- 1Laboratory of Neurobiology, Department of Biology, Faculty of Chemistry and Biology, University of Santiago of Chile, Santiago, Chile
- 2Center for the Development of Nanoscience and Nanotechnology (CEDENNA), Santiago, Chile
Analgesic efficacy of methadone in cancer and chronic non-cancer pains is greater than that of other opioids, probably because of its unique pharmacokinetics properties and also because it targets glutamatergic receptors in addition to µ-opioid receptors. However, methadone has drawbacks which are clearly related to dosing and treatment duration. The authors hypothesized that the antinociceptive efficacy of methadone could be synergistically potentiated by magnesium and copper salts in a preclinical mouse model of chronic pain, using the intraplantar formalin test as algesimetric tool. The spared nerve injury mice model was used to generate mononeuropathy. A low dose (0.25%) formalin was injected in the neuropathic limb in order to give rise only to Phase I response, resulting from direct activation by formalin of nociceptive primary afferents. Licking/biting of the formalin-injected limb was evaluated as nociceptive behavior during a 35-min observation period. Dose-response curves for intraperitoneal magnesium sulfate (10, 30, 100, and 300 mg/kg i.p.), copper sulfate (0.1, 0.3, 1, and 3 mg/kg i.p.) and methadone (0.1, 0.3, 1, and 3 mg/kg i.p.) allowed to combine them in equieffective doses and to determine their interaction by isobolographic analysis. Magnesium sulfate, copper sulfate and methadone dose-dependently decreased the nociceptive response evoked by formalin injection, the respective ED50 being 76.38, 1.18, and 0.50 mg/kg i.p. Isobolographic analysis showed a superadditive interaction for magnesium and methadone. Indeed, despite that both ED50 are obviously equieffective, the ED50 for the MgSO4/methadone combination contained less than one third of the methadone having the ED50 for methadone alone. For the CuSO4/methadone combination, the interaction was only additive. Extrapolated to clinical settings, the results suggest that magnesium salts might be used to improve synergistically the efficacy of methadone in neuropathy, which would allow to reduce the dose of methadone and its associated side effects.
Introduction
Among the variety of opioid drugs currently used to manage cancer and chronic non-cancer pains, methadone is well positioned because: (i) methadone has no known active metabolites, it is well absorbed by oral and rectal routes, suffers less first pass metabolization and has a lesser interindividual variation in bioavailability than oral morphine (Gourlay et al., 1986); (ii) analgesic efficacy during chronic dosing is greater (Davis and Walsh, 2001) and opioid escalation is lesser (Mercadante et al., 1998) in patients treated with methadone than those treated with morphine; (iii) methadone displays antagonistic properties at the N-methyl-D-aspartate (NMDA) receptor (Ebert et al., 1995; Gorman et al., 1997), which is known to be involved in chronic pain; and (iv) methadone acts as an inhibitor of 5-hydroxytryptamine and norepinephrine uptake (Codd et al., 1995), a mechanism classically associated to pain control by tricyclic antidepressants, particularly important in the case of neuropathic pain.
Nevertheless, besides to those adverse effects that are common for all opioids (i.e., addiction, sedation, nausea, and respiratory depression), methadone has some drawbacks. First, its long and variable half-life can lead to accumulation and associated side effects in some patients, such as respiratory arrest in patients without prior opioid treatment and in those with a history of sleep apnea, severe asthma or respiratory failure (Bruera and Sweeney, 2002; Brown et al., 2004). Second, methadone interacts with other drugs that inhibit or activate the cytochrome P450 system, which is involved in the methadone metabolism (Iribarne et al., 1996; Herrlin et al., 2000). Third, weight gain (Dyer and White, 1997) and sexual dysfunction (Spring et al., 1992) are commonly reported among patients on methadone maintenance. Although these drawbacks may discourage the use of methadone for chronic non-cancer pain, therapeutic methadone use has increased 167.0% from 2000 to 2014 globally and 205.2% in the United States (Manchikanti et al., 2018). The drawbacks of chronic methadone administration are clearly related to dosing and treatment duration both for cancer (McPherson et al., 2018) and non-cancer (Els et al., 2017) pain. Consequently, the possibility of enhancing the analgesic effect of methadone (and therefore of reducing its dose) by combining it with other non-opioid antinociceptive drugs could significantly help to reduce the side effects and risks associated with the therapeutic use of this drug. Combination of methadone with tricyclic antidepressants (Banks et al., 2010; Schreiber et al., 2014), methylphenidate (Schreiber et al., 2017), delta9-tetrahydrocannabinol (Cichewicz et al., 1999) and ketamine (Pelissier et al., 2003) have already been reported, but almost in acute preclinical pain models. Methadone/ketamine (de Godoy et al., 2013) and methadone/ibuprofen (Ferrer-Brechner and Ganz, 1984) combinations have been used as antinociceptive agents in the clinic, but controlled studies reported that ketamine alone is more effective than the methadone/ketamine combination (Rigo et al., 2017) and that at the long-term nonsteroidal anti-inflammatory drugs (NSAIDs) can result in increased risk of gastrointestinal and cardiovascular side-effects (Wehling, 2014; Ho et al., 2018).
Here, we propose to study whether the antinociceptive efficacy of methadone could be potentiated by magnesium and copper salts in a preclinical model of chronic pain. Magnesium ions are coactivators of the activity of many enzymes and regulate the conductance of the NMDA receptor channel in the central nervous system (Swaminathan, 2003; Seo and Park, 2008), which play a crucial role in the mechanisms of chronic pain. Clinical trials showed that systemic Mg2+, used as adjuvant medication of opioids (mostly morphine, but also fentanyl and tramadol), significantly reduced opioid consumption in acute intraoperative and post-operative pain complaints (Bujalska-Zadrożny et al., 2017), but no similar data exist regarding chronic pain syndromes (Kreutzwiser and Tawfic, 2019). On the other hand, copper has demonstrated antinociceptive properties against various pain modalities in preclinical studies hot plate, tail flick tests, and in the writhing test (Tamba et al., 2013), formalin test (Cazanga et al., 2018), adjuvant arthritic rat pain model (Okuyama et al., 1987), and that it may enhance the peripheral analgesic effect of fenoprofen (Gumilar et al., 2012)) and the central analgesic effect of ketamine (Cazanga et al., 2018), but copper has not yet been tested as antinociceptive agent in humans via systemic route.
Thus, the aim of the present study was to evaluate the antinociceptive effect of magnesium and copper salts in a neuropathic mice model using the intraplantar formalin test, and to examine whether their effects may interact synergistically with methadone-induced antinociception using isobolographic analysis.
Materials and Methods
Animals
Naïve outbred CF1 male adult mice weighing 28–33 g were used for the study. Animals were housed 6 per cage and maintained with controlled temperature (21 ± 1°C) and light conditions (12:12 h light-dark cycle, lights on at 7:00 am). In total, one hundred and five mice were used in the experiments. Animals had ad libitum access to food and water and were allowed to habituate to the housing facility for one week before the beginning of experiments. The experimental procedure was achieved during the light phase, between 9:00 am and 12:00 am, in a quiet room. The housing conditions and experimental procedures were approved by the Bioethics Committee of the University of Santiago de Chile, and were in agreement with the ethical guidelines published by the International Association for the Study of Pain and with the Guide for the Care and Use of Laboratory Animals of NIH (National Research Council, 2011). To determine the number of required mice in each experimental group, we conducted a sample size power analysis by using the G*Power 3 Software (Faul et al., 2007). All the experimental measurements were performed in blinded condition. Each mouse was sacrificed at the end of the experiment by a carbon dioxide overdose.
Neuropathy
Neuropathy was induced by using the spared nerve injury mice model proposed by Omori et al. (2009), which is a modification of the spared nerve injury rat model described by Decosterd and Woolf (2000) resulting in early, prolonged, and robust changes in mechanical sensitivity and thermal responsiveness that closely mimic many features of clinical neuropathic pain. In the original rat model of Decosterd and Woolf (2000), two of the three terminal distal branches of the sciatic nerve were axotomized (the tibial and common peroneal nerves), sparing only one (the sural nerve), whereas in the present mice version of the model only the sural nerve was transected, sparing the tibial and common peroneal nerves. Since the sural nerve contains almost no motor fibers (Peyronnard and Charron, 1982; Schmalbruch, 1986), this procedure allowed to generate a neuropathic pain model in mice (Omori et al., 2009) and rats (Bravo et al., 2014) in which the posture and motor functions of the hindpaw are preserved, without affecting the evaluation of pain-like responses of the paw. Briefly, animals were anesthetized with 400 mg/kg i.p. of 7% chloral hydrate solution (w/v) and a skin incision approximately 10 mm long was made in the right hindpaw at the level of sciatic nerve. The subcutaneous tissue was dissected, and the biceps femoris muscle was freed from the pelvic and vertebral heads to expose the sciatic nerve. The nerve path was then followed until its split into three branches: the sural, common peroneal, and tibial nerves. The sural nerve was cut 2 mm from its emergence, and the overlying tissues were sutured in layers. During the following 2 days after surgery, animals were daily given 3 mg/kg s.c. of the analgesic ketoprofen and 5 mg/kg s.c. of the antimicrobial agent enrofloxacin. The neural lesion described above resulted in thermal hyperalgesia of the mouse hindpaw, as measured in the hot-plate test, that persisted for at least 28 days (data not shown).
Drugs
Magnesium sulfate heptahydrate (Fresenius Kabi, Santiago, Chile) and copper sulfate pentahydrate (Winkler, Santiago, Chile) were dissolved in physiological saline (0.9% NaCl) and administered via i.p. route, in a volume 0.5 ml. Doses of magnesium sulfate were 10, 30, 100, and 300 mg/kg (four groups of five mice each) and doses of copper sulfate were 0.1, 0.3, 1, and 3 mg/kg (four groups of five mice each). Methadone chlorhydrate (Laboratorio Biosano, Santiago, Chile) was administered i.p. (0.5 ml) at doses of 0.1, 0.3, 1, and 3 mg/kg (four groups of five mice each). Controls groups (five mice) received 0.5 ml of 0.9% NaCl. Thus, each mouse was given only one injection of a determined drug dose or of the solvent used.
Behavioral Assessment: Formalin Test
The intraplantar formalin test was chosen instead mechanical or thermal pain testing, because these later are mostly based on evoked withdrawal responses that do not measure pain itself but the threshold of hyperactive reflexes that accompany pain. To run the formalin test in mice with spared nerve injury, it was used a lower formalin concentration (0.25%) than those regularly utilized for formalin testing in healthy mice (2 to 5%). This low formalin concentration gives rise only to Phase I response (direct activation by formalin of the transient receptor potential ankyrin 1, TRPA1, existing in nociceptive primary afferents) but not to Phase II response (secondary activation of nociceptive primary afferents by molecules released by neighboring injured cells, via formalin covalent crosslinks to proteins that disrupt cells membranes) when applied to neuropathic mice (see below in Results, and also see Abe et al., 2011), thereby minimizing interaction during pain testing with the neurogenic inflammation process that occurs in most models of experimental neuropathy with peripheral lesion (Meacham et al., 2017). Therefore, with this paradigm of low formalin concentration, the pain response is mostly due to direct nociceptor stimulation, as occurs with mechanical and thermal nociceptive stimuli, but with the advantage of measuring the pain response itself to nociceptor activation and not merely the threshold for eliciting a pain response as usually occurs with mechanical and thermal testing.
The animals were acclimatized in the experimental room 2 h before beginning of experiments. Fifteen min before the behavioral evaluation, mice were given a single injection either of saline (controls), MgSO4 alone, CuSO4 alone, methadone alone, MgSO4 plus methadone, or CuSO4 plus methadone. Behavioral testing was carried out by a researcher who was blind to the particular drug treatment given to each animal. For this, mice were situated into an acrylic cylinder (25 cm high x 25 cm in diameter) enclosed by two mirrors placed perpendicularly to each other. Previous to testing, each mouse was positioned into the cylinder for 10 min to acclimatize and minimize stress. Mice were then gently restrained and 20 μl of 0.25% formalin solution were injected either into the plantar surface of the right hindlimb. The intraplantar formalin test was performed as described by Cazanga et al. (2018). The nociceptive behavior evaluated was the licking/biting of the injected limb and the test was run during a 35-min observation period starting from the time of formalin administration, which was divided into seven blocks of 5-min each. A nociceptive score was determined for each block by measuring the number of seconds that the animals spent the nociceptive behavior (licking/biting the formalin-injected limb).
As it is known (e.g., Zhao et al., 2003), the time course of the nociceptive response to formalin is usually studied by plotting the individual nociceptive scores obtained during the first 10 min following intraplantar formalin injection (the so-called Phase I) and between 10 and 35 min after formalin (the so-called Phase II). Since no Phase II response could be observed with the intraplantar injection of 0.25% formalin (see the time-course of nociceptive scores in Figures 1 and 2), in the present study the data was analyzed as compiled for the 35-min total time of observation. By summating the seven individual nociceptive scores (NS) recorded during the total time of observation, a global nociceptive score (∑NS) was obtained. This was subsequently used to calculate the antinociceptive effect of each dose of drug, as:
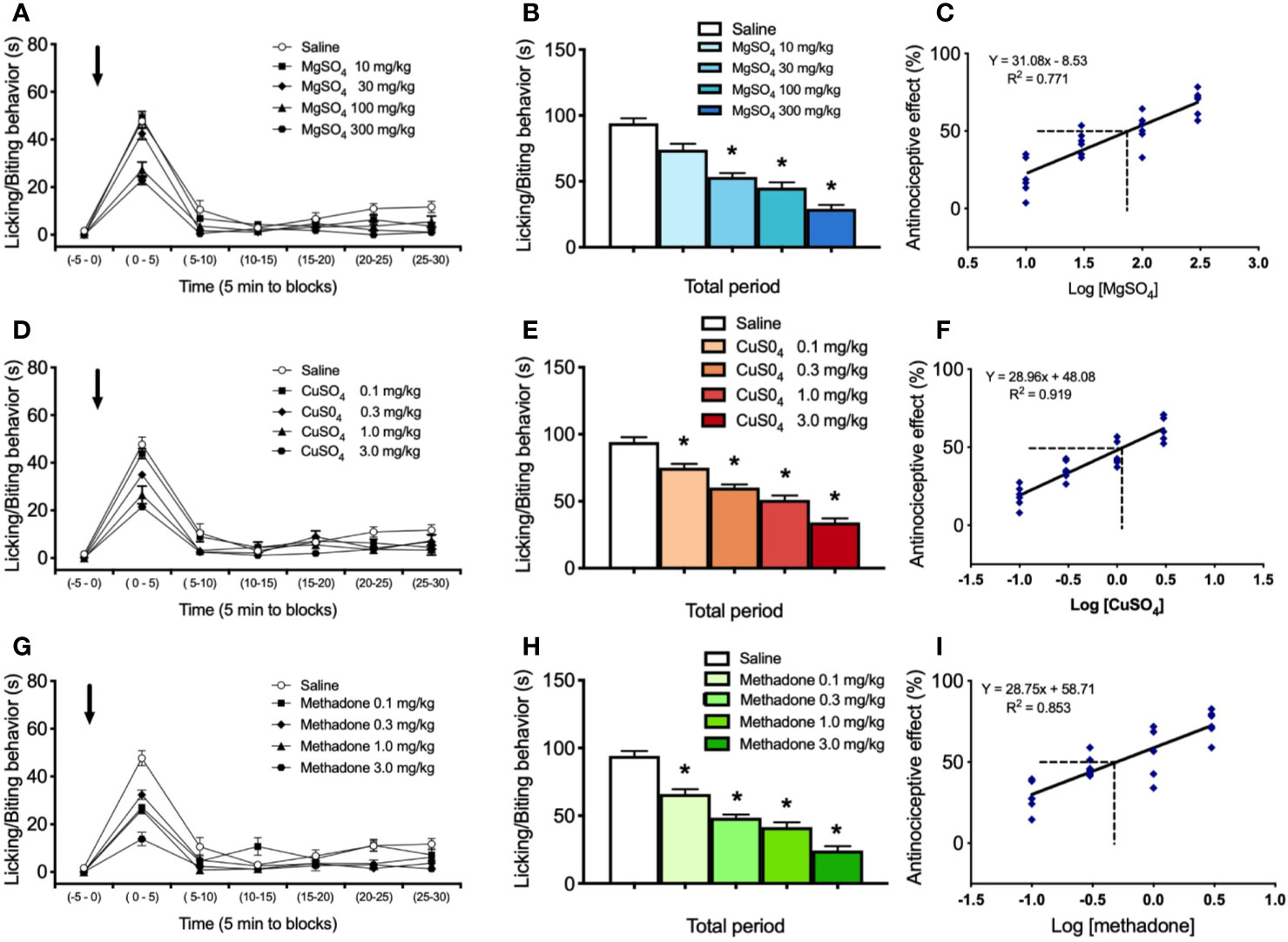
Figure 1 Effect of i.p. administration of saline, MgSO4, CuSO4, or methadone on licking/biting behavior elicited by intraplantar administration of 0.25% formalin in the neuropathic hindlimb of mice. Saline, MgSO4, CuSO4, or methadone were administered as a single i.p. injection 15 min before intraplantar formalin administration. (A) Time-course of effects of saline and 10, 30, 100, and 300 mg/kg of MgSO4 in nociceptive response, expressed as seconds spending licking/biting activity. (B) Global nociceptive score (∑NS) of licking/biting behavior for Total time of observation in intraplantar formalin test after i.p. administration of saline or increasing doses of MgSO4. (C) Dose-response data representing the antinociceptive effect (%) of MgSO4, expressed as dose logarithm. The ED50 was calculated from the regression line and is shown with segmented line. (D) Time-course of effects of saline and 0.1, 0.3, 1, and 3 mg/kg of CuSO4 in nociceptive response, expressed as seconds spending licking/biting activity. (E) Global nociceptive score (∑NS) of licking/biting behavior for Total time of observation in intraplantar formalin test after i.p. administration of saline or increasing doses of CuSO4. (F) Dose-response data representing the antinociceptive effect (%) of CuSO4, expressed as dose logarithm. The ED50 was calculated from the regression line and is shown with segmented line. (G) Time-course of effects of saline and 0.1, 0.3, 1, and 3 mg/kg of methadone in nociceptive response, expressed as seconds spending licking/biting activity. (H) Global nociceptive score (∑NS) of licking/biting behavior for Total time of observation in intraplantar formalin test after i.p. administration of saline or increasing doses of methadone. (I) Dose-response data representing the antinociceptive effect (%) of methadone, expressed as dose logarithm. The ED50 was calculated from the regression line and is shown with segmented line. For (A, D, G): Arrows indicate formalin injection. For (B, E, H): Each bar represents the mean ± SEM of 5 independent determinations. Intergroup statistics were compared by One-way ANOVA followed by Bonferroni’s multiple comparison post hoc test (*p < 0.01). For (C, F, I): The equation for linear regression and the goodness of fit (R2) are shown in each graph.
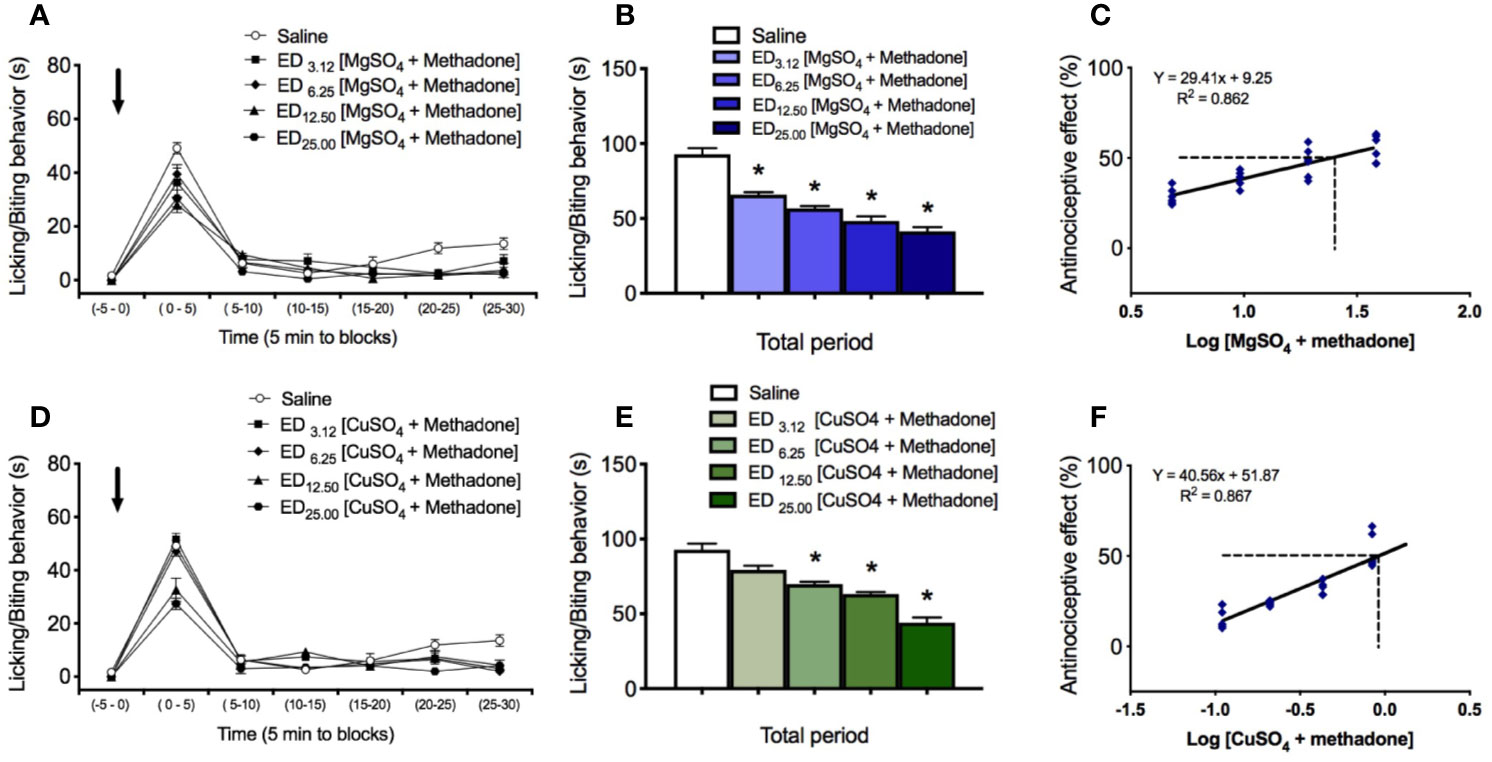
Figure 2 Effect of i.p. administration of saline and of the combinations of either magnesium sulfate or copper sulfate with methadone, on licking/biting behavior elicited by intraplantar administration of 0.25% formalin in the neuropathic hindlimb of mice. Saline and combinations MgSO4/methadone or CuSO4/methadone, were administered as a single i.p. injection 15 min before intraplantar formalin administration. (A) Time course of effects of saline and MgSO4/methadone combinations administered in fixed ratios of 1/2, 1/4, 1/8, and 1/16 of their respective ED50, expressed as seconds spending licking/biting activity. (B) Global nociceptive score (∑NS) of licking/biting behavior for Total time of observation in intraplantar formalin test after i.p. administration of saline or increasing equieffective doses of MgSO4/ketamine combination. (C) Dose-response data representing the antinociceptive effect (%) of MgSO4/methadone combination, expressed as dose logarithm. The respective ED50 were calculated from the regression lines and are shown in each figure with segmented line. (D) Time course of effects of saline and CuSO4/methadone combinations administered in fixed ratios of 1/2, 1/4, 1/8, and 1/16 of their respective ED50, expressed as seconds spending licking/biting activity. (E) Global nociceptive score (∑NS) of licking/biting behavior for Total time of observation in intraplantar formalin test after i.p. administration of saline or increasing equieffective doses of CuSO4/ketamine combination. (F) Dose-response data representing the antinociceptive effect (%) of CuSO4/methadone combination, expressed as dose logarithm. The respective ED50 were calculated from the regression lines and are shown in each figure with segmented line. For (A, D): Arrows indicate formalin injection. For (B, E): Each bar represents the mean ± SEM of five independent determinations. Intergroup statistics were compared by One-way ANOVA followed by Bonferroni’s multiple comparison post hoc test (*p < 0.01). For (C, F): The equation for linear regression and the goodness of fit (R2) are shown in each graph.
where ∑NSsaline is the algebraic sum of the scores under saline and ∑NSdrug is the algebraic sum of the scores under drug. Plotting the Antinociceptive effect (%) against log dose allowed for obtaining the ED50 (effective dose that produce the 50% of the maximal effect) by linear regression analysis.
Isobolographic Analysis
Evaluation of the interactions of MgSO4 and CuSO4 with methadone was performed by using isobolographic analysis (Tallarida, 2000; Tallarida, 2006). The isobologram is a graphic method that involves calculating the theoretical additive dose for each level of effect and their statistical comparison with the combination dose that causes the same effect experimentally. Equieffective doses of the drugs alone are necessary to calculate the expected dose in a combination. To this end, for each drug we defined the dose that produces 50% of maximal effect (ED50) by using a linear regression analysis from the dose-response curve of four increasing doses of MgSO4, CuSO4, or methadone, as stated above. Once the ED50 of each of the three drugs was obtained, a graph was constructed by placing in the x-axis the ED50 of MgSO4 or that of CuSO4, and on the y-axis the ED50 of methadone. The union of the two points by a straight line (isobole), also known as line of additivity, allowed establishing the expected theoretical additivity ED50 of each ion salt with methadone in the middle of the isobole. Then, a dose–response curve for the co-administration of MgSO4 with methadone (four groups of five mice each) or CuSO4 with methadone (four groups of five mice each) was carried out, by administering the combination in fixed ratios of 1/2, 1/4, 1/8, and 1/16 of their respective ED50. Each combination of each ion salt with methadone was administered as a single i.p. injection, 15 min before the intraplantar formalin injection. The relation between the experimental value (experimental ED50) of the combination with to the theoretical value (theoretical additivity ED50) determines the type of interaction: if the value is located under the line of additivity and it is statistically different from the theoretical value, the interaction is synergistic or superadditive (effect greater than the sum of the individual effects of drugs); if it is not statistically different from the theoretical value, the interaction is simple additivity (equal effect than the sum of each drug); conversely, if the experimental value is located above the line of additivity and is statistically different from the theoretical value, it is a subadditive or antagonistic interaction. This relation can be calculated by the interaction index (γ = experimental ED50/theoretical additive ED50) between the drugs tested. This index, when smaller than 1 corresponds to a synergistic interaction, when equal to 1 corresponds to an additive interaction, and when greater than 1 is an antagonistic interaction.
Analysis of Results
The results of scores obtained were expressed as means ± S.E.M., while the computed ED50 values included the 95% confidence intervals. To characterize the interaction between the drugs studied, an isobolographic analysis was performed using a custom Microsoft Excel macro program based on the method described by Ronald J. Tallarida (2000; 2006; 2016), and the interaction index calculated. The results were examined using Student’s t-test for unpaired data. To compare the effect of the different doses of each drug or their combinations, the results were examined using one-way analysis of variance (ANOVA) followed by the Bonferroni post hoc multiple comparisons test. The statistical analyses were made by using Prism 7.0 Software (GraphPad Software Inc, San Diego, CA). Significance was accepted at an alpha level of 0.05.
Results
Antinociceptive Effect of Magnesium Sulfate, Copper Sulfate, and Methadone in Neuropathic Mice
Intraplantar administration of 0.25% formalin in the neuropathic hindpaw of mice under drug-free condition (saline controls) induced a score of nociceptive licking/biting behavior amounting to 91.9 ± 4.1 s for the total time of observation (n=5; Figure 1B). Administration of magnesium sulfate i.p. induced a dose-dependent reduction of the nociceptive response induced by 0.25% formalin (Figure 1A). Indeed, the licking/biting behavior scores amounted to 74.0 ± 5.8 s, 55.8 ± 3.7 s, 45.6 ± 4.6 s, and 32.1 ± 3.5 s, for doses of 10, 30, 100, and 300 mg/kg of MgSO4, respectively (n=5 for each group; Figure 1B), all the nociceptive scores being significantly lower to that obtained after saline administration (*p < 0.01). The calculated value of ED50 for MgSO4 was 76.38 mg/kg with a 95% confidence interval (95% CI) of 55.21 mg/kg to 105.66 mg/kg (Figure 1C).
Administration of copper sulfate i.p. dose-dependently reduced the formalin-induced nociceptive response (Figure 1D), the licking/biting behavior scores amounting to 75.1 ± 3.7 s, 59.8 ± 3.3 s, 49.6 ± 4.1 s, and 34.1 ± 3.4 s, for doses of 0.1, 0.3, 1, and 3 mg/kg of CuSO4, respectively (n=5 for each group; Figure 1E). All these nociceptive scores were significantly lower to that obtained after saline administration (*p < 0.01). The calculated ED50 for CuSO4 was 1.18 mg/kg with a 95% CI of 0.98 mg/kg to 1.42 mg/kg (Figure 1F).
Methadone i.p. administration induced a dose-dependent reduction of the nociceptive licking/biting scores induced by formalin administration (Figure 1G). Nociceptive scores were 67.4 ± 4.9 s, 49.0 ± 2.6 s, 41.2 ± 4.2 s, and 25.2 ± 3.2 s, for doses of 0.3, 1, 1, and 3 mg/kg of methadone, respectively (n=5 for each group; Figure 1H). All doses of methadone produced significantly lower nociceptive scores compared to saline (*p < 0.01). The ED50 value for methadone was 0.50 mg/kg with a 95% confidence interval (95% CI) of 0.33 mg/kg to 0.75 mg/kg (Figure 1I).
Antinociceptive Effect of the Combinations of Either Magnesium Sulfate or Copper Sulfate With Methadone in Neuropathic Mice
The administration of the combination of magnesium sulfate and methadone, in equieffective proportions of their respective ED50, induced a dose-dependent reduction of the rubbing/scratching behavior scores in the intraplantar formalin test (Figure 2A). For the total period of observation after formalin injection, association of MgSO4 and methadone administered in fixed ratios of 1/2, 1/4, 1/8, and 1/16 of their respective ED50, produced nociceptive licking/biting scores of 67.1 ± 2.8 s, 58.2 ± 2.6 s, 47.6 ± 3.3 s, and 38.0 ± 3.8 s, respectively (n=5 for each group; Figure 2B). All these nociceptive scores were significantly lower than the obtained under saline administration (*p < 0.001). The experimental ED50 for the MgSO4/methadone association was 24.29 mg/kg (with 95% CI from 18.20 mg/kg to 32.41 mg/kg, Figure 2C), which can be decomposed in 24.13 mg/kg MgSO4 plus 0.16 mg/kg methadone. Isobolographic analysis for the administration of MgSO4/methadone combination showed that the experimental ED50 was significantly lower than the theoretical additive ED50 (p < 0.05, two-tailed Student’s t-test), with an interaction index γ = 0.632, which means a superadditive effect (Figure 3A).
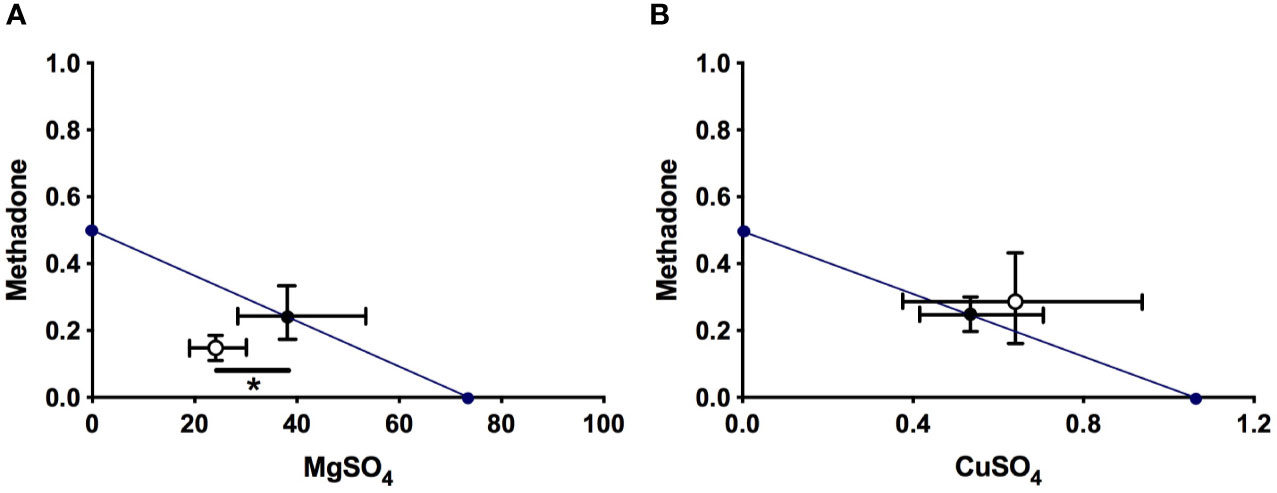
Figure 3 Isobolograms of interaction for MgSO4/methadone (A) and CuSO4/methadone (B) combinations in neuropathic mice, for total observation period in the intraplantar formalin test. The black circle on the straight line represents the point of theoretical additivity of the combination, whereas the white circle corresponds to the experimental point. In (A): The experimental point was significantly different from the theoretical point (mean ± SEM; *p < 0.05, two-tailed Student t test), indicating superadditive interaction. The standard errors for MgSO4 and methadone are resolved into MgSO4 (abscissa scale) and methadone (ordinate scale) components and shown by horizontal and vertical bars, respectively. In (B): The experimental point was not significantly different from the theoretical point (mean ± SEM; not significant, two-tailed Student t test), indicating an additive interaction of both drugs. The standard errors for CuSO4 and methadone are resolved into CuSO4 (abscissa scale) and methadone (ordinate scale) components and shown by horizontal and vertical bars, respectively.
The administration of the combination of copper sulfate and methadone, in fixed ratios of 1/2, 1/4, 1/8, and 1/16 of their respective ED50, produced nociceptive licking/biting scores of 67.1 ± 4.8 s, 58.2 ± 3.6 s, 47.6 ± 3.3 s, and 38.0 ± 4.8 s, respectively (n=5 for each group; Figure 2E). The three higher doses of combined copper sulfate and methadone administered led to significantly lower nociceptive scores, compared to saline administration (*p < 0.01), and the calculated experimental ED50 for the CuSO4/methadone association was 0.90 mg/kg (with 95% CI from 0.68 mg/kg to 1.32 mg/kg, Figure 2F), which can be decomposed in 0.63 mg/kg CuSO4 plus 0.27 mg/kg methadone. Isobolographic analysis for the administration of MgSO4/methadone combination showed that the experimental ED50 did not significantly differ from the theoretical additive ED50 (two-tailed Student’s t-test), with an interaction index γ = 1.148, which means simple additivity (Figure 3B).
Discussion
The present results showed that both magnesium sulfate and copper sulfate produced dose-dependent antinociceptive effects in the intraplantar formalin test. This is in agreement with expectations since magnesium and copper salts have been found to induce dose-dependent antinociception in the hot-plate, tail-flick, and writhing tests (Tamba et al., 2013). Besides, the antinociceptive effects showed by magnesium and copper ions also coincide with those obtained in other studies using formalin-induced pain, where systemic magnesium improved the antinociceptive effect of ketamine (Vujović et al., 2017) and copper-NSAIDs complexes exhibited higher antinociceptive effect than NSAIDs alone (Gumilar et al., 2012). The foregoing results also showed that the MgSO4/methadone and CuSO4/methadone combinations produced higher antinociception than methadone alone, an opiate agonist that has proven to be effective in thermal and mechanical pain models (Lemberg et al., 2006), as well as in chemonociception (Miranda et al., 2014) and different models of neuropathic pain (Erichsen et al., 2005).
The main and most important result of the present study was that MgSO4 and methadone interacted synergistically in the intraplantar formalin pain model, which means that there was a potentiation of the antinociceptive effect of the drugs when given in combination. In fact, the ED50 of methadone alone was 0.50 mg/kg while the equieffective dose of the MgSO4/methadone combination only contained 0.16 mg/kg methadone, which means that the addition of MgSO4 allowed the methadone content of the dose to be reduced to less than one third. If extrapolated to humans, this might be an important finding because the side-effects of methadone in clinical settings are related to dosing and treatment duration, both for cancer (McPherson et al., 2018) and non-cancer (Els et al., 2017) pains. Indeed, according to the guidelines from the American Pain Society (Chou et al., 2014) and from the experts group of the Hospice and Palliative Care (McPherson, 2016), rotation to methadone of opioid-tolerant patients with cancer pain should be based on dose calculations, as exact opioid/methadone ratios. The same apply to chronic non-cancer pain, where the risk for addiction increases with increasing opioid doses (Huffman et al., 2015), and sleep-disordered breathing and respiratory depression may result in opioid-associated deaths demonstrating a clear relationship to dose (Walker et al., 2007; Jungquist et al., 2012).
It seems worth to remark that the superadditive interaction between MgSO4 and methadone, detected by isobolographic analysis upon intraplantar formalin testing, originated from parallel regression lines obtained in the dose-response plots of the individual drugs, meaning that the potency ratio for these two drugs remained constant during testing of formalin-induced pain in neuropathic rats (Tallarida, 2000; Tallarida, 2006; Tallarida, 2016). Theoretically, superadditivity in the effect of two simultaneously administered antinociceptive drugs implies that the combined molecules act on anatomically and/or functionally different substrates for nociceptive processing, which may represent different neurons, different receptors in the same neuron, or even different sites of binding in the same receptor. In this regard, it is well known that magnesium induce antinociception by antagonistic binding to NMDA receptors (Traynelis et al., 2010), while the antinociceptive effect of methadone can be explained by both agonism at µ opiate receptors and antagonism on NMDA receptors (Gorman et al., 1997).
Methadone is a potent inhibitor of [3H]MK-801 binding, a specific uncompetitive NMDA receptor antagonist, with a Ki of 0.85 ± 0.31 µM (Ebert et al., 1995). Gorman et al. (1997) reported moderate affinity, but still in the low µM range, for the displacement of [3H]MK-801 by l-, d-, and dl-methadone (Ki of 3.4 ± 0.3, 7.4 ± 1.2, and 8.3 ± 1.2, respectively) from non-competitive NMDA receptor sites in the rat forebrain. More recently, Matsui and Williams (2010) showed that the NMDA current induced by iontophoretic application of L-aspartate in locus coeruleus neurons was dose-dependently inhibited by l/d methadone with an IC50 value for l/d-methadone of 3.5 ± 0.3 µM, which was statistically similar to the IC50 of d-and l- methadone enantiomers. Since methadone blocked the inward but not the outward current in the NMDA channel, it can be concluded that, in addition to µ-opioid receptor binding, at low µM concentration methadone could act as a non stereoselective, uncompetitive, voltage-dependent pore blocker of the NMDA receptor. Despite that the MgSO4/methadone combination reported here was synergistic, it seems apparent that the effects of Mg2+ and methadone were redundant at the NMDA receptor, because both magnesium ions (Traynelis et al., 2010) and methadone (Gorman et al., 1997; Matsui and Williams, 2010) are open channel blockers that act as voltage-dependent uncompetitive NMDA receptor antagonists in the same site of the NMDA channel. In such a case, one drug substitutes for the other and only additivity should be expected when the two drugs are given simultaneously. Thus, it is likely that the synergy of the MgSO4/methadone combination reported here arose from the interaction between the blocking properties of magnesium ions in the NMDA receptor channels and the agonistic properties of methadone in µ opioid receptors. With regard to this, it has been reported that binding of opioid agonists and antagonists in brain homogenates is allosterically promoted by Mg2+ in a concentration-dependent manner (Rodriguez et al., 1992), which could be at the base of the synergistic effect of magnesium on methadone-induced antinociception. A rather similar synergistic interaction between methadone and ketamine in neuropathic rats has previously been reported (Pelissier et al., 2003), but the translational potential of such a combination is likely to be impaired by the well-known undesirable psychomimetic effects of ketamine (Persson, 2013). In contrast, magnesium is cheap and well tolerated by oral route, as children have been safely treated from chronic constipation with 2 ml/kg daily of milk of magnesia, i.e., 160 mg magnesium hydroxide/kg/day (Loening-Baucke and Pashankar, 2006).
As it is known, subcutaneously injected formalin into the mouse paw gives rise to phase I response by direct activation of nociceptive primary afferents via TRPA1 channels (McNamara et al., 2007), and to phase II response (or inflammatory phase) corresponding to a secondary activation of nociceptive primary afferents by histamine, bradykinin, cytokines, and substance P, among others mediators released by inflammatory cells (Zouikr et al., 2015). Various forms of experimental neuropathy induced by peripheral injury (Kingery et al., 1999; Botz et al., 2013; Gallo et al., 2017) have been associated to neurogenic inflammation, eliciting the release of substance P, calcitonin gene-related peptide, neurokinin A, endothelin-3, cytokines, among others cellular mediators of inflammation. Since both formalin administration and the neuropathic process upregulate rather similar inflammatory mediators, this makes difficult the interpretation of nociceptive data during phase II response. Therefore, we utilized a paradigm of low formalin concentration, as proposed by Abe et al., 2011, where only phase I pain response can be observed.
Unlike the superadditive interaction between magnesium salt and methadone, copper ions give rise only to an additive effect in the intraplantar formalin test when administered together with methadone. This different interaction, obtained through isobologram analysis of data, could be related to the already reported different mode of binding of Cu2+ on NMDA channels, where copper acts as a high-affinity NMDA receptor antagonist characterized by a voltage-independent mechanism of action (Herrlin et al., 2000).
Although it remains yet uncertain the mechanism underlying the ability of magnesium sulfate to exert a synergistic action upon the methadone antinociceptive effect, this issue could constitute a potential basis for future clinical applications addressed to lower methadone dosing together with a lowering of its side-effects. As pointed out elsewhere (Erichsen et al., 2005), it is not possible to determine synergism in humans due to scientific, practical and ethical reasons, and thus prior to testing drug interaction in clinical trials, studies on preclinical drug combinations should be carried out in animals to obtain the basis and rationale for further studies in humans. Among opioids, methadone should be well positioned for treat neuropathic pain because of its unique ability to target NMDA receptors in addition to its well-known effect on µ opioid receptors. However, therapeutic guidelines relegate strong opioids, including methadone, to third-line therapy in neuropathic pain mainly because of safety concerns (Finnerup et al., 2015). Drug combination strategies aimed to reduce methadone dosing—and therefore its side-effects—could be a promising therapeutic approach to optimize opioid analgesia under neuropathic pain conditions, provided the drug co-administered with methadone does not give rise to important side-effects by its own. To this end, magnesium salts probably represent the best alternative, since the European Food Safety Authority (EFSA) of the European Union states that the upper limit for magnesium (i.e., the daily dose that does not produce any observable adverse effect in healthy adult humans) is as high as 350 mg, while toxic hypermagnesemia is only seen at oral doses greater than 2500 mg daily (Scientific Committee on Food, Scientific Panel on Dietetic Products, Nutrition and Allergies, 2006). Although further studies are necessary to examine in detail the mechanism underlying the synergistic interaction between magnesium ions and methadone, it can be concluded that this association could represent a potential therapeutic strategy aimed to treat some forms of chronic pain in humans, which deserves more investigation in clinical settings.
Data Availability Statement
The datasets generated for this study are available on request to the corresponding author.
Ethics Statement
The animal study was reviewed and approved by Bioethics Committee of the University of Santiago de Chile.
Author Contributions
TP and LC provided ideas or concepts for definition of intellectual context, particularly designed and performed the experiments. VG, VC, and TP performed research. AH, TP, and LC contributed new reagents/analytic tools. VG, AH, TP, and LC analyzed data. AH and LC wrote the paper. All authors of this paper have read and approved the final version of the manuscript.
Funding
This work was supported by the Fondecyt Project (grant 1181622) and the Centers of Excellence with Basal/Conicyt financing, CEDENNA (grant AFB180001).
Conflict of Interest
The authors declare that the research was conducted in the absence of any commercial or financial relationships that could be construed as a potential conflict of interest.
Acknowledgments
We thank Ms. Cristina Arenas and Mr José López for technical support and animal care.
References
Abe, K., Fujii, Y., Nojima, H. (2011). Evaluation of hyperalgesia in spared nerve injury model using mechanical, thermal, and chemical stimuli in the mouse. Neurol. Res. 33, 656–662. doi: 10.1179/1743132810Y.0000000019
Banks, M. L., Rice, K. C., Negus, S. S. (2010). Antinociceptive interactions between mu-opioid receptor agonists and the serotonin uptake inhibitor clomipramine in rhesus monkeys: role of mu agonist efficacy. J. Pharmacol. Exp. Ther. 335, 497–505. doi: 10.1124/jpet.110.169276
Botz, B., Imreh, A., Sándor, K., Elekes, K., Szolcsányi, J., Reglődi, D., et al. (2013). Role of Pituitary Adenylate-Cyclase Activating Polypeptide and Tac1 gene derived tachykinins in sensory, motor and vascular functions under normal and neuropathic conditions. Peptides 43, 105–112. doi: 10.1016/j.peptides.2013.03.003
Bravo, D., Ibarra, P., Retamal, J., Pelissier, T., Laurido, C., Hernandez, A., et al. (2014). Pannexin 1: a novel participant in neuropathic pain signaling in the rat spinal cord. Pain 155, 2108–2115. doi: 10.1016/j.pain.2014.07.024
Brown, R., Kraus, C., Fleming, M., Reddy, S. (2004). Methadone: applied pharmacology and use as adjunctive treatment in chronic pain. Postgrad. Med. J. 80, 654–659. doi: 10.1136/pgmj.2004.022988
Bruera, E., Sweeney, C. (2002). Methadone use in cancer patients with pain: a review. J. Palliat. Med. 5, 127–138. doi: 10.1089/10966210252785097
Bujalska-Zadrożny, M., Tatarkiewicz, J., Kulik, K., Filip, M., Naruszewicz, M. (2017). Magnesium enhances opioid-induced analgesia - What we have learnt in the past decades? Eur. J. Pharm. Sci. 99, 113–127. doi: 10.1016/j.ejps.2016.11.020
Cazanga, V., Hernandez, A., Morales, B., Pelissier, T., Constandil, L. (2018). Antinociception induced by copper salt revisited: Interaction with ketamine in formalin-induced intraplantar and orofacial pain in mice. J. Oral. Facial Pain Headache 32, 247–257. doi: 10.11607/ofph.1961
Chou, R., Cruciani, R. A., Fiellin, D. A., Compton, P., Farrar, J. T., Haigney, M. C., et al. (2014). Methadone safety: a clinical practice guideline from the American Pain Society and College of Problems on Drug Dependence, in collaboration with the Heart Rhythm Society. J. Pain 15, 321–337. doi: 10.1016/j.jpain.2014.01.494
Cichewicz, D. L., Martin, Z. L., Smith, F. L., Welch, S. P. (1999). Enhancement mu opioid antinociception by oral Δ9-tetrahydrocannabinol: dose-response analysis and receptor identification. J. Pharmacol. Exp. Ther. 289, 859–867.
Codd, E., Shank, R., Schupsky, J., Raffa, R. (1995). Serotonin and norepinephrine uptake inhibiting activity of centrally acting analgesics: structural determinants and role in antinociception. J. Pharmacol. Exp. Ther. 274, 1263–1270.
Davis, M. P., Walsh, D. (2001). Methadone for relief of cancer pain: a review of pharmacokinetics, pharmacodynamics, drug interactions and protocols of administration. Support Care Cancer 9, 73–83. doi: 10.1007/s005200000180
de Godoy, M. C., Dalmolin, G. D., Rigo, F. K., Rossato, M. F., de Menezes, M. S., Alvarez, M. A., et al. (2013). Management of chronic neuropathic pain of different causes with the combination of oral methadone along with ketamine: A report of 18 cases. Eur. J. Anaesthesiol. 30, 638–640. doi: 10.1097/EJA.0b013e32835f9a3b
Decosterd, I., Woolf, C. J. (2000). Spared nerve injury: an animal model of persistent peripheral neuropathic pain. Pain 87, 149–158. doi: 10.1016/s0304-3959(00)00276-1
Dyer, K. R., White, J. M. (1997). Patterns of symptom complaints in methadone maintenance patients. Addiction 92, 1445–1455. doi: 10.1111/j.1360-0443.1997.tb02866.x
Ebert, B., Andersen, S., Krogsgaard-Larsen, P. (1995). Ketobemidone, methadone and pethidine are non-competitive N-methyl-D-aspartate (NMDA) antagonists in the rat cortex and spinal cord. Neurosci. Lett. 187, 165–168. doi: 10.1016/0304-3940(95)11364-3
Els, C., Jackson, T. D., Kunyk, D., Lappi, V. G., Sonnenberg, B., Hagtvedt, R., et al. (2017). Adverse events associated with medium- and long-term use of opioids for chronic non-cancer pain: an overview of Cochrane Reviews. Cochrane Database Syst. Rev. 10, CD012509. doi: 10.1002/14651858.CD012509.pub2
Erichsen, H. K., Hao, J. X., Xu, X. J., Blackburn-Munro, G. (2005). Comparative actions of the opioid analgesics morphine, methadone and codeine in rat models of peripheral and central neuropathic pain. Pain 116, 347–358. doi: 10.1016/j.pain.2005.05.004
Faul, F., Erdfelder, E., Lang, A. G., Buchner, A. (2007). G*Power 3: a flexible statistical power analysis program for the social, behavioral, and biomedical sciences. Behav. Res. Methods 39, 175–191. doi: 10.3758/bf03193146
Ferrer-Brechner, T., Ganz, P. (1984). Combination therapy with ibuprofen and methadone for chronic cancer pain. Am. J. Med. 77, 78–83. doi: 10.1016/s0002-9343(84)80023-6
Finnerup, N. B., Attal, N., Haroutounian, S., McNicol, E., Baron, R., Dworkin, R. H., et al. (2015). Pharmacotherapy for neuropathic pain in adults: A systematic review and meta-analysis. Lancet Neurol. 14, 162–173. doi: 10.1016/S1474-4422(14)70251-0
Gallo, A., Leerink, M., Michot, B., Ahmed, E., Forget, P., Mouraux, A., et al. (2017). Bilateral tactile hypersensitivity and neuroimmune responses after spared nerve injury in mice lacking vasoactive intestinal peptide. Exp. Neurol. 293, 62–73. doi: 10.1016/j.expneurol.2017.03.019
Gorman, A. L., Elliott, K. J., Inturrisi, C. E. (1997). The d- and l-isomers of methadone bind to the non-competitive site on the N-methyl-D-aspartate (NMDA) receptor in rat forebrain and spinal cord. Neurosci. Lett. 223, 5–8. doi: 10.1016/s0304-3940(97)13391-2
Gourlay, G. K., Cherry, D. A., Cousins, M. J. (1986). A comparative study of the efficacy and pharmacokinetics of oral methadone and morphine in the treatment of severe pain in patients with cancer. Pain 25, 297–312. doi: 10.1016/0304-3959(86)90234-4
Gumilar, F., Agotegaray, M., Bras, C., Gandini, N. A., Minetti, A., Quinzani, O. (2012). Anti-nociceptive activity and toxicity evaluation of Cu(II)-fenoprofenate complexes in mice. Eur. J. Pharmacol. 675, 32–39. doi: 10.1016/j.ejphar.2011.11.049
Herrlin, K., Segerdahl, M., Gustafsson, L. L., Kalso, E. (2000). Methadone, ciprofloxacin, and adverse drug reactions. Lancet 356, 2069–2070. doi: 10.1016/S0140-6736(00)03409-7
Ho, K. Y., Gwee, K. A., Cheng, Y. K., Yoon, K. H., Hee, H. T., Omar, A. R. (2018). Nonsteroidal anti-inflammatory drugs in chronic pain: implications of new data for clinical practice. J. Pain Res. 11, 1937–1948. doi: 10.2147/JPR.S168188
Huffman, K. L., Shella, E. R., Sweis, G., Griffith, S. D., Scheman, J., Covington, E. C. (2015). Nonopioid substance use disorders and opioid dose predict therapeutic opioid addiction. J. Pain 16, 126–134. doi: 10.1016/j.jpain.2014.10.011
Iribarne, C., Berthou, F., Baird, S., Dreano, Y., Picart, D., Bail, J. P., et al. (1996). Involvement of cytochrome P450 3A4 enzyme in the N-demethylation of methadone in human liver microsomes. Chem. Res. Toxicol. 9, 365–973. doi: 10.1021/tx950116m
Jungquist, C. R., Flannery, M., Perlis, M. L., Grace, J. T. (2012). Relationship of chronic pain and opioid use with respiratory disturbance during sleep. Pain Manage. Nurs. 13, 70–79. doi: 10.1016/j.pmn.2010.04.003
Kingery, W. S., Castellote, J. M., Maze, M. (1999). Methylprednisolone prevents the development of autotomy and neuropathic edema in rats, but has no effect on nociceptive thresholds. Pain 80, 555–566. doi: 10.1016/s0304-3959(98)00251-6
Kreutzwiser, D., Tawfic, Q. A. (2019). Expanding role of NMDA receptor antagonists in the management of pain. CNS Drugs 33, 347–374. doi: 10.1007/s40263-019-00618-2
Lemberg, K., Kontinen, V. K., Viljakka, K., Kylänlahti, I., Yli-Kauhaluoma, J., Kalso, E. (2006). Morphine, oxycodone, methadone and its enantiomers in different models of nociception in the rat. Anesth. Analg. 102, 1768–1774. doi: 10.1213/01.ane.0000205751.88422.41
Loening-Baucke, V., Pashankar, D. S. (2006). A randomized, prospective, comparison study of polyethylene glycol 3350 without electrolytes and milk of magnesia for children with constipation and fecal incontinence. Pediatrics 118, 528–535. doi: 10.1542/peds.2006-0220
Manchikanti, L., Kaye, A. M., Knezevic, N. N., McAnally, H., Slavin, K., Trescot, A. M., et al. (2018). Responsible, safe, and effective prescription of opioids for chronic non-cancer pain: American Society of Interventional Pain Physicians (ASIPP) Guidelines. Pain Physician 20 (2S), S3–S92.
Matsui, A., Williams, J. T. (2010). Activation of µ-opioid receptors and block of Kir3 potassium channels and NMDA receptor conductance by L- and D-methadone in rat locus coeruleus. Br. J. Pharmacol. 161, 1403–1413. doi: 10.1111/j.1476-5381.2010.00967.x
McNamara, C. R., Mandel-Brehm, J., Bautista, D. M., Siemens, J., Deranian, K. L., Zhao, M., et al. (2007). TRPA1 mediates formalin-induced pain. Proc. Natl. Acad. Sci. U.S.A. 104, 13525–13530. doi: 10.1073/pnas.0705924104
McPherson, M. L., Costantino, R. C., McPherson, A. L. (2018). Methadone: Maximizing Safety and Efficacy for Pain Control in Patients with Cancer. Hematol. Oncol. Clin. North Am. 32, 405–415. doi: 10.1016/j.hoc.2018.01.004
McPherson, M. L. (2016). Methadone safety guidelines for hospice and palliative care. AAHPM Q. 17, 8–9. doi: 10.1016/j.jpainsymman.2018.12.001
Meacham, K., Shepherd, A., Mohapatra, D. P., Haroutounian, S. (2017). Neuropathic pain: Central vs. peripheral mechanisms. Curr. Pain Headache Rep. 21, 28–28. doi: 10.1007/s11916-017-0629-5
Mercadante, S., Casuccio, A., Agnello, A., Serretta, R., Calderone, L., Barresi, L. (1998). Morphine versus methadone in the pain treatment of advanced-cancer patients followed up at home. J. Clin. Oncol. 16, 3656–3661. doi: 10.1200/JCO.1998.16.11.3656
Miranda, H. F., Noriega, V., Zanetta, P., Prieto, J. C., Prieto-Rayo, J. C., Aranda, N., et al. (2014). Isobolographic analysis of the opioid-opioid interactions in a tonic and a phasic mouse model of induced nociceptive pain. J. BioMed. Sci. 21, 62–62. doi: 10.1186/s12929-014-0062-6
National Research Council (2011). Guide for the Care, and Use of Laboratory Animals. 8th Edn. (Washington DC: The National Academies Press).
Okuyama, S., Hashimoto, S., Aihara, H., Willingham, W. M., Sorenson, J. R. (1987). Copper complexes of nonsteroidal antiinflammatory agents: analgesic activity and possible opioid receptor activation. Agents Actions 21, 130–144. doi: 10.1007/bf01974933
Omori, Y., Kagaya, K., Enomoto, R., Sasaki, A., Andoh, T., Nojima, H., et al. (2009). A mouse model of sural nerve injury-induced neuropathy: gabapentin inhibits pain-related behaviors and the hyperactivity of wide-dynamic range neurons in the dorsal horn. J. Pharmacol. Sci. 109, 532–539. doi: 10.1254/jphs.08319fp
Pelissier, T., Laurido, C., Kramer, V., Hernández, A., Paeile, C. (2003). Antinociceptive interactions of ketamine with morphine or methadone in mononeuropathic rats. Eur. J. Pharmacol. 477, 23–28. doi: 10.1016/s0014-2999(03)02192-7
Persson, J. (2013). Ketamine in pain management. CNS Neurosci. Ther. 19, 396–402. doi: 10.1111/cns.12111
Peyronnard, J. M., Charron, L. (1982). Motor and sensory neurons of the rat sural nerve: a horseradish peroxidase study. Muscle Nerve 5, 654–660. doi: 10.1002/mus.880050811
Rigo, F. K., Trevisan, G., Godoy, M. C., Rossato, M. F., Dalmolin, G. D., Silva, M. A., et al. (2017). Management of neuropathic chronic pain with methadone combined with ketamine: A randomized, double blind, active-controlled clinical trial. Pain Physician 20, 207–215.
Rodriguez, F. D., Bardaji, E., Traynor, J. R. (1992). Differential effects of Mg2+ and other divalent cations on the binding of tritiated opioid ligands. J. Neurochem. 59, 467–472. doi: 10.1111/j.1471-4159.1992.tb09393.x
Schmalbruch, H. (1986). Fiber composition of the rat sciatic nerve. Anat. Rec. 215, 71–81. doi: 10.1002/ar.1092150111
Schreiber, S., Barak, Y., Hostovsky, A., Baratz-Goldstein, R., Volis, I., Rubovitch, V., et al. (2014). Interaction of different antidepressants with acute and chronic methadone in mice, and possible clinical implications. J. Mol. Neurosci. 52, 598–604. doi: 10.1007/s12031-013-0115-4
Schreiber, S., Bader, M., Rubovitch, V., Pick, C. G. (2017). Interaction between methylphenidate, methadone and different antidepressant drugs on antinociception in mice, and possible clinical implications. World J. Biol. Psychiatry 18, 300–307. doi: 10.3109/15622975.2015.1086492
Scientific Committee on Food, Scientific Panel on Dietetic Products, Nutrition and Allergies (2006). Tolerable upper intake levels for vitamins and minerals (European Food Safety Authority (EFSA), European Union), 107–116.
Seo, J. W., Park, T. J. (2008). Magnesium metabolism. Electrolyte Blood Press 6, 86–95. doi: 10.5049/EBP.2008.6.2.86
Spring, W. D., Jr., Willenbring, M. L., Maddux, T. L. (1992). Sexual dysfunction and psychological distress in methadone maintenance. Int. J. Addict. 27, 1325–1334. doi: 10.3109/10826089209047354
Tallarida, R. J. (2000). Drug Synergism and Dose-Effect Data Analysis (Chapman & Hall/CRC: Boca Raton).
Tallarida, R. J. (2006). An overview of drug combination analysis with isobolograms. J. Pharmacol. Exp. Ther. 319, 1–7. doi: 10.1124/jpet.106.104117
Tallarida, R. J. (2016). Drug combinations: Tests and analysis with isoboles. Curr. Protoc. Pharmacol. 72, 9.19.1–9.19.9. doi: 10.1002/0471141755.ph0919s72
Tamba, B. I., Leon, M. M., Petreus, T. (2013). Common trace elements alleviate pain in an experimental mouse model. J. Neurosci. Res. 91, 554–561. doi: 10.1002/jnr.23191
Traynelis, S. F., Wollmuth, L. P., McBain, C. J., Menniti, F. S., Vance, K. M., Ogden, K. K., et al. (2010). Glutamate receptor ion channels: structure, regulation, and function. Pharmacol. Rev. 62, 405–496. doi: 10.1124/pr.109.002451
Vujović, K. S., Vučković, S., Vasović, D., Medić, B., Knežević, N., Prostran, M. (2017). Additive and antagonistic antinociceptive interactions between magnesium sulfate and ketamine in the rat formalin test. Acta Neurobiol. Exp. (Wars) 77, 137–146. doi: 10.21307/ane-2017-046
Walker, J. M., Farney, R. J., Rhondeau, S. M., Boyle, K. M., Valentine, K., Cloward, T. V., et al. (2007). Chronic opioid use is a risk factor for the development of central sleep apnea and ataxic breathing. J. Clin. Sleep Med. 3, 455–461. doi: 10.5664/jcsm.26908
Wehling, M. (2014). Non-steroidal anti-inflammatory drug use in chronic pain conditions with special emphasis on the elderly and patients with relevant comorbidities: management and mitigation of risks and adverse effects. Eur. J. Clin. Pharmacol. 70, 1159–1172. doi: 10.1007/s00228-014-1734-6
Zhao, C. S., Tao, Y. X., Tall, J. M., Donovan, D. M., Meyer, R. A., Raja, S. N. (2003). Role of μ-opioid receptors in formalin-induced pain behavior in mice. Exp. Neurol. 184, 839–845. doi: 10.1016/S0014-4886(03)00346-7
Keywords: neuropathic pain, magnesium, isobolographic study, methadone, pain treatment
Citation: González V, Pelissier T, Cazanga V, Hernández A and Constandil L (2020) Magnesium Salt, a Simple Strategy to Improve Methadone Analgesia in Chronic Pain: An Isobolographic Preclinical Study in Neuropathic Mice. Front. Pharmacol. 11:566. doi: 10.3389/fphar.2020.00566
Received: 19 November 2019; Accepted: 14 April 2020;
Published: 08 May 2020.
Edited by:
Gonzalo E. Yevenes, University of Concepcion, ChileReviewed by:
Hugo F. Miranda, University of Chile, ChileLuis Gandía, Autonomous University of Madrid, Spain
Copyright © 2020 González, Pelissier, Cazanga, Hernández and Constandil. This is an open-access article distributed under the terms of the Creative Commons Attribution License (CC BY). The use, distribution or reproduction in other forums is permitted, provided the original author(s) and the copyright owner(s) are credited and that the original publication in this journal is cited, in accordance with accepted academic practice. No use, distribution or reproduction is permitted which does not comply with these terms.
*Correspondence: Luis Constandil, luis.constandil@usach.cl