- 1Institute of Cardiovascular Disease of Integrated Traditional Chinese and Western Medicine, Shuguang Hospital Affiliated to Shanghai University of Traditional Chinese Medicine, Shanghai, China
- 2Branch of National Clinical Research Center for Chinese Medicine Cardiology, Shuguang Hospital Affiliated to Shanghai University of Traditional Chinese Medicine, Shanghai, China
- 3Department of Cardiovascular Disease, Shuguang Hospital Affiliated to Shanghai University of Traditional Chinese Medicine, Shanghai, China
Acute myocardial infarction (AMI) is a severe ischemic disease with high morbidity and mortality worldwide. Maladaptive cardiac remodeling is a series of abnormalities in cardiac structure and function that occurs following myocardial infarction (MI). The pathophysiology of this process can be separated into two distinct phases: the initial inflammatory response, and the subsequent longer-term scar revision that includes the regression of inflammation, neovascularization, and fibrotic scar formation. Extracellular vesicles are nano-sized lipid bilayer vesicles released into the extracellular environment by eukaryotic cells, containing bioinformatic transmitters which are essential mediators of intercellular communication. EVs of different cellular origins play an essential role in cardiac remodeling after myocardial infarction. In this review, we first introduce the pathophysiology of post-infarction cardiac remodeling, as well as the biogenesis, classification, delivery, and functions of EVs. Then, we explore the dual role of these small molecule transmitters delivered by EVs in post-infarction cardiac remodeling, including the double-edged sword of pro-and anti-inflammation, and pro-and anti-fibrosis, which is significant for post-infarction cardiac repair. Finally, we discuss the pharmacological and engineered targeting of EVs for promoting heart repair after MI, thus revealing the potential value of targeted modulation of EVs and its use as a drug delivery vehicle in the therapeutic process of post-infarction cardiac remodeling.
1 Introduction
Among the leading causes of death and disability worldwide are acute myocardial infarction (AMI) and end-stage heart failure (HF), causing approximately 17 million deaths annually and accounting for 30% of all deaths globally (Ong et al., 2018; Roth et al., 2020). Coronary atherosclerosis underlies the development of myocardial infarction (MI), which contributes to vessel lumen narrowing and ultimately elicits myocardial necrosis due to hypoxia/ischemia. Currently, the primary goal of clinical treatment for such disease is to promptly restore flow in the occluded vessel and restore the ischemic myocardium, commonly performed by direct percutaneous coronary intervention. Intriguingly, however, such interventions can contribute to cardiomyocyte death and myocardial injury (Schirone et al., 2022). Consequently, novel therapies and interventions are urgently required to attenuate adverse ventricular remodeling and mitigate HF.
The heart undergoes a series of structural and functional alterations to adapt to cardiac injury induced by MI, which is known as cardiac remodeling (Zhao et al., 2020). Specifically, cardiac remodeling is a complex pathophysiological process, including inflammation, mitochondria dysfunction, oxidative stress, cardiac hypertrophy, and cardiac fibrosis. The entire process can be divided into two stages, the inflammatory phase and the fibrotic scar revision phase (Schirone et al., 2017; Liu H et al., 2020). The initial phase involves the exposure of intracellular contents into the extracellular environment due to cell necrosis, called damage-associated molecular patterns (DAMPs), triggering and exacerbating sterile inflammation. While the latter phase is attributed to the transdifferentiation of fibroblasts into myofibroblasts stimulated by multiple factors, including inflammatory factors, angiotensin II, and mechanical stress stimulation, which secrete collagen and facilitate scar repair in the infarcted region and maintain the structural integrity of the ventricle (Prabhu and Frangogiannis, 2016; Peet et al., 2020).
During cardiac remodeling, numerous immune cells recruitment to the infarct area, interact with cardiac fibroblasts (CFs) which crucially coordinates the balance between profibrotic and antifibrotic effects (Zaidi et al., 2021). In the initial inflammatory phase of AMI, the recruitment and activation of immune cells, such as macrophages, neutrophils, and monocytes, not only secrete inflammatory mediators to aggravate the inflammation but also shift towards an anti-inflammatory phenotype, which can prevent the release of their contents into the extracellular environment by removing necrotic cellular debris from the MI zone to attenuate inflammation (Ong et al., 2018; Andreadou et al., 2019; Doran et al., 2020). Besides this, the crosstalk between other diverse cells, including mesenchymal stem cells, endothelial cells (ECs), and cardiomyocytes is also essential for maintaining dynamic balance throughout the whole process. Interestingly, however, these cells secrete large numbers of EVs, which carry numerous bioactive substances, including miRNAs, lipids, and proteins. The bioactive substances not only have similar roles to parental cells but are also essential regulators of intercellular communication (Xiong et al., 2021). In general, there are three major subtypes of EVs, apoptotic bodies (ABs), microvesicles (MVs), and exosomes (Sanchez-Alonso et al., 2018), of which the most comprehensively explored are exosomes. In contrast, the least well-studied are ABs.
This review summarizes the dual roles of EVs derived from diverse cells in postinfarction cardiac remodeling, including pro-inflammatory and anti-inflammatory effects in the initial postinfarction inflammatory response and profibrotic and antifibrotic effects in the scar repair phase. Importantly, we discuss the role of crosstalk between these EVs in achieving a coordinated dynamic balance between the inflammatory response and the fibrotic process, which is significant for maladaptive cardiac remodeling after MI. In parallel, we also discuss therapeutic opportunities for targeting EVs that play a significant role in post-infarction cardiac remodeling, including pharmacological preconditioning and engineered modification. These elements will be beneficial in expanding therapeutic strategies for post-infarction cardiac remodeling.
2 Pathophysiological process of cardiac remodeling after myocardial infarction
AMI results from myocardial blood flow obstruction due to atherosclerotic plaque rupture or erosion (Ibanez et al., 2018). AMI is currently treated mainly by revascularization procedures such as thrombolysis and coronary intervention. However, recanalizing occluded vessels under long-term ischemic conditions causes additional myocardial damage, termed “I/R injury” (Yellon and Hausenloy, 2007). Such a process is accompanied by a blockage of blood flow, a sudden and dramatic interruption of oxygen and nutrient supply in the blood, a short cessation of mitochondrial oxidative phosphorylation (Frank et al., 2012), a dramatic decrease in available intracellular adenosine triphosphate (ATP), and a reduction in cardiomyocyte contractile function and cardiac stiffness and contracture minutes after the onset of ischemia (Jennings and Reimer, 1991). In the meantime, the myocardial ischemic injury may contribute to substantial cardiomyocyte death via different mechanisms. At the outset of reperfusion, cytosolic calcium overload and massive reactive oxygen species (ROS) generation rupture the mitochondrial membrane and release the contents, which in turn activate the caspase-mediated intrinsic pathway of apoptosis (Schirone et al., 2022). On the other hand, phosphorylation of receptor-interacting protein kinase 3 (RIPK3) is recruited and activated by the receptor-interacting protein kinase 1 (RIPK1), which in turn triggers necroptosis and exacerbate the pathological process after MI (Rodriguez et al., 2016).
The large volume of dead and injured cardiomyocyte contents (including heat shock proteins, organelle debris, and nuclear debris) enter the interstitial matrix, namely, DAMPs (Frangogiannis, 2012; Timmers et al., 2012). Simultaneously, innate immunity is triggered when pattern recognition receptors (PRRs) on leukocytes recognize DAMPs (Suetomi et al., 2019; Silvis et al., 2020). Immediately afterwards, the Toll-like receptor signaling pathway and other numerous inflammatory pathways are activated, thereby inducing proinflammatory cytokine expression, including interleukin 6 (IL-6), interleukin 1β (IL-1β), and tumor necrosis factor α (TNF-α), as well as the expression of chemokines, including monocyte chemotactic protein-1 (MCP-1/CCL2) (Frangogiannis, 2007). Additionally, neutrophils and inflammatory monocytes recruited by chemokine CCL2 activation are recruited to the infarcted heart (Dewald et al., 2005; Ley et al., 2007). On the other hand, when tissues are damaged, nuclear factor κB (NF-κB) is activated in ROS-dependent way and increases the expression of specific cellular genes, which can also eventually stimulate the growth of cells associated with inflammation and immune responses and exacerbate the inflammation even further (Kabe et al., 2005; Sun, 2009).
Short-term inflammatory responses contribute to debris removal, while long-term inflammation can facilitate extracellular matrix degradation and cell death, resulting in infarct size expansion. Consequently, timely resolution of the inflammation is essential for maintaining post-infarction remodeling. At the same time, apoptosis and clearance of neutrophils in the infarcted area is a hallmark that inflammation is beginning to subside (Frangogiannis, 2012; Westman et al., 2016; Ong et al., 2018). As leukocyte infiltration removes dead cells and matrix debris from the infarct and represses inflammatory mediator release, the anti-inflammatory monocyte subsets become dominant (Frangogiannis, 2008; Prabhu and Frangogiannis, 2016). Furthermore, suppressive subsets of other immune cells like lymphocytes, monocytes, and anti-inflammatory macrophages can suppress inflammation in the infarcted heart. Macrophages, in particular, play a crucial role in this process by shifting from a pro-inflammatory to an anti-inflammatory phenotype (Yan et al., 2013). In this way, the post-infarction pathological process moves from the pro-inflammatory response phase to the anti-inflammatory response phase, collectively referred to as the inflammatory response phase (within 3–7 days after MI), and eventually to the scar repair phase (7 days after MI) (Liu S et al., 2020).
In the cardiac tissue remodeling phase, fibroblast proliferation, extracellular matrix secretion, and eventually fibrotic scar maturation (Kologrivova et al., 2021). During the scar proliferation phase, subpopulations of monocytes and macrophages activate and recruit mesenchymal repair cells and primarily fibroblast-like cells by secreting growth factors (Frangogiannis, 2014). There is evidence that CFs originate from circulating progenitor stem cells (PSCs) recruited into the ventricular myocardium after birth and distribute throughout the normal myocardium as strands and sheets between myocardial muscle fibers and are critical effector cells in the scar repair process (Visconti and Markwald, 2006). In the normal physiological state, CFs account for 60%–70% of total cardiac cell numbers (Porter and Turner, 2009). They secrete collagen, which provides structural scaffolding for cardiomyocytes, coordinates myocardial motion, and mediates the onset of electrical activity (Bretherton et al., 2020). Under pathological conditions, fibroblasts transdifferentiate into myofibroblasts (MFBs) in response to various stimuli, including renin-angiotensin system activation, mechanochemical signaling, and release of transforming growth factor β (TGF-β), inflammatory factors (Frangogiannis, 2015; Han et al., 2022).
MFBs possess characteristics of both fibroblasts and smooth muscle cells, and secrete collagen to promote myocardial fibrosis. Meanwhile, these cells are analogous to smooth muscle cell contraction (as present in the vascular system), which can be modulated by circulating factors and neurohormones, including angiotensin II (van den Borne et al., 2010). Apoptosis of most repair cells indicates the end of the proliferative phase, and as the development of MI pathophysiology, the scar matures via a steady increase in collagen cross-linking (Frangogiannis, 2012). However, persistent MFBs differentiation and activation, as well as extracellular matrix (ECM) deposition, contribute to a decreased compliance of the myocardium (Umbarkar et al., 2021). On the other hand, progressive decline in the delivery of oxygen and nutrients induces atrophy and cell death in the cardiomyocytes, contributing to progressive acute left ventricular dilatation and, ultimately, dysfunction and heart failure (Gibb et al., 2020). Thus, controlling the fibrosis process and achieving a dynamic balance between pro-and anti-fibrosis are of great significance for post-infarction repair. Given the complexity of post-infarction cardiac remodeling, this review focuses on two significant aspects: inflammatory response and fibrotic scar repair.
3 Biogenesis, sorting, delivery, and outcome of extracellular vesicles
EVs are nanoscale lipid particles generated by the endosomal system of eukaryotes and secreted into the extracellular environment (Borges et al., 2013; van der Pol et al., 2014; Yanez-Mo et al., 2015; Zaborowski et al., 2015). Currently, there is still no exact criteria on the nomenclature of EVs. Various names are used, such as exosomes, microparticles, MVs, ABs. Remarkably, the nomenclature of “extracellular vesicles” proposed by Chistiakov mainly used in this review (Chistiakov et al., 2015), which means that EVs are typically categorized subsets by their size, formation, marker proteins, release mechanisms, and lipid composition, including three types of plasma membrane exfoliated vesicles: exosomes (30–150 nm), MVs (1,000–1,000 nm), and ABs (500–2,000 nm) (Liu H et al., 2021). Exosomes are phospholipid bilayers membrane-enclosed vesicles (Pegtel and Gould, 2019), which were initially discovered in sheep reticulocytes in 1983 and then were named “exosomes” by Johnstone in 1987 (Pan and Johnstone, 1983; Johnstone et al., 1987). Several studies have indicated that the inward budding of the early endosomal membrane forms early endosomes and then matures into late endosomes which are often packed with small intraluminal vesicles (ILVs) and evolve into multivesicular bodies (MVBs) (Ohayon et al., 2021). After a series of intracellular transport, the degradation of vesicles occurs when some MVBs fuse with lysosomes. While other MVBs release ILVs such as exosomes when fusing with the plasma membrane (Kowal et al., 2014; Villarroya-Beltri et al., 2016). These exosomes can be detected in diverse bodily fluids, such as breast milk, saliva, blood, urine (Pisitkun et al., 2004; Caby et al., 2005; Admyre et al., 2007; Palanisamy et al., 2010). Simultaneously, numerous studies suggest that a broad range of cell types, including cardiomyocytes, immune cells, progenitor cells, fibroblasts, and stem cells can generate exosomes (Zitvogel et al., 1998; Doyle and Wang, 2019).
MVs were first described by Peter Wolf in 1967 and are generated by the outward budding of plasma membrane (Wolf, 1967), and they are also the result of dynamic interaction between redistribution of phosphatidylserine (PS) and the remodeling of cytoskeletal proteins (Zwaal and Schroit, 1997; Leventis and Grinstein, 2010); proteins and phospholipids are distributed very heterogeneously and form microdomains within the membrane. Aminophospholipid translocases tightly control this asymmetric distribution of PS on plasma membranes. Moreover, flippase is a translocase that translocates PS from the outer leaflet to the inner leaflet of the membrane, while floppase transfers phospholipids from the inner to the outer leaflet of the plasma membrane (Zwaal and Schroit, 1997; Hugel et al., 2005). Immediately afterwards, actin-myosin interactions promote cytoskeleton contraction, aid cell membranes’ budding, and ultimately form MVs (McConnell et al., 2009; Muralidharan-Chari et al., 2009). The number of these generated MVs relies on the physiological state and microenvironment of the donor cells while consumed MVs depends on the physiological state and microenvironment of the recipient cells (Zaborowski et al., 2015).
These EVs can enter the recipient cells via fusing with cellular membranes; on the other hand, it also enters the recipient cells via different endocytic pathways, including phagocytosis clathrin-mediated endocytosis, caveolae, or lipid raft-mediated endocytosis (Feng et al., 2010; Tian et al., 2014; Costa Verdera et al., 2017; Hou and Chen, 2021). Moreover, classical intercellular adhesion molecules also mediate the binding of EVs to the target cells (Zhao et al., 2015); specifically, on the one hand, TIM family members (TIM1, TIM4) PS transmembrane receptors are used by target cells for exosomes entry (Miyanishi et al., 2007), on the other hand, exosomes derived from B cells containing a 2,3-linked dialkylated glycoprotein can be captured by lymph node and spleen CD169+ macrophages (Saunderson et al., 2014). At the same time, macrophages uptake exosome via C-type lectins expressed in macrophages and galectin-5 exposed to exosome (Barres et al., 2010). Meanwhile, multiple mechanisms have been demonstrated to be associated with regulating the biogenesis of EVs, especially the endosomal sorting complex required for transport (ESCRT) or non-ESCRT mechanisms (Assil et al., 2015). ESCRT consists of four core subunits (ESCRT-0; ESCRT-I; ESCRT-II; ESCRT-III), where ESCRT-0 mediates substrate recognition and sorting, ESCRT-I and ESCRT-II are critical for mediating endosomal membrane inward budding, and ESCRT-III is responsible for shearing the neck of the budding body, thereby orchestrating the formation of an inward budding vesicle of MVBs (Hurley and Hanson, 2010; Hanson and Cashikar, 2012; Tarasov et al., 2021). Considering the biological complexity of EVs and is susceptible to the environment, there are yet no established criteria to distinguish different types of EVs, which limits the development of related studies (Amosse et al., 2017) (Figure 1).
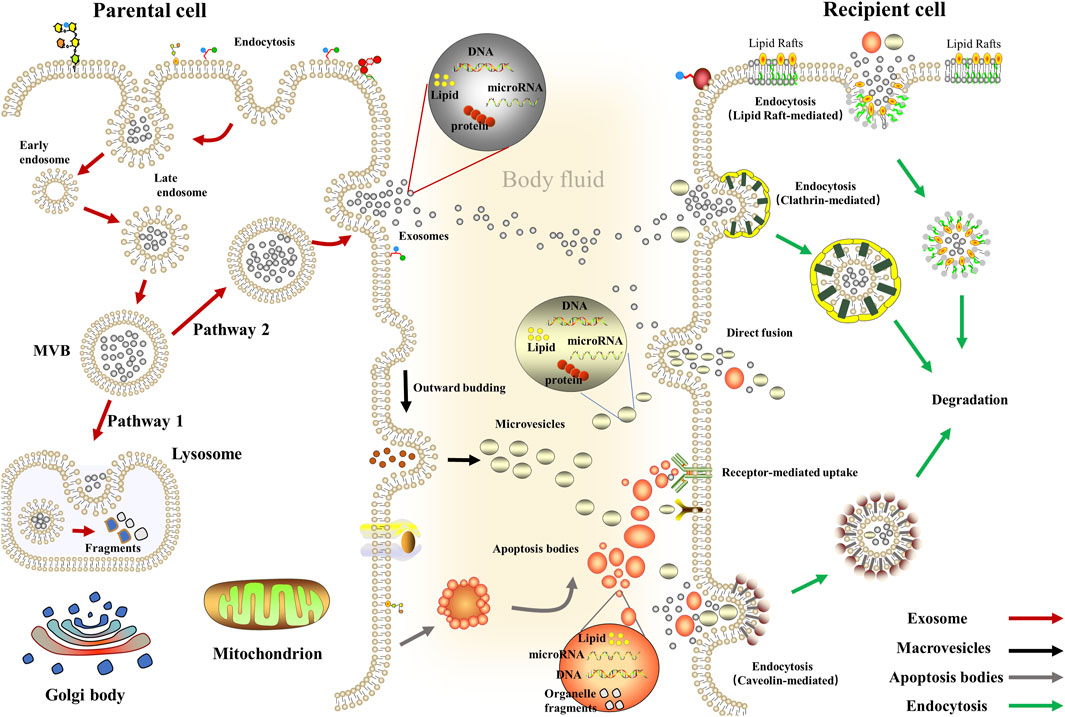
FIGURE 1. Biogenesis and delivery of extracellular vesicles. Exosomes: Inward budding of the early endosomal membrane forms early endosomes and then matures into late endosomes and evolves into MVBs. Then, some MVBs fuse with lysosomes while other MVBs fuses with the plasma membrane to release exosomes (van Niel et al., 2018); Macrovesicles: Actin-myosin interactions promote cytoskeleton contraction, aid cell membranes’ budding, and ultimately form macrovesicles (McConnell et al., 2009); Apoptosis Bodies: The cytosolic membrane is wrinkled and invaginated, dividing and wrapping the cytoplasm, containing DNA material and organelles, forming Apoptosis Bodies (Liu J et al., 2018).
4 Composition and physiological function of extracellular vesicles
Initially, EVs were considered waste products released by cells. Later studies, however, demonstrated that EVs mediate the transmission of intercellular molecular biological information and play a crucial role in intercellular communication (Hessvik and Llorente, 2018; Harmati et al., 2021). Besides, EVs can transport bioactive cargoes, including RNA, DNA, proteins, and lipids (Chen F et al., 2020). Indeed, it both retains many of the biological properties of their parental cells and regulates recipient cells’ physiological or pathological processes (Xing et al., 2021). Of the three types of EVs, exosomes are derived from MVBs, which budding inward from a raft-like domain and, therefore, have a slightly different lipid content than the cell membrane. Exosomes are rich in cholesterol, sphingomyelin, glycerophosphocholine, and phosphatidylcholine (Laulagnier et al., 2004; Raposo and Stoorvogel, 2013; Janas et al., 2015), and membrane surface markers such as integrins, Flotillin 1, CD63, CD81, Alix, TSG101, and cell adhesion molecules (CAMs) (Lotvall et al., 2014). Due to its small size, protective lipid bilayer, and surface receptors, exosome can mediate long-distance, inter-tissue systemic crosstalk (Mathieu et al., 2019; Pelissier Vatter et al., 2021).
The lipid composition and markers of MVs depend mainly on the composition of their cell membrane and also show particular antigens concerning the membrane composition of the original cell. For example, T cell MVs carry CD4 or T cell receptors (Choudhuri et al., 2014). Monocyte-derived MVs show CD14, while CD31, CD34, CD51, CD62E, CD144 and CD146 expressed on endothelium-derived MVs (Koga et al., 2005). Microvesicular cholesterol and hyperphosphatidylserine expose a lipid composition different from that of exosomes. Addition, the shape and density of MVs are irregular as well as the surface does not have any tetrapeptide (Muralidharan-Chari et al., 2010; Choudhuri et al., 2014). These vesicles consist of a lipid bilayer and have mRNAs, miRNAs, biologically active lipids, metabolites, and protein in which surface phospholipid bilayer (Ratajczak and Ratajczak, 2020), it also expresses the number of transmembrane proteins, such as integrins and selectins as in the parental cell membrane (Bobrie et al., 2011; Raposo and Stoorvogel, 2013). However, MVs contain a large number of cytoplasmic and plasma membrane proteins, such as cytoskeletal proteins, heat shock proteins, and protein tetrapeptides that accumulate on the surface of the plasma membrane, which is 100-fold more concentrated in MVs than in cell lysates (Escola et al., 1998; Zoller, 2009; Di Vizio et al., 2012; Morello et al., 2013), and contribute significantly to intercellular communication (Cocucci et al., 2009). On the other hand, the function of MVs depends on the cell types from which they originate (Muralidharan-Chari et al., 2010). For example, immune cell-derived MVs are involved in inflammatory responses, while endothelial cell-derived MVs are related to blood vessels (Morel et al., 2004). In addition to this, the procoagulant properties of MVs were reported for the first time in 1946 by Chargaff and West and later confirmed by Wolf in 1967, who described them as “platelet dust” (Wolf, 1967; Morel et al., 2004).
In recent years, there have been concerns regarding the relationship between EVs and diseases, with numerous studies focusing on cancer (Prieto-Vila et al., 2021), Parkinson’s (D'Anca et al., 2019), infectious diseases (Rodrigues et al., 2018), kidney diseases (Thongboonkerd, 2019), and cardiovascular diseases, resulting in substantial influential outcomes. A great deal of research in cardiovascular disease suggests that EVs play an essential role in cardiac pathophysiology (Njock et al., 2015), and EVs of diverse origins are necessary for maintaining cardiac function and homeostasis via influencing and interacting with each other (Yu and Wang, 2019). These subgroups exhibit a wide range of functions, making them an important source of potential biomarkers for early diagnosis, therapeutic drug delivery systems, or vaccine production systems (Bordanaba-Florit et al., 2021).
5 Mechanism of extracellular vesicles involved in cardiac remodeling after myocardial infarction
Numerous studies have shown that EVs have similar biological properties to parental cells. Interestingly, however, multiple cells produce EVs after MI, including cardiomyocytes, immune cells, ECs, progenitor cells, fibroblasts, and stem cells (Fujita et al., 2019; Berezin and Berezin, 2020). The presence of pro-inflammatory M1 macrophages (M1-MØ) and reparative M2 macrophages (M2- MØ) as well as N1 (Ly6G+CD206-) and N2 (Ly6G+CD206+) neutrophil phenotypes in the infarcted heart mean immune cells exhibit functions and phenotypic heterogeneity (Ma et al., 2016; Peet et al., 2020). N1 neutrophils are pro-inflammatory, with high expression of pro-inflammatory markers, such as TNF-α, IL-1b, macrophage inflammatory protein 1α (CCL3), and interleukin 12a (IL-12a), while anti-inflammatory Cd206 and interleukin 10 (IL-10) are highly expressed in N2 neutrophils. Similarly, monocytes differentiate into pro-inflammatory and anti-inflammatory. EVs of different origins and subtypes also have complex and diverse roles similar to their parental cells and play a dual role in post-infarction cardiac remodeling. These EVs maintain a dynamic balance between pro-and anti-inflammatory, pro-and anti-fibrotic. Thus, it is significant to investigate the involvement of EVs in post-infarction cardiac remodeling, providing new avenues to open up targeted therapeutic strategies.
5.1 Mechanism of extracellular vesicles associated with the initial inflammatory response following myocardial infarction
5.1.1 Mechanism of extracellular vesicles associated with the pro-inflammatory response after myocardial infarction
After MI, the I/R injury contributes to the increased release of various EVs from the heart, which on the one hand, increases the expression of pro-inflammatory cytokines by promoting M1-type polarization of macrophages, on the other hand, these EVs generated by I/R injury stimulate the inflammatory response via transferring miR-155-5p to macrophages activating the JAK2/STAT1 pathway (Ge et al., 2021). Furthermore, a large number of monocytes infiltrate the infarct zone and differentiate into pro-inflammatory macrophages, which generated exosomes containing pro-inflammatory miRNAs. When transported into CFs, these exosomes promote significant expression of inflammatory cytokines, including TNF-α, CCL2, IL-1β, and IL-6 in CFs, thereby exacerbating the inflammatory response in the infarct area (Wang et al., 2017). On the other hand, M1 macrophage-secreted exosomes can also transfer specific miR-155 to ECs, preventing migration and proliferation, affecting angiogenesis and repair, which in turn exert a pro-inflammatory effect (Liu Y et al., 2020).
Beyond that point, as one of the most critical members of the immune cell family, neutrophils play an essential role in this phase. It is well known that neutrophils are professional phagocytes that help to clear necrotic cells. However, recent studies have shown that excessive neutrophil accumulation or delayed clearance of neutrophils is detrimental and can act by secreting EVs (van der Pol et al., 2016). Depending on the production mechanism, neutrophil-derived EVs can be classified into two subtypes: neutrophil-derived MVs (NDMVs) and neutrophil-derived trails (NDTRs). Pro-inflammatory miRNAs are highly expressed in the NDTRs, which are found in tissues where neutrophils migrate while inducing pro-inflammatory macrophage polarization and exacerbating the inflammatory response and cardiac injury (Ma, 2021; Youn et al., 2021). On the other hand, NDMVs can evoke an acute inflammatory response by stimulating ECs to produce inflammatory mediators, including MCP-1 and IL-6 and tissue factor as well as increase microvascular endothelial permeability (Mesri and Altieri, 1999; Ajikumar et al., 2019). Numerous factors influence the increase of neutrophils. Generally, myocardial injury mobilizes neutrophils rapidly from the spleen to the peripheral blood, where they engage in transcriptional activation prior to reaching the damaged area. AMI triggers the transient release of cardiomyocyte-and endothelium-derived EVs. Ly6C + monocytes infiltrating the infarcted heart are stimulated to release chemokines and inflammatory cytokines by these vesicles, exacerbating the local inflammatory response (Loyer et al., 2018). Further studies demonstrated that endothelial cell-derived EVs mediated neutrophil mobilization from the spleen via EC-EV-VCAM-1 after AMI and induced transcriptional activation of blood neutrophils to facilitate miRNA-126-mRNA targeting (Akbar et al., 2022). At the same time, ABs are produced when a substantial number of cells appear apoptotic in infarct zones. However, these ABs do not contribute to the release of intracellular contents and trigger an inflammatory response due to their intact membrane structure, while a large number of necrotic cell contents are secreted into the extracellular area to trigger a dangerous related pattern and trigger a severe inflammatory reaction (Anversa et al., 1998).
Besides this, the current clinical treatment of MI is mainly through percutaneous coronary intervention (PCI). Available studies demonstrate that post-PCI vascular inflammation involves complex interactions between multiple cell types, these cells release proinflammatory cytokines while recruiting monocytes and neutrophils for transendothelial migration to surrounding tissues (Tucker et al., 2021), and EVs, as an important medium of intercellular communication, may play an important role in this process (S et al., 2013). On the other hand, Neutrophils mediate platelet aggregation and thrombus formation, while activating endothelial cells, macrophages and T lymphocytes to release inflammatory cytokines and promote an inflammatory cascade response (Vaidya et al., 2021). However, whether these immune cells release EVs in the process and whether these EVs play a role remains to be explored. Given the current context, future research directions regarding EVs in the regulation of microvascular inflammation after PCI may focus on several directions. Firstly, EVs can be used as potential biomarkers to assess the degree of vascular inflammation; secondly, they may be critical mediators of intercellular communication, broadening the understanding of pathological mechanisms associated with the microvascular inflammation after PCI; thirdly, EVs can be used as drug carriers or targets to alleviate microvascular inflammation after PCI.
5.1.2 Mechanism of extracellular vesicles associated with the anti-inflammatory response following myocardial infarction
Macrophages are functionally and subtypically polarized into the classically activated M1 phenotype and the alternatively activated M2 phenotype. Despite its simplicity, this classification is still more accepted at this stage. M2 phenotype macrophages can mediate inflammation resolution by secreting anti-inflammatory mediators during days 3–5 following MI. Furthermore, it has been shown that the co-culture of the MSCs and macrophages can contribute to sustained high expression of CD206 and IL-10 and low expression of interleukin 12 (IL-12) and TNF-α on the surface of macrophages, resulting in a shift of macrophages towards the M2 phenotype (Chiossone et al., 2016). It has also been shown that mesenchymal stroma-derived exosomes promote macrophage polarization toward the M2 phenotype, which may attenuate the inflammatory cascade response and enhance subsequent repair activity, thereby limiting the infarct size. The mechanism is primarily associated with a large number of miR-182 transported in these exosomes, which regulate the phenotypic transition of macrophages via the TLR4/NF-κB pathway (Zhao J. et al., 2019). These studies suggest that exosomes of different cellular origins that interact with macrophages may be one of the mechanisms by which the myocardium is protected.
NDMVs are involved in the anti-inflammatory response as well as the pro-inflammatory response. These EVs have been demonstrated to have a significant anti-inflammatory effect on interacting cells, mainly by reducing the production of activated cytokines, including IL-1β, TNF-α, IL-6, IL-10, and IL-12 (Byrne and Reen, 2002; Eken et al., 2008; Ren et al., 2008; Eken et al., 2010; Alvarez-Jimenez et al., 2018). Apart from this, neutrophils exert their anti-inflammatory action by secreting EVs, such as NDMVs containing anti-inflammatory miRNAs in foci of inflammation (Youn et al., 2021). At the same time, the administration of neutrophil-derived EVs carrying membrane-linked protein A1 (AnxA1) inhibited inflammation. Overexpression of AnxA1 activated the STAT3 signaling pathway to inhibit neutrophil infiltration during myocardial I/R injury (Dalli et al., 2008; Zhao C. et al., 2019). On the other hand, it has been shown that leukocyte-derived MVs (LMVs) impede the leukocyte inflammatory responses to lipopolysaccharide and stimulate anti-inflammatory cytokine transforming growth factor β1 (TGF-β1) secretion (Gasser and Schifferli, 2004). Specifically, membrane-linked protein 1 (an anti-inflammatory protein) expressed on the surface of these particles exerts this effect (Dalli et al., 2008). In vitro, the particles are taken up by B cells and monocytes, thereby modulating their activation to an anti-inflammatory phenotype. Such phenomenon mediates the immune cells’ anti-inflammatory response in the early phase of acute infarction (Koppler et al., 2006).
Regulatory T (Treg) cells from the thymus or peripheral lymphoid organs are a subtype of T-cells (Richards et al., 2015). Inflammatory cell infiltration and macrophage polarization can be improved through paracrine action and direct contact. It inhibits cardiomyocytic apoptosis and is considered a potential target for reducing the inflammatory response to myocardial I/R injury. Recent studies have indicated that miRNA-181a is associated with immune regulation in cardiovascular disease. Furthermore, Wei et al. showed that miRNA-181a overexpression significantly suppressed inflammatory responses and increased Treg cell ratios by targeting the critical inflammatory transcription factor c-Fos. Additionally, miRNA-181a also attenuated the activation of dendritic cells (DCs) stimulated by oxidized low-density lipoprotein (ox-LDL). It is evident that miRNA-181a delivered by MSC-derived exosomes (MSC-Exos) combines the immunosuppressive effect of miRNA-181a with the cellular targeting ability of MSC-Exos and ultimately exerts a more substantial therapeutic effect on myocardial I/R injury (Wei et al., 2019).
Bone marrow mesenchymal stem cell (BMMSC) transplantation is considered potential therapeutic approach for MI; however, the body’s immune response can contribute to significant early death of transplant cells (Karantalis et al., 2014). Increasing evidence points specifically to the BMMSC-derived exosomes have immunomodulatory, anti-inflammatory effects and can be used as an alternative to stem cell transplantation (Moghaddam et al., 2019). The NF-κB pathway acts as a coordinator in inflammation and inhibiting the NF-κB pathway prevents ventricular remodeling and cardiac rupture in mice with acute myocardial infarction (Pramanik et al., 2018). EVs of BMMSCs can regulate the inflammatory microenvironment via regulating the BCL6/MD2/NF-κB signaling pathway, thereby delivering miR-302d-3p and attenuating the inflammatory response during cardiac remodeling after AMI (Liu et al., 2022). At the same time, it has also been shown that FNDC5 pretreated BMMSCs can secrete more exosomes that reduce the secretion of pro-inflammatory cytokines by macrophages while increasing the secretion of anti-inflammatory cytokines. MSCs-Exo and FNDC5-MSCs-Exo lessen the infiltration of inflammatory cells in ischemic heart tissue and improve the secretion of pro-inflammatory cytokines, thereby improving the inflammatory response in the heart early after myocardial infarction (Ning et al., 2021).
In addition, adipose mesenchymal stem cell-derived exosomes (ADMSC-Exos) exert anti-inflammatory effects by promoting macrophage M2 polarization and activating the S1P/SK1/S1PR1 signaling pathway to improve the inflammatory response in MI (Deng et al., 2019). Adipose stromal cells (ADSCs) can reduce MI severity (Kim et al., 2017), and ADSCs derived exosomes overexpression miR-126 have previously been shown to reduce inflammatory cytokines secreted by damaged cardiomyocytes (Luo et al., 2017). Furthermore, exosomes from miR-93-5p overexpressing ADSCs further targete TLR4 to downregulate the expression of inflammatory cytokines such as IL-6, IL-1β, and TNF-α in hypoxia-treated cardiomyocytes, thereby reducing autophagy-related proteins (LC3II and Atg7) expression and inflammation-related proteins (TLR4 and NF-κB p65) in cardiac tissue and ultimately alleviated inflammatory response (Liu D et al., 2018; Pan W et al., 2019).
At the same time, further work in this field of research contributes to paramount observations or considerations to pre-clinical research using tissue engineering or matrices carrying multifunctional MSC-EVs as an alternative therapeutic approach with associated anti-inflammatory or fibrotic benefits. EVs from porcine cardiac adipose tissue-derived MSC (cATMSC) combined with biocompatible cardiac scaffolds are capable of effective delivery in post-infarct myocardial tissue, decreasing expression of inflammatory TNF-α, increasing expression of IL-1ra, blocking IL-1α/β inflammatory effects and inducing anti-inflammatory IL-10 expression, which in turn attenuates the local inflammatory response in the myocardium. Meanwhile, the reduction of inflammatory mediators modulates the expression and activity of TGF-β, metallopeptidases, and other pro-fibrotic mediators, thereby reducing collagen synthesis and deposition and myocardial vascularization and fibrosis (Monguio-Tortajada et al., 2021; Monguio-Tortajada et al., 2022). Yet producing effective formulations of EVs and meeting the requirements for standardized clinical-grade biomanufacturing and regulatory, large-scale, GMP-compliant issues for EV production and clinical application remain a huge challenge (Courageux et al., 2022; Soler-Botija et al., 2022).
5.2 Mechanism of extracellular vesicles involved in scar repair after myocardial infarction
5.2.1 Mechanism of extracellular vesicles involved in promoting scar repair
CFs are the primary effector cells in the scar repair phase of myocardial fibrosis, which transdifferentiate to MFBs in response to different stimuli, secrete collagen, and are the primary source of ECM. The scar repair phase involves several processes such as pro-angiogenesis and pro-differentiation of CFs into MFBs. CircUbe3a from M2 macrophage-derived exosomes promotes the proliferation or migration of CFs, and transdifferentiation via the miR-138-5p/RhoC axis, thereby mediating post-infarction myocardial fibrotic repair (Wang Y. et al., 2021). On the other hand, EVs generated from cardiosphere-derived cells (CDC-EVs) can polarize M1-MØ to a proangiogenic phenotype dependent on upward regulation of arginase to contribute to scar repair (Mentkowski et al., 2020). Moreover, several studies suggested that the expansion and activation of CD4+ T-cells in the heart associated with post-infarction remodeling. Exosomes generated from activated CD4+ T-cells carrying miR-142-3p, which mediates local MFBs activation through the miR-142-3p-WNT signaling cascade, secreting TGF-β, and regulating the APC-GSK-β-linked protein signaling cascade to confer a profibrotic effect. Aggravates post-ischemic myocardial fibrosis (Cai et al., 2020). Though EVs affect the post-infarction microenvironment by transporting miRNAs, cardiovascular risk factors, including sex, age, genetics, smoking, overweight, and obesity, affect apoptosis and activation of the mother cells of EVs, which in turn affect the physicochemical properties of these vesicles. Thus, most miRNAs are transported by cell-free and EV-free.
Cardiomyocyte-derived exosomes facilitate the formation of myocardial fibrosis via cardiomyocyte-fibroblast interactions. Previous study demonstrated that cardiomyocyte-derived exosomes enriched with miR-208a can promote the proliferation and differentiation of fibroblast toward MFBs (Yang et al., 2018). The molecular analysis then showed that miR-208a blocked the expression of Dyrk (bispecific tyrosine phosphorylation kinase), thereby upregulating fibrotic gene expression via the nuclear translocation of NFAT. Additionally, miR-217 was enriched in exosomes derived from cardiomyocyte and enhanced fibroblast activity by targeting the PTEN signaling cascade under pressure overload (Nie et al., 2018). At the same time, WNT3a in exosomes containing WNT protein can effectively trigger WNT/β-catenin signaling. In the presence of TGF-β, WNT3a can effectively enhance the pro-fibrotic response of human CFs (Dzialo et al., 2019). In addition, human umbilical cord mesenchymal stem cell-derived exosomes (hUCMSC-Exos) can promote CFs into FMBs and secrete collagen into inflammatory environment, thereby promoting cardiac fibrotic scar repair (Shi et al., 2019).
During the fibrotic repair phase of post-myocardial infarction, the precursors of ECs such as late endothelial progenitor cells (EPCs) can repair the heart through paracrine mechanisms. Several studies have demonstrated that human endothelial histocyte-derived exosomes enhance the proliferation and angiogenesis of CFs in vitro (Ke et al., 2017). In addition, cardiac mesenchymal stem cells (C-MSCs), a novel subpopulation of MSCs derived from cardiac tissue, have been shown to play an irreplaceable role in cardiac regeneration. Notch signaling is thought to contribute to cardiac repair after myocardial injury. EVs derived from Notch1 overexpressed C-MSCs are essential in promoting angiogenesis and cardiac fibroblast proliferation, preventing cell death, and facilitating myocardial fibrosis repair (Xuan et al., 2020).
Furthermore, in a MI model, it has been demonstrated that ABs released by transplanted MSCs can enhance angiogenesis and improve recovery of cardiac function by regulating macroautophagy/autophagy in recipient ECs. BMMSCs undergo extensive apoptosis immediately after transplantation and release ABs phagocytosed by recipient ECs. Then, these ABs activate lysosomal function and promote the expression of TFEB (transcription factor EB), the master gene for lysosomal biogenesis and autophagy in ECs. Notably, TFEB is involved in the expression of autophagy-related genes in ECs and boosts angiogenesis and recovery of cardiac function after MI (Liu H et al., 2020). It has been shown that myocardial endocytic target and silence CDIP1(cell death involved p53 target 1) gene via exosomal miRNA-21-5p, thereby downregulating activated caspase-3, which in turn inhibits apoptosis of ECs under ischemic and hypoxic conditions and facilitates post-MI angiogenesis and regeneration, which in turn involved in post-infarction scar repair (Liao et al., 2021).
5.2.2 Mechanism of extracellular vesicles involved in the anti-scar repair
Scar repair is essential for maintaining cardiac structure in the early stage of MI, which involves angiogenesis and MFBs activation. Fibrotic scar repair is a double-edged sword, inadequate repair can contribute to cardiac rupture, and excessive and sustained fibrotic repair can lead to ventricular systolic-diastolic dysfunction and ultimately left heart failure (Ma et al., 2013; Ma et al., 2017). Harmonizing the dynamic balance of pro-and anti-fibrosis is crucial for post-infarction cardiac remodeling.
BMMSC-derived M1 macrophage exosomes inhibit angiogenesis and myocardial regeneration following MI by activating the MALAT1/miR-25-3p/CDC42 signaling pathway as well as MEK/ERK axis, thereby impeding scar repair (Chen et al., 2021). These EV-loaded miRNAs are significant for the pathological process of fibrosis in addition to their involvement in the early anti-inflammatory response to cardiac remodeling. Previous studies have indicated that MSCs derived EVs containing miR-212-5p may ameliorate myocardial fibrosis after MI by inhibiting the NLRC5/VEGF/TGF-β1/SMAD signaling pathway, as evidenced by reducing the expression of TGF-β1, collagen type I (Col I), and α-smooth muscle actin (α-SMA) (Wu et al., 2022). At the same time, MSC-derived extracellular vesicles (MSC-EVs) were also able to inhibit hypoxia-mediated cardiac fibroblast activation, in turn, exerting antifibrotic effects (Chen et al., 2014), secondly, these vesicles could be internalized by cardiac stem cells. Treatment of CSCs with MSC-Exo in an AMI model enhanced cell proliferation implantation and capillary density and reduced fibrotic area (Zhang et al., 2016). On the other hand, miR-199a-3p in exosomes released by BMMSCs targeted to the region of cardiac injury inhibited mTOR activation in myocardial tissue and subsequently activated induction of autophagy. Excessive autophagy eliminated the fibrotic fraction in the heart and attenuated tissue damage (Fan C et al., 2022). Concurrently, these exosomes can promote premature senescence of MFBs in vitro and enhance microvascular regeneration under stress, and ultimately improve cardiac function via modulating platelet-derived growth factor receptor-β (PDGFR-β) (Chen J et al., 2020; Wang et al., 2021a). Adipose-derived mesenchymal stem cells secreted miR-671-containing exosomes that attenuated myocardial fibrosis by inhibiting the TGFBR2/Smad2 signaling pathway (Wang et al., 2021b).
Cardiovascular progenitor cells (CVPCs) derived from human pluripotent stem cells are a promising source of myocardial repair with EVs mediating intercellular communication. Intramyocardial injection of HCVPC EVs into AMI mice on day 28 after MI significantly improved cardiac function and attenuated fibrosis while improving vascularization and cardiomyocyte survival in the marginal zone (Wu et al., 2020). In addition, it has been shown that ISX-9 is a small molecule with prosurvival, antioxidant, and regenerative properties, and that induction of CPCs by ISX-9 is an attractive cell-based cardiac regeneration therapy, and that these EVs contain a unique set of bioactive miRNAs, among which miR-373 has anti-fibrotic substantial effects. These new discoveries have important implications for preventing post-myocardial infarction remodeling (Xuan et al., 2019).
In preclinical studies, EVs derived from Cardiosphere-derived cells (CDCs) facilitated myogenesis and angiogenesis, ameliorated fibrosis, modulated immune responses, and generally improved cardiac function (Marban, 2018). MSCs mediate their disease-modifying biological activity by secreting paracrine factors, including EVs. Furthermore, like CDC-EVs, MSC-EVs contain a plethora of RNA species, like miRNAs. For instance, MSC-EVs from bone marrow-derived MSCs are enriched in miR-22 and have anti-fibrotic and anti-apoptotic properties in a mouse model of AMI (Feng et al., 2014). This demonstrates a significate role in regulating fibrotic repair after infarction by intervening in extracellular vesicle-loading cargos (Figure 2).
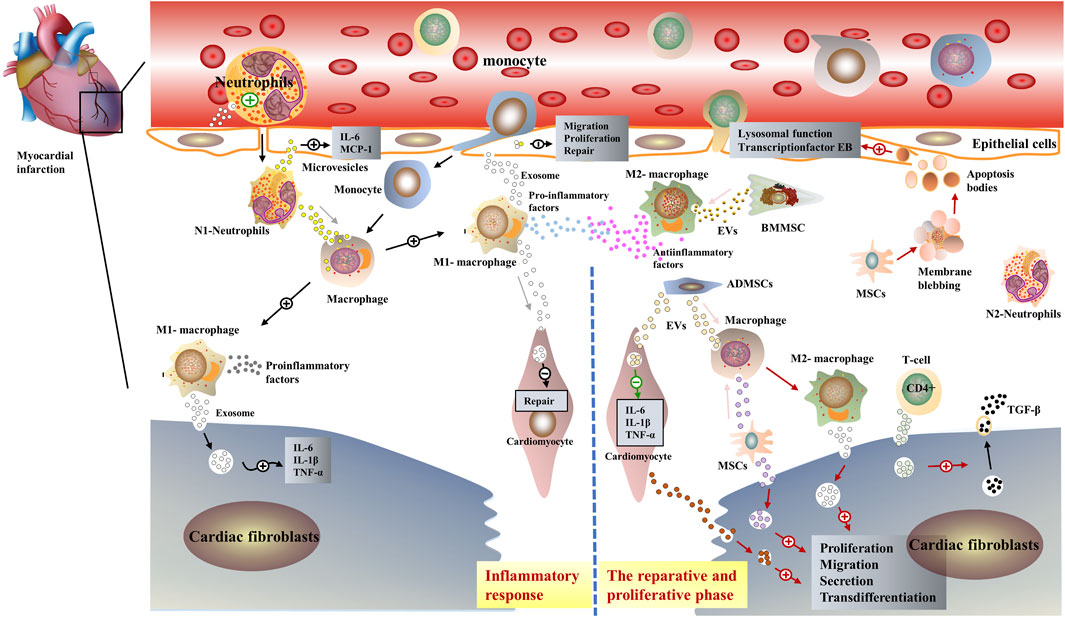
FIGURE 2. Schematic representation of the role of extracellular vesicles in post-infarction cardiac remodeling. EVs have similar biological properties to parental cells, in the early inflammatory phase post-infarction, immune cells such as pro-inflammatory M1-MØ and neutrophils, secret exosomes containing pro-inflammatory miRNAs. Interaction with myocardial fibroblasts and endothelial cells exacerbates the inflammatory response in the infarcted area (Xiong et al., 2021). Subsequently, anti-inflammatory M2-MØ and immune cell-derived exosomes such as neutrophils dominate, interacting with mesenchymal stem cells as well as cardiomyocytes to exert anti-inflammatory effects (Wu et al., 2019).
6 Therapeutic strategies for targeting extracellular vesicles in regulating cardiac remodeling after myocardial infarction
6.1 Engineered extracellular vesicle-based therapeutics
Although native EVs make great achievements in preclinical research, realizing the precisely controllable release of these cargos for the target regions remains a challenge (Fan Z et al., 2022). Accordingly, to enhance the ability of EVs to load cargoes, improve the controllability and precision of vesicular cargoes released in the infarct region, further attenuate inflammation and maintain the homeostasis of fibrotic scar repair following MI, researchers distill experiences and inspirations from nano materials-based drug delivery research and engineer modification of extracellular vesicles, including bioengineering, chemical engineering, and physical engineering (Man et al., 2020; Nasiri Kenari et al., 2020; Wu et al., 2021). Targeted manipulation of the extracellular vesicles’ genes and proteins is an essential part of bioengineering, which can direct EVs to a specific tissue or organ and increase their utilization. For instance, EVs derived from cardiosphere-derived stem cells are labeled by infarcted heart targeting peptide, so-called “cardiac-homing peptide”, the vesicles recruit to the infarct area and improve cardiac function by attenuating cardiac fibrosis, inducing angiogenesis, and promoting cardiomyocyte proliferation (Vandergriff et al., 2018). Meanwhile, there are also several studies demonstrated that HEK 293 cells transfecting with vectors encoding CTP-Lamp2b can derive exosomes with cardiac-targeting peptide (CTP)-Lamp2b on their membrane (CTP-Exo) (Kim et al., 2018), these vesicles can transport curcumin directly to the heart and improve cardiac function by regulating the PTEN/Akt/Bax signaling pathway associated with cardiomyocyte apoptosis via upregulating miR-144-3p (Kang et al., 2021). Furthermore, MSCs-derived exosomes modified with ischemic myocardium-targeting peptide CSTSMLKAC (IMTP) can specifically target ischemic myocardium, attenuating inflammation and apoptosis, promoting angiogenesis, and restoring cardiac function (Wang et al., 2018). On the other hand, Tian et al. found that exosomes (Hypo-Exo) derived from bone marrow mesenchymal stem cells (BMMSCs) under hypoxic conditions have excellent protections against ischemic diseases. The combination of Hypo-Exo with “CSTSMLKAC” peptide, an ischemic myocardial targeting peptide, significantly reduced apoptosis after MI via targeting Exo in ischemic heart regions, suggesting that IMT-Exo could be a novel drug carrier to enhance the specificity of drug delivery in ischemic diseases (Zhu et al., 2018). These findings demonstrated that the delivery of various cargoes in cardiac-targeted peptide-modified exosomes may be a promising strategy for MI treatment.
Alginate hydrogel possesses favorable biocompatibility and has been widely applied in tissue engineering and cellular engineering (Ruvinov and Cohen, 2016). Researchers have constructed an injectable conductive hydrogel to bind exosomes derived from human umbilical cord mesenchymal stem cells and injecting the hydrogel into injured rat hearts effectively prolongs the retention time of exosomes in the ischemic myocardium (Zou et al., 2021). Dendritic cell-derived exosomes (DEX) can activate Treg cells (Sakaguchi, 2004), and contribute to an early shift of macrophage subsets from an inflammatory M1 phenotype towards a reparative M2 phenotype after MI, thereby improving the immune microenvironment in the infarct area and offering the possibility of improving post-infarcted cardiac function. However, they have a short retention time and the therapeutic effects are transient. Accordingly, Researchers have developed a new drug delivery system that contains a sodium alginate hydrogel that continuously releases DEX, an approach that significantly increases therapeutic efficacy (Zhang et al., 2021). In addition, some investigators have also found that by combining the advantages of natural EVs and novel nanomaterials, engineered vesicles with biofilm/synthetic material chimeras were successfully constructed to enhance further the targeting and loading of natural vesicles with therapeutic molecules. Jin et al. found that engineered vesicles can effectively load microRNA-21 or curcumin for targeted delivery to achieve precise release of therapeutic molecules in macrophages, modulate the phenotypic transformation of macrophages, and effectively control inflammation levels (Andaloussi et al., 2013) (Table 1).
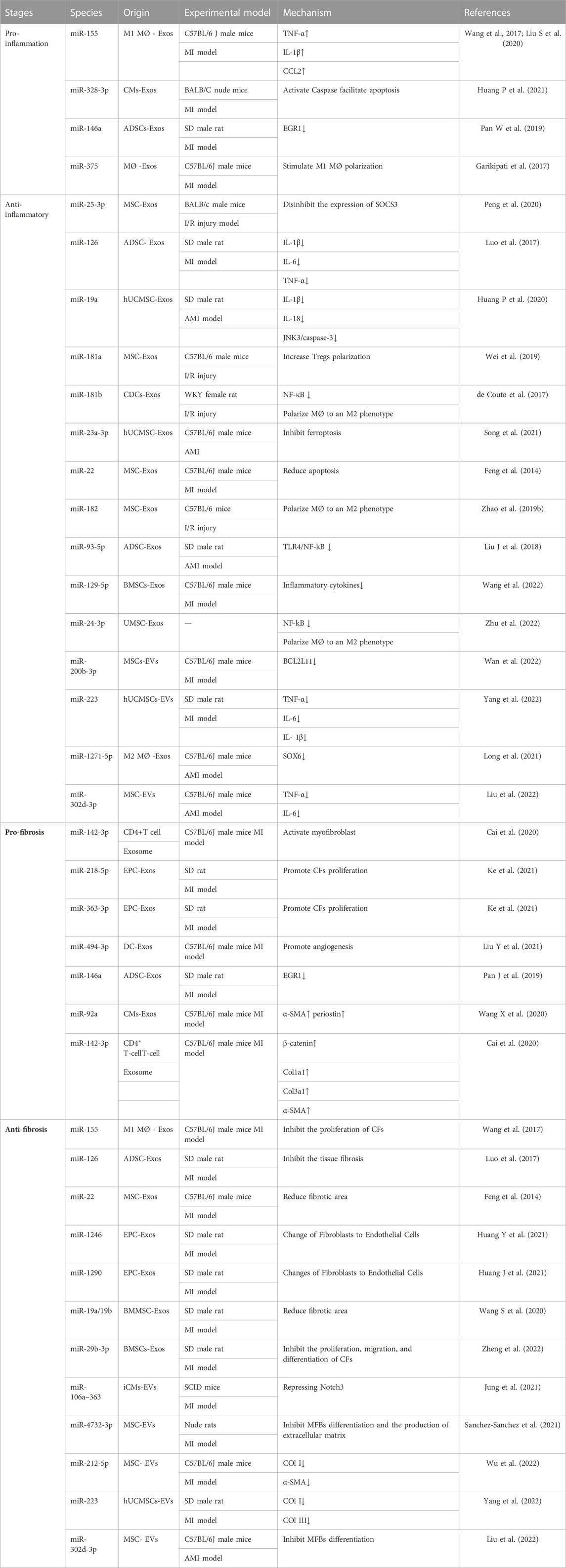
TABLE 1. Specific application and mechanism of miRNAs carried by EVs in post-infarction cardiac remodeling.
6.2 Drug pretreatment of extracellular vesicles to enhance its therapeutic effect
In addition to the engineered modification of EVs, several types of research focus on the drug regulation of EVs and make several significant findings.
Numerous experimental and clinical studies demonstrate that myocardium is protected from ischemic left ventricular remodeling by long-term antagonisty of the purinergic GPCR P2Y12 (Roubille et al., 2012; Nanhwan et al., 2014; Vilahur et al., 2016; Bansilal et al., 2018). Ticagrelor is a selective and reversible P2Y12 receptor antagonist that induces exosome release from CPCs, resulting in the release of anti-apoptotic HSP70. Simultaneous continuous pretreatment of cardiomyocytes with HCPC derived exosomes exposed to low-dose of ticagrelor attenuates hypoxia-induced apoptosis via acute phosphorylation and activation of ERK42/44, resulting in myocardial protection effect (Casieri et al., 2020). This research has clinical implications for enhancing the endogenous exosomal anti-hypoxic response and prevent the heart from ischemic injury by developing new non-invasive pharmacological approaches. In addition, atorvastatin pretreatment has been demonstrated to enhance the function of BMMSC-derived exosomes in angiogenesis, cardiomyocyte protection, and long non-coding RNA H19 (lncRNA H19) is a mediator of regulating miR-675 expression and promoting atorvastatin pretreatment MSC-Exo (MSCATV-Exo) effect in angiogenesis. Importantly, lncRNA H19 and its downstream signaling pathways mediate the cardioprotective effects of MSCATV-Exo (Huang L et al., 2020). Other studies have shown that sulpiride/valsartan can improve cardiac function and ameliorate myocardial fibrosis by downregulating miR-181a in exosomes in a rodent chronic MI model (Vaskova et al., 2020).
TCM has been practiced and developed for thousands of years. In recent decades, considerable progress and achievements have been made in studying the mechanism of TCM using modern molecular biology techniques (Zhou et al., 2016). One of the hotspots is the research on the targeting and regulation of EVs using TCM, such as the regulation of stem cells and immune cells. Stem cell transplantation is a promising therapeutic alternative to facilitate myocardial repair following MI, however, its clinical application is limited by the low preservation and survival rates of implanted cells (Feyen et al., 2016). In recent years, new strategies have been developed, including combined cell therapy with BMMSC exosomes, which have been demonstrated that such strategies have anti-apoptotic, anti-inflammatory, and pro-angiogenic effects. Transplantation of exogenous vesicles into the ischemic heart within 30 min of MI significantly modulates the ischemic environment, including decreasing inflammatory IL-6 and TNF-α, enhancing SDF-1 expression and MSC survival. Additionally, several studies have demonstrated that combined pretreatment with hypoxia and the herbal compound Tongxinluo can effectively achieve better performance in facilitating cardiac repair through increasing CXCR4 expression, which provides new insights into stem cell therapy for cardiac rehabilitation (Xiong et al., 2022). Furthermore, Ruan et al. suggested that Suxiao Jiuxin pills modulate myocardial MSC-Exos, causing structural genetic chromatin remodeling in recipient cardiomyocytes and accelerating cardiomyocyte propagation (Ruan et al., 2018). Accordingly, researchers rolled out several studies to enhance EVs targeting, thus making them better delivery vehicles (Veerman et al., 2019).
7 Clinical applications and regulation of extracellular vesicles
Clinical applications of EVs are novel therapeutic modalities, including vaccines, diagnostic criteria, and drug delivery. The idea of using EVs as anti-tumor vaccines originated in the last century. Basic studies suggested that DEX can promote T-cell dependent antitumor effect, and phase I clinical trials (to vaccinate peptide-pulses DEX for patients with cancer) demonstrated that the feasibility and safety of inoculation of DEX (Escudier et al., 2005; Morse et al., 2005). Immediately after, Sophie et al. developed a second-generation DEX with enhanced immunostimulatory properties and produced large-scale IFN-γ-DEX vaccines while conducting phase II clinical trials (NCT01159288) under the guidance of Institut Gustave Roussy in France, in which DEX was administered to patients with non-small cell lung cancer with the aim of increasing progression-free survival (Viaud et al., 2011; Lener et al., 2015). In respiratory diseases, preclinical studies have established that MSC-Exos can ameliorate most of the pathological changes caused by lung infections. And a clinical study (NCT04602104) for treating acute respiratory distress syndrome (ARDS) with MSC-Exos Nebulizer is currently recruiting patients, this study explores a new approach to treat ARDS and assesses its safety by administering human mesenchymal stem cell exosomes (hMSC-Exos) aerosol inhalation to patients. It has also been shown that ExoFlo™, derived from allogeneic bone marrow mesenchymal stem cells, can reconstitute immunity, downregulate cytokine storm and restore oxygenation in patients with severe COVID-19, demonstrating the promise of ExoFlo for the treatment of COVID-19 (Sengupta et al., 2020). In renal disease, intra-arterial and intravenous administration of MSC-Exos to patients with grade III-IV chronic kidney disease in phase II/III clinical trials was shown to ameliorate inflammation and renal function. No adverse events associated with MSC-Exos administration were observed in subjects during the 1-year follow-up period (Nassar et al., 2016; Guo et al., 2020). In cardiovascular system diseases, a phase II randomized clinical trial of intravenous ischemia-tolerant MSCs (itMSCs) in patients with non-ischemic cardiomyopathy showed that single-dose of intravenous itMSCs was safe and well tolerated compared with controls, increasing the patient’s 6-min walk distance while eliciting systemic immunomodulatory effects associated with improved LVEF (Butler et al., 2017). Examples of these clinical trials showed the great therapeutic potential of EVs.
The complex properties of EVs make their specification and characterization difficult. Thus, in addition to controlling the final product by specification and characterization, the quality of EVs must be ensured by quality control of the raw material and regulation of the manufacturing process (Tsuchiya et al., 2022). EV manufacturing must be performed in accordance with Good Practice (GxP) regulations, which are a collection of quality guidelines and regulations designed to ensure that biological/medical products are safe, meet their intended use and follow quality processes in manufacturing, control, storage and distribution (Good Manufacturing, Good Laboratory, Good Distribution, Good Clinical, Good Scientific Practice) (Soekmadji et al., 2020). The biological activity of EV used as a therapeutic agent must be tested in a qualified bioassay, called a “potency assay" (Lener et al., 2015). EVs are subject to detailed guidelines for the clinical application and manufacture of novel biomedical products in the EU. In Australia, the government’s Therapeutic Goods Administration (TGA) office provides rules and guidelines related to the manufacture and use of therapeutic agents often adopted in EU rules. In the United States, EV-based therapeutics for human use are regulated by the Center for Biologics Evaluation and Research (CBER) within the Food and Drug Administration (FDA) (Lener et al., 2015). (Figure 3).
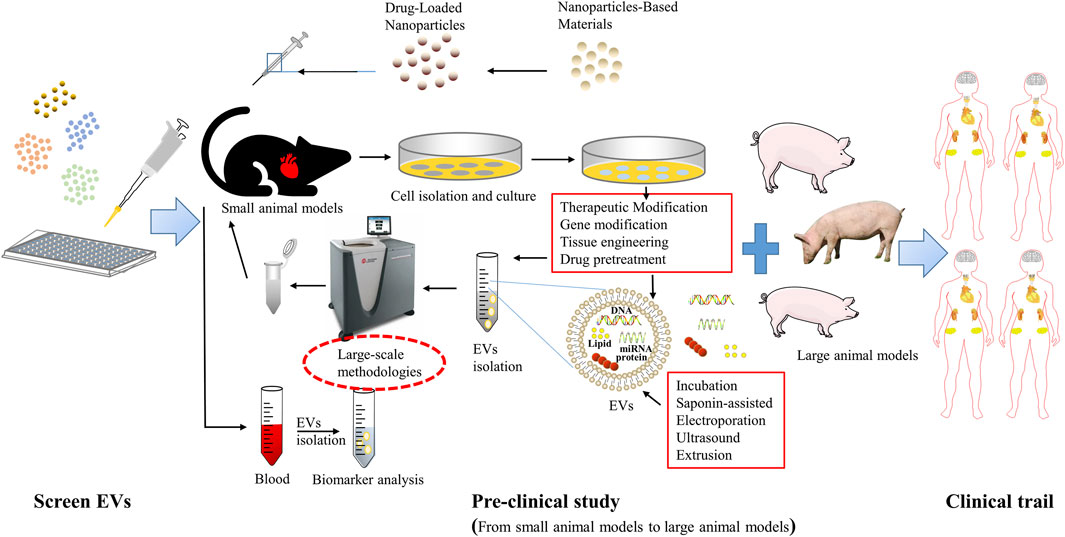
FIGURE 3. Extracellular vesicle-based therapeutics toward clinical application. The first is a basic study to identify potential EVs, followed by preclinical studies, including small animal in vivo trials and large animal in vivo trials, as well as researches to evaluate the manufacturing process, quality control, and stability (CMC); and finally a phase III randomized clinical trial to further evaluate its safety and efficacy.
8 Conclusion
Post-infarction cardiac remodeling is a complex and diverse pathological process consisting of two main phases: inflammatory response and fibrotic scar repair. Sudden and substantial cardiomyocyte death triggers a vigorous inflammatory response and subsequently activated MFBs, which secrete collagen, leading to myocardial fibrosis and consequent scar formation. Moderate myocardial fibrosis plays a critical protective role, maintaining the structural integrity of the chambers and preventing cardiac rupture, while persistent myocardial fibrosis can lead to cardiac stiffness and diastolic dysfunction, eventually causing HF. The search for new measures and approaches that can attenuate the post-infarction inflammatory response as well as maintain the dynamic balance of fibrosis repair is particularly critical. EVs of different cellular origins play a dual role in the early post-infarction inflammatory response and the subsequent repair of fibrotic scars. During the early inflammatory phase, these vesicles are involved in both pro-inflammatory and anti-inflammatory responses, and therefore, by achieving effective control of the release of pro-inflammatory vesicles and the delivery of their cargoes is of great importance to contain the early adverse persistent inflammatory response after infarction. At the same time, these vesicles play a dual role of pro-fibrotic and anti-fibrotic effects in the subsequent fibrotic scar repair phase, a property that also shows the importance of maintaining the dynamic balance of post-infarction fibrotic repair by modulating EVs. Currently, research on targeted regulation of EVs in cardiovascular diseases has focused on drug pretreatment as well as engineered modifications.
Research associated with the intercellular delivery of functional nucleic acids and proteins by EVs has demonstrated their advantages as cargo carriers, such as low immunogenicity, high stability and biocompatibility, long cycle life, and ability to cross the blood-brain barrier. These properties make EVs an important endogenous carrier for delivering therapeutic drugs, however, the current research on extracellular vesicle drug delivery is mainly focused on cancer and neurological related diseases, while preclinical studies on cardiovascular diseases are rare. The reasons for this are limited by the isolation of endogenous vesicles, storage, mass production techniques, low efficiency of targeted drug delivery and cellular uptake, on the one hand, and the unknown biological distribution of drug-loaded vesicles in the individuals treated with them, on the other hand, which may lead to difficult challenges in managing the use of exosomes in standardized methods related to their isolation, quantification and outcome analysis. Currently, EVs were isolated by Polymer precipitation, Differential ultracentrifugation, Field-flow fractionation, and Gradient density ultracentrifugation. These methods always lacking in yield and purity. Distinct purification methods of EVs exist in relation to the physical or molecular characteristics of isolated EVs preparations and affect the results of downstream analysis. The best separation strategy for EVs is by applying different methods of isolation in order to achieve a desirable recovery and purity and report in detail about standardized approaches for isolation, this research is particularly important, especially in the context of promising clinical applications of EVs(Clos-Sansalvador et al., 2022). Second, drug loading cannot disrupt the membrane structure and content of EVs, and finding effective ways to load therapeutic drugs into these EVs remains a great challenge. Although, the use of EVs as drug delivery carriers is still in the developmental stage. However, with a deeper understanding of the physiological properties of EVs, improved isolation and drug delivery techniques for EVs, the understanding and conclusion of exosome-loaded drugs in other diseases (cancer, hepatitis, Parkinson’s disease, etc.) will help develop or expand future therapeutic approaches for post-infarction cardiac remodeling, mitigate the inflammatory response during post-infarction cardiac remodeling, precisely regulate the dynamic balance between pro- and anti-fibrosis, and ultimately the transition from basic research to clinical applications.
Author contributions
PC: Writing original draft and designing the figures. XW and QL: Revising the manuscript and visualization. TY: Revising the manuscript and providing good ideas. HZ and HQ: Conceiving and designing this review.
Funding
This work was supported by the Three-year Action Plan of Shanghai Shenkang Medical Development Center (grant no. SHDC2020CR1053B, SHDC2020CR6012-003); the Shanghai Municipal Health and Family Planning Commission (grant no. ZY-(2018–2020)-CCCX-2003); the Shanghai Medical Leading Talents Program (grant no. 0462015); the Shanghai Shenkang Medical Development Center Emerging Frontier Technology Joint Research Project (grant no. SHDC12018125); the Science and Technology Support Project of Shanghai Science and Technology Commission (grant nos. 20S21901800 and 18401932800).
Conflict of interest
The authors declare that the research was conducted in the absence of any commercial or financial relationships that could be construed as a potential conflict of interest.
The reviewer HG declared a shared affiliation, with the authors to the handling editor at the time of the review.
Publisher’s note
All claims expressed in this article are solely those of the authors and do not necessarily represent those of their affiliated organizations, or those of the publisher, the editors and the reviewers. Any product that may be evaluated in this article, or claim that may be made by its manufacturer, is not guaranteed or endorsed by the publisher.
References
Admyre, C., Johansson, S. M., Qazi, K. R., Filen, J. J., Lahesmaa, R., Norman, M., et al. (2007). Exosomes with immune modulatory features are present in human breast milk. J. Immunol. 179 (3), 1969–1978. doi:10.4049/jimmunol.179.3.1969
Ajikumar, A., Long, M. B., Heath, P. R., Wharton, S. B., Ince, P. G., Ridger, V. C., et al. (2019). Neutrophil-derived microvesicle induced dysfunction of brain microvascular endothelial cells in vitro. Int. J. Mol. Sci. 20 (20), 5227. doi:10.3390/ijms20205227
Akbar, N., Braithwaite, A. T., Corr, E. M., Koelwyn, G. J., van Solingen, C., Cochain, C., et al. (2022). Rapid neutrophil mobilisation by VCAM-1+ endothelial extracellular vesicles. Cardiovasc Res. 2022, cvac012. doi:10.1093/cvr/cvac012
Alvarez-Jimenez, V. D., Leyva-Paredes, K., Garcia-Martinez, M., Vazquez-Flores, L., Garcia-Paredes, V. G., Campillo-Navarro, M., et al. (2018). Extracellular vesicles released from Mycobacterium tuberculosis-infected neutrophils promote macrophage autophagy and decrease intracellular mycobacterial survival. Front. Immunol. 9, 272. doi:10.3389/fimmu.2018.00272
Amosse, J., Martinez, M. C., and Le Lay, S. (2017). Extracellular vesicles and cardiovascular disease therapy. Stem Cell Investig. 4, 102. doi:10.21037/sci.2017.11.07
Andaloussi, S. E. L., Mager, I., Breakefield, X. O., and Wood, M. J. (2013). Extracellular vesicles: Biology and emerging therapeutic opportunities. Nat. Rev. Drug Discov. 12 (5), 347–357. doi:10.1038/nrd3978
Andreadou, I., Cabrera-Fuentes, H. A., Devaux, Y., Frangogiannis, N. G., Frantz, S., Guzik, T., et al. (2019). Immune cells as targets for cardioprotection: New players and novel therapeutic opportunities. Cardiovasc Res. 115 (7), 1117–1130. doi:10.1093/cvr/cvz050
Anversa, P., Cheng, W., Liu, Y., Leri, A., Redaelli, G., and Kajstura, J. (1998). Apoptosis and myocardial infarction. Basic Res. Cardiol. 93, 8–12. doi:10.1007/s003950050195
Assil, S., Webster, B., and Dreux, M. (2015). Regulation of the host antiviral state by intercellular communications. Viruses 7 (8), 4707–4733. doi:10.3390/v7082840
Bansilal, S., Bonaca, M. P., Cornel, J. H., Storey, R. F., Bhatt, D. L., Steg, P. G., et al. (2018). Ticagrelor for secondary prevention of atherothrombotic events in patients with multivessel coronary disease. J. Am. Coll. Cardiol. 71 (5), 489–496. doi:10.1016/j.jacc.2017.11.050
Barres, C., Blanc, L., Bette-Bobillo, P., Andre, S., Mamoun, R., Gabius, H. J., et al. (2010). Galectin-5 is bound onto the surface of rat reticulocyte exosomes and modulates vesicle uptake by macrophages. Blood 115 (3), 696–705. doi:10.1182/blood-2009-07-231449
Berezin, A. E., and Berezin, A. A. (2020). Extracellular endothelial cell-derived vesicles: Emerging role in cardiac and vascular remodeling in heart failure. Front. Cardiovasc Med. 7, 47. doi:10.3389/fcvm.2020.00047
Bobrie, A., Colombo, M., Raposo, G., and Thery, C. (2011). Exosome secretion: Molecular mechanisms and roles in immune responses. Traffic 12 (12), 1659–1668. doi:10.1111/j.1600-0854.2011.01225.x
Bordanaba-Florit, G., Royo, F., Kruglik, S. G., and Falcon-Perez, J. M. (2021). Using single-vesicle technologies to unravel the heterogeneity of extracellular vesicles. Nat. Protoc. 16 (7), 3163–3185. doi:10.1038/s41596-021-00551-z
Borges, F. T., Reis, L. A., and Schor, N. (2013). Extracellular vesicles: Structure, function, and potential clinical uses in renal diseases. Braz J. Med. Biol. Res. 46 (10), 824–830. doi:10.1590/1414-431X20132964
Bretherton, R., Bugg, D., Olszewski, E., and Davis, J. (2020). Regulators of cardiac fibroblast cell state. Matrix Biol. 91-92, 117–135. doi:10.1016/j.matbio.2020.04.002
Butler, J., Epstein, S. E., Greene, S. J., Quyyumi, A. A., Sikora, S., Kim, R. J., et al. (2017). Intravenous allogeneic mesenchymal stem cells for nonischemic cardiomyopathy: Safety and efficacy results of a phase II-A randomized trial. Circ. Res. 120 (2), 332–340. doi:10.1161/CIRCRESAHA.116.309717
Byrne, A., and Reen, D. J. (2002). Lipopolysaccharide induces rapid production of IL-10 by monocytes in the presence of apoptotic neutrophils. J. Immunol. 168 (4), 1968–1977. doi:10.4049/jimmunol.168.4.1968
Caby, M. P., Lankar, D., Vincendeau-Scherrer, C., Raposo, G., and Bonnerot, C. (2005). Exosomal-like vesicles are present in human blood plasma. Int. Immunol. 17 (7), 879–887. doi:10.1093/intimm/dxh267
Cai, L., Chao, G., Li, W., Zhu, J., Li, F., Qi, B., et al. (2020). Activated CD4(+) T cells-derived exosomal miR-142-3p boosts post-ischemic ventricular remodeling by activating myofibroblast. Aging (Albany NY) 12 (8), 7380–7396. doi:10.18632/aging.103084
Casieri, V., Matteucci, M., Pasanisi, E. M., Papa, A., Barile, L., Fritsche-Danielson, R., et al. (2020). Ticagrelor enhances release of anti-hypoxic cardiac progenitor cell-derived exosomes through increasing cell proliferation in vitro. Sci. Rep. 10 (1), 2494. doi:10.1038/s41598-020-59225-7
Chen, B., Luo, L., Wei, X., Gong, D., Li, Z., Li, S., et al. (2021). M1 bone marrow-derived macrophage-derived extracellular vesicles inhibit angiogenesis and myocardial regeneration following myocardial infarction via the MALAT1/MicroRNA-25-3p/CDC42 Axis. Oxid. Med. Cell Longev. 2021, 9959746. doi:10.1155/2021/9959746
Chen, F., Li, X., Zhao, J., Geng, J., Xie, J., and Xu, B. (2020). Bone marrow mesenchymal stem cell-derived exosomes attenuate cardiac hypertrophy and fibrosis in pressure overload induced remodeling. Vitro Cell Dev. Biol. Anim. 56 (7), 567–576. doi:10.1007/s11626-020-00481-2
Chen, J., Fei, X., Wang, J., and Cai, Z. (2020). Tumor-derived extracellular vesicles: Regulators of tumor microenvironment and the enlightenment in tumor therapy. Pharmacol. Res. 159, 105041. doi:10.1016/j.phrs.2020.105041
Chen, P., Wu, R., Zhu, W., Jiang, Z., Xu, Y., Chen, H., et al. (2014). Hypoxia preconditioned mesenchymal stem cells prevent cardiac fibroblast activation and collagen production via leptin. PLoS One 9 (8), e103587. doi:10.1371/journal.pone.0103587
Chiossone, L., Conte, R., Spaggiari, G. M., Serra, M., Romei, C., Bellora, F., et al. (2016). Mesenchymal stromal cells induce peculiar alternatively activated macrophages capable of dampening both innate and adaptive immune responses. Stem Cells 34 (7), 1909–1921. doi:10.1002/stem.2369
Chistiakov, D. A., Orekhov, A. N., and Bobryshev, Y. V. (2015). Extracellular vesicles and atherosclerotic disease. Cell Mol. Life Sci. 72 (14), 2697–2708. doi:10.1007/s00018-015-1906-2
Choudhuri, K., Llodra, J., Roth, E. W., Tsai, J., Gordo, S., Wucherpfennig, K. W., et al. (2014). Polarized release of T-cell-receptor-enriched microvesicles at the immunological synapse. Nature 507 (7490), 118–123. doi:10.1038/nature12951
Clos-Sansalvador, M., Monguio-Tortajada, M., Roura, S., Franquesa, M., and Borras, F. E. (2022). Commonly used methods for extracellular vesicles' enrichment: Implications in downstream analyses and use. Eur. J. Cell Biol. 101 (3), 151227. doi:10.1016/j.ejcb.2022.151227
Cocucci, E., Racchetti, G., and Meldolesi, J. (2009). Shedding microvesicles: Artefacts no more. Trends Cell Biol. 19 (2), 43–51. doi:10.1016/j.tcb.2008.11.003
Costa Verdera, H., Gitz-Francois, J. J., Schiffelers, R. M., and Vader, P. (2017). Cellular uptake of extracellular vesicles is mediated by clathrin-independent endocytosis and macropinocytosis. J. Control Release 266, 100–108. doi:10.1016/j.jconrel.2017.09.019
Courageux, Y., Monguio-Tortajada, M., Prat-Vidal, C., Bayes-Genis, A., and Roura, S. (2022). Clinical translation of mesenchymal stromal cell extracellular vesicles: Considerations on scientific rationale and production requisites. J. Cell Mol. Med. 26 (3), 937–939. doi:10.1111/jcmm.17112
D'Anca, M., Fenoglio, C., Serpente, M., Arosio, B., Cesari, M., Scarpini, E. A., et al. (2019). Exosome determinants of physiological aging and age-related neurodegenerative diseases. Front. Aging Neurosci. 11, 232. doi:10.3389/fnagi.2019.00232
Dalli, J., Norling, L. V., Renshaw, D., Cooper, D., Leung, K. Y., and Perretti, M. (2008). Annexin 1 mediates the rapid anti-inflammatory effects of neutrophil-derived microparticles. Blood 112 (6), 2512–2519. doi:10.1182/blood-2008-02-140533
de Couto, G., Gallet, R., Cambier, L., Jaghatspanyan, E., Makkar, N., Dawkins, J. F., et al. (2017). Exosomal MicroRNA transfer into macrophages mediates cellular postconditioning. Circulation 136 (2), 200–214. doi:10.1161/CIRCULATIONAHA.116.024590
Deng, S., Zhou, X., Ge, Z., Song, Y., Wang, H., Liu, X., et al. (2019). Exosomes from adipose-derived mesenchymal stem cells ameliorate cardiac damage after myocardial infarction by activating S1P/SK1/S1PR1 signaling and promoting macrophage M2 polarization. Int. J. Biochem. Cell Biol. 114, 105564. doi:10.1016/j.biocel.2019.105564
Dewald, O., Zymek, P., Winkelmann, K., Koerting, A., Ren, G., Abou-Khamis, T., et al. (2005). CCL2/Monocyte Chemoattractant Protein-1 regulates inflammatory responses critical to healing myocardial infarcts. Circ. Res. 96 (8), 881–889. doi:10.1161/01.RES.0000163017.13772.3a
Di Vizio, D., Morello, M., Dudley, A. C., Schow, P. W., Adam, R. M., Morley, S., et al. (2012). Large oncosomes in human prostate cancer tissues and in the circulation of mice with metastatic disease. Am. J. Pathol. 181 (5), 1573–1584. doi:10.1016/j.ajpath.2012.07.030
Doran, A. C., Yurdagul, A., Tabas, I., Dou, G., Tian, R., Liu, X., et al. (2020). Efferocytosis in health and diseaseChimeric apoptotic bodies functionalized with natural membrane and modular delivery system for inflammation modulation. Nat. Rev. ImmunolSci Adv. 206 (430), 254eaba2987–267. doi:10.1038/s41577-019-0240-610.1126/sciadv.aba2987
Doyle, L. M., and Wang, M. Z. (2019). Overview of extracellular vesicles, their origin, composition, purpose, and methods for exosome isolation and analysis. Cells 8 (7), 727. doi:10.3390/cells8070727
Dzialo, E., Rudnik, M., Koning, R. I., Czepiel, M., Tkacz, K., Baj-Krzyworzeka, M., et al. (2019). WNT3a and WNT5a transported by exosomes activate WNT signaling pathways in human cardiac fibroblasts. Int. J. Mol. Sci. 20 (6), 1436. doi:10.3390/ijms20061436
Eken, C., Gasser, O., Zenhaeusern, G., Oehri, I., Hess, C., and Schifferli, J. A. (2008). Polymorphonuclear neutrophil-derived ectosomes interfere with the maturation of monocyte-derived dendritic cells. J. Immunol. 180 (2), 817–824. doi:10.4049/jimmunol.180.2.817
Eken, C., Martin, P. J., Sadallah, S., Treves, S., Schaller, M., and Schifferli, J. A. (2010). Ectosomes released by polymorphonuclear neutrophils induce a MerTK-dependent anti-inflammatory pathway in macrophages. J. Biol. Chem. 285 (51), 39914–39921. doi:10.1074/jbc.M110.126748
Escola, J. M., Kleijmeer, M. J., Stoorvogel, W., Griffith, J. M., Yoshie, O., and Geuze, H. J. (1998). Selective enrichment of tetraspan proteins on the internal vesicles of multivesicular endosomes and on exosomes secreted by human B-lymphocytes. J. Biol. Chem. 273 (32), 20121–20127. doi:10.1074/jbc.273.32.20121
Escudier, B., Dorval, T., Chaput, N., Andre, F., Caby, M. P., Novault, S., et al. (2005). Vaccination of metastatic melanoma patients with autologous dendritic cell (DC) derived-exosomes: Results of thefirst phase I clinical trial. J. Transl. Med. 3 (1), 10. doi:10.1186/1479-5876-3-10
Fan, C., Wang, Q., Chen, Y., Ye, T., and Fan, Y. (2022). Exosomes derived from bone mesenchymal stem cells attenuate myocardial fibrosis both in vivo and in vitro via autophagy activation: The key role of miR-199a-3p/mTOR pathway. Hum. Cell 35 (3), 817–835. doi:10.1007/s13577-022-00680-x
Fan, Z., Jiang, C., Wang, Y., Wang, K., Marsh, J., Zhang, D., et al. (2022). Engineered extracellular vesicles as intelligent nanosystems for next-generation nanomedicine. Nanoscale Horiz. 7 (7), 682–714. doi:10.1039/d2nh00070a
Feng, D., Zhao, W. L., Ye, Y. Y., Bai, X. C., Liu, R. Q., Chang, L. F., et al. (2010). Cellular internalization of exosomes occurs through phagocytosis. Traffic 11 (5), 675–687. doi:10.1111/j.1600-0854.2010.01041.x
Feng, Y., Huang, W., Wani, M., Yu, X., and Ashraf, M. (2014). Ischemic preconditioning potentiates the protective effect of stem cells through secretion of exosomes by targeting Mecp2 via miR-22. PLoS One 9 (2), e88685. doi:10.1371/journal.pone.0088685
Feyen, D. A. M., Gaetani, R., Doevendans, P. A., and Sluijter, J. P. G. (2016). Stem cell-based therapy: Improving myocardial cell delivery. Adv. Drug Deliv. Rev. 106, 104–115. doi:10.1016/j.addr.2016.04.023
Frangogiannis, N. G. (2007). Chemokines in ischemia and reperfusion. Thromb. Haemost. 97 (5), 738–747. doi:10.1160/th07-01-0022
Frangogiannis, N. G. (2015). Pathophysiology of myocardial infarction. Compr. Physiol. 5 (4), 1841–1875. doi:10.1002/cphy.c150006
Frangogiannis, N. G. (2012). Regulation of the inflammatory response in cardiac repair. Circ. Res. 110 (1), 159–173. doi:10.1161/CIRCRESAHA.111.243162
Frangogiannis, N. G. (2008). The immune system and cardiac repair. Pharmacol. Res. 58 (2), 88–111. doi:10.1016/j.phrs.2008.06.007
Frangogiannis, N. G. (2014). The inflammatory response in myocardial injury, repair, and remodelling. Nat. Rev. Cardiol. 11 (5), 255–265. doi:10.1038/nrcardio.2014.28
Frank, A., Bonney, M., Bonney, S., Weitzel, L., Koeppen, M., and Eckle, T. (2012). Myocardial ischemia reperfusion injury: From basic science to clinical bedside. Semin. Cardiothorac. Vasc. Anesth. 16 (3), 123–132. doi:10.1177/1089253211436350
Fujita, J., Tohyama, S., Kishino, Y., Okada, M., and Morita, Y. (2019). Concise review: Genetic and epigenetic regulation of cardiac differentiation from human pluripotent stem cells. Stem Cells 37 (8), 992–1002. doi:10.1002/stem.3027
Garikipati, V. N. S., Verma, S. K., Jolardarashi, D., Cheng, Z., Ibetti, J., Cimini, M., et al. (2017). Therapeutic inhibition of miR-375 attenuates post-myocardial infarction inflammatory response and left ventricular dysfunction via PDK-1-AKT signalling axis. Cardiovasc Res. 113 (8), 938–949. doi:10.1093/cvr/cvx052
Gasser, O., and Schifferli, J. A. (2004). Activated polymorphonuclear neutrophils disseminate anti-inflammatory microparticles by ectocytosis. Blood 104 (8), 2543–2548. doi:10.1182/blood-2004-01-0361
Ge, X., Meng, Q., Wei, L., Liu, J., Li, M., Liang, X., et al. (2021). Myocardial ischemia-reperfusion induced cardiac extracellular vesicles harbour proinflammatory features and aggravate heart injury. J. Extracell. Vesicles 10 (4), e12072. doi:10.1002/jev2.12072
Gibb, A. A., Lazaropoulos, M. P., and Elrod, J. W. (2020). Myofibroblasts and fibrosis: Mitochondrial and metabolic control of cellular differentiation. Circ. Res. 127 (3), 427–447. doi:10.1161/CIRCRESAHA.120.316958
Guo, M., Yin, Z., Chen, F., and Lei, P. (2020). Mesenchymal stem cell-derived exosome: A promising alternative in the therapy of alzheimer's disease. Alzheimers Res. Ther. 12 (1), 109. doi:10.1186/s13195-020-00670-x
Han, X., Zhang, Y., Zhang, X., Ji, H., Wang, W., Qiao, O., et al. (2022). Targeting adipokines: A new strategy for the treatment of myocardial fibrosis. Pharmacol. Res. 181, 106257. doi:10.1016/j.phrs.2022.106257
Hanson, P. I., and Cashikar, A. (2012). Multivesicular body morphogenesis. Annu. Rev. Cell Dev. Biol. 28, 337–362. doi:10.1146/annurev-cellbio-092910-154152
Harmati, M., Bukva, M., Boroczky, T., Buzas, K., and Gyukity-Sebestyen, E. (2021). The role of the metabolite cargo of extracellular vesicles in tumor progression. Cancer Metastasis Rev. 40 (4), 1203–1221. doi:10.1007/s10555-021-10014-2
Hessvik, N. P., and Llorente, A. (2018). Current knowledge on exosome biogenesis and release. Cell Mol. Life Sci. 75 (2), 193–208. doi:10.1007/s00018-017-2595-9
Hou, P. P., and Chen, H. Z. (2021). Extracellular vesicles in the tumor immune microenvironment. Cancer Lett. 516, 48–56. doi:10.1016/j.canlet.2021.05.032
Huang, J., Wang, F., Sun, X., Chu, X., Jiang, R., Wang, Y., et al. (2021). Myocardial infarction cardiomyocytes-derived exosomal miR-328-3p promote apoptosis via Caspase signaling. Am. J. Transl. Res. 13 (4), 2365–2378.
Huang, L., Yang, L., Ding, Y., Jiang, X., Xia, Z., and You, Z. (2020). Human umbilical cord mesenchymal stem cells-derived exosomes transfers microRNA-19a to protect cardiomyocytes from acute myocardial infarction by targeting SOX6. Cell Cycle 19 (3), 339–353. doi:10.1080/15384101.2019.1711305
Huang, P., Wang, L., Li, Q., Tian, X., Xu, J., Xu, J., et al. (2020). Atorvastatin enhances the therapeutic efficacy of mesenchymal stem cells-derived exosomes in acute myocardial infarction via up-regulating long non-coding RNA H19. Cardiovasc Res. 116 (2), 353–367. doi:10.1093/cvr/cvz139
Huang, Y., Chen, L., Feng, Z., Chen, W., Yan, S., Yang, R., et al. (2021). EPC-derived exosomal miR-1246 and miR-1290 regulate phenotypic changes of fibroblasts to endothelial cells to exert protective effects on myocardial infarction by targeting ELF5 and SP1. Front. Cell Dev. Biol. 9, 647763. doi:10.3389/fcell.2021.647763
Hugel, B., Martinez, M. C., Kunzelmann, C., and Freyssinet, J. M. (2005). Membrane microparticles: Two sides of the coin. Physiol. (Bethesda) 20, 22–27. doi:10.1152/physiol.00029.2004
Hurley, J. H., and Hanson, P. I. (2010). Membrane budding and scission by the ESCRT machinery: it's all in the neck. Nat. Rev. Mol. Cell Biol. 11 (8), 556–566. doi:10.1038/nrm2937
Ibanez, B., James, S., Agewall, S., Antunes, M. J., Bucciarelli-Ducci, C., Bueno, H., et al. (2018). 2017 ESC Guidelines for the management of acute myocardial infarction in patients presenting with ST-segment elevation: The Task Force for the management of acute myocardial infarction in patients presenting with ST-segment elevation of the European Society of Cardiology (ESC). Eur. Heart J. 39 (2), 119–177. doi:10.1093/eurheartj/ehx393
Janas, T., Janas, M. M., Sapon, K., and Janas, T. (2015). Mechanisms of RNA loading into exosomes. FEBS Lett. 589 (13), 1391–1398. doi:10.1016/j.febslet.2015.04.036
Jennings, R. B., and Reimer, K. A. (1991). The cell biology of acute myocardial ischemia. Annu. Rev. Med. 42, 225–246. doi:10.1146/annurev.me.42.020191.001301
Johnstone, R. M., Adam, M., Hammond, J. R., Orr, L., and Turbide, C. (1987). Vesicle formation during reticulocyte maturation. Association of plasma membrane activities with released vesicles (exosomes). J. Biol. Chem. 262 (19), 9412–9420. doi:10.1016/s0021-9258(18)48095-7
Jung, J. H., Ikeda, G., Tada, Y., von Bornstadt, D., Santoso, M. R., Wahlquist, C., et al. (2021). miR-106a-363 cluster in extracellular vesicles promotes endogenous myocardial repair via Notch3 pathway in ischemic heart injury. Basic Res. Cardiol. 116 (1), 19. doi:10.1007/s00395-021-00858-8
Kabe, Y., Ando, K., Hirao, S., Yoshida, M., and Handa, H. (2005). Redox regulation of NF-kappaB activation: Distinct redox regulation between the cytoplasm and the nucleus. Antioxid. Redox Signal 7 (3-4), 395–403. doi:10.1089/ars.2005.7.395
Kang, J. Y., Kim, H., Mun, D., Yun, N., and Joung, B. (2021). Co-delivery of curcumin and miRNA-144-3p using heart-targeted extracellular vesicles enhances the therapeutic efficacy for myocardial infarction. J. Control Release 331, 62–73. doi:10.1016/j.jconrel.2021.01.018
Karantalis, V., DiFede, D. L., Gerstenblith, G., Pham, S., Symes, J., Zambrano, J. P., et al. (2014). Autologous mesenchymal stem cells produce concordant improvements in regional function, tissue perfusion, and fibrotic burden when administered to patients undergoing coronary artery bypass grafting: The Prospective Randomized Study of Mesenchymal Stem Cell Therapy in Patients Undergoing Cardiac Surgery (PROMETHEUS) trial. Circ. Res. 114 (8), 1302–1310. doi:10.1161/CIRCRESAHA.114.303180
Ke, X., Yang, D., Liang, J., Wang, X., Wu, S., Wang, X., et al. (2017). Human endothelial progenitor cell-derived exosomes increase proliferation and angiogenesis in cardiac fibroblasts by promoting the mesenchymal-endothelial transition and reducing high mobility group box 1 protein B1 expression. DNA Cell Biol. 36 (11), 1018–1028. doi:10.1089/dna.2017.3836
Ke, X., Yang, R., Wu, F., Wang, X., Liang, J., Hu, X., et al. (2021). Exosomal miR-218-5p/miR-363-3p from endothelial progenitor cells ameliorate myocardial infarction by targeting the p53/JMY signaling pathway. Oxid. Med. Cell Longev. 2021, 5529430. doi:10.1155/2021/5529430
Kim, H., Yun, N., Mun, D., Kang, J. Y., Lee, S. H., Park, H., et al. (2018). Cardiac-specific delivery by cardiac tissue-targeting peptide-expressing exosomes. Biochem. Biophys. Res. Commun. 499 (4), 803–808. doi:10.1016/j.bbrc.2018.03.227
Kim, J. H., Joo, H. J., Kim, M., Choi, S. C., Lee, J. I., Hong, S. J., et al. (2017). Transplantation of adipose-derived stem cell sheet attenuates adverse cardiac remodeling in acute myocardial infarction. Tissue Eng. Part A 23 (1-2), 1–11. doi:10.1089/ten.TEA.2016.0023
Koga, H., Sugiyama, S., Kugiyama, K., Watanabe, K., Fukushima, H., Tanaka, T., et al. (2005). Elevated levels of VE-cadherin-positive endothelial microparticles in patients with type 2 diabetes mellitus and coronary artery disease. J. Am. Coll. Cardiol. 45 (10), 1622–1630. doi:10.1016/j.jacc.2005.02.047
Kologrivova, I., Shtatolkina, M., Suslova, T., and Ryabov, V. (2021). Cells of the immune system in cardiac remodeling: Main players in resolution of inflammation and repair after myocardial infarction. Front. Immunol. 12, 664457. doi:10.3389/fimmu.2021.664457
Koppler, B., Cohen, C., Schlondorff, D., and Mack, M. (2006). Differential mechanisms of microparticle transfer toB cells and monocytes: Anti-inflammatory propertiesof microparticles. Eur. J. Immunol. 36 (3), 648–660. doi:10.1002/eji.200535435
Kowal, J., Tkach, M., and Thery, C. (2014). Biogenesis and secretion of exosomes. Curr. Opin. Cell Biol. 29, 116–125. doi:10.1016/j.ceb.2014.05.004
Laulagnier, K., Motta, C., Hamdi, S., Roy, S., Fauvelle, F., Pageaux, J. F., et al. (2004). Mast cell- and dendritic cell-derived exosomes display a specific lipid composition and an unusual membrane organization. Biochem. J. 380, 161–171. doi:10.1042/BJ20031594
Lener, T., Gimona, M., Aigner, L., Borger, V., Buzas, E., Camussi, G., et al. (2015). Applying extracellular vesicles based therapeutics in clinical trials - an ISEV position paper. J. Extracell. Vesicles 4, 30087. doi:10.3402/jev.v4.30087
Leventis, P. A., and Grinstein, S. (2010). The distribution and function of phosphatidylserine in cellular membranes. Annu. Rev. Biophys. 39, 407–427. doi:10.1146/annurev.biophys.093008.131234
Ley, K., Laudanna, C., Cybulsky, M. I., and Nourshargh, S. (2007). Getting to the site of inflammation: The leukocyte adhesion cascade updated. Nat. Rev. Immunol. 7 (9), 678–689. doi:10.1038/nri2156
Liao, Z., Chen, Y., Duan, C., Zhu, K., Huang, R., Zhao, H., et al. (2021). Cardiac telocytes inhibit cardiac microvascular endothelial cell apoptosis through exosomal miRNA-21-5p-targeted cdip1 silencing to improve angiogenesis following myocardial infarction. Theranostics 11 (1), 268–291. doi:10.7150/thno.47021
Liu, D., Kou, X., Chen, C., Liu, S., Liu, Y., Yu, W., et al. (2018). Circulating apoptotic bodies maintain mesenchymal stem cell homeostasis and ameliorate osteopenia via transferring multiple cellular factors. Cell Res. 28 (9), 918–933. doi:10.1038/s41422-018-0070-2
Liu, H., Liu, S., Qiu, X., Yang, X., Bao, L., Pu, F., et al. (2020). Donor MSCs release apoptotic bodies to improve myocardial infarction via autophagy regulation in recipient cells. Autophagy 16 (12), 2140–2155. doi:10.1080/15548627.2020.1717128
Liu, H., Zhang, Y., Yuan, J., Gao, W., Zhong, X., Yao, K., et al. (2021). Dendritic cellderived exosomal miR4943p promotes angiogenesis following myocardial infarction. Int. J. Mol. Med. 47 (1), 315–325. doi:10.3892/ijmm.2020.4776
Liu, S., Chen, J., Shi, J., Zhou, W., Wang, L., Fang, W., et al. (2020). M1-like macrophage-derived exosomes suppress angiogenesis and exacerbate cardiac dysfunction in a myocardial infarction microenvironment. Basic Res. Cardiol. 115 (2), 22. doi:10.1007/s00395-020-0781-7
Liu, Y., Guan, R., Yan, J., Zhu, Y., Sun, S., and Qu, Y. (2022). Mesenchymal stem cell-derived extracellular vesicle-shuttled microRNA-302d-3p represses inflammation and cardiac remodeling following acute myocardial infarction. J. Cardiovasc Transl. Res. 15, 754–771. doi:10.1007/s12265-021-10200-1
Liu, Y., Xia, Y., Smollar, J., Mao, W., and Wan, Y. (2021). The roles of small extracellular vesicles in lung cancer: Molecular pathology, mechanisms, diagnostics, and therapeutics. Biochim. Biophys. Acta Rev. Cancer 1876 (1), 188539. doi:10.1016/j.bbcan.2021.188539
Liu, Y., Xu, J., Wu, M., Kang, L., and Xu, B. (2020). The effector cells and cellular mediators of immune system involved in cardiac inflammation and fibrosis after myocardial infarction. J. Cell Physiol. 235 (12), 8996–9004. doi:10.1002/jcp.29732
Liu, J, J., Jiang, M., Deng, S., Lu, J., Huang, H., Zhang, Y., et al. (2018). miR-93-5p-Containing exosomes treatment attenuates acute myocardial infarction-induced myocardial damage. Mol. Ther. Nucleic Acids 11, 103–115. doi:10.1016/j.omtn.2018.01.010
Long, R., Gao, L., Li, Y., Li, G., Qin, P., Wei, Z., et al. (2021). M2 macrophage-derived exosomes carry miR-1271-5p to alleviate cardiac injury in acute myocardial infarction through down-regulating SOX6. Mol. Immunol. 136, 26–35. doi:10.1016/j.molimm.2021.05.006
Lotvall, J., Hill, A. F., Hochberg, F., Buzas, E. I., Di Vizio, D., Gardiner, C., et al. (2014). Minimal experimental requirements for definition of extracellular vesicles and their functions: A position statement from the international society for extracellular vesicles. J. Extracell. Vesicles 3, 26913. doi:10.3402/jev.v3.26913
Loyer, X., Zlatanova, I., Devue, C., Yin, M., Howangyin, K. Y., Klaihmon, P., et al. (2018). Intra-cardiac release of extracellular vesicles shapes inflammation following myocardial infarction. Circ. Res. 123 (1), 100–106. doi:10.1161/CIRCRESAHA.117.311326
Luo, Q., Guo, D., Liu, G., Chen, G., Hang, M., and Jin, M. (2017). Exosomes from MiR-126-overexpressing adscs are therapeutic in relieving acute myocardial ischaemic injury. Cell Physiol. Biochem. 44 (6), 2105–2116. doi:10.1159/000485949
Ma, Y., Halade, G. V., Zhang, J., Ramirez, T. A., Levin, D., Voorhees, A., et al. (2013). Matrix metalloproteinase-28 deletion exacerbates cardiac dysfunction and rupture after myocardial infarction in mice by inhibiting M2 macrophage activation. Circ. Res. 112 (4), 675–688. doi:10.1161/CIRCRESAHA.111.300502
Ma, Y., Iyer, R. P., Jung, M., Czubryt, M. P., and Lindsey, M. L. (2017). Cardiac fibroblast activation post-myocardial infarction: Current knowledge gaps. Trends Pharmacol. Sci. 38 (5), 448–458. doi:10.1016/j.tips.2017.03.001
Ma, Y. (2021). Role of neutrophils in cardiac injury and repair following myocardial infarction. Cells 10 (7), 1676. doi:10.3390/cells10071676
Ma, Y., Yabluchanskiy, A., Iyer, R. P., Cannon, P. L., Flynn, E. R., Jung, M., et al. (2016). Temporal neutrophil polarization following myocardial infarction. Cardiovasc Res. 110 (1), 51–61. doi:10.1093/cvr/cvw024
Man, K., Brunet, M. Y., Jones, M. C., and Cox, S. C. (2020). Engineered extracellular vesicles: Tailored-made nanomaterials for medical applications. Nanomater. (Basel) 10 (9), 1838. doi:10.3390/nano10091838
Marban, E. (2018). The secret life of exosomes: What bees can teach us about next-generation therapeutics. J. Am. Coll. Cardiol. 71 (2), 193–200. doi:10.1016/j.jacc.2017.11.013
Mathieu, M., Martin-Jaular, L., Lavieu, G., and Thery, C. (2019). Specificities of secretion and uptake of exosomes and other extracellular vesicles for cell-to-cell communication. Nat. Cell Biol. 21 (1), 9–17. doi:10.1038/s41556-018-0250-9
McConnell, R. E., Higginbotham, J. N., Shifrin, D. A., Tabb, D. L., Coffey, R. J., and Tyska, M. J. (2009). The enterocyte microvillus is a vesicle-generating organelle. J. Cell Biol. 185 (7), 1285–1298. doi:10.1083/jcb.200902147
Mentkowski, K. I., Mursleen, A., Snitzer, J. D., Euscher, L. M., and Lang, J. K. (2020). CDC-derived extracellular vesicles reprogram inflammatory macrophages to an arginase 1-dependent proangiogenic phenotype. Am. J. Physiol. Heart Circ. Physiol. 318 (6), H1447–H1460. doi:10.1152/ajpheart.00155.2020
Mesri, M., and Altieri, D. C. (1999). Leukocyte microparticles stimulate endothelial cell cytokine release and tissue factor induction in a JNK1 signaling pathway. J. Biol. Chem. 274 (33), 23111–23118. doi:10.1074/jbc.274.33.23111
Miyanishi, M., Tada, K., Koike, M., Uchiyama, Y., Kitamura, T., and Nagata, S. (2007). Identification of Tim4 as a phosphatidylserine receptor. Nature 450 (7168), 435–439. doi:10.1038/nature06307
Moghaddam, A. S., Afshari, J. T., Esmaeili, S. A., Saburi, E., Joneidi, Z., and Momtazi-Borojeni, A. A. (2019). Cardioprotective microRNAs: Lessons from stem cell-derived exosomal microRNAs to treat cardiovascular disease. Atherosclerosis 285, 1–9. doi:10.1016/j.atherosclerosis.2019.03.016
Monguio-Tortajada, M., Prat-Vidal, C., Martinez-Falguera, D., Teis, A., Soler-Botija, C., Courageux, Y., et al. (2022). Acellular cardiac scaffolds enriched with MSC-derived extracellular vesicles limit ventricular remodelling and exert local and systemic immunomodulation in a myocardial infarction porcine model. Theranostics 12 (10), 4656–4670. doi:10.7150/thno.72289
Monguio-Tortajada, M., Prat-Vidal, C., Moron-Font, M., Clos-Sansalvador, M., Calle, A., Gastelurrutia, P., et al. (2021). Local administration of porcine immunomodulatory, chemotactic and angiogenic extracellular vesicles using engineered cardiac scaffolds for myocardial infarction. Bioact. Mater 6 (10), 3314–3327. doi:10.1016/j.bioactmat.2021.02.026
Morel, O., Toti, F., Hugel, B., and Freyssinet, J. M. (2004). Cellular microparticles: A disseminated storage pool of bioactive vascular effectors. Curr. Opin. Hematol. 11 (3), 156–164. doi:10.1097/01.moh.0000131441.10020.87
Morello, M., Minciacchi, V. R., de Candia, P., Yang, J., Posadas, E., Kim, H., et al. (2013). Large oncosomes mediate intercellular transfer of functional microRNA. Cell Cycle 12 (22), 3526–3536. doi:10.4161/cc.26539
Morse, M. A., Garst, J., Osada, T., Khan, S., Hobeika, A., Clay, T. M., et al. (2005). A phase I study of dexosome immunotherapy in patients with advanced non-small cell lung cancer. J. Transl. Med. 3 (1), 9. doi:10.1186/1479-5876-3-9
Muralidharan-Chari, V., Clancy, J., Plou, C., Romao, M., Chavrier, P., Raposo, G., et al. (2009). ARF6-regulated shedding of tumor cell-derived plasma membrane microvesicles. Curr. Biol. 19 (22), 1875–1885. doi:10.1016/j.cub.2009.09.059
Muralidharan-Chari, V., Clancy, J. W., Sedgwick, A., and D'Souza-Schorey, C. (2010). Microvesicles: Mediators of extracellular communication during cancer progression. J. Cell Sci. 123, 1603–1611. doi:10.1242/jcs.064386
Nanhwan, M. K., Ling, S., Kodakandla, M., Nylander, S., Ye, Y., and Birnbaum, Y. (2014). Chronic treatment with ticagrelor limits myocardial infarct size: An adenosine and cyclooxygenase-2-dependent effect. Arterioscler. Thromb. Vasc. Biol. 34 (9), 2078–2085. doi:10.1161/ATVBAHA.114.304002
Nasiri Kenari, A., Cheng, L., and Hill, A. F. (2020). Methods for loading therapeutics into extracellular vesicles and generating extracellular vesicles mimetic-nanovesicles. Methods 177, 103–113. doi:10.1016/j.ymeth.2020.01.001
Nassar, W., El-Ansary, M., Sabry, D., Mostafa, M. A., Fayad, T., Kotb, E., et al. (2016). Umbilical cord mesenchymal stem cells derived extracellular vesicles can safely ameliorate the progression of chronic kidney diseases. Biomater. Res. 20, 21. doi:10.1186/s40824-016-0068-0
Nie, X., Fan, J., Li, H., Yin, Z., Zhao, Y., Dai, B., et al. (2018). miR-217 promotes cardiac hypertrophy and dysfunction by targeting PTEN. Mol. Ther. Nucleic Acids 12, 254–266. doi:10.1016/j.omtn.2018.05.013
Ning, H., Chen, H., Deng, J., Xiao, C., Xu, M., Shan, L., et al. (2021). Exosomes secreted by FNDC5-BMMSCs protect myocardial infarction by anti-inflammation and macrophage polarization via NF-κB signaling pathway and Nrf2/HO-1 axis. Stem Cell Res. Ther. 12 (1), 519. doi:10.1186/s13287-021-02591-4
Njock, M. S., Cheng, H. S., Dang, L. T., Nazari-Jahantigh, M., Lau, A. C., Boudreau, E., et al. (2015). Endothelial cells suppress monocyte activation through secretion of extracellular vesicles containing antiinflammatory microRNAs. Blood 125 (20), 3202–3212. doi:10.1182/blood-2014-11-611046
Ohayon, L., Zhang, X., and Dutta, P. (2021). The role of extracellular vesicles in regulating local and systemic inflammation in cardiovascular disease. Pharmacol. Res. 170, 105692. doi:10.1016/j.phrs.2021.105692
Ong, S. B., Hernandez-Resendiz, S., Crespo-Avilan, G. E., Mukhametshina, R. T., Kwek, X. Y., Cabrera-Fuentes, H. A., et al. (2018). Inflammation following acute myocardial infarction: Multiple players, dynamic roles, and novel therapeutic opportunities. Pharmacol. Ther. 186, 73–87. doi:10.1016/j.pharmthera.2018.01.001
Palanisamy, V., Sharma, S., Deshpande, A., Zhou, H., Gimzewski, J., and Wong, D. T. (2010). Nanostructural and transcriptomic analyses of human saliva derived exosomes. PLoS One 5 (1), e8577. doi:10.1371/journal.pone.0008577
Pan, B. T., and Johnstone, R. M. (1983). Fate of the transferrin receptor during maturation of sheep reticulocytes in vitro: Selective externalization of the receptor. Cell 33 (3), 967–978. doi:10.1016/0092-8674(83)90040-5
Pan, J., Alimujiang, M., Chen, Q., Shi, H., and Luo, X. (2019). Exosomes derived from miR-146a-modified adipose-derived stem cells attenuate acute myocardial infarction-induced myocardial damage via downregulation of early growth response factor 1. J. Cell Biochem. 120 (3), 4433–4443. doi:10.1002/jcb.27731
Pan, W., Zhu, Y., Meng, X., Zhang, C., Yang, Y., and Bei, Y. (2019). Immunomodulation by exosomes in myocardial infarction. J. Cardiovasc Transl. Res. 12 (1), 28–36. doi:10.1007/s12265-018-9836-7
Peet, C., Ivetic, A., Bromage, D. I., and Shah, A. M. (2020). Cardiac monocytes and macrophages after myocardial infarction. Cardiovasc Res. 116 (6), 1101–1112. doi:10.1093/cvr/cvz336
Pegtel, D. M., and Gould, S. J. (2019). Exosomes. Annu. Rev. Biochem. 88, 487–514. doi:10.1146/annurev-biochem-013118-111902
Pelissier Vatter, F. A., Cioffi, M., Hanna, S. J., Castarede, I., Caielli, S., Pascual, V., et al. (2021). Extracellular vesicle- and particle-mediated communication shapes innate and adaptive immune responses. J. Exp. Med. 218 (8), e20202579. doi:10.1084/jem.20202579
Peng, Y., Zhao, J. L., Peng, Z. Y., Xu, W. F., and Yu, G. L. (2020). Exosomal miR-25-3p from mesenchymal stem cells alleviates myocardial infarction by targeting pro-apoptotic proteins and EZH2. Cell Death Dis. 11 (5), 317. doi:10.1038/s41419-020-2545-6
Pisitkun, T., Shen, R. F., and Knepper, M. A. (2004). Identification and proteomic profiling of exosomes in human urine. Proc. Natl. Acad. Sci. U. S. A. 101 (36), 13368–13373. doi:10.1073/pnas.0403453101
Porter, K. E., and Turner, N. A. (2009). Cardiac fibroblasts: At the heart of myocardial remodeling. Pharmacol. Ther. 123 (2), 255–278. doi:10.1016/j.pharmthera.2009.05.002
Prabhu, S. D., and Frangogiannis, N. G. (2016). The biological basis for cardiac repair after myocardial infarction: From inflammation to fibrosis. Circ. Res. 119 (1), 91–112. doi:10.1161/CIRCRESAHA.116.303577
Pramanik, K. C., Makena, M. R., Bhowmick, K., and Pandey, M. K. (2018). Advancement of NF-κB signaling pathway: A novel target in pancreatic cancer. Int. J. Mol. Sci. 19 (12), 3890. doi:10.3390/ijms19123890
Prieto-Vila, M., Yoshioka, Y., and Ochiya, T. (2021). Biological functions driven by mRNAs carried by extracellular vesicles in cancer. Front. Cell Dev. Biol. 9, 620498. doi:10.3389/fcell.2021.620498
Raposo, G., and Stoorvogel, W. (2013). Extracellular vesicles: Exosomes, microvesicles, and friends. J. Cell Biol. 200 (4), 373–383. doi:10.1083/jcb.201211138
Ratajczak, M. Z., and Ratajczak, J. (2020). Extracellular microvesicles/exosomes: Discovery, disbelief, acceptance, and the future? Leukemia 34 (12), 3126–3135. doi:10.1038/s41375-020-01041-z
Ren, Y., Xie, Y., Jiang, G., Fan, J., Yeung, J., Li, W., et al. (2008). Apoptotic cells protect mice against lipopolysaccharide-induced shock. J. Immunol. 180 (7), 4978–4985. doi:10.4049/jimmunol.180.7.4978
Richards, D. M., Delacher, M., Goldfarb, Y., Kagebein, D., Hofer, A. C., Abramson, J., et al. (2015). Treg cell differentiation: From thymus to peripheral tissue. Prog. Mol. Biol. Transl. Sci. 136, 175–205. doi:10.1016/bs.pmbts.2015.07.014
Rodrigues, M., Fan, J., Lyon, C., Wan, M., and Hu, Y. (2018). Role of extracellular vesicles in viral and bacterial infections: Pathogenesis, diagnostics, and therapeutics. Theranostics 8 (10), 2709–2721. doi:10.7150/thno.20576
Rodriguez, D. A., Weinlich, R., Brown, S., Guy, C., Fitzgerald, P., Dillon, C. P., et al. (2016). Characterization of RIPK3-mediated phosphorylation of the activation loop of MLKL during necroptosis. Cell Death Differ. 23 (1), 76–88. doi:10.1038/cdd.2015.70
Roth, G. A., Mensah, G. A., Johnson, C. O., Addolorato, G., Ammirati, E., Baddour, L. M., et al. (2020). Global burden of cardiovascular diseases and risk factors, 1990-2019: Update from the GBD 2019 study. J. Am. Coll. Cardiol. 76 (25), 2982–3021. doi:10.1016/j.jacc.2020.11.010
Roubille, F., Lairez, O., Mewton, N., Rioufol, G., Ranc, S., Sanchez, I., et al. (2012). Cardioprotection by clopidogrel in acute ST-elevated myocardial infarction patients: A retrospective analysis. Basic Res. Cardiol. 107 (4), 275. doi:10.1007/s00395-012-0275-3
Ruan, X. F., Li, Y. J., Ju, C. W., Shen, Y., Lei, W., Chen, C., et al. (2018). Exosomes from Suxiao Jiuxin pill-treated cardiac mesenchymal stem cells decrease H3K27 demethylase UTX expression in mouse cardiomyocytes in vitro. Acta Pharmacol. Sin. 39 (4), 579–586. doi:10.1038/aps.2018.18
Ruvinov, E., and Cohen, S. (2016). Alginate biomaterial for the treatment of myocardial infarction: Progress, translational strategies, and clinical outlook: From ocean algae to patient bedside. Adv. Drug Deliv. Rev. 96, 54–76. doi:10.1016/j.addr.2015.04.021
Sakaguchi, S. (2004). Naturally arising CD4+ regulatory t cells for immunologic self-tolerance and negative control of immune responses. Annu. Rev. Immunol. 22, 531–562. doi:10.1146/annurev.immunol.21.120601.141122
Sanchez-Alonso, S., Alcaraz-Serna, A., Sanchez-Madrid, F., and Alfranca, A. (2018). Extracellular vesicle-mediated immune regulation of tissue remodeling and angiogenesis after myocardial infarction. Front. Immunol. 9, 2799. doi:10.3389/fimmu.2018.02799
Sanchez-Sanchez, R., Gomez-Ferrer, M., Reinal, I., Buigues, M., Villanueva-Badenas, E., Ontoria-Oviedo, I., et al. (2021). miR-4732-3p in extracellular vesicles from mesenchymal stromal cells is cardioprotective during myocardial ischemia. Front. Cell Dev. Biol. 9, 734143. doi:10.3389/fcell.2021.734143
Saunderson, S. C., Dunn, A. C., Crocker, P. R., and McLellan, A. D. (2014). CD169 mediates the capture of exosomes in spleen and lymph node. Blood 123 (2), 208–216. doi:10.1182/blood-2013-03-489732
Schirone, L., Forte, M., D'Ambrosio, L., Valenti, V., Vecchio, D., Schiavon, S., et al. (2022). An overview of the molecular mechanisms associated with myocardial ischemic injury: State of the art and translational perspectives. Cells 11 (7), 1165. doi:10.3390/cells11071165
Schirone, L., Forte, M., Palmerio, S., Yee, D., Nocella, C., Angelini, F., et al. (2017). A review of the molecular mechanisms underlying the development and progression of cardiac remodeling. Oxid. Med. Cell Longev. 2017, 3920195. doi:10.1155/2017/3920195
Sengupta, V., Sengupta, S., Lazo, A., Woods, P., Nolan, A., and Bremer, N. (2020). Exosomes derived from bone marrow mesenchymal stem cells as treatment for severe COVID-19. Stem Cells Dev. 29 (12), 747–754. doi:10.1089/scd.2020.0080
Shi, Y., Yang, Y., Guo, Q., Gao, Q., Ding, Y., Wang, H., et al. (2019). Exosomes derived from human umbilical cord mesenchymal stem cells promote fibroblast-to-myofibroblast differentiation in inflammatory environments and benefit cardioprotective effects. Stem Cells Dev. 28 (12), 799–811. doi:10.1089/scd.2018.0242
Silvis, M. J. M., Kaffka Genaamd Dengler, S. E., Odille, C. A., Mishra, M., van der Kaaij, N. P., Doevendans, P. A., et al. (2020). Damage-associated molecular patterns in myocardial infarction and heart transplantation: The road to translational success. Front. Immunol. 11, 599511. doi:10.3389/fimmu.2020.599511
Soekmadji, C., Li, B., Huang, Y., Wang, H., An, T., Liu, C., et al. (2020). The future of Extracellular Vesicles as Theranostics - an ISEV meeting report. J. Extracell. Vesicles 9 (1), 1809766. doi:10.1080/20013078.2020.1809766
Soler-Botija, C., Monguio-Tortajada, M., Munizaga-Larroude, M., Galvez-Monton, C., Bayes-Genis, A., and Roura, S. (2022). Mechanisms governing the therapeutic effect of mesenchymal stromal cell-derived extracellular vesicles: A scoping review of preclinical evidence. Biomed. Pharmacother. 147, 112683. doi:10.1016/j.biopha.2022.112683
Song, Y., Wang, B., Zhu, X., Hu, J., Sun, J., Xuan, J., et al. (2021). Human umbilical cord blood-derived MSCs exosome attenuate myocardial injury by inhibiting ferroptosis in acute myocardial infarction mice. Cell Biol. Toxicol. 37 (1), 51–64. doi:10.1007/s10565-020-09530-8
Suetomi, T., Miyamoto, S., and Brown, J. H. (2019). Inflammation in nonischemic heart disease: Initiation by cardiomyocyte CaMKII and NLRP3 inflammasome signaling. Am. J. Physiol. Heart Circ. Physiol. 317 (5), H877–H890. doi:10.1152/ajpheart.00223.2019
Sun, Y. (2009). Myocardial repair/remodelling following infarction: Roles of local factors. Cardiovasc Res. 81 (3), 482–490. doi:10.1093/cvr/cvn333
Tarasov, V. V., Svistunov, A. A., Chubarev, V. N., Dostdar, S. A., Sokolov, A. V., Brzecka, A., et al. (2021). Extracellular vesicles in cancer nanomedicine. Semin. Cancer Biol. 69, 212–225. doi:10.1016/j.semcancer.2019.08.017
Thongboonkerd, V. (2019). Roles for exosome in various kidney diseases and disorders. Front. Pharmacol. 10, 1655. doi:10.3389/fphar.2019.01655
Tian, T., Zhu, Y. L., Zhou, Y. Y., Liang, G. F., Wang, Y. Y., Hu, F. H., et al. (2014). Exosome uptake through clathrin-mediated endocytosis and macropinocytosis and mediating miR-21 delivery. J. Biol. Chem. 289 (32), 22258–22267. doi:10.1074/jbc.M114.588046
Timmers, L., Pasterkamp, G., de Hoog, V. C., Arslan, F., Appelman, Y., and de Kleijn, D. P. (2012). The innate immune response in reperfused myocardium. Cardiovasc Res. 94 (2), 276–283. doi:10.1093/cvr/cvs018
Tsuchiya, A., Terai, S., Horiguchi, I., Homma, Y., Saito, A., Nakamura, N., et al. (2022). Basic points to consider regarding the preparation of extracellular vesicles and their clinical applications in Japan. Regen. Ther. 21, 19–24. doi:10.1016/j.reth.2022.05.003
Tucker, B., Vaidya, K., Cochran, B. J., and Patel, S. (2021). Inflammation during percutaneous coronary intervention-prognostic value, mechanisms and therapeutic targets. Cells 10 (6), 1391. doi:10.3390/cells10061391
Umbarkar, P., Singh, A. P., Tousif, S., Zhang, Q., Sethu, P., and Lal, H. (2021). Repurposing Nintedanib for pathological cardiac remodeling and dysfunction. Pharmacol. Res. 169, 105605. doi:10.1016/j.phrs.2021.105605
Vaidya, K., Tucker, B., Kurup, R., Khandkar, C., Pandzic, E., Barraclough, J., et al. (2021). Colchicine inhibits neutrophil extracellular trap formation in patients with acute coronary syndrome after percutaneous coronary intervention. J. Am. Heart Assoc. 10 (1), e018993. doi:10.1161/JAHA.120.018993
van den Borne, S. W., Diez, J., Blankesteijn, W. M., Verjans, J., Hofstra, L., and Narula, J. (2010). Myocardial remodeling after infarction: The role of myofibroblasts. Nat. Rev. Cardiol. 7 (1), 30–37. doi:10.1038/nrcardio.2009.199
van der Pol, E., Boing, A. N., Gool, E. L., and Nieuwland, R. (2016). Recent developments in the nomenclature, presence, isolation, detection and clinical impact of extracellular vesicles. J. Thromb. Haemost. 14 (1), 48–56. doi:10.1111/jth.13190
van der Pol, E., Coumans, F. A., Grootemaat, A. E., Gardiner, C., Sargent, I. L., Harrison, P., et al. (2014). Particle size distribution of exosomes and microvesicles determined by transmission electron microscopy, flow cytometry, nanoparticle tracking analysis, and resistive pulse sensing. J. Thromb. Haemost. 12 (7), 1182–1192. doi:10.1111/jth.12602
van Niel, G., D'Angelo, G., and Raposo, G. (2018). Shedding light on the cell biology of extracellular vesicles. Nat. Rev. Mol. Cell Biol. 19 (4), 213–228. doi:10.1038/nrm.2017.125
Vandergriff, A., Huang, K., Shen, D., Hu, S., Hensley, M. T., Caranasos, T. G., et al. (2018). Targeting regenerative exosomes to myocardial infarction using cardiac homing peptide. Theranostics 8 (7), 1869–1878. doi:10.7150/thno.20524
Vaskova, E., Ikeda, G., Tada, Y., Wahlquist, C., Mercola, M., and Yang, P. C. (2020). Sacubitril/valsartan improves cardiac function and decreases myocardial fibrosis via downregulation of exosomal miR-181a in a rodent chronic myocardial infarction model. J. Am. Heart Assoc. 9 (13), e015640. doi:10.1161/JAHA.119.015640
Veerman, R. E., Gucluler Akpinar, G., Eldh, M., and Gabrielsson, S. (2019). Immune cell-derived extracellular vesicles - functions and therapeutic applications. Trends Mol. Med. 25 (5), 382–394. doi:10.1016/j.molmed.2019.02.003
Viaud, S., Ploix, S., Lapierre, V., Thery, C., Commere, P. H., Tramalloni, D., et al. (2011). Updated technology to produce highly immunogenic dendritic cell-derived exosomes of clinical grade: A critical role of interferon-γ. J. Immunother. 34 (1), 65–75. doi:10.1097/CJI.0b013e3181fe535b
Vilahur, G., Gutierrez, M., Casani, L., Varela, L., Capdevila, A., Pons-Llado, G., et al. (2016). Protective effects of ticagrelor on myocardial injury after infarction. Circulation 134 (22), 1708–1719. doi:10.1161/CIRCULATIONAHA.116.024014
Villarroya-Beltri, C., Baixauli, F., Mittelbrunn, M., Fernandez-Delgado, I., Torralba, D., Moreno-Gonzalo, O., et al. (2016). ISGylation controls exosome secretion by promoting lysosomal degradation of MVB proteins. Nat. Commun. 7, 13588. doi:10.1038/ncomms13588
Visconti, R. P., and Markwald, R. R. (2006). Recruitment of new cells into the postnatal heart: Potential modification of phenotype by periostin. Ann. N. Y. Acad. Sci. 1080, 19–33. doi:10.1196/annals.1380.003
Wan, J., Lin, S., Yu, Z., Song, Z., Lin, X., Xu, R., et al. (2022). Protective effects of MicroRNA-200b-3p encapsulated by mesenchymal stem cells-secreted extracellular vesicles in myocardial infarction via regulating BCL2L11. J. Am. Heart Assoc. 11 (12), e024330. doi:10.1161/JAHA.121.024330
Wang, C., Zhang, C., Liu, L., Xi, A., Chen, B., Li, Y., et al. (2017). Macrophage-derived mir-155-containing exosomes suppress fibroblast proliferation and promote fibroblast inflammation during cardiac injury. Mol. Ther. 25 (1), 192–204. doi:10.1016/j.ymthe.2016.09.001
Wang, S., Dong, J., Li, L., Wu, R., Xu, L., Ren, Y., et al. (2022). Exosomes derived from miR-129-5p modified bone marrow mesenchymal stem cells represses ventricular remolding of mice with myocardial infarction. J. Tissue Eng. Regen. Med. 16 (2), 177–187. doi:10.1002/term.3268
Wang, S., Li, L., Liu, T., Jiang, W., and Hu, X. (2020). miR-19a/19b-loaded exosomes in combination with mesenchymal stem cell transplantation in a preclinical model of myocardial infarction. Regen. Med. 15 (6), 1749–1759. doi:10.2217/rme-2019-0136
Wang, X., Bai, L., Liu, X., Shen, W., Tian, H., Liu, W., et al. (2021a). Cardiac microvascular functions improved by MSC-derived exosomes attenuate cardiac fibrosis after ischemia-reperfusion via PDGFR-beta modulation. Int. J. Cardiol. 344, 13–24. doi:10.1016/j.ijcard.2021.09.017
Wang, X., Chen, Y., Zhao, Z., Meng, Q., Yu, Y., Sun, J., et al. (2018). Engineered exosomes with ischemic myocardium-targeting peptide for targeted therapy in myocardial infarction. J. Am. Heart Assoc. 7 (15), e008737. doi:10.1161/JAHA.118.008737
Wang, X., Morelli, M. B., Matarese, A., Sardu, C., and Santulli, G. (2020). Cardiomyocyte-derived exosomal microRNA-92a mediates post-ischemic myofibroblast activation both in vitro and ex vivo. Esc. Heart Fail 7 (1), 284–288. doi:10.1002/ehf2.12584
Wang, X., Zhu, Y., Wu, C., Liu, W., He, Y., and Yang, Q. (2021b). Adipose-derived mesenchymal stem cells-derived exosomes carry MicroRNA-671 to alleviate myocardial infarction through inactivating the TGFBR2/smad2 Axis. Inflammation 44 (5), 1815–1830. doi:10.1007/s10753-021-01460-9
Wang, Y., Li, C., Zhao, R., Qiu, Z., Shen, C., Wang, Z., et al. (2021c). CircUbe3a from M2 macrophage-derived small extracellular vesicles mediates myocardial fibrosis after acute myocardial infarction. Theranostics 11 (13), 6315–6333. doi:10.7150/thno.52843
Wei, Z., Qiao, S., Zhao, J., Liu, Y., Li, Q., Wei, Z., et al. (2019). miRNA-181a over-expression in mesenchymal stem cell-derived exosomes influenced inflammatory response after myocardial ischemia-reperfusion injury. Life Sci. 232, 116632. doi:10.1016/j.lfs.2019.116632
Westman, P. C., Lipinski, M. J., Luger, D., Waksman, R., Bonow, R. O., Wu, E., et al. (2016). Inflammation as a driver of adverse left ventricular remodeling after acute myocardial infarction. J. Am. Coll. Cardiol. 67 (17), 2050–2060. doi:10.1016/j.jacc.2016.01.073
Wolf, P. (1967). The nature and significance of platelet products in human plasma. Br. J. Haematol. 13 (3), 269–288. doi:10.1111/j.1365-2141.1967.tb08741.x
Wu, P., Zhang, B., Ocansey, D. K. W., Xu, W., and Qian, H. (2021). Extracellular vesicles: A bright star of nanomedicine. Biomaterials 269, 120467. doi:10.1016/j.biomaterials.2020.120467
Wu, Q., Wang, J., Tan, W. L. W., Jiang, Y., Wang, S., Li, Q., et al. (2020). Extracellular vesicles from human embryonic stem cell-derived cardiovascular progenitor cells promote cardiac infarct healing through reducing cardiomyocyte death and promoting angiogenesis. Cell Death Dis. 11 (5), 354. doi:10.1038/s41419-020-2508-y
Wu, R., Gao, W., Yao, K., and Ge, J. (2019). Roles of exosomes derived from immune cells in cardiovascular diseases. Front. Immunol. 10, 648. doi:10.3389/fimmu.2019.00648
Wu, Y., Peng, W., Fang, M., Wu, M., and Wu, M. (2022). MSCs-derived extracellular vesicles carrying miR-212-5p alleviate myocardial infarction-induced cardiac fibrosis via NLRC5/VEGF/TGF-β1/SMAD Axis. J. Cardiovasc Transl. Res. 15 (2), 302–316. doi:10.1007/s12265-021-10156-2
Xing, Y., Sun, X., Dou, Y., Wang, M., Zhao, Y., Yang, Q., et al. (2021). The immuno-modulation effect of macrophage-derived extracellular vesicles in chronic inflammatory diseases. Front. Immunol. 12, 785728. doi:10.3389/fimmu.2021.785728
Xiong, Y., Tang, R., Xu, J., Jiang, W., Gong, Z., Zhang, L., et al. (2022). Sequential transplantation of exosomes and mesenchymal stem cells pretreated with a combination of hypoxia and Tongxinluo efficiently facilitates cardiac repair. Stem Cell Res. Ther. 13 (1), 63. doi:10.1186/s13287-022-02736-z
Xiong, Y. Y., Gong, Z. T., Tang, R. J., and Yang, Y. J. (2021). The pivotal roles of exosomes derived from endogenous immune cells and exogenous stem cells in myocardial repair after acute myocardial infarction. Theranostics 11 (3), 1046–1058. doi:10.7150/thno.53326
Xuan, W., Khan, M., and Ashraf, M. (2020). Extracellular vesicles from Notch activated cardiac mesenchymal stem cells promote myocyte proliferation and neovasculogenesis. Front. Cell Dev. Biol. 8, 11. doi:10.3389/fcell.2020.00011
Xuan, W., Wang, L., Xu, M., Weintraub, N. L., and Ashraf, M. (2019). miRNAs in extracellular vesicles from iPS-derived cardiac progenitor cells effectively reduce fibrosis and promote angiogenesis in infarcted heart. Stem Cells Int. 2019, 3726392. doi:10.1155/2019/3726392
Yan, X., Anzai, A., Katsumata, Y., Matsuhashi, T., Ito, K., Endo, J., et al. (2013). Temporal dynamics of cardiac immune cell accumulation following acute myocardial infarction. J. Mol. Cell Cardiol. 62, 24–35. doi:10.1016/j.yjmcc.2013.04.023
Yanez-Mo, M., Siljander, P. R., Andreu, Z., Zavec, A. B., Borras, F. E., Buzas, E. I., et al. (2015). Biological properties of extracellular vesicles and their physiological functions. J. Extracell. Vesicles 4, 27066. doi:10.3402/jev.v4.27066
Yang, J., Yu, X., Xue, F., Li, Y., Liu, W., and Zhang, S. (2018). Exosomes derived from cardiomyocytes promote cardiac fibrosis via myocyte-fibroblast cross-talk. Am. J. Transl. Res. 10 (12), 4350–4366.
Yang, M., Liao, M., Liu, R., Zhang, Q., Zhang, S., He, Y., et al. (2022). Human umbilical cord mesenchymal stem cell-derived extracellular vesicles loaded with miR-223 ameliorate myocardial infarction through P53/S100A9 axis. Genomics 114 (3), 110319. doi:10.1016/j.ygeno.2022.110319
Yellon, D. M., and Hausenloy, D. J. (2007). Myocardial reperfusion injury. N. Engl. J. Med. 357 (11), 1121–1135. doi:10.1056/NEJMra071667
Youn, Y. J., Shrestha, S., Lee, Y. B., Kim, J. K., Lee, J. H., Hur, K., et al. (2021). Neutrophil-derived trail is a proinflammatory subtype of neutrophil-derived extracellular vesicles. Theranostics 11 (6), 2770–2787. doi:10.7150/thno.51756
Yu, H., and Wang, Z. (2019). Cardiomyocyte-derived exosomes: Biological functions and potential therapeutic implications. Front. Physiol. 10, 1049. doi:10.3389/fphys.2019.01049
Zaborowski, M. P., Balaj, L., Breakefield, X. O., and Lai, C. P. (2015). Extracellular vesicles: Composition, biological relevance, and methods of study. Bioscience 65 (8), 783–797. doi:10.1093/biosci/biv084
Zaidi, Y., Aguilar, E. G., Troncoso, M., Ilatovskaya, D. V., and DeLeon-Pennell, K. Y. (2021). Immune regulation of cardiac fibrosis post myocardial infarction. Cell Signal 77, 109837. doi:10.1016/j.cellsig.2020.109837
Zhang, Y., Cai, Z., Shen, Y., Lu, Q., Gao, W., Zhong, X., et al. (2021). Hydrogel-load exosomes derived from dendritic cells improve cardiac function via Treg cells and the polarization of macrophages following myocardial infarction. J. Nanobiotechnology 19 (1), 271. doi:10.1186/s12951-021-01016-x
Zhang, Z., Yang, J., Yan, W., Li, Y., Shen, Z., and Asahara, T. (2016). Pretreatment of cardiac stem cells with exosomes derived from mesenchymal stem cells enhances myocardial repair. J. Am. Heart Assoc. 5 (1), e002856. doi:10.1161/JAHA.115.002856
Zhao, C., Zhang, B., Jiang, J., Wang, Y., and Wu, Y. (2019a). Retracted: Up-regulation of ANXA1 suppresses polymorphonuclear neutrophil infiltration and myeloperoxidase activity by activating STAT3 signaling pathway in rat models of myocardial ischemia-reperfusion injury. Cell Signal 62, 109325. doi:10.1016/j.cellsig.2019.05.010
Zhao, J., Li, X., Hu, J., Chen, F., Qiao, S., Sun, X., et al. (2019b). Mesenchymal stromal cell-derived exosomes attenuate myocardial ischaemia-reperfusion injury through miR-182-regulated macrophage polarization. Cardiovasc Res. 115 (7), 1205–1216. doi:10.1093/cvr/cvz040
Zhao, W., Zhao, J., and Rong, J. (2020). Pharmacological modulation of cardiac remodeling after myocardial infarction. Oxid. Med. Cell Longev. 2020, 8815349. doi:10.1155/2020/8815349
Zhao, W., Zheng, X. L., and Zhao, S. P. (2015). Exosome and its roles in cardiovascular diseases. Heart Fail Rev. 20 (3), 337–348. doi:10.1007/s10741-014-9469-0
Zheng, J., Zhang, X., Cai, W., Yang, Y., Guo, T., Li, J., et al. (2022). Bone marrow mesenchymal stem cell-derived exosomal microRNA-29b-3p promotes angiogenesis and ventricular remodeling in rats with myocardial infarction by targeting ADAMTS16. Cardiovasc Toxicol. 22 (8), 689–700. doi:10.1007/s12012-022-09745-7
Zhou, W., Wang, J., Wu, Z., Huang, C., Lu, A., and Wang, Y. (2016). Systems pharmacology exploration of botanic drug pairs reveals the mechanism for treating different diseases. Sci. Rep. 6, 36985. doi:10.1038/srep36985
Zhu, F., Chen, Y., Li, J., Yang, Z., Lin, Y., Jiang, B., et al. (2022). Human umbilical cord mesenchymal stem cell-derived exosomes attenuate myocardial infarction injury via miR-24-3p-promoted M2 macrophage polarization. Adv. Biol. (Weinh) 6, e2200074. doi:10.1002/adbi.202200074
Zhu, L. P., Tian, T., Wang, J. Y., He, J. N., Chen, T., Pan, M., et al. (2018). Hypoxia-elicited mesenchymal stem cell-derived exosomes facilitates cardiac repair through miR-125b-mediated prevention of cell death in myocardial infarction. Theranostics 8 (22), 6163–6177. doi:10.7150/thno.28021
Zitvogel, L., Regnault, A., Lozier, A., Wolfers, J., Flament, C., Tenza, D., et al. (1998). Eradication of established murine tumors using a novel cell-free vaccine: Dendritic cell-derived exosomes. Nat. Med. 4 (5), 594–600. doi:10.1038/nm0598-594
Zoller, M. (2009). Tetraspanins: Push and pull in suppressing and promoting metastasis. Nat. Rev. Cancer 9 (1), 40–55. doi:10.1038/nrc2543
Zou, Y., Li, L., Li, Y., Chen, S., Xie, X., Jin, X., et al. (2021). Restoring cardiac functions after myocardial infarction-ischemia/reperfusion via an exosome anchoring conductive hydrogel. ACS Appl. Mater Interfaces 13 (48), 56892–56908. doi:10.1021/acsami.1c16481
Zwaal, R. F., and Schroit, A. J. (1997). Pathophysiologic implications of membrane phospholipid asymmetry in blood cells. Blood 89 (4), 1121–1132. doi:10.1182/blood.v89.4.1121
Glossary
AMI acute myocardial infarction
EVs Extracellular vesicles
HF heart failure
MI myocardial infarction
I/R ischemia-reperfusion
DAMPs damage-associated molecular patterns
CFs cardiac fibroblasts
MSCs mesenchymal stem cells
CPCs cardiac progenitor cells
MVs microvesicles
ABs apoptotic bodies
RIPK3 receptor-interacting protein kinase three
RIPK1 receptor-interacting protein kinase 1
PRRs pattern recognition receptors
TNF tumor necrosis factor
IL-1β interleukin 1β
IL-6 interleukin 6
MCP-1 monocyte chemotactic protein-1
ATP adenosine triphosphate
NF-κB nuclear factor κB
ECs endothelial cells
PSCs progenitor stem cells
MFBs myofibroblasts
TGF-β transforming growth factor β
ECM extracellular matrix
ILVs intraluminal vesicles
MVBs multivesicular bodies
PS phosphatidylserine
ESCRT endosomal sorting complex required for transport
CAMs cell adhesion molecules
CCL3 macrophage inflammatory protein 1 α
IL-1b interleukin 1b
IL-12a interleukin 12a
TNF-α tumor necrosis factor
IL-10 interleukin 10
CCL-2 chemokine (C-C motif) ligand 2
NDMVs neutrophil-derived MVs
NDTRs neutrophil-derived trails
AnxA1 membrane-linked protein A1
LMVs leukocyte-derived MVs
TGF-β1 transforming growth factor β1
DCs dendritic cells
ox-LDL oxidized low-density lipoprotein
BMMSC Bone marrow mesenchymal stem cell
ADSCs adipose stromal cells
Dyrk bispecific tyrosine phosphorylation kinase
MSC-Exos MSC-derived exosomes
hUCMSC-Exos human umbilical cord mesenchymal stem cell-derived exosomes
EPCs endothelial progenitor cells
CD31 cluster of differentiation 31
TCM traditional chinese medicine
C-MSCs cardiac mesenchymal stem cells
TFEB transcription factor EB
CDIP1 cell death involved p53 target 1
α-SMA α-smooth muscle actin
Col I collagen type I
MSC-EVs MSC-derived extracellular vesicles
PDGFR-β platelet-derived growth factor receptor-β
HPSC human pluripotent stem cell
CVPCs cardiovascular progenitor cells
CHP cardiac homing peptide
CDCs cardiosphere-derived cells
PCs progenitor cells
iCMs human iPSC-derived cardiomyocytes
IMTP ischemic myocardium-targeting peptide
AMSC-Exos adipose mesenchymal stem cell-derived exosomes
EGR1 growth response factor 1
M1-MØ M1 macrophages
M2-MØ M2 macrophages
ADSCs-Exos exosomes secreted by adipose-derived stem cells
CDCs-Exos exosomes secreted by Cardiosphere-derived cells
EPC-Exos exosomes secreted by endothelial progenitor cells
BMMSC-Exos exosomes derived from bone marrow mesenchymal stem cells
DC-Exos dendritic cells derived exosomes
CMs-Exos cardiomyocytes-derived exosomes
UMSC-Exos umbilical cord MSC-derived exosomes
DEX dendritic cell-derived exosomes
ARDS acute respiratory distress syndrome
hMSC-Exos human mesenchymal stem cell exosomes
itMSCs ischemia-tolerant MSCs
Keywords: myocardial infarction, cardiac remodeling, extracellular vesicles, cargo delivery, drug delivery
Citation: Cheng P, Wang X, Liu Q, Yang T, Qu H and Zhou H (2023) Extracellular vesicles mediate biological information delivery: A double-edged sword in cardiac remodeling after myocardial infarction. Front. Pharmacol. 14:1067992. doi: 10.3389/fphar.2023.1067992
Received: 12 October 2022; Accepted: 13 February 2023;
Published: 22 February 2023.
Edited by:
Gui-Rong Li, Xiamen University, ChinaReviewed by:
Hai-dong Guo, Shanghai University of Traditional Chinese Medicine, ChinaMuneyoshi Okada, Kitasato University, Japan
Alexander E. Berezin, Zaporizhia State Medical University, Ukraine
Copyright © 2023 Cheng, Wang, Liu, Yang, Qu and Zhou. This is an open-access article distributed under the terms of the Creative Commons Attribution License (CC BY). The use, distribution or reproduction in other forums is permitted, provided the original author(s) and the copyright owner(s) are credited and that the original publication in this journal is cited, in accordance with accepted academic practice. No use, distribution or reproduction is permitted which does not comply with these terms.
*Correspondence: Huiyan Qu, sgyyjxb@163.com; Hua Zhou, zhouhua@shutcm.edu.cn