pbio.1001719
Boisson-Dernier, A., Roy, S., Kritsas, K., Grobei, M. A., Jaciubek, M., Schroeder, J. I., et al. (2009). Disruption of the pollen-expressed FERONIA homologs ANXUR1 and ANXUR2 triggers pollen tube discharge. Development 136, 3279–3288. doi: 10.1242/dev.040071
Chen, J., Yu, F., Liu, Y., Du, C., Li, X., Zhu, S., et al. (2016). FERONIA interacts with ABI2-type phosphatases to facilitate signaling cross-talk between abscisic acid and RALF peptide in Arabidopsis. Proc. Natl. Acad. Sci. U.S.A. 113, E5519–E5527. doi: 10.1073/pnas.1608449113
Chen, M., Zhao, Y., Zhuo, C., Lu, S., and Guo, Z. (2015). Overexpression of a NF-YC transcription factor from bermudagrass confers tolerance to drought and salinity in transgenic rice. Plant Biotechnol. J. 13, 482–491. doi: 10.1111/pbi.12270
Chinnusamy, V., Schumaker, K., and Zhu, J. K. (2004). Molecular genetic perspectives on cross-talk and specificity in abiotic stress signalling in plants. J. Exp. Bot. 55, 225–236. doi: 10.1093/jxb/erh005
Choi, H. I., Park, H. J., Park, J. H., Kim, S., Im, M. Y., Seo, H. H., et al. (2005). Arabidopsis calcium-dependent protein kinase AtCPK32 interacts with ABF4, a transcriptional regulator of abscisic acid-responsive gene expression, and modulates its activity. Plant Physiol. 139, 1750–1761. doi: 10.1104/pp.105.069757
Deslauriers, S. D., and Larsen, P. B. (2010). FERONIA is a key modulator of brassinosteroid and ethylene responsiveness in Arabidopsis hypocotyls. Mol. Plant 3, 626–640. doi: 10.1093/mp/ssq015
Du, Y. T., Zhao, M. J., Wang, C. T., Gao, Y., Wang, Y. X., Liu, Y. W., et al. (2018). Identification and characterization of GmMYB118 responses to drought and salt stress. BMC Plant Biol. 18:320. doi: 10.1186/s12870-018-1551-7
Duan, Q., Kita, D., Li, C., Cheung, A. Y., and Wu, H. M. (2010). FERONIA receptor-like kinase regulates RHO GTPase signaling of root hair development. Proc. Natl. Acad .Sci. U.S.A. 107, 17821–17826. doi: 10.1073/pnas.1005366107
Edwards, R., Dixon, D. P., and Walbot, V. (2000). Plant glutathione S-transferases: enzymes with multiple functions in sickness and in health. Trends Plant Sci. 5, 193–198. doi: 10.1016/s1360-1385(00)01601-0
Feng, L., Gao, Z., Xiao, G., Huang, R., and Zhang, H. (2014). Leucine-rich repeat receptor-like kinase FON1 regulates drought stress and seed germination by activating the expression of ABA-responsive genes in rice. Plant Mol. Biol. Rep. 32, 1158–1168. doi: 10.1007/s11105-014-0718-0
Gilroy, S., Bialasek, M., Suzuki, N., Gorecka, M., Devireddy, A. R., Karpinski, S., et al. (2016). ROS, calcium, and electric signals: key mediators of rapid systemic signaling in plants. Plant Physiol. 171, 1606–1615. doi: 10.1104/pp.16.00434
Guo, H., Li, L., Ye, H., Yu, X., Algreen, A., and Yin, Y. (2009). Three related receptor-like kinases are required for optimal cell elongation in Arabidopsis thaliana. Proc. Natl. Acad .Sci. U.S.A. 106, 7648–7653. doi: 10.1073/pnas.0812346106
Haruta, M., Sabat, G., Stecker, K., Minkoff, B. B., and Sussman, M. R. (2014). A peptide hormone and its receptor protein kinase regulate plant cell expansion. Science 343, 408–411. doi: 10.1126/science.1244454
He, G. H., Xu, J. Y., Wang, Y. X., Liu, J. M., Li, P. S., Chen, M., et al. (2016). Drought-responsive WRKY transcription factor genes TaWRKY1 and TaWRKY33 from wheat confer drought and/or heat resistance in Arabidopsis. BMC Plant Biol. 16:116. doi: 10.1186/s12870-016-0806-4
Hematy, K., and Hofte, H. (2008). Novel receptor kinases involved in growth regulation. Curr. Opin. Plant Biol. 11, 321–328. doi: 10.1016/j.pbi.2008.02.008
Hematy, K., Sado, P. E., Van Tuinen, A., Rochange, S., Desnos, T., Balzergue, S., et al. (2007). A receptor-like kinase mediates the response of Arabidopsis cells to the inhibition of cellulose synthesis. Curr. Biol. 17, 922–931. doi: 10.1016/j.cub.2007.05.018
Huck, N., Moore, J. M., Federer, M., and Grossniklaus, U. (2003). The Arabidopsis mutant feronia disrupts the female gametophytic control of pollen tube reception. Development 130, 2149–2159. doi: 10.1242/dev.00458
Hundertmark, M., and Hincha, D. K. (2008). LEA (late embryogenesis abundant) proteins and their encoding genes in Arabidopsis thaliana. BMC Genomics 9:118. doi: 10.1186/1471-2164-9-118
Jha, B., Sharma, A., and Mishra, A. (2011). Expression of SbGSTU (tau class glutathione S-transferase) gene isolated from Salicornia brachiata in tobacco for salt tolerance. Mol. Biol. Rep. 38, 4823–4832. doi: 10.1007/s11033-010-0625-x
Kessler, S. A., Shimosato-Asano, H., Keinath, N. F., Wuest, S. E., Ingram, G., Panstruga, R., et al. (2010). Conserved molecular components for pollen tube reception and fungal invasion. Science 330, 968–971. doi: 10.1126/science.1195211
Le, D. T., Nishiyama, R., Watanabe, Y., Mochida, K., Yamaguchi-Shinozaki, K., Shinozaki, K., et al. (2011). Genome-wide expression profiling of soybean two-component system genes in soybean root and shoot tissues under dehydration stress. DNA Res. 18, 17–29. doi: 10.1093/dnares/dsq032
Li, C., Yeh, F. L., Cheung, A. Y., Duan, Q., Kita, D., Liu, M. C., et al. (2015). Glycosylphosphatidylinositol-anchored proteins as chaperones and co-receptors for FERONIA receptor kinase signaling in Arabidopsis. eLife 4:e06587. doi: 10.7554/eLife.06587
Lindner, H., Muller, L. M., Boisson-Dernier, A., and Grossniklaus, U. (2012). CrRLK1L receptor-like kinases: not just another brick in the wall. Curr. Opin. Plant Biol. 15, 659–669. doi: 10.1016/j.pbi.2012.07.003
Liu, P. L., Du, L., Huang, Y., Gao, S. M., and Yu, M. (2017). Origin and diversification of leucine-rich repeat receptor-like protein kinase (LRR-RLK) genes in plants. BMC Evol. Biol. 17:47. doi: 10.1186/s12862-017-0891-5
Luan, S., Lan, W., and Chul Lee, S. (2009). Potassium nutrition, sodium toxicity, and calcium signaling: connections through the CBL-CIPK network. Curr. Opin. Plant Biol. 12, 339–346. doi: 10.1016/j.pbi.2009.05.003
Mao, D., Yu, F., Li, J., Van de Poel, B., Tan, D., Li, J., et al. (2015). FERONIA receptor kinase interacts with S-adenosylmethionine synthetase and suppresses S-adenosylmethionine production and ethylene biosynthesis in Arabidopsis. Plant Cell Environ. 38, 2566–2574. doi: 10.1111/pce.12570
Marè, C., Mazzucotelli, E., Crosatti, C., Francia, E., Stanca, A. M., and Cattivelli, L. (2004). Hv-WRKY38: a new transcription factor involved in cold- and drought-response in barley. Plant Mol. Biol. 55, 399–416. doi: 10.1007/s11103-004-0906-7
Mittler, R. (2006). Abiotic stress, the field environment and stress combination. Trends Plant Sci. 11, 15–19. doi: 10.1016/j.tplants.2005.11.002
Miyazaki, S., Murata, T., Sakurai-Ozato, N., Kubo, M., Demura, T., Fukuda, H., et al. (2009). ANXUR1 and 2, sister genes to FERONIA/SIRENE, are male factors for coordinated fertilization. Curr. Biol. 19, 1327–1331. doi: 10.1016/j.cub.2009.06.064
Nelson, S. K., and Oliver, M. J. (2017). A soil-plate based pipeline for assessing cereal root growth in response to polyethylene glycol (PEG)-induced water deficit stress. Front. Plant Sci. 8:1272. doi: 10.3389/fpls.2017.01272
Nguyen, Q. N., Lee, Y. S., Cho, L. H., Jeong, H. J., An, G., and Jung, K. H. (2015). Genome-wide identification and analysis of Catharanthus roseus RLK1-like kinases in rice. Planta 241, 603–613. doi: 10.1007/s00425-014-2203-2
Niu, E., Cai, C., Zheng, Y., Shang, X., Fang, L., and Guo, W. (2016). Genome-wide analysis of CrRLK1L gene family in Gossypium and identification of candidate CrRLK1L genes related to fiber development. Mol. Genet. Genomics 291, 1137–1154. doi: 10.1007/s00438-016-1169-0
Qi, J., Song, C. P., Wang, B., Zhou, J., Kangasjarvi, J., Zhu, J. K., et al. (2018). Reactive oxygen species signaling and stomatal movement in plant responses to drought stress and pathogen attack. J. Integr. Plant Biol. 60, 805–826. doi: 10.1111/jipb.12654
Ramachandra Reddy, A., Chaitanya, K. V., and Vivekanandan, M. (2004). Drought-induced responses of photosynthesis and antioxidant metabolism in higher plants. J. Plant Physiol. 161, 1189–1202. doi: 10.1016/j.jplph.2004.01.013
Rich, S. M., and Watt, M. (2013). Soil conditions and cereal root system architecture: review and considerations for linking Darwin and Weaver. J. Exp. Bot. 64, 1193–1208. doi: 10.1093/jxb/ert043
Riechmann, J. L., Heard, J., Martin, G., Reuber, L., Jiang, C., Keddie, J., et al. (2000). Arabidopsis transcription factors: genome-wide comparative analysis among eukaryotes. Science 290, 2105–2110. doi: 10.1126/science.290.5499.2105
Robbins, N. E. II, and Dinneny, J. R. (2015). The divining root: moisture-driven responses of roots at the micro- and macro-scale. J. Exp. Bot. 66, 2145–2154. doi: 10.1093/jxb/eru496
Rotman, N., Rozier, F., Boavida, L., Dumas, C., Berger, F., and Faure, J. E. (2003). Female control of male gamete delivery during fertilization in Arabidopsis thaliana. Curr. Biol. 13, 432–436. doi: 10.1016/s0960-9822(03)00093-9
Sarkar, T., Thankappan, R., Mishra, G. P., and Nawade, B. D. (2019). Advances in the development and use of DREB for improved abiotic stress tolerance in transgenic crop plants. Physiol. Mol. Biol. Plants 25, 1323–1334. doi: 10.1007/s12298-019-00711-2
Schulze-Muth, P., Irmler, S., Schröder, G., and Schröder, J. (1996). Novel type of receptor-like protein kinase from a higher plant (Catharanthus roseus). cDNA, gene, intramolecular autophosphorylation, and identification of a threonine important for auto- and substrate phosphorylation. J. Biol. Chem. 271, 26684–26689. doi: 10.1074/jbc.271.43.26684
Shahnejat-Bushehri, S., Tarkowska, D., Sakuraba, Y., and Balazadeh, S. (2016). Arabidopsis NAC transcription factor JUB1 regulates GA/BR metabolism and signalling. Nat. Plants 2:16013. doi: 10.1038/nplants.2016.13
Shi, W. Y., Du, Y. T., Ma, J., Min, D. H., Jin, L. G., Chen, J., et al. (2018). The WRKY transcription factor GmWRKY12 confers drought and salt tolerance in Soybean. Int. J. Mol. Sci. 19:4087. doi: 10.3390/ijms19124087
Shinozaki, K., and Yamaguchi-Shinozaki, K. (2007). Gene networks involved in drought stress response and tolerance. J. Exp. Bot. 58, 221–227. doi: 10.1093/jxb/erl164
Shiu, S. H., Karlowski, W. M., Pan, R., Tzeng, Y. H., Mayer, K. F., and Li, W. H. (2004). Comparative analysis of the receptor-like kinase family in Arabidopsis and rice. Plant Cell 16, 1220–1234. doi: 10.1105/tpc.020834
Thynne, E., Saur, I. M. L., Simbaqueba, J., Ogilvie, H. A., Gonzalez-Cendales, Y., Mead, O., et al. (2017). Fungal phytopathogens encode functional homologues of plant rapid alkalinization factor (RALF) peptides. Mol. Plant Pathol. 18, 811–824. doi: 10.1111/mpp.12444
Tran, L. S., Nakashima, K., Sakuma, Y., Simpson, S. D., Fujita, Y., Maruyama, K., et al. (2004). Isolation and functional analysis of Arabidopsis stress-inducible NAC transcription factors that bind to a drought-responsive cis-element in the early responsive to dehydration stress 1 promoter. Plant Cell 16, 2481–2498. doi: 10.1105/tpc.104.022699
Vaid, N., Pandey, P., Srivastava, V. K., and Tuteja, N. (2015). Pea lectin receptor-like kinase functions in salinity adaptation without yield penalty, by alleviating osmotic and ionic stresses and upregulating stress-responsive genes. Plant Mol. Biol. 88, 193–206. doi: 10.1007/s11103-015-0319-9
Walker, J. C. (1994). Structure and function of the receptor-like protein kinases of higher plants. Plant Mol. Biol. 26, 1599–1609. doi: 10.1007/BF00016492
Walker, J. C., and Zhang, R. (1990). Relationship of a putative receptor protein kinase from maize to the S-locus glycoproteins of Brassica. Nature 345, 743–746. doi: 10.1038/345743a0
Wang, G., Ellendorff, U., Kemp, B., Mansfield, J. W., Forsyth, A., Mitchell, K., et al. (2008). A genome-wide functional investigation into the roles of receptor-like proteins in Arabidopsis. Plant Physiol. 147, 503–517. doi: 10.1104/pp.108.119487
Wang, N., Zhang, W., Qin, M., Li, S., Qiao, M., Liu, Z., et al. (2017). Drought Tolerance Conferred in Soybean (Glycine max. L) by GmMYB84, a Novel R2R3-MYB Transcription Factor. Plant Cell Physiol. 58, 1764–1776. doi: 10.1093/pcp/pcx111
Xiang, Y., Tang, N., Du, H., Ye, H., and Xiong, L. (2008). Characterization of OsbZIP23 as a key player of the basic leucine zipper transcription factor family for conferring abscisic acid sensitivity and salinity and drought tolerance in rice. Plant Physiol. 148, 1938–1952. doi: 10.1104/pp.108.128199
Yang, T., Chaudhuri, S., Yang, L., Du, L., and Poovaiah, B. W. (2010). A calcium/calmodulin-regulated member of the receptor-like kinase family confers cold tolerance in plants. J. Biol. Chem. 285, 7119–7126. doi: 10.1074/jbc.M109.035659
Keywords: CrRLK1L, genome-wide analysis, drought, salt, soybean
Citation: Wang Z-Q, Yu T-F, Sun G-Z, Zheng J-C, Chen J, Zhou Y-B, Chen M, Ma Y-Z, Wei W-L and Xu Z-S (2021) Genome-Wide Analysis of the Catharanthus roseus RLK1-Like in Soybean and GmCrRLK1L20 Responds to Drought and Salt Stresses. Front. Plant Sci. 12:614909. doi: 10.3389/fpls.2021.614909
Received: 07 October 2020; Accepted: 15 February 2021;
Published: 18 March 2021.
Edited by:
Eric Von Wettberg, University of Vermont, United StatesReviewed by:
Richard Odongo Magwanga, Institute of Cotton, Chinese Academy of Agricultural Sciences, ChinaErtugrul Filiz, Duzce University, Turkey
Xiaoli Sun, Heilongjiang Bayi Agricultural University, China
Copyright © 2021 Wang, Yu, Sun, Zheng, Chen, Zhou, Chen, Ma, Wei and Xu. This is an open-access article distributed under the terms of the Creative Commons Attribution License (CC BY). The use, distribution or reproduction in other forums is permitted, provided the original author(s) and the copyright owner(s) are credited and that the original publication in this journal is cited, in accordance with accepted academic practice. No use, distribution or reproduction is permitted which does not comply with these terms.
*Correspondence: Zhao-Shi Xu, xuzhaoshi@caas.cn; Wen-Liang Wei, whwenliang@163.com
†These authors have contributed equally to this work