- 1The Kennedy Institute of Rheumatology, NDORMS, University of Oxford, Oxford, United Kingdom
- 2Department of Internal Medicine, Ashtead Hospital, Ashtead, United Kingdom
Two of the molecular families closely associated with mediating communication between the brain and immune system are cytokines and the kynurenine metabolites of tryptophan. Both groups regulate neuron and glial activity in the central nervous system (CNS) and leukocyte function in the immune system, although neither group alone completely explains neuroimmune function, disease occurrence or severity. This essay suggests that the two families perform complementary functions generating an integrated network. The kynurenine pathway determines overall neuronal excitability and plasticity by modulating glutamate receptors and GPR35 activity across the CNS, and regulates general features of immune cell status, surveillance and tolerance which often involves the Aryl Hydrocarbon Receptor (AHR). Equally, cytokines and chemokines define and regulate specific populations of neurons, glia or immune system leukocytes, generating more specific responses within restricted CNS regions or leukocyte populations. In addition, as there is a much larger variety of these compounds, their homing properties enable the superimposition of dynamic variations of cell activity upon local, spatially limited, cell populations. This would in principle allow the targeting of potential treatments to restricted regions of the CNS. The proposed synergistic interface of ‘tonic’ kynurenine pathway affecting baseline activity and the superimposed ‘phasic’ cytokine system would constitute an integrated network explaining some features of neuroimmune communication. The concept would broaden the scope for the development of new treatments for disorders involving both the CNS and immune systems, with safer and more effective agents targeted to specific CNS regions.
Introduction
Many neuropsychiatric disorders are accompanied by abnormal regulation of the immune system, while many immune system disorders are influenced by the central nervous system (CNS) (Gibney and Drexhage, 2013; Kipnis, 2016; Dantzer, 2018; Reardon et al., 2018). The bi-directional ‘neuroimmune interface’ believed to underlie these interactions depends partly on mediators such as cytokines which, together with other neuroactive compounds including neurotransmitters and modulators, affect neuronal and glial function in the CNS. Conversely CNS neurons and glia, in addition to producing neurotransmitters and neuromodulators, generate some of the immune system mediators which regulate leukocyte function, establishing the potential for a two-way flow of information between the CNS and immune system. Components of the kynurenine pathway of tryptophan oxidation modulate neuronal excitability and neuro-glial plasticity in the CNS (Stone and Darlington, 2002; Badawy, 2017) but also regulate fundamental aspects of the immune system including inflammatory balance, immunosurveillance and tolerance (Belladonna et al., 2009; Mandi and Vecsei, 2012; Proietti et al., 2020; Gargaro et al., 2021).
Despite this overlap, neither the immune system mediators nor tryptophan metabolites alone provide a complete explanation for disorders which may involve both systems. It is therefore proposed that the two families of compounds function as an integrated inter-dependent network in which the broad changes in neural excitability and synaptic plasticity in the CNS, and the generalized control of immune system inflammatory balance and tolerance are modulated by kynurenines. This activity would determine the basal level of cell function across the neuroimmune divide. Cytokines and chemokines could then superimpose a layer of greater regional and functional refinement which generates the specificity required for organ and tissue selective bi-directional communication.
This perspective begins with a brief summary of kynurenine and cytokine biology, followed by a more detailed expansion of the integration concept with examples of interactions between cytokines, kynurenines, the CNS and immune system. The concept of ‘volume transmission’ in the CNS is discussed as the structural basis within which those molecular interactions can occur, with comments on the contribution of the blood-brain barrier (BBB) and glia. Finally, there is an exploration of the possible mechanisms by which the different actions of tryptophan metabolites and immune mediators can achieve the functional inter-dependence and tissue specificity for a neuroimmune interface with regional selectivity.
Cytokines and kynurenines
Immune system mediators and the central nervous system
Cytokines and chemokines are immune system mediators which define leukocyte phenotypes and regulate innate and adaptive immunity. They determine the balance of pro- and anti-inflammatory activity, and the susceptibility to autoimmune disorders, infection and malignancy. Importantly, the immune system mediators also have potent actions in the CNS originally recognized by their involvement in ‘sickness behavior’ which affects multiple affective and intellectual dimensions (mood, rationality, cognition) with symptoms of hyperthermia, apathy, reduced locomotion and socialization, and a centrally mediated loss of appetite, all indicating CNS involvement (Kent et al., 1992; Dantzer et al., 2008). Interleukin-1β (IL-1β) was considered primarily responsible for sickness behavior as its levels in the blood and brain correlated with symptoms in rodents and humans (Konsman et al., 2008; Harden et al., 2011; Felger and Miller, 2012), intracerebral administration reproduced the symptoms, and they were blocked by IL-1-receptor antagonist (IL-1ra) (Konsman et al., 2008; Huang et al., 2010). Subsequently it was realized that IL-1β has wide-ranging influences on neuronal excitability, synaptic plasticity, neurogenesis, cognitive function and neuro-degeneration – effects also prevented by IL-1ra or genetic deletion of IL1B (Koo and Duman, 2008; Spulber and Schultzberg, 2010; Clausen et al., 2011; Sugama et al., 2011; Haroon et al., 2012; Takemiya et al., 2017; Salvador et al., 2021). Antagonism of IL-1β also interfered with brain development (Spulber et al., 2011).
Other cytokines can affect the CNS (Felger and Miller, 2012; Galic et al., 2012; Haroon et al., 2012; Felger and Lotrich, 2013; Miller et al., 2013; Kipnis, 2016; Dantzer, 2018; Salvador et al., 2021), some examples of which are summarized in Table 1. Many, including IL-1β, IL-6 and Tumor Necrosis Factor (TNF) can be released by activated astrocytes or microglia (Heppner et al., 2015) as well as immune system cells, contributing to normal function and pathological CNS conditions (Deverman and Patterson, 2009). Increased levels of IL-1β in the basal ganglia and nucleus accumbens have been associated with modified behavioral control and affective state. They may contribute to anxiety, fear and depression in human subjects (Felger and Lotrich, 2013; Miller et al., 2013) and possibly underlie Major Depressive Disorder (MDD) or bipolar disorder (BD) (Harrison et al., 2009). Immune mediators may have special importance in neurodevelopmental disorders such as autism and schizophrenia (Deverman and Patterson, 2009), with lifelong effects on cell proliferation, migration, synaptic, and extrasynaptic junctions (Garay and McAllister, 2010; Martineau, 2013).
Chemokines (“chemotactic cytokines”) govern the movement and localization of cells (Ubogu et al., 2006; Lai and Mueller, 2021; Sawant et al., 2021) and are therefore crucial in the communication between cells of the immune system, neurons and glia. Fractalkine (CX3CL1) has received much interest as it is produced constitutively by neurons and restrains microglial activation. The deletion of fractalkine impairs CNS development and neurogenesis (Harrison et al., 1998; Cardona et al., 2006).
The kynurenine pathway
The kynurenine pathway (Figure 1) accounts for over 90% of the metabolism of free tryptophan, which is oxidized initially to L-kynurenine primarily by indoleamine-2, 3-dioxygenase-1 (IDO1) (Stone, 1993; Stone and Darlington, 2002; Badawy, 2018; Platten et al., 2019). Interferon-γ was found to induce IDO1 expression in antigen presenting cells (APCs) (Yoshida et al., 1981; Yasui et al., 1986; Figure 2). This was considered to explain its inhibition of infection (Pfefferkorn, 1984) and the suppression of maternal lymphocyte attack on allogeneic embryos (Munn et al., 1998, 2002), partly by local tryptophan depletion and partly by the generation of downstream kynurenine metabolites. These observations introduced the concept of kynurenine metabolites as major factors in immunological function and tolerance.
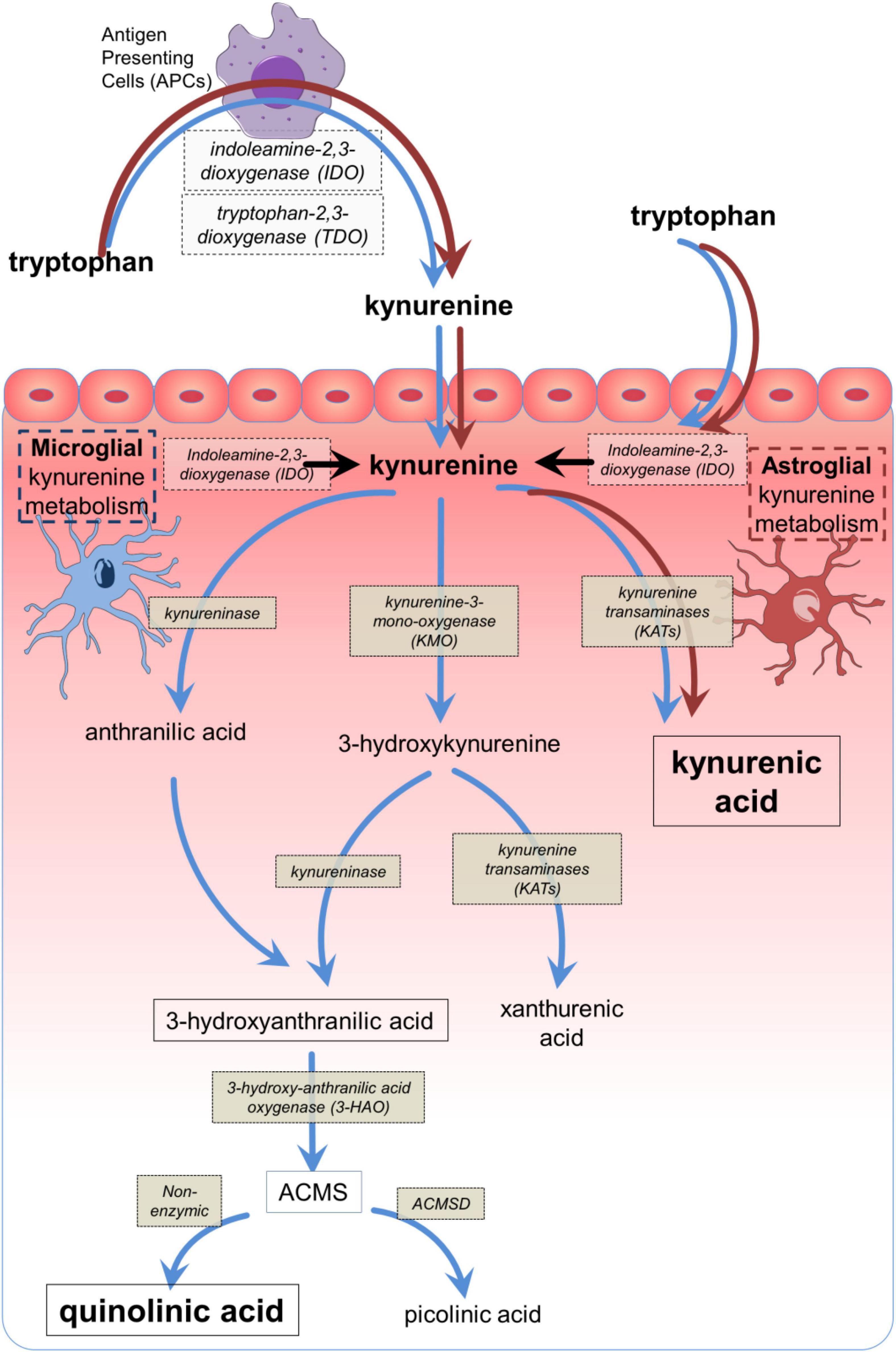
Figure 1. The main components of the kynurenine pathway. The main biologically active compounds of the kynurenine pathway, with their corresponding synthetic or catabolic enzymes from tryptophan to quinolinic acid or picolinic acid, depending on the presence or absence of ACMSD. The dark (brown) arrows indicate the dominant pathway which can be expressed by many cell types, especially antigen presenting cells including macrophages and their CNS counterparts, microglia. The light (blue) arrows indicate the abbreviated metabolism of tryptophan to kynurenic acid via kynurenine aminotransferases (KAT) which is expressed in astrocytes. The conversion of AA to 3HAA has been reported in mammalian tissues (Kotake et al., 1956; Kashiwamata et al., 1966; Baran and Schwarcz, 1990) but depends on the tissue and species (Saito et al., 1993; Fujigaki et al., 1998). ACMS, 2-amino-3-carboxy muconic acid semialdehyde; ACMSD, 2-amino-3-carboxy muconic acid semialdehyde decarboxylase.
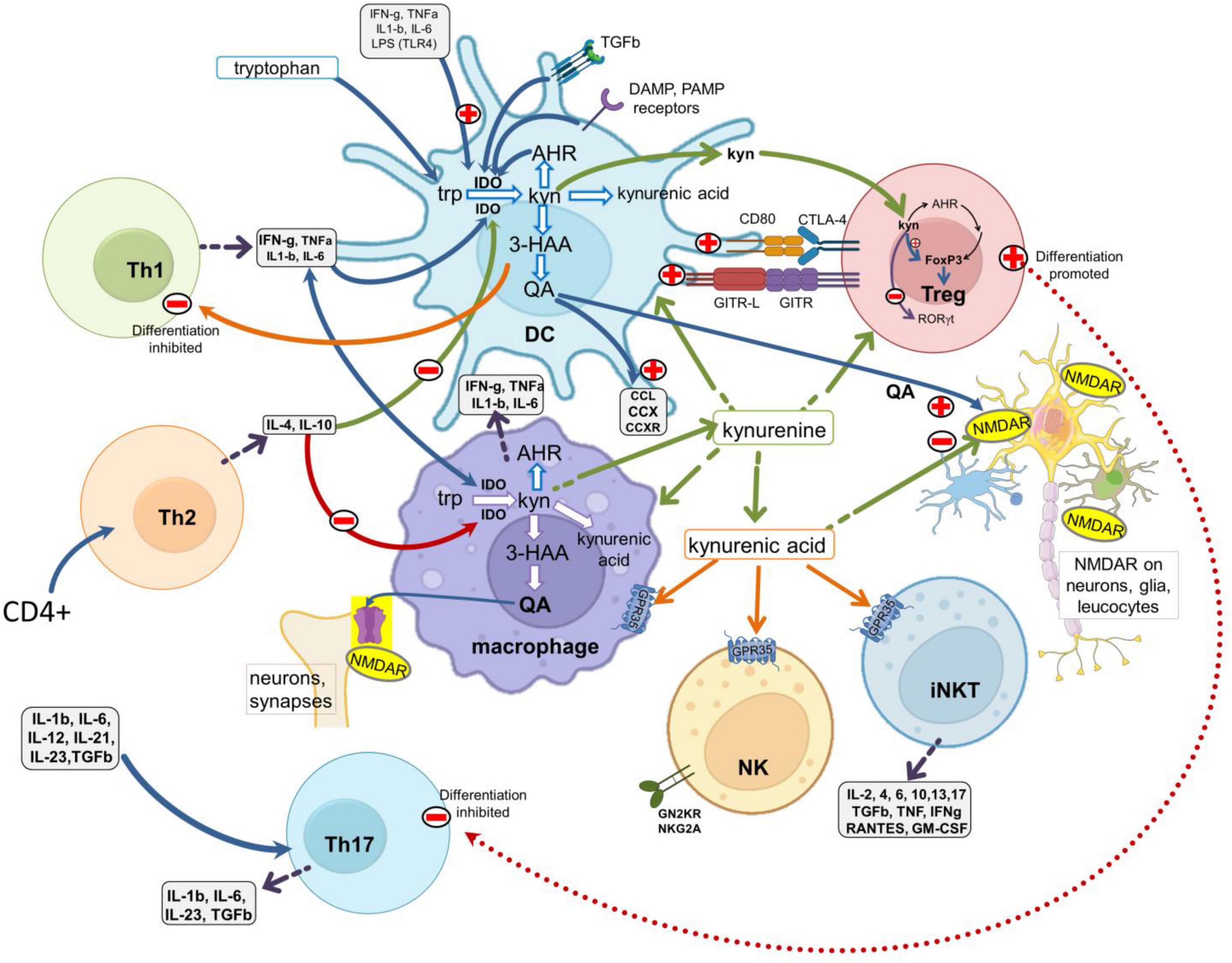
Figure 2. Interactions between the kynurenine pathway and the immune system. A schematic of major leukocyte populations showing the main sites of production and activity of the kynurenine pathway metabolites. The pathway is expressed constitutively in antigen presenting cells (DCs and macrophages) and is regulated by (a) ligation of the CD80/CD86 (B7) complex with CTLA-4 expressed by Treg cells; (b) the glucocorticoid-induced TNF receptor-related protein (GITR) and its ligand GITR-L; (c) the activation of receptors for inflammatory cytokines such as IFN-γ, IL-1β, IL-6, TNF, and with TLRs activated by Pathogen Associated Molecular Patterns (PAMPs, such as LPS, viral dsRNA and mammalian nucleotides) or Damage Associated Molecular Patterns (DAMPS). Activation of the kynurenine pathway generates 3HK, 3HAA, quinolinic acid and kynurenic acid. 3HAA inhibits Th1 cell activity, quinolinic acid is an agonist at glutamate (NMDA) receptors and kynurenic acid is an antagonist at glutamate receptors but can also activate GPR35 protein. NMDA receptors and GPR35 are expressed by many leukocytes and neurons in the CNS. Kynurenine produced by APCs acts as a paracrine agent to enter and influence lymphocytes, activating AHR to promote FoxP3 and Treg differentiation, but suppressing Th17 generation. TLRs, toll-like receptors; 3HAA, 3-hydroxyanthranilic acid; AHR, aryl hydrocarbon receptors.
Activation of the kynurenine pathway can be induced by molecular products of tissue injury or microbial fragments such as lipopolysaccharides (LPS), often acting via TLRs (Figure 2). These “sensory” routes complement the cytokine-kynurenine feedback cycles (Mezrich et al., 2010; Litzenburger et al., 2014; Li et al., 2016) which maintain kynurenine pathway activity beyond the early phases of induction in the phenomenon of ‘infectious tolerance’ (Grohmann et al., 2003; Andersson et al., 2008; Belladonna et al., 2009).
In the CNS, one product of the kynurenine pathway – quinolinic acid (Figures 1, 2) – is a selective agonist at glutamate receptors which respond to N-methyl-D-aspartate (NMDA) (Stone and Perkins, 1981; Perkins and Stone, 1983; Stone, 1993). The fundamental importance of NMDA receptors (NMDARs) lies in their contribution to excitatory synaptic transmission and the modulation of calcium fluxes and synaptic plasticity underlying learning and cognitive behavior (Traynelis et al., 2010; Stone and Darlington, 2013; Dubois and Liu, 2021; Kilonzo et al., 2021; McQuail et al., 2021; Schonewille et al., 2021). When over-activated by NMDA or quinolinic acid they can promote neuronal loss (Schwarcz et al., 1983; Stone et al., 2007; Guillemin, 2012; Stone and Darlington, 2013).
In contrast, kynurenic acid (Figures 1, 2) is an antagonist at all three glutamate receptor subtypes responding respectively to NMDA, α-amino-3-hydroxy-5-methyl-4-isoxazole-propionic acid (AMPA) or kainic acid (Perkins and Stone, 1982), but it is most potent at blocking postsynaptic NMDARs (Perkins and Stone, 1982, 1985; Ganong et al., 1983; Brooks et al., 1986; Ganong and Cotman, 1986) since it blocks the binding sites for glutamate and the co-agonist glycine (Ascher and Nowak, 1987; Birch et al., 1988; Danysz et al., 1989; Henderson et al., 1990). Glutamate antagonism accounts for the learning and cognitive deficits induced by kynurenic acid or procedures which increase its levels in the CNS (Stone and Darlington, 2002, 2013; Heisler and O’Connor, 2015). Increasing kynurenic acid levels before birth can lead to cognitive dysfunction in the offspring (Pocivavsek et al., 2019), corresponding to changes in CNS structure and synaptic function (Forrest et al., 2013a,b, 2015; Khalil et al., 2014; Pisar et al., 2014).
In addition to blocking these ionotropic glutamate receptors, cell activity may be affected by kynurenic acid activating the Aryl Hydrocarbon Receptor (AHR) (Opitz et al., 2011; Bessede et al., 2014) or the GPR35 protein (Wang et al., 2006; Berlinguer-Palmini et al., 2013; Alkondon et al., 2015; Mackenzie and Milligan, 2015; Resta et al., 2016), while paradoxical stimulant effects can arise indirectly by blocking the activation of inhibitory neurons. The overall effects of kynurenic acid therefore depend on the pattern of neuronal activity in the network of excitatory and inhibitory pathways (Stone, 2021). The balance between quinolinic acid and kynurenic acid influence neuronal plasticity, development, cognitive function, recovery after injury and specific aspects of behavior such as pain perception (Ciapala et al., 2021) and addiction (Morales-Puerto et al., 2021).
The kynurenine pathway is present constitutively in APCs such as macrophages and dendritic cells (Guillemin et al., 2001, 2005a; Jones et al., 2015). The interplay between these and other immune system cells determines the levels of innate and adaptive immune system functioning. Key intracellular enzymes of the kynurenine pathway are regulated by pro- and anti-inflammatory cytokines and feedback circuits such as those centered around the AHR (Belladonna et al., 2006; Opitz et al., 2011; Bessede et al., 2014; Litzenburger et al., 2014; Li et al., 2016).
The initial enzymes: IDO1, IDO2, TDO
Although the first enzyme of the kynurenine pathway – IDO1 – is induced primarily by IFN-γ (Yoshida et al., 1981; Yasui et al., 1986), activation is also produced or potentiated by type-1 interferons, IL-1β, IL-6, TNF, LPS and viral dsRNA (Hissong et al., 1995; Hissong and Carlin, 1997; Babcock and Carlin, 2000; Robinson et al., 2003, 2005; Dai and Zhu, 2010; Watts et al., 2011; Bessede et al., 2014; Murray et al., 2015; Yeung et al., 2015; Mondanelli et al., 2020). These factors usually activate IDO1 via Toll-Like Receptors (TLRs) in APCs. TLR9 activation by CpG oligodeoxynucleotides increases expression of the B7 (CD80/86) protein complex on DCs which induced IDO1 expression. IDO1 can use a wide range of indole-derived compounds as substrates, including melatonin and indolic amines generated by the intestinal microbiota (see Section “Dietary and microbial influences”).
Cytokines associated with anti-inflammatory resolution and tissue recovery, such as IL-4 and IL-10 inhibit IDO1 expression (Musso et al., 1994; Mueller and Schwarz, 2010), while IDO1 reduces IL-10 expression, creating a stable feedback level of IL-10. This is greatly reduced in IDO(–/–) mice but is restored in double knockouts of IDO1 and IL-10 (Metghalchi et al., 2015; Laumet et al., 2018).
IFN-γ also induces KMO, KAT and kynureninase in circulating monocytes (McIlroy et al., 2005; Varga et al., 2015) and DCs, even in the absence of IDO1 (Belladonna et al., 2006). The increased generation of 3-hydroxynurenine (3HK), 3-hydroxyanthranilic acid (3HAA) and quinolinic acid are important in the immune system and CNS as noted below.
Exposure to stress induces expression of cytokines and IDO1 (Lu et al., 2013; Tian et al., 2014; Lavieri et al., 2016; Pearson-Leary et al., 2016; Rowland et al., 2018; Johnson et al., 2019; Kim et al., 2019; Upthegrove and Khandaker, 2020; Lumertz et al., 2022). Mice lacking IDO1 or treated with the inhibitor 1-methyl-tryptophan do not show the loss of spontaneous mobility and social interaction which characterizes depression in the forced swim test (O’Connor et al., 2009a,b; Raison et al., 2010; Alexander et al., 2013; Lawson et al., 2013). IDO1 induction in the CNS contributes to depressive symptoms associated with pain (Alberati-Giani et al., 1996; Guillemin et al., 2003; Kim et al., 2012). Kynurenic acid may be a major factor in this, since its blockade of NMDARs can suppress chronic pain (Pineda-Farias et al., 2013; Vecsei et al., 2013).
IDO2 is less active than IDO1 but mediates the same metabolism of tryptophan to kynurenine (Fatokun et al., 2013) and may be important in the activity of IDO1 (Metz et al., 2014). IDO2 can be induced by activation of the AHR and its role in immunomodulation includes regulation of antibody production (Merlo et al., 2014). IDO2 exhibits some non-enzymic actions on targets such as GAPDH and Runx (Mondanelli et al., 2021; Merlo et al., 2022), some of which may not involve tryptophan oxidation (Mondanelli et al., 2021). Despite having a spectrum of cellular targets (Prendergast et al., 2014), deleting IDO2 does not produce any behavioral changes (Too et al., 2016).
Tryptophan is also metabolized to kynurenine by tryptophan-2,3-dioxygenase (TDO2) an enzyme with greater substrate selectivity for tryptophan. TDO is expressed mainly in the liver (Badawy, 2017, 2018), but is a key link between the external environment and the kynurenine pathway. Exposure to physical or mental stress induces activity in the hypothalamo-pituitary-adrenal (HPA) axis leading to an increased synthesis of adrenal gluco-corticosteroids. These hormones induce and activate hepatic TDO, contributing to the regulation of systemic kynurenine levels. Stress is also associated with heightened immune system activity in the brain, with social withdrawal and anxiety, increased neuronal activity and microglial activation (Yin et al., 2019). Cognitive and emotional changes may be induced, potentially leading to mental illness (de Kloet et al., 2005; Davidson and McEwen, 2012), even when the stress is encountered during gestation (Lupien et al., 2009). These negative effects of stress on cognition have been linked to the activation of tryptophan metabolism by TDO (Kiank et al., 2010; Miura et al., 2011).
Limitations and uncertainties: Concentrations and correlations
Arguments about the roles of tryptophan catabolites and cytokines in the CNS often center around the relationship between their systemic and central concentrations. Several studies of blood and CSF cytokine concentrations have shown parallel qualitative and quantitative changes, including those on TGF-β (Liu et al., 2022) with one report of IL-6 levels in blood correlating highly with mood (Gadad et al., 2021). The CSF: serum ratio of IL-6 levels was sufficiently consistent to act as a potential biomarker of intracranial aneurysms (Kaminska et al., 2021), while increased levels of IL-6 in rat brain parenchyma occurred in parallel with plasma and CSF values and with the severity of traumatic brain injury (Chatzipanteli et al., 2012).
However, levels of cytokines in the tissues and organs can be highly variable. A cytokine panel used in patients with tuberculous meningitis indicated that all (except IL-10) were present in brain at levels lower than in CSF (Loxton et al., 2021), while a panel of 36 cytokines used by Lepennetier et al. (2019) exhibited little correlation between serum and CSF levels in a variety of neurological disorders. Similarly, measurements of TNF, IFN-γ, IL-6, IL-8, and IL-10 revealed no correlation between plasma and CSF levels (Ellison et al., 2005), and a meta-analysis of patients with MDD found no relationships between IL-6 or TNF levels in blood and CSF or with PET-scan markers of inflammation (Enache et al., 2019). A highly instructive study concluded that the levels of several cytokines were not correlated between serum and CSF following peripheral (knee) surgery and that the central levels exceeded those in the periphery (Bromander et al., 2012) leading to the conclusion that even a peripheral inflammatory stimulus could induce a substantial CNS inflammatory response.
In the case of kynurenines, many studies of depression have reported increased activity along the kynurenine pathway but this is not a universal observation (Quak et al., 2014; Dahl et al., 2015). There is a high variability which imposes limits on interpretation (Birnbaum et al., 2018; Ogyu et al., 2018; Birnbaum and Weinberger, 2020) and the weakness of correlations between compound concentrations and disease symptoms has been highlighted in meta-analyses. It has been suggested that this reflects variations in diagnostic criteria (Arnone et al., 2018; Hunt et al., 2020; Pedraz-Petrozzi et al., 2020; Serafini et al., 2020). The need for greater precision of diagnosis has been raised by Sublette et al. (2011) who reported changes in cytokine and kynurenine concentrations only in suicidally depressed patients, a finding consistent with other evidence (Brundin et al., 2017).
From uncertainty to hypothesis
Overall, therefore, the data suggest that the CNS presence of complex molecules does not lend itself to meaningful correlations with symptoms or with the levels of other compounds – such as kynurenines – with very different permeabilities and kinetics. These difficulties may disappear under the present hypothesis, as discussed in depth below, since the essential correlations to examine are not between cytokines or between kynurenine metabolites, but are the cross-correlations between the two families of compounds, reflecting the complementary nature of their contributions. Such measurements of course still need the appropriate attention to diagnosis, cohort variations, and clearer definition of the CNS regions relevant to a particular disorder or symptom.
An integrated kynurenine-cytokine system
Clearly, the cytokine and kynurenine families play fundamental and wide-ranging roles in the immune system and CNS, with confusion arising on how to relate either of these groups to disease symptoms or severity. The hypothesis proposed here is that the two sets of compounds exert complementary actions so that integration between them is required to explain aspects of disease. This idea will be expanded in the following sections by considering in more detail how that integration could arise, with an emphasis firstly on molecular interactions and then secondly on spatial factors. Subsequent sections will then discuss the structural and functional aspects of how and where these interactions can occur, focusing on volume transmission and the roles of glial cells and the BBB.
Cytokine – Kynurenine interactions: Molecular aspects
Some of the more prominent interactions between cytokines or chemokines and the kynurenine metabolites of tryptophan are summarized in Table 1 and major actions of kynurenines on the immune system are shown in Figure 3.
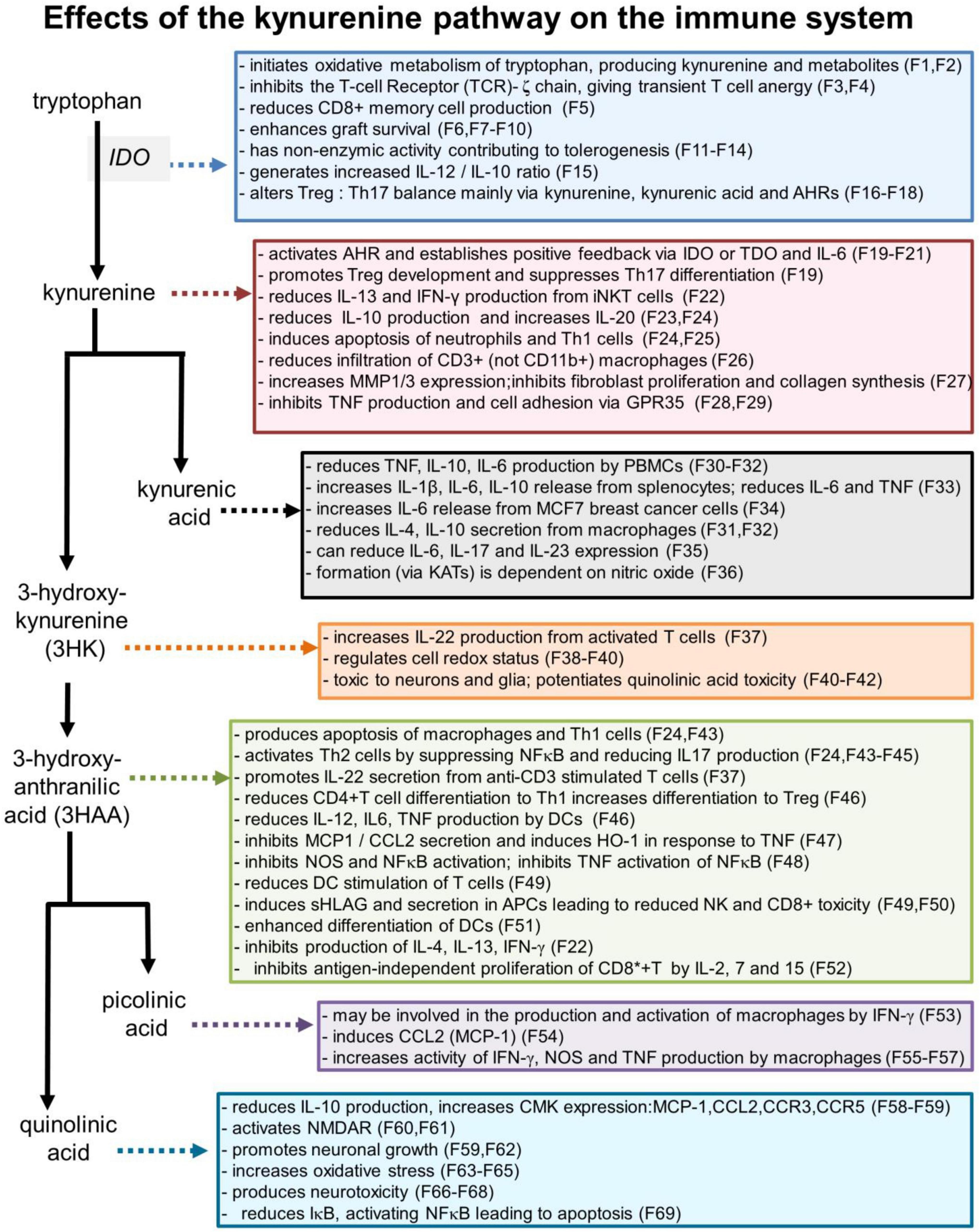
Figure 3. Selected effects of kynurenine pathway components on the immune system. Numbers refer to the corresponding references which are listed in a separate section of the references list, but which are summarized as follows: F1: Badawy, 2017; F2: Badawy, 2018; F3: Fallarino et al., 2006; F4: Eleftheriadis et al., 2016; F5: Kirillova et al., 2008; F6: He et al., 2015; F7: Zhang et al., 2018; F8: Huang et al., 2017; F9: Bock et al., 2013; F10: Xie et al., 2015; F11: Pallotta et al., 2011; F12: Belladonna et al., 2006; F13: Orabona et al., 2012; F14: Albini et al., 2017; F15: Juhl et al., 2020; F16: Favre et al., 2010; F17: Porro et al., 2020; F18: Baban et al., 2009; F19: Mezrich et al., 2010; F20: Litzenburger et al., 2014; F21: Li et al., 2016; F22: Molano et al., 2008; F23: Harden et al., 2016; F24: Fallarino et al., 2002; F25: El-Zaatari et al., 2014; P26: Elizei et al., 2015; F27: Poormasjedi-Meibod et al., 2016; F28: Barth et al., 2009; F29: Kolodziej et al., 2011; F30: Wang et al., 2006; F31: Metghalchi et al., 2015; F32: Fallarini et al., 2010; F33: Malaczewska et al., 2016; F34: DiNatale et al., 2010; F35: Elizei et al., 2017; F36: Luchowski and Urbanska, 2007; F37: Lowe et al., 2014; F38: Giles et al., 2003; F39: Goldstein et al., 2000; F40: Okuda et al., 1998; F41: Chiarugi et al., 2001; F42: Guidetti and Schwarcz, 1999; F43: Hayashi et al., 2007; F44: Terness et al., 2002; F45: Lee et al., 2010; F46: Lee et al., 2013; F47: Pae et al., 2006; F48: Sekkai et al., 1997; F49: Lopez et al., 2008; F50: Lopez et al., 2006; F51: Hill et al., 2007; F52: Weber et al., 2006; F53: Varesio et al., 1990; F54: Bosco et al., 2004; F55: Pastorino et al., 2004; F56: Ladomersky et al., 2018; F57: Melillo et al., 1993; F58: Croitoru-Lamoury et al., 2003; F59: Guillemin et al., 2003; F60: Stone, 1993; F61: Stone and Perkins, 1981; F62: Hernandez-Martinez et al., 2017; F63: Kubicova et al., 2013; F64: Santamaria et al., 2001; F65: Perez-De La Cruz et al., 2012; F66: Guillemin, 2012; F67: Schwarcz et al., 2010; F68: Bhat et al., 2020; F69: Qin et al., 2000.
Immune mediators can interact with glutamate-mediated transmission. TNF, for example, enhances release of glutamate from neurons and enhances its exocytotic release from glia (Santello et al., 2011; Santello and Volterra, 2012; Wang et al., 2017; Shim et al., 2018). Some of this release arises from glutaminase activity (Ye et al., 2013; Milewski et al., 2019) but glutamate uptake transporters are also inhibited (Olmos and Llado, 2014; Clark and Vissel, 2016).
Stimulation of NMDAR promotes TNF expression (McNearney et al., 2010) and TNF enhances NMDAR activity (Youn et al., 2008; Han and Whelan, 2010; Woods et al., 2021) including synaptic depolarization (Xu et al., 2006; Kawasaki et al., 2008; Li et al., 2009) mainly through TNFR1 receptors (Zhang et al., 2011; Del Rivero et al., 2019). This interaction extends to a regulation of NMDAR subunit composition (Weaver-Mikaere et al., 2013).
Interactions with AMPAR are less clear, since TNF modulates the expression and activity of AMPAR (Beattie et al., 2002; Stellwagen et al., 2005) and TNFR1 blockade reduces
AMPAR expression (Woolf and Salter, 2000; Beattie et al., 2002; Cingolani et al., 2008; Santello and Volterra, 2012). However, AMPAR-induced depolarization was not affected by TNF in other studies (Yang et al., 2002; Kawasaki et al., 2008; Xu et al., 2010). A negative feedback cycle, dependent on IDO1, stabilizes IL-10 expression (Metghalchi et al., 2015) thereby regulating AMPAR expression and glutamate-modulated neural excitability in the CNS (Savina et al., 2013).
Inflammatory mediators such as IL-6, IL-1β, and IFN-γ activate IDO1 and other kynurenine pathway enzymes primarily in APCs and CNS glia (Figure 2; Heyes et al., 1992a; Guillemin et al., 2001, 2005a; Andre et al., 2008; O’Connor et al., 2009b; Yamada et al., 2009; Jones et al., 2015; Murray et al., 2015; Yeung et al., 2015). Anti-inflammatory mediators such as IL-4 and IL-10 normally inhibit IDO1 expression (Musso et al., 1994). Since kynurenine enters and leaves cells by transmembrane diffusion and active transport (Belladonna et al., 2008; Sinclair et al., 2018) its production by APCs gives it a paracrine signaling role to cells which do not express IDO constitutively (Guillemin et al., 2005b; Andersson et al., 2008; Belladonna et al., 2008, 2009). This is crucial for immune function since kynurenine induces FoxP3 expression in CD4+ T cells, promoting differentiation to regulatory T cells (Tregs), but suppresses Retinoic Acid Receptor-related Orphan Receptor-γt (RORγt) which promotes differentiation to Th17 inflammatory cells (Figure 2). Dysregulation of the Treg: Th17 ratio has been associated with several autoimmune disorders (Favre et al., 2010; Jurado-Manzano et al., 2017) and may be dependent on epigenetic factors affecting IDO1 (Kawalkowska et al., 2019; Kmiolek et al., 2020; Huang et al., 2021). Treg control of the immune system is enhanced by their expression of CD39 and CD73 which hydrolyze ATP to adenosine, an important modulator of many neuronal activities (Stone et al., 2009). This nucleoside potently inhibits the release of synaptic neurotransmitters and other neuroactive compounds in the CNS and peripheral autonomic nerve terminals (Stone et al., 2007; Antonioli et al., 2013) contributing substantially to neuroimmune communication.
In addition to these direct actions of kynurenine and its metabolites, the localized consumption of tryptophan by IDO reduces the proportion of loaded tryptophanyl-tRNA, thereby activating General Controller Non-derepressible-2-kinase (GCN2k) and leading to reduced proliferation and increased apoptosis (Liu et al., 2014). Activation of GCN2k is synergistic with IL-12 and IL-6 in macrophages, leading to further IDO1 induction. The kynurenine generated will enhance Treg formation and produce an anti-inflammatory bias. However, kynurenine also promotes inflammasome activation in astrocytes, generating IL-1β (Zhang et al., 2020).
The effects of kynurenine are cell and context-dependent since it enhances IL-6 and IL-13 production by mast cells but inhibits IL-13 release from iNKT cells probably via AHR (Figure 2; Stone et al., 2007). This may be a factor linking kynurenine with obesity and autoimmune dysfunction (Nguyen et al., 2014; Stockinger et al., 2014; Moyer et al., 2016; Stone et al., 2018). Kynurenine inhibits expression of the Natural Killer (NK) cell activating lectin receptor NKG2, thus suppressing their anti-tumor properties. In human monocyte-derived cells, kynurenic acid reduces IL-6 and TNF release via AHR (DiNatale et al., 2010) and enhances IL-1β, IL-6 and TNF release, while suppressing IL-10 (Metghalchi et al., 2015).
An excellent example of the intimate functional relationship between immune mediators and kynurenine metabolites is the sickness behavior, described above. While early work implicated IL-1β, the behavior is also prevented by IDO1 inactivation (Jung et al., 2009; Gibney et al., 2013) emphasizing that downstream kynurenine metabolites make a significant to the cytokine-induced symptoms.
Changes of CNS function can be induced by depleting peripheral CD4+T cells (Bauer et al., 2007; Wolf et al., 2009; Kipnis et al., 2012). This leads to hippocampal neuronal loss and reduced cognition which is prevented by IL-4, indicating that neuronal viability and functional integrity are maintained by immune mediators secreted by peripheral lymphocytes (Kipnis et al., 2012; Kamimura and Murakami, 2019). Many such influences are probably mediated by the itinerant leukocyte populations passing through the CNS parenchyma.
3-Hydroxyanthranilic acid and anthranilic acid
A key catabolite of kynurenine is 3-hydroxy-anthranilic acid (3HAA) (Figure 1). 3HAA entrains an anti-inflammatory cycle in which IFN-γ generated by antigenic activation of Th1 cells induces IDO1 in monocytes and APCs. The 3HAA generated by these cells then inhibits Th1 cells and promotes Th2 activity, thus reducing inflammatory activity (Fallarino et al., 2002). This will be enhanced by the paracrine transport of kynurenine into T cells (Sinclair et al., 2018), promoting Treg formation and further suppressing effector T cells. The result is an inhibition of pro-inflammatory cytokine release from Th1, CD8+, and NK cells (Figures 2, 3; Fallarino et al., 2002; Hayashi et al., 2007) while promoting anti-inflammatory Th2 activity (Fallarino et al., 2002, 2006; Terness et al., 2002; Darlington et al., 2010; Krause et al., 2011).
The ratio between anthranilic acid and 3HAA correlates with inflammatory status in many disorders (Forrest et al., 2006; Darlington et al., 2010; Heng et al., 2020; Huang et al., 2020; Jusof et al., 2022; Yan et al., 2022) and 3HAA contributes to allograft survival (He et al., 2015), synergistically with AHR activation (Gargaro et al., 2019). Kynurenine, kynurenic acid and 3HAA reduce NK cell activation and proliferation, potentially facilitating tumor development (Fallarino et al., 2002; Terness et al., 2002; Hayashi et al., 2007). The tolerogenic efficacy of 3HAA is partly achieved by promoting TGF-β generation and its maintenance of IDO+ CD8+ cells.
Quinolinic acid
Inflammatory activation of IDO in peripheral cells will increase plasma levels of kynurenine and 3HK which enter the CNS by diffusion and by the amino acid transporter LAT-1 (SLC7A5) (Sinclair et al., 2018; Figure 4). Kynurenine is then metabolized to kynurenic acid (in astrocytes) and quinolinic acid (in microglia and neurons) (Figure 1), (Guillemin et al., 2001; Sathyasaikumar et al., 2022) which leave the CNS slowly via the acidic amino acid transporter, blocked by probenecid (Figure 4). Stimulation of macrophages by IFN-γ therefore increases the synthesis and release of quinolinic acid (Heyes et al., 1992a; Chiarugi et al., 2000; Guillemin et al., 2005a; O’Connor et al., 2009a,b; Garrison et al., 2018) which tends to accumulate in the CNS. As an agonist at NMDARs (Stone and Perkins, 1981; Stone, 1993) quinolinate induces neuronal loss even at low concentrations if maintained for several days (Schwarcz et al., 1983; Whetsell and Schwarcz, 1989; Giulian et al., 1990; Guillemin, 2012) and has been linked with neurodegenerative disorders including Alzheimer’s disease (Majlath et al., 2014), Huntington’s disease (Schwarcz et al., 2010; Thevanavakkam et al., 2010), multiple sclerosis and amyotrophic lateral sclerosis (Guillemin et al., 2005b; Maya et al., 2018). High micromolar levels have been recorded in patients with immunodeficiency viruses, possibly contributing to the associated dementia (Heyes et al., 1992b; Kandanearatchi and Brew, 2012). It is likely that the pro-oxidant activity of quinolinic acid (Platenik et al., 2001; Santamaria et al., 2001; Seminotti et al., 2016; Hernandez-Martinez et al., 2017) and its subcellular targets leading to effects on apoptosis and autophagy (Silva-Islas et al., 2022) may also be relevant.
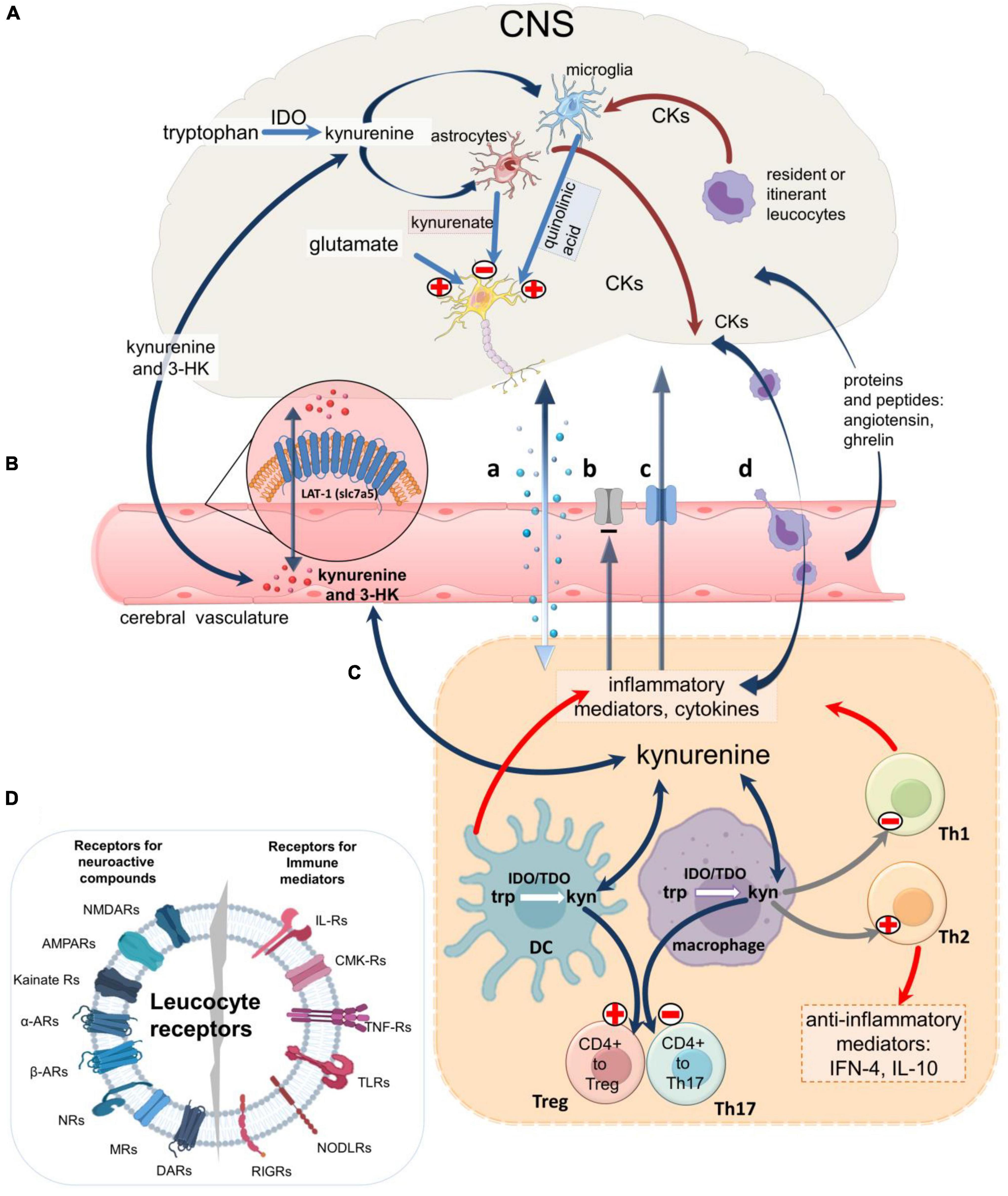
Figure 4. An overview of neuroimmune communication. Routes of communication between the CNS and immune systems including the blood-brain barrier. (A) IDO1 expressed in microglia produces kynurenic acid and quinolinic acid, a selective agonist on cellular NMDARs. Astrocytes produces only kynurenic acid which blocks receptors for endogenous glutamate on neurons, glia, and leukocytes, while glia and leukocytes resident in or passing through the CNS (‘itinerant’ cells) produce a variety of neuroactive cytokines and other inflammatory mediators which modulate glial activity and neuron excitability. (B) Kynurenine and 3HK generated in the CNS or in peripheral tissues cross the blood-brain barrier in both directions partly by diffusion and partly by the active Large Neutral Amino Acid Transporter (LAT-1). Larger immune mediator molecules gain access to the CNS by diffusion in areas of higher permeability (a), although some mediators may be excluded by persistent efflux transporters (b) and several have dedicated active transporters (c). Alternatively, immune mediators can be secreted by leukocytes which have crossed the cerebrovascular endothelium (d). (C) In all compartments the cytokines can regulate kynurenine pathway enzymes, partly via the balance of pro-inflammatory and anti-inflammatory interleukins. In the immune system kynurenine initiates a feedback generation of IDO and more kynurenine via Aryl Hydrocarbon Receptors. The kynurenine generated determines the expression of FoxP3 and RORγt and thus CD4 + differentiation to Treg or inflammatory T cells. (D) Leukocyte receptors for immune system mediators and neuroactive compounds produced by neurons and glia, emphasizing their susceptibility to ligands produced by cells of the immune system and the peripheral and central nervous systems.
Importantly, quinolinic acid induces the expression of several key chemokines (including CCL2, CCL5, CXCL8, and CX3CL1) and chemokine receptors (CXCR4, CCR3, CCR5) (Guillemin et al., 2003). The resulting modulation of immune cell migration and mediator activity could in the CNS would exert a strong influence over neuronal function.
Kynurenic acid
In general, kynurenic acid is anti-inflammatory, suppressing TNF, IL-4 and IL-23 production by activated monocytes and reducing production of neutrophil peptides (Tiszlavicz et al., 2011). It also inhibits CD4+ cell differentiation to the Th17 phenotype via AHR (Wirthgen et al., 2018; Walczak et al., 2020a,b) and is an endogenous activator of GPR35 (Wang et al., 2006; Mackenzie and Milligan, 2015; Resta et al., 2016; Figure 2). This may carry novel implications for understanding the roles of kynurenic acid as new consequences of GPR35 activation are discovered such as those involving mitochondrial functioning (Wyant et al., 2022).
Kynurenic acid is released from neurons and glial cells (Figure 4; Turski et al., 1989; Swartz et al., 1990; Gobert et al., 2011; Heredi et al., 2019; Notarangelo et al., 2019). Activation of catecholamine β1- or β2-adrenoreceptors increased the levels of kynurenic acid in vivo or in brain slices or glial cultures (Luchowska et al., 2009), possibly accounting for some effects of catecholamines on the CNS and behavior.
Kynurenic acid blocks the ionotropic receptors for glutamate found on leukocytes – NMDA, AMPAR and kainate receptors (Lombardi et al., 2001; Boldyrev et al., 2004, 2012; Kvaratskhelia et al., 2009; Mashkina et al., 2010; Bhandage et al., 2017; Stone, 2020) (Figures 3, 4). The NMDA or AMPA receptors, and metabotropic glutamate receptors mGlu1R and mGlu5R (Pacheco et al., 2004) are analogous to those in the CNS and are associated with calcium influx and changes in cell proliferation, activation and differentiation (Lombardi et al., 2001; Nohara et al., 2015).
It is of interest that NMDAR activation induced the release of kynurenate in vivo, representing a further link between immune cell sensitivity and the CNS since glutamate generated by neuroglial activity will modulate itinerant leukocyte activity via their complement of glutamate receptors (Turski et al., 1989; Heredi et al., 2019). Glutamate induces release of anti-inflammatory IL-8, IL-10 and other cytokines from microglia and lymphocytes (Kvaratskhelia et al., 2009), so blockade of these receptors by kynurenic acid (Lombardi et al., 2001) may indirectly affect the release of several immune system mediators.
Modifying the endogenous levels of kynurenic acid for therapeutic purposes is a major and promising field of investigation (Stone, 2000a,b; Dounay et al., 2013, 2015; Kozak et al., 2014; Jayawickrama et al., 2015; Smith et al., 2016; Zadori et al., 2016; Jacobs et al., 2017; Majlath et al., 2018), especially as the neuroglial and leukocyte glutamate receptors are blocked by the same antagonists (Lombardi et al., 2001; Boldyrev et al., 2004; Kvaratskhelia et al., 2009; Mashkina et al., 2010; Bhandage et al., 2017) and respond similarly to changes in calcium availability (Nohara et al., 2015). Indeed, in isolated cell culture experiments, without the uptake and removal of kynurenate into cells or circulatory vessels, kynurenate is a highly potent antagonist at leukocyte NMDARs (IC50 of 400 nM) (Lombardi et al., 2001).
Both quinolinic acid and kynurenic acid have been implicated in Huntington’s disease (Forrest et al., 2010; Thevanavakkam et al., 2010; Stone et al., 2012), stroke (Darlington et al., 2007), multiple sclerosis (Sundaram et al., 2014; Boros et al., 2018; Platten et al., 2019), amyotrophic lateral sclerosis (Lee et al., 2017; Lovelace et al., 2017), schizophrenia (Wonodi et al., 2011; Stone and Darlington, 2013; Erhardt et al., 2017) and suicidality (Brundin et al., 2016, 2017) among other neurological and psychiatric disorders. Kindler et al. (2020) quantified kynurenine metabolites in blood and brain tissue of patients with schizophrenia. Several, including kynurenic acid, were increased in the prefrontal cortex, a region characterized by high levels of proinflammatory cytokines (Figure 4). The same population exhibited increased IDO1 activity (defined as the kynurenine: tryptophan ratio) in plasma, correlating inversely with patients’ attention. The results were consistent with elevated kynurenate concentrations causing abnormal mental behavior by blocking NMDA receptors. Quantification of kynurenine metabolites in the blood in MDD, BD and schizophrenia supported increased tryptophan metabolism, but with distinct metabolite profiles for the three disorders (Marx et al., 2021).
Picolinic and xanthurenic acids
Picolinic acid may have cell-protective and anabolic activity (Heng et al., 2016; Lovelace et al., 2017; Duque et al., 2020), with a possible pathological relevance in lupus nephritis for which it may be a suitable biomarker (Anekthanakul et al., 2021). Picolinic acid modulates macrophage metabolism and secretion of Macrophage Inflammatory Protein-1 (MIP-1) (Bosco et al., 2000; Rapisarda et al., 2002; Manzari et al., 2007).
Xanthurenic acid (Figure 2) can modulate neuronal activity and synaptic transmission in the CNS (Neale et al., 2013; Roussel et al., 2016; Sathyasaikumar et al., 2017). It appears to interact chiefly with metabotropic glutamate receptors and AHR but other targets may remain unidentified (Fazio et al., 2017). Xanthurenic acid has also been linked to the development of diabetes (Connick and Stone, 1985).
Cytokine – Kynurenine interactions: Spatial aspects
The production of neuro-glial transmitters, neuromodulators, neurotrophins, cytokines and chemokines in the CNS yields a complex inter-cellular matrix (Section “Structural and functional factors underlying cytokine-kynurenine integration”) but some regional specificity is required to produce the varied, dynamic regulation of different behaviors, sensations (including pain), emotional reactions, and intellectual function, resulting from activity across the CNS and immune system. While most actions of kynurenine and its metabolites will be a generalized regulation of CNS development, excitability and plasticity, together with an organism-wide modulation of immune function, the present hypothesis argues that the reciprocal effects of immune system mediators on the CNS will produce a more localized, focused control of neuronal and glial activity. A mechanism is required to explain how such anatomical and functional complementarity could be generated between two such apparently complex and diffuse environments as the immune system and CNS.
Certainly one factor would be the distribution of receptors. Kynurenine pathway targets are widespread, including the glutamate receptors expressed by CNS neurons and glia, and many populations of leukocytes can be activated by quinolinic acid and blocked by kynurenic acid. Alternative targets for kynurenate such as AHR and GPR35 are present in a large fraction of cells, while effects on oxidative processes and redox status will affect almost all cell types. A highly specific distribution of receptors was first noted for IL-1 (Farrar et al., 1987) but has since been described for many others (Cayrol and Girard, 2022) with receptors located on different cells or groups (Gour and Wills-Karp, 2015; Hurdayal and Brombacher, 2017; Johnston and Bryce, 2017; Fairlie-Clarke et al., 2018; Younger et al., 2019; Wilson, 2021). In contrast, cytokines are often produced by distinct populations of cells, giving the compounds marked differences in kinetics and regional specificity (Scheller et al., 2021; Evavold and Kagan, 2022).
Viewed together, these various considerations contribute to the ability of individual cytokines to define specific aspects of CNS or immune system function superimposed on the more generalized regulation of cell behavior produced by the kynurenine pathway. This view could form the basis of selectivity mechanisms already recognized in peripheral tissues but now applied to the CNS, such as homing.
Homing
The term ‘homing’ refers to the directed migration of different cell types to appropriate locations affected by environmental or pathological changes. The concept is well established in peripheral tissues with the differential targeting of cells to various tumors or specific cell types within tumors. The homing factors are primarily cytokines and chemokines (Mikhak et al., 2006; Hanninen et al., 2011; Lupsa et al., 2018; Hoeppli et al., 2019; Xiong et al., 2020). The roles of CCR3 in prostate cancer (Guerard et al., 2021) and CXCR4 in leukemias (Burger and Buerkle, 2007) are good examples although many others including CXCR2 (Hoerning et al., 2011; Whilding et al., 2019) and the CCR2 driven homing of IL-23 expressing cells (Kara et al., 2015) have been well studied.
The spatial resolution of homing can be increased by the formation of complex molecular entities between classical cellular receptors and cytokine receptors. The dopamine receptor family, for example, has wide-ranging involvement in immune cell activity (Matt and Gaskill, 2020), extending to the formation of heteromeric complexes with other agents. The D5 dopamine receptors complex with chemokine receptor CCR9 (Osorio-Barrios et al., 2021) is an essential contributor to the homing of CD4+ T cells to the intestinal mucosa, and inhibiting its formation suppresses the phenomenon. The homing of CD4+ T cells is partly regulated by the interaction between the two T cell differentiating transcription factors FoxP3 and RORγt. The former complexes with AHR to enhance homing while RORγt inhibits homing (Xiong et al., 2020). As these are key targets of kynurenine and its downstream metabolites, this example emphasizes the importance of an integrated cytokine-kynurenine interface and its potential relevance to cellular and molecular complementarity. The value of targeting cytokines has been intensively explored for systemic autoimmune disorders such as arthritis (Malemud and Reddy, 2008; Hausmann, 2019; Kyttaris, 2019).
In the CNS, homing is likely to depend on the varied distributions of cytokines and chemokines localized to small regions of CNS and to specific cell types (Figure 4). One example is IL-1β which, although synthesized and released widely from neurons, astrocytes and microglia, acts on receptors that are restricted largely to granule cells of the dentate gyrus (Ban et al., 1993). This provides a mechanism by which changed levels of peripheral IL-1β may enter the CNS to produce effects that are spatially limited to those cells possessing receptors. The sensitivity of those receptors may be varied by up- or down-regulation within different, restricted areas of CNS, and the differential expression of receptor subunits will also present a different pharmacology (concentration dependence, sensitivity to antagonists etc.) which may be functionally relevant, especially if affected by disease.
Disorders of the CNS are already known in which cell homing may be a powerful factor. In multiple sclerosis, for example, the induced movement of inflammatory Th17 cells into early lesions is thought to be important in establishing myelitic plaque formation and development, and can determine the course of symptoms (Poeta et al., 2019).
Contact complementarity
The concept of homing was developed for cells attracted to a relatively homogeneous tissue or cell type but may not be adequate in the CNS. A more accurate view for neuroimmune communication might be based on ‘contact complementarity.’ This would involve a large number of molecular sites with which migrating cells make contact to interact and function in a complementary fashion. Those sites may encompass only a few – perhaps single – cells. Small groups of leukocytes would express a unique profile of ‘contact complementarity factors’ which interact with corresponding target molecules in the CNS. The most obvious such links would be the immune mediators and their receptors. Similarly, the chemical products of neurons or glia would act on leucocytic cytokine, chemokine, and neurotransmitter receptors (in addition to receptors on other neurons and glia).
Contact complementarity may resemble the spatial location of cells determined by their specific mix of proteins. In mouse brain, for example, microglia have high levels of CD11b, F4/80 and FcγRI in the hippocampus whereas in the frontal cortex CD11b is lower and CX3CR1 (fractalkine) is higher (de Haas et al., 2008). The difference emphasizes that complementarity could be based not only on the presence of a particular molecule, but also on the relative expression of several molecules.
The ability of the proposed mechanism to account for neuroimmune selectivity can be illustrated mathematically. If a group of leukocytes interacted with CNS cells based on the expression of a single molecule then, based on the existence of 100 relevant cytokines and related molecules influencing the CNS, there could be around 100 neuro-immunologically distinguishable regions of CNS. However, since the cell interactions could involve any number of mediators, in different combinations and at densities which may vary in time, there could be many millions of distinguishable CNS regions. This variety could generate localized activity affecting small groups of CNS neurons depending on the state of the cells concerned, local physiological inputs and the specific local extracellular chemical composition. It is this spectrum of contacts which could be prominently affected by the widespread changes in cell activity and polarization produced by environmentally modulated changes in IDO1 expression and its tryptophan metabolites.
Not surprisingly there will be exceptions to these rules, as some cytokines show a degree of non-selectivity for their receptors. Although some pairings are highly specific, as with the interaction between CXCL12 and its receptor CXCR4, some ligands can affect several receptor populations, and individual receptor subtypes may respond to multiple ligands (Deverman and Patterson, 2009). This implies that even at the ligand-receptor level, there is variability in the precise variety of receptors, and the relative concentrations of several ligands able to act on them. However, it is not the presence of any single ligand which will determine cell behavior, but the combined and relative presence of several.
Even the relatively few leukocytes within the CNS parenchyma or meninges would contribute to this regional complexity, since their products would access receptors on thousands of CNS cells (around 1 million per microliter). Complexity would be further enhanced by the diffusion and dilution gradients of compounds from several leukocytes.
In the CNS, the expression of complementarity factors will be influenced by the level of local neuronal activity (Figure 4). Similarly, glial polarization and activation, influenced by neuronal activity will alter the supply and removal of substrates and products. Changes in the kynurenine pathway (in neurons, astrocytes, glia or leukocytes), will produce corresponding effects on neuronal excitability. Leukocytes whose expression of complementarity factors or kynurenines has been modified by peripheral events will play a major part in these interactions, communicating and translating peripheral events in the immune system into neuronal modulation in the CNS.
The overall outcome of these interactions will be to generate a complex intercellular chemical matrix which would generate a different ‘map grid reference’ for each point within the CNS, rather like the point-to-point representations of the body surface in the sensory neocortex. The degree of commonality or overlap between cells in this chemical map will then (as for a zip or post code) determine the size and composition of each region.
Temporal aspects
There may also be a temporal aspect to cytokine-kynurenine interaction. Activation of IDO1 can occur over the first few hours of an appropriate stimulus, remaining elevated for 24 h or more, whereas IL-1β, IL-6 and TNF decline much more rapidly (Clanchy et al., 2022). Enzymes downstream of IDO1 (Figure 1) may become active in a similar time frame, even in cells lacking IDO (Belladonna et al., 2006).
In contrast, cytokines can exhibit a very wide range of time courses which are likely to amplify functional differences between the cytokines, as for IL-1 or IL-6 (Grellner, 2002; Uchida et al., 2007; Bai et al., 2008), IL-18 (Barrientos et al., 2009; Witt et al., 2011), IL-4 and IL-10 (van Meegeren et al., 2013), IL-15 (Shamsi et al., 2015), and IL-17 (Loof et al., 2016). Younger et al. (2019) summarize data showing that cytokines and their receptors are affected by injury on time scales of a few hours to several days, with similar conclusions after stress (Fatouros and Jamurtas, 2016). However, emphasizing the spectrum of cytokine activity, the administration of LPS to rats induced a peak of TNF and IL-1 expression in the plasma approximately 1 h later, while IL-1β, IL-1ra and IL-8 levels were detected 2–5 h after an allergy challenge in humans (Wagenmann et al., 2005). The use of fresh tissue may be more helpful, but the stimulation (by PMA) of fresh blood cells collected after burn injuries indicated peak levels of IL-6 or IL-8 several days later, a time frame far slower than kynurenine responses (Finnerty et al., 2006; Csontos et al., 2010). Cytokine induction after strokes can be similarly delayed (Nayak et al., 2012).
The existence of such a breadth in the temporal range of cytokine expression and activity would overlap that of the kynurenine pathway, such that the initiation, development or termination of cytokine activity could be modulated by the more generalized effects of kynurenine and its metabolites. This gives rise to the proposal that kynurenines provide a continually shifting ‘tonic’ baseline of cell activity, onto which the ‘phasic’ effects of immune system mediators are superimposed.
Structural and functional factors underlying cytokine-kynurenine integration
If, as proposed here, the kynurenine pathway and cytokines function in a complementary fashion, partly accounting for the weak or conflicting correlations between tissue levels of either family of compounds in relation to disease symptoms or severity, how and where does this integration occur? This section addresses the question by considering the most important concept of ‘volume transmission,’ with additional comments on the contributions of glial cells and the BBB. Together, these features define the physical, structural factors involved in the neuroimmune interface.
Mechanisms of integration: Volume transmission
The classical concept of synaptic transmission by fast neurotransmitters was that they acted at tightly apposed synaptic junctions, on a timescale of milliseconds, to modulate the polarization of neurons. Using the dominant amino acid glutamate as an example, fast transmission was mediated by AMPA and kainate receptors, with a slower excitation by NMDARs which generated much of the plasticity-based changes underlying behavior and cognition (Kandanearatchi and Brew, 2012; Dounay et al., 2013; Stone and Darlington, 2013; Varga et al., 2015; Kilonzo et al., 2021; McQuail et al., 2021). The subsequent activation of G-protein linked metabotropic glutamate receptors (Pacheco et al., 2004) extends the time frame of neuromodulation from seconds to minutes or hours.
This view became modified by the recognition that slow neurotransmitters such as the monoamines were often released from axonal varicosities into a much larger volume of extracellular space, and this idea evolved further into the concept of “volume transmission” (Figure 5). The term encompasses the evidence that the release of most neuroactive compounds is not confined to the synaptic gap, but can arise from neuron terminals, somata, dendrites, axons, microglia, and astrocytes (Fuxe et al., 2007; Guidolin et al., 2007; Fuxe and Borroto-Escuela, 2016). This applies to fast transmitters as much as to peptides and proteins such as cytokines. Since receptors for many of the released compounds are also expressed on a broad range of cells (Christensen et al., 2016a,b; Figures 2, 3), their activation will depend on the overall quantity and time course of release from all these sources. These parameters will be highly variable since, for example, glutamate release is partly exocytotic from neurons and microglia and partly by efflux transporters, whereas astrocytes secrete glutamate in a more diffuse, continuous non-exocytotic manner (Malarkey and Parpura, 2008; Cresto et al., 2019; Mahmoud et al., 2019). The final concentration of any particular compound will therefore be a complex balance between synthesis, release and removal by transporters (Figure 5), with all these processes being subject to the rate and pattern of neuronal activity and the state of rest or activation of the glia (Acarin et al., 2000; Nimmerjahn et al., 2005; Paolicelli and Gross, 2011; Paolicelli et al., 2011; Erta et al., 2012; Trettel et al., 2020).
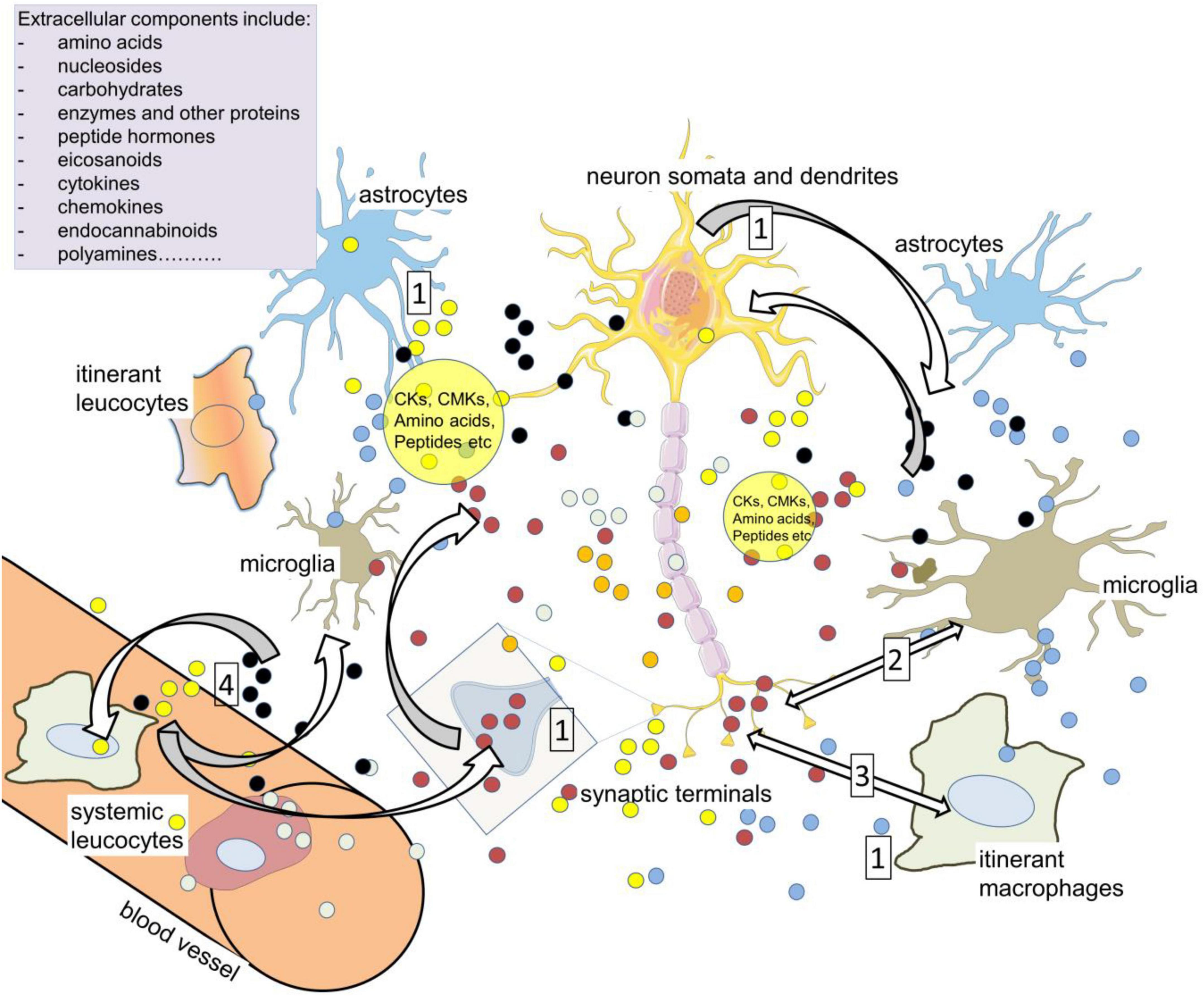
Figure 5. Volume transmission as a vehicle of neuroimmune communication. Compounds in the extracellular spaces of the CNS are released from neurons, glia, axons (including varicosities), cell bodies and dendrites in addition to synaptic terminals (1). Among the most active, relevant compounds are neurotransmitters such as glutamate, cytokines (CKs), and chemokines (CMKs), all of which can influence cell activity depending on the local expression of receptors and transporters, and all of which can act on – and be released by – resident and itinerant leukocytes. The large volume of brain tissue exposed to so many sources of active compounds led to the concept of ‘volume transmission.’ The primary neuroactive substances promote CK release (2) while CK receptors can release neurotransmitters and related compounds (3). The cytokines can also directly modulate neuron and synaptic function, affecting excitability and plasticity. The major neuroactive kynurenine metabolites include kynurenic acid (glutamate antagonist) and quinolinic acid (NMDAR agonist). The extracellular compounds may act on leukocytes in the CNS which later re-enter the circulation and interact with peripheral leukocytes (4). Kynurenine can enter and leave cells relatively readily, acting as a paracrine agent to maintain or enhance levels in nearby cells.
Since CNS cells secrete and respond to a variety of growth factors and cytokines, including IL-1β, IL-6, and TNF in addition to conventional neurotransmitters and neuromodulators (such as purines, peptides, endocannabinoids etc.), volume transmission will generate a highly complex medium in which active compounds can modulate the activity of neurons and glia with the appropriate receptors or targets at much greater distances than across synapses (Figure 3). The cell groups affected would be limited in size and localized to a restricted region of the CNS as determined by homing and complementarity factors, as discussed above. Theoretically, however, every cell in the CNS is exposed to a different extracellular medium composition, allowing almost a cell-to-cell communication between the CNS and immune system.
Neuroimmune transfer of information
Indeed, a central tenet of the neuroimmune concept is that leukocyte populations will participate in these chemical exchanges, responding to neuroglial secreted transmitters, modulators and cytokines, but also adding their various products of cytokines and proteins into the extracellular matrix. This will apply to resident and itinerant leukocytes in the CNS, so that the information on neuron and glial activity represented by the chemical composition of the CNS extracellular medium will be imprinted onto the cohort of leukocytes present in the CNS at that time. As those leukocytes eventually return to the systemic circulation, any subtle changes in phenotype will influence their interactions with other immune system cells, modifying their activity or differentiation and proliferation such that even a small number of cells ‘conditioned’ by the CNS extracellular medium could have a significant impact on immune system function.
Equally, of course, the molecular information in leukocytes entering the CNS will be determined by the state of the immune system in the previous minutes or hours, allowing the imprinting of that information onto neuron and glial activity.
It has been suggested that the level of local neural activity influences cytokine production and receptor expression which then generate a differential attraction of immune system cells into the CNS, further linking neural activity with neuroimmune communication (Kamimura and Murakami, 2019). Such a mechanism could well be a factor in refining these concepts.
Additional transfer mechanisms
In addition to the release and uptake of molecules by transporters or diffusion, cells may secrete high molecular weight compounds such as proteins and nucleic acids packaged into vesicles (exosomes) up to 100 nm diameter (Agnati and Fuxe, 2014). Transfer of the chemokine receptor and viral docking protein CCR5 provides an example of molecules which can be distributed to other cells in secreted exosomes (Camussi et al., 2011; Guo et al., 2018). Cells can also communicate by the formation of ‘nanotubes’ (Agnati et al., 2010; Agnati and Fuxe, 2014). These inter-cellular structures, approximately 200 nm in diameter, are up to ∼50 μm in length through which large molecules and complete intracellular vesicles can be transferred. They can be formed between different cell populations such as neurons and astrocytes, expanding the potential biological importance of moving high molecular components between phenotypically dissimilar pools. These processes may transfer key molecules from stressed or injured cells to healthy ones (Agnati and Fuxe, 2014). Most importantly for the present discussion is the possibility that they may allow the transfer of cell membrane molecules and receptors for neuromodulatory compounds (Bukoreshtliev et al., 2009; Agnati et al., 2010), creating a shifting pattern of cell phenotypes dependent on chemical influences and temporal changes.
Mechanisms of integration: The blood-brain barrier
In addition to the complexity of volume transmission, the presence of a BBB will itself modify the movement of compounds to and from the CNS (Figure 4), contributing to the contradictory correlations noted above (section “Limitations and uncertainties: Concentrations and correlations”). Despite their protein nature and size (IL-1β is ∼17.5 kDa), many immune system mediators can cross the BBB structurally unchanged even in adult animals (Broadwell and Sofroniew, 1993; Capuron and Miller, 2011) reaching concentrations which can influence animal behavior (Banks et al., 2001, 2002). IL-1α, IL-1β, and IL-1ra have active transport into the CNS (Banks et al., 1989, 1994; Gutierrez et al., 1994). These are specific processes, as unrelated cytokines such as IL-2 were not transported (Waguespack et al., 1994). Conversely the active transport of IL-6 is not affected by IL-1 (Banks et al., 1994). Brain region-specific actions of some cytokines may result from regional variations in BBB function and selectivity (Moinuddin et al., 2000). In so far as neuroglial activity may influence specific transport processes such as these, it would contribute to general features of neuroimmune communication.
As with much larger proteins, cytokines (including chemokines) can also enter the CNS through leaky regions such as the circumventricular organs (CVO) (Konsman et al., 2008). Some, including IL-1β, act on cerebrovascular endothelial cells to stimulate the production of more IL-1β which is secreted into the brain parenchyma (Figure 4). As small amounts of IL-1β reach the parenchyma, they may activate glial cells to induce further IL-1β production and release into the extracellular space of the CNS, able to spread its influence by acting successively on other glia and neurons. Other proteins known to cross in CVO areas include IL-17A (Chen et al., 2022), angiotensin (McKinley and Oldfield, 1998), and ghrelin (Uriarte et al., 2021).
Some smaller compounds ‘leak’ across the capillary endothelial layer outside the CVO to reach biologically active concentrations within the CNS. A classical example is morphine which crosses the barrier poorly but still sufficiently to induce a rapid and effective analgesia (Banks et al., 1995). Some compounds bypass the barrier by transcellular processes involving movement by cytoskeletal networks, while some are moved within intracellular vesicles which afford protection from modification. Molecules moving in this way include large molecular weight proteins such as insulin and transferrin and smaller but critically important trophic peptides such as NGF, BDNF (Crowe and Morgan, 1992; Pan et al., 1998a,b) the glycoprotein erythropoietin and related compounds (Banks et al., 2004).
Leukocytes
Intact leukocytes can cross or by-pass the BBB giving them, and the cytokines they express, direct access to the CNS parenchyma. In particular, elements of later stage, adaptive immune activity including T cells are able to access the CNS via diapedesis across the choroid plexus and by the binding between chemokine CCL20 and its chemoattractant receptor CCR6 (Axtell and Steinman, 2009). This system is particularly important in the access of Treg and Th17 cells and their penetration into regions of the limbic system (Sallusto et al., 2012). Here again IL-1β is involved as it acts on its receptor on endothelial, ependymal and astrocytic cells to induce the expression of CCL2 and promote cell migration. Indeed, IL-1β is often viewed as a ‘Trojan horse’ of the immune system as it increases the overall permeability of the BBB (Shaftel et al., 2007), facilitating the ingress of other cytokines and intact leukocytes (Figure 4). TNF plays a significant role in the attraction of monocytes into the CNS (D’Mello et al., 2009).
Leukocytes also gain access from the vascular circulation to the arachnoid mater, from where they can enter the CSF and the brain parenchyma through the meninges (Schlager et al., 2016). Among the leukocytes with this ability are several functionally distinct subsets of CD4+ T-cells, with evidence that marked changes of learning are associated with neuronal loss in the hippocampus following the depletion of peripheral CD4+ T cells, implying that the presence of these cells makes a significant contribution to neuronal and synaptic plasticity (Wolf et al., 2009). It is also claimed that populations of CD4+ T lymphocytes are partly responsible for neuronal damage (Liblau et al., 2013) although this can also be caused by CD8+ T cells penetrating the CNS and releasing destructive enzymes and cytokines (Medana et al., 2000; Huse et al., 2008; Liblau et al., 2013).
Mechanisms of integration: Glial cells
Microglia are derived embryologically from monocytic myeloid cells and phenotypically they resemble systemic macrophages (Ginhoux et al., 2010). They produce a variety of cytokines including IL-6, IL-1β, and TNF, and chemokines such as CCL1, CCL5, CCL12, some of which induce the migration of systemic immune system cells (Hanisch, 2002; Kettenmann et al., 2010; Kraft and Harry, 2011). They may also produce eicosanoids, growth factors, purines, neuropeptides, polyamines, proteases and other compounds which might fulfill niche roles in specific aspects of immuno-neural communication (Sternberg, 2006; Hanisch and Kettenmann, 2007; Muzio et al., 2007; Fabry et al., 2008; Ekdahl et al., 2009; Talbot et al., 2016; Pavlov and Tracey, 2017; Kanashiro et al., 2020; Scheiblich et al., 2020). Microglia also identify and destroy aberrant and non-self cells, often presenting fragments as antigens (Miyanishi et al., 2021).
Once systemic monocytes have crossed into the CNS, the mediators they release activate resident microglia to become motile and to secrete mediators which modify neuronal and synaptic function (Ransohoff and Perry, 2009; Spulber and Schultzberg, 2010; Garay et al., 2013; Giovanoli et al., 2016). The activation of TLR4 on microglia induces their transformation from a stabilizing M2 to a reactive M1 phenotype (Rodriguez-Gomez et al., 2020). This may be crucial in the ability of microglia to influence neuronal degeneration, regeneration and neurogenesis in the aftermath of CNS injury or infection (Leiter et al., 2016; Diaz-Aparicio et al., 2020).
It has been suggested that a population of immune system-derived ‘extra-neural’ cells exists in the meninges and choroid tissue. While their relationship to other cell types is not clear, they may respond to foreign molecules and regulate central neuroglial activity by secreting their own spectrum of compounds (Becher et al., 2006; Tambuyzer et al., 2009), generating a ‘microglia-cytokine axis’ (Werneburg et al., 2017). These cells may play a specialized role in regulating axo-dendritic development and synapse formation, affecting network development and neuronal plasticity (Paolicelli and Gross, 2011; Paolicelli et al., 2011).
Receptors
Integration of the CNS and immune systems exists even at the level of individual receptors. The synaptic regions of neurons have a concentrated expression of IL-1 receptor (IL-1R) which can occur physically associated with the GluN2B subunit of NMDARs (Gardoni et al., 2011). Both IL-1β and NMDA can modify the expression of these complexes and their precise localization within the synapse. Immune system mediators secreted by microglia can modulate synaptic neurotransmission by altering the ratio of glutamate expression and sensitivity to NMDA, AMPA or kainate (Bauer et al., 2007; Bessis et al., 2007; Masgrau et al., 2017). Indeed, lymphocyte-generated cytokines can induce the expression, in microglia, of receptors and transporters for glutamate GABA, acetylcholine and catecholamines (Lisak et al., 2009), providing an almost direct ability of cytokines to interact with these and modulate neuronal firing and plasticity. These molecular interactions can be bi-directional: IFN-γ potentiates GABA-mediated neuronal inhibition (Flood et al., 2019) while GABA inhibits microglial activation (Bjurstom et al., 2008).
A novel perspective on the neuroimmune relationship has been gained from interfering with the D3 dopamine receptor, which is localized exclusively to CNS microglia. Blockade or deletion of D3 caused activation of those glia, with symptoms of depression and increased levels of IL-1β, IL-6, and TNF in dopaminergic neurons (Wang et al., 2020; Bassett et al., 2021).
Neuronal influences on immune system cells
The discussion of immune system effects on the CNS is probably easier to conceptualize than the converse interaction, so it is important to note experimental examples showing how CNS activity directly influences the immune system.
The reciprocal production of secreted neuro-glial transmitters (Biber et al., 2007), neuromodulators and cytokines is reflected in the existence of receptors for glutamate on glial cells and leukocytes, illustrated by the glutamate-induced release of IL-8 and IL-10 from lymphocytes (Kvaratskhelia et al., 2009). The release of IL-10 from microglia is potentiated by presynaptic NMDAR activity leading to increased postsynaptic activation of non-NMDA (AMPA and kainate) receptors (Paetau et al., 2017; Stone, 2021). Kynurenic acid blocks many of these receptors, indirectly affecting mediator release.
Intracerebral injections of NMDA increased the number of NK cells in the systemic circulation, with increased levels of TNF. These responses were reduced by mild stress acting on CNS efferent glutamate-mediated (NMDAR-activated) pathways demonstrating their ability to influence systemic immunological function (Podlacha et al., 2016).
Mild stress also increased CNS microglial activation and cytokine expression (Ben-Shaanan et al., 2016; Jones et al., 2018; Du Preez et al., 2021). However, the cytokine changes were detected in both the CNS microglia and in systemic macrophages (Dantzer, 2006) but were not the basolateral amygdala or perirhinal cortex (Jones et al., 2018). The changes occurred in parallel with improved learning and so were not dependent on cytokine movements from periphery to CNS. They were presumably induced by stress-induced neuroglial activation in the hippocampal region from where they reached the systemic circulation.
Efferent neuroimmune communication is clear in the stimulation of limbic dopaminergic reward neurons of the Ventral Tegmental Area, which activates the peripheral immune system (Luscher and Malenka, 2011; Matt and Gaskill, 2020). Dopamine is important in movement and locomotion, and in the regulation of affective states and psychotic disorders. Microglial dopamine receptors (Luscher and Malenka, 2011; Matt and Gaskill, 2020) mediate structural and biochemical changes. Most lymphocyte populations release dopamine (Fan et al., 2018; Talhada et al., 2018) and express dopamine receptors (Cosentino et al., 2007; Huck et al., 2015; Levite, 2016; Talhada et al., 2018) although there are differences between T cell subsets, and between resting and activated cells (Besser et al., 2005; Watanabe et al., 2006). Leukocyte dopamine receptors, and those on CNS and systemic macrophages, affect cytokine production, cell adhesion properties, and mobility (Kirillova et al., 2008; Mignini et al., 2013; Coley et al., 2015; Nolan et al., 2019). The Th17 population of CD4+T cells is involved in the dopaminergic regulation of multiple sclerosis, arthritis, inflammatory bowel disease and Parkinson’s disease (Melnikov et al., 2018, 2020; Capellino, 2020), supporting the profound mutual influence of the immune and nervous systems.
Dietary and microbial influences
The potential role of intestinal microbiota in modulating the host neuroimmune systems has been the subject of several reviews (Anderson et al., 2019; Cryan et al., 2019; Berding et al., 2021; Spichak et al., 2021). Tryptophan is not synthesized by mammalian tissues and is mainly obtained from dietary sources. In contrast, bacteria synthesize tryptophan in the shikimate pathway via anthranilic acid and, while the tryptophan is then available for protein synthesis, bacteria also catabolize it along the kynurenine pathway. Since tryptophan, kynurenine and 3HK are transported readily across the intestinal epithelium, their movements will affect levels of kynurenine and its metabolites in the bacteria and host. Changes in diet, disease or drugs affecting the intestinal microbiome will then have a significant impact on host neural, immune and behavioral factors. This view may be related to the concept that anthranilic acid levels seem to reflect the presence of infection or inflammation (Darlington et al., 2010; Badawy, 2018).
The recent discovery that the bacterial anthranilic acid derivative Pseudomonas Quorum Sensor (PQS; 2-heptyl-3-hydroxy-4-quinolone) modulates cytokine release and IDO expression in murine and human cells is an important extension to this concept. It reveals that a microbially important molecule, derived from the metabolism of tryptophan and which is not produced by mammals, can have highly significant effects on the host immune system (Ogbechi et al., 2022). The implication would be that changes in bacterial or host tryptophan metabolism could have reciprocal effects on both organisms as an example of ‘inter-kingdom signaling’ (Pacheco and Sperandio, 2009) between prokaryotes and eukaryotes.
Since IDO1 substrates include several indole derivatives produced by bacteria, the kynurenine pathway may represent a critical evolutionary development to detoxify and destroy chemicals not produced by the host organism. This would be consistent with the expression of AHR and their activation by kynurenine or kynurenic acid leading to the activation of catabolic cytochrome oxidases as well as the expression of transcription factors such as FoxP3 and RORγt which define the differentiation of Treg and Th17 cells respectively (Omenetti and Pizarro, 2015; Morris et al., 2017; Su et al., 2022). The Th17 cells concentrated in the intestinal walls and Peyer’s patches could exert a profound influence on host immunity (Bhaumik and Basu, 2017; Cheng et al., 2019; Chen and Tang, 2021), modulated by dietary and microbial tryptophan and anthranilic acid. Interestingly, one of the most potent known activators of AHR is FICZ, an endogenous metabolite produced by the photo-oxidation of tryptophan.
Implications for drug development
To date, much drug development research has concentrated on individual compounds or families and specific molecular targets but this may lead to, at best, treatments for small cohorts of patients or, at worst, failure to achieve significant results across a varied, inadequately characterized population. If major examples of molecular interaction, such as has been attempted here, prove to be valid then a combined screening of relevant compounds in different families might be more fruitful, leading to therapeutic agents or combination thereof, which are more beneficial for more patients.
In the case of cytokines and kynurenines recent results lend support to this view with evidence that cytokines and kynurenine metabolites overlap in their relevance to clinical disorders. Comai et al. (2022) have noted that the respective contributions of these molecular groups may contribute to the differential etiology of MDD and BD. This is consistent with current clinical views on these conditions, but the introduction of a molecular basis for their differences could lead to an increasingly refined diagnosis and selection of patients for drug trials. That, in turn, could lead to drugs which are much more disease and symptom-specific.
Reinforcing this argument, few cytokine-related drug candidates have reached clinical trials, or those that have done so have proved insufficiently active or selective (Poeta et al., 2019; Lai and Mueller, 2021). The difficulty may lie in the potency of many cytokines coupled with the need to target a limited number of immune system cells or a restricted region of CNS. Reaching a sufficient concentration of drug within a limited volume of tissue will almost certainly be associated with unwanted effects elsewhere, especially given the relative non-selectivity of some cytokine receptors (Deverman and Patterson, 2009).
The kynurenine pathway is also the subject of much activity in drug discovery, with several clinical trials in progress (Pires et al., 2022). Autoimmune disorders represent a major focus for kynurenine-related drug development (Baumgartner et al., 2019; Huang et al., 2020, 2021; Ogbechi et al., 2020; Krupa and Kowalska, 2021) together with cancer (Prendergast et al., 2017, 2018; Muller et al., 2019; Opitz et al., 2020; Walczak et al., 2020a,b) and other conditions (Dounay et al., 2013, 2015; Kozak et al., 2014; Jayawickrama et al., 2015; Smith et al., 2016; Zadori et al., 2016; Jacobs et al., 2017).
In the CNS, both quinolinic acid and kynurenic acid have been implicated in Huntington’s disease (Stone, 1993, 2000a,b; Stone and Darlington, 2002; Thevanavakkam et al., 2010), stroke (Darlington et al., 2007), multiple sclerosis and related conditions (Sundaram et al., 2014; Lee et al., 2017; Lovelace et al., 2017; Boros et al., 2018; Majlath et al., 2018; Platten et al., 2019), schizophrenia (Erhardt et al., 2017), MDD, suicidality (Brundin et al., 2016, 2017) and other disorders.
For more than 50 years it has been thought that some depressive disorders are caused by lowered levels of 5HT in the CNS, supported by the apparent therapeutic success of Selective Serotonin Reuptake Inhibitors (SSRIs), even though counseling can be more effective (Mackay et al., 2009). However, the serotonin hypothesis has been brought into serious doubt by Moncrieff et al. (2022) who concluded that there is a lack of firm supporting that view. This may make it more likely that the kynurenine pathway has a more important role in depressive disorders and may be the target of future antidepressant therapies. Broadening the understanding of kynurenine pathway activity and interactions across the neuroimmune interface may promote this change of perspective to the benefit of many patients with a variety of complex disorders.
Summary and conclusion
The concept of a neuroimmune interface attempts to explain how systemic insults can affect brain function and how aspects of behavior affect the immune system. The kynurenine pathway is viewed as pivotal in these interactions, mediating the translation of CNS neural activity to changes in the surveillance, defensive and tolerance activities of the immune system. Conversely, the latent presence of the kynurenine pathway in cells of the immune system, under the control of cytokines and chemokines, enables immune system activity to influence the CNS. Similarly, activity in peripheral immune cells, with the immunologically active and neuroactive factors they produce represents an additional force modulating neuronal and glial functions, contributing to subtle changes in a variety of behaviors. Overall, the low intensity modulation of neuronal excitability and synaptic plasticity by glutamate, NMDARs and the kynurenine pathway, it is proposed, lays the shifting foundation of a ‘tonic’ modulation of neuronal and glial activity upon which the ‘phasic’ effects of immune system modulators are superimposed at a localized, perhaps even cellular, level. Efforts to explain clinical disorders and to develop new diagnostic tools or treatments should take into account both the immune system mediators and kynurenine pathway metabolites for a more complete explanation and understanding of the neuroimmune and immuno-neural interface. The potential for producing highly localized changes in selected CNS regions could improve the safety, tolerability and efficacy of drugs for a range of psychiatric and neurological disorders as well as autoimmune conditions.
Author contributions
TS wrote the initial draft. FC, Y-SH, N-YC, LD, RW, and TS contributed to the subsequent discussions and modifications. All authors contributed to the article and approved the submitted version.
Funding
Y-SH was supported by Wellcome Trust funding reference: 104025/Z/14/Z Cambridge.
Conflict of interest
The authors declare that the research was conducted in the absence of any commercial or financial relationships that could be construed as a potential conflict of interest.
Publisher’s note
All claims expressed in this article are solely those of the authors and do not necessarily represent those of their affiliated organizations, or those of the publisher, the editors and the reviewers. Any product that may be evaluated in this article, or claim that may be made by its manufacturer, is not guaranteed or endorsed by the publisher.
Abbreviations
3HK, 3-hydroxy-kynurenine; 3HAA, 3-hydroxyanthranilic acid; AHR, aryl hydrocarbon receptor; BDNF, brain-derived neurotrophic factor; CNS, central nervous system; CSF, cerebrospinal fluid; GM-CSF, granulocyte-macrophage colony-stimulating factor; IDO-1, indoleamine 2, 3-dioxygenase-1; IDO2, indoleamine 2, 3-dioxygenase 2; KAT, kynurenine aminotransferase; KMO, kynurenine monooxygenase; kynurenine-3-hydroxylase; NMDA, N–methyl–D–aspartate; TDO2, tryptophan 2,3-dioxygenase; TLR, toll-like receptor.
References
Acarin, L., Gonzalez, B., and Castellano, B. (2000). Neuronal, astroglial and microglial cytokine expression after an excitotoxic lesion in the immature rat brain. Eur. J. Neurosci. 12, 3505–3520. doi: 10.1046/j.1460-9568.2000.00226.x
Agnati, L. F., and Fuxe, K. (2014). Extracellular-vesicle type of volume transmission and tunnelling-nanotube type of wiring transmission add a new dimension to brain neuro-glial networks. Philos. Trans. R. Soc. B 369:20130505. doi: 10.1098/rstb.2013.0505
Agnati, L. F., Guidolin, D., Guescini, M., Genedani, S., and Fuxe, K. (2010). Understanding wiring and volume transmission. Brain Res. Rev. 64, 137–159. doi: 10.1016/j.brainresrev.2010.03.003
Alberati-Giani, D., RicciardiCastagnoli, P., Kohler, C., and Cesura, A. M. (1996). Regulation of the kynurenine metabolic pathway by interferon-gamma in murine cloned macrophages and microglial cells. J. Neurochem. 66, 996–1004. doi: 10.1046/j.1471-4159.1996.66030996.x
Albini, E., Rosini, V., Gargaro, M., Mondanelli, G., Belladonna, M. L., Pallotta, M. T., et al. (2017). Distinct roles of immunoreceptor tyrosine-based motifs in immunosuppressive indoleamine 2,3-dioxygenase-1. J. Cell. Mol. Med. 21, 165–176. doi: 10.1111/jcmm.12954
Alboni, S., Benatti, C., Montanari, C., Tascedda, F., and Brunello, N. (2013). Chronic antidepressant treatments resulted in altered expression of genes involved in inflammation in the rat hypothalamus. Eur. J. Pharmacol. 721, 158–167. doi: 10.1016/j.ejphar.2013.08.046
Alboni, S., Cervia, D., Sugama, S., and Conti, B. (2010). Interleukin-18 in the CNS. J. Neuroinflamm. 7:9. doi: 10.1186/1742-2094-7-9
Alboni, S., Montanari, C., Benatti, C., Blom, J. M. C., Simone, M., Brunello, N., et al. (2011). Constitutive and LPS-regulated expression of interleukin-18 receptor beta variants in the mouse brain. Brain Behav. Immun. 25, 483–493. doi: 10.1016/j.bbi.2010.11.011
Alexander, G. M., Reichenberger, E., Peterlin, B. L., Perreault, M. J., Grothusen, J. R., and Schwartzman, R. J. (2013). Plasma amino acids changes in complex regional pain syndrome. Pain Res. Treat. 2013:742407. doi: 10.1155/2013/742407
Alkondon, M., Pereira, E. F. R., Todd, S. W., Randall, W. R., Lane, M. V., and Albuquerque, E. X. (2015). Functional G-protein-coupled receptor 35 is expressed by neurons in the CA1 field of the hippocampus. Biochem. Pharmacol. 93, 506–518. doi: 10.1016/j.bcp.2014.12.009
Anderson, G., and Rodriguez, M. (2011). Multiple sclerosis, seizures and anti-epileptics: Role of IL-18. IDO and melatonin. Eur. J. Neurol. 18, 680–685. doi: 10.1111/j.1468-1331.2010.03257.x
Anderson, R., Theron, A. J., and Rapoport, B. L. (2019). Immunopathogenesis of immune checkpoint inhibitor-related adverse events: Roles of the intestinal microbiome and Th17 cells. Front. Immunol. 10:2254. doi: 10.3389/fimmu.2019.02254
Andersson, J., Tran, D. Q., Pesu, M., Davidson, T. S., Ramsey, H., O’Shea, J. J., et al. (2008). CD4(+)FoxP3(+) regulatory T cells confer infectious tolerance in a TGF-beta-dependent manner. J. Exp. Med. 205, 1975–1981. doi: 10.1084/jem.20080308
Andre, C., O’Connor, J. C., Kelley, K. W., Lestage, J., Dantzer, R., and Castanon, N. (2008). Spatio-temporal differences in the profile of murine brain expression of proinflammatory cytokines and indoleamine 2,3-dioxygenase in response to peripheral lipopolysaccharide administration. J. Neuroimmunol. 200, 90–99. doi: 10.1016/j.jneuroim.2008.06.011
Anekthanakul, K., Manocheewa, S., Chienwichai, K., Sathirapongsasuti, N., Kiyakara, C., Khoomrung, A., et al. (2021). Predicting lupus membranous nephritis using reduced picolinic acid to tryptophan ratio as a urinary biomarker. iScience 24:103355. doi: 10.1016/j.isci.2021.103355
Aniszewska, A., Chlodzinska, N., Bartkowska, K., Winnicka, M. M., Turlejski, K., and Djavadian, R. L. (2015). The expression of interleukin-6 and its receptor in various brain regions and their roles in exploratory behavior and stress responses. J. Neuroimmunol. 284, 1–9. doi: 10.1016/j.jneuroim.2015.05.001
Antonioli, L., Pacher, P., Vizi, E. S., and Kasko, G. (2013). CD39 and CD73 in immunity and inflammation. Trends Mol. Med. 19, 355–367. doi: 10.1016/j.molmed.2013.03.005
Arnone, D., Saraykar, S., Salem, H., Teixeira, A. L., Dantzer, R., and Selvaraj, S. (2018). Role of kynurenine pathway and its metabolites in mood disorders: A systematic review and meta-analysis of clinical studies. Neurosci. Biobehav. Rev. 92, 477–485. doi: 10.1016/j.neubiorev.2018.05.031
Ascher, P., and Nowak, L. (1987). Electrophysiological studies of NMDA receptors. Trends Neurosci. 10, 284–288. doi: 10.1016/0166-2236(87)90174-3
Axtell, R. C., and Steinman, L. (2009). Gaining entry to an uninflamed brain. Nat. Immunol. 10, 453–455. doi: 10.1038/ni0509-453
Baban, B., Chandler, P. R., Sharma, M. D., Pihkala, J., Koni, P. A., Munn, D. H., et al. (2009). IDO activates regulatory T cells and blocks their conversion into Th17-Like T cells. J. Immunol. 183, 2475–2483. doi: 10.4049/jimmunol.0900986
Babcock, T. A., and Carlin, J. M. (2000). Transcriptional activation of indoleamine dioxygenase by interleukin 1 and tumor necrosis factor alpha in interferon-treated epithelial cells. Cytokine 12, 588–594. doi: 10.1006/cyto.1999.0661
Badawy, A. A. B. (2018). Kynurenic and quinolinic acids: The main players of the kynurenine pathway and opponents in inflammatory disease. Med. Hypotheses 118, 129–138. doi: 10.1016/j.mehy.2018.06.021
Badawy, A. A.-B. (2017). Kynurenine pathway of tryptophan metabolism: Regulatory and functional aspects. Int. J. Tryp. Res. 10:1178646917691938. doi: 10.1177/1178646917691938
Bai, R. F., Wan, L. H., and Shi, M. S. (2008). The time-dependent expressions of IL-1 beta, COX-2, MCP-1 rnRNA in skin wounds of rabbits. Forensic Sci. Int. 175, 193–197. doi: 10.1016/j.forsciint.2007.07.006
Balasingam, V., and Yong, V. W. (1996). Attenuation of astroglial reactivity by interleukin-10. J. Neurosci. 16, 2945–2955. doi: 10.1523/JNEUROSCI.16-09-02945.1996
Ban, E. E., Sarlieve, L. L., and Haour, F. G. (1993). Interleukin-1 binding-sites on astrocytes. Neuroscience 52, 725–733. doi: 10.1016/0306-4522(93)90421-B
Banks, W. A., Farr, S. A., and Morley, J. E. (2002). Entry of blood-borne cytokines into the central nervous system: Effects on cognitive processes. Neuroimmunomodulation 10, 319–327. doi: 10.1159/000071472
Banks, W. A., Farr, S. A., La Scola, M. E., and Morley, J. E. (2001). Intravenous human interleukin-1 alpha impairs memory processing in mice: Dependence on blood-brain barrier transport into posterior division of the septum. J. Pharmacol. Exp. Ther. 299, 536–541.
Banks, W. A., Jumbe, N. L., Farrell, C. L., Niehoff, M. L., and Heatherington, A. C. (2004). Passage of erythropoietic agents across the blood-brain barrier: A comparison of human and murine erythropoietin and the analog darbepoetin –α. Eur. J. Pharmacol. 505, 93–101. doi: 10.1016/j.ejphar.2004.10.035
Banks, W. A., Kastin, A. J., and Broadwell, R. D. (1995). Passage of cytokines across the blood-brain barrier. Neuroimmunomodulation 2, 241–248. doi: 10.1159/000097202
Banks, W. A., Kastin, A. J., and Durham, D. A. (1989). Birectional transport of interleukin-1-alpha across the blood-brain-barrier. Brain Res. Bull. 23, 433–437. doi: 10.1016/0361-9230(89)90185-8
Banks, W. A., Kastin, A. J., and Gutierrez, E. G. (1994). Penetration of interleukin-6 across the murine blood-brain-barrier. Neurosci. Lett. 179, 53–56. doi: 10.1016/0304-3940(94)90933-4
Baran, H., and Schwarcz, R. (1990). Presence of 3-hydroxyanthranilic acid in rat-tissues and evidence for its production from anthranilic acid in the brain. J. Neurochem. 55, 738–744. doi: 10.1111/j.1471-4159.1990.tb04553.x
Barrientos, R. M., Frank, M. G., Hein, A. M., Higgins, E. A., Watkins, L. R., Rudy, J. W., et al. (2009). Time course of hippocampal IL-1 beta and memory consolidation impairments in aging rats following peripheral infection. Brain Behav. Immun. 23, 46–54. doi: 10.1016/j.bbi.2008.07.002
Barrientos, R. M., Sprunger, D. B., Campeau, S., Watkins, L. R., Rudy, J. W., and Maier, S. F. (2004). BDNF mRNA expression in rat hippocampus following contextual learning is blocked by intrahippocampal IL-1 beta administration. J. Neuroimmunol. 155, 119–126. doi: 10.1016/j.jneuroim.2004.06.009
Barth, M. C., Ahluwalia, N., Anderson, T. J. T., Hardy, G. J., Sinha, S., Alvarez-Cardona, J. A., et al. (2009). Kynurenic Acid triggers firm arrest of leukocytes to vascular endothelium under flow conditions. J. Biol. Chem. 284, 19189–19195. doi: 10.1074/jbc.M109.024042
Bassett, B., Subramaniyam, S., Fan, Y., Varney, S., Pan, H., Carneiro, A. M. D., et al. (2021). Minocycline alleviates depression-like symptoms by rescuing decrease in neurogenesis in dorsal hippocampus via blocking microglia activation/phagocytosis. Brain Behav. Immun. 91, 519–530. doi: 10.1016/j.bbi.2020.11.009
Bauer, S., Kerr, B. J., and Patterson, P. H. (2007). The neuropoietic cytokine family in development, plasticity, disease and injury. Nat. Rev. Neurosci. 8, 221–232. doi: 10.1038/nrn2054
Baumgartner, R., Forteza, M. J., and Ketelhuth, D. F. J. (2019). The interplay between cytokines and the kynurenine pathway in inflammation and atherosclerosis. Cytokine 122:154148. doi: 10.1016/j.cyto.2017.09.004
Beattie, E. C., Stellwagen, D., Morishita, W., Bresnahan, J. C., Ha, B. K., Von Zastrow, M., et al. (2002). Control of synaptic strength by glial TNF-α. Science 295, 2282–2285. doi: 10.1126/science.1067859
Becher, B., Bechmann, I., and Greter, M. (2006). Antigen presentation in autoimmunity and CNS inflammation: How T lymphocytes recognize the brain. J. Mol. Med. 84, 532–543. doi: 10.1007/s00109-006-0065-1
Belladonna, M. L., Grohmann, U., Guidetti, P., Volpi, C., Bianchi, R., Fioretti, M. C., et al. (2006). Kynurenine pathway enzymes in dendritic cells initiate tolerogenesis in the absence of functional IDO. J. Immunol. 177, 130–137. doi: 10.4049/jimmunol.177.1.130
Belladonna, M. L., Orabona, C., Grohmann, U., and Puccetti, P. (2009). TGF-β and kynurenines as the key to infectious tolerance. Trends Mol. Med. 15, 41–49. doi: 10.1016/j.molmed.2008.11.006
Belladonna, M. L., Volpi, C., Bianchi, R., Vacca, C., Orabona, C., Pallotta, M. T., et al. (2008). Cutting edge: Autocrine TGF-beta sustains default tolerogenesis by IDO-competent dendritic cells. J. Immunol. 181, 5194–5198. doi: 10.4049/jimmunol.181.8.5194
Ben-Shaanan, T. L., Azulay-Debby, H., Dubovik, T., Starosvetsky, E., Korin, B., Schiller, M., et al. (2016). Activation of the reward system boosts innate and adaptive immunity. Nat. Med. 22, 940–944. doi: 10.1038/nm.4133
Berding, K., Vlckova, K., Marx, W., Schellekens, H., Stanton, C., Clarke, G., et al. (2021). Diet and the microbiota-gut-brain axis: Sowing the seeds of good mental health. Adv. Nutr. 12, 1239–1285. doi: 10.1093/advances/nmaa181
Berlinguer-Palmini, R., Masi, A., Narducci, R., Cavone, L., Maratea, D., Cozzi, A., et al. (2013). GPR35 Activation reduces Ca2+ transients and contributes to the kynurenic acid-dependent reduction of synaptic activity at CA3-CA1 synapses. PLoS One 8:e82180. doi: 10.1371/journal.pone.0082180
Bessede, A., Gargaro, M., Pallotta, M. T., Matino, D., Servillo, G., Brunacci, C., et al. (2014). Aryl hydrocarbon receptor control of a disease tolerance defence pathway. Nature 511, 184–189. doi: 10.1038/nature13323
Besser, M. J., Ganor, Y., and Levite, M. (2005). Dopamine by itself activates either D2, D3 or D1/D5 dopaminergic receptors in normal human T-cells and triggers the selective secretion of either IL-10, TNF-α or both. J. Neuroimmunol. 169, 161–171. doi: 10.1016/j.jneuroim.2005.07.013
Bessis, A., Bechade, C., Bernard, D., and Roumier, A. (2007). Microglial control of neuronal death and synaptic properties. Glia 55, 233–238. doi: 10.1002/glia.20459
Bhandage, A. K., Jin, Z., Hellgren, C., Korol, S. V., Nowak, K., Williamsson, L., et al. (2017). AMPA, NMDA and kainate glutamate receptor subunits are expressed in human peripheral blood mononuclear cells (PBMCs) where the expression of GluK4 is altered by pregnancy and GluN2D by depression in pregnant women. J. Neuroimmunol. 305, 51–58. doi: 10.1016/j.jneuroim.2017.01.013
Bhat, A., Tan, V., Heng, B., Lovejoy, D. B., Sakharkar, M. K., Essa, M. M., et al. (2020). Roflumilast, a cAMP-specific phosphodiesterase-4 inhibitor, reduces oxidative stress and improves synapse functions in human cortical neurons exposed to the excitotoxin quinolinic acid. ACS Chem. Neurosci. 11, 4405–4415. doi: 10.1021/acschemneuro.0c00636
Bhaumik, S., and Basu, R. (2017). Cellular and molecular dynamics of th17 differentiation and its developmental plasticity in the intestinal immune response. Front. Immunol. 8:254. doi: 10.3389/fimmu.2017.00254
Biber, K., Neumann, H., Inoue, K., and Boddeke, H. W. (2007). Neuronal ‘On’ and ‘Off’ signals control microglia. Trends Neurosci. 30, 596–602. doi: 10.1016/j.tins.2007.08.007
Birch, P. J., Grossman, C. J., and Hayes, A. G. (1988). Kynurenic acid antagonises responses to NMDA via an action at the strychnine-insensitive glycine receptor. Eur. J. Pharmacol. 154, 85–87. doi: 10.1016/0014-2999(88)90367-6
Birnbaum, R., and Weinberger, D. R. (2020). A genetics perspective on the role of the (neuro)immune system in schizophrenia. Schizophr. Res. 217, 105–113. doi: 10.1016/j.schres.2019.02.005
Birnbaum, R., Jaffe, A. E., Chen, Q., Shin, J. H., Kleinman, J. E., Hyde, T. M., et al. (2018). Investigating the neuroimmunogenic architecture of schizophrenia. Mol. Psychiatr. 18, 1251–1260. doi: 10.1038/mp.2017.89
Bjurstom, H., Wang, J., Ericsson, I., Bengtsson, M., Liu, Y., Kumar-Mendu, S., et al. (2008). GABA, a natural immunomodulator of T lymphocytes. J. Neuroimmunol. 205, 44–50. doi: 10.1016/j.jneuroim.2008.08.017
Bock, F., Rossner, S., Onderka, J., Lechmann, M., Pallotta, M. T., Fallarino, F., et al. (2013). Topical application of soluble CD83 induces IDO-mediated immune modulation, increases Foxp3(+) T cells, and prolongs allogeneic corneal graft survival. J. Immunol. 191, 1965–1975. doi: 10.4049/jimmunol.1201531
Boldyrev, A. A., Bryushkova, E. A., and Vladychenskaya, E. A. (2012). NMDA receptors in immune competent cells. Biochemistry (Moscow) 77, 128–134. doi: 10.1016/j.bbrc.2004.09.019
Boldyrev, A. A., Kazey, V. I., Leinsoo, T. A., Mashkina, A. P., Tyulina, O. V., Johnson, P., et al. (2004). Rodent lymphocytes express functionally active glutamate receptors. Biochem. Biophys. Res. Commun. 324, 133–139.
Boros, F. A., Bohar, Z., and Vecsei, L. (2018). Genetic alterations affecting the genes encoding the enzymes of the kynurenine pathway and their association with human diseases. Mutat. Res. 776, 32–45. doi: 10.1016/j.mrrev.2018.03.001
Bosco, M. C., Puppo, M., Pastorino, S., Mi, Z. H., Melillo, G., Massazza, S., et al. (2004). Hypoxia selectively inhibits monocyte chemoattractant protein-1 production by macrophages. J. Immunol. 172, 1681–1690. doi: 10.4049/jimmunol.172.3.1681
Bosco, M. C., Rapisarda, A., Massazza, S., Melillo, G., Young, H., and Varesio, L. (2000). The tryptophan catabolite picolinic acid selectively induces the chemokines macrophage inflammatory protein-1 alpha and-1 beta in macrophages. J. Immunol. 164, 3283–3291. doi: 10.4049/jimmunol.164.6.3283
Bossu, P., Ciaramella, A., Salani, F., Vanni, D., Palladino, I., Caltagirone, C., et al. (2010). Interleukin-18, from neuroinflammation to Alzheimer’s disease. Curr. Pharmaceut. Des. 16, 4213–4224. doi: 10.2174/138161210794519147
Broadwell, R. D., and Sofroniew, M. V. (1993). Serum proteins by-pass the blood-brain barrier for extraeellular entry to the central nervous system. Exp. Neurol. 120, 245–263.
Bromander, S., Anckarsater, R., Kristiansson, M., Blennow, K., Zetterberg, H., and Anckarsater, H. (2012). Changes in serum and cerebrospinal fluid cytokines in response to non-neurological surgery: An observational study. J. Neuroinflamm. 9:242. doi: 10.1186/1742-2094-9-242
Brooker, G., and Mocchetti, I. (2001). Interleukin-10 prevents glutamate-mediated cerebellar granule cell death by blocking caspase-3-like activity. J. Neurosci. 21, 3104–3112. doi: 10.1523/JNEUROSCI.21-09-03104.2001
Brooks, P. A., Smith, D. A. S., Stone, T. W., and Kelly, J. S. (1986). Postsynaptic action of kynurenic acid in the rat dentate gyrus. Neurosci. Lett. 66, 96–100.
Brown, J. A., Sherrod, S. D., Goodwin, C. R., Brewer, B., Yang, L., Garbett, K. A., et al. (2014). Metabolic consequences of interleukin-6 challenge in developing neurons and astroglia. J. Neuroinflamm. 11:183. doi: 10.1186/s12974-014-0183-6
Brundin, L., Bryleva, E. Y., and Rajamani, K. T. (2017). Role of inflammation in suicide: From mechanisms to treatment. Neuropsychopharmacology 42, 271–283. doi: 10.1038/npp.2016.116
Brundin, L., Sellgren, C. M., Lim, C. K., Grit, J., Palsson, E., Landen, M., et al. (2016). An enzyme in the kynurenine pathway that governs vulnerability to suicidal behavior by regulating excitotoxicity and neuroinflammation. Transl. Psychiatr. 6:e865. doi: 10.1038/tp.2016.133
Bukoreshtliev, N. V., Wang, X., Hodneland, E., Gurke, S., Barroso, J. F., and Gerdes, H. H. (2009). Selective block of tunneling nanotube (TNT) formation inhibits intercellular organelle transfer between PC12 cells. FEBS Lett. 583, 1481–1488. doi: 10.1016/j.febslet.2009.03.065
Burger, J. A., and Buerkle, A. (2007). The CXCR4 chemokine receptor in acute and chronic leukaemia: A marrow homing receptor and potential therapeutic target. Br. J. Haematol. 137, 288–296. doi: 10.1111/j.1365-2141.2007.06590.x
Burmeister, A. R., and Marriott, I. (2018). The Interleukin-10 family of cytokines and their role in the CNS. Front. Cell. Neurosci. 12:458. doi: 10.3389/fncel.2018.00458
Burton, M. D., Sparkman, N. L., and Johnson, R. W. (2011). Inhibition of interleukin-6 trans-signaling in the brain facilitates recovery from lipopolysaccharide-induced sickness behavior. J. Neuroinflamm. 8:54. doi: 10.1186/1742-2094-8-54
Camussi, G., Deregibus, M. C., Bruno, S., Grange, C., Fonsato, V., and Tetta, C. (2011). Exosome/microvesicle-mediated epigenetic reprogramming of cells. Am. J. Cancer Res. 1, 98–110.
Capellino, S. (2020). Dopaminergic agents in rheumatoid arthritis. J. Neuroimmunol. Pharmacol. 15, 48–56. doi: 10.1007/s11481-019-09850-5
Capuron, L., and Miller, A. H. (2011). Immune system to brain signaling: Neuropsychopharmacological implications. Pharmacol. Ther. 130, 226–238. doi: 10.1016/j.pharmthera.2011.01.014
Cardona, A. E., Pioro, E. P., Sasse, M. E., Kostenko, V., Cardona, S. M., Dijkstra, I. M., et al. (2006). Control of microglial neurotoxicity by the fractalkine receptor. Nat. Neurosci. 9, 917–924. doi: 10.1038/nn1715
Cayrol, C., and Girard, J. P. (2022). Interleukin-33 (IL-33): A critical review of its biology and the mechanisms involved in its release as a potent extracellular cytokine. Cytokine 156:155891. doi: 10.1016/j.cyto.2022.155891
Chatzipanteli, K., Vitarbo, E., Alonso, O. F., Bramlett, H. M., and Dietrich, W. D. (2012). Temporal profile of cerebrospinal fluid, plasma, and brain interleukin-6 after normothermic fluid-percussion brain injury: Effect of secondary hypoxia. Ther. Hypothermia Temp. Manag. 2, 167–175. doi: 10.1089/ther.2012.0016
Chen, H., Tang, X. Q., Li, J., Hu, B. Y., Yang, W. Q., Zhan, M., et al. (2022). IL-17 crosses the blood-brain barrier to trigger neuroinflammation: A novel mechanism in nitroglycerin-induced chronic migraine. J. Headache Pain 23:1. doi: 10.1186/s10194-021-01374-9
Chen, P., and Tang, X. Q. (2021). Gut microbiota as regulators of Th17/Treg balance in patients with myasthenia gravis. Front. Immunol. 12:803101. doi: 10.3389/fimmu.2021.803101
Cheng, H. Y., Guan, X., Chen, D. K., and Ma, W. T. (2019). The Th17/Treg cell balance: A gut microbiota-modulated story. Microorganisms 7:583. doi: 10.3390/microorganisms7120583
Chiarugi, A., Dello Sbarba, P., Paccagnini, A., Donnini, S., Filippi, S., and Moroni, F. (2000). Combined inhibition of indoleamine 2,3-dioxygenase and nitric oxide synthase modulates neurotoxin release by interferon-gamma-activated macrophages. J. Leukocyte Biol. 68, 260–266.
Chiarugi, A., Meli, E., and Moroni, F. (2001). Similarities and differences in the neuronal death processes activated by 3OH-kynurenine and quinolinic acid. J. Neurochem. 77, 1310–1318. doi: 10.1046/j.1471-4159.2001.00335.x
Choi, G. B., Yim, Y. W., Wong, H. L., Kim, S., Kim, H., Kim, S. V., et al. (2016). The maternal interleukin-17a pathway in mice promotes autism-like phenotypes in offspring. Science 351, 933–939. doi: 10.1126/science.aad0314
Christensen, P. C., Samadi-Bahrami, Z., Pavlov, V., Stys, P. K., and Moore, G. R. W. (2016a). Ionotropic glutamate receptor expression in human white matter. Neurosci. Lett. 630, 1–8. doi: 10.1016/j.neulet.2016.07.030
Christensen, P. C., Welch, N. C., Brideau, C., and Stys, P. K. (2016b). Functional ionotropic glutamate receptors on peripheral axons and myelin. Muscle Nerve 54, 451–459. doi: 10.1002/mus.25078
Ciapala, K., Mika, J., and Rojewska, E. (2021). The kynurenine pathway as a potential target for neuropathic pain therapy design: From basic research to clinical perspectives. Int. J. Mol. Sci. 22:11055. doi: 10.3390/ijms222011055
Cingolani, L. A., Thalhammer, A., Yu, L. M. Y., Catalano, M., Ramos, T., Colicos, M. A., et al. (2008). Activity-dependent regulation of synaptic AMPA receptor composition and abundance by beta 3 integrins. Neuron 58, 749–762. doi: 10.1016/j.neuron.2008.04.011
Clanchy, F. I. L., Huang, Y.-S., Ogbechi, J., Darlington, L. G., Williams, R. O., and Stone, T. W. (2022). Induction of IDO1 and kynurenine by serine proteases subtilisin, prostate specific antigen, CD26/DPP4 and HtrA: A new mode of immunosuppression? Front. Immunol. 13:832989. doi: 10.3389/fimmu.2022.832989
Clark, I. A., and Vissel, B. (2016). Excess cerebral TNF causing glutamate excitotoxicity rationalizes treatment of neurodegenerative diseases and neurogenic pain by anti-TNF agents. J. Neuroinflamm. 13:236. doi: 10.1186/s12974-016-0708-2
Clausen, F., Hanell, A., Israelsson, C., Hedin, J., Ebendal, T., Mir, A. K., et al. (2011). Neutralization of interleukin-1 beta reduces cerebral edema and tissue loss and improves late cognitive outcome following traumatic brain injury in mice. Eur. J. Neurosci. 34, 110–123. doi: 10.1111/j.1460-9568.2011.07723.x
Coley, J. S., Calderon, T. M., Gaskill, P. J., Eugenin, E. A., and Berman, J. W. (2015). Dopamine Increases CD14(+)CD16(+) monocyte migration and adhesion in the context of substance abuse and HIV neuropathogenesis. PLoS One 10:ARe0117450. doi: 10.1371/journal.pone.0117450
Comai, S., Melloni, E., Lorenzi, C., Bollettini, I., Vai, B., Zanardi, R., et al. (2022). Selective association of cytokine levels and kynurenine/tryptophan ratio with alterations in white matter microstructure in bipolar but not in unipolar depression. Eur. Neuropsychopharmacol. 55, 96–109. doi: 10.1016/j.euroneuro.2021.11.003
Connick, J. H., and Stone, T. W. (1985). The role of kynurenines in diabetes mellitus. Med. Hypotheses 18, 371–376.
Cosentino, M., Fietta, A. M., Ferrari, M., Rasini, E., Bombelli, R., Carcano, E., et al. (2007). Human CD4(+) CD25(+) regulatory T cells selectively express tyrosine hydroxylase and contain endogenous catecholamines subserving an autocrine/paracrine inhibitory functional loop. Blood 109, 632–642. doi: 10.1182/blood-2006-01-028423
Cresto, N., Pillet, L. E., Billuart, P., and Rouach, N. (2019). Do astrocytes play a role in intellectual disabilities? Trends Neurosci. 42, 518–527. doi: 10.1016/j.tins.2019.05.011
Croitoru-Lamoury, J., Guillemin, G. J., Boussin, F. D., Mognetti, B., Gigout, L. I., Cheret, A., et al. (2003). Expression of chemokines and their receptors in human and simian astrocytes: Evidence for a central role of TNF-α and IFN-γ in CXCR4 and CCR5 modulation. Glia 41, 354–370. doi: 10.1002/glia.10181
Crowe, A., and Morgan, E. H. (1992). Iron and transferrin uptake by brain and cerebrospinal-fluid in the rat. Brain Res. 592, 8–16. doi: 10.1016/0006-8993(92)91652-U
Cryan, J. F., O’Riordan, K. J., Cowan, C. S. M., Sandhu, K. V., Bastiaanssen, T. F. S., Boehme, M., et al. (2019). The microbiota-gut-brain axis. Physiol. Rev. 99, 1877–2013. doi: 10.1152/physrev.00018.2018
Csontos, C., Foldi, V., Palinkas, L., Bogar, L., Roth, E., Weber, G., et al. (2010). Time course of pro- and anti-inflammatory cytokine levels in patients with burns–prognostic value of interleukin-10. Burns 36, 483–494. doi: 10.1016/j.burns.2009.10.0009
Dahl, J., Andreassen, O. A., Verkerk, R., Malt, U. F., Sandvik, L., Brundin, L., et al. (2015). Ongoing episode of major depressive disorder is not associated with elevated plasma levels of kynurenine pathway markers. Psychoneuroendocrinology 56, 12–22. doi: 10.1016/j.psyneuen.2015.02.011
Dai, X., and Zhu, B. T. (2010). Indoleamine 2,3-dioxygenase tissue distribution and cellular localization in mice: Implications for its biological functions. J. Histochem. Cytochem. 58, 17–28. doi: 10.1369/jhc.2009.953604
Dantzer, R. (2006). Cytokine, sickness behavior, and depression. Neurol. Clin. 24, 441–460. doi: 10.1016/j.ncl.2006.03.003
Dantzer, R. (2018). Neuroimmune interactions: From the brain to the immune system and vice versa. Physiol. Rev. 98, 477–504. doi: 10.1152/physrev.00039.2016
Dantzer, R., O’Connor, J. C., Freund, G. G., Johnson, R. W., and Kelley, K. W. (2008). From inflammation to sickness and depression: When the immune system subjugates the brain. Nat. Rev. Neurosci. 9, 46–57. doi: 10.1038/nrn2297
Danysz, W., Fadda, E., Wroblewski, J. T., and Costa, E. (1989). Kynurenate and 2-amino-5-phosphonovalerate interact with multiple bmding sites of the N-methyl-D-aspartate-sensitive glutamate receptor domain. Neurosci. Lett. 96, 340–344. doi: 10.1016/0304-3940(89)90402-3
Darlington, L. G., Forrest, C. M., Mackay, G. M., Stoy, N., Smith, R. A., Smith, A. J., et al. (2010). On the biological significance of the 3-hydroxyanthranilic acid: Anthranilic acid ratio. Int. J. Tryptophan Res. 3, 51–59. doi: 10.4137/IJTR.S4282
Darlington, L. G., Mackay, G. M., Forrest, C. M., Stoy, N., George, C., and Stone, T. W. (2007). Altered kynurenine metabolism correlates with infarct volume in stroke. Eur. J. Neurosci. 26, 2211–2221. doi: 10.1111/j.1460-9568.2007.05838.x
Davidson, R. J., and McEwen, B. S. (2012). Social influences on neuroplasticity: Stress and interventions to promote well-being. Nat. Neurosci. 15, 689–695. doi: 10.1038/nn.3093
de Haas, A. H., Boddeke, H. W. G. M., and Biber, K. (2008). Region-specific expression of immunoregulatory proteins on microglia in the healthy CNS. Glia 56, 888–894. doi: 10.1002/glia.20663
de Kloet, E. R., Joels, M., and Holsboer, F. (2005). Stress and the brain: From adaptation to disease. Nat. Rev. Neurosci. 6, 463–475. doi: 10.1038/nrn1683
Debnath, M., and Berk, M. (2017). Functional implications of the IL-23/IL-17 immune axis in schizophrenia. Mol. Neurobiol. 54, 8170–8178. doi: 10.1007/s12035-016-0309-1
Del Rivero, T., Fischer, R., Yang, F., Swanson, K. A., and Bethea, J. R. (2019). Tumor necrosis factor receptor 1 inhibition is therapeutic for neuropathic pain in males but not in females. Pain 160, 922–931. doi: 10.1097/j.pain.0000000000001470
Deverman, B. E., and Patterson, P. H. (2009). Cytokines and CNS development. Neuron 64, 61–78. doi: 10.1016/j.neuron.2009.09.002
Diaz-Aparicio, I., Paris, I., Sierra-Torre, V., Plaza-Zabala, A., Rodriguez-Iglesias, N., Marquez-Ropero, M., et al. (2020). Microglia actively remodel adult hippocampal neurogenesis through the phagocytosis secretome. J. Neurosci. 40, 1453–1482. doi: 10.1523/JNEUROSCI.0993-19.2019
DiNatale, B. C., Murray, I. A., Schroeder, J. C., Flaveny, C. A., Lahoti, T. S., Laurenzana, E. M., et al. (2010). Kynurenic acid is a potent endogenous aryl hydrocarbon receptor ligand that synergistically induces interleukin-6 in the presence of inflammatory signaling. Toxicol. Sci. 115, 89–97. doi: 10.1093/toxsci/kfq024
D’Mello, C. H., Le, T., and Swain, M. G. (2009). Cerebral microglia recruit monocytes into the brain in response to tumor necrosis factor alpha signaling during peripheral organ inflammation. J. Neurosci. 29, 2089–2102. doi: 10.1523/JNEUROSCI.3567-08.2009
Dounay, A. B., Anderson, M., Bechle, B. M., Evrard, E., Gan, X., Kim, J. Y., et al. (2013). PF-04859989 as a template for structure-based drug design: Identification of new pyrazole series of irreversible KAT II inhibitors with improved lipophilic efficiency. Bioorg. Med. Chem. Lett. 23, 1961–1966. doi: 10.1016/j.bmcl.2013.02.039
Dounay, A. B., Tuttle, J. B., and Verhoestt, P. R. (2015). Challenges and opportunities in the discovery of new therapeutics targeting the kynurenine pathway. J. Med. Chem. 58, 8762–8782. doi: 10.1021/acs.jmedchem.5b00461
Du Preez, A., Onorato, D., Eiben, I., Musaelyan, K., Egeland, M., Zunszain, P. A., et al. (2021). Chronic stress followed by social isolation promotes depressive-like behavior, alters microglial and astrocyte biology and reduces hippocampal neurogenesis in male mice. Brain Behav. Immun. 91, 24–47. doi: 10.1016/j.bbi.2020.07.015
Dubois, C. J., and Liu, S. J. (2021). GluN2D NMDA receptors gate fear extinction learning and interneuron plasticity. Front. Synaptic Neurosci. 13:681068. doi: 10.3389/fnsyn.2021.681068
Duque, G., Vidal, C., Li, W., Al Saedi, A., Khalil, M., Lim, C. K., et al. (2020). Picolinic acid, a catabolite of tryptophan, has an anabolic effect on bone in vivo. J. Bone Min. Res. 35, 2275–2288. doi: 10.1002/jbmr.4125
Ekdahl, C. T., Kokaia, Z., and Lindvall, O. (2009). Brain inflammation and adult neurogenesis: The dual role of microglia. Neuroscience 158, 1021–1029. doi: 10.1016/j.neuroscience.2008.06.052
Eleftheriadis, T., Pissas, G., Antoniadi, G., Tsogka, K., Sounidaki, M., Liakopoulos, V., et al. (2016). Indoleamine 2,3-dioxygenase downregulates T-cell receptor complex zeta-chain and c-Myc, and reduces proliferation, lactate dehydrogenase levels and mitochondrial glutaminase in human T-cells. Mol. Med. Rep. 13, 925–932. doi: 10.3892/mmr.2015.4595
Elizei, S. S., Poormasjedi-Meibod, M. S., Li, Y. Y., Jalili, R. B., and Ghahary, A. (2015). Effects of kynurenine on CD3+ and macrophages in wound healing. Wound Repair Regen. 23, 90–97. doi: 10.1111/wrr.12252
Elizei, S. S., Poormasjedi-Meibod, M. S., Wang, X., Kheirandish, M., and Ghahary, A. (2017). Kynurenic acid downregulates IL-17/1L-23 axis in vitro. Mol.Cell. Biochem. 431, 55–65. doi: 10.1007/s11010-017-2975-3
Ellison, V. J., Mocatta, T. J., Winterbourn, C. C., Darlow, B. A., Volpe, J. J., and Inder, T. E. (2005). The relationship of CSF and plasma cytokine levels to cerebral white matter injury in the premature newborn. Pediatr. Res. 57, 282–286. doi: 10.1203/01.PDR.0000148286.53572.95
El-Zaatari, M., Chang, Y. M., Zhang, M., Franz, M., Shreiner, A., McDermott, A. J., et al. (2014). Tryptophan catabolism restricts IFN-γ-expressing neutrophils and Clostridium difficile immunopathology. J. Immunol. 193, 807–816. doi: 10.4049/jimmunol.1302913
Enache, D., Pariante, C. M., and Mondelli, V. (2019). Markers of central inflammation in major depressive disorder: A systematic review and meta-analysis of studies examining cerebrospinal fluid, positron emission tomography and post-mortem brain tissue. Brain Behav. Immun. 81, 24–40. doi: 10.1016/j.bbi.2019.06.015
Erhardt, S., Schwieler, L., Imbeault, S., and Engberg, G. (2017). The kynurenine pathway in schizophrenia and bipolar disorder. Neuropharmacology 112, 297–306. doi: 10.1016/j.neuropharm.2016.05.020
Erta, M., Quintana, A., and Hidalgo, J. (2012). Interleukin-6, a major cytokine in the central nervous system. Int. J. Biol. Sci. 8, 1254–1266. doi: 10.7150/ijbs.4679
Evavold, C. L., and Kagan, J. C. (2022). Diverse control mechanisms of the interleukin-1 cytokine family. Front. Cell Dev. Biol. 10:910983. doi: 10.3389/fcell.2022.910983
Fabry, Z., Schreiber, H. A., Harris, M. G., and Sandor, M. (2008). Sensing the microenvironment of the central nervous system: Immune cells in the central nervous system and their pharmacological manipulation. Curr. Opin. Pharmacol. 8, 496–507. doi: 10.1016/j.coph.2008.07.009
Fairlie-Clarke, K., Barbour, M., Wilson, C., Hridi, S. U., Allan, D., and Jiang, H. R. (2018). Expression and function of IL-33/ST2 axis in the central nervous system under normal and diseased conditions. Front. Immunol. 9:2596. doi: 10.3389/fimmu.2018.02596
Fallarini, S., Magliulo, L., Paoletti, T., de Lalla, C., and Lombardi, G. (2010). Expression of functional GPR35 in human iNKT cells. Biochem. Biophys. Res. Commun. 398, 420–425. doi: 10.1016/j.bbrc.2010.06.091
Fallarino, F., Grohmann, U., You, S., McGrath, B. C., Cavener, D. R., Vacca, C., et al. (2006). The combined effects of tryptophan starvation and tryptophan catabolites down-regulate T cell receptor zeta-chain and induce a regulatory phenotype in naive T cells. J. Immunol. 176, 6752–6761. doi: 10.4049/jimmunol.176.11.6752
Fallarino, I., Grohmann, U., Vacca, C., Bianchi, R., Orabona, C., Spreca, A., et al. (2002). T cell apoptosis by tryptophan catabolism. Cell Death Differ. 9, 1069–1077. doi: 10.1038/sj.cdd.4401073
Fan, Y., Chen, Z. L., Pathak, J. L., Carneiro, A. M. D., and Chung, C. Y. (2018). Differential regulation of adhesion and phagocytosis of resting and activated microglia by dopamine. Front. Cell. Neurosci. 12:309. doi: 10.3389/fncel.2018.00309
Farrar, W. L., Kilian, P. L., Ruff, M. R., Hill, J. M., and Pert, C. B. (1987). Visualization and characterization of interleukin-1 receptors in brain. J. Immunol. 139, 459–463.
Fatokun, A. A., Hunt, N. H., and Ball, H. J. (2013). Indoleamine 2,3-dioxygenase 2 (IDO2) and the kynurenine pathway: Characteristics and potential roles in health and disease. Amino Acids 45, 1319–1329. doi: 10.1007/s00726-013-1602-1
Fatouros, I. G., and Jamurtas, A. (2016). Insights into the molecular etiology of exercise-induced inflammation: Opportunities for optimizing performance. J. Inflamm. Res. 9, 175–186. doi: 10.2147/JIR.S114635
Favre, D., Mold, J., Hunt, P. W., Kanwar, B., Loke, P., Seu, L., et al. (2010). Tryptophan catabolism by indoleamine 2,3-dioxygenase 1 alters the balance of T(H)17 to regulatory t cells in HIV disease. Sci. Transl. Med. 2:32ra36. doi: 10.1126/scitranslmed.3000632
Fazio, F., Lionetto, L., Curto, M., Iacovelli, L., Copeland, C. S., Neale, S. A., et al. (2017). Cinnabarinic acid and xanthurenic acid: Two kynurenine metabolites that interact with metabotropic glutamate receptors. Neuropharmacology 112, 365–372. doi: 10.1016/j.neuropharm.2016.06.020
Felderhoff-Mueser, U., Schmidt, O. I., Oberholzer, A., Buhrer, C., and Stahel, P. F. (2005). IL-18: A key player in neuroinflammation and neurodegeneration? Trends Neurosci. 28, 487–493. doi: 10.1016/j.tins.2005.06.008
Felger, J. C., and Lotrich, F. E. (2013). Inflammatory cytokines in depression: Neurobiological mechanisms and therapeutic implications. Neuroscience 246, 199–229. doi: 10.1016/j.neuroscience.2013.04.060
Felger, N., and Miller, A. H. (2012). Cytokine effects on the basal ganglia and dopamine function: The subcortical source of inflammatory malaise. Front. Neuroendocrinol. 33:315–327. doi: 10.1016/j.yfrne.2012.09.003
Finnerty, C. C., Herndon, D. N., Przkora, R., Pereira, C. C., Oliveira, H. M., Querioz, D. M. M., et al. (2006). Cytokine expression profile over time in severely burned pediatric patients. Shock 26, 13–19. doi: 10.1097/01.shk.0000223120.26394.7d
Fleshner, M., Goehler, L. E., Hermann, J., Relton, J. K., Maier, S. F., and Watkins, L. R. (1995). Interleukin-1β induced corticosterone elevation and hypothalamic NE depletion is vagally mediated. Brain Res. Bull. 37, 605–610. doi: 10.1016/0361-9230(95)00051-F
Flood, L., Korol, S. V., Ekselius, L., Birnir, B., and Jin, Z. (2019). Interferon-gamma potentiates GABA(A) receptor-mediated inhibitory currents in rat hippocampal CA1 pyramidal neurons. J. Immunol. 337:577050. doi: 10.1016/j.jneuroim.2019.577050
Forrest, C. M., Khalil, O. S., Pisar, M., Darlington, L. G., and Stone, T. W. (2013a). Prenatal inhibition of the tryptophan–kynurenine pathway alters synaptic plasticity and protein expression in the rat hippocampus. Brain Res. 1504, 1–15. doi: 10.1016/j.brainres.2013.01.031
Forrest, C. M., Khalil, O. S., Pisar, M., McNair, K., Kornisiuk, E., Snitcofsky, M., et al. (2013b). Changes in synaptic transmission and protein expression in the brains of adult offspring after prenatal inhibition of the kynurenine pathway. Neuroscience 254, 241–259. doi: 10.1016/j.neuroscience.2013.09.034
Forrest, C. M., Mackay, G. M., Oxford, L., Stoy, N., Stone, T. W., and Darlington, L. G. (2006). Kynurenine pathway metabolism in patients with osteoporosis after 2 years of drug treatment. Clin. Exp. Pharmacol. Physiol. 33, 1078–1087. doi: 10.1111/j.1440-1681.2006.04490
Forrest, C. M., Mackay, G. M., Stoy, N., Spiden, S. L., Taylor, R., Stone, T. W., et al. (2010). Blood levels of kynurenines, interleukin IL-23 and sHLA-G at different stages of Huntington’s disease. J. Neurochem. 112, 112–122. doi: 10.1111/j.1471-4159.2009.06442.x
Forrest, C. M., McNair, K., Pisar, M., Khalil, O. S., Darlington, L. G., and Stone, T. W. (2015). Altered hippocampal plasticity by prenatal kynurenine administration, kynurenine-3-monoxygenase (KMO) deletion or galantamine. Neuroscience 310, 91–105. doi: 10.1016/j.neuroscience.2015.09.022
Fujigaki, S., Saito, K., Takemura, M., Fujii, H., Wada, H., Noma, A., et al. (1998). Species differences in L-tryptophan-kynurenine pathway metabolism: Quantification of anthranilic acid and its related enzymes. Arch. Biochem. Biophys. 358, 329–335. doi: 10.1006/abbi.1998.0861
Fuxe, K., and Borroto-Escuela, D. O. (2016). Volume transmission and receptor-receptor interactions in heteroreceptor complexes: Understanding the role of new concepts for brain communication. Neural Regen. Res. 11, 1220–1223. doi: 10.4103/1673-5374.189168
Fuxe, K., Dahlstrom, A., Hoistad, M., Marcellino, D., Jansson, A., Rivera, A., et al. (2007). From the Golgi-Cajal mapping to the transmitter-based characterization of the neuronal networks leading to two modes of brain communication: Wiring and volume transmission. Brain Res. Rev. 55, 17–54. doi: 10.1016/j.brainresrev.2007.02.009
Gadad, B. S., Vargas-Medrano, J., Ramos, E. I., Najera, K., Fagan, M., Forero, A., et al. (2021). Altered levels of interleukins and neurotrophic growth factors in mood disorders and suicidality: An analysis from periphery to central nervous system. Transl. Psychiatr. 11:341. doi: 10.1038/s41398-021-01452-1
Gajtko, A., Bakk, E., Hegedus, K., Ducza, L., and Hollo, K. (2020). IL-1 beta induced cytokine expression by spinal astrocytes can play a role in the maintenance of chronic inflammatory pain. Front. Physiol. 11:543331. doi: 10.3389/fphys.2020.543331
Galic, M. A., Riazi, K., and Pittman, Q. J. (2012). Cytokines and brain excitability. Front. Neuroendocrinol. 33:116–125. doi: 10.1016/j.yfrne.2011.12.002
Ganong, A. H., and Cotman, C. W. (1986). Kynurenic acid and quinolinic acid act at NMDA receptors in the rat hippocampus. J. Pharmacol. Exp. Ther. 236, 293–299.
Ganong, A. H., Lanthorn, T. H., and Cotman, C. W. (1983). Kynurenic acid inhibits synaptic and acidic amino acid-induced responses in the rat hippocampus and spinal-cord. Brain Res. 273, 170–174. doi: 10.1016/0006-8993(83)91108-3
Garay, P. A., and McAllister, A. K. (2010). Novel roles for immune molecules in neural development: Implications for neurodevelopmental disorders. Front. Syn. Neurosci. 2:136. doi: 10.3389/fnsyn.2010.00136
Garay, P. A., Hsiao, E. Y., Patterson, P. H., and McAllister, A. K. (2013). Maternal immune activation causes age- and region-specific changes in brain cytokines in offspring throughout development. Brain Behav. Immun. 31, 54–68. doi: 10.1016/j.bbi.2012.07.008
Gardoni, F., Boraso, M., Zianni, E., Corsini, E., Galli, C. L., Cattabeni, F., et al. (2011). Distribution of interleukin-1 receptor complex at the synaptic membrane driven by interleukin-1 beta and NMDA stimulation. J. Neuroinflamm. 8:14. doi: 10.1186/1742-2094-8-14
Gargaro, M., Manni, G., Scalisi, G., Puccetti, P., and Fallarino, F. (2021). Tryptophan metabolites at the crossroad of immune-cell interaction via the aryl hydrocarbon receptor: Implications for tumor immunotherapy. Int. J. Mol. Sci. 22:AR4644. doi: 10.3390/ijms22094644
Gargaro, M., Vacca, C., Massari, S., Scalisi, G., Manni, G., Mondanelli, G., et al. (2019). Engagement of nuclear coactivator 7 by 3-hydroxyanthranilic acid enhances activation of aryl hydrocarbon receptor in immunoregulatory dendritic cells. Front. Immunol. 10:1973. doi: 10.3389/fimmu.2019.01973
Garrison, A. M., Parrott, J. M., Tunon, A., Delgado, J., Redus, L., and O’Connor, J. C. (2018). Kynurenine pathway metabolic balance influences microglia activity: Targeting kynurenine monooxygenase to dampen neuroinflammation. Psychoneuroendocrinology 4, 1–10. doi: 10.1016/j.psyneuen.2018.04.019
Gibney, S. M., and Drexhage, H. A. (2013). Evidence for a dysregulated immune system in the etiology of psychiatric disorders. J. Neuroimmunol. Pharmacol. 8, 900–920. doi: 10.1007/s11481-013-9462-8S-2013
Gibney, S. M., McGuinness, B., Prendergast, C., Harkin, A., and Connor, T. J. (2013). Poly I:C-induced activation of the immune response accompanied by depression and anxiety-like behavior behaviors, kynurenine pathway activation and reduced BDNF expression. Brain Behav. Immun. 28, 170–181. doi: 10.1016/j.bbi.2012.11.010
Giles, G. I., Collins, C. A., Stone, T. W., and Jacob, C. (2003). Electrochemical and in vitro evaluation of the redox properties of kynurenine species. Biochem. Biophys. Res. Commun. 300, 719–724. doi: 10.1016/S0006-291X(02)02917-0
Ginhoux, F., Greter, M., Leboeuf, M., Nandi, S., See, P., Gokhan, S., et al. (2010). Fate mapping analysis reveals that adult microglia derive from primitive macrophages. Science 330, 841–845. doi: 10.1126/science.1194637
Giovanoli, S., Weber-Stadlbauer, U., Schedlowski, M., Meyer, U., and Engler, H. (2016). Prenatal immune activation causes hippocampal synaptic deficits in the absence of overt microglia anomalies. Brain Behav. Immun. 55, 25–38. doi: 10.1016/j.bbi.2015.09.015
Giulian, D., Vaca, K., and Noonan, C. A. (1990). Secretion of neurotoxins by mononuclear phagocytes infected with HIV-1. Science 250, 1593–1596. doi: 10.1126/science.2148832
Gobert, A., Rivet, J. M., Billiras, R., Parsons, F., and Millan, M. J. (2011). Simultaneous quantification of D- vs. L-serine, taurine, kynurenate, phosphoethanolamine and diverse amino acids in frontocortical dialysates of freely-moving rats: Differential modulation by N-methyl-D-aspartate (n.d.) and other pharmacological agents. J. Neurosci. Methods 202, 143–157. doi: 10.1016/j.jneumeth.2011.08.040
Goldstein, L. E., Leopold, M. C., Huang, X. D., Atwood, C. S., Saunders, A. J., Hartshorn, M., et al. (2000). 3-Hydroxy-kynurenine and 3-hydroxyanthranilic acid generate hydrogen peroxide and promote alpha-crystallin cross-linking by metal ion reduction. Biochemistry 39, 7266–7275. doi: 10.1021/bi992997s
Gomez-Nicola, D., Valle-Argos, B., Pallas-Bazarra, N., and Nieto-Sampedro, M. (2011). Interleukin-15 regulates proliferation and self-renewal of adult neural stem cells. Mol. Biol. Stem Cell 22, 1960–1970. doi: 10.1091/mbc.E11-01-0053
Goshen, I., Kreisel, T., Ben-Menachem-Zido, O., Licht, T., Weidenfeld, J., Ben-Hur, T., et al. (2008). Brain interleukin-1 mediates chronic stress-induced depression in mice via adrenocortical activation and hippocampal neurogenesis suppression. Mol. Psychiatr. 13, 717–728. doi: 10.1038/sj.mp.4002055
Gour, N., and Wills-Karp, M. (2015). IL-4 and IL-13 signaling in allergic airway disease. Cytokine 75, 68–78. doi: 10.1016/j.cyto.2015.05.014
Grellner, W. (2002). Time-dependent immunohistochemical detection of proinflammatory cytokines (IL-1 beta, IL-6, TNF-α) in human skin wounds. Forensic Sci. Int. 130, 90–96. doi: 10.1016/S0379-0738(02)00342-0
Grohmann, U., Fallarino, F., and Puccetti, P. (2003). Tolerance, DCs and tryptophan: Much ado about IDO. Trends Immunol. 24, 242–248. doi: 10.1016/S1471-4906(03)00072-3
Gruol, D. L. (2015). IL-6 regulation of synaptic function in the CNS. Neuropharmacology 96, 42–54. doi: 10.1016/j.neuropharm.2014.10.023
Gruol, D. L., Melkonian, C., Ly, K., Sisouvanthong, J., Tan, Y., and Roberts, A. J. (2020). Alcohol and IL-6 alter expression of synaptic proteins in cerebellum of transgenic mice with increased astrocyte expression of IL-6. Neuroscience 442, 124–137. doi: 10.1016/j.neuroscience.2020.06.043
Guerard, A., Laurent, V., Fromont, G., Esteve, D., Gilhodes, J., Bonnelye, E., et al. (2021). The chemokine receptor CCR3 is potentially involved in the homing of prostate cancer cells to bone: Implication of bone-marrow adipocytes. Int. J. Mol. Sci. 22:1994. doi: 10.3390/ijms22041994
Guidetti, P., and Schwarcz, R. (1999). 3-hydroxykynurenine potentiates quinolinate but not NMDA toxicity in the rat striatum. Eur. J. Neurosci. 11, 3857–3863. doi: 10.1046/j.1460-9568.1999.00806.x
Guidolin, D., Fuxe, K., Neri, G., Nussdorfer, G. G., and Agnati, L. F. (2007). On the role of receptor-receptor interactions and volume transmission in learning and memory. Brain Res. Rev. 55, 119–133. doi: 10.1016/j.brainresrev.2007.02.004
Guillemin, G. J. (2012). Quinolinic acid, the inescapable neurotoxin. FEBS J. 279, 1356–1365. doi: 10.1111/j.1742-4658.2012.08485.x
Guillemin, G. J., Croitoru-Lamoury, J., Dormont, D., Armati, P. J., and Brew, B. J. (2003). Quinolinic acid upregulates chemokine production and chemokine receptor expression in astrocytes. Glia 41, 371–381. doi: 10.1002/glia.10175
Guillemin, G. J., Kerr, S. J., Smythe, G. A., Smith, D. G., Kapoor, V., Armati, P. J., et al. (2001). Kynurenine pathway metabolism in human astrocytes: A paradox for neuronal protection. J. Neurochem. 78, 842–853. doi: 10.1046/j.1471-4159.2001.00498.x
Guillemin, G. J., Meininger, V., and Brew, B. J. (2005b). Implications for the kynurenine pathway and quinolinic acid in amyotrophic lateral sclerosis. Neurodegen. Dis. 2, 166–176. doi: 10.1159/000089622
Guillemin, G. J., Smythe, G., Takikawa, O., and Brew, B. J. (2005a). Expression of indoleamine 2,3-dioxygenase and production of quinolinic acid by human microglia, astrocytes, and neurons. Glia 49, 15–23. doi: 10.1002/glia.20090
Gumusoglu, S. B., Hing, B., Wen, Q., Chilukuri, A. S. S., Dewitt, J. J., Scroggins, S. M., et al. (2020). Chronic maternal interleukin-17 and autism-related cortical gene expression, neurobiology, and behavior. Neuropsychopharmacology 45, 1008–1017. doi: 10.1038/s41386-020-0640-0
Guo, D. F., Chen, Y. H., Wang, S. J., Yu, L., Shen, Y., Zhong, H. J., et al. (2018). Exosomes from heat-stressed tumour cells inhibit tumour growth by converting regulatory T cells to Th17 cells via IL-6. Immunology 154, 132–143. doi: 10.1111/imm.12874
Gutierrez, E. G., Banks, W. A., and Kastin, A. J. (1994). Blood-borne interleukin-1 rec-tor antagonist crosses the blood-brain-barrier. J. Neuroimmunol. 55, 153–160. doi: 10.1016/0165-5728(94)90005-1
Han, P., and Whelan, P. J. (2010). Tumor necrosis factor-a enhances glutamatergic transmission onto spinal motoneurons. J. Neurotrauma 27, 287–292. doi: 10.1089/neu.2009.1016
Hanisch, U. K. (2002). Microglia as a source and target of cytokines. Glia 40, 140–155. doi: 10.1002/glia.10161
Hanisch, U. K., and Kettenmann, H. (2007). Microglia: Active sensor and versatile effector cells in the normal and pathologic brain. Nat. Neurosci. 10, 1387–1394. doi: 10.1038/nn1997
Hanninen, A., Maksimow, M., Alam, C., Morgan, D. J., and Jalkanen, S. (2011). Ly6C supports preferential homing of central memory CD8(+) T cells into lymph nodes. Eur. J. Immunol. 41, 634–644. doi: 10.1002/eji.201040760
Harden, J. L., Lewis, S. M., Lish, S. R., Suarez-Farinas, M., Gareau, D., Lentini, T., et al. (2016). The tryptophan metabolism enzyme L-kynureninase is a novel inflammatory factor in psoriasis and other inflammatory diseases. J. Allergy Clin. Immunol. 137, 1830–1840. doi: 10.1016/j.jaci.2015.09.055
Harden, L. M., du Plessis, I., Roth, J., Loram, L. C., Poole, S., and Laburn, H. P. (2011). Differences in the relative involvement of peripherally released interleukin (IL)-6, brain IL-1 beta and prostanoids in mediating lipopolysaccharide- induced fever and sickness behavior. Psychoneuroendocrinology 36, 608–622. doi: 10.1016/j.psyneuen.2010.09.003
Haroon, E., Raison, C. L., and Miller, A. H. (2012). Psychoneuroimmunology meets neuropharmacology: Translational implications of the impact of inflammation on behavior. Neuropsychopharmacology 37, 137–162. doi: 10.1038/npp.2011.205
Harrison, J. K., Jiang, Y., Chen, S., Xia, Y., Maciejewski, D., McNamara, R. K., et al. (1998). Role for neuronally derived fractalkine in mediating interactions between neurons and CX3CR1-expressing microglia. Proc. Natl. Acad. Sci. U.S.A. 95, 10896–10901. doi: 10.1073/pnas.95.18.10896
Harrison, N. A., Brydon, L., Walker, C., Gray, M. A., Dolan, R. J., and Critchley, H. D. (2009). Neural origins of human sickness in interoceptive responses to inflammation. Biol. Psychiatr. 66, 415–422. doi: 10.1016/j.biopsych.2009.03.007
Hausmann, J. S. (2019). Targeting cytokines to treat autoinflammatory diseases. Clin. Immunol. 206, 23–32. doi: 10.1016/j.clim.2018.10.016
Hayashi, T., Mo, J. H., Gong, X., Rossetto, C., Jang, A., Beck, L., et al. (2007). 3- Hydroxyanthranilic acid inhibits PDK1 activation and suppresses experimental asthma by inducing T cell apoptosis. Proc. Natl. Acad. Sci. U.S.A. 104, 18619–18624. doi: 10.1073/pnas.0709261104
He, M. L., Lv, Z. Y., Shi, X., Yang, T., Zhang, Y., Li, T. Y., et al. (2017). Interleukin-10 release from astrocytes suppresses neuronal apoptosis via the TLR2/NF kappa B pathway in a neonatal rat model of hypoxic-ischemic brain damage. J. Neurochem. 142, 920–933. doi: 10.1111/jnc.14126
He, Y., Zhou, S., Liu, H., Shen, B., Zhao, H., Peng, K., et al. (2015). Indoleamine 2, 3-dioxgenase transfected mesenchymal stem cells induce kidney allograft tolerance by increasing the production and function of regulatory T cells. Transplantation 99, 1829–1838. doi: 10.1097/TP.0000000000000856
Heisler, J. M., and O’Connor, J. C. (2015). Indoleamine 2,3-dioxygenase-dependent neurotoxic kynurenine metabolism mediates inflammation-induced deficit in recognition memory. Brain Behav. Immun. 50, 115–124. doi: 10.1016/j.bbi.2015.06.022
Henderson, G., Johnson, J. W., and Ascher, P. (1990). Competitive antagonists and partial agonists at the glycine modulatory site of the mouse N-methyl-D-aspartate receptor. J. Physiol. 430, 189–212. doi: 10.1113/jphysiol.1990.sp018288
Heng, B., Bilgin, A. A., Lovejoy, D. B., Tan, V. X., Milioli, H. H., Gluch, L., et al. (2020). Differential kynurenine pathway metabolism in highly metastatic aggressive breast cancer subtypes: Beyond IDO1-induced immunosuppression. Breast Cancer Res. 20:113. doi: 10.1186/s13058-020-01351-1
Heng, B., Lim, C. K., Lovejoy, D. B., Bessede, A., Gluch, L., and Guillemin, G. J. (2016). Understanding the role of the kynurenine pathway in human breast cancer immunobiology. Oncotarget 7, 6506–6520. doi: 10.18632/oncotarget.6467
Heppner, F. L., Ransohoff, R. M., and Becher, B. (2015). Immune attack: The role of inflammation in Alzheimer disease. Nat. Rev. Neurosci. 16, 358–372. doi: 10.1038/nrn3880
Heredi, J., Cseh, E. K., Berko, A. M., Veres, G., Zadori, D., Toldi, J., et al. (2019). Investigating KYNA production and kynurenergic manipulation on acute mouse brain slice preparations. Brain Res. Bull. 146, 185–191. doi: 10.1016/j.brainresbull.2018.12.014
Hernandez, R. V., Puro, A. C., Manos, J. C., Huitron-Resendiz, S., Reyes, K. C., Liu, K., et al. (2016). Transgenic mice with increased astrocyte expression of IL-6 show altered effects of acute ethanol on synaptic function. Neuropharmacology 103, 27–43. doi: 10.1016/j.neuropharm.2015.12.015
Hernandez-Martinez, J. M., Forrest, C. M., Darlington, L. G., Smith, R. A., and Stone, T. W. (2017). Quinolinic acid induces neuritogenesis in SH-SY5Y neuroblastoma cells independently of NMDA receptor activation. Eur. J. Neurosci. 45, 700–711. doi: 10.1111/ejn.13499
Herrera, G., Calfa, G., Schioth, H. B., Lasaga, M., and Scimonelli, T. (2019). Memory consolidation impairment induced by Interleukin-1 beta is associated with changes in hippocampal structural plasticity. Behav. Brain Res. 370:111969. doi: 10.1016/j.bbr.2019.111969
Heyes, M. P., Jordan, E. K., Lee, K., Saito, K., Frank, J. A., Snoy, P. J., et al. (1992b). Relationship of neurologic status in macaques infected with the simian immunodeficiency virus to cerebrospinal-fluid quinolinic acid and kynurenic acid. Brain Res. 570, 237–250. doi: 10.1016/0006-8993(92)90587-Y
Heyes, M. P., Saito, K., and Markey, S. P. (1992a). Human macrophages convert l-tryptophan into the neurotoxin quinolinic acid. Biochem. J. 283, 633–635. doi: 10.1042/bj2830633
Hill, M., Tanguy-Royer, S., Royer, P., Chauveau, C., Asghar, K., Tesson, L., et al. (2007). IDO expands human CD4+CD25high regulatory T cells by promoting maturation of LPS-treated dendritic cells. Eur. J. Immunol. 37, 3054–3062. doi: 10.1002/eji.200636704
Hissong, B. D., and Carlin, J. M. (1997). Potentiation of interferon-induced indoleamine 2,3-dioxygenase mRNA in human mononuclear phagocytes by lipopolysaccharide and interleukin-1. J. Interferon Cytokine Res. 17, 387–393. doi: 10.1089/jir.1997.17.387
Hissong, B. D., Byrne, G., Padilla, M. I., and Carlin, J. M. (1995). Up-Regulation of interferon-induced indoleamine 2,3-dioxygenase in human macrophage cultures by lipopolysaccharide, muramyl tripeptide, and interleukin-1. Cell. Immunol. 160, 264–269. doi: 10.1016/0008-8749(95)80037-J
Hoeppli, R. E., MacDonald, K. N., Leclair, P., Fung, V. C. W., Mojibian, M., Gillies, J., et al. (2019). Tailoring the homing capacity of human tregs for directed migration to sites of Th1-inflammation or intestinal regions. Am. J. Transplant. 19, 62–76. doi: 10.1111/ajt.14936
Hoerning, A., Koss, K., Datta, D., Calzadilla, K., Benitez, F., Hoyer, P. F., et al. (2011). Subsets of human CD4(+) regulatory T cells express the peripheral homing receptor CXCR3. Eur. J. Immunol. 41, 2291–2302. doi: 10.1002/eji.201041095
Hong, J., and Hutton, G. J. (2010). Regulatory effects of interferon-beta on osteopontin and interleukin-17 expression in multiple sclerosis. J. Interferon Cytokine Res. 30, 751–757. doi: 10.1089/jir.2010.0082
Hridi, S. U., Barbour, M., Wilson, C., Franssen, A. J., Harte, T., Bushell, T. J., et al. (2021). Increased Levels of IL-16 in the central nervous system during neuroinflammation are associated with infiltrating immune cells and resident glial cells. Biology (Basel) 10:472. doi: 10.3390/biology10060472
Hridi, S. U., Franssen, A. J. P. M., Jiang, H. R., and Bushell, T. J. (2019). Interleukin-16 inhibits sodium channel function and GluA1 phosphorylation via CD4-and CD9-independent mechanisms to reduce hippocampal neuronal excitability and synaptic activity. Mol. Cell Neurosci. 95, 71–78. doi: 10.1016/j.mcn.2019.01.002
Huang, F., Chen, M. G., Chen, W. Q., Gu, J., Yuan, J., Xue, Y., et al. (2017). Human gingiva-derived mesenchymal stem cells inhibit xeno-graft-versus-host disease via CD39-CD73-adenosine and IDO signals. Front. Immunol. 8:68. doi: 10.3389/fimmu.2017.00068
Huang, K. F., Huang, W. T., Lin, K. C., Lin, M. T., and Chang, C. P. (2010). Interleukin-1 receptor antagonist inhibits the release of glutamate, hydroxyl radicals, and prostaglandin E-2 in the hypothalamus during pyrogen-induced fever in rabbits. Eur. J. Pharmacol. 629, 125–131. doi: 10.1016/j.ejphar.2009.11.060
Huang, Y. S., Cheng, S. N., Chueh, S. H., Tsai, Y. L., Liou, N. H., Guo, Y. W., et al. (2009). Effects of interleukin-15 on neuronal differentiation of neural stem cells. Brain Res. 1304, 38–48. doi: 10.1016/j.brainres.2009.09.009
Huang, Y. S., Ogbechi, J., Clanchy, F., Williams, R. O., and Stone, T. W. (2020). Kynurenine metabolites in peripheral disorders and neuroinflammation. Front. Immunol. 11:388. doi: 10.3389/fimmu.2020.00388
Huang, Y. S., Tseng, W. Y., Clanchy, F., Topping, L. M., Ogbechi, J., McNamee, K., et al. (2021). Pharmacological modulation of T cell immunity results in long-term remission of autoimmune arthritis. Proc. Natl. Acad. Sci. U.S.A. 118:e2100939118. doi: 10.1073/pnas.2100939118
Huck, J. H. J., Freyer, D., Boettcher, C., Mladinov, M., Muselmann-Genschow, C., Thielke, M., et al. (2015). De novo expression of dopamine D2 receptors on microglia after stroke. J. Cereb. Blood Flow Metab. 35, 1804–1811. doi: 10.1038/jcbfm.2015.128
Hunt, C., Cordeiro, T. M. E., Suchting, R., de Dios, C., Leal, V. A. C., Soares, J. C., et al. (2020). Effect of immune activation on the kynurenine pathway and depression symptoms–a systematic review and meta-analysis. Neurosci. Biobehav. Rev. 118, 514–523. doi: 10.1016/j.neubiorev.2020.08.010
Hurdayal, R., and Brombacher, F. (2017). Interleukin-4 receptor alpha: From innate to adaptive immunity in murine models of cutaneous leishmaniasis. Front. Immunol. 8:1354. doi: 10.3389/fimmu.2017.01354
Huse, M., Quann, E. J., and Davis, M. M. (2008). Shouts, whispers and the kiss of death: Directional secretion in T cells. Nat. Immunol. 9, 1105–1111. doi: 10.1038/ni.f.215
Jacobs, K. R., Castellano-Gonzalez, G., Guillemin, G. J., and Lovejoy, D. B. (2017). Major developments in the design of inhibitors along the kynurenine pathway. Curr. Med. Chem. 24, 2471–2495. doi: 10.2174/0929867324666170502123114
Jayawickrama, G. S., Sadig, R. R., Sun, G., Nematollahi, A., Nadvi, N. A., Hanrahan, J. R., et al. (2015). Kynurenine aminotransferases and the prospects of inhibitors for the treatment of schizophrenia. Curr. Med. Chem. 22, 2902–2918. doi: 10.2174/0929867322666150608094054
Johnson, J. D., Barnard, D. F., Kulp, A. C., and Mehta, D. M. (2019). Neuroendocrine regulation of brain cytokines after psychological stress. J. Endocr. Soc. 3, 1302–1320. doi: 10.1210/js.2019-00053
Johnston, L. K., and Bryce, P. J. (2017). Understanding interleukin 33 and its roles in eosinophil development. Front. Med. 4:51. doi: 10.3389/fmed.2017.00051
Jones, M. E., Lebonville, C. L., Paniccia, J. E., Balentine, M. E., Reissner, K. J., and Lysle, D. T. (2018). Hippocampal interleukin-1 mediates stress-enhanced fear learning: A potential role for astrocyte-derived interleukin-1 beta. Brain Behav. Immun. 67, 355–363. doi: 10.1016/j.bbi.2017.09.016
Jones, S. P., Franco, N. F., Varney, B., Sundaram, G., Brown, D. A., de Bie, J., et al. (2015). Expression of the kynurenine pathway in human peripheral blood mononuclear cells: Implications for inflammatory and neurodegenerative disease. PLoS One 10:e0131389. doi: 10.1371/journal.pone.0131389
Juhl, M., Follin, B., Gad, M., Larsen, J., Kastrup, J., and Ekblond, A. (2020). Adipose tissue-derived stromal cells induce a highly trophic environment while reducing maturation of monocyte-derived dendritic cells. Stem Cells Int. 2020:8868909. doi: 10.1155/2020/8868909
Jung, I. D., Lee, M. G., Chang, J. H., Lee, J. S., Jeong, Y. I., Lee, C. M., et al. (2009). Blockade of indoleamine 2,3-dioxygenase protects mice against lipopolysaccharide-induced endotoxin shock. J. Immunol. 182, 3146–3154. doi: 10.4049/jimmunol.0803104
Jurado-Manzano, B. B., Zavala-Reyes, D., Turrubiartes-Martinez, E. A., Portales-Perez, D. P., Gonzalez-Amaro, R., and Layseca-Espinosa, E. (2017). FICZ generates human tDCs that induce CD4(+) CD25(high) Foxp3(+) treg-like cell differentiation. Immunol. Lett. 190, 84–92. doi: 10.1016/j.imlet.2017.07.013
Jusof, F. F., Lim, C. K., Aziz, F. N., Soe, H. J., Raju, C. S., Sekaran, S. D., et al. (2022). The Cytokines CXCL10 and CCL2 and the kynurenine metabolite anthranilic acid accurately predict patients at risk of developing dengue with warning signs. J. Infect. Dis. 29:jiac273. doi: 10.1093/infdis/jiac273
Kai, K., Komohara, Y., Esumi, S., Fujiwara, Y., Yamamoto, T., Uekawa, K., et al. (2021). Macrophage/microglia-derived IL-1beta induces glioblastoma growth via the STAT3/NF-kappa B pathway. Hum. Cell 35, 226–237. doi: 10.1007/s13577-021-00619-8
Kamimura, D., and Murakami, M. (2019). Neural stimulations regulate the infiltration of immune cells into the CNS. J. Int. Med. 286, 259–267. doi: 10.1111/joim.12912
Kaminska, J., Dymicka-Piekarska, V., Chrzanowski, R., Sawicki, K., Milewska, A. J., Zinczuk, J., et al. (2021). IL-6 quotient (The ratio of cerebrospinal fluid IL-6 to serum IL-6) as a biomarker of an unruptured intracranial aneurysm. J. Inflamm. Res. 14, 6103–6114. doi: 10.2147/JIR.S335618
Kanashiro, A., Hiroki, C. H., da Fonseca, D. M., Birbrair, A., Ferreira, R. G., Bassi, G. S., et al. (2020). The role of neutrophils in neuro-immune modulation. Pharmacol. Res. 151:104580. doi: 10.1016/j.phrs.2019.104580
Kandanearatchi, A., and Brew, B. J. (2012). The kynurenine pathway and quinolinic acid: Pivotal roles in HIV associated neurocognitive disorders. FEBS J. 279, 1366–1374. doi: 10.1111/j.1742-4658.2012.08500.x
Kaplanski, G. (2018). Interleukin-18: Biological properties and role in disease pathogenesis. Immunol. Rev. 281, 138–153. doi: 10.1111/imr.12616
Kara, E. E., McKenzie, D. R., Bastow, C. R., Gregor, C. E., Fenix, K. A., Ogunniyi, A. D., et al. (2015). CCR2 defines in vivo development and homing of IL-23-driven GM-CSF-producing Th17 cells. Nat. Commun. 6:8644. doi: 10.1038/ncomms9644
Kashiwamata, S., Nakashima, K., and Kotake, Y. (1966). Anthranilic acid hydroxylation by rabbitliver microsomes. Biochim. Biophys. Acta Enzymol. Biol. Oxid. 113, 244–254. doi: 10.1016/s0926-6593(66)80065-6
Kastin, A. J., Akerstrom, V., and Pan, W. H. (2003). Interleukin-10 as a CNS therapeutic: The obstacle of the blood-brain/blood-spinal cord barrier. Mol. Brain 114, 168–171. doi: 10.1016/S0169-328X(03)00167-0
Kawalkowska, J. Z., Ogbechi, J., Venables, P. J., and Williams, R. O. (2019). cIAP1/2 inhibition synergizes with TNF inhibition in autoimmunity by down-regulating IL-17A and inducing T-regs. Sci. Adv. 5:eaaw5422. doi: 10.1126/sciadv.aaw5422
Kawasaki, Y., Zhang, L., Cheng, J. K., and Ji, R. R. (2008). Cytokine mechanisms of central sensitization: Distinct and overlapping role of interleukin-1beta, interleukin-6, and tumor necrosis factor-alpha in regulating synaptic and neuronal activity in the superficial spinal cord. J. Neurosci. 28, 5189–5194. doi: 10.1523/JNEUROSCI.3338-07.2008
Kelly, A., Lynch, A., Vereker, E., Nolan, Y., Queenan, P., Whittaker, E., et al. (2001). The anti-inflammatory cytokine, interleukin (IL)-10, blocks the inhibitory effect of IL-1β on long term potentiation. A role for JNK. J. Biol. Chem. 276, 45564–45572. doi: 10.1074/jbc.M108757200
Kent, S., Bluthe, R. M., Kelley, K. W., and Dantzer, R. (1992). Sickness behavior as a new target for drug development. Trends Pharmacol. Sci. 13, 24–28. doi: 10.1016/0165-6147(92)90012-U
Kettenmann, H., Hanisch, U. K., Noda, M., and Verkhratsky, A. (2010). Physiology of microglia. Physiol. Rev. 91, 461–553. doi: 10.1152/physrev.00011.2010
Khalil, O. S., Pisar, M., Forrest, C. M., Vincenten, M. C. J., Darlington, L. G., and Stone, T. W. (2014). Prenatal inhibition of the kynurenine pathway leads to structural changes in the hippocampus of adult rat offspring. Eur. J. Neurosci. 39, 1558–1571. doi: 10.1111/ejn.12535
Kiank, C., Zeden, J. P., Drude, S., Domanska, G., Fusch, G., Otten, W., et al. (2010). Psychological stress-induced, IDO1-dependent tryptophan catabolism: Implications on immunosuppression in mice and humans. PLoS One 5:e11825. doi: 10.1371/journal.pone.0011825
Kilonzo, K., van der Veen, B., Teutsch, J., Schulz, S., Kapanaiah, S. K. T., Liss, B., et al. (2021). Delayed-matching-to-position working memory in mice relies on NMDA-receptors in prefrontal pyramidal cells. Sci. Rep. 11:AR8788. doi: 10.1038/s41598-021-88200-z
Kim, H., Chen, L., Lim, G., Sung, B., Wang, S. X., McCabe, M. F., et al. (2012). Brain indoleamine 2,3-dioxygenase contributes to the comorbidity of pain and depression. J. Clin. Invest. 122, 2940–2954. doi: 10.1172/JCI61884
Kim, Y. K., Amidfar, M., and Won, E. (2019). A review on inflammatory cytokine-induced alterations of the brain as potential neural biomarkers in post-traumatic stress disorder. Progr. Neuro Psychopharmacol. Biol. Psychiatr. 91, 103–112. doi: 10.1016/j.pnpbp.2018.06.008
Kindler, J., Lim, C. K., Weickert, C. S., Boerrigter, D., Galletly, C., Liu, D., et al. (2020). Dysregulation of kynurenine metabolism is related to proinflammatory cytokines, attention, and prefrontal cortex volume in schizophrenia. Mol. Psychiatr. 25, 2860–2872. doi: 10.1038/s41380-019-0401-9
Kipnis, J. (2016). Multifaceted interactions between adaptive immunity and the central nervous system. Science 353, 766–771. doi: 10.1126/science.aag2638
Kipnis, J., Gadani, S., and Derecki, N. C. (2012). Pro-cognitive properties of T cells. Nat. Rev. Immunol. 12, 662–668. doi: 10.1038/nri3280
Kirillova, G. P., Hrutkay, R. J., Shurin, M. R., Shurin, G. V., Tourkova, I. L., and Vanyukov, M. M. (2008). Dopamine receptors in human lymphocytes: Radioligand binding and quantitative RT-PCR assays. J. Neurosci. Methods 174, 272–280. doi: 10.1016/j.jneumeth.2008.07.018
Kmiolek, T., Rzeszotarska, E., Wajda, A., Walczuk, E., Kuca-Warnawin, E., Romanowska-Prochnicka, K., et al. (2020). The interplay between transcriptional factors and MicroRNAs as an important factor for Th17/Treg balance in RA patients. Int. J. Mol. Sci. 21:AR7169. doi: 10.3390/ijms21197169
Kolodziej, L. R., Paleolog, E. M., and Williams, R. O. (2011). Kynurenine metabolism in health and disease. Amino Acids 41, 1173–1183. doi: 10.1007/s00726-010-0787-9
Konsman, J. P., Veeneman, J., Combe, C., Poole, S., Luheshi, G. N., and Dantzer, R. (2008). Central nervous action of interleukin-1 mediates activation of limbic structures and behavioral depression in response to peripheral administration of bacterial lipopolysaccharide. Eur. J. Neurosci. 28, 2499–2510. doi: 10.1111/j.1460-9568.2008.06549.x
Koo, J. W., and Duman, R. S. (2008). IL-1 beta is an essential mediator of the antineurogenic and anhedonic effects of stress. Proc. Natl. Acad. Sci. U.S.A. 105, 751–756. doi: 10.1073/pnas.0708092105
Kotake, Y., Shibata, Y., and Toratani, A. (1956). On the hydroxylation of anthranilic acid and kynurenine. Proc. Jpn. Acad. 32, 774–777.
Kozak, R., Campbell, B. M., Strick, C. A., Horner, W., Hoffmann, W. E., Kiss, T., et al. (2014). Reduction of brain kynurenic acid improves cognitive function. J. Neurosci. 34, 10592–10602. doi: 10.1523/jneurosci.1107-14.2014
Kraft, A. D., and Harry, G. J. (2011). Features of microglia and neuroinflammation relevant to environmental exposure and neurotoxicity. Int. J. Environ. Res. Public Health 8, 2980–3018. doi: 10.3390/ijerph8072980
Krause, D., Suh, H. S., Tarassishin, L., Cui, Q. L., Durafourt, B. A., Choi, N., et al. (2011). The tryptophan metabolite 3-hydroxyanthranilic acid plays anti-inflammatory and neuroprotective roles during inflammation. Am. J. Pathol. 179, 1360–1372. doi: 10.1016/j.ajpath.2011.05.048
Krupa, A., and Kowalska, I. (2021). The kynurenine pathway – new linkage between innate and adaptive immunity in autoimmune endocrinopathies. Inter. J. Mol. Sci. 22:9879. doi: 10.3390/ijms22189879
Kubicova, L., Hadacek, F., and Chobot, V. (2013). Quinolinic acid: Neurotoxin or oxidative stress modulator? Int. J. Mol. Sci. 14, 21328–21338. doi: 10.3390/ijms141121328
Kvaratskhelia, E., Maisuradze, E., Dabrundashvili, N. G., Natsvlishvili, N., Zhuravliova, E., and Mikeladze, D. G. (2009). N-Methyl-D-aspartate and sigma-ligands change the production of interleukins 8 and 10 in lymphocytes through modulation of the NMDA glutamate receptor. Neuroimmunomodulation 16, 201–207. doi: 10.1159/000204234
Kwilasz, A. J., Grace, P. M., Serbedzija, P., Maier, S. F., and Watkins, L. R. (2015). The therapeutic potential of interleukin-10 in neuroimmune diseases. Neuropharmacology 96, 55–69. doi: 10.1016/j.neuropharm.2014.10.020
Kyttaris, V. C. (2019). Targeting cytokines to treat autoimmunity. Clin. Immunol. 206:108251. doi: 10.1016/j.clim.2019.108251
Ladomersky, E., Zhai, L., Lenzen, A., Lauing, K. L., Qian, J., Scholtens, D. M., et al. (2018). IDO1 inhibition synergizes with radiation and PD-1 blockade to durably increase survival against advanced glioblastoma. Clin. Cancer Res. 24, 2559–2573. doi: 10.1158/1078-0432.CCR-17-3573
Laffer, B., Bauer, D., Wasmuth, S., Busch, M., Jalilvand, T. V., Thanos, S., et al. (2019). Loss of IL-10 promotes differentiation of microglia to a M1 phenotype. Front. Cell. Neurosci. 13:430. doi: 10.3389/fncel.2019.00430
Lai, A. Y., Swayze, R. D., El-Husseini, A., and Song, C. (2006). Interleukin-1 beta modulates AMPA receptor expression and phosphorylation in hippocampal neurons. J. Neuroimmunol. 175, 97–106. doi: 10.1016/j.jneuroim.2006.03.001
Lai, W. Y., and Mueller, A. I. (2021). Latest update on chemokine receptors as therapeutic targets. Biochem. Soc. Trans. 49, 1385–1395. doi: 10.1042/BST20201114
Laumet, G., Edralin, J. D., Chiang, A. C. A., Dantzer, R., Heijnen, C. J., and Kavelaars, A. (2018). Resolution of inflammation-induced depression requires T lymphocytes and endogenous brain interleukin-10 signaling. Neuropsychopharmacology 43, 2597–2605. doi: 10.1038/s41386-018-0154-1
Lavieri, R., Rubartelli, A., and Carta, S. (2016). Redox stress unbalances the inflammatory cytokine network: Role in autoinflammatory patients and healthy subjects. J. Leukocyte Biol. 99, 79–86. doi: 10.1189/jlb.3MR0415-159R
Lawson, M. A., McCusker, R. H., and Kelley, K. W. (2013). Interleukin-1 beta converting enzyme is necessary for development of depression-like behavior following intracerebroventricular administration of lipopolysaccharide to mice. J. Neuroinflamm. 10:54. doi: 10.1186/1742-2094-10-54
Ledeboer, A., Brevé, J. J., Poole, S., Tilders, F. J., and Van Dam, A. M. (2000). Interleukin-10, interleukin-4, and transforming growth factor-β differentially regulate lipopolysaccharide-induced production of pro-inflammatory cytokines and nitric oxide in co-cultures of rat astroglial and microglial cells. Glia 30, 134–142. doi: 10.1002/(SICI)1098-1136(200004)30:2<134::AID-GLIA3<3.0.CO;2-3
Lee, J. M., Tan, V., Lovejoy, D., Braidy, N., Rowe, D. B., Brew, B. J., et al. (2017). Involvement of quinolinic acid in the neuropathogenesis of amyotrophic lateral sclerosis. Neuropharmacology 112, 346–364. doi: 10.1016/j.neuropharm.2016.05.011
Lee, S. M., Lee, Y.-S., Choi, J. H., Park, S. G., Choi, L. W., Joo, Y. D., et al. (2010). Tryptophan metabolite 3-hydroxyanthranilic acid selectively induces activated T cell death via intracellular GSH depletion. Immunol. Lett. 132, 53–60. doi: 10.1016/j.imlet.2010.05.008
Lee, T. S., and Chau, L. Y. (2002). Heme oxygenase-1 mediates the anti-inflammatory effect of interleukin-10 in mice. Nat. Med. 8, 240–246. doi: 10.1038/nm0302-240
Lee, W. H., Seo, D., Lim, S. G., and Suk, K. (2019). Reverse signaling of tumor necrosis factor superfamily proteins in macrophages and microglia: Superfamily portrait in the neuroimmune interface. Front. Immunol. 10:AR262. doi: 10.3389/fimmu.2019.00262
Lee, W. S., Lee, S. M., Kim, M. K., Park, S. G., Choi, I. W., Choi, I., et al. (2013). The tryptophan metabolite 3-hydroxyanthranilic acid suppresses T cell responses by inhibiting dendritic cell activation. Int. Immunopharmacol. 17, 721–726. doi: 10.1016/j.intimp.2013.08.018
Leiter, O., Kempermann, G., and Walker, T. L. (2016). A common language: How neuroimmunological cross talk regulates adult hippocampal neurogenesis. Stem Cells Int. 2016:1681590. doi: 10.1155/2016/1681590
Lepennetier, G., Hracsko, Z., Unger, M., Van Griensven, M., Grummel, V., Krumbholz, M., et al. (2019). Cytokine and immune cell profiling in the cerebrospinal fluid of patients with neuro-inflammatory diseases. J. Neuroinflamm. 16:219. doi: 10.1186/s12974-019-1601
Levite, M. (2016). Dopamine and T cells: Dopamine receptors and potent effects on T cells, dopamine production in T cells, and abnormalities in the dopaminergic system in T cells in autoimmune, neurological and psychiatric diseases. Acta Physiol. 216, 42–89. doi: 10.1111/apha.12476
Lewitus, G. M., Pribiag, H., Duseja, R., St-Hilaire, M., and Stellwagen, D. (2014). An adaptive role of TNF-α in the regulation of striatal synapses. J. Neurosci. 34, 6146–6155. doi: 10.1523/JNEUROSCI.3481-13.2014
Li, J., Xie, W., Zhang, J. M., and Baccei, M. L. (2009). Peripheral nerve injury sensitizes neonatal dorsal horn neurons to tumor necrosis factor-alpha. Mol. Pain 5:10. doi: 10.1186/1744-8069-5-10
Li, M., Li, Z., Yao, Y., Jin, W. N., Wood, K., Liu, Q., et al. (2017). Astrocyte-derived interleukin-15 exacerbates ischemic brain injury via propagation of cellular immunity. Proc. Nat. Acad. Sci. U.S.A. 114, E396–E405. doi: 10.1073/pnas.1612930114
Li, Q. S., Harden, J. L., Anderson, C. D., and Egilmez, N. K. (2016). Tolerogenic phenotype of IFN-γ-induced IDO+ dendritic cells is maintained via an autocrine IDO-kynurenine / AhR-IDO loop. J. Immunol. 197, 962–970. doi: 10.4049/jimmunol.1502615
Liblau, R. S., Gonzalez-Dunia, D., Wiendl, H., and Zipp, F. (2013). Neurons as targets for T cells in the nervous system. Trends Neurosci. 36, 315–324. doi: 10.1016/j.tins.2013.01.008
Lisak, R. P., Benjamins, J. A., Bealmear, B., Nedelkoska, L., Studzinski, D., Retland, E., et al. (2009). Differential effects of Th1, monocyte/macrophage and Th2 cytokine mixtures on early gene expression for molecules associated with metabolism, signaling and regulation in central nervous system mixed glial cell cultures. J. Neuroinflamm. 6:4. doi: 10.1186/1742-2094-6-4
Litzenburger, U. M., Opitz, C. A., Sahm, F., Rauschenbach, K. J., Trump, S., Winter, M., et al. (2014). Constitutive IDO expression in human cancer is sustained by an autocrine signaling loop involving IL-6, STAT3 and the AHR. Oncotarget 5, 1038–1051. doi: 10.18632/oncotarget.1637
Liu, H., Huang, L., Bradley, J., Liu, K., Bardhan, K., Ron, D., et al. (2014). GCN2-dependent metabolic stress is essential for endotoxemic cytokine induction and pathology. Mol. Cell. Biol. 34, 428–438. doi: 10.1128/MCB.00946-13
Liu, T., Jiang, C. Y., Fujita, T., Luo, S. W., and Kumamoto, E. (2013). Enhancement by interleukin-1 beta of AMPA and NMDA receptor-mediated currents in adult rat spinal superficial dorsal horn neurons. Mol. Pain 9:16. doi: 10.1186/1744-8069-9-16
Liu, X., Chen, Y., Wang, H., Wei, Y., Yuan, Y., Zhou, Q., et al. (2021). Microglia-derived IL-1 beta promoted neuronal apoptosis through ER stress-mediated signaling pathway PERK/eIF2 alpha/ATF4/CHOP upon arsenic exposure. J. Hazard. Mater. 417:125997. doi: 10.1016/j.jhazmat.2021.125997
Liu, Y. C., Hsiao, H. T., Wang, J. C. F., Wen, T. C., and Chen, S. L. (2022). TGF-beta 1 in plasma and cerebrospinal fluid can be used as a biological indicator of chronic pain in patients with osteoarthritis. PLoS One 17:e0262074. doi: 10.1371/journal.pone.0262074
Lobo-Silva, D., Carriche, G. M., Gil, C. A., Roque, S., and Saraiva, M. (2016). Balancing the immune response in the brain: IL-10 and its regulation. J. Neuroinflamm. 13:297. doi: 10.1186/s12974-016-0763-8
Lombardi, G., Dianzani, C., Miglio, G., Canonico, P. L., and Fantozzi, R. (2001). Characterization of ionotropic glutamate receptors in human lymphocytes. Br. J. Pharmacol. 133, 936–944. doi: 10.1038/sj.bjp.0704134
Loof, T., Kramer, S., Gaedeke, J., Neumayer, H. H., and Peters, H. (2016). IL-17 expression in the time course of acute anti-Thy1 glomerulonephritis. PLoS One 11:e0156480. doi: 10.1371/journal.pone.0156480
Lopez, A. S., Alegre, E., Diaz-Lagares, A., Garcia-Giron, C., Coma, M. J., and Gonzalez, A. (2008). Effect of 3-hydroxyanthranilic acid in the immunosuppressive molecules indoleamine dioxygenase and HLA-G in macrophages. Immunol. Lett. 117, 91–95. doi: 10.1016/j.imlet.2008.01.001
Lopez, A. S., Alegre, E., LeMaoult, J., Carosella, E., and Gonzalez, A. (2006). Regulatory role of tryptophan degradation pathway in HLA-G expression by human monocyte-derived dendritic cells. Mol. Immunol. 43, 2151–2160. doi: 10.1016/j.molimm.2006.01.007
Lovelace, M. D., Varney, B., Sundaram, G., Lennon, M. J., Lim, C. K., Jacobs, K., et al. (2017). Recent evidence for an expanded role of the kynurenine pathway of tryptophan metabolism in neurological diseases. Neuropharmacology 112, 373–388. doi: 10.1016/j.neuropharm.2016.03.024
Lowe, M. M., Mold, J. E., Kanwar, B., Huang, Y., Louie, A., Pollastri, M. P., et al. (2014). Identification of cinnabarinic acid as a novel endogenous aryl hydrocarbon receptor ligand that drives IL-22 production. PLoS One 9:e87877. doi: 10.1371/journal.pone.0087877
Loxton, N. W., Rohlwink, U. K., Tshavhungwe, M., Dlamini, L., Shey, M., Enslin, N., et al. (2021). A pilot study of inflammatory mediators in brain extracellular fluid in paediatric TBM. PLoS One 16:e0246997. doi: 10.1371/journal.pone.0246997
Lu, X. T., Zhao, Y. X., Zhang, Y., and Jiang, F. (2013). Psychological stress, vascular inflammation, and atherogenesis: Potential roles of circulating cytokines. J. Cardiovasc. Pharmacol. 62, 6–12. doi: 10.1097/FJC.0b013e3182858fac
Luchowska, E., Kloc, R., Olajossy, B., Wnuk, S., Wielosz, M., Owe-Larsson, B., et al. (2009). Beta-adrenergic enhancement of brain kynurenic acid production mediated via cAMP-related protein kinase a signalling. Prog. Neuropsychopharmacol. Biol. Psychiatr. 33, 519–529. doi: 10.1016/j.pnpbp.2009.02.002
Luchowski, P., and Urbanska, E. M. (2007). SNAP and SIN-1 increase brain production of kynurenic acid. Eur. J. Pharmacol. 563, 130–133. doi: 10.1016/j.ejphar.2007.02.044
Lumertz, F. S., Kestering-Ferreira, E., Orso, R., Creutzberg, K. C., Tractenberg, S. G., Stocchero, B. A., et al. (2022). Effects of early life stress on brain cytokines: A systematic review and meta-analysis of rodent studies. Neurosci. Biobehav. Rev. 139:104746. doi: 10.1016/j.neubiorev.2022.104746
Lupien, S. J., McEwen, B. S., Gunnar, M. R., and Heim, C. (2009). Effects of stress throughout the lifespan on the brain, behavior and cognition. Nat. Rev. Neurosci. 10, 434–445. doi: 10.1038/nrn2639
Lupsa, N., Ersek, B., Horvath, A., Bencsik, A., Lajko, E., Sillo, P., et al. (2018). Skin-homing CD8(+) T cells preferentially express GPI-anchored peptidase inhibitor 16, an inhibitor of cathepsin K. Eur. J. Immunol. 48, 1944–1957. doi: 10.1002/eji.201847552
Luscher, C., and Malenka, R. C. (2011). Drug-evoked synaptic plasticity in addiction: From molecular changes to circuit remodelling. Neuron 69, 650–663. doi: 10.1016/j.neuron.2011.01.017
Mackay, G. M., Forrest, C. M., Christofides, J., Bridel, M. A., Mitchell, S., Cowlard, R., et al. (2009). Kynurenine metabolites and inflammation markers in depressed patients treated with fluoxetine or counselling. Clin. Exp. Pharmacol. Physiol. 36, 425–435. doi: 10.1111/j.1440-1681.2008.05077.x
Mackenzie, A. E., and Milligan, G. (2015). The emerging pharmacology and function of GPR35 in the nervous system. Neuropharmacology 113, 661–671. doi: 10.1016/j.neuropharm.2015.07.035
Mahmoud, S., Gharagozloo, M., Simard, C., and Gris, D. (2019). Astrocytes maintain glutamate homeostasis in the CNS by controlling the balance between glutamate uptake and release. Cells 8:184. doi: 10.3390/cells8020184
Mai, C. L., Wei, X., Gui, W. S., Xu, Y. N., Zhang, U., Lin, Z. J., et al. (2019). Differential regulation of GSK-3 beta in spinal dorsal horn and in hippocampus mediated by interleukin-1beta contributes to pain hypersensitivity and memory deficits following peripheral nerve injury. Mol. Pain 15:1744806919826789. doi: 10.1177/1744806919826789
Majlath, Z., Annus, A., and Vecsei, L. (2018). Kynurenine system and multiple sclerosis, pathomechanism and drug targets with an emphasis on laquinimod. Curr. Drug Targets 19, 805–814. doi: 10.2174/1389450117666161223125417
Majlath, Z., Toldi, J., and Vecsei, L. (2014). The potential role of kynurenines in Alzheimer’s disease: Pathomechanism and therapeutic possibilities by influencing the glutamate receptors. J. Neural Transm. 121, 881–889. doi: 10.1007/s00702-013-1135-5
Malaczewska, J., Siwicki, A. K., Wojcik, R. A., Turski, W. A., and Kaczorek, E. (2016). The effect of kynurenic acid on the synthesis of selected cytokines by murine splenocytes – in vitro and ex vivo studies. Cent. Eur. J. Immunol. 1, 39–46. doi: 10.5114/ceji.2016.58815
Malarkey, E. B., and Parpura, V. (2008). Mechanisms of glutamate release from astrocytes. Neurochem. Int. 52, 142–154. doi: 10.1016/j.neuint.2007.06.005
Malemud, C. J., and Reddy, S. K. (2008). Targeting cytokines, chemokines and adhesion molecules in rheumatoid arthritis. Curr. Rheumatol. Rev. 4, 219–234. doi: 10.2174/157339708786263933
Mandi, Y., and Vecsei, L. (2012). The kynurenine system and immunoregulation. J. Neural Transm. 119, 197–209. doi: 10.1007/s00702-011-0681-y
Manzari, B., Kudlow, J. E., Fardin, P., Merello, E., Ottaviano, C., Puppo, M., et al. (2007). Induction of macrophage glutamine: Fructose-6-phosphate amidotransferase expression by hypoxia and by picolinic acid. Int. J. Immunopathol. Pharmacol. 20, 47–58. doi: 10.1177/039463200702000106
Martineau, M. (2013). Gliotransmission: Focus on exocytotic release of L-glutamate and D-serine from astrocytes. Biochem. Soc. Trans. 41, 1557–1561. doi: 10.1042/BST20130195
Marx, W., McGuiness, A. J., Rocks, T., Ruusunen, A., Cleminson, J., Walker, A. J., et al. (2021). The kynurenine pathway in major depressive disorder, bipolar disorder, and schizophrenia: A meta-analysis of 101 studies. Mol. Psychiatr. 26, 4158–4178. doi: 10.1038/s41380-020-00951-9
Masgrau, R., Guaza, C., Ransohoff, R. M., and Galea, E. (2017). Should we stop saying ‘Glia’ and ‘Neuroinflammation’? J. Mol. Med. 23, 486–500. doi: 10.1016/j.molmed.2017.04.005
Mashkina, A. P., Cizkova, D., Vanicky, I., and Boldyrev, A. A. (2010). NMDA receptors are expressed in lymphocytes activated both in vitro and in vivo. Cell. Mol. Neurobiol. 30, 901–907. doi: 10.1007/s10571-010-9519-7
Maslinska, D. (2001). The cytokine network and interleukin-15 (IL-15) in brain development. Folia Neuropathol. 39, 43–47.
Matt, S. M., and Gaskill, P. J. (2020). Where is dopamine and how do immune cells see it?: Dopamine-Mediated immune cell function in health and disease. J. Neuroimmune Pharmacol. 15, 114–164. doi: 10.1007/s11481-019-09851-4
Maya, S., Prakash, T., and Goli, D. (2018). Effect of wedelolactone and gallic acid on quinolinic acid-induced neurotoxicity and impaired motor function: Significance to sporadic amyotrophic lateral sclerosis. Neurotoxicology 68, 1–12. doi: 10.1016/j.neuro.2018.06.015.
McIlroy, D., Tanguy-Royer, S., Le Meur, N., Guisle, I., Royer, P. J., Leger, J., et al. (2005). Profiling dendritic cell maturation with dedicated microarrays. J. Leukocyte Biol. 78, 794–803. doi: 10.1189/jlb.0105029
McKinley, M. J., and Oldfield, B. J. (1998). The brain as an endocrine target for peptide hormones. Trends Endocrinol. Metab. 9, 349–354. doi: 10.1016/S1043-2760(98)00092-7
McNearney, T. A., Ma, Y. H., Chen, Y. P., Taglialatela, G., Yin, H. Z., Zhang, W. R., et al. (2010). A peripheral neuroimmune link: Glutamate agonists upregulate NMDA NR1 receptor mRNA and protein, vimentin, TNF-α, and RANTES in cultured human synoviocytes. Am. J. Physiol. 298, R584–R598. doi: 10.1152/ajpregu.00452.2010
McQuail, J. A., Beas, S., Kelly, K. B., Hernandez, C. M., Bizon, J. L., and Frazier, C. J. (2021). Attenuated NMDAR signaling on fast-spiking interneurons in prefrontal cortex contributes to age-related decline of cognitive flexibility. Neuropharmacology 197:108720. doi: 10.1016/j.neuropharm.2021.108720
Medana, I. M., Gallimore, A., Oxenius, A., Martinic, M. M. A., Wekerle, H., and Neumann, H. (2000). MHC class I-restricted killing of neurons by virus-specific CD8(+) T lymphocytes is effected through the Fas/FasL, but not the perforin pathway. Eur. J. Immunol. 30, 3623–3633. doi: 10.1002/1521-4141(200012)30:12<3623::AID-IMMU3623<3.0.CO;2-F
Melillo, G., Cox, G. W., Radzioch, D., and Varesio, L. (1993). Picolinic-acid, a catabolite of l-tryptophan, a co-stimulus for the induction of reactive nitrogen intermediate production in murine macrophages. J. Immunol. 150, 4031–4040.
Melnikov, M., Rogovskii, V., Boyko, A., and Pashenkov, M. (2018). The influence of biogenic amines on Th17-mediated immune response in multiple sclerosis. Mult. Scler. Relat. Dis. 21, 19–23. doi: 10.1016/j.msard.2018.02.012
Melnikov, M., Rogovskii, V., Boyko, A., and Pashenkov, M. (2020). Dopaminergic therapeutics in multiple sclerosis: Focus on Th17-cell functions. J. Neuroimmune Pharmacol. 15, 37–47. doi: 10.1007/s11481-019-09852-3
Merlo, L. M. F., Peng, W. D., DuHadaway, J. B., Montgomery, J. D., Prendergast, G. C., Muller, A. J., et al. (2022). The immunomodulatory enzyme IDO2 mediates autoimmune arthritis through a nonenzymatic mechanism. J. Immunol. 208, 571–581. doi: 10.4049/jimmunol.2100705
Merlo, L. M., Pigott, E., DuHadaway, J. B., Grabler, S., Metz, R., Prendergast, G. C., et al. (2014). IDO2 is a critical mediator of autoantibody production and inflammatory pathogenesis in a mouse model of autoimmune arthritis. J. Immunol. 192, 2082–2090. doi: 10.4049/jimmunol.1303012
Metghalchi, S., Ponnuswamy, P., Simon, T., Haddad, Y., Laurans, L., Clement, M., et al. (2015). Indoleamine 2,3-dioxygenase fine-tunes immune homeostasis in atherosclerosis and colitis through repression of interleukin-10 production. Cell Metab. 22, 460–471. doi: 10.1016/j.cmet.2015.07.004
Metz, R., Smith, C., DuHadaway, J. B., Chandler, P., Baban, B., Merlo, L. M. F., et al. (2014). IDO2 is critical for IDO1-mediated T-cell regulation and exerts a non-redundant function in inflammation. Int. Immunol. 26, 357–367. doi: 10.1093/intimm/dxt073
Mezrich, J. D., Fechner, J. H., Zhang, X., Johnson, B. P., Burlingham, W. J., and Bradfield, C. A. (2010). An interaction between kynurenine and the aryl hydrocarbon receptor can generate regulatory T cells. J. Immunol. 185, 3190–3198. doi: 10.4049/jimmunol.0903670
Mignini, F., Sabbatini, M., Capacchietti, M., Amantini, C., Bianchi, E., Artico, M., et al. (2013). T-cell sub-populations express a different pattern of dopaminergic markers in intra- and extra-thymic compartments. J. Biol. Reg. Homeost. Agents 27, 463–475.
Mikhak, Z., Fleming, C. M., Medoff, B. D., Thomas, S. Y., Tager, A. M., Campanella, G. S., et al. (2006). STAT1 in peripheral tissue differentially regulates homing of antigen-specific Th1 and Th2 cells. J. Immunol. 176, 4959–4967. doi: 10.4049/jimmunol.176.8.4959
Milewski, K., Bogacinska-Karas, M., Hilgier, W., Albrecht, J., and Zielinska, M. (2019). TNF-α increases STAT3-mediated expression of glutaminase isoform KGA in cultured rat astrocytes. Cytokine 123:154774. doi: 10.1016/j.cyto.2019.154774
Miller, A. H., Haroon, E., Raison, C. L., and Felger, J. C. (2013). Cytokine targets in the brain: Impact on neurotransmitters and neurocircuits. Depress. Anxiety 30, 297–306. doi: 10.1002/da.22084
Millett, C. E., Harder, J., Locascio, J. J., Shanahan, M., Santone, G., Fichorova, R. N., et al. (2020). TNF-α and its soluble receptors mediate the relationship between prior severe mood episodes and cognitive dysfunction in euthymic bipolar disorder. Brain Behav. Immun. 88, 403–410. doi: 10.1016/j.bbi.2020.04.003
Milovanovic, J., Arsenijevic, A., Stojanovic, B., Kanjevac, T., Arsenijevic, D., Radosavljevic, G., et al. (2020). Interleukin-17 in chronic inflammatory neurological diseases. Front. Immunol. 11:947. doi: 10.3389/fimmu.2020.00947
Miura, H., Ando, Y., Noda, Y., Isobe, K., and Ozaki, N. (2011). Long-lasting effects of inescapable-predator stress on brain tryptophan metabolism and the behavior of juvenile mice. Stress Int. J. Biol. Stress 14, 262–272. doi: 10.3109/10253890.2010.541539
Miyanishi, K., Sato, A., Kihara, N., Utsunomiya, R., and Tanaka, J. (2021). Synaptic elimination by microglia and disturbed higher brain functions. Neurochem. Int. 142:104901. doi: 10.1016/j.neuint.2020.104901
Moinuddin, A., Morley, J. E., and Banks, W. A. (2000). Regional variations in the transport of interleukin-l alpha across the blood-brain barrier in ICR and aging SAMP8 mice. Neuroimmunomodulation 8, 165–170. doi: 10.1159/000054814
Molano, A., Illarionov, P. A., Besra, G. S., Putterman, C., and Porcelli, S. A. (2008). Modulation of invariant natural killer T cell cytokine responses by indoleamine 2,3-dioxygenase. Immunol. Lett. 117, 81–90. doi: 10.1016/j.imlet.2007.12.013
Molina-Holgado, E., Vela, J. M., Arévalo-Martín, A., and Guaza, C. (2001). LPS/IFN-γ cytotoxicity in oligodendroglial cells: Role of nitric oxide and protection by the anti-inflammatory cytokine IL-10. Eur. J. Neurosci. 13, 493–502. doi: 10.1046/j.0953-816x.2000.01412.x
Moncrieff, J., Cooper, R. E., Stockmann, T., Amendola, S., Hengartner, M. P., and Horowitz, M. A. (2022). The serotonin theory of depression: A systematic umbrella review of the evidence. Mol. Psychiatr. Ahead of print. doi: 10.1038/s41380-022-01661-0
Mondanelli, G., Coletti, A., Greco, F. A., Pallotta, M. T., Orabona, C., Iacono, A., et al. (2020). Positive allosteric modulation of indoleamine 2,3-dioxygenase 1 restrains neuroinflammation. Proc. Natl. Acad. Sci. U.S.A. 117, 3848–3857. doi: 10.1073/pnas.1918215117
Mondanelli, G., Mandarano, M., Belladonna, M. L., Suvieri, C., Pelliccia, C., Bellezza, G., et al. (2021). Current challenges for IDO2 as target in cancer immunotherapy. Front. Immunol. 12:679953. doi: 10.3389/fimmu.2021.679953
Morales-Puerto, N., Gimenez-Gomez, P., Perez-Hernandez, M., Abuin-Martinez, C., de Biedma-Elduayen, L. G., Vidal, R., et al. (2021). Addiction and the kynurenine pathway: A new dancing couple? Pharmacol. Therap. 223, 107807. doi: 10.1016/j.pharmthera.2021.107807
Morris, G., Berk, M., Carvalho, A., Caso, J. R., Sanz, Y., Walder, K., et al. (2017). The role of the microbial metabolites including tryptophan catabolites and short chain fatty acids in the pathophysiology of immune-inflammatory and neuroimmune disease. Mol. Neurobiol. 54, 4432–4451. doi: 10.1007/s12035-016-0004-2
Moyer, B. J., Rojas, I. Y., Kerley-Hamilton, J. S., Hazlett, H. F., Nemani, K. V., Trask, H. W., et al. (2016). Inhibition of the aryl hydrocarbon receptor prevents Western diet-induced obesity. Model for AHR activation by kynurenine via oxidized-LDL, TLR2/4, TGF beta, and IDO1. Toxicol. Appl. Pharmacol. 300, 13–24. doi: 10.1016/j.taap.2016.03.011
Mueller, N., and Schwarz, M. J. (2010). The role of the immune system in schizophrenia. Curr. Immunol. Rev. 6, 213–220.
Muller, A. J., Manfredi, M. G., Zakharia, Y., and Prendergast, G. C. (2019). Inhibiting IDO pathways to treat cancer: Lessons from the ECHO-301 trial and beyond. Semin. Immunpathol. 41, 41–48. doi: 10.1007/s00281-018-0702-0
Munn, D. H., Sharma, M. D., Lee, J. R., Jhaver, K. G., Johnson, T. S., Keskin, D. B., et al. (2002). Potential regulatory function of human dendritic cells expressing indoleamine 2,3-dioxygenase. Science 297, 1867–1870. doi: 10.1126/science.1073514
Munn, D. H., Zhou, M., Attwood, J. T., Bondarev, I., Conway, S. J., Marshall, B., et al. (1998). Prevention of allogeneic fetal rejection by tryptophan catabolism. Science 281, 1191–1193. doi: 10.1126/science.281.5380.1191
Murray, C., Griffin, E. W., O’Loughlin, E., Lyons, A., Sherwin, E., Ahmed, S., et al. (2015). Interdependent and independent roles of type I interferons and IL-6 in innate immune, neuroinflammatory and sickness behavior responses to systemic poly I:C. Brain Behav. Immun. 48, 274–286. doi: 10.1016/j.bbi.2015.04.009
Musso, T., Varesio, L., Zhang, X. Y., Rowe, T. K., Ferrara, P., Ortaldo, J. R., et al. (1994). IL-4 and IL-13 Induce LSK, a Csk-like tyrosine kinase, in human monocytes. J. Exp. Med. 180, 2383–2388. doi: 10.1084/jem.180.6.2383
Muzio, L., Martino, G., and Furlan, R. (2007). Multifaceted aspects of inflammation in multiple sclerosis: The role of microglia. J. Immunol. 191, 39–44. doi: 10.1016/j.jneuroim.2007.09.016
Nakanishi, K. (2018). Unique action of interleukin-18 on T cells and other immune cells. Front. Immunol. 9:763. doi: 10.3389/fimmu.2018.00763
Nayak, A. R., Kashyap, R. S., Kabra, D., Purohit, H. J., Taori, G. M., and Daginawala, H. F. (2012). Time course of inflammatory cytokines in acute ischemic stroke patients and their relation to inter-alfa trypsin inhibitor heavy chain 4 and outcome. Ann. Indian Acad. Neurol. 15, 181–185. doi: 10.4103/0972-2327.99707
Neale, S. A., Copeland, C. S., Uebele, V. N., Thomson, F. J., and Salt, T. E. (2013). Modulation of hippocampal synaptic transmission by the kynurenine pathway member xanthurenic acid and other VGLUT Inhibitors. Neuropsychopharmacology 38, 1060–1067. doi: 10.1038/npp.2013.4
Nenov, M. N., Malkov, A. E., Konakov, M. V., and Levin, S. G. (2019). Interleukin-10 and transforming growth factor-beta-1 facilitate long-term potentiation in CA1 region of hippocampus. Biochem. Biophys. Res. Commun. 518, 486–491. doi: 10.1016/j.bbrc.2019.08.072
Nguyen, N. T., Nakahama, T., Le, D. H., Son, L. V., Chu, H. H., and Kishimoto, T. (2014). Aryl hydrocarbon receptor and kynurenine: Recent advances in autoimmune disease research. Front. Immunol. 5:551. doi: 10.3389/fimmu.2014.00551
Nimmerjahn, A., Kirchhoff, F., and Helmchen, F. (2005). Resting microglial cells are highly dynamic surveillants of brain parenchyma in vivo. Science 308, 1314–1318. doi: 10.1126/science.1110647
Nohara, L. L., Stanwood, S. R., Omilusik, K. D., and Jefferies, W. A. (2015). Tweeters, woofers and horns: The complex orchestration of calcium currents in T lymphocytes. Front. Immunol. 6:234. doi: 10.3389/fimmu.2015.00234
Nolan, R. A., Muir, R., Runner, K., Haddad, E. K., and Gaskill, P. J. (2019). Role of macrophage dopamine receptors in mediating cytokine production: Implications for neuroinflammation in the context of HIV-associated neurocognitive disorders. J. Neuroimmun. Pharmacol. 14, 134–156. doi: 10.1007/s11481-018-9825-2
Notarangelo, F. M., Beggiato, S., and Schwarcz, R. (2019). Assessment of prenatal kynurenine metabolism using tissue slices: Focus on the neosynthesis of kynurenic acid in mice. Develop. Neurosci. 41, 102–111. doi: 10.1159/000499736
O’Connor, J. C., Lawson, M. A., Andre, C., Moreau, M., Lestage, J., Castanon, N., et al. (2009a). Lipopolysaccharide-induced depressive-like behavior is mediated by indoleamine 2,3-dioxygenase activation in mice. Mol. Psychiatry 14, 511–522. doi: 10.1038/sj.mp.4002148
O’Connor, J. C., Andre, C., Wang, Y. X., Lawson, M. A., Szegedi, S. S., Lestage, J., et al. (2009b). Interferon-gamma and tumor necrosis factor-alpha mediate the upregulation of indoleamine 2,3-dioxygenase and the induction of depressive-like behavior in mice in response to Bacillus Calmette-Guerin. J. Neurosci. 29, 4200–4209. doi: 10.1523/JNEUROSCI.5032-08.2009
Ogbechi, J., Clanchy, F. I., Huang, Y.-S., Stone, T. W., and Williams, R. O. (2020). IDO activation, inflammation and musculoskeletal disease. Exp. Gerontol. 131:110820.
Ogbechi, J., Huang, Y.-S., Clanchy, F. I. L., Pantazi, E., Topping, L. M., Darlington, L. G., et al. (2022). Modulation of immune cell function, IDO expression and kynurenine production by the quorum sensor 2-heptyl-3-hydroxy-4-quinolone (PQS). Front. Immunol. 13:1001956. doi: 10.3389/fimmu.2022.1001956
Ogyu, K., Kubo, K., Noda, Y., Iwata, Y., Tsugawa, S., Omura, Y., et al. (2018). Kynurenine pathway in depression: A systematic review and meta-analysis. Neurosci. Biobehav. Rev. 90, 16–25. doi: 10.1016/j.neubiorev.2018.03.023
Okuda, S., Nishiyama, N., Saito, H., and Katsuki, H. (1998). 3-Hydroxykynurenine, an endogenous oxidative stress generator, causes neuronal cell death with apoptotic features and region selectivity. J. Neurochem. 70, 299–307. doi: 10.1046/j.1471-4159.1998.70010299.x
Olmos, G., and Llado, J. (2014). Tumor necrosis factor alpha: A link between neuroinflammation and excitotoxicity. Mediat. Inflamm. 2014:861231. doi: 10.1155/2014/861231
Omenetti, S., and Pizarro, T. T. (2015). The Treg/Th17 axis: A dynamic balance regulated by the gut microbiome. Front. Immunol. 6:639. doi: 10.3389/fimmu.2015.00639
Opitz, C. A., Litzenburger, U. M., Sahm, F., Ott, M., Tritschler, I., Trump, S., et al. (2011). An endogenous tumour-promoting ligand of the human aryl hydrocarbon receptor. Nature 478, 197–203. doi: 10.1038/nature10491
Opitz, C. A., Patterson, L. F. S., Mohapatra, S. R., Dewi, D. L., Sadik, A., Platten, M., et al. (2020). The therapeutic potential of targeting tryptophan catabolism in cancer. Br. J. Cancer 122, 30–44. doi: 10.1038/s41416-019-0664-6
Orabona, C., Pallotta, M. T., and Grohmann, U. (2012). Different partners, opposite outcomes: A new perspective of the immunobiology of indoleamine 2,3-dioxygenase. Mol. Med. 18, 834–842. doi: 10.2119/molmed.2012.00029
Oruckaptan, H. H., Ozisik, P., Atilla, P., Tuncel, M., Kilinc, K., Geyik, P. O., et al. (2009). Systemic Administration of Interleukin-10 attenuates early ischemic response following spinal cord ischemia reperfusion injury in rats. J. Surg. Res. 155, 345–356. doi: 10.1016/j.jss.2008.07.013
Osorio-Barrios, F., Navarro, G., Campos, J., Ugalde, V., Prado, C., Raich, L., et al. (2021). The heteromeric complex formed by dopamine receptor D-5 and CCR9 leads the gut homing of CD4(+) T cells upon inflammation. Cell Mol. Gastroenterol. Hepatol. 12, 489–506. doi: 10.1016/j.jcmgh.2021.04.006
Pacheco, A. R., and Sperandio, V. (2009). Inter-kingdom signaling: Chemical language between bacteria and host. Curr. Opin. Microbiol. 12, 192–198. doi: 10.1016/j.mib.2009.01.006
Pacheco, R., Ciruela, F., Casado, V., Mallol, J., Gallart, T., Lluis, C., et al. (2004). Group I metabotropic glutamate receptors mediate a dual role of glutamate in T cell activation. J. Biol. Chem. 279, 33352–33358. doi: 10.1074/jbc.M401761200
Pae, H. O., Oh, G. S., Lee, B. S., Rim, J. S., Kim, Y. M., and Chung, H. T. (2006). 3-Hydroxy-anthranilic acid, one of L-tryptophan metabolites, inhibits monocyte chemoattractant protein-1 secretion and vascular cell adhesion molecule-1 expression via heme oxygenase-1 induction in human umbilical vein endothelial cells. Atherosclerosis 187, 274–284. doi: 10.1016/j.atherosclerosis.2005.09.010
Paetau, S., Rolova, T., Ning, L., and Gahmberg, C. G. (2017). Neuronal ICAM-5 inhibits microglia adhesion and phagocytosis and promotes an anti-inflammatory response in LPS Stimulated Microglia. Front. Mol. Neurosci. 10:431. doi: 10.3389/fnmol.2017.00431
Pallotta, M. T., Orabona, C., Volpi, C., Vacca, C., Belladonna, M. L., Bianchi, R., et al. (2011). Indoleamine 2,3- dioxygenase is a signaling protein in long-term tolerance by dendritic cells. Nat. Immunol. 12, 870–878. doi: 10.1038/ni.2077
Pan, W. H., Wu, X. J., He, Y., Hung, H. C., and Huang, E. Y. K. (2013). Brain interleukin-15 in neuroinflammation and behavior. Neurosci. Biobehav. Rev. 37, 184–192. doi: 10.1016/j.neubiorev.2012.11.009
Pan, W., Banks, W. A., and Kastin, A. J. (1998b). Permeability of the blood–brain barrier to neurotrophins. Brain Res. 788, 87–94.
Pan, W., Banks, W. A., Fasold, M. B., Bluth, J., and Kastin, A. J. (1998a). Transport of brain-derived neurotrophic factor across the blood–brain barrier. Neuropharmacology 12, 1553–1561. doi: 10.1016/S0028-3908(98)00141-5
Paolicelli, R. C., and Gross, C. T. (2011). Microglia in development: Linking brain wiring to brain environment. Neuron Glia Biol. 7, 77–83. doi: 10.1017/S1740925X12000105
Paolicelli, R. C., Bolasco, G., Pagani, F., Maggi, L., Scianni, M., Panzanelli, P., et al. (2011). Synaptic pruning by microglia is necessary for normal brain development. Science 333, 1456–1458. doi: 10.1126/science.1202529
Pastorino, S., Carta, L., Puppo, M., Melillo, G., Bosco, M. C., and Varesio, L. (2004). Picolinic acid- or desferrioxamine-inducible autocrine activation of macrophages engineered to produce IFN-γ: An approach for gene therapy. Gene Ther. 11, 560–568. doi: 10.1038/sj.gt.3302217
Patel, R. R., Wolfe, S. A., Bajo, M., Abeynaike, S., Pahng, A., and Borgonetti, V. (2021). IL-10 normalizes aberrant amygdala GABA transmission and reverses anxiety-like behavior and dependence-induced escalation of alcohol intake. Prog. Neurobiol. 199:101952. doi: 10.1016/j.pneurobio.2020.101952
Patterson, S. L. (2015). Immune dysregulation and cognitive vulnerability in the aging brain: Interactions of microglia, IL-1 beta, BDNF and synaptic plasticity. Neuropharmacology 96, 11–18. doi: 10.1016/j.neuropharm.2014.12.020
Pavlov, V. A., and Tracey, K. J. (2017). Neural regulation of immunity: Molecular mechanisms and clinical translation. Nat. Neurosci. 20, 156–166. doi: 10.1038/nn.4477
Pearson-Leary, J., Osborne, D. M., and McNay, E. C. (2016). Role of glia in stress-induced enhancement and impairment of memory. Front. Integr. Neurosci. 9:63. doi: 10.3389/fnint.2015.00063
Pedraz-Petrozzi, B., Elyamany, O., Rummel, C., and Mulert, C. (2020). Effects of inflammation on the kynurenine pathway in schizophrenia–a systematic review. J. Neuroinflamm. 17:56. doi: 10.1186/s12974-020-1721-z
Perez-Asensio, F. J., Perpiñá, U., Planas, A. M., and Pozas, E. (2013). Interleukin-10 regulates progenitor differentiation and modulates neurogenesis in adult brain. J. Cell Sci. 126, 4208–4219. doi: 10.1242/jcs.127803
Perez-De La Cruz, V., Carrillo-Mora, P., and Santamaria, A. (2012). Quinolinic acid, an endogenous molecule combining excitotoxicity, oxidative stress and other toxic mechanisms. Intern. J. Tryptophan Res. 5, 1–8. doi: 10.4137/IJTR.S8158
Perkins, M. N., and Stone, T. W. (1982). An iontophoretic investigation of the action of convulsant kynurenines and their interaction with the endogenous excitant quinolinic acid. Brain Res. 247, 184–187. doi: 10.1016/0006-8993(82)91048-4
Perkins, M. N., and Stone, T. W. (1983). The pharmacology and regional variations of quinolinic acid evoked excitations in the rat CNS. J. Pharmacol. Exp. Therap. 226, 551–557.
Perkins, M. N., and Stone, T. W. (1985). Actions of kynurenic acid and quinolinic acid in the rat hippocampus in vivo. Exp. Neurol. 88, 570–579.
Pfefferkorn, E. R. (1984). Interferon-gamma blocks the growth of toxoplasma-gondii in human-fibroblasts by inducing the host-cells to degrade tryptophan. Proc. Nat. Acad. Sci. U.S.A. 81, 908–912. doi: 10.1073/pnas.81.3.908
Pietrzak, A., Misiak-Tloczek, A., and Brzezinska-Baszczyk, E. (2009). Interleukin (IL)-10 inhibits RANTES-, tumour necrosis factor (TNF)- and nerve growth factor (NGF)-induced mast cell migratory response but is not a mast cell chemoattractant. Immunol. Lett. 123, 46–51. doi: 10.1016/j.imlet.2009.02.003
Pineda-Farias, J. B., Perez-Severiano, F., Gonzalez-Esquivel, D. F., Barragan-Iglesias, P., Bravo-Hernandez, M., Cervantes-Duran, C., et al. (2013). The l-kynurenine-probenecid combination reduces neuropathic pain in rats. Europ. J. Pain 17, 1365–1373. doi: 10.1002/j.1532-2149.2013.00305.x
Pires, A. S., Sundaram, G., Heng, B., Krishnamurthy, S., Brew, B. J., and Guillemin, G. J. (2022). Recent advances in clinical trials targeting the kynurenine pathway. Pharmacol. Therap. 236:108055. doi: 10.1016/j.pharmthera.2021.108055
Pisar, M., Forrest, C. M., Khalil, O. S., McNair, K., Vincenten, M. C. J., Qasem, S., et al. (2014). Modified neocortical and cerebellar protein expression and morphology following prenatal inhibition of the kynurenine pathway. Brain Res. 1576, 1–17. doi: 10.1016/j.brainres.2014.06.016
Platenik, J., Stopka, P., Vejrazka, M., and Stipek, S. (2001). Quinolinic acid-iron(II) complexes: Slow autoxidation, but enhanced hydroxyl radical production in the Fenton reaction. Free Radic. Res. 34, 445–459. doi: 10.1080/10715760100300391
Platten, M., Nollen, E. A. A., Rohrig, U. F., Fallarino, F., and Opitz, C. A. (2019). Tryptophan metabolism as a common therapeutic target in cancer, neurodegeneration and beyond. Nat. Rev. Drug Discov. 18, 379–401. doi: 10.1038/s41573-019-0016-5
Pocivavsek, A., Elmer, G. I., and Schwarcz, R. (2019). Inhibition of kynurenine aminotransferase II attenuates hippocampus-dependent memory deficit in adult rats treated prenatally with kynurenine. Hippocampus 29, 73–77. doi: 10.1002/hipo.23040
Podlacha, M., Glac, W., Listowska, M., Grembecka, B., Majkutewicz, I., Myslinska, D., et al. (2016). Medial septal NMDA glutamate receptors are involved in modulation of blood natural killer cell activity in rats. J. Neuroimmune Pharmacol. 11, 121–132. doi: 10.1007/s11481-015-9632-y
Poeta, V. M., Massara, M., Capucetti, A., and Bonecchi, R. (2019). Chemokines and chemokine receptors: New targets for cancer immunotherapy. Front. Immunol. 10:379. doi: 10.3389/fimmu.2019.00379
Poormasjedi-Meibod, M. S., Elizei, S. S., Leung, V., Jalili, R. B., Ko, F., and Ghahary, A. (2016). Kynurenine modulates MMP-1 and Type-I collagen expression via aryl hydrocarbon receptor activation in dermal fibroblasts. J. Cell. Physiol. 231, 2749–2760. doi: 10.1002/jcp.25383
Porro, C., Cianciulli, A., and Panaro, M. A. (2020). The regulatory role of IL-10 in neurodegenerative diseases. Biomolecules 10:1017. doi: 10.3390/biom10071017
Prendergast, G. C., Malachowski, W. P., DuHadaway, J. B., and Muller, A. J. (2017). Discovery of IDO1 inhibitors: From bench to bedside. Cancer Res. 77, 6795–6811. doi: 10.1158/0008-5472.CAN-17-2285
Prendergast, G. C., Metz, R., Muller, A. J., Merlo, L. M. F., and Mandik-Nayak, L. (2014). IDO2 in immunomodulation and autoimmune disease. Front. Immunol. 5:585. doi: 10.3389/fimmu.2014.00585
Prendergast, G. C., Mondal, A., Dey, S., Laury-Kleintop, L. D., and Muller, A. J. (2018). Inflammatory reprogramming with IDO1 inhibitors: Turning immunologically unresponsive ‘cold’ tumors ‘hot’. Trends Cancer 4, 38–58. doi: 10.1016/j.trecan.2017.11.005
Pribiag, H., and Stellwagen, D. (2013). TNF-α downregulates inhibitory neurotransmission through protein phosphatase 1-dependent trafficking of GABA(A) receptors. J. Neurosci. 33, 15879–15893. doi: 10.1523/JNEUROSCI.0530-13.2013
Proietti, E., Rossini, S., Grohmann, U., and Mondanelli, G. (2020). Polyamines and kynurenines at the intersection of immune modulation. Trends Immunol. 41, 1037–1050. doi: 10.1016/j.it.2020.09.007
Qin, Z. H., Wang, Y. M., and Chase, T. N. (2000). Quinolinic acid neurotoxicity via caspase-3 and NFκB. A caspase-3-like protease is involved in NF-kappa B activation induced by stimulation of N-methyl-D-aspartate receptors in rat striatum. Mol. Brain Res. 80, 111–122. doi: 10.1016/S0169-328X(00)00147-9
Quak, J., Doornbos, B., Roest, A. M., Duivis, H. E., Vogelzangs, N., Nolen, W. A., et al. (2014). Does tryptophan degradation along the kynurenine pathway mediate the association between pro-inflammatory immune activity and depressive symptoms? Psychoneuroendocrinology 45, 202–210. doi: 10.1016/j.psyneuen.2014.03.013
Rage, F., Silhol, M., and Tapia-Arancibia, L. (2006). IL-1 beta regulation of BDNF expression in rat cultured hypothalamic neurons depends on the presence of glial cells. Neurochem. Int. 49, 433–441. doi: 10.1016/j.neuint.2006.03.002
Raison, C. L., Dantzer, R., Kelley, K. W., Lawson, M. A., Woolwine, B. J., Vogt, G., et al. (2010). CSF concentrations of brain tryptophan and kynurenines during immune stimulation with IFN-alpha: Relationship to CNS immune responses and depression. Mol. Psychiatr. 15, 393–403. doi: 10.1038/mp.2009.116
Ransohoff, R. M., and Perry, V. H. (2009). Microglial physiology: Unique stimuli, specialized responses. Ann. Rev. Immunol. 27, 119–145. doi: 10.1146/annurev.immunol.021908.132528
Rapisarda, A., Pastorino, S., Massazza, S., Varesio, L., and Bosco, M. C. (2002). Antagonistic effect of picolinic acid and interferon-gamma on macrophage inflammatory protein-1 alpha/beta production. Cell. Immunol. 220, 70–80. doi: 10.1016/S0008-8749(03)00008-X
Reardon, C., Murray, K., and Lomax, A. E. (2018). Neuroimmune communication in health and disease. Physiol. Rev. 98, 2287–2316. doi: 10.1152/physrev.00035
Rentzos, M., and Rombos, A. (2012). The role of IL-15 in central nervous system disorders. Acta Neurol. Scand. 125, 77–82. doi: 10.1111/j.1600-0404.2011.01524.x
Resta, F., Masi, A., Sili, M., Laurino, A., Moroni, F., and Mannaioni, G. (2016). Kynurenic acid and zaprinast induce analgesia by modulating HCN channels through GPR35 activation. Neuropharmacology 108, 136–143. doi: 10.1016/j.neuropharm.2016.04.038
Rizzo, F. R., Musella, A., De Vito, F., Fresegna, D., Bullitta, S., Vanni, V., et al. (2018). Tumor necrosis factor and interleukin-1 beta modulate synaptic plasticity during neuroinflammation. Neural Plast. 2018:8430123. doi: 10.1155/2018/8430123
Robinson, C. M., Hale, P. T., and Carlin, J. M. (2005). The role of IFN-γ and TNF-α-responsive regulatory elements in the synergistic induction of indoleamine dioxygenase. J. Interferon Cytokine Res. 25, 20–30. doi: 10.1089/jir.2005.25.20
Robinson, C. M., Shirey, K. A., and Carlin, J. M. (2003). Synergistic transcriptional activation of indoleamine dioxygenase by IFN-γ and TNF-α. J Interferon Cytokine Res. 23, 413–421. doi: 10.1089/107999003322277829
Rodriguez-Gomez, J. A., Kavanagh, E., Engskog-Vlachos, P., Engskog, M. K. R., Herrera, A. J., Espinosa-Oliva, A. M., et al. (2020). Microglia: Agents of the CNS pro-inflammatory response. Cells 9:1717. doi: 10.3390/cells9071717
Roussel, G., Bessede, A., Klein, C., Maitre, M., and Mensah-Nyagan, A. G. (2016). Xanthurenic acid is localized in neurons in the central nervous system. Neuroscience 329, 226–238. doi: 10.1016/j.neuroscience.2016.05.006
Rowland, T., Perry, B. I., Upthegrove, R., Barnes, N., Chatterjee, J., Gallacher, D., et al. (2018). Neurotrophins, cytokines, oxidative stress mediators and mood state in bipolar disorder: Systematic review and meta-analyses. Br. J. Psychiatr. 213, 514–525. doi: 10.1192/bjp.2018.144
Saito, K., Crowley, J. S., Markey, S. P., and Heyes, M. P. (1993). A mechanism for increased quinolinic acid formation following acute systemic immune stimulation. J. Biol. Chem. 268, 15496–15503.
Sallmann, S., Juttler, E., Prinz, S., Petersen, N., Knopf, U., Weiser, T., et al. (2000). Induction of interleukin-6 by depolarization of neurons. J. Neurosci. 20, 8637–8642.
Sallusto, F., Impellizzieri, D., Basso, C., Laroni, A., Uccelli, A., Lanzavecchia, A., et al. (2012). T-cell trafficking in the central nervous system. Immunol. Rev. 248, 216–227. doi: 10.1111/j.1600-065X.2012.01140.x
Salvador, A. F., de Lima, K. A., and Kipnis, J. (2021). Neuromodulation by the immune system: A focus on cytokines. Nat. Revs. Immunol. 21, 526–541. doi: 10.1038/s41577-021-00508-z
Santamaria, A., Galvan-Arzate, S., Lisy, V., Ali, S. F., Duhart, H. M., Osorio-Rico, L., et al. (2001). Quinolinic acid induces oxidative stress in rat brain synaptosomes. Neuroreport 12, 871–874. doi: 10.1097/00001756-200103260-00049
Santello, M., and Volterra, A. (2012). TNF-a in synaptic function: Switching gears. Trends Neurosci. 35, 638–647. doi: 10.1016/j.tins.2012.06.001
Santello, M., Bezzi, P., and Volterra, A. (2011). TNF-α controls glutamatergic gliotransmission in the hippocampal dentate gyrus. Neuron 69, 988–1001. doi: 10.1016/j.neuron.2011.02.003
Sathyasaikumar, K. V., de la Cruz, V. P., Pineda, B., Cervantes, G. I. V., Ortega, D. R., Donley, D. W., et al. (2022). Cellular localization of kynurenine 3-monooxygenase in the brain: Challenging the dogma. Antioxidants 11:315. doi: 10.3390/antiox11020315
Sathyasaikumar, K. V., Tararina, M., Wu, H. Q., Neale, S. A., Weisz, F., Salt, T. E., et al. (2017). Xanthurenic acid formation from 3-hydroxykynurenine in the mammalian brain: Neurochemical characterization and physiological effects. Neuroscience 367, 85–97. doi: 10.1016/j.neuroscience.2017.10.006
Savina, T. A., Shchipakina, T. G., Levin, S. G., and Godukhin, O. V. (2013). Interleukin-10 prevents the hypoxia-induced decreases in expressions of AMPA receptor subunit GluA1 and alpha subunit of Ca2+/caLmodulin-dependent protein kinase II in hippocampal neurons. Neurosci. Lett. 534, 279–284. doi: 10.1016/j.neulet.2012.11.023
Sawada, M., Suzumura, A., Hosoya, H., Marunouchi, T., and Nagatsu, T. (1999). Interleukin-10 inhibits both production of cytokines and expression of cytokine receptors in microglia. J. Neurochem. 72, 1466–1471.
Sawant, K. V., Sepuru, K. M., Lowry, E., Penaranda, B., Frevert, C. W., Garofalo, R. P., et al. (2021). Neutrophil recruitment by chemokines Cxcl1/KC and Cxcl2/MIP2: Role of Cxcr2 activation and glycosaminoglycan interactions. J. Leukocyte Biol. 109, 199–229. doi: 10.1002/JLB.3A0820-207R
Scheiblich, H., Trombly, M., Ramirez, A., and Heneka, M. T. (2020). Neuroimmune connections in aging and neurodegenerative diseases. Trends Immunol. 41, 300–312. doi: 10.1016/j.it.2020.02.002
Scheller, J., Berg, A., Moll, J. M., Floss, D. M., and Jungesblut, C. (2021). Current status and relevance of single nucleotide polymorphisms in IL-6-/ IL-12-type cytokine receptors. Cytokine 148:155550. doi: 10.1016/j.cyto.2021.155550
Schlager, C., Korner, H., Krueger, M., Vidoli, S., Haberl, M., Mielke, D., et al. (2016). Effector T-cell trafficking between the leptomeninges and the cerebrospinal fluid. Nature 530, 349–350. doi: 10.1038/nature16939
Schonewille, M., Girasole, A. E., Rostaing, P., Mailhes-Hamon, C., Ayon, A., Nelson, A. B., et al. (2021). NMDARs in granule cells contribute to parallel fiber-purkinje cell synaptic plasticity and motor learning. Proc. Nat. Acad. Sci. U.S.A. 118:e2102635118. doi: 10.1073/pnas.2102635118
Schwarcz, R., Guidetti, P., Sathyasaikumar, K. V., and Muchowski, P. J. (2010). Of mice, rats and men: Revisiting the quinolinic acid hypothesis of Huntington’s disease. Prog. Neurobiol. 90, 230–245. doi: 10.1016/j.pneurobio.2009.04.005
Schwarcz, R., Whetsell, W. O., and Mangano, R. M. (1983). Quinolinic acid–an endogenous metabolites that produces axon-sparing lesions in rat brain. Science 219, 316–318. doi: 10.1126/science.6849138
Schwieler, L., Larsson, M. K., Skogh, E., Kegel, M. E., Orhan, F., Abdelmoaty, S., et al. (2015). Increased levels of IL-6 in the cerebrospinal fluid of patients with chronic schizophrenia–significance for activation of the kynurenine pathway. J. Psychiatr. Neurosci. 40, 126–133. doi: 10.1503/jpn.140126
Sekkai, D., Guittet, O., Lemaire, G., Tenu, J. P., and Lepoivre, M. (1997). Inhibition of nitric oxide synthase expression and activity in macrophages by 3-hydroxyanthranilic acid, a tryptophan metabolite. Arch. Biochem. Biophys. 340, 117–123. doi: 10.1006/abbi.1997.9913
Seminotti, B., Amaral, A. U., Ribeiro, R. T., Rodrigues, M. D. N., Colin-Gonzalez, A. L., Leipnitz, G., et al. (2016). Oxidative stress, disrupted energy metabolism, and altered signaling pathways in glutaryl-coa dehydrogenase knockout mice: Potential implications of quinolinic acid toxicity in the neuropathology of glutaric acidemia Type I. Mol. Neurobiol. 53, 6459–6475. doi: 10.1007/s12035-015-9548-9
Serafini, G., Parisi, V. M., Aguglia, A., Amerio, A., Sampogna, G., Fiorillo, A., et al. (2020). A specific inflammatory profile underlying suicide risk? systematic review of the main literature findings. Intern. J. Environ. Res. Public Health 17:2393. doi: 10.3390/ijerph17072393
Shaftel, S. S., Carlson, T. J., Olschowka, J. A., Kyrkanides, S., Matousek, S. B., and O’Banion, M. K. (2007). Chronic interleukin-1 beta expression in mouse brain leads to leukocyte infiltration and neutrophil-independent blood brain barrier permeability without overt neurodegeneration. J. Neurosci. 27, 9301–9309. doi: 10.1523/JNEUROSCI.1418-07.2007
Shamsi, M. M., Hassan, Z. M., Quinn, L. S., Gharakhanlou, R., Baghersad, L., and Mahdavi, M. (2015). Time course of IL-15 expression after acute resistance exercise in trained rats: Effect of diabetes and skeletal muscle phenotype. Endocrine 49, 396–403. doi: 10.1007/s12020-014-0501-x
Shim, H. G., Jang, S. S., Kim, S. H., Hwang, E. M., Min, J. O., Kim, H. Y., et al. (2018). TNF-α increases the intrinsic excitability of cerebellar purkinje cells through elevating glutamate release in Bergmann Glia. Sci. Rep. 8:11589. doi: 10.1038/s41598-018-29786-9
Silva-Islas, C. A., Santana-Martinez, R. A., Leon-Contreras, J. C., Barrera-Oviedo, D., Pedraza-Chaverri, J., Hernandez-Pando, R., et al. (2022). Quinolinic acid induces alterations in neuronal subcellular compartments, blocks autophagy flux and activates necroptosis and apoptosis in rat striatum. Mol. Neurobiol. 59, 6632–6651. doi: 10.1007/s12035-022-02986-1
Sinclair, L. V., Neyens, D., Ramsay, G., Taylor, P. M., and Cantrell, D. A. (2018). Single cell analysis of kynurenine and system L amino acid transport in T cells. Nat. Commun. 9:1981. doi: 10.1038/S41467-018-04366-7
Skundric, D. S., Cruikshank, W. W., Montgomery, P. C., Lisak, R. P., and Tse, H. Y. (2015). Emerging role of IL-16 in cytokine-mediated regulation of multiple sclerosis. Cytokine 75, 234–248. doi: 10.1016/j.cyto.2015.01.005
Smith, J. R., Jamie, J. F., and Guillemin, G. J. (2016). Kynurenine-3-monooxygenase: A review of structure, mechanism, and inhibitors. Drug Discov. 21, 315–324. doi: 10.1016/j.drudis.2015.11.001
Spichak, S., Bastiaanssen, T. F. S., Berding, K., Vlckova, K., Clarke, G., Dinan, T. G., et al. (2021). Mining microbes for mental health: Determining the role of microbial metabolic pathways in human brain health and disease. Neurosci. Biobehav. Rev. 125, 698–761. doi: 10.1016/j.neubiorev.2021.02.044
Spooren, A., Kolmus, K., Laureys, G., Clinckers, R., De Keyser, J., Haegeman, G., et al. (2011). Interleukin-6, a mental cytokine. Brain Res. Rev. 67, 157–183. doi: 10.1016/j.brainresrev.2011.01.002
Spulber, S., and Schultzberg, M. (2010). Connection between inflammatory processes and transmitter function-modulatory effects of interleukin-1. Prog. Neurobiol. 90, 256–262. doi: 10.1016/j.pneurobio.2009.10.015
Spulber, S., Bartfai, T., Winblad, B., and Schultzberg, M. (2011). Morphological and behavioral changes induced by transgenic overexpression of interleukin-1ra in the brain. J. Neurosci. Res. 89, 142–152. doi: 10.1002/jnr.22534
Stellwagen, D., Beattie, E. C., Seo, J. Y., and Malenka, R. C. (2005). Differential regulation of AMPA receptor and GABA receptor trafficking by tumor necrosis factor-alpha. J. Neurosci. 25, 3219–3228. doi: 10.1523/JNEUROSCI.4486-04.2005
Sternberg, E. M. (2006). Neural regulation of innate immunity: A coordinated nonspecific host response to pathogens. Nat. Rev. Immunol. 6, 318–328. doi: 10.1038/nri1810
Stockinger, B., Di Meglio, P., Gialitakis, M., and Duarte, J. H. (2014). The aryl hydrocarbon receptor: Multitasking in the immune system. Ann. Rev. Immunol. 32, 403–432. doi: 10.1146/annurev-immunol-032713-120245
Stone, T. W. (1993). The neuropharmacology of quinolinic and kynurenic acids. Pharmacol. Rev. 45, 309–379.
Stone, T. W. (2000a). The development and therapeutic potential of kynurenic acid and kynurenine derivatives for CNS neuroprotection. Trends Pharmacol. Sci. 21, 149–154. doi: 10.1111/cns.13768
Stone, T. W. (2020). Does kynurenic acid act on nicotinic receptors? An assessment of the evidence. J. Neurochem. 152, 627–649. doi: 10.1111/jnc.14907
Stone, T. W. (2021). Relationships and interactions between ionotropic glutamate receptors and nicotinic receptors in the CNS neuroscience. Neuroscience 468, 321–365. doi: 10.1016/j.neuroscience.2021.06.007
Stone, T. W., and Darlington, L. G. (2002). Endogenous kynurenines as targets for drug discovery and development. Nat. Rev. Drug Discov. 1, 609–620. doi: 10.1038/nrd870
Stone, T. W., and Darlington, L. G. (2013). The kynurenine pathway as a therapeutic target in cognitive and neurodegenerative disorders. Br. J. Pharmacol. 169, 1211–1227. doi: 10.1111/bph.12230
Stone, T. W., and Perkins, M. N. (1981). Quinolinic acid: A potent endogenous excitant at amino acid receptors in CNS. Eur. J. Pharmacol. 72, 411–412. doi: 10.1016/0014-2999(81)90587-2
Stone, T. W., Ceruti, S., and Abbracchio, M. P. (2009). “Adenosine receptors and neurological disease: Neuroprotection and neurodegeneration,” in Adenosine receptors in health and disease, Vol. 193, eds C. N. Wilson and S. J. Mustafa (Berlin: Springer-Verlag), 535–587. doi: 10.1007/978-3-540-89615-917
Stone, T. W., Forrest, C. M., Mackay, G. M., Stoy, N., and Darlington, L. G. (2007). Tryptophan, adenosine, neurodegeneration and neuroprotection. Metab. Brain Dis. 22, 337–352. doi: 10.1007/s11011-007-9064-3
Stone, T. W., Forrest, C. M., Stoy, N., and Darlington, L. G. (2012). Involvement of kynurenines in Huntington’s disease and stroke-induced brain damage. J. Neural Transm. 119, 261–274. doi: 10.1007/s00702-011-0676-8
Stone, T. W., McPherson, M., and Darlington, L. G. (2018). Obesity and cancer: Existing and new hypotheses for a causal connection. EBioMedicine. 30, 14–28. doi: 10.1016/j.ebiom.2018.02.022
Storelli, E., Cassina, N., Rasini, E., Marino, F., and Cosentino, M. (2019). Do Th17 Lymphocytes and IL-17 contribute to Parkinson’s disease? A systematic review of available evidence. Front. Neurol. 10:13. doi: 10.3389/fneur.2019.00013
Streit, W. J., Hurley, S. D., McGraw, T. S., and Semple-Rowland, S. L. (2000). Comparative evaluation of cytokine profiles and reactive gliosis supports a critical role for interleukin-6 in neuron-glia signaling during regeneration. J. Neurosci. Res. 61, 10–20. doi: 10.1002/1097-4547(20000701)61:1<10::AID-JNR2<3.0.CO;2-E
Strle, K., Zhou, J. H., Shen, W. H., Broussard, S. R., Johnson, R. W., Freund, G. G., et al. (2001). Interleukin-10 in the brain. Crit. Rev. Immunol. 21, 427–449.
Su, X., Gao, Y., and Yang, R. (2022). Gut microbiota-derived tryptophan metabolites maintain gut and systemic homeostasis. Cells 11:2296. doi: 10.3390/cells11152296
Sublette, M. E., Galfalvy, H. C., Fuchs, D., Lapidus, M., Grunebaum, M. F., Oquendo, M. A., et al. (2011). Plasma kynurenine levels are elevated in suicide attempters with major depressive disorder. Brain Behav. Immun. 25, 1272–1278. doi: 10.1016/j.bbi.2011.05.002
Sugama, S., and Conti, B. (2008). Interleukin-18 and stress. Brain Res. Rev. 58, 85–95. doi: 10.1016/j.brainresrev.2007.11.003
Sugama, S., Takenouchi, T., Sekiyama, K., Kitani, H., and Hashimoto, M. (2011). Immunological responses of astroglia in the rat brain under acute stress: Interleukin-1β co-localized in astroglia. Neuroscience 192, 429–437. doi: 10.1016/j.neuroscience.2011.06.051
Sugino, H., Futamura, T., Mitsumoto, Y., Maeda, K., and Marunaka, Y. (2009). Atypical antipsychotics suppress production of proinflammatory cytokines and up-regulate interleukin-10 in lipopolysaccharide-treated mice. Progr. Neuro Psychopharmacol. Biol. Psychiatr. 33, 303–307. doi: 10.1016/j.pnpbp.2008.12.006
Sundaram, G., Brew, B. J., Jones, S. P., Adams, S., Lim, C. K., and Guillemin, G. J. (2014). Quinolinic acid toxicity on oligodendroglial cells: Relevance for multiple sclerosis and therapeutic strategies. J. Neuroinflamm. 11:204. doi: 10.1186/s12974-014-0204-5
Swardfager, W., Winer, D. A., Herrmann, N., Winer, S., and Lanctot, K. L. (2013). Interleukin-17 in post-stroke neurodegeneration. Neurosci. Biobehav. Rev. 37, 436–447. doi: 10.1016/j.neubiorev.2013.01.021
Swartz, K. J., During, M. J., Freese, A., and Beal, M. F. (1990). Cerebral synthesis and release of kynurenic acid–an endogenous antagonist of excitatory amino-acid receptors. J. Neurosci. 10, 2965–2973.
Syed, A., Alamdar, S., He, L., Shi, Y., and Mahmood, S. (2021). Elevated levels ofIL-18 associated with schizophrenia and first episode psychosis: A systematic review and meta-analysis. Early Interv. Psychiatr. 15, 896–905. doi: 10.1111/eip.13031
Takemiya, T., Fumizawa, K., Yamagata, K., Iwakura, Y., and Kawakami, M. (2017). Brain Interleukin-1 facilitates of a water maze spatial memory task in young mice. Front. Neurosci. 11:202. doi: 10.3389/fnbeh.2017.00202
Talbot, S., Foster, S. L., and Woolf, C. J. (2016). Neuroimmunity: Physiology and pathology. Ann. Rev. Immunol. 34, 421–447. doi: 10.1146/annurev-immunol-041015-055340
Talhada, D., Rabenstein, M., and Ruscher, K. (2018). The role of dopaminergic immune cell signalling in poststroke inflammation. Ther. Adv. Neurol. Dis. 11, 1–11. doi: 10.1177/1756286418774225
Tambuyzer, B. R., Ponsaerts, P., and Nouwen, E. J. (2009). Microglia: Gatekeepers of central nervous system immunology. J Leukocyte Biol. 85, 352–370. doi: 10.1189/jlb.0608385
Terness, P., Bauer, T. M., Rose, L., Dufter, C., Watzlik, A., Simon, H., et al. (2002). Inhibition of allogeneic T cell proliferation by indoleamine 2,3-dioxygenase-expressing dendritic cells: Mediation of suppression by tryptophan metabolites. J. Exp. Med. 196, 447–457. doi: 10.1084/jem.20020052
Thevanavakkam, M. A., Schwarcz, R., Muchowski, P. J., and Giorgini, F. (2010). Targeting kynurenine 3-monooxygenase (KMO): Implications for therapy in Huntington’s disease. CNS Neurol. Disord. Drug Targets 9, 791–800.
Thompson, C. D., Zurko, J. C., Hanna, B. F., Hellenbrand, D. J., and Hanna, A. (2013). The therapeutic role of interleukin-10 after spinal cord injury. J. Neurotrauma 39, 1311–1324. doi: 10.1089/neu.2012.2651
Tian, R., Hou, G. L., Li, D., and Yuan, T. F. (2014). A possible change process of inflammatory cytokines in the prolonged chronic stress and its ultimate implications for health. Sci. World J. 2014:780616. doi: 10.1155/2014/780616
Tiszlavicz, Z., Németh, B., Fülöp, F., Vécsei, L., Tápai, K., Ocsovszky, I., et al. (2011). Different inhibitory effects of kynurenic acid and a novel kynurenic acid analogue on tumour necrosis factor-α (TNF-α) production by mononuclear cells, HMGB1 production by monocytes and HNP1-3 secretion by neutrophils. Arch. Pharmacol. 383, 447–455. doi: 10.1007/s00210-011-0605-2
Tong, L., Prieto, G. A., Kramar, E. A., Smith, E. D., Cribbs, D. H., Lynch, G., et al. (2012). Brain-derived neurotrophic factor-dependent synaptic plasticity is suppressed by interleukin-1 beta via p38 mitogen-activated protein kinase. J. Neurosci. 32, 17714–17724. doi: 10.1523/JNEUROSCI.1253-12.2012
Too, L. K., Li, K. M., Suarna, C., Maghzal, G. J., Stocker, R., McGregor, I. S., et al. (2016). Deletion of TDO2, IDO-1 and IDO-2 differentially affects mouse behavior and cognitive function. Behav. Brain Res. 312, 102–117. doi: 10.1016/j.bbr.2016.06.018
Traynelis, S. F., Wollmuth, L. P., McBain, C. J., Menniti, F., Vance, K. M., Ogden, K. K., et al. (2010). Glutamate receptor ion channels: Structure, regulation, and function. Pharmacol. Rev. 62, 405–496. doi: 10.1124/pr.109.002451
Trettel, F., Di Castro, M. A., and Limatola, C. (2020). Chemokines: Key molecules that orchestrate communication among neurons, microglia and astrocytes to preserve brain function. Neuroscience 439, 230–240. doi: 10.1016/j.neuroscience.2019.07.035
Turski, W. A., Gramsbergen, J. B., Traitler, H., and Schwarcz, R. (1989). Rat brain slices produce and liberate kynurenic acid upon exposure to L-kynurenine. J. Neurochem. 52, 1629–1636. doi: 10.1111/j.1471-4159.1989.tb09218.x
Ubogu, E. E., Cossoy, M. B., and Ransohoff, R. M. (2006). The expression and function of chemokines involved in CNS inflammation. Trends Pharmacol. Sci. 27, 48–55. doi: 10.1016/j.tips.2005.11.002
Uchida, T., Kinoshita, M., Fukasawa, M., Habu, Y., Shinomiya, N., and Seki, S. (2007). IL-18 time-dependently modulates Th1/Th2 cytokine production by ligand-activated NKT cells. Eur. J. Pharmacol. 37, 966–977. doi: 10.1002/eji.200636465
Upthegrove, R., and Khandaker, G. M. (2020). Cytokines, oxidative stress and cellular markers of inflammation in schizophrenia. Curr. Topics Behav. Neurosci. 44, 49–66. doi: 10.1007/7854_2018_88
Uriarte, M., De Francesco, P. N., Fernandez, G., Castrogiovanni, D., D’Arcangelo, M., Imbernon, M., et al. (2021). Circulating ghrelin crosses the blood-cerebrospinal fluid barrier via growth hormone secretagogue receptor dependent and independent mechanisms. Mol. Cell. Endocrinol. 538:111449. doi: 10.1016/j.mce.2021.111449
van Meegeren, M. E. R., Roosendaal, G., van Veghel, K., Mastbergen, S. C., and Lafeber, F. P. J. G. (2013). A short time window to profit from protection of blood-induced cartilage damage by IL-4 plus IL-10. Rheumatology 52, 1563–1571. doi: 10.1093/rheumatology/ket005
Varesio, L., Clayton, M., Blasi, E., Ruffman, R., and Radzioch, D. (1990). Picolinic acid, a catabolite of tryptophan, as the second signal in the activation of IFN-γ-primed macrophages. J. Immunol. 145, 4265–4271.
Varga, D., Heredi, J., Kanvasi, Z., Ruszka, M., Kis, Z., Ono, E., et al. (2015). Systemic L-kynurenine sulfate administration disrupts object recognition memory, alters open field behavior and decreases c-Fos immunopositivity in C57BI/6 mice. Front. Behav. Neurosci. 9:00157. doi: 10.3389/fnbeh.2015.00157
Vecsei, L., Szalardy, L., Fulop, F., and Toldi, J. (2013). Kynurenines in the CNS: Recent advances and new questions. Nat. Rev. Drug Discov. 12, 64–82. doi: 10.1038/nrd3793
Viviani, B., Gardoni, F., Bartesaghi, S., Corsini, E., Facchi, A., Galli, C. L., et al. (2006). Interleukin-1 beta released by gp120 drives neural death through tyrosine phosphorylation and trafficking of NMDA receptors. J. Biol. Chem. 281, 30212–30222. doi: 10.1074/jbc.M602156200
Wagenmann, M., Schumacher, L., and Bachert, C. (2005). The time course of the bilateral release of cytokines and mediators after unilateral nasal allergen challenge. Allergy 60, 1132–1138. doi: 10.1111/j.1398-9995.2005.00867.x
Waguespack, P. J., Banks, W. A., and Kastin, A. J. (1994). Interleukin-2 does not cross the blood-brain-barrier by a saturable transport-system. Brain Res. Bull. 34, 103–109. doi: 10.1016/0361-9230(94)90005-1
Waisman, A., Hauptmann, J., and Regen, T. (2015). The role of IL-17 in CNS diseases. Acta Neuropathol. 129, 625–637. doi: 10.1007/s00401-015-1402-7
Walczak, K., Langner, E., Makuch-Kocka, A., Szelest, M., Szalast, K., Marciniak, S., et al. (2020a). Effect of tryptophan-derived AHR ligands, kynurenine, kynurenic acid and FICZ, on proliferation, cell cycle regulation and cell death of melanoma cells-in vitro studies. Int. J. Mol. Sci. 21:AR7946. doi: 10.3390/ijms21217946
Walczak, K., Wnorowski, A., Turski, W. A., and Plech, T. (2020b). Kynurenic acid and cancer : Facts and controversies. Cell. Mol. Life Sci. 77, 1531–1550. doi: 10.1007/s00018-019-03332-w
Wang, J., Lai, S., Li, G., Zhou, T., Wang, B., Cao, F., et al. (2020). Microglial activation contributes to depressive-like behavior in dopamine D3 receptor knockout mice. Brain Behav. Immunol. 83, 226–238. doi: 10.1016/j.bbi.2019.10.016
Wang, J., Simonavicius, N., Wu, X., Swaminath, G., Reagan, J., Tian, H., et al. (2006). Kynurenic acid as a ligand for orphan G protein-coupled receptor GPR35. J. Biol. Chem. 281, 22021–22028. doi: 10.1074/jbc.M603503200
Wang, K. Z., Ye, L., Lu, H. F., Chen, H. L., Zhang, Y. Y., Huang, Y. L., et al. (2017). TNF-α promotes extracellular vesicle release in mouse astrocytes through glutaminase. J. Neuroinflamm. 14:87. doi: 10.1186/s12974-017-0853-2
Watanabe, Y., Nakayama, T., Nagakubo, D., Hieshima, K., Jin, Z., Katou, F., et al. (2006). Dopamine selectively induces migration and homing of naive CD8(+) T cells via dopamine receptor D3. J. Immunol. 176, 848–856. doi: 10.4049/jimmunol.176.2.848
Watts, S. W., Shaw, S., Burnett, R., and Dorrance, A. M. (2011). Indoleamine 2,3-dioxygenase in periaortic fat: Mechanisms of inhibition of contraction. Am. J. Physiol. Heart Circ. Physiol. 301, H1236–H1247. doi: 10.1152/ajpheart.00384.2011
Weaver-Mikaere, L., Gunn, A. J., Mitchell, M. D., Bennet, L., and Fraser, M. (2013). LPS and TNF-α modulate AMPA/NMDA receptor subunit expression and induce PGE2 and glutamate release in preterm fetal ovine mixed glial cultures. J. Neuroinflamm. 10:153. doi: 10.1186/1742-2094-10-153
Weber, W. P., Feder-Mengus, C., Chiarugi, A., Rosenthal, R., Reschner, A., Schumacher, R., et al. (2006). Differential effects of the tryptophan metabolite 3-hydroxyanthranilic acid on the proliferation of human CD8(+) T cells induced by TCR triggering or homeostatic cytokines. Eur. J. Immunol. 36, 296–304. doi: 10.1002/eji.200535616
Werneburg, S., Feinberg, P. A., Johnson, K. M., and Schafer, D. P. (2017). A microglia-cytokine axis to modulate synaptic connectivity and function. Curr. Opin. Neurobiol. 47, 138–145. doi: 10.1016/j.conb.2017.10.002
Whetsell, W. O., and Schwarcz, R. (1989). Prolonged exposure to submicromolar concentrations of quinolinic acid causes excitotoxic damage in organotypic cultures of rat corticostriatal system. Neurosci. Lett. 97, 271–275. doi: 10.1016/0304-3940(89)90609-5
Whilding, L. M., Halim, L., Draper, B., Parente-Pereira, A. C., Zabinski, T., Davies, D. M., et al. (2019). CAR T-cells targeting the integrin alpha v beta 6 and co-expressing the chemokine receptor CXCR2 demonstrate enhanced homing and efficacy against several solid malignancies. Cancers 11:674. doi: 10.3390/cancers11050674
Wilson, S. E. (2021). Interleukin-1 and transforming growth factor beta: Commonly opposing, but sometimes supporting, master regulators of the corneal wound healing response to injury. Invest. Ophthalmol. Vis. Sci. 62:8. doi: 10.1167/iovs.62.4.8
Wirthgen, E., Hoeflich, A., Rebl, A., and Guenther, J. (2018). Kynurenic acid: The janus-faced role of an immunomodulatory tryptophan metabolite and its link to pathological conditions. Front. Immunol. 8:1957. doi: 10.3389/fimmu.2017.01957
Witt, J., Barisic, S., Sawodny, O., Ederer, M., Kulms, D., and Sauter, T. (2011). Modeling time delay in the NF kappa B signaling pathway following low dose IL-1 stimulation. Eurasip J. Bioinform. Syst. Biol. 2011:3. doi: 10.1186/1687-4153-2011-3
Wojkowska, D. V., Szpakowski, P., and Glabinski, A. (2017). Interleukin 17A promotes lymphocytes adhesion and induces CCL2 and CXCL1 release from brain endothelial cells. Int. J. Molec. Sci. 18:1000. doi: 10.3390/ijms18051000
Wolf, S. A., Steiner, B., Akpinarli, A., Kammertoens, T., Nassenstein, C., Braun, A., et al. (2009). CD4-positive T lymphocytes provide a neuroimmunological link in the control of adult hippocampal neurogenesis. J. Immunol. 182, 3979–3984. doi: 10.4049/jimmunol.0801218
Wonodi, I., Stine, O. C., Sathyasaikumar, K. V., Roberts, R. C., Mitchell, B. D., Hong, L. E., et al. (2011). Downregulated kynurenine 3-monooxygenase gene expression and enzyme activity in schizophrenia and genetic association with schizophrenia endophenotypes. Arch. Gen. Psychiatr. 68, 665–674. doi: 10.1001/archgenpsychiatry.2011.71
Woods, C., Marques-Lopes, J., Controreggi, N. H., Milner, T. A., Pickel, V. M., Wang, G., et al. (2021). TNF-α Receptor Type 1 activation in the hypothalamic paraventricular nucleus contributes to glutamate signalling and angiotensin II-dependent hypertension. J. Neurosci. 41, 1349–1362. doi: 10.1523/jneurosci.2360-19.2020
Woolf, C. J., and Salter, M. W. (2000). Neuroscience–neuronal plasticity: Increasing the gain in pain. Science 288, 1765–1768. doi: 10.1126/science.288.5472.1765
Wyant, G. A., Yu, W., Doulamis, I. P., Nomoto, R. S., Saeed, M. Y., Duignan, T., et al. (2022). Mitochondrial remodeling and ischemic protection by G protein-coupled receptor 35 agonists. Science 377, 621–629. doi: 10.1126/science.abm1638
Xie, F. T., Cao, J. S., Zhao, J., Yu, Y., Qi, F., and Dai, X. C. (2015). IDO expressing dendritic cells suppress allograft rejection of small bowel transplantation in mice by expansion of Foxp3(+) regulatory T cells. Transplant. Immunol. 33, 69–77. doi: 10.1016/j.trim.2015.05.003
Xiong, L. F., Dean, J. W., Fu, Z., Oliff, K. N., Bostick, J. W., Ye, J., et al. (2020). AHR-Foxp3-ROR gamma t axis controls gut homing of CD4(+) T cells by regulating GPR15. Sci. Immunol. 5:eaaz7277. doi: 10.1126/sciimmunol.aaz7277
Xu, J. T., Xin, W. J., Zang, Y., Wu, C. Y., and Liu, X. G. (2006). The role of tumor necrosis factor-alpha in the neuropathic pain induced by Lumbar 5 ventral root transection in rat. Pain 123, 306–321. doi: 10.1016/j.pain.2006.03.011
Xu, Z. Z., Zhang, L., Liu, T., Park, J. Y., Berta, T., Yang, R., et al. (2010). Resolvins RvE1 and RvD1 attenuate inflammatory pain via central and peripheral actions. Nat. Med. 16, 592–597. doi: 10.1038/nm.2123
Yamada, A., Akimoto, H., Kagawa, S., Guillemin, G. J., and Takikawa, O. (2009). Proinflammatory cytokine interferon-gamma increases induction of indoleamine 2,3-dioxygenase in monocytic cells primed with amyloid beta peptide 1-42: Implications for the pathogenesis of Alzheimer’s disease. J. Neurochem. 110, 791–800. doi: 10.1111/j.1471-4159.2009.06175.x
Yan, J., Han, V. X., Heng, B., Guillemin, G. J., and Bandodkar, S. (2022). Development of a translational inflammation panel for the quantification of cerebrospinal fluid pterin, tryptophan-kynurenine and nitric oxide pathway metabolites. EBiomedicine 77:103917. doi: 10.1016/j.ebiom.2022.103917
Yang, J., Jiang, Z., Fitzgerald, D. C., Ma, C., Yu, S., Li, H., et al. (2009). Adult neural stem cells expressing IL-10 confer potent immunomodulation and remyelination in experimental autoimmune encephalitis. J. Clin. Invest. 119, 3678–3691. doi: 10.1172/JCI37914
Yang, L., Lindholm, K., Konishi, Y., Li, R., and Shen, Y. (2002). Target depletion of distinct tumor necrosis factor receptor subtypes reveals hippocampal neuron death and survival through different signal transduction pathways. J. Neurosci. 22, 3025–3032. doi: 10.1523/JNEUROSCI.22-08-03025.2002
Yang, M. J., Li, S., Yang, C. S., Wang, X. J., Chang, S. M., and Sun, G. X. (2017). Dynamic alterations of the levels of tumor necrosis factor-alpha, interleukin-6, and interleukin-1 beta in rat primary motor cortex during transhemispheric functional reorganization after contralateral seventh cervical spinal nerve root transfer following brachial plexus avulsion injuries. Neuroreport 28, 279–284. doi: 10.1097/WNR.0000000000000743
Yanik, B. M., Dauch, J. R., and Cheng, H. T. (2020). Interleukin-10 reduces neurogenic Inflammation and pain behavior in a mouse model of type 2 diabetes. J. Pain Res. 13, 3499–3512. doi: 10.2147/JPR.S264136
Yasui, H., Takai, K., Yoshida, R., and Hayaishi, O. (1986). Interferon enhances tryptophan metabolism by inducing pulmonary indoleamine 2,3-dioxygenase: Its possible occurrence in cancer patients. Proc. Natl. Acad. Sci. U.S.A. 83, 6622–6626. doi: 10.1073/pnas.83.17.6622
Ye, L., Huang, Y. L., Zhao, L. X., Li, Y. J., Sun, L. J., Zhou, Y., et al. (2013). IL-1 and TNF- induce neurotoxicity through glutamate production: A potential role for neuronal glutaminase. J. Neurochem. 125, 897–908. doi: 10.1111/jnc.12263
Yeung, A. W. S., Terentis, A. C., King, N. J. C., and Thomas, S. R. (2015). Role of indoleamine 2,3-dioxygenase in health and disease. Clin. Sci. 129, 601–672. doi: 10.1042/CS20140392
Yin, W. Y., Gallagher, N. R., Sawicki, C. M., McKim, D. B., Godbout, J. P., and Sheridan, J. F. (2019). Repeated social defeat in female mice induces anxiety-like behavior associated with enhanced myelopoiesis and increased monocyte accumulation in the brain. Brain Behav. Immunol. 78, 131–142. doi: 10.1016/j.bbi.2019.01.015
Yoshida, R., Imanishi, J., Oku, T., Kishida, T., and Hayaishi, O. (1981). Induction of pulmonary indoleamine 2,3-dioxygenase by interferon. Proc. Nat. Acad. Sci. U.S.A. 78, 129–132. doi: 10.1073/pnas.78.1.129
Youn, D. H., Wang, H., and Jeong, S. J. (2008). Exogenous tumor necrosis factor alpha rapidly alters synaptic and sensory transmission in the adult rat spinal cord dorsal horn. J. Neurosci. Res. 86, 2867–2875. doi: 10.1002/jnr.21726
Younger, D., Murugan, M., Rao, K. V. R., Wu, L. J., and Chandra, N. (2019). Microglia receptors in animal models of traumatic brain injury. Mol. Neurobiol. 56, 5202–5228. doi: 10.1007/s12035-018-1428-7
Zadori, D., Veres, G., Szalardy, L., Klivenyi, P., Fulop, F., Toldi, J., et al. (2016). Inhibitors of the kynurenine pathway as neurotherapeutics: A patent review 2012-2015. Expert Opin. Therap. Patents 26, 815–832. doi: 10.1080/13543776.2016.1189531
Zhang, L., Berta, T., Xu, Z. Z., Liu, T., Park, J. Y., and Ji, R. R. (2011). TNF-α contributes to spinal cord synaptic plasticity and inflammatory pain: Distinct role of TNF receptor subtypes 1 and 2. Pain 15, 419–427. doi: 10.1016/j.pain.2010.11.014
Zhang, Q., Liao, Y., Liu, Z., Dai, Y., Li, Y., Li, Y., et al. (2021). Interleukin-17 and ischaemic stroke. Immunology 162, 179–193. doi: 10.1111/imm.13265
Zhang, Q., Sun, Y., He, Z., Xu, Y., Li, X., Ding, J., et al. (2020). Kynurenine regulates NLRP2 inflammasome in astrocytes and its implications in depression. Brain Behav. Immunol. 88, 471–481. doi: 10.1016/j.bbi.2020.04.016
Zhang, Y. J., Wang, Y. C., Wu, G. F., Zhang, W., Wang, X. J., Cai, W. X., et al. (2018). Prolonged skin grafts survival time by IFN-γ in allogeneic skin transplantation model during acute rejection through IFN-γ/STAT3/IDO pathway in epidermal layer. Biochem. Biophys. Res. Commun. 496, 436–442. doi: 10.1016/j.bbrc.2017.12.152
Zhou, Z., Peng, X., Insolera, R., Fink, D. J., and Mata, M. (2009). Interleukin-10 provides direct trophic support to neurons. J. Neurochem. 110, 1617–1627. doi: 10.1111/j.1471-4159.2009.06263.x
Keywords: neuroimmune interface, kynurenine, kynurenic acid, quinolinic acid, cytokines, immune-neural communication, glutamate, homing
Citation: Stone TW, Clanchy FIL, Huang Y-S, Chiang N-Y, Darlington LG and Williams RO (2022) An integrated cytokine and kynurenine network as the basis of neuroimmune communication. Front. Neurosci. 16:1002004. doi: 10.3389/fnins.2022.1002004
Received: 24 July 2022; Accepted: 31 October 2022;
Published: 24 November 2022.
Edited by:
Stefano Comai, University of Padua, ItalyReviewed by:
Chai K. Lim, Macquarie University, AustraliaJohanna M. Gostner, Medical University of Innsbruck, Austria
Copyright © 2022 Stone, Clanchy, Huang, Chiang, Darlington and Williams. This is an open-access article distributed under the terms of the Creative Commons Attribution License (CC BY). The use, distribution or reproduction in other forums is permitted, provided the original author(s) and the copyright owner(s) are credited and that the original publication in this journal is cited, in accordance with accepted academic practice. No use, distribution or reproduction is permitted which does not comply with these terms.
*Correspondence: Trevor W. Stone, Trevor.Stone@kennedy.ox.ac.uk
†ORCID: Trevor W. Stone, orcid.org/0000-0002-5532-0031; Yi-Shu Huang, orcid.org/0000-0001-8770-9298; Richard O. Williams, orcid.org/0000-0001-5473-5270