Neurosensory development of the four brainstem-projecting sensory systems and their integration in the telencephalon
- 1Department of Biology, The University of Iowa, Iowa City, IA, United States
- 2Department of Otolaryngology, The University of Iowa, Iowa City, IA, United States
- 3Department of Physiology and Cell Biology, School of Medicine, University of Nevada, Reno, Reno, NV, United States
Somatosensory, taste, vestibular, and auditory information is first processed in the brainstem. From the brainstem, the respective information is relayed to specific regions within the cortex, where these inputs are further processed and integrated with other sensory systems to provide a comprehensive sensory experience. We provide the organization, genetics, and various neuronal connections of four sensory systems: trigeminal, taste, vestibular, and auditory systems. The development of trigeminal fibers is comparable to many sensory systems, for they project mostly contralaterally from the brainstem or spinal cord to the telencephalon. Taste bud information is primarily projected ipsilaterally through the thalamus to reach the insula. The vestibular fibers develop bilateral connections that eventually reach multiple areas of the cortex to provide a complex map. The auditory fibers project in a tonotopic contour to the auditory cortex. The spatial and tonotopic organization of trigeminal and auditory neuron projections are distinct from the taste and vestibular systems. The individual sensory projections within the cortex provide multi-sensory integration in the telencephalon that depends on context-dependent tertiary connections to integrate other cortical sensory systems across the four modalities.
Introduction
Across vertebrates, the brainstem contains discrete nuclei which receive input from distinct peripheral sensory neurons. Neurons from these brainstem nuclei project to higher-order nuclei, permitting the relay of the specific sensory signal to unique areas of the telencephalon, where integration across sensory systems occurs. The telencephalon, or forebrain, is the last to form and develops as a bilateral expansion from a single end of the neuropore in the lancelet and the ascidians (Fritzsch and Martin, 2022). We will analyze four sensory systems (trigeminal, taste, vestibular, and auditory) from their peripheral nerve entry point within the hindbrain through their projection to the telencephalon. In addition, we will focus on the various genes expressed as well as the organizational principles for each system. For instance, while a topological organization exists for the somatosensory and the auditory projections to the telencephalon, the taste, and vestibular projections are not topologically organized. Moreover, higher-order interactions are provided to integrate the cohesive perception in the telencephalon that is documented chiefly in human organization.
Neurons of the peripheral nervous system (PNS) depend on specific genes for proper development (Kobayashi et al., 2019; Dvorakova et al., 2020; Xu et al., 2021). Most brainstem-projecting neurons differentiate by Neurog2 expression from epibranchial placodes (Fode et al., 1998; Dennis et al., 2019), whereas all other remaining placodally derived neurons depend on Neurog1 expression in mammals (Ma et al., 1998). There is a sequential expression of Neurog1/Neurog2 in the spinal cord of the dorsal root ganglia (DRGs; Ma et al., 1999; Meltzer et al., 2021). DRGs in the spinal cord depend on Sox10 followed by Neurog2 for large and mediums sized neurons, and by Neurog1 for small neurons and all depend on Pou4f1 (Huang et al., 2001; Meltzer et al., 2021). In contrast, epibranchial and dorsal placodes develop independently of Sox10 (Mao et al., 2013; Reiprich and Wegner, 2015). In mammals, the trigeminal, facial, glossopharyngeal, and vagus neurons depend on Neurog1, whereas the epibranchial neurons (geniculate, petrosal, and nodose) require Neurog2.
The central nervous system (CNS) consists of the brainstem and spinal cord. In the brainstem, the dorsal alar plate is innervated by placodally derived peripheral sensory neurons, which depend on Neurog1 or Neurog2 (Fode et al., 1998). On the other hand, the spinal cord receives DRG projections which depend on Neurog1/2 (Ma et al., 1999). These fibers extend ventrally to the basal plate in the spinal cord, including the muscle spindles (Lai et al., 2016; Meltzer et al., 2021). The CNS is divided dorsoventrally into the alar and basal plate, the former depending on choroid plexus formation along the dorsal part of the brainstem (Figure 1). Choroid plexus development requires Lmx1a/b expression, and without Lmx1a/b, neither the choroid plexus nor many of the dorsal alar plate structures form (Glover et al., 2018; Chizhikov et al., 2021). Downstream of Lmx1a/b is Gdf7, which is also necessary for roof plate formation (Lee et al., 2000; Mishima et al., 2009). The dorsoventral identity of neural progenitors within the developing dorsal brainstem and spinal cord are subdivided into eight or six distinct domains, respectively (Lai et al., 2016; Hernandez-Miranda et al., 2017; Hirsch et al., 2021). The most dorsal domain, dA1, is defined by an expression of Atoh1 across the brainstem and spinal cord. Ventral to dA1 is the dA2 domain (Neurog1/2 expression), followed by the dA3 domain (Ascl1, Phox2b, and Tlx3 expression) rostrally, which forms the solitary tract (Qian et al., 2001). Caudally to the more rostral dA2/3, we have two neuronal populations of the brainstem: one is presented as a duplication of Ptf1a expression (dA4/dB1), whereas another set of unique populations from dB2 (Atoh1, Phox2b, and Lbx1) expression that forms, among others, the lateral vestibular nucleus (LVN) in rhombomere r4 (Chen et al., 2012; Lunde et al., 2019). While some of these domain populations in the hindbrain are maintained through the spinal cord, such as Atoh1, some gene expression differences lead to the absence of two domains in the spinal cord that are present in the brainstem (Hernandez-Miranda et al., 2017). Although Atoh1 expression is continuous from the spinal cord to the cerebellum, separate nuclei are forming in this expression domain: the cerebellum (r0-1), the auditory nuclei (r2-5), and the pontine nuclei [r6-7 (Bermingham et al., 2001; Nichols and Bruce, 2006; Lowenstein et al., 2022)]. Further work is needed to detail the various nuclei and their specific migration to coalesce into distinct nuclei (r8-11; Rose et al., 2009; Watson et al., 2017a).
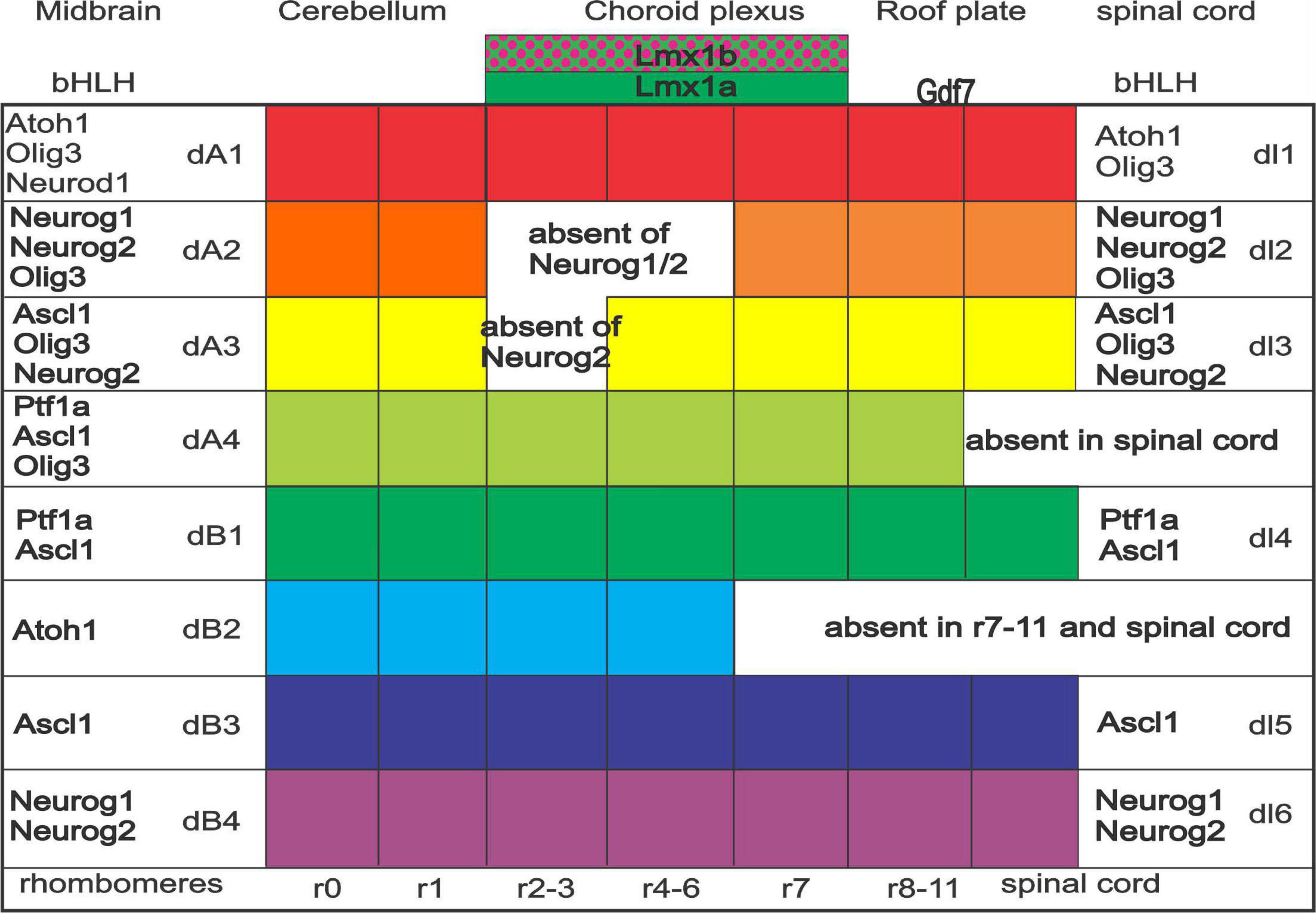
Figure 1. Expression of bHLH genes across spinal cord and brainstem. Specific and, in part, overlapping genes are expressed. Note that the spinal cord has only six (dl1-6) whereas the brainstem forms into eight expressions (dA1-4 and dB1-4). Particular expression is continuing, whereas specific gaps are unique among dA2 and dA3 (absence of Neurog1 and or Neurog2), dA4 (Ptf1a, Ascl1, and Olig3), and dB2 (late expression of Atoh1). Rhombomeres are expressed in certain combined expressions (e.g., r2-3). Note that the choroid plexus depends on Lmx1a/b and Gdf7. Modified and combined after (Iskusnykh et al., 2016; Lai et al., 2016; Hernandez-Miranda et al., 2017; Chizhikov et al., 2021; Lowenstein et al., 2022).
Second-order neuronal projections from the trigeminal, solitary tract, vestibular, and cochlear nuclei project in a relay to several additional nuclei before reaching the thalamus, whereas other second-order predictions are direct. Compared to the trigeminal projection that is common across vertebrates, information from taste, vestibular, and auditory nuclei is limited, and in addition, there is a unique loss of lateral line and electroreception in some species that are instead developing the auditory nuclei from frogs and amniotes (Grothe et al., 2004; Wullimann and Grothe, 2013; Fritzsch, 2021).
The bipartite of the telencephalon has long been seen as an exclusive input from the olfactory system of cyclostomes that is now shown to receive significant input from the trigeminal to the telencephalon in the lamprey (Suryanarayana et al., 2020, 2021), showing a basic telencephalon across vertebrates. Open questions concern the taste, vestibular, lateral line, electroreception, and auditory system in lampreys that show certain connections in gnathostomes (Striedter and Northcutt, 2019). It is unclear how the auditory system evolved beyond rudimentary insights that it is such a central system in mammals, frogs, reptiles, and birds (Briscoe and Ragsdale, 2019; Tosches and Laurent, 2019). Likewise, the taste system forms in all vertebrates but is unclear for higher-order connections to the telencephalon (Elliott et al., 2022a). The vestibular system has a basic understanding of the gnathostomes (Elliott et al., 2022a). Interestingly, there are multiple integrations of sensory systems. For example, auditory and visual input, taste and olfaction, and of vestibular and trigeminal (review in Shepherd, 2006; Majka et al., 2019; Chou et al., 2020; Mizoguchi et al., 2020; Rauschecker, 2021). We will provide the similarity of higher-order interactions of the four sensory systems and how they integrate multisensory modalities in the telencephalon with the four sensory systems.
Structure of the sensory systems
Somatosensory receptors receive touch, proprioception, pain, and thermoreception, which project via trigeminal neurons to nuclei in the brainstem. Two major brainstem nuclei (principle and descending tract) send second-order projections mostly to the contralateral thalamus, and from there, the projections end up in the somatosensory topological cortex. Tertiary connections integrate other cortical sensory system inputs to direct motor output.
Taste buds, the peripheral end organs of gustation, contain chemosensory transducing cells. These cells are in the five different sensory taste buds innervated by four distinct sensory neurons. Three epibranchial placodes develop from Neurog2-expressing ectoderm to provide innervation from the oral cavity, particularly the tongue, and end in the solitary tract (ST) from r3-r11. From there, neurons project to the parabrachial nucleus (PB) to spread directly or indirectly in the thalamus and the insular cortex. Interactions of the olfactory system with vision, auditory, and vestibular provide an integrative taste perspective.
Vestibular hair cells and vestibular neurons detect and send, respectively, linear, and angular acceleration information to the brainstem. Various organizations and sensory epithelia are dedicated to detecting this directional information in gnathostomes. Vestibular neurons depend on Neurog1 and provide innervation of hair cells at the periphery and end up in four distinct vestibular nuclei, as well as a part of the cerebellum (r1-r8) centrally. Within the cerebellum, vestibular neurons project a selected input to the uvula and nodulus. All spinal cord motoneurons receive an ipsilateral projection from the lateral vestibular nuclei (LVN), whereas three other vestibular neurons (superior, medial, and descending vestibular nuclei; SVN, MVN, and DVN) project to the three ocular motoneurons (III, IV, and VI). Bilateral outputs project the dorsal tegmental nucleus, the thalamus, and the parieto-insular vestibular cortex. Additional connections of auditory, visual, and taste information with vestibular input interact to drive higher-order spatial representations.
Auditory sensory neurons are unique neurons that evolved after the segregation of the basilar papilla developed. In mammals, auditory spiral ganglion neurons depend on Neurog1 to innervate Atoh1-dependent hair cells. In mammals, three cochlear nuclei form only in r2-r5. Like the hair cells at the periphery, these cochlear nuclei depend upon Atoh1 expression. Bilateral auditory nuclei interact with the superior olive nuclei (SOC) to go beyond the inferior colliculi (IC) to reach the medial geniculate body (MGB). The auditory cortex (aC) is the main target of the MGB, and auditory cortex neurons interact with those from vision, somatosensory, taste, and vestibular systems.
Structure of the sensory systems and genes needed for their development
Somatosensory sensory inputs extend from the spinal cord to the trigeminal nuclei to provide somatosensory perception, giving rise to four brainstem PNS inputs (trigeminal, facial, glossopharyngeal, and vagal). These inputs reach rostrally from r2 and blend with the spinal cord past r11 caudally (Gray, 2013; Hans et al., 2020). The trigeminal nerve’s ophthalmic, maxillary, and mandibular branches project centrally in a ventral-to-dorsal orientation, ending with the small addition of other sensory neurons (Erzurumlu et al., 2010). The somatosensory input is comparable in the brainstem and the spinal cord: The spinal cord formed from Neurog2 first to develop the largest and intermediate DRGs, followed by the population of Neurog1-dependent small DRGs (Ma et al., 1999; Meltzer et al., 2021). In contrast, brainstem-derived PNS neurons are exclusively developed from Neurog1-expressing cells (Ma et al., 1998) to innervate a mix of placode and neural crest (trigeminal) or neural crest-derived facial, glossopharyngeal (superior ganglion), and vagal sensory neurons (jugular ganglion). Most of the largest neurons reach the motoneurons in the spinal cord, except for the input from the trigeminal neurons.
A unique set of mesencephalic trigeminal neurons (MesV or MTN) develop that reach the brainstem and branch into a distal projection to innervate the muscle spindles of the trigeminal motoneurons (Fritzsch and Northcutt, 1993; Lipovsek et al., 2017; Ter-Avetisyan et al., 2018). The MesV develops in the absence of Neurog1 (Ma et al., 1998; Marzban et al., 2019), independent of the neural crest (Lipovsek et al., 2017). The largest proprioceptor neurons of the spinal cord depend on Atoh1, but these continuations are interrupted at the hindbrain to generate brainstem neurons giving rise to auditory and other neurons, the cuneate and gracile to continue the lateral lemniscus (Kuypers and Tuerk, 1964; Bermingham et al., 2001; Lai et al., 2016).
Most trigeminal and proximal brainstem PNS neurons depend on Eya1/2, which is upstream of Sox2 expression (Xu et al., 2021; Zhang et al., 2021). In addition, Six1, Irx, Pax3, Dlx, Tbx, and Fox1 are expressed early. Sox2 functions as a pioneer factor that regulates the expression of various bHLH genes; the trigeminal neurons depend on Neurog1 expression (Ma et al., 1998; Kobayashi et al., 2019). Downstream of Neurog1 are Neurod1 and Pou4f1, which interact with Isl1 to regulate all trigeminal ganglion neuron development. The first PNS neurons depend on Tbx1/3, which overlaps with the ophthalmic and maxillary ganglions. Further subdivisions are driven by Oc2 (maxillary and mandibular ganglion) and Oc1 and Hmx2 (mandibular ganglion), forming discrete central projections to the CNS (Erzurumlu et al., 2010). An extensive set of distinct genes specify various sensory inputs and proteins fully described in the spinal cord input (Abraira and Ginty, 2013; Handler and Ginty, 2021) and the trigeminal ganglion as well as the MesV (Figures 2A–D). The expression of neurotrophin receptors differs between the spinal cord and trigeminal system for TrkA, TrkB, and TrkC. DRGs and trigeminal neurons depend on Neurog1 and mostly TrkA (Ma et al., 1999). Loss of TrkC leads to the absence of DRGs innervating the muscle spindles, but since about 50% of muscle spindles form in the absence of TrkC suggests many MesV neurons remain (Matsuo et al., 1999; Huang and Reichardt, 2003). Bdnf, which preferentially binds to TrkB, reduces the number of MesV neurons when lost but seems not to affect the muscle spindles, whereas Merkel cells and hair innervation require TrkC. The interaction between TrkC and possibly TrkB remains unclear for the remaining trigeminal neurons. In summary, our data show a different dependence of trigeminal and spinal cord concerning Neurog1/2 (spinal cord) compared to trigeminal, which depends on the placode and neural crest ganglions. A unique formation forms in the MesV that provide muscle spindle innervation but seems to develop independently of Neurog1, which diversifies into a set of neurons that depend on unique neurotrophins (Handler and Ginty, 2021; Meltzer et al., 2021).
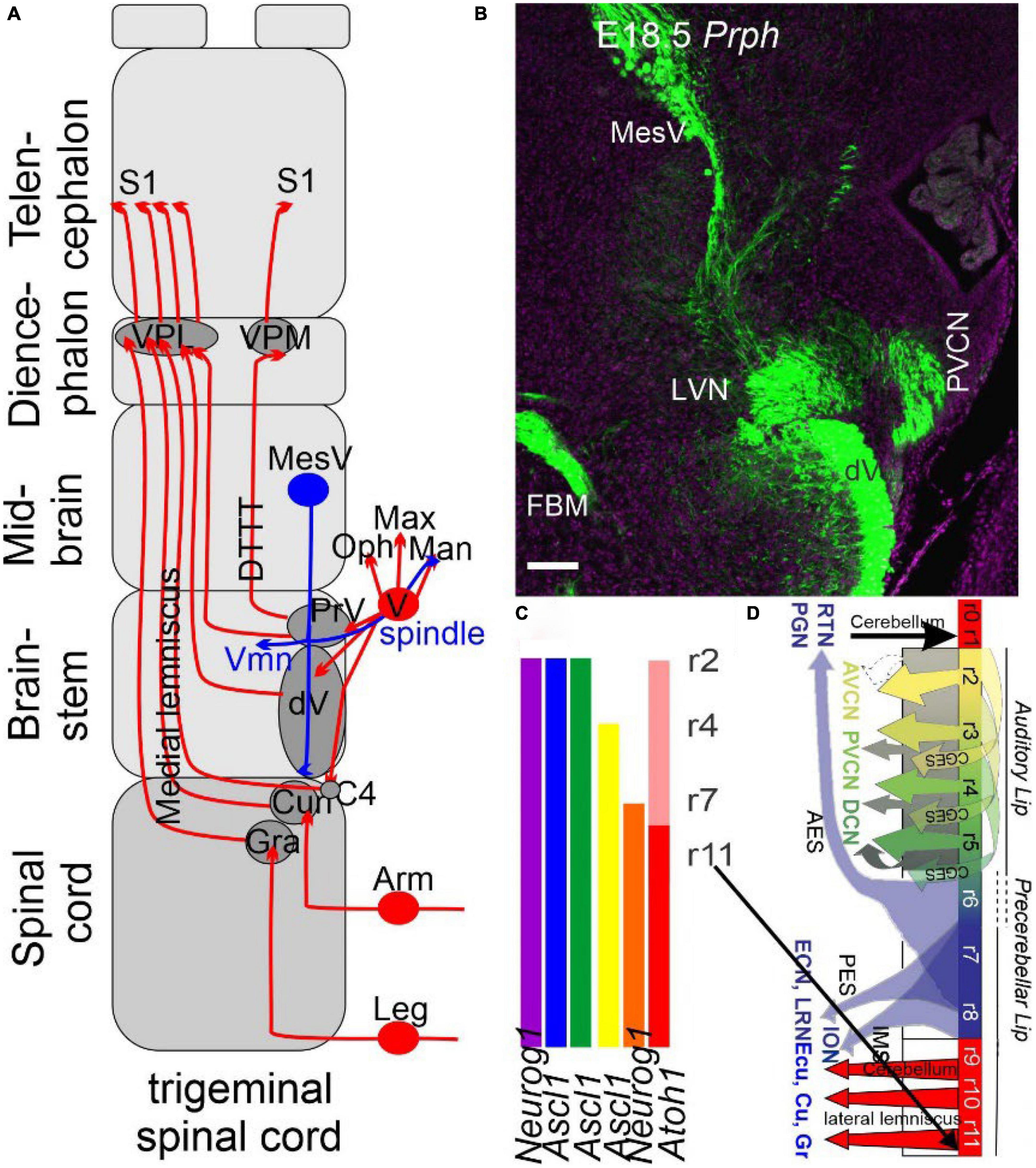
Figure 2. The trigeminal is projecting identical to the spinal cord. (A) Three trigeminal ganglia (ophthalmic, maxillary, and mandibular) project ipsi- and contralateral fibers from r2, whereas r3 of the principal trigeminal (PrV) and the descending trigeminal fibers (Vd) cross to the contralateral side, joining as medial lemniscus with the gracile (Gr) and cuneate (Cu) to reach the ventral posterior lateral nucleus (VPL) and ventral posterior medial nucleus (VPM) to reach the somatosensory cortex (S1). A unique projection is a mesencephalic tract (MesV, blue) to provide spindle fibers branching to the extent of the trigeminal motoneurons (Vmn) and the mandibular fibers. (B) A cross-section shows that all fibers are positive for peripherin (Prph), thus labeling the auditory, trigeminal, vestibular, facial, and MesV. (C) The organization of the primary central fibers is formed from Atoh1, Neurog1, and Ascl1, which have different central projections in the brainstem in dA1-4 and dB1-2. (D) Details of Atoh1 show the formation of the granular neurons of the cerebellum (r0-1), cochlear (r2-5, AVCN, PVCN, and DCN), precerebellum (r6-8, PGN, and RTN) and reaches out the lemniscus fibers (r7-11) to become the gracile (Gr), cuneate (Cu), and the external cuneate (Ecu). The input from the inferior olive neurons (ION) is projecting to the contralateral cerebellum. AES, anterior extramural stream; CGES, cochlear granule cell extramural stream; Ecu, external cuneate; IES, intramural migratory stream; ION, inferior olivary nucleus; PES, posterior extramural stream; PGN, pontine gray nucleus; RTN, reticulotegmental nucleus. Modified after (Bermingham et al., 2001; Farago et al., 2006; Rose et al., 2009; Elliott et al., 2021a, 2022a).
Taste sensory information develops a unique step for taste buds in the oral cavity. Fungiform papillae depend on sonic hedgehog (Shh) and Wnt (Hall et al., 2003; Liu et al., 2007), while circumvallate papilla formations are regulated by FGF10 and its receptors Spry1-2 (Petersen et al., 2011). Sox2 expression and innervation are required for continued taste bud development (Okubo et al., 2006; Fan et al., 2019). Taste buds are not differentiated when Sox2 is conditionally deleted. The absence of Neurog2 results in a reduction of Sox2 expression (Okubo et al., 2006; Ohmoto et al., 2017) and causes taste bud formation to abort beyond a limited differentiation of small, single taste buds (Fan et al., 2019). Bitter-sensing taste buds seem to depend on Eya1, suggesting a unique expression of these sensory cells (Ohmoto et al., 2020). The differentiation of specific taste bud cells depends on numerous factors, only some of which have been identified (Pou2f3, Ascl1, and Eya1).
Neurons delaminate from the epithelium and migrate to achieve a final position near where three cranial nerves (facial, glossopharyngeal, and vagus) develop. Upstream of Neurog2, which defines the epibranchial placodes (Fode et al., 1998; O’Neill et al., 2012), is the expression of Eya1, Sox2, and Pax2. Downstream is the expression of Neurod1, Isl1, Pou4f1, Phox2b, and Foxg1 (Alsina, 2020), which are needed to differentiate into the distal part of epibranchial neurons. Phox2b is explicitly required for a visceral sensory neuron fate (D’Autréaux et al., 2011), whereas other genes are needed for differentiation, such as a set of genes, Coe1, Drg11, and Dcx. This set of genes is only turned on after the migrating cells have left the placode (Freter et al., 2012; Smith et al., 2015). Following neuronal differentiation, developing gustatory neurons express Ntrk2 (TrkB), required for the survival of all epibranchial neurons (Fritzsch et al., 1997). Ligands for the TrkB receptor, BDNF and NT-4, are expressed in the epibranchial neuron placodes and the taste buds (von Bartheld and Fritzsch, 2006; Huang and Krimm, 2010; Nosrat et al., 2012). Axons reach the correct target by making multiple decisions at several different locations along the path to the taste buds (Figures 3A–C). In summary, the development of both taste neurons and taste buds depends on a series of genes independent of one another: peripheral axons of neurons reach the taste epithelium. These two cell types become interdependent.
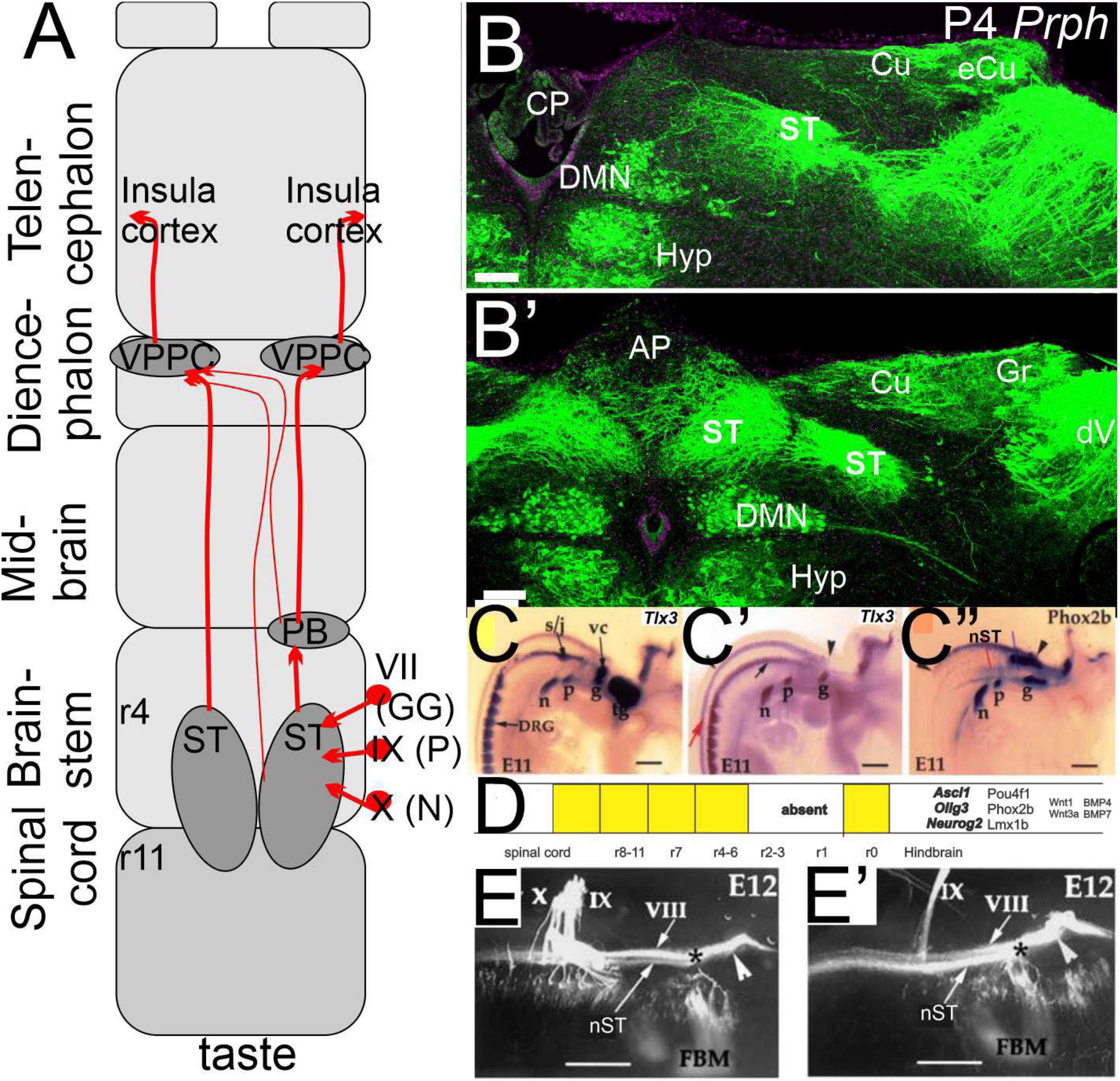
Figure 3. The taste buds are projecting via the solitary tract to innervate the insula cortex. (A) Three peripheral neurons (geniculate, petrosal, and nodose) project from r4-11 bilaterally to extend via the parabrachial nucleus (PB) and the thalamus (VPPC) to the insula cortex. (B,B’) sections show the solitary tract that is initially ventral to the vestibular nuclei that will replace more caudal to the external cuneate, cuneate, and gracile (eCu, Cu, and Gr). Note that the bilateral interaction is particularly large dorsal to the dorsal motor neurons (DMN) that interact with the area postrema (AP) that is replaced by the choroid plexus (CP). Central projection is positive for Tlx3 (C,C’) and Phox2b (C”), which define the dA3 (D). Central projections show an identical pattern after the Tlx3 is ablated (E,E’). Modified after (Elliott et al., 2021a, 2022a; Roper et al., 2022). *VII entry point.
Vestibular neurons innervate vestibular hair cells located within the three canal cristae and two (mammals) or three (most gnathostomes; Fritzsch et al., 2002; Duncan and Cox, 2020) macula (Maklad and Fritzsch, 1999; Straka et al., 2014). Two vestibular neurons project to the vestibular hair cells (Desai et al., 2005): type I and type II, which innervate the two types of vestibular hair cells. The central projection of these neurons shows an incomplete and partial overlap of their target regions (Chagnaud et al., 2017; Elliott and Straka, 2022) that expands from the cerebellum rostrally to r8 caudally (Maklad and Fritzsch, 2003; Straka et al., 2014; Glover, 2020).
Vestibular neurons depend on certain common genes for specification and differentiation. For instance, no neurons develop without Neurog1 (Ma et al., 2000). Upstream of Neurog1 is the expression of the Brg1-based SWI/SNF chromatin-remodeling complex, which interacts with the neurosensory-specific transcriptional regulators of Eya1/Six1 (Xu et al., 2021). These regulator genes induce the expression of Sox2 (Dvorakova et al., 2020) to promote proneurosensory-lineage specification, such as upregulating Neurog1 expression (Reiprich and Wegner, 2015; Kageyama et al., 2019). Downstream of Neurog1 is Neurod1, which is important for neuronal differentiation; many neurons are lost without it. In addition, without Neurod1 ectopic hair cells develop (Jahan et al., 2010; Macova et al., 2019). Pou4f1, Isl1, and bHLHb5, among others, are also needed for normal vestibular or auditory neuron development (Filova et al., 2020). The initial induction of an otic placode depends on Foxi3 (Birol et al., 2016) and Fg3/7/10 (Urness et al., 2011). Any differentiation of neurons or hair cells from this placode requires the expression of Eya1 and Pax2/8 (Zou et al., 2004; Bouchard et al., 2010). A disorganized development occurs following the loss of several genes, including Lmx1a/b, Gata3, Dicer, and Shh, among others (see reviews Riccomagno et al., 2002; Kersigo et al., 2011; Duncan and Fritzsch, 2013; Elliott and Gordy, 2020; Chizhikov et al., 2021). The loss of distinct neurons following specific gene deletion remains unclear (Diaz and Glover, 2021).
Atoh1 is an essential gene for all mechanosensory hair cell development (Bermingham et al., 1999) beyond undifferentiated cells (Fritzsch et al., 2005). Vestibular hair cells differentiate into two types in amniotes: type I and type II (Elliott and Straka, 2022). The macula are divided into two distinct areas and are innervated by neurons that send central processes selectively to either the cerebellum or the brainstem, depending on the area of the macula that they innervate (Maklad and Fritzsch, 2003; Balmer and Trussell, 2019). Emx2 is only expressed in one of these distinct areas (Holley et al., 2010; Jiang et al., 2017) and requires a detailed analysis of central connections to the cerebellum or the brainstem (Figures 4A–C). Hair cells within the utricle and saccule show a polarity that provides 360 degrees of sensitivity. In contrast, hair cells within a particular canal crista are all polarized in the same direction (Fritzsch et al., 2002; Elliott and Gordy, 2020). In summary, the vestibular neurons and hair cells require specific genes for proper development.
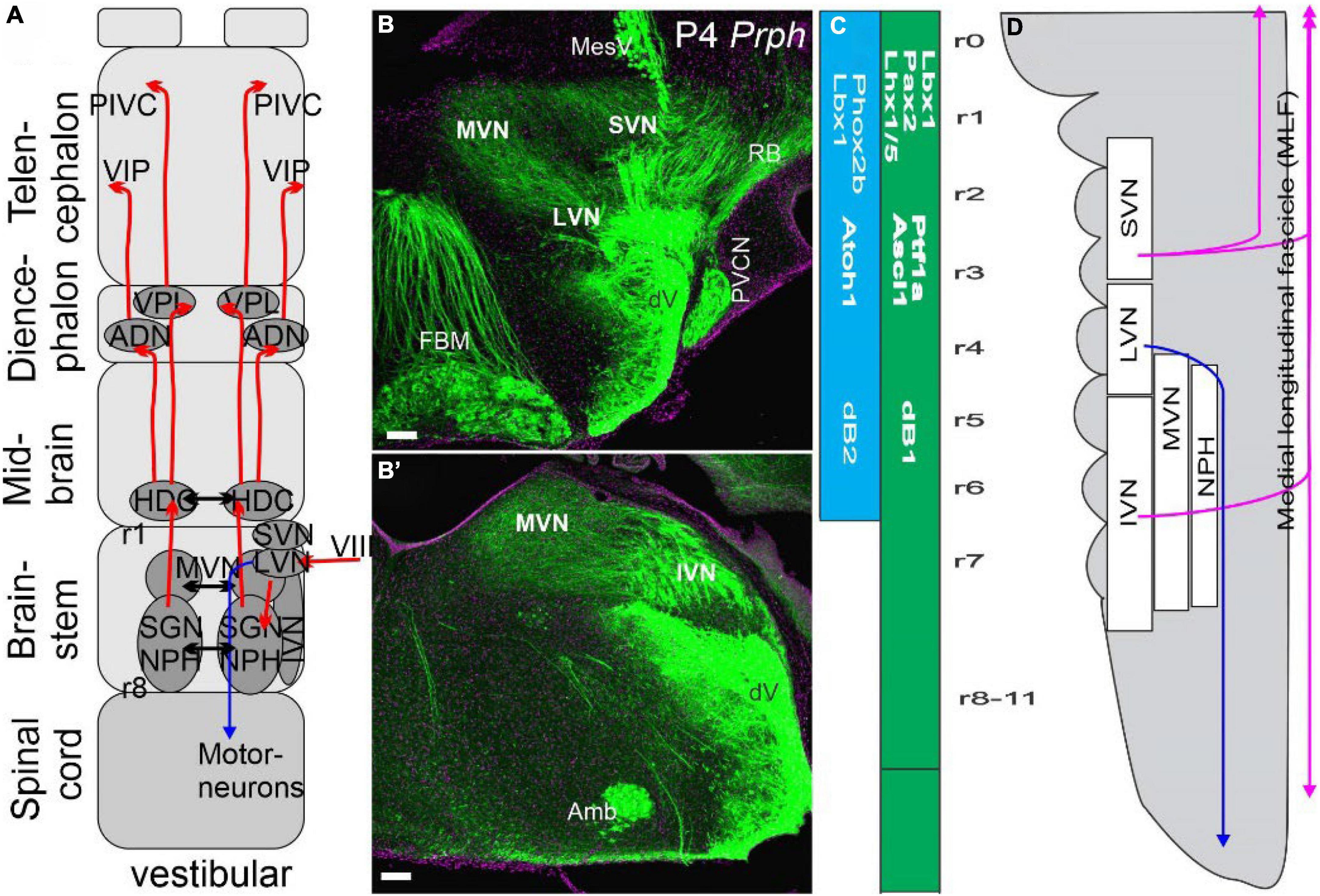
Figure 4. Vestibular neurons innervate to reach the cortex bilaterally via four nuclei. (A) Three vestibular nuclei send fibers bilaterally to the thalamus directly via the ventral posterior lateral nucleus (VPL) and indirectly via several steps to reach the anterior dorsal thalamus (AND). From there, projections extend to the distinct areas of the vestibular cortical area PIVC and the entorhinal cortex (VIP). Separate ipsilateral projections come from LVN to project to spinal motoneurons (in blue). (B,B’) A cross-section shows Prph expression in vestibular nuclei prominent LVN, SVN, and MVN that will continue as the IVN. (C) Two areas have been identified that form in dB1 and dB2. Note that dB2 is found between r1-r6 and is dependent on Phox2b and Lbx1. In contrast, dB1 continues with r0 to the spinal cord and is positive for Ptf1a, Ascl1, and Lhx1/5. (D) SVN, LVN, and IVN are ongoing, whereas the MVN is a separate, more medial organization next to the nucleus propositus hypoglossi (NPH). Note that ventral and dorsal fibers are indicated in color (blue for LVN, lilac for MLF). Modified after (Elliott et al., 2021a, 2022a; Elliott and Straka, 2022).
Auditory neurons innervate auditory hair cells located within the basilar papilla or cochlea. As with the vestibular system, there are two types of auditory neurons (type I and type II) that innervate two types of hair cells: inner hair cells (IHCs) and outer hair cells (OHCs) (Grabner and Moser, 2018; Jean et al., 2019; Moser, 2020). In mammals, these are arranged in a highly stereotyped organization with one row of IHCs and three rows of OHCs. The central projection of auditory neurons shows a highly organized tonotopic organization in auditory nuclei (Fritzsch et al., 2019).
As with vestibular neurons, auditory neurons also require the expression of Neurog1 as these neurons never develop in the absence of its expression (Ma et al., 2000). Neurog1 interacts with transcriptional regulators to promote the development of auditory neurons, the spiral ganglion neurons (SGN). In addition to Neurog1, these neurons depend on Gata3, Pax2, and Lmx1a/b: without any of these three transcription regulators, all SGNs are lost (Bouchard et al., 2010; Duncan and Fritzsch, 2013; Chizhikov et al., 2021). Downstream of Neurog1 is Neurod1 which regulates the differentiation of sensory neurons and their projections to the organ of Corti and the ventral cochlear nuclei (Jahan et al., 2010; Macova et al., 2019). In addition, SGNs require the expression of Pou4f1, Isl1, and bHLHb5, among others (Filova et al., 2020).
Eya1, followed by Sox2, is needed to initiate hair cell development (Dvorakova et al., 2020; Xu et al., 2021). As with vestibular hair cells, auditory hair cells also critically depend upon Atoh1 expression (Bermingham et al., 1999). Downstream of Atoh1, hair cell differentiation requires Pou4f1 and Gfi1 (Xiang et al., 2003; Hertzano et al., 2004; Eng et al., 2007). Cochlear hair cells also need Pax2, Gata3, Lmx1a/b, and Dicer: without each case, there are no hair cells formed in the cochlear sac (Bouchard et al., 2010; Kersigo et al., 2011; Duncan and Fritzsch, 2013; Chizhikov et al., 2021). Unique genes are needed for the specific differentiation of IHCs (Nakano et al., 2020) and OHCs (Lorenzen et al., 2015; Chessum et al., 2018; Wiwatpanit et al., 2018). For instance, Emx2 is needed to differentiate OHCs (Holley et al., 2010; Kindt et al., 2021). The total length of hair cells in the cochlea depends on Neurog1, Neurod1, Foxg1, Prox1, and n-Myc (Matei et al., 2005; Pauley et al., 2006; Fritzsch et al., 2010; Kopecky et al., 2011; Filova et al., 2020). Hair cells must be polarized with stereocilia aligned in a stair-step organization to function optimally. Specific genes are expressed that establish the distinct polarity of both vestibular and auditory hair cells (Montcouquiol et al., 2003). This polarity develops through steps, resulting in a kinocilium on one end (for vestibular hair cells; cochlear hair cells lack a kinocilium) connected with stereocilia that form a staircase (Fritzsch and Elliott, 2017; Elliott and Straka, 2022). After the polarity is established, Pcdh15 and Chd23 interact as tip links between taller and shorter stereocilia to respond to proper stimulation. Hair cells require a channel, Tmc1/2, to open, allowing an influx of K+ ions (Pan et al., 2013; Shibata et al., 2016; Erives and Fritzsch, 2020).
Somatosensory—Developing distinct mechanosensory innervation
Nuclei dedicated for nociception (pain), thermosensation (temperature), pruriception (itch), mechanosensation (cutaneous/touch), and proprioception (limb and body position) are found in the brainstem and spinal cord (Erzurumlu et al., 2010; Lai et al., 2016; Meltzer et al., 2021). An extensive set of different neuronal categorizations is dependent on their size (largest and fast conducting Aα fibers, a variety of medium-sized conducting Aβ and Aδ fibers, and the thinnest unmyelinated C fibers) and provides proprioception (Ia, Ib, and II) that innervates the muscle spindle and tendon organs. The four mechanosensory cells are Merkel cells, Meissner, Ruffini, and Pacinian corpuscles (Abraira and Ginty, 2013; Handler and Ginty, 2021). Most of the innervation is driven by low-threshold mechanoreceptors (LTMR) for detecting mechanical stimuli, but there is also specific innervation for high-threshold mechanoreceptors (HTMR), which provide noxious input by thin, unmyelinated fibers (Meltzer et al., 2021).
Merkel cells depend on the local upregulation of Atoh1 (Maricich et al., 2009) and Pou4f3 for normal development. Like many other sensory innervations, these cells depend on Piezo2 for mechanosensory cation channels, including the Aβ-LTMR innervation. Merkel cells are local next to the keratinocyte cells and show multiple indentations with desmosomes to interact with Merkel cells. An exciting feature is the presence of Piezo2 in the afferent fiber and the Merkel cells, suggesting an interaction between the complex. Synapses form in Merkel cells, releasing them upon proper stimulations (Handler and Ginty, 2021). Compared to the TrkC-dependent Merkel cells that respond to forces of about 10 Hz, some fibers react to specific frequencies around 100 Hz (Meissner cells, which depend on TrkB). In comparison, Pacinian corpuscles are most sensitive to about 200–700 Hz and require Ret for proper innervation (Handler and Ginty, 2021). In summary, a wide variety of mechanosensory inputs depend on Piezo2 to drive obviously in Merkel cells, a unique bHLH gene-dependent formation.
Central nuclei differ between the brainstem and the spinal cord
A large set of expressed genes and proteins regulate the different central projections in the spinal cord (Lai et al., 2016; Handler and Ginty, 2021; Loutit et al., 2021) and the brainstem (Hernandez-Miranda et al., 2017; Hirsch et al., 2021). Whereas the spinal cord receives mechanosensory fibers from proprioceptors (muscle spindles), Aβ, Aδ, and C fibers, the trigeminal system in the hindbrain receives only Aβ, Aδ, and C from the trigeminal neurons to project the principle and the descending trigeminal branches that extend to C4. A separate projection from proprioceptors reaches the trigeminal motoneurons through bifurcations (Matsuo et al., 1999; Ter-Avetisyan et al., 2018) and provides information from the dental gums along the descending track (Lazarov, 2002). Trigeminal motoneurons are a part of the branchiomotor nuclei that belong to the facial, ambiguous, and dorsal motor nuclei (DMNs) that express Phox2a/b and Lmx1b (Figures 1, 2; Fritzsch et al., 2017). At least four dorsal nuclei receive trigeminal innervation, defined by the Ascl1 gene (dA3, dA4, dB1, and dB3). In contrast, only three nuclei receive somatosensory information in the spinal cord (dl3-5; Figures 2A–D). A continuation of Neurog1/2 (dl2) and Atoh1 (dl1) defines the following expression in the spinal cord to regulate. Interestingly, this continued expression of Atoh1 from the spinal cord to the cerebellum (dl1, dA1) shows a gap for Neurog1 (dl2, dA2 to r7 and expression of r1) to add the cerebellum Purkinje cells (Hawkes, 2012; Obana et al., 2018). In the brainstem, the dorsal Atoh1 expressing column gives rise to cerebellar granule cells (r1), the cochlear nuclei (r2-5), the pontine nuclei (r6-7), the pre-cerebellar nuclei (r7-8) and the external cuneate, the cuneate and the gracile (r9-11; review in Farago et al., 2006; Rose et al., 2009; Gray, 2013; Watson et al., 2017a; Arimura et al., 2019; Hirsch et al., 2021; Pop et al., 2021), that project to the cerebellum (pontine, external cuneate, inferior olivary nucleus; ION). Some areas expand from dA4 to expand from the ION, and depend on Ptf1a (Yamada et al., 2014; Iskusnykh et al., 2016; Hirsch et al., 2021), a unique contribution to the brainstem (Hernandez-Miranda et al., 2017).
Rodents best describe the detailed terminals of trigeminal neurons (Erzurumlu and Gaspar, 2020). Central projection of these neurons depends on Hoxa2, Lmx1b, Epha4/7, Slit2, Robo1/2, Neuropilin1, and Sema3a to define the maxillary branch that innervates the unique whiskers for normal central innervation of r2 (mandibular) and r3 (maxillary). Five rows of the maxillary projection were formed to develop a ‘barrelette.’ Robo deletions can cause a duplication from both ipsilateral and contralateral fibers to reach the somatosensory cortex (Iwasato and Erzurumlu, 2018). Retinoic acid (RA) influences the patterning of Hoxa2 (Glover et al., 2006). Lmx1b acts upstream of Fgf8 and limits the rostral distribution of Hoxa2 to r2 (Glover et al., 2018). Fate mapping shows the principal trigeminal nucleus (PrV) is derived from r2 and r3 and the spinal cord (caudalis; SpV) to present an inverted projection that starts in the ophthalmic region to the more dorsal mandibular fibers in a topographical projection of the face. Lower jaws and lips project from the mandibular ganglia to r2, whereas the maxillary fibers project to r3 to innervate the ‘barrelette’ (Erzurumlu et al., 2010). Second order trigeminal neurons give rise to the contralateral lemniscal of SpV, whereas the PrV projects to the thalamus to innervate the VPM (Erzurumlu et al., 2010; Iwasato and Erzurumlu, 2018). Mutants have disorganized central projections that lack the ‘barrelette’ formation (Drg11, Nmdar1, and Lmx1b; reviewed in Erzurumlu and Gaspar, 2020).
In humans, we can define between the PrV, which forms a dorsomedial division, and a ventrolateral division, likely belonging to r2 and r3, respectively. The dorsomedial provides the dorsal trigeminothalamic tract (DTTT; Figure 1A) to reach the ipsilateral VPM, whereas the ventrolateral division, the ventral and dorsal trigeminothalamic tracts (VTTT, DTTT) that crosses to innervate, like other trigeminal lemniscus fibers, the VPM next to the VPL in humans (Suer, 2021) and monkeys (Andrew et al., 2020). It requires a detailed analysis in mice for the ipsilateral projections from r2. A discrete projection from the PrV projects predominantly ipsilateral fibers. Atoh1 is expressed from the cerebellum to the spinal cord but shows a regular formation to generate granular cells, auditory nuclei, pontine, and ION, and receives the external cuneate, cuneate and gracile between r1-r11 (Figure 2). At least four Ascl1 positive genes (dA3, dA4, dB1, and dB3) are driving, in part, the development of trigeminal nuclei. In summary, the spinal and trigeminal central projections are contralateral and are, in part, like the medial lemniscus in the SpV, cuneate, and gracile.
The thalamus receives the topological somatosensory inputs
In mice, fibers reach the VPM to innervate as ‘barreloids’ the thalamic nucleus (Erzurumlu et al., 2010) from r3 projections, while the spinal fibers enter as the contralateral medial lemniscus (SpV, gracile, and cuneate) to innervate the VPL (Iwasato and Erzurumlu, 2018). Interesting bilateral information is described in gums and teeth that show faster ipsilateral projections in humans and monkeys (Andrew et al., 2020; Hihara et al., 2020). We have a somatosensory, topological innervation (Figures 1A, 5A) to project to the mammalian cortex with a primary and secondary innervation (Liao et al., 2020; Qi et al., 2020; Zilles and Palomero-Gallagher, 2021). Based on the earliest description of Brodmann’s work, they defined cortical organizations that were further distinct, showing the ‘homunculus’ by Penfield. In addition, lampreys and gnathostomes show a similar direct trigeminal projection that reaches, with VPM/VPL, the cortex (Briscoe and Ragsdale, 2019; Suryanarayana et al., 2020).
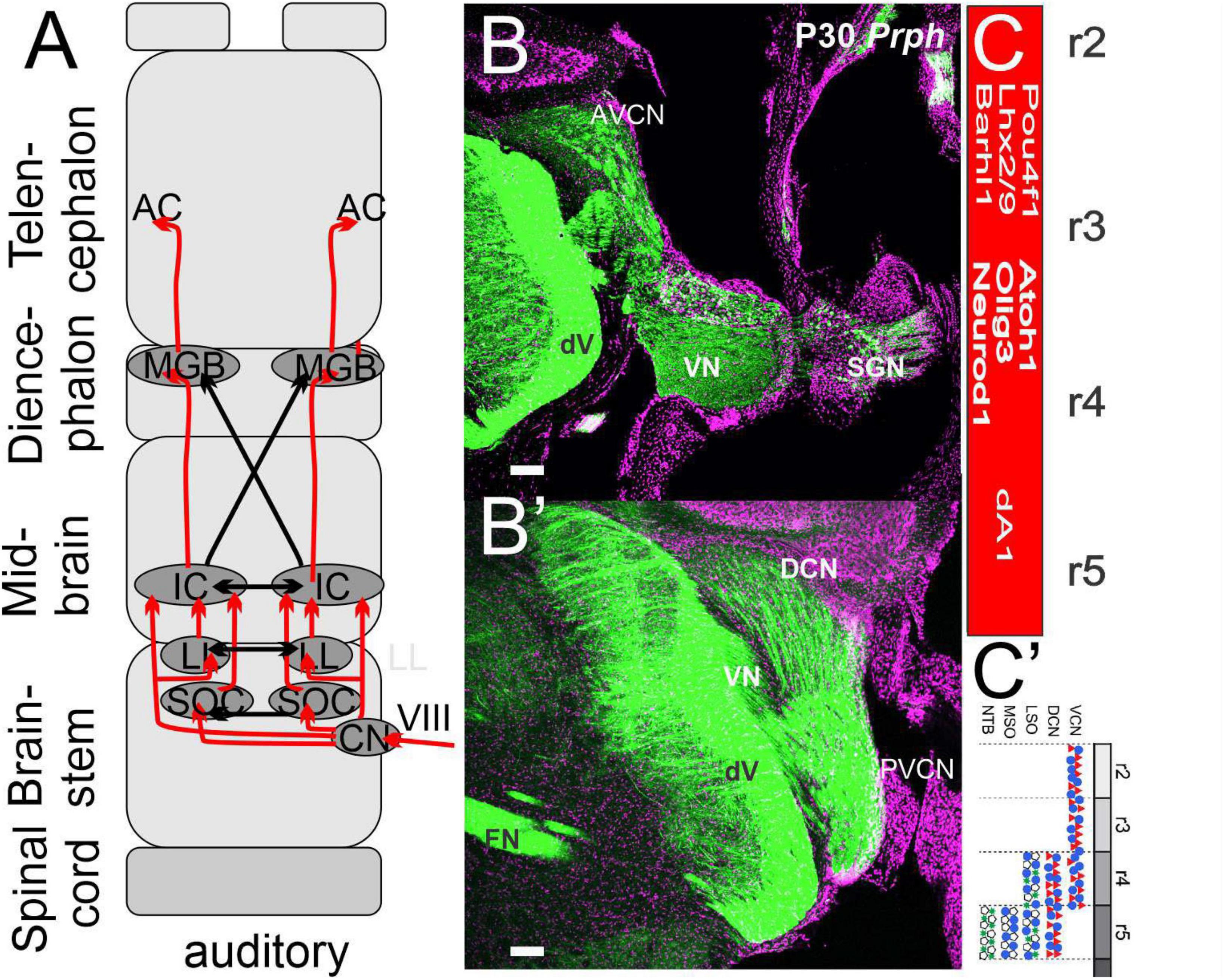
Figure 5. Cochlear neurons innervate three nuclei forming bilaterally in the auditory cortex. (A) Three cochlear nuclei (AVCN, PVCN, and DCN) project bilaterally to an intermediate set of SOCs and LL to innervate directly or indirectly the IC. Crossing fibers are prominent that project to the auditory cortex (A1). (B,B’) Sections reach different areas, shown with Prph. (C,C’) A single cochlear nuclei depend on Atoh1, Olig3, and Neurod1 giving rise to the SOC from r4/5. Modified after (Lipovsek and Wingate, 2018; Elliott et al., 2021a, 2022a).
Mice, and other rodents, have a unique organization, the barrel field, a significant innervation that can be manipulated by loss and gain of input (Erzurumlu and Gaspar, 2020). The cortical organization is affected by changing the pattern of central projections. For example, sensory receptors of whiskers differentiate after birth and can change the pattern from the brainstem (barrelettes), thalamus (barreloids), and the cortex (barrel field). Further, manipulations have studied the effect of plasticity on morphological and physiological plasticity. Fgf8 defines the rostromedial neocortical primordia that organize somatosensory cortical maps. Calcium signal drives the thalamus and barrel field before sensory input is present, and without calcium, it results in hyperexcitability to provide projections to the barrel field organization (Antón-Bolaños et al., 2019). The organization is controlled by specific transcription factors with dendrites that suggest the self-organization of a proto-map assembly to drive thalamocortical sensory circuits (Steiner et al., 2020). Cross-modal plasticity can substitute input modality during development, which has important implications for plasticity and evolution after surgical replacement of part of the cortex (Pallas, 2001; Kral and Pallas, 2011). In summary, the developing somatosensory input generates the topological input that all mammals and other vertebrates provide. The role of innervation depends on specific inputs to create the barrel field in mice and can be used as cortical reorganizations.
Taste buds innervate four sensory neurons from five-sensory receptors
Four types of taste bud cells have 30 to 150 tall columnar cells in the orofacial innervation. The largest population (about 50% of cells) are Type I cells, glia-like cells. Type II and Type III cells are receptor cells for the 5 basic taste qualities (sweet, salty, sour, bitter, and umami). Type IV cells are considered immature cells that do not extend to the taste and differentiate into other cell types over the next 20 days (Perea-Martinez et al., 2013; Ohmoto et al., 2020). Type II, III, and IV contribute to 19, 15, and 14% of taste bud cells, respectively (Yang et al., 2020).
Type II taste cells are chemosensors that express G protein-coupled taste receptors (Tas1Rs, Tas2Rs) to detect sugars, amino acids, and bitter compounds. Sugars bind to the dimeric receptor Tas1R2/Tas1R3 while umami activates the dimeric receptor Tas1R1/Tas1R3. It is unknown whether Tas2Rs form dimers in bitterness (Kinnamon and Finger, 2019; Ohmoto et al., 2020; Roper, 2020). Salt/NaCl taste responds to a subset of Type II cells to release neurotransmitters (Bigiani, 2021). Type III taste cells form conventional synapses with sensory afferent fibers and respond to sour tastes (Roper, 2020). These cells respond to a combination of (1) intracellular acidification and (2) proton (H+) influx through apical OTOP1 channels (Teng et al., 2019; Zhang Y. et al., 2020).
Six neurotransmitters have been identified in taste buds: ATP, 5-HT, GABA, ACh, glutamate, and noradrenaline (see review Roper and Chaudhari, 2017; Roper, 2021), with receptors expressed on the postsynaptic sensory afferent terminals. Individual taste cells and gustatory afferent nerve fibers respond either to single tastes (sweet, salty, sour, and umami) or, more broadly, to several different taste qualities (Wooding and Ramirez, 2020) that are more compatible with cross-fiber or combined tastes (Roper, 2020). A subset of neurons innervates taste buds that express the mechanosensitive channel Piezo1, which may play a role in the tactile responsiveness of taste buds (Dvoryanchikov et al., 2017). In summary, three sensory cells (sweet, bitter, and umami) are sensory Type II cells, whereas sour cells are Type III cells that synapse onto neurons. Salt sensation is unclear to date.
The solitary tract depends primarily on three genes
Central projections of three different nerves for taste perception (geniculate, nodose, and petrosal) show initially entirely overlapping terminal fields (Figures 3A–E); each nerve occupies a discrete, overlapping territory, suggesting a weak oral topography (Hill and May, 2007; Lundy and Norgren, 2015). A ‘homotopy’ is more pronounced in lampreys and lower gnathostomes than in mammals (Finger, 1978; Fritzsch and Northcutt, 1993; Daghfous et al., 2020). It raises the question of the functionality of constant input (Finger, 2008) and suggests further investigation (Lundy and Norgren, 2015).
Centrally, taste information ends up in the nucleus of the solitary tract (ST or NST; Rose et al., 2009; Gray, 2013; Hernandez-Miranda et al., 2017). The development of the ST depends on a combination of bHLH genes (Ascl1, Neurog2, and Olig3) that outline the dA3 brainstem through the spinal cord (Figures 3A,B). The genes, Tlx3, Lmx1b, and Phox2b are co-expressed in the neurons of the ST but show a different longitudinal expression that is prominent for Phox2b in the dA3 (r1-r11) that end before the DRGs (Qian et al., 2001; Hirsch et al., 2021). Phox2b is essential for the viscerosensory fate that determines the dA3 that convert into dB3-like development (D’Autréaux et al., 2011). In contrast, Tlx3 expressing neurons project from the cerebellum and continue from r4 through the spinal cord (Figure 3; Qian et al., 2001). Lmx1b is expressed throughout the cerebellum and spinal cord (Mishima et al., 2009). A fusion of the left and right ST forms a prominent input that reaches r8-11 and forms the area postrema that expands ventrally to the gracile and overlaps with the hyoglossus (XII; Gray, 2013).
A subpopulation, which expresses the dA3 markers Phox2b/Lmx1b (Figures 3C–E), is localized to the ST by projecting to the parabrachial nucleus (PB) and reaching out to the pre-locus coeruleus (pLC) complex in the prepontine hindbrain (r0-1; Watson et al., 2017b; Gasparini et al., 2021) to subdivide PB as depending on Lmx1b and Phox2b. A large regulation provides homeostatic functions, hunger, thirst, sodium appetite, taste aversion, and cardiorespiratory influence (Karthik et al., 2022). Other ST reaches ventrally and caudally to reach different cervical and thoracic spinal cord segments to innervate pre-motor neurons (Hirsch et al., 2021), among many other connections (Lundy and Norgren, 2015). In summary, taste buds send taste information through three cranial nerves (VII, IX, and X) to the hindbrain that develops specific gene expression (dA3) to innervate the solitary tract (r4-11) that retains a rough orthotopic organization.
Thalamus provides the input to the insula
Solitary tract neurons send output projections to a variety of different targets, including the PB as one of the heaviest targets to receive input from the isthmus (r0) and rhombomere 1 (r1; Watson et al., 2017b). The PB neurons relay via gustatory and general visceral input to the “gustatory/visceral” thalamic region that also receives input from the ST directly (Figure 3A). The thalamic gustatory relay is referred to as ventral posterior thalamic nucleus parvocellular (VPPC) and is defined as a ‘taste relay’ (Herbert et al., 1990; Lundy and Norgren, 2015). This unique, predominantly ipsilateral connection breaks down into a complex interaction from the VPPC that receives fibers from the ipsi- and, to a lesser extent, the contralateral PB. The largest PB injections reach the medial septum, olfactory tubercle, and receive the gustatory cortex (Saper and Loewy, 1980; Lundy and Norgren, 2015). The orthotopic organization in the ST is not preserved in higher-order nuclei, making the need for any orthotopic primary map even fuzzier (Lundy and Norgren, 2015). PB neurons project axons indirectly to the insular cortex via VPPC (reviewed in Lundy and Norgren, 2015; Gasparini et al., 2021).
In primates, including humans, the central gustatory pathway bypasses the PB, projects directly to the VPPC, and reaches the granular region of the insular cortex (Lorenzo, 2021). Highly conserved second-order neurons project taste information to the cortex (Lundy and Norgren, 2015; Vendrell-Llopis and Yaksi, 2015; Staszko and Boughter, 2020). The major terminal field in the insula region interacts with other inputs, in addition to taste (Fain, 2019; Staszko et al., 2020). In contrast to the clear-cut neuroanatomical pathways for taste, a uniform interpretation of taste coding has yet to emerge in the insula cortex. Imaging studies have conflicting reports (Chen et al., 2011, 2021), and many studies report a broad distribution of taste neurons throughout the insula cortex with no spatial organization (Staszko et al., 2020), including fMRI studies in human subjects (Canna et al., 2019; Avery et al., 2020). Gustatory responses were elicited from the anterior tongue and posterior tongue, and pharyngeal responses were located caudal to the anterior tongue (Hanamori et al., 1998). The reciprocal connection between the cortex, PB, and ST indicates descending projection from forebrain areas converging the brainstem gustatory neurons, the PB, and the ST into an integrated interaction. In summary (Figure 3A), gustatory PB neurons reach the taste cortex without or with a relay with the thalamus and have reciprocal connections with the gustatory and visceral cortex directly from the PB.
Vestibular nuclei develop from two areas in the hindbrain
Vestibular neurons innervate the semicircular canals, and otolithic organs at the periphery and project centrally to the vestibular nuclei (Straka et al., 2014). Mammals have four defined nuclei: the descending, lateral, medial, and superior vestibular nuclei (DVN, LVN, MVN, and SVN). Nuclei that form the SVN are from r1-3, LVN mostly from r4, DVN from r5-8, and MVN from r2-8 (Chagnaud et al., 2017; Glover, 2020; Elliott and Straka, 2022). Vestibular afferents project partially overlapping and separate within the vestibular nuclei (Figures 3A–D). There is no topologically organized central or peripheral organization compared to the trigeminal system, which is more akin to the taste input (Fritzsch et al., 2019). Within the overlap of different central projections, one can distinguish them into incomplete projections in partial central information. For example, the cerebellum receives only fibers from the saccule to the uvula, whereas the posterior canal is prominent to the nodulus and has a limited expansion to the uvula (Maklad and Fritzsch, 2003; Balmer and Trussell, 2019), like the remaining vestibular organization (Elliott and Straka, 2022). Central projections depend on the expression of specific genes. For example, in the absence of Neurod1, Gata3, or Fzd3, there is an overlap of cochlear and vestibular central projections (reviewed in Duncan and Fritzsch, 2013; Duncan et al., 2019; Filova et al., 2020; Stoner et al., 2021). Lmx1a/b double null mice cross afferents to the contralateral side after the choroid plexus is eliminated (Chizhikov et al., 2021).
Vestibular nuclei develop from at least two longitudinal central nuclei progenitor populations: dB1 and dB2 (Hernandez-Miranda et al., 2017; Hirsch et al., 2021). dB1 expresses Ascl1 and Ptf1 from the spinal cord to the cerebellum (r0-r11, spinal cord). Additional genes expressed are Lbx1, Pax2, and Lhx1/5 (Diaz and Glover, 2021). In contrast, a unique gene expression is present in dB2, Phox2b, and Lbx1, from r1 to r6. A later manifestation of Atoh1 is reported (Hirsch et al., 2021). Gene expression of Hoxb1 overlaps with r4 and depends on Phox2b and Lbx1 that define the LVN, reaching the ipsilateral spinal motor neurons (Chen et al., 2012). Further, developing mice (E13-15; Lunde et al., 2019) show a nearly equal expression of Esrrg, Maf, and Pou3f1 in LVN/MVN. In contrast, Lhx1/5, Evx2, and Foxp2 are expressed in the nearby MVN in r5 that project in a different direction with additional expression of several other genes (Lunde et al., 2019). In addition, a reduced expression level in Onecut1-3 is higher in the MVN compared to the LVN (Glover, 2020). Additional expression is possible from other origins (Figures 4A,D). In particular, dB4 is positive for bHLH genes Neurog1 and Neurog2 (Diaz and Glover, 2021). Gene expression changes by migration later, following in part the information from neurons developing spinal and brainstem neurons (Lai et al., 2016; Hernandez-Miranda et al., 2017). Further work is needed to define the contribution of at least two earliest gene expressions (dB1, dB2) that originate along the longitudinal gene expression from r1-r8 (Diaz and Glover, 2021; Hirsch et al., 2021). In summary, at least two areas are defined to add distinct vestibular nuclei (Figures 3A,C,D) that indicate other origins, making it a complex migration from different neuronal populations (reviewed in Diaz and Glover, 2021).
Neurons from the thalamus project to the insula to provide vestibular information
Semicircular canal organs converge with otolithic organs to generate an end-organ-specific central organization that is incompletely presented in overlapping and distinct functional inputs (Straka et al., 2014; Elliott and Straka, 2022). Considerable overlap of vestibular end-organs precludes defining a classical sensory map as the organizational principle (Chagnaud et al., 2017). Three major 2o order output fibers are determined from the vestibular nuclei:
1. The LVN gives rise to the ipsilateral projection to the spinal cord motoneurons via the lateral vestibulospinal tract (LVST).
2. The MVN, SVN, and DVN project bilaterally to the ocular motoneurons (III, IV, and VI).
3. Additional fibers project to the lateral mammillary and dorsal tegmental nucleus to extend via the anterodorsal and ventral posterior lateral thalamus (AND, VPL) to the parietal-insula vestibular (PIVC) and the ventral intraparietal cortex (VIP).
We concentrate on the thalamocortical interaction (Figure 4A), providing a limited discussion on the two motor outputs of the LVN to the spinal cord, as all other vestibular fibers project to the ocular motoneurons (see review Fritzsch et al., 2017; Glover, 2020; Elliott and Straka, 2022). Within this connection is the cerebellum that receives and emits fine control of movements and storage of velocity and activates visual-vestibular integration using the nucleus propositus hypoglossal and contacts with the parabrachial nucleus to deal with cardiovascular and respiratory control (Cullen et al., 2021; Dieterich and Brandt, 2021; Elliott and Straka, 2022). The vestibular-ocular reflex (VOR) provides active and passive head-body motion, whereas the optokinetic reflex (OKN) provides visuomotor responses (Figure 3A).
Vestibular projections are bilateral, primarily from the vestibular nuclei to the thalamus and cortex. Certain areas may project ipsilaterally and contralaterally to the cortex. It appears that certain stimuli project via ipsilateral connections to the extent of the PIVC in humans. However, other stimuli project bilaterally or even a dominant contralateral input that reaches across three different levels to innervate the cortex (Dieterich et al., 2017), showing an asymmetric interaction from vestibular nuclei (VN) followed by a dominant contralateral input that crosses back except for the thalamus (Dieterich and Brandt, 2018). At least three midline crossings are located: (1) at the level of the vestibular nuclei; (2) at the pontine level above the vestibular nuclei; (3) at the mesencephalic level (Dieterich and Brandt, 2021). Vestibular nuclei project via a relay to pass through the nucleus propositus hypoglossal (NPH) and reach the supragenual nucleus (SGNu) that plays a role in the head direction network (HDC; Cullen et al., 2021; Taube and Yoder, 2021). From here, projections expand to the anterior vestibular-thalamic pathway (ADN) in the thalamus, and, from there, it projects to the limbic and entorhinal cortex. In addition, vestibular projections project bilaterally to the ventral posterolateral nucleus (VPL) that innervates the parietal insula of the vestibular cortical area (PIVC). In addition to the parietal-insula vestibular area (PIVC), vital information is sent to the visual temporal Sylvian area (VTS) in the retroinsular cortex, the superior temporal gyrus (STG), the inferior parietal lobule (IPL), the anterior cingulum, the hippocampus, and area 6a. Posterolateral thalamic nuclei project bilaterally via VOPL, VPM, and VL (Dieterich et al., 2017) to end up in the PIVC, but some fibers from the brainstem can project directly to the PIVC. Overall, fiber tracking data in humans shows that both the VPL (crossed and uncrossed projections) and VP (uncrossed projections) thalamus serve as vestibular relay stations for brainstem projections to (or from) the vestibular cortex.
Moreover, the ventral intraparietal areas (VIP) play a role in the vestibular circuits, where they interact with somatosensory and visual information to interact with the optokinetic (reviewed in Dieterich and Brandt, 2021). In summary, consistent with the interactions from the brainstem, there is a bilateral projection from the vestibular nuclei to the cortex, except for the lack of crossing fibers in the thalamus. The cortical areas innervated are the parietal insula, limbic and entorhinal cortex, and contacts the premotor cortical areas.
Auditory nuclei depend on Atoh1
The expression of Atoh1 in r2-5 (Wang et al., 2005) is needed to develop the anterior-ventral, posterior-ventral, and dorsal cochlear nuclei (AVCN, PVCN, and DCN; Figures 4A–C). Within dA1 is the expression of the bHLH gene Olig3 and Lhx2/9, Barhl1/2, and Pou4f1 to differentiate cells into neurons (Hernandez-Miranda et al., 2017). Atoh1 expression depends on the choroid plexus formation to initiate Lmx1a/b to distinguish cochlear nuclei (Chizhikov et al., 2021). In addition, there is a loss of Wnt3a in Lmx1a/b double null mice (Elliott et al., 2021b). Furthermore, specific migration reaches out to add to dA1 and at least dA4 that depends on Ptf1a (Iskusnykh et al., 2016; Lipovsek et al., 2017; Hirsch et al., 2021). Cochlear nuclei are active before hair cells (Marrs and Spirou, 2012). Previous work showed that the SGN central projection typically develops in the absence of Atoh1 and, thus, lacks hair cells and cochlear nuclei (Elliott et al., 2017). Future differentiation will generate a large set of cochlear nuclei that must be traced from the earliest gene expression to establish into bushy, globular, spherical, stellate, and pyramidal neurons (Malmierca, 2015; Oertel and Cao, 2020). In addition, unipolar brush cells, granule cells, and fusiform cells develop in the DCN; each is uniquely positive for calbindin, calretinin, and parvalbumin (reviewed in Caspary and Llano, 2018; Elliott et al., 2022b).
In addition to the cochlear nuclei (Figures 5A–C), several neurons migrate more ventrally to develop mostly from r4 to generate the medial olivocochlear nuclei (MOCs; Farago et al., 2006; Di Bonito et al., 2017). Different projections innervate ipsi- and contralateral MOCs (Marrs et al., 2013; Lipovsek and Wingate, 2018; Kandler et al., 2020; Grothe, 2021). For example, the development of detailed innervation shows an exuberant innervation of the MNTB followed by the development of the calyx of Held with time (Holcomb et al., 2013) that may decline in density formation with age (Elliott et al., 2022b). SGN fibers end up in a tonotopic organization that follows a base to apex progression to the MOCs. Both time and intensity are used to identify the unique frequency for future interactions (Grothe, 2021; Kandler et al., 2021). Innervation shows a bilateral projection from each cochlear nuclei to reach the lateral lemniscus to extend to the inferior colliculi (Macova et al., 2019; Syka, 2020). In summary (Figure 5), Atoh1 is developing the three cochlear nuclei and designing the MOCs into distinct innervation to create massive calyx formations.
Inferior colliculi and medial geniculate bodies project to the auditory cortex
Ascending projections to the inferior colliculi (IC) are bilateral projections either directly from the cochlear nucleus or indirectly from the cochlear nuclei (AVCN, PVCN, and DCN) through the MOCs and the lateral lemniscus, all while maintaining the tonotopic organization (Malmierca, 2015; Macova et al., 2019; Syka, 2020). The IC projects from the unilateral medial geniculate ganglion (MGB) that have limited crossing fibers in the IC (Grothe, 2021). Limited data (Gurung and Fritzsch, 2004; Razak et al., 2009) shows the earliest projection that has a prominent expression of Neurod1 to the IC to project to the auditory nucleus (Malone et al., 2021). The central part of the IC is organized in a tonotopic organization that responds to both direct and indirect fibers (Malmierca, 2015; Budinger, 2020).
The MGB consists of several subareas, the major parts being the ventral (MGV), medial (MGM), and dorsal (MGD) divisions (Budinger, 2020). Its main ascending input, from the ventral nucleus of the medial geniculate body, provides connections that comprise only a fraction of the inputs to the auditory cortex (A1; ∼18%), where these ascending signals are integrated with feedback connections from ipsilateral (∼70%) and contralateral (∼10%) cortex (Malone et al., 2021). The MGV constitutes the tonotopic lemniscal part of the MGB (Figure 5A). The MGD and MGM are usually assigned to the largely non-tonotopically organized, non-lemniscal position (Malmierca, 2015; Budinger, 2020). MGB and IC receive direct input from the auditory cortex to influence information processing (Budinger, 2020; King, 2020). In summary, a bilateral relay from the IC is mostly unilateral to the MGB, which expands bilaterally in the auditory cortex.
Integration of sensory inputs
Within the cortex, inputs from the various sensory systems interact at a tertiary level with inputs from other systems to provide an integrated sensory experience. Cortical integration of somatosensory, taste, vestibular, and auditory systems with other sensory and motor systems will be discussed below. Unfortunately, much of the detailed description is presented in humans, with a few exceptions from other mammals. Moreover, the detailed description will require additional analysis to evaluate the distinct genes needed for the integrated perspective.
Somatosensory integration
Cortical somatosensory projections interact tertiarily with those from other sensory systems to form a cohesive perception (Hans et al., 2020). For example, multisensory integration depends on auditory, visual, olfactory, and somatosensory interaction (Meehan et al., 2017). Somatosensory neurons show integration properties like those of visual stimuli. Neural responses account for the perceived direction of the stimuli across all stimulus conditions tested, highlighting the somatosensory system, a vector average mechanism to compute tactile motion direction that bears striking similarities to its visual counterpart (Pei et al., 2011; Goodman and Bensmaia, 2021). In addition, the vibrissa information from the primary motor cortex (M1) modulates sensory processing in the primary somatosensory barrel cortex to provide interneurons in vivo that of somatostatin interneurons decreased during whisking, suggesting the firing rates during whisking depended on M1 activity (Figure 6A). A circuitry exists by which inputs from the motor cortex influence activity in the somatosensory cortex (Lee et al., 2013).
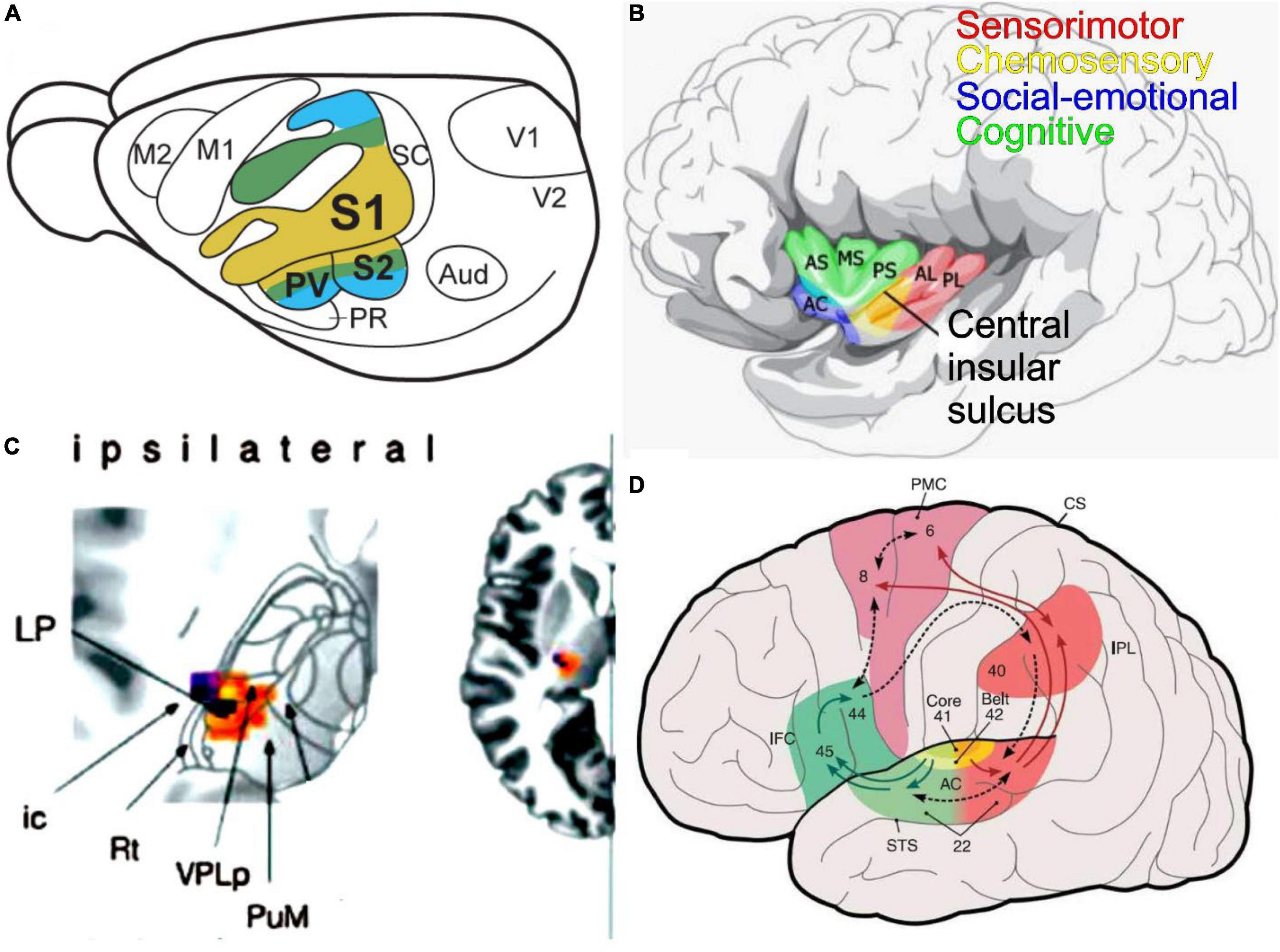
Figure 6. An integrated perspective of fur sensory inputs is presented. (A) Primary somatosensory (S1) has a topological projection that duplicates the secondary fibers (S2, PV)—taken from (Liao et al., 2020). (B) Within the insula are the sensorimotor, chemosensory, social-emotional, and cognitive interactions. AC, accessory gyrus; AL, anterior long insular gyrus; AS, anterior short insular gyrus; MS, middle short insular gyrus; PL, posterior long insular gyrus; PS, posterior short insular gyrus; VPPC, ventral posterior thalamic nucleus parvocellular. It was modified after (Zhang M. et al., 2020). (C) The ipsilateral inputs are shown to reach out via VPL to the cortex (insertion right). Modified after (Dieterich and Brandt, 2021). (D) The auditory cortex (AC, area 41, 42, 22) provides output to Brocca’s area (inferior frontal cortex, IFC; area 45, 46) for speech production, where it interacts with the premotor cortex (PMC, are 6, 8). Interaction is provided from the inferior parietal cortex (IPL, area 40) to interact with the superior temporal lobe (STS, area 22). Numbers provided by Brodmann. Taken from Rauschecker and Scott (2009).
A secondary somatosensory area (S2) and the ventral parietal area (PV) are organized mirror-symmetrically relative to the primary somatosensory area in humans. Bilateral spatiotemporal integration in S2/PV takes place over a sizeable cortical area and presents the properties of spatiotemporal integration in anterior parietal regions. Importantly, S2/PV showed significant temporal integration while spatial integration was reduced (Zhu et al., 2007; Caldwell et al., 2019).
A tactile and visual interactive response to activation of various brain regions shows the effects of visuotactile multisensory integration on the amount of brain activation in the somatosensory cortical regions. Tactile stimulation-induced cortical activations occur in the left primary sensory-motor cortex (SM1) and secondary somatosensory cortex (S2). In the visuotactile stimulation task, cortical activations can happen in both primary S1 and secondary S2 and can reach the posterior parietal cortex by activation (Kwon et al., 2017). A visual and somatosensory input is reliable, reducing reliance on the vestibular system. Postural training can alter sensory organization after a visual feedback-vestibular activation training protocol, suggesting a possible sensory reweighting through vestibular adaptation and/or habituation occurs (Appiah-Kubi and Wright, 2019) that helps indicate with the somatosensory input, in particular the tendon of the limbs in humans (Peterka and Loughlin, 2004). Multisensory work in rodents suggested a segregation of mechanically driven, temperature-selective and gustatory responses that provide stimuli in the oral somatosensory cortex (Clemens et al., 2018). Furthermore, the mid-dorsal insula and the primary and secondary somatosensory cortex suggest that it involves a specific set of brain regions of the oral-sensory integration processes (Wistehube et al., 2018).
Beyond a hierarchical model of multisensory integration, a unimodal input converges onto higher multisensory areas that integrate multimodal information and guide decision-making and behavior. The superior temporal sulcus, lateral occipital–temporal cortex, posterior parietal cortex, and ventrolateral frontal cortex have been implicated in higher cortical multisensory processing (Meehan et al., 2017; Zhou et al., 2019). The involvement of primary sensory areas in multisensory processing indicates a multisensory integration that may involve more distributed neural networks beyond classic hierarchical multisensory-specific areas, including primary sensory cortices of the somatosensory input, including the associated somatosensory information (Brunert and Rothermel, 2020).
Somatosensory-auditory integrations
In the mouse, primary (S1) and secondary (S2) somatosensory cortices pair sound with whisker stimulation, modulating tactile responses in both S1 and S2. After local mutually inhibitory S2 circuit can spectrally select tactile versus auditory inputs suggesting a multisensory integration that provides a key role for S2 in combining auditory and tactile information (Zhang M. et al., 2020), Magnetoencephalography can be used to study auditory stimulation by cortical activity in the primary somatosensory cortex. Somatosensory–acoustic interaction reaches area 3b from the thalamus during the early stages of synaptic transmission (Sugiyama et al., 2018).
Somatosensory-vestibular interactions
Vestibular stimulation can speed tactile detection and indicates vestibular facilitation of somatosensory processing (Pfeiffer et al., 2018). Specifically, somatosensory- vestibular interactions affect the perceived timing of tactile stimuli (Moro and Harris, 2018). Caloric vestibular stimulation can result in somatosensory stimulation (Bretas et al., 2020) that can be combined with vestibular and postural training to improve balance in healthy young adults (Appiah-Kubi and Wright, 2019; Ferrè and Haggard, 2020). Area 3a extends from the somatosensory cortex and reaches the superior parietal lobule of the parietal-insula vestibular area (PIVC), and the extrastriate regions (Shaikh et al., 2020).
Somatosensory-taste interactions
Cross-modal correspondences occur between tastes and the somatosensory attributions of food and beverage products (Spence and Ngo, 2012). Beyond cross-modal integration occurs with somatosensory with sweet tastes (Spence, 2022). Results show that flavor perception must affect somatosensory stimuli as components of flavor perception and taste modulators (Green and Nachtigal, 2012). In mice, ascending projections from trigeminal neurons reach the parabrachial nucleus (PB). Trigeminal fibers use TRPV1 and TRPA1 to play a role in thermosensation and pain communication with parabrachial taste neurons (Li et al., 2021; Rhyu et al., 2021). In summary, mammals’ somatosensory cortical regions (S1, S2, and PV) have integrated with the motor cortex and visual, auditory, vestibular, taste, and olfactory cortical areas.
Conclusion of somatosensory inputs
Trigeminal input (Figures 2A, 6A) spans the spinal cord through the hindbrain to organize a topological input (leg > arm > face). Fibers reach the medial lemniscus, which projects to the VPL/VPM to innervate the principal (S1) and secondary somatosensory fibers (S2, PV). A tertiary interaction is evident in the motor cortex and has visual, auditory, vestibular, olfactory, and taste interactions to generate a cohesive perception. Further work is needed to detail the various sensory inputs to coordinate with the trigeminal primary (S1) and secondary (S2, PV) into a cohesive interaction.
Taste integration
The solitary tract (ST) innervates, directly and indirectly, the parabrachial nuclei (PB, Figure 3A), from which primarily ipsilateral fibers extend the thalamus in mice (Lundy and Norgren, 2015; Gasparini et al., 2021). Neurons also directly reach the thalamus from ST to reach the gustatory and taste relay, referred to as ventral posterior thalamic nucleus parvocellular (VPPC). The unique ipsilateral connection and, to a lesser extent, the contralateral PB reach the insular cortex, which develops a reciprocal connection with gustatory and visceral afferents. The insula functions from sensory processing to representing feelings and emotions, autonomical and motor control, risk prediction and decision-making, bodily- and self-awareness, and complex social functions like empathy (Gogolla, 2017). Moreover, the insula subserves various human functions ranging from sensory and affective processing to high-level cognition (Uddin et al., 2017).
Beyond function, the loss of the right insula affects ipsilateral taste recognition, whereas the left-hemispheric stroke patients’ taste recognition on the right side of the tongue suggests that taste information from both sides of the tongue passes through the left insula (Pritchard et al., 1999). The insular cortex may be organized into a hedonic or “viscerotropic” map rather than one ordered according to taste quality. In contrast to a spatial map ordered by taste quality, the insular cortex interacts with other sensory inputs, integrating taste, somatosensation, and olfaction into a cohesive experience of food intake (Shepherd, 2006; Schier and Spector, 2019). Once it reaches the mouth, the taste, texture, and retronasal smell of the food deliver sensations of olfaction and taste (Figure 5B). The cortical presentation of both spatial and temporal divisions of taste coding changes with experience. Cortical taste representation is not organized in a spatially discrete map but instead is plastic and spatially dispersed that uses temporal information to encode many types of ingested stimuli (Staszko et al., 2020).
In contrast to visual, auditory, and somatosensory cortices that are topographically organized, the spatial organization of taste responses in the gustatory insular cortex responses shows no sign of spatial clustering or topography. Thus, the idea that taste qualities in the insula are sparse and distributed is analogous to the representation of odorants in the piriform cortex (Chen et al., 2021). In humans, recent studies do not support the topographic model of taste quality representation, but rather taste quality is a distributed pattern within gustatory regions of the insula (Avery, 2021). Several indications suggest that information about taste stimuli is more profound when paired with odors than when presented alone (Lorenzo, 2021). These data show that a lack of direct projections from the olfactory bulb or piriform cortex may be conveyed via the gustatory cortex, amygdala, or lateral hypothalamus, suggesting a convergence of information of olfaction and taste (Lorenzo, 2021).
Four functionally distinct regions have been identified in the human insula (Figure 5B): (1) a sensorimotor region located in the mid-posterior insula; (2) a central olfactory-gustatory region; (3) a socio-emotional region in the anterior-ventral insula; and (4) a cognitive anterior-dorsal region (Uddin et al., 2017). Trigeminal and olfactory input converges and integrates within the insular region (Hummel et al., 2009) which is expanded for spicy stimulations in humans (Han et al., 2021; Spence et al., 2021). Somatosensory manifestations represent a large proportion of responses elicited by electrical stimulation of the insular cortex in humans. Likewise, input from the auditory system and the parieto-insular vestibular cortex reach the insular cortex. The posterior granular insula in monkeys integrates vestibular inputs and proprioceptive, visual motion, and auditory activities comparable to humans (Evrard, 2019). However, an interaction between auditory, speech influence, and vestibular integration with taste input is unclear (Uddin et al., 2017). A poly-modal integration likely contributes to “higher” vestibular functions, including self-motion perception in a body- (proprioceptive) and the world- (audio-visual) centered referential system that could be organized into a dorsoventral gradient (Evrard, 2019).
Taste-auditory integrations
Tasting in the presence of auditory stimuli of various frequencies or silence suggests that low frequencies increase red wine’s perception and aromatic intensity (Crisinel and Spence, 2009; Burzynska et al., 2019). Moreover, cross-modal interactions can affect the background music on the drink’s taste (Hauck and Hecht, 2019).
Taste-vestibular interactions
Vestibular stimulation can speed tactile detection and indicates vestibular facilitation of somatosensory processing (Pfeiffer et al., 2018). Caloric vestibular stimulation can result in somatosensory stimulation (Bretas et al., 2020) combined with vestibular and postural training (Appiah-Kubi and Wright, 2019; Ferrè and Haggard, 2020).
Taste-somatosensory interactions
Cross-modal correspondences between taste and the somatosensory attributions of food and beverage products (Spence and Ngo, 2012). Beyond cross-modal integration with somatosensory in particular, sweet flavors (Spence, 2022) that connect to drinking have unclear interactions (Green and Nachtigal, 2012).
Conclusion of taste inputs
The taste bud projection (Figures 3A, 6B) from the three visceral ganglia reaches r4-11 to form the solitary tract. Fibers reach from the ST to the PB and go bilaterally to the VPPC to innervate the insula. To generate a cohesive perception, a tertiary interaction is limited to somatosensory, olfactory, auditory, and vestibular interactions.
Vestibular integration
In monkeys, the PIVC seems to be the dominant multi-modal vestibular cortical area, which is closely connected to the other regions, the vestibular nucleus, and to the opposite hemisphere (Figure 4A). Neurons are focused on VPL and ADN but have additional projections from MVN and SVN (Dieterich and Brandt, 2018). The thalamus is multimodal and encodes vestibular signals to project somatosensory, proprioceptive, visual, and auditory sensory information (Figure 4A). From here, the VPL is important as multisensory inputs to have a perceptual perception of head and body motion that are directly innervating the parietal-insula of the vestibular cortical area (PIVC) and the ventral intraparietal areas (VIP; Cullen, 2019). A strong connection exists with 3a and 2v that interacts with multiple connections to play a role in the frontal eye field. Notable inputs are the medial superior temporal area that plays a role in visual motion that interacts with vestibular and visual stimulation (Cullen, 2019). Without any doubt, the visual-vestibular interactions are directly and indirectly involved in optokinetic stimulation and caloric irrigation in normal and partial deletion of various regions (Dieterich and Brandt, 2019; Cullen et al., 2021). Two areas, the lateral entorhinal and postrhinal cortex contain center-bearing and center-distance cells (Figure 4C), which discharge based on the animal’s orientation and distance to the center of its environment (Taube and Yoder, 2021). Going beyond the visual/vestibular interactions, we provide an overview of somatosensory, taste, and auditory integration. Because this vestibular cortical network is so widely distributed, it could impact multiple neurocognitive functions in health and disease. The most impact is dependent on Caloric Vestibular Stimulation (CVS) or Galvanic Vestibular Stimulation (GVS) to cause an integrated perspective (Ferrè and Haggard, 2020).
Vestibular-somatosensory interactions
Following the peripersonal space can be used to define upper limbs for vestibular signals (Pfeiffer et al., 2018). For example, vestibular stimulation can speed tactile detection and indicates vestibular facilitation of somatosensory processing, suggesting that vestibular inputs dynamically interact with multisensory input (Pfeiffer et al., 2018). Caloric vestibular stimulation can result in somatosensory activity (Bretas et al., 2020) that can be activated after somatosensory reduction and can combine vestibular and postural training to improve balance in healthy young adults and likely in those with deficits (Appiah-Kubi and Wright, 2019; Ferrè and Haggard, 2020).
Vestibular-taste interactions
Pairing a novel taste with an offensive vestibular stimulation result in conditioned taste aversions in rats and humans. Vestibular system involvement in gustatory conditioning was compared in sham-lesioned or labyrinth ectomized rats with control rats for oral rejection reactions (Ossenkopp et al., 2003). Previous work showed that vestibular input could influence several aspects of consumer behavior to affect food taste (Biswas et al., 2019). Robust taste aversions can be solicited in rats and mice affected after the bilateral loss of labyrinth function, suggesting a direct interaction of the magnet on the vestibular system in Het mutant mice (Cote, 2020).
Vestibular-auditory interactions
Rhythm can be biased by passive motion, which suggests that vestibular input may play a vital role in the effect of movement on auditory rhythm processing (Phillips-Silver and Trainor, 2008; Phillips-Silver et al., 2020). Recent evidence suggests that simultaneous auditory–vestibular training facilitates short-term auditory plasticity, producing stronger oscillator connections in the acoustic network (Tichko et al., 2021). Most importantly, a combined vestibular and cochlear prosthesis may restore hearing and balance in patients who have lost both (Phillips et al., 2020). A significant interaction between different sensory modalities during stimulation with a combined vestibular and cochlear prosthesis can help challenge stimulation strategies to simultaneously restore auditory and vestibular function after such an implant (Phillips et al., 2020).
Conclusion of vestibular inputs
Vestibular information (Figures 4A, 6C) is sent from the periphery to four vestibular nuclei (spanning r1-r8) along vestibular sensory neurons. Ascending projections are bilateral to innervate the ventral posterolateral nucleus (VPL) and anterior vestibular-thalamic pathway (ADN) to the parietal insula of the vestibular cortical area (PIVC) and the ventral intraparietal regions (VIP). Higher-order integration beyond the demonstrated visual-vestibular interaction is limited to somatosensory, taste, and, importantly, auditory-vestibular interactions.
Auditory integration
The auditory cortex (A1) is a central hub located at a pivotal position within the auditory system, receiving a tonotopic input from the medial geniculate body (MGB) and playing a role in the sensation, perception (Figure 5A), and interpretation of the acoustic environment (Malone et al., 2021). ‘Receptive fields’ (RF) end up a particular stimulus dimension: the choice of acoustic stimulus dimensions to explore are related to the basic perceptual attributes such as spectral pitch (frequency), loudness (intensity), periodicity, or virtual pitch (amplitude modulations, harmonic series), timbre (spectral envelope), and sound location (interaural time, level differences, and spectral shape). In humans, hemispheric asymmetries between the left and suitable auditory cortex form in gross anatomical features and cortical microcircuitry (Budinger, 2020; Rauschecker, 2021).
There are two major classes of neurons in the neocortex: principal cells and interneurons. The auditory cortex is organized into six horizontal layers, another defining feature of the neocortex. It has anatomical and functional vertical columns and has intense interhemispheric connections between the auditory cortices of both hemispheres, which cross the midline via the (caudal body of the) corpus callosum (Budinger, 2020; Malone et al., 2021). Primary sensory cortices like A1, S1 (somatosensory), and V1(visual) are not purely unimodal but also process other non-matched sensory and non-sensory information. Projections from V1 and, to a lesser degree, from S1 toward A1 mainly arise from subgranular layers and provide feedforward organizations (Budinger, 2020).
The auditory corticobulbar system includes projections from the nuclei of the medial geniculate body (MGB), the inferior colliculi (IC), the lateral lemniscus (NLL), superior olivary complex (SOC), and the cochlear nuclear complex (CN) including the medial olivary efferents that project out to the outer hair cells (OHCs; Simmons et al., 2011; King, 2020). The prominent corticothalamic, corticocollicular, colliculofugal, and olivocochlear connections descend from auditory pathways that include long-range connections (Malmierca, 2015; Budinger, 2020; King, 2020). Overall, the feedback loop might modulate auditory response properties of neurons in the midbrain and hindbrain, adapting their sensitivity to sound frequency, intensity, and location (Budinger, 2020; King, 2020).
As previously mentioned, the auditory cortex interacts with multiple other sensory projections as tertiary fibers (Figure 5D). For example, auditory soundscape or visual landscape can influence the perception in a real, multisensory environment (Pheasant et al., 2010; Zhao et al., 2018). An interesting McGurke effect showed an interaction between visual and auditory stimuli, highlighting a higher interaction of otherwise discrete stimulations (Spence and Soto-Faraco, 2010). Most interestingly, multisensory interactions are audiovisual speech perception, in which visual speech substantially enhances auditory speech processes (Plass et al., 2020) that be more robust in audio-visual speech responses than what would have been expected from the summation of the audio and visual speech responses, suggesting that multisensory integration occurs (O’Sullivan et al., 2021).
Auditory-somatosensory interactions
Typical, auditory-somatosensory (AS) humans can multisensory stimulus pairs yield significant reaction time facilitation relative to their unisensory counterparts that exceeded probability (Murray et al., 2005). Multisensory interactions across space suggest perceptual-cognitive phenomena of sensory-cognitive processing (Foxe et al., 2002). Further, integration of auditory and somatosensory information in speech processing can be used in a bimodal perceptual task, suggesting somatosensory information on sound categorization can affect adults more than children (Trudeau-Fisette et al., 2019). This results in somatosensory cross-modal reorganization of the auditory cortex in adults with early stage, mild-moderate age-related hearing loss [ARHL (Cardon and Sharma, 2018)]. Pairing sound with whisker stimulation modulates tactile responses in both S1 and S2, with the most prominent modulation being robust inhibition in S2 (Zhang M. et al., 2020). Mutually inhibitory activation from the S2 circuit can spectrally select tactile versus auditory inputs in mice (Zhang M. et al., 2020).
Auditory-taste interactions
In contrast to vision and somatosensory interaction, very few examples exist supporting some level of interaction between the auditory and taste systems (Keast and Breslin, 2003; Zampini and Spence, 2012). The strongest is provided through a cross interaction between taste and auditory pitch (Holt-Hansen, 1968; Spence, 2011). Most recent evidence suggests an attenuation of taste neophobia induced by taste familiarity and is auditory context-dependent in mice (Grau-Perales and Gallo, 2020). Context dependency involved dopaminergic activity mediated by D1 receptors, which might be responsible for adequately acquiring safe taste recognition memory (Grau-Perales et al., 2021). In humans, associations between auditory attributes and a number of the commonly agreed basic tastes are now recently added as ‘saltiness’ (Wang et al., 2021), among other interactions of taste with auditory interactions (Spence et al., 2021).
Auditory-vestibular interactions
Since rhythm can be biased by passive motion, it suggests that vestibular input may play a vital role in the effect of movement on auditory rhythm processing (Phillips-Silver and Trainor, 2008; Phillips-Silver et al., 2020). Recent evidence suggests that simultaneous auditory-vestibular training facilitates short-term auditory plasticity, producing stronger oscillator connections in the auditory network (Tichko et al., 2021). Most importantly, it can be combined in a vestibular and cochlear prosthesis to restore hearing and balance in patients who have lost both (Phillips et al., 2020). A significant interaction between different sensory modalities during stimulation with a combined vestibular and cochlear prosthesis can help challenge stimulation strategies to simultaneously restore auditory and vestibular function after such an implant (Phillips et al., 2020).
Conclusion on auditory integration
Cochlear spiral ganglia (Figures 5A, 6D) are unique compared to the nearby vestibular ganglia and project tonotopically to innervate the cochlear nuclei that form from r2-5. A notable development migrates from the cochlear nuclei (r4) to receive order projections. Projections are bilateral to innervate via IC and MGB to innervate the A1. Higher-order auditory interactions are limited to somatosensory, taste, and, importantly, the auditory-vestibular interactions.
A combined perspective of the four brainstem sensory systems
The peripheral sensory innervation depends on unique regional cell types (Merkel cells, free endings, taste buds, and mechanosensory hair cells). Trigeminal, vestibular, auditory and taste neurons depend on the bHLH genes, Neurog1/2. Central organization depends on Atoh1 (dA1) for auditory, Phox2b/Tbx3 (dA3) for taste, Phox2b/Ptf1a (dB1/2) for vestibular and Ascl1/Neurog1 (dB3/4) for trigeminal to integrate through migration into the distinct brainstem organization. Output is prominent mainly to the contralateral thalamus (trigeminal), ipsilateral via an intermediate (PB, taste) to the thalamus, bilaterally through the midbrain intermediate to the thalamus in the vestibular, and bilaterally from the auditory a secondary (SOC, LL) to the tertiary (IC) and the thalamus (MGB). From here, a topological organization projects to the somatosensory (S1) and the auditory telencephalon (A1), whereas the taste (insula) and vestibular (VPL, PIVC) are not organized topologically. Higher-order interactions are demonstrated between trigeminal and auditory, between taste and vestibular, between the auditory and the vestibular, and between taste and trigeminal to integrate the cohesive perception of the telencephalon mostly documented in human.
Data availability statement
The original contributions presented in this study are included in the article/supplementary material, further inquiries can be directed to the corresponding author.
Author contributions
All authors listed have made a substantial, direct, and intellectual contribution to the work, and approved it for publication.
Acknowledgments
We thank members for their comments on this manuscript. Grants to EY and BF supported this work from the National Institutes of Health (DC016099, DC015252, DC015135, AG060504, and AG051443).
Conflict of interest
The authors declare that the research was conducted in the absence of any commercial or financial relationships that could be construed as a potential conflict of interest.
Publisher’s note
All claims expressed in this article are solely those of the authors and do not necessarily represent those of their affiliated organizations, or those of the publisher, the editors and the reviewers. Any product that may be evaluated in this article, or claim that may be made by its manufacturer, is not guaranteed or endorsed by the publisher.
References
Abraira, V. E., and Ginty, D. D. (2013). The sensory neurons of touch. Neuron 79, 618–639. doi: 10.1016/j.neuron.2013.07.051
Alsina, B. (2020). Mechanisms of cell specification and differentiation in vertebrate cranial sensory systems. Curr. Opin. Cell Biol. 67, 79–85. doi: 10.1016/j.ceb.2020.08.006
Andrew, D. L. E., May, P. J., and Warren, S. (2020). Morphologic characterization of trigeminothalamic terminal arbors arising from the principal nucleus in the macaque. Front. Neuroanat. 14:562673. doi: 10.3389/fnana.2020.562673
Antón-Bolaños, N., Sempere-Ferràndez, A., Guillamón-Vivancos, T., Martini, F. J., Pérez-Saiz, L., Gezelius, H., et al. (2019). Prenatal activity from thalamic neurons governs the emergence of functional cortical maps in mice. Science 364, 987–990. doi: 10.1126/science.aav7617
Appiah-Kubi, K. O., and Wright, W. (2019). Vestibular training promotes adaptation of multisensory integration in postural control. Gait Posture 73, 215–220. doi: 10.1016/j.gaitpost.2019.07.197
Arimura, N., Dewa, K. I., Okada, M., Yanagawa, Y., Taya, S. I., and Hoshino, M. (2019). Comprehensive and cell-type-based characterization of the dorsal midbrain during development. Genes Cells 24, 41–59. doi: 10.1111/gtc.12656
Avery, J. A. (2021). Against gustotopic representation in the human brain: There is no Cartesian restaurant. Curr. Opin. Physiol. 20, 23–28. doi: 10.1016/j.cophys.2021.01.005
Avery, J. A., Liu, A. G., Ingeholm, J. E., Riddell, C. D., Gotts, S. J., and Martin, A. (2020). Taste quality representation in the human brain. J. Neurosci. 40, 1042–1052. doi: 10.1523/JNEUROSCI.1751-19.2019
Balmer, T. S., and Trussell, L. O. (2019). Selective targeting of unipolar brush cell subtypes by cerebellar mossy fibers. eLife 8:e44964. doi: 10.7554/eLife.44964
Bermingham, N. A., Hassan, B. A., Price, S. D., Vollrath, M. A., Ben-Arie, N., Eatock, R. A., et al. (1999). Math1: An essential gene for the generation of inner ear hair cells. Science 284, 1837–1841. doi: 10.1126/science.284.5421.1837
Bermingham, N. A., Hassan, B. A., Wang, V. Y., Fernandez, M., Banfi, S., Bellen, H. J., et al. (2001). Proprioceptor pathway development is dependent on Math1. Neuron 30, 411–422. doi: 10.1016/s0896-6273(01)00305-1
Bigiani, M. (2021). “Salt taste,” in The senses, ed. B. Fritzsch (Amsterdam: Elsevier), 247–263. doi: 10.1016/B978-0-12-809324-5.23910-2
Birol, O., Ohyama, T., Edlund, R. K., Drakou, K., Georgiades, P., and Groves, A. K. (2016). The mouse Foxi3 transcription factor is necessary for the development of posterior placodes. Dev. Biol. 409, 139–151. doi: 10.1016/j.ydbio.2015.09.022
Biswas, D., Szocs, C., and Abell, A. (2019). Extending the boundaries of sensory marketing and examining the sixth sensory system: Effects of vestibular sensations for sitting versus standing postures on food taste perception. J. Consum. Res. 46, 708–724. doi: 10.1093/jcr/ucz018
Bouchard, M., de Caprona, D., Busslinger, M., Xu, P., and Fritzsch, B. (2010). Pax2 and Pax8 cooperate in mouse inner ear morphogenesis and innervation. BMC Dev. Biol. 10:89. doi: 10.1186/1471-213X-10-89
Bretas, R. V., Taoka, M., Suzuki, H., and Iriki, A. (2020). Secondary somatosensory cortex of primates: Beyond body maps, toward conscious self-in-the-world maps. Exp. Brain Res. 238, 259–272. doi: 10.1007/s00221-020-05727-9
Briscoe, S. D., and Ragsdale, C. W. (2019). Evolution of the chordate telencephalon. Curr. Biol. 29, R647–R662. doi: 10.1016/j.cub.2019.05.026
Brunert, D., and Rothermel, M. (2020). Cortical multisensory integration—a special role of the agranular insular cortex? Pflügers Arch. 472, 671–672. doi: 10.1007/s00424-020-02400-6
Budinger, E. (2020). “Primary auditory cortex and the thalamo-cortico-thalamic circuitry,” in The senses, ed. B. Fritzsch doi: 10.1016/B978-0-12-805408-6.00022-1 (Amsterdam: Elsevier), 623–656.
Burzynska, J., Wang, Q. J., Spence, C., and Bastian, S. E. P. (2019). Taste the bass: Low frequencies increase the perception of body and aromatic intensity in red wine. Multisens. Res. 32, 429–454. doi: 10.1163/22134808-20191406
Caldwell, D. J., Cronin, J. A., Wu, J., Weaver, K. E., Ko, A. L., Rao, R. P., et al. (2019). Direct stimulation of somatosensory cortex results in slower reaction times compared to peripheral touch in humans. Sci. Rep. 9:3292. doi: 10.1038/s41598-019-38619-2
Canna, A., Prinster, A., Cantone, E., Ponticorvo, S., Russo, A. G., Di Salle, F., et al. (2019). Intensity-related distribution of sweet and bitter taste fMRI responses in the insular cortex. Hum. Brain Mapp. 40, 3631–3646. doi: 10.1002/hbm.24621
Cardon, G., and Sharma, A. (2018). Somatosensory cross-modal reorganization in adults with age-related, early-stage hearing loss. Front. Hum. Neurosci. 12:172. doi: 10.3389/fnhum.2018.00172
Caspary, D. M., and Llano, D. A. (2018). “Aging processes in the subcortical auditory system,” in The oxford handbook of the auditory brainstem, ed. K. Kandler (Oxford: Oxford University Press), 639–679.
Chagnaud, B. P., Engelmann, J., Fritzsch, B., Glover, J. C., and Straka, H. (2017). Sensing external and self-motion with hair cells: A comparison of the lateral line and vestibular systems from a developmental and evolutionary perspective. Brain Behav. Evol. 90, 98–116. doi: 10.1159/000456646
Chen, K., Kogan, J. F., and Fontanini, A. (2021). Spatially distributed representation of taste quality in the gustatory insular cortex of behaving mice. Curr. Biol. 31, 247–256.e4. doi: 10.1016/j.cub.2020.10.014
Chen, X., Gabitto, M., Peng, Y., Ryba, N. J., and Zuker, C. S. (2011). A gustotopic map of taste qualities in the mammalian brain. Science 333, 1262–1266. doi: 10.1126/science.1204076
Chen, Y., Takano-Maruyama, M., Fritzsch, B., and Gaufo, G. O. (2012). Hoxb1 controls anteroposterior identity of vestibular projection neurons. PLoS One 7:e34762. doi: 10.1371/journal.pone.0034762
Chessum, L., Matern, M. S., Kelly, M. C., Johnson, S. L., Ogawa, Y., Milon, B., et al. (2018). Helios is a key transcriptional regulator of outer hair cell maturation. Nature 563, 696–700. doi: 10.1038/s41586-018-0728-4
Chizhikov, V. V., Iskusnykh, I. Y., Fattakhov, N., and Fritzsch, B. (2021). Lmx1a and Lmx1b are redundantly required for the development of multiple components of the mammalian auditory system. Neuroscience 452, 247–264. doi: 10.1016/j.neuroscience.2020.11.013
Chou, X. L., Fang, Q., Yan, L., Zhong, W., Peng, B., Li, H., et al. (2020). Contextual and cross-modality modulation of auditory cortical processing through pulvinar mediated suppression. eLife 9:e54157. doi: 10.7554/eLife.54157
Clemens, A. M., Delgado, Y. F., Mehlman, M. L., Mishra, P., and Brecht, M. (2018). Multisensory and motor representations in rat oral somatosensory cortex. Sci. Rep. 8:13556. doi: 10.1038/s41598-018-31710-0
Cote, J. (2020). Het mouse model suggests vestibular system mediates magnetic field effects. Biophys. J. 118:613a. doi: 10.1016/j.bpj.2019.11.3313
Crisinel, A.-S., and Spence, C. (2009). Implicit association between basic tastes and pitch. Neurosci. Lett. 464, 39–42. doi: 10.1016/j.neulet.2009.08.016
Cullen, K. E. (2019). Vestibular processing during natural self-motion: Implications for perception and action. Nat. Rev. Neurosci. 20, 346–363. doi: 10.1038/s41583-019-0153-1
Cullen, K. E., Zobeini, O. A., Woboonsaksakul, K. P., Wang, L., Stanley, O. R., Leavitt, O. M. E., et al. (2021). “Self-motion,” in The senses, ed. B. Fritzsch doi: 10.1016/B978-0-12-809324-5.24178-3 (Amsterdam: Elsevier), 483–495.
Daghfous, G., Auclair, F., Blumenthal, F., Suntres, T., Lamarre-Bourret, J., Mansouri, M., et al. (2020). Sensory cutaneous papillae in the sea lamprey (Petromyzon marinus L.): I. Neuroanatomy and physiology. J. Comp. Neurol. 528, 664–686. doi: 10.1002/cne.24787
D’Autréaux, F., Coppola, E., Hirsch, M. R., Birchmeier, C., and Brunet, J. F. (2011). Homeoprotein Phox2b commands a somatic-to-visceral switch in cranial sensory pathways. Proc. Natl. Acad. Sci. U.S.A. 108, 20018–20023. doi: 10.1073/pnas.1110416108
Dennis, D. J., Han, S., and Schuurmans, C. (2019). bHLH transcription factors in neural development, disease, and reprogramming. Brain Res. 1705, 48–65. doi: 10.1016/j.brainres.2018.03.013
Desai, S. S., Zeh, C., and Lysakowski, A. (2005). Comparative morphology of rodent vestibular periphery. I. Saccular and utricular maculae. J. Neurophysiol. 93, 251–266.
Di Bonito, M., Studer, M., and Puelles, L. (2017). Nuclear derivatives and axonal projections originating from rhombomere 4 in the mouse hindbrain. Brain Struct. Funct. 222, 3509–3542. doi: 10.1007/s00429-017-1416-0
Diaz, C., and Glover, J. C. (2021). The vestibular column in the mouse: A rhombomeric perspective. Front. Neuroanat. 15:806815. doi: 10.3389/fnana.2021.806815
Dieterich, M., and Brandt, T. (2018). Global orientation in space and the lateralization of brain functions. Curr. Opin. Neurol. 31, 96–104. doi: 10.1097/WCO.0000000000000516
Dieterich, M., and Brandt, T. (2019). Perception of verticality and vestibular disorders of balance and falls. Front. Neurol. 10:172. doi: 10.3389/fneur.2019.00172
Dieterich, M., and Brandt, T. (2021). “Structural and functional imaging of the human bilateral vestibular network from the brainstem to the cortical hemispheres,” in The senses, ed. B. Fritzsch (Amsterdam: Elsevier), 414–431. doi: 10.1016/B978-0-12-809324-5.23886-8
Dieterich, M., Kirsch, V., and Brandt, T. (2017). Right-sided dominance of the bilateral vestibular system in the upper brainstem and thalamus. J. Neurol. 264, 55–62. doi: 10.1007/s00415-017-8453-8
Duncan, J. S., and Cox, B. C. (2020). “Anatomy and development of the inner ear,” in Reference module in neuroscience and biobehavioral psychology, ed. J. Stein doi: 10.1016/B978-0-12-809324-5.24161-8 (Amsterdam: Elsevier).
Duncan, J. S., and Fritzsch, B. (2013). Continued expression of GATA3 is necessary for cochlear neurosensory development. PLoS One 8:e62046. doi: 10.1371/journal.pone.0062046
Duncan, J. S., Fritzsch, B., Houston, D. W., Ketchum, E. M., Kersigo, J., Deans, M. R., et al. (2019). Topologically correct central projections of tetrapod inner ear afferents require Fzd3. Sci. Rep. 9:10298. doi: 10.1038/s41598-019-46553-6
Dvorakova, M., Macova, I., Bohuslavova, R., Anderova, M., Fritzsch, B., and Pavlinkova, G. (2020). Early ear neuronal development, but not olfactory or lens development, can proceed without SOX2. Dev. Biol. 457, 43–56. doi: 10.1016/j.ydbio.2019.09.003
Dvoryanchikov, G., Hernandez, D., Roebber, J. K., Hill, D. L., Roper, S. D., and Chaudhari, N. (2017). Transcriptomes and neurotransmitter profiles of classes of gustatory and somatosensory neurons in the geniculate ganglion. Nat. Commun. 8:760. doi: 10.1038/s41467-017-01095-1
Elliott, K. L., Sokolowski, B., Yamoah, E. N., and Fritzsch, B. (2022a). An integrated perspective of commonalities and differences across sensory receptors and their distinct inputs. doi: 10.1201/9781003092810-10 Boca Raton, FL: Wiley.
Elliott, K. L., Fritzsch, B., Yamoah, E. N., and Zine, A. (2022b). Age-related hearing loss: Sensory and neural etiology and their interdependence. Front. Aging Neurosci. 14:814528. doi: 10.3389/fnagi.2022.814528
Elliott, K. L., and Gordy, C. (2020). “Ear vestibular neurosensory development,” in The senses, ed. B. Fritzsch doi: 10.1016/B978-0-12-809324-5.24141-2 (Amsterdam: Elsevier), 145–161.
Elliott, K. L., Kersigo, J., Lee, J. H., Jahan, I., Pavlinkova, G., Fritzsch, B., et al. (2021a). Developmental changes in peripherin-eGFP expression in spiral ganglion neurons. Front. Cell. Neurosci. 15:678113. doi: 10.3389/fncel.2021.678113
Elliott, K. L., Pavlínková, G., Chizhikov, V. V., Yamoah, E. N., and Fritzsch, B. (2021b). Development in the mammalian auditory system depends on transcription factors. Int. J. Mol. Sci. 22:4189.
Elliott, K. L., Kersigo, J., Pan, N., Jahan, I., and Fritzsch, B. (2017). Spiral ganglion neuron projection development to the hindbrain in mice lacking peripheral and/or central target differentiation. Front. Neural Circuits 11:25. doi: 10.3389/fncir.2017.00025
Elliott, K. L., and Straka, H. (2022). Assembly and functional organization of the vestibular system, eds B. Fritzsch and K. L. Elliott doi: 10.1201/9781003092810-6 (Boca Raton, FL: Wiley), 135–174.
Eng, S. R., Dykes, I. M., Lanier, J., Fedtsova, N., and Turner, E. E. (2007). POU-domain factor Brn3a regulates both distinct and common programs of gene expression in the spinal and trigeminal sensory ganglia. Neural Develop. 2:3. doi: 10.1186/1749-8104-2-3
Erives, A., and Fritzsch, B. (2020). A screen for gene paralogies delineating evolutionary branching order of early metazoa. G3 10, 811–826. doi: 10.1534/g3.119.400951
Erzurumlu, R. S., and Gaspar, P. (2020). How the barrel cortex became a working model for developmental plasticity: A historical perspective. J. Neurosci. 40, 6460–6473. doi: 10.1523/JNEUROSCI.0582-20.2020
Erzurumlu, R. S., Murakami, Y., and Rijli, F. M. (2010). Mapping the face in the somatosensory brainstem. Nat. Rev. Neurosci. 11, 252–263. doi: 10.1038/nrn2804
Evrard, H. C. (2019). The organization of the primate insular cortex. Front. Neuroanat. 13:43. doi: 10.3389/fnana.2019.00043
Fain, G. L. (2019). Sensory transduction. doi: 10.1093/oso/9780198835028.001.0001 Oxford: Oxford University Press.
Fan, D., Chettouh, Z., Consalez, G. G., and Brunet, J.-F. (2019). Taste bud formation depends on taste nerves. eLife 8:e49226. doi: 10.7554/eLife.49226.017
Farago, A. F., Awatramani, R. B., and Dymecki, S. M. (2006). Assembly of the brainstem cochlear nuclear complex is revealed by intersectional and subtractive genetic fate maps. Neuron 50, 205–218. doi: 10.1016/j.neuron.2006.03.014
Ferrè, E. R., and Haggard, P. (2020). Vestibular cognition: State-of-the-art and future directions. Cogn. Neuropsychol. 37, 413–420. doi: 10.1080/02643294.2020.1736018
Filova, I., Dvorakova, M., Bohuslavova, R., Pavlinek, A., Elliott, K. L., Vochyanova, S., et al. (2020). Combined Atoh1 and Neurod1 deletion reveals autonomous growth of auditory nerve fibers. Mol. Neurobiol. 57, 5307–5323. doi: 10.1007/s12035-020-02092-0
Finger, T. E. (1978). Gustatory pathways in the bullhead catfish. II. Facial lobe connections. J. Comp. Neurol. 180, 691–705. doi: 10.1002/cne.901800404
Finger, T. E. (2008). Sorting food from stones: The vagal taste system in Goldfish, Carassius auratus. J. Comp. Physiol. A 194, 135–143. doi: 10.1007/s00359-007-0276-0
Fode, C., Gradwohl, G., Morin, X., Dierich, A., LeMeur, M., Goridis, C., et al. (1998). The bHLH protein NEUROGENIN 2 is a determination factor for epibranchial placode–derived sensory neurons. Neuron 20, 483–494. doi: 10.1016/s0896-6273(00)80989-7
Foxe, J. J., Wylie, G. R., Martinez, A., Schroeder, C. E., Javitt, D. C., Guilfoyle, D., et al. (2002). Auditory-somatosensory multisensory processing in auditory association cortex: An fMRI study. J. Neurophysiol. 88, 540–543. doi: 10.1152/jn.2002.88.1.540
Freter, S., Muta, Y., O’Neill, P., Vassilev, V. S., Kuraku, S., and Ladher, R. K. (2012). Pax2 modulates proliferation during specification of the otic and epibranchial placodes. Dev. Dyn. 241, 1716–1728. doi: 10.1002/dvdy.23856
Fritzsch, B. (2021). An integrated perspective of evolution and development: From genes to function to ear, lateral line and electroreception. Diversity 13:364. doi: 10.3390/d13080364
Fritzsch, B., Beisel, K., Jones, K., Farinas, I., Maklad, A., Lee, J., et al. (2002). Development and evolution of inner ear sensory epithelia and their innervation. J. Neurobiol. 53, 143–156. doi: 10.1002/neu.10098
Fritzsch, B., Dillard, M., Lavado, A., Harvey, N. L., and Jahan, I. (2010). Canal cristae growth and fiber extension to the outer hair cells of the mouse ear require Prox1 activity. PLoS One 5:e9377. doi: 10.1371/journal.pone.0009377
Fritzsch, B., and Elliott, K. L. (2017). Gene, cell, and organ multiplication drives inner ear evolution. Dev. Biol. 431, 3–15. doi: 10.1016/j.ydbio.2017.08.034
Fritzsch, B., Elliott, K. L., and Glover, J. C. (2017). Gaskell revisited: New insights into spinal autonomics necessitate a revised motor neuron nomenclature. Cell Tissue Res. 370, 195–209. doi: 10.1007/s00441-017-2676-y
Fritzsch, B., Elliott, K. L., and Pavlinkova, G. (2019). Primary sensory map formations reflect unique needs and molecular cues specific to each sensory system. F1000Res. 8:F1000FacultyRev-345. doi: 10.12688/f1000research.17717.1
Fritzsch, B., and Martin, P. R. (2022). Vision and retina evolution: How to develop a retina. IBRO Neurosci. Rep. 12, 240–248.
Fritzsch, B., Matei, V., Nichols, D., Bermingham, N., Jones, K., Beisel, K., et al. (2005). Atoh1 null mice show directed afferent fiber growth to undifferentiated ear sensory epithelia followed by incomplete fiber retention. Dev. Dyn. 233, 570–583. doi: 10.1002/dvdy.20370
Fritzsch, B., and Northcutt, G. (1993). Cranial and spinal nerve organization in amphioxus and lampreys: Evidence for an ancestral craniate pattern. Cells Tissues Organs 148, 96–109. doi: 10.1159/000147529
Fritzsch, B., Sarai, P., Barbacid, M., and Silos-Santiago, I. (1997). Mice with a targeted disruption of the neurotrophin receptor trkB lose their gustatory ganglion cells early but do develop taste buds. Int. J. Dev. Neurosci. 15, 563–576. doi: 10.1016/s0736-5748(96)00111-6
Gasparini, S., Resch, J. M., Gore, A. M., Peltekian, L., and Geerling, J. C. (2021). Pre-locus coeruleus neurons in rat and mouse. Am. J. Physiol. Regul Integr. Comp. Physiol. 320, R342–R361. doi: 10.1152/ajpregu.00261.2020
Glover, J. C. (2020). “Development and evolution of vestibulo-ocular reflex circuitry,” in The senses, ed. B. Fritzsch (Amsterdam: Elsevier), 309–325. doi: 10.1002/cne.902270408
Glover, J. C., Elliott, K. L., Erives, A., Chizhikov, V. V., and Fritzsch, B. (2018). Wilhelm His’ lasting insights into hindbrain and cranial ganglia development and evolution. Dev. Biol. 444, S14–S24. doi: 10.1016/j.ydbio.2018.02.001
Glover, J. C., Renaud, J. S., and Rijli, F. M. (2006). Retinoic acid and hindbrain patterning. J. Neurobiol. 66, 705–725. doi: 10.1002/neu.20272
Goodman, J. M., and Bensmaia, S. J. (2021). “The neural mechanisms of touch and proprioception at the somatosensory periphery,” in The senses, ed. B. Fritzsch (Amsterdam: Elsevier), 2–27. doi: 10.1155/2020/3069639
Grabner, C. P., and Moser, T. (2018). Individual synaptic vesicles mediate stimulated exocytosis from cochlear inner hair cells. Proc. Natl. Acad. Sci. U.S.A. 115, 12811–12816. doi: 10.1073/pnas.1811814115
Grau-Perales, A., and Gallo, M. (2020). The auditory context-dependent attenuation of taste neophobia depends on D1 dopamine receptor activity in mice. Behav. Brain Res. 391:112687. doi: 10.1016/j.bbr.2020.112687
Grau-Perales, A. B., Gámiz, F., and Gallo, M. (2021). Effect of hippocampal 6-OHDA lesions on the contextual modulation of taste recognition memory. Behav. Brain Res. 409:113320. doi: 10.1016/j.bbr.2021.113320
Gray, P. (2013). Transcription factors define the neuroanatomical organization of the medullary reticular formation. Front. Neuroanat. 7:7. doi: 10.3389/fnana.2013.00007
Green, B. G., and Nachtigal, D. (2012). Somatosensory factors in taste perception: Effects of active tasting and solution temperature. Physiol. Behav. 107, 488–495. doi: 10.1016/j.physbeh.2012.05.010
Grothe, B. (2021). “The auditory system function – An integrative perspective,” in The senses, ed. B. Fritzsch doi: 10.1016/B978-0-12-805408-6.00009-9 (Amsterdam: Elsevier), 1–17.
Grothe, B., Carr, C. E., Casseday, J. H., Fritzsch, B., and Köppl, C. (2004). The evolution of central pathways and their neural processing patterns, Evolution of the vertebrate auditory system. doi: 10.1007/978-1-4419-8957-4_10 Berlin: Springer, 289–359.
Gurung, B., and Fritzsch, B. (2004). Time course of embryonic midbrain and thalamic auditory connection development in mice as revealed by carbocyanine dye tracing. J. Comp. Neurol. 479, 309–327. doi: 10.1002/cne.20328
Hall, J. M., Bell, M. L., and Finger, T. E. (2003). Disruption of sonic hedgehog signaling alters growth and patterning of lingual taste papillae. Dev. Biol. 255, 263–277. doi: 10.1016/S0012-1606(02)00048-9
Han, P., Müller, L., and Hummel, T. (2021). Peri-threshold trigeminal stimulation with capsaicin increases taste sensitivity in humans. Chemosens. Percept. 15, 1–7. doi: 10.1007/s12078-021-09285-4
Hanamori, T., Kunitake, T., Kato, K., and Kannan, H. (1998). Responses of neurons in the insular cortex to gustatory, visceral, and nociceptive stimuli in rats. J. Neurophysiol. 79, 2535–2545. doi: 10.1152/jn.1998.79.5.2535
Handler, A., and Ginty, D. D. (2021). The mechanosensory neurons of touch and their mechanisms of activation. Nat. Rev. Neurosci. 22, 521–537. doi: 10.1038/s41583-021-00489-x
Hans, J., Broman, J., and van Domburg, P. (2020). The somatosensory system, clinical neuroanatomy. doi: 10.1007/978-3-030-41878-6_4 Berlin: Springer, 171–255.
Hauck, P., and Hecht, H. (2019). Having a drink with Tchaikovsky: The crossmodal influence of background music on the taste of beverages. Multisens. Res. 32, 1–24. doi: 10.1163/22134808-20181321
Hawkes, R. (2012). Pattern formation during development of the embryonic cerebellum. Front. Neuroanat. 6:10. doi: 10.3389/fnana.2012.00010
Herbert, H., Moga, M. M., and Saper, C. B. (1990). Connections of the parabrachial nucleus with the nucleus of the solitary tract and the medullary reticular formation in the rat. J. Comp. Neurol. 293, 540–580. doi: 10.1002/cne.902930404
Hernandez-Miranda, L. R., Müller, T., and Birchmeier, C. (2017). The dorsal spinal cord and hindbrain: From developmental mechanisms to functional circuits. Dev. Biol. 432, 34–42. doi: 10.1016/j.ydbio.2016.10.008
Hertzano, R., Montcouquiol, M., Rashi-Elkeles, S., Elkon, R., Yücel, R., Frankel, W. N., et al. (2004). Transcription profiling of inner ears from Pou4f3 ddl/ddl identifies Gfi1 as a target of the Pou4f3 deafness gene. Hum. Mol. Genet. 13, 2143–2153. doi: 10.1093/hmg/ddh218
Hihara, H., Kanetaka, H., Kanno, A., Shimada, E., Koeda, S., Kawashima, R., et al. (2020). Somatosensory evoked magnetic fields of periodontal mechanoreceptors. Heliyon 6:e03244. doi: 10.1016/j.heliyon.2020.e03244
Hill, D. L., and May, O. L. (2007). “Development and plasticity of the gustatory portion of nucleus of the solitary tract,” in The role of the nucleus of the solitary tract in gustatory processing, ed. R. M. Bradley doi: 10.1201/9781420005974.ch6 (Boca Raton, FL: CRC Press).
Hirsch, D., Kohl, A., Wang, Y., and Sela-Donenfeld, D. (2021). Axonal projection patterns of the dorsal interneuron populations in the embryonic hindbrain. Front. Neuroanat. 15:793161. doi: 10.3389/fnana.2021.793161
Holcomb, P. S., Hoffpauir, B. K., Hoyson, M. C., Jackson, D. R., Deerinck, T. J., Marrs, G. S., et al. (2013). Synaptic inputs compete during rapid formation of the calyx of Held: A new model system for neural development. J. Neurosci. 33, 12954–12969. doi: 10.1523/JNEUROSCI.1087-13.2013
Holley, M., Rhodes, C., Kneebone, A., Herde, M. K., Fleming, M., and Steel, K. P. (2010). Emx2 and early hair cell development in the mouse inner ear. Dev. Biol. 340, 547–556. doi: 10.1016/j.ydbio.2010.02.004
Holt-Hansen, K. (1968). Taste and pitch. Percept. Mot. Skills 27, 59–68. doi: 10.2466/pms.1968.27.1.59
Huang, E. J., Liu, W., Fritzsch, B., Bianchi, L. M., Reichardt, L. F., and Xiang, M. (2001). Brn3a is a transcriptional regulator of soma size, target field innervation and axon pathfinding of inner ear sensory neurons. Development 128, 2421–2432. doi: 10.1242/dev.128.13.2421
Huang, E. J., and Reichardt, L. F. (2003). Trk receptors: Roles in neuronal signal transduction. Annu. Rev. Biochem. 72, 609–642. doi: 10.1146/annurev.biochem.72.121801.161629
Huang, T., and Krimm, R. F. (2010). Developmental expression of Bdnf, Ntf4/5, and TrkB in the mouse peripheral taste system. Dev. Dyn. 239, 2637–2646. doi: 10.1002/dvdy.22412
Hummel, T., Iannilli, E., Frasnelli, J., Boyle, J., and Gerber, J. (2009). Central processing of trigeminal activation in humans. Ann. N. Y. Acad. Sci. 1170, 190–195. doi: 10.1111/j.1749-6632.2009.03910.x
Iskusnykh, I. Y., Steshina, E. Y., and Chizhikov, V. V. (2016). Loss of Ptf1a leads to a widespread cell-fate misspecification in the brainstem, affecting the development of somatosensory and viscerosensory nuclei. J. Neurosci. 36, 2691–2710. doi: 10.1523/JNEUROSCI.2526-15.2016
Iwasato, T., and Erzurumlu, R. S. (2018). Development of tactile sensory circuits in the CNS. Curr. Opin. Neurobiol. 53, 66–75. doi: 10.1016/j.conb.2018.06.001
Jahan, I., Pan, N., Kersigo, J., and Fritzsch, B. (2010). Neurod1 suppresses hair cell differentiation in ear ganglia and regulates hair cell subtype development in the cochlea. PLoS One 5:e11661. doi: 10.1371/journal.pone.0011661
Jean, P., Özçete, Ö. D., Tarchini, B., and Moser, T. (2019). Intrinsic planar polarity mechanisms influence the position-dependent regulation of synapse properties in inner hair cells. Proc. Natl. Acad. Sci. U.S.A. 116, 9084–9093. doi: 10.1073/pnas.1818358116
Jiang, T., Kindt, K., and Wu, D. K. (2017). Transcription factor Emx2 controls stereociliary bundle orientation of sensory hair cells. eLife 6:e23661. doi: 10.7554/eLife.23661
Kageyama, R., Shimojo, H., and Ohtsuka, T. (2019). Dynamic control of neural stem cells by bHLH factors. Neurosci. Res. 138, 12–18. doi: 10.1016/j.neures.2018.09.005
Kandler, K., Lee, J., and Pecka, M. (2020). “2.28 – The superior olivary complex,” in The senses: A comprehensive reference, 2nd Edn, ed. B. Fritzsch (Oxford: Elsevier), 533–555. doi: 10.1016/B978-0-12-805408-6.00021-X
Kandler, K., Lee, J., and Pecka, M. (2021). “The superior olivary complex,” in The senses, ed. B. Fritzsch (Amsterdam: Elsevier), 533–555.
Karthik, S., Huan, D., Delgado, Y., Laing, J. J., Peltekian, L., Iversen, G., et al. (2022). Molecular ontology of the parabrachial nucleus. J. Comp. Neurol. 530, 1658–1699. doi: 10.1002/cne.25307
Keast, R. S., and Breslin, P. A. (2003). An overview of binary taste–taste interactions. Food Qual. Prefer. 14, 111–124. doi: 10.1016/S0950-3293(02)00110-6
Kersigo, J., D’Angelo, A., Gray, B. D., Soukup, G. A., and Fritzsch, B. (2011). The role of sensory organs and the forebrain for the development of the craniofacial shape as revealed by Foxg1-cre-mediated microRNA loss. Genesis 49, 326–341. doi: 10.1002/dvg.20714
Kindt, K. S., Akturk, A., Jarysta, A., Day, M., Beirl, A., Flonard, M., et al. (2021). EMX2-GPR156-Gαi reverses hair cell orientation in mechanosensory epithelia. Nat. Commun. 12:2861. doi: 10.1038/s41467-021-22997-1
King, A. J. (2020). “Feedback systems: Descending pathways and adaptive coding in the auditory system,” in The senses, ed. B. Fritzsch (Amsterdam: Elsevier), 732–748. doi: 10.7554/eLife.73289
Kinnamon, S. C., and Finger, T. E. (2019). Recent advances in taste transduction and signaling. F1000Res. 8:F1000FacultyRev-2117. doi: 10.12688/f1000research.21099.1
Kobayashi, T., Piao, W., Takamura, T., Kori, H., Miyachi, H., Kitano, S., et al. (2019). Enhanced lysosomal degradation maintains the quiescent state of neural stem cells. Nat. Commun. 10:5446. doi: 10.1038/s41467-019-13203-4
Kopecky, B., Santi, P., Johnson, S., Schmitz, H., and Fritzsch, B. (2011). Conditional deletion of N-Myc disrupts neurosensory and non-sensory development of the ear. Dev. Dyn. 240, 1373–1390. doi: 10.1002/dvdy.22620
Kral, A., and Pallas, S. L. (2011). “Development of the auditory cortex,” in The auditory cortex, eds J. A. Winer and C. E. Schreiner (Berlin: Springer), 443–463. doi: 10.1007/978-1-4419-0074-6_21
Kuypers, H. G., and Tuerk, J. (1964). The distribution of cortical fibres within the nuclei cuneatus and gracilis in the cat. J. Anat. 98, 143–162.
Kwon, H. G., Jang, S. H., and Lee, M. Y. (2017). Effects of visual information regarding tactile stimulation on the somatosensory cortical activation: A functional MRI study. Neural Regen. Res. 12, 1119–1123. doi: 10.4103/1673-5374.211191
Lai, H. C., Seal, R. P., and Johnson, J. E. (2016). Making sense out of spinal cord somatosensory development. Development 143, 3434–3448. doi: 10.1242/dev.139592
Lazarov, N. E. (2002). Comparative analysis of the chemical neuroanatomy of the mammalian trigeminal ganglion and mesencephalic trigeminal nucleus. Prog. Neurobiol. 66, 19–59. doi: 10.1016/s0301-0082(01)00021-1
Lee, K. J., Dietrich, P., and Jessell, T. M. (2000). Genetic ablation reveals that the roof plate is essential for dorsal interneuron specification. Nature 403, 734–740. doi: 10.1038/35001507
Lee, S., Kruglikov, I., Huang, Z. J., Fishell, G., and Rudy, B. (2013). A disinhibitory circuit mediates motor integration in the somatosensory cortex. Nat. Neurosci. 16, 1662–1670. doi: 10.1038/nn.3544
Li, J., Ali, M. S. S., and Lemon, C. H. (2021). TRPV1-lineage somatosensory fibers communicate with taste neurons in the mouse parabrachial nucleus. bioRxiv [Preprint] doi: 10.1523/JNEUROSCI.0927-21.2021
Liao, C.-C., Qi, H.-X., Reed, J. L., and Kaas, J. H. (2020). “The somatosensory system of primates,” in The senses, ed. B. Fritzsch doi: 10.1016/B978-0-12-805408-6.00028-2 (Amsterdam: Elsevier), 180–197.
Lipovsek, M., Ledderose, J., Butts, T., Lafont, T., Kiecker, C., Wizenmann, A., et al. (2017). The emergence of mesencephalic trigeminal neurons. Neural Dev. 12:11. doi: 10.1186/s13064-017-0088-z
Lipovsek, M., and Wingate, R. J. (2018). Conserved and divergent development of brainstem vestibular and auditory nuclei. eLife 7:e40232. doi: 10.7554/eLife.40232
Liu, F., Thirumangalathu, S., Gallant, N. M., Yang, S. H., Stoick-Cooper, C. L., Reddy, S. T., et al. (2007). Wnt-beta-catenin signaling initiates taste papilla development. Nat. Genet. 39, 106–112. doi: 10.1038/ng1932
Lorenzen, S. M., Duggan, A., Osipovich, A. B., Magnuson, M. A., and García-Añoveros, J. (2015). Insm1 promotes neurogenic proliferation in delaminated otic progenitors. Mech. Dev. 138(Pt 3), 233–245. doi: 10.1016/j.mod.2015.11.001
Lorenzo, P. M. D. (2021). Neural coding of food is a multisensory, sensorimotor function. Nutrients 13:398. doi: 10.3390/nu13020398
Loutit, A. J., Vickery, R. M., and Potas, J. R. (2021). Functional organization and connectivity of the dorsal column nuclei complex reveals a sensorimotor integration and distribution hub. J. Comp. Neurol. 529, 187–220. doi: 10.1002/cne.24942
Lowenstein, E. D., Cui, K., and Hernandez-Miranda, L. R. (2022). Regulation of early cerebellar development. FEBS J. [Epub ahead of print]. doi: 10.1111/febs.16426
Lunde, A., Okaty, B. W., Dymecki, S. M., and Glover, J. C. (2019). Molecular profiling defines evolutionarily conserved transcription factor signatures of major vestibulospinal neuron groups. Eneuro 6:ENEURO.0475-18.2019. doi: 10.1523/ENEURO.0475-18.2019
Lundy, R. F. Jr., and Norgren, R. (2015). “Gustatory system,” in The rat nervous system, ed. G. Paxinos doi: 10.1016/B978-0-12-374245-2.00026-7 (Amsterdam: Elsevier), 733–760.
Ma, Q., Anderson, D. J., and Fritzsch, B. (2000). Neurogenin 1 null mutant ears develop fewer, morphologically normal hair cells in smaller sensory epithelia devoid of innervation. J. Assoc. Res. Otolaryngol. 1, 129–143. doi: 10.1007/s101620010017
Ma, Q., Chen, Z., del Barco Barrantes, I., De La Pompa, J. L., and Anderson, D. J. (1998). neurogenin1 is essential for the determination of neuronal precursors for proximal cranial sensory ganglia. Neuron 20, 469–482. doi: 10.1016/s0896-6273(00)80988-5
Ma, Q., Fode, C., Guillemot, F., and Anderson, D. J. (1999). Neurogenin1 and neurogenin2 control two distinct waves of neurogenesis in developing dorsal root ganglia. Genes Dev. 13, 1717–1728. doi: 10.1101/gad.13.13.1717
Macova, I., Pysanenko, K., Chumak, T., Dvorakova, M., Bohuslavova, R., Syka, J., et al. (2019). Neurod1 is essential for the primary tonotopic organization and related auditory information processing in the midbrain. J. Neurosci. 39, 984–1004. doi: 10.1523/JNEUROSCI.2557-18.2018
Majka, P., Rosa, M. G., Bai, S., Chan, J. M., Huo, B.-X., Jermakow, N., et al. (2019). Unidirectional monosynaptic connections from auditory areas to the primary visual cortex in the marmoset monkey. Brain Struct. Funct. 224, 111–131. doi: 10.1007/s00429-018-1764-4
Maklad, A., and Fritzsch, B. (1999). Incomplete segregation of endorgan-specific vestibular ganglion cells in mice and rats. J. Vestib. Res. 9, 387–399. doi: 10.3233/VES-1999-9601
Maklad, A., and Fritzsch, B. (2003). Partial segregation of posterior crista and saccular fibers to the nodulus and uvula of the cerebellum in mice, and its development. Brain Res. Dev. Brain Res. 140, 223–236. doi: 10.1016/s0165-3806(02)00609-0
Malmierca, M. S. (2015). “Auditory system,” in The rat nervous system, ed. G. Paxinos (Cambridge, MA: Academic Press), 865–946.
Malone, B. J., Hasenstaub, A. R., and Schreiner, C. E. (2021). “Primary auditory cortex II. Some functional considerations,” in The senses, ed. B. Fritzsch doi: 10.1016/B978-0-12-809324-5.24268-5 (Amsterdam: Elsevier), 657–680.
Mao, C.-A., Cho, J.-H., Wang, J., Gao, Z., Pan, P., Tsai, W.-W., et al. (2013). Reprogramming amacrine and photoreceptor progenitors into retinal ganglion cells by replacing Neurod1 with Atoh7. Development 140, 541–551. doi: 10.1242/dev.085886
Maricich, S. M., Wellnitz, S. A., Nelson, A. M., Lesniak, D. R., Gerling, G. J., Lumpkin, E. A., et al. (2009). Merkel cells are essential for light-touch responses. Science 324, 1580–1582. doi: 10.1126/science.1172890
Marrs, G. S., Morgan, W. J., Howell, D. M., Spirou, G. A., and Mathers, P. H. (2013). Embryonic origins of the mouse superior olivary complex. Dev. Neurobiol. 73, 384–398. doi: 10.1002/dneu.22069
Marrs, G. S., and Spirou, G. A. (2012). Embryonic assembly of auditory circuits: Spiral ganglion and brainstem. J. Physiol. 590, 2391–2408. doi: 10.1113/jphysiol.2011.226886
Marzban, H., Rahimi-Balaei, M., and Hawkes, R. (2019). Early trigeminal ganglion afferents enter the cerebellum before the Purkinje cells are born and target the nuclear transitory zone. Brain Struct. Funct. 224, 2421–2436.
Matei, V., Pauley, S., Kaing, S., Rowitch, D., Beisel, K., Morris, K., et al. (2005). Smaller inner ear sensory epithelia in Neurog1 null mice are related to earlier hair cell cycle exit. Dev. Dyn. 234, 633–650. doi: 10.1002/dvdy.20551
Matsuo, S., Ichikawa, H., Silos-Santiago, I., Arends, J., Henderson, T., Kiyomiya, K., et al. (1999). Proprioceptive afferents survive in the masseter muscle of trkC knockout mice. Neuroscience 95, 209–216. doi: 10.1016/s0306-4522(99)00424-8
Meehan, T. P., Bressler, S. L., Tang, W., Astafiev, S. V., Sylvester, C. M., Shulman, G. L., et al. (2017). Top-down cortical interactions in visuospatial attention. Brain Struct. Funct. 222, 3127–3145. doi: 10.1007/s00429-017-1390-6
Meltzer, S., Santiago, C., Sharma, N., and Ginty, D. D. (2021). The cellular and molecular basis of somatosensory neuron development. Neuron 109, 3736–3757. doi: 10.1016/j.neuron.2021.09.004
Mishima, Y., Lindgren, A. G., Chizhikov, V. V., Johnson, R. L., and Millen, K. J. (2009). Overlapping function of Lmx1a and Lmx1b in anterior hindbrain roof plate formation and cerebellar growth. J. Neurosci. 29, 11377–11384. doi: 10.1523/JNEUROSCI.0969-09.2009
Mizoguchi, N., Muramoto, K., and Kobayashi, M. (2020). Olfactory signals from the main olfactory bulb converge with taste information from the chorda tympani nerve in the agranular insular cortex of rats. Pflügers Arch. 472, 721–732. doi: 10.1007/s00424-020-02399-w
Montcouquiol, M., Rachel, R. A., Lanford, P. J., Copeland, N. G., Jenkins, N. A., and Kelley, M. W. (2003). Identification of Vangl2 and Scrb1 as planar polarity genes in mammals. Nature 423, 173–177. doi: 10.1038/nature01618
Moro, S. S., and Harris, L. R. (2018). Vestibular–somatosensory interactions affect the perceived timing of tactile stimuli. Exp. Brain Res. 236, 2877–2885. doi: 10.1007/s00221-018-5346-8
Moser, T. (2020). “Presynaptic physiology of cochlear inner hair cells,” in The senses, ed. B. Fritzsch (Amsterdam: Elsevier), 441–467. doi: 10.1016/B978-0-12-809324-5.24185-0
Murray, M. M., Molholm, S., Michel, C. M., Heslenfeld, D. J., Ritter, W., Javitt, D. C., et al. (2005). Grabbing your ear: Rapid auditory–somatosensory multisensory interactions in low-level sensory cortices are not constrained by stimulus alignment. Cereb. Cortex 15, 963–974. doi: 10.1093/cercor/bhh197
Nakano, Y., Wiechert, S., Fritzsch, B., and Bánfi, B. (2020). Inhibition of a transcriptional repressor rescues hearing in a splicing factor–deficient mouse. Life Sci. Alliance 3:e202000841. doi: 10.26508/lsa.202000841
Nichols, D. H., and Bruce, L. L. (2006). Migratory routes and fates of cells transcribing the Wnt-1 gene in the murine hindbrain. Dev. Dyn. 235, 285–300. doi: 10.1002/dvdy.20611
Nosrat, I. V., Margolskee, R. F., and Nosrat, C. A. (2012). Targeted taste cell-specific overexpression of brain-derived neurotrophic factor in adult taste buds elevates phosphorylated TrkB protein levels in taste cells, increases taste bud size, and promotes gustatory innervation. J. Biol. Chem. 287, 16791–16800. doi: 10.1074/jbc.M111.328476
Obana, E. A., Zhou, Q., Furmanski, O., and Doughty, M. L. (2018). Conditional deletion of Neurog1 in the cerebellum of postnatal mice delays inhibitory interneuron maturation. J. Neurosci. Res. 96, 1560–1575. doi: 10.1002/jnr.24247
Oertel, D., and Cao, X.-J. (2020). “2.27 – The ventral cochlear nucleus,” in The senses: A comprehensive reference, 2nd Edn, ed. B. Fritzsch doi: 10.1016/B978-0-12-809324-5.23880-7 (Oxford: Elsevier), 517–532.
Ohmoto, M., Kitamoto, S., and Hirota, J. (2020). Expression of Eya1 in mouse taste buds. Cell Tissue Res. 383, 979–986. doi: 10.1007/s00441-020-03311-9
Ohmoto, M., Ren, W., Nishiguchi, Y., Hirota, J., Jiang, P., and Matsumoto, I. (2017). Genetic lineage tracing in taste tissues using Sox2-CreERT2 strain. Chem. Senses 42, 547–552. doi: 10.1093/chemse/bjx032
Okubo, T., Pevny, L. H., and Hogan, B. L. (2006). Sox2 is required for development of taste bud sensory cells. Genes Dev. 20, 2654–2659.
O’Neill, P., Mak, S.-S., Fritzsch, B., Ladher, R. K., and Baker, C. V. (2012). The amniote paratympanic organ develops from a previously undiscovered sensory placode. Nat. Commun. 3:1041. doi: 10.1038/ncomms2036
Ossenkopp, K.-P., Parker, L. A., Limebeer, C. L., Burton, P., Fudge, M. A., and Cross-Mellor, S. K. (2003). Vestibular lesions selectively abolish body rotation-induced, but not lithium-induced, conditioned taste aversions (oral rejection responses) in rats. Behav. Neurosci. 117, 105–112. doi: 10.1037/0735-7044.117.1.105
O’Sullivan, A. E., Crosse, M. J., Di Liberto, G. M., de Cheveigné, A., and Lalor, E. C. (2021). Neurophysiological indices of audiovisual speech processing reveal a hierarchy of multisensory integration effects. J. Neurosci. 41, 4991–5003. doi: 10.1523/JNEUROSCI.0906-20.2021
Pallas, S. L. (2001). Intrinsic and extrinsic factors that shape neocortical specification. Trends Neurosci. 24, 417–423. doi: 10.1016/s0166-2236(00)01853-1
Pan, B., Géléoc, G. S., Asai, Y., Horwitz, G. C., Kurima, K., Ishikawa, K., et al. (2013). TMC1 and TMC2 are components of the mechanotransduction channel in hair cells of the mammalian inner ear. Neuron 79, 504–515. doi: 10.1016/j.neuron.2013.06.019
Pauley, S., Lai, E., and Fritzsch, B. (2006). Foxg1 is required for morphogenesis and histogenesis of the mammalian inner ear. Dev. Dyn. 235, 2470–2482. doi: 10.1002/dvdy.20839
Pei, Y.-C., Hsiao, S. S., Craig, J. C., and Bensmaia, S. J. (2011). Neural mechanisms of tactile motion integration in somatosensory cortex. Neuron 69, 536–547. doi: 10.1016/j.neuron.2010.12.033
Perea-Martinez, I., Nagai, T., and Chaudhari, N. (2013). Functional cell types in taste buds have distinct longevities. PLoS One 8:e53399. doi: 10.1371/journal.pone.0053399
Peterka, R. J., and Loughlin, P. J. (2004). Dynamic regulation of sensorimotor integration in human postural control. J. Neurophysiol. 91, 410–423. doi: 10.1152/jn.00516.2003
Petersen, C. I., Jheon, A. H., Mostowfi, P., Charles, C., Ching, S., Thirumangalathu, S., et al. (2011). FGF signaling regulates the number of posterior taste papillae by controlling progenitor field size. PLoS Genet. 7:e1002098. doi: 10.1371/journal.pgen.1002098
Pfeiffer, C., Noel, J. P., Serino, A., and Blanke, O. (2018). Vestibular modulation of peripersonal space boundaries. Eur. J. Neurosci. 47, 800–811. doi: 10.1111/ejn.13872
Pheasant, R. J., Fisher, M. N., Watts, G. R., Whitaker, D. J., and Horoshenkov, K. V. (2010). The importance of auditory-visual interaction in the construction of ‘tranquil space’. J. Environ. Psychol. 30, 501–509. doi: 10.1016/j.jenvp.2010.03.006
Phillips, J. O., Ling, L., Nowack, A., Rebollar, B., and Rubinstein, J. T. (2020). Interactions between auditory and vestibular modalities during stimulation with a combined vestibular and cochlear prosthesis. Audiol. Neurotol. 25, 96–108. doi: 10.1159/000503846
Phillips-Silver, J., and Trainor, L. J. (2008). Vestibular influence on auditory metrical interpretation. Brain Cogn. 67, 94–102. doi: 10.1016/j.bandc.2007.11.007
Phillips-Silver, J., VanMeter, J. W., and Rauschecker, J. P. (2020). Auditory-vestibulomotor temporal processing and crossmodal plasticity for musical rhythm in the early blind. bioRxiv [Preprint] doi: 10.1101/2020.03.23.987727
Plass, J., Brang, D., Suzuki, S., and Grabowecky, M. (2020). Vision perceptually restores auditory spectral dynamics in speech. Proc. Natl. Acad. Sci. U.S.A. 117, 16920–16927. doi: 10.1073/pnas.2002887117
Pop, I. V., Espinosa, F., Blevins, C. J., Okafor, P. C., Ogujiofor, O. W., Goyal, M., et al. (2021). Structure of long-range direct and indirect spinocerebellar pathways as well as local spinal circuits mediating proprioception. J. Neurosci. 42, 581–600. doi: 10.1523/JNEUROSCI.2157-20.2021
Pritchard, T. C., Macaluso, D. A., and Eslinger, P. J. (1999). Taste perception in patients with insular cortex lesions. Behav. Neurosci. 113, 663–671. doi: 10.1037/0735-7044.113.4.663
Qi, H.-X., Liao, C.-C., Reed, J. L., and Kaas, J. H. (2020). “Cortical and subcortical plasticity after sensory loss in the somatosensory system of primates,” in The senses, ed. B. Fritzsch doi: 10.1016/B978-0-12-809324-5.24228-4 (Amsterdam: Elsevier), 399–418.
Qian, Y., Fritzsch, B., Shirasawa, S., Chen, C.-L., Choi, Y., and Ma, Q. (2001). Formation of brainstem (nor) adrenergic centers and first-order relay visceral sensory neurons is dependent on homeodomain protein Rnx/Tlx3. Genes Dev. 15, 2533–2545. doi: 10.1101/gad.921501
Rauschecker, J. P. (2021). “The auditory cortex of primates including man with reference to speech,” in The senses, ed. B. Fritzsch doi: 10.1016/B978-0-12-805408-6.00029-4 (Amsterdam: Elsevier), 791–811.
Rauschecker, J. P., and Scott, S. K. (2009). Maps and streams in the auditory cortex: Nonhuman primates illuminate human speech processing. Nat. Neurosci. 12, 718–724. doi: 10.1038/nn.2331
Razak, K. A., Zumsteg, T., and Fuzessery, Z. M. (2009). Development of auditory thalamocortical connections in the pallid bat, Antrozous pallidus. J. Comp. Neurol. 515, 231–242. doi: 10.1002/cne.22050
Reiprich, S., and Wegner, M. (2015). From CNS stem cells to neurons and glia: Sox for everyone. Cell Tissue Res. 359, 111–124. doi: 10.1007/s00441-014-1909-6
Rhyu, M.-R., Kim, Y., and Lyall, V. (2021). Interactions between chemesthesis and taste: Role of TRPA1 and TRPV1. Int. J. Mol. Sci. 22:3360. doi: 10.3390/ijms22073360
Riccomagno, M. M., Martinu, L., Mulheisen, M., Wu, D. K., and Epstein, D. J. (2002). Specification of the mammalian cochlea is dependent on sonic hedgehog. Genes Dev. 16, 2365–2378. doi: 10.1101/gad.1013302
Roper, S. (2020). “Microphysiology of taste buds,” in The senses, ed. B. Fritzsch (Amsterdam: Elsevier), 187–210. doi: 10.1523/JNEUROSCI.12-04-01127.1992
Roper, S. D. (2021). Encoding taste: From receptors to perception. Handb. Exp. Pharmacol. 275, 53–90. doi: 10.1007/164_2021_559
Roper, S. D., and Chaudhari, N. (2017). Taste buds: Cells, signals and synapses. Nat. Rev. Neurosci. 18, 485–497. doi: 10.1038/nrn.2017.68
Roper, S. D., Krimm, R. F., and Fritzsch, B. (2022). in Taste buds explained: From taste sensing to taste processing in the forebrain, eds B. Fritzsch and K. L. Elliott doi: 10.1201/9781003092810-5 (Boca Raton, FL: Wiley), 111–134.
Rose, M. F., Ahmad, K. A., Thaller, C., and Zoghbi, H. Y. (2009). Excitatory neurons of the proprioceptive, interoceptive, and arousal hindbrain networks share a developmental requirement for Math1. Proc. Natl. Acad. Sci. U.S.A. 106, 22462–22467. doi: 10.1073/pnas.0911579106
Saper, C., and Loewy, A. (1980). Efferent connections of the parabrachial nucleus in the rat. Brain Res. 197, 291–317. doi: 10.1016/0006-8993(80)91117-8
Schier, L. A., and Spector, A. C. (2019). The functional and neurobiological properties of bad taste. Physiol. Rev. 99, 605–663. doi: 10.1152/physrev.00044.2017
Shaikh, A. G., Zee, D. S., Taube, J., and Kheradmand, A. (2020). “Visual–vestibular interactions,” in Multisensory perception, doi: 10.1016/B978-0-12-812492-5.00009-7 (Amsterdam: Elsevier), 201–219.
Shepherd, G. M. (2006). Smell images and the flavour system in the human brain. Nature 444, 316–321. doi: 10.1038/nature05405
Shibata, S. B., Ranum, P. T., Moteki, H., Pan, B., Goodwin, A. T., Goodman, S. S., et al. (2016). RNA interference prevents autosomal-dominant hearing loss. Am. J. Hum. Genet. 98, 1101–1113. doi: 10.1016/j.ajhg.2016.03.028
Simmons, D., Duncan, J., de Caprona, D. C., and Fritzsch, B. (2011). “Development of the inner ear efferent system,” in Auditory and vestibular efferents, doi: 10.1007/978-1-4419-7070-1_7 (New York, NY: Springer), 187–216.
Smith, A. C., Fleenor, S. J., and Begbie, J. (2015). Changes in gene expression and cell shape characterise stages of epibranchial placode-derived neuron maturation in the chick. J. Anat. 227, 89–102. doi: 10.1111/joa.12333
Spence, C. (2011). Crossmodal correspondences: A tutorial review. Atten. Percept. Psychophys. 73, 971–995. doi: 10.3758/s13414-010-0073-7
Spence, C. (2022). Multisensory contributions to affective touch. Curr. Opin. Behav. Sci. 43, 40–45. doi: 10.1016/j.cobeha.2021.08.003
Spence, C., and Ngo, M. K. (2012). Assessing the shape symbolism of the taste, flavour, and texture of foods and beverages. Flavour 1, 1–13. doi: 10.1186/2044-7248-1-12
Spence, C., and Soto-Faraco, S. (2010). Auditory perception: Interactions with vision. Oxford Handb. Audit. Sci. Hear. 3, 271–296. doi: 10.1093/oxfordhb/9780199233557.013.0012
Spence, C., Wang, Q. J., Reinoso-Carvalho, F., and Keller, S. (2021). Commercializing sonic seasoning in multisensory offline experiential events and online tasting experiences. Front. Psychol. 12:740354. doi: 10.3389/fpsyg.2021.740354
Staszko, S., and Boughter, J. (2020). “Taste pathways, representation and processing in the brain,” in The senses, ed. B. Fritzsch doi: 10.1016/B978-0-12-809324-5.23891-1 (Amsterdam: Elsevier), 280–297.
Staszko, S. M., Boughter, J. D. Jr., and Fletcher, M. L. (2020). Taste coding strategies in insular cortex. Exp. Biol. Med. 245, 448–455. doi: 10.1177/1535370220909096
Steiner, L., Federspiel, A., Slavova, N., Wiest, R., Grunt, S., Steinlin, M., et al. (2020). Functional topography of the thalamo-cortical system during development and its relation to cognition. Neuroimage 223:117361. doi: 10.1016/j.neuroimage.2020.117361
Stoner, Z. A., Ketchum, E. M., Sheltz-Kempf, S., Blinkiewicz, P. V., Elliott, K. L., and Duncan, J. S. (2021). Fzd3 expression within inner ear afferent neurons is necessary for central pathfinding. Front. Neurosci. 15:779871. doi: 10.3389/fnins.2021.779871
Straka, H., Fritzsch, B., and Glover, J. C. (2014). Connecting ears to eye muscles: Evolution of a ‘simple’ reflex arc. Brain Behav. Evol. 83, 162–175. doi: 10.1159/000357833
Striedter, G. F., and Northcutt, R. G. (2019). Brains through time: A natural history of vertebrates. doi: 10.1093/oso/9780195125689.001.0001 Oxford: Oxford University Press.
Suer, M. (2021). “Anatomy of the trigeminal nerve,” in Trigeminal nerve pain: A guide to clinical management, ed. A. Abd-Elsayed (Cham: Springer International Publishing), 5–16.
Sugiyama, S., Takeuchi, N., Inui, K., Nishihara, M., and Shioiri, T. (2018). Effect of acceleration of auditory inputs on the primary somatosensory cortex in humans. Sci. Rep. 8:12883.
Suryanarayana, S. M., Pérez-Fernández, J., Robertson, B., and Grillner, S. (2020). The evolutionary origin of visual and somatosensory representation in the vertebrate pallium. Nat. Ecol. Evol. 4, 639–651.
Suryanarayana, S. M., Pérez-Fernández, J., Robertson, B., and Grillner, S. (2021). Olfaction in lamprey pallium revisited—Dual projections of mitral and tufted cells. Cell Rep. 34:108596. doi: 10.1016/j.celrep.2020.108596
Syka, J. (2020). Age-related changes in the auditory brainstem and inferior colliculus, aging and hearing. Berlin: Springer, 67–96.
Taube, J. S., and Yoder, R. M. (2021). “The impact of vestibular signals on cells responsible for orientation and navigation,” in The senses, ed. B. Fritzsch (Amsterdam: Elsevier), 496–511.
Teng, B., Wilson, C. E., Tu, Y.-H., Joshi, N. R., Kinnamon, S. C., and Liman, E. R. (2019). Cellular and neural responses to sour stimuli require the proton channel Otop1. Curr. Biol. 29, 3647–3656.e5. doi: 10.1016/j.cub.2019.08.077
Ter-Avetisyan, G., Dumoulin, A., Herrel, A., Schmidt, H., Strump, J., Afzal, S., et al. (2018). Loss of axon bifurcation in mesencephalic trigeminal neurons impairs the maximal biting force in Npr2-deficient mice. Front. Cell. Neurosci. 12:153. doi: 10.3389/fncel.2018.00153
Tichko, P., Kim, J. C., and Large, E. W. (2021). Bouncing the network: A dynamical systems model of auditory–vestibular interactions underlying infants’ perception of musical rhythm. Dev. Sci. 24:e13103. doi: 10.1111/desc.13103
Tosches, M. A., and Laurent, G. (2019). Evolution of neuronal identity in the cerebral cortex. Curr. Opin. Neurobiol. 56, 199–208.
Trudeau-Fisette, P., Ito, T., and Ménard, L. (2019). Auditory and somatosensory interaction in speech perception in children and adults. Front. Hum. Neurosci. 13:344. doi: 10.3389/fnhum.2019.00344
Uddin, L. Q., Nomi, J. S., Hébert-Seropian, B., Ghaziri, J., and Boucher, O. (2017). Structure and function of the human insula. J. Clin. Neurophysiol. 34, 300–306.
Urness, L. D., Bleyl, S. B., Wright, T. J., Moon, A. M., and Mansour, S. L. (2011). Redundant and dosage sensitive requirements for Fgf3 and Fgf10 in cardiovascular development. Dev. Biol. 356, 383–397. doi: 10.1016/j.ydbio.2011.05.671
Vendrell-Llopis, N., and Yaksi, E. (2015). Evolutionary conserved brainstem circuits encode category, concentration and mixtures of taste. Sci. Rep. 5:17825. doi: 10.1038/srep17825
von Bartheld, C. S., and Fritzsch, B. (2006). Comparative analysis of neurotrophin receptors and ligands in vertebrate neurons: Tools for evolutionary stability or changes in neural circuits? Brain Behav. Evol. 68, 157–172.
Wang, Q. J., Keller, S., and Spence, C. (2021). Metacognition and crossmodal correspondences between auditory attributes and saltiness in a large sample study. Multisens. Res. 34, 785–805. doi: 10.1163/22134808-bja10055
Wang, V. Y., Rose, M. F., and Zoghbi, H. Y. (2005). Math1 expression redefines the rhombic lip derivatives and reveals novel lineages within the brainstem and cerebellum. Neuron 48, 31–43. doi: 10.1016/j.neuron.2005.08.024
Watson, C., Mitchelle, A., and Puelles, L. (2017a). 2.02 A new mammalian brain ontology based on developmental gene expression. Evol. Nervous Syst.
Watson, C., Shimogori, T., and Puelles, L. (2017b). Mouse Fgf8-Cre-LacZ lineage analysis defines the territory of the postnatal mammalian isthmus. J. Comp. Neurol. 525, 2782–2799. doi: 10.1002/cne.24242
Wistehube, T., Rullmann, M., Wiacek, C., Braun, P., and Pleger, B. (2018). Fat perception in the human frontal operculum, insular and somatosensory cortex. Sci. Rep. 8:11825. doi: 10.1038/s41598-018-30366-0
Wiwatpanit, T., Lorenzen, S. M., Cantú, J. A., Foo, C. Z., Hogan, A. K., Márquez, F., et al. (2018). Trans-differentiation of outer hair cells into inner hair cells in the absence of INSM1. Nature 563, 691–695.
Wooding, S., and Ramirez, V. (2020). “Taste genetics,” in The senses, ed. B. Fritzsch (Amsterdam: Elsevier), 264–279.
Wullimann, M. F., and Grothe, B. (2013). “The central nervous organization of the lateral line system,” in The lateral line system, eds S. Coombs, H. Bleckmann, R. Fay, and A. Popper (New York, NY: Springer), 195–251.
Xiang, M., Maklad, A., Pirvola, U., and Fritzsch, B. (2003). Brn3c null mutant mice show long-term, incomplete retention of some afferent inner ear innervation. BMC Neurosci. 4:2. doi: 10.1186/1471-2202-4-2
Xu, J., Li, J., Zhang, T., Jiang, H., Ramakrishnan, A., Fritzsch, B., et al. (2021). Chromatin remodelers and lineage-specific factors interact to target enhancers to establish proneurosensory fate within otic ectoderm. Proc. Natl. Acad. Sci. U.S.A. 118:e2025196118. doi: 10.1073/pnas.2025196118
Yamada, M., Seto, Y., Taya, S., Owa, T., Inoue, Y. U., Inoue, T., et al. (2014). Specification of spatial identities of cerebellar neuron progenitors by ptf1a and atoh1 for proper production of GABAergic and glutamatergic neurons. J. Neurosci. 34, 4786–4800. doi: 10.1523/JNEUROSCI.2722-13.2014
Yang, R., Dzowo, Y. K., Wilson, C. E., Russell, R. L., Kidd, G. J., Salcedo, E., et al. (2020). Three-dimensional reconstructions of mouse circumvallate taste buds using serial blockface scanning electron microscopy: I. Cell types and the apical region of the taste bud. J. Comp. Neurol. 528, 756–771. doi: 10.1002/cne.24779
Zampini, M., and Spence, C. (2012). “Assessing the role of visual and auditory cues in multisensory perception of flavor,” in The neural bases of multisensory processes, eds M. M. Murray and M. T. Wallace (Boca Raton, FL: CRC Press).
Zhang, M., Kwon, S. E., Ben-Johny, M., O’Connor, D. H., and Issa, J. B. (2020). Spectral hallmark of auditory-tactile interactions in the mouse somatosensory cortex. Commun. Biol. 3:64. doi: 10.1038/s42003-020-0788-5
Zhang, Y., Lu, W.-J., Bulkley, D. P., Liang, J., Ralko, A., Han, S., et al. (2020). Hedgehog pathway activation through nanobody-mediated conformational blockade of the Patched sterol conduit. Proc. Natl. Acad. Sci. U.S.A. 117, 28838–28846. doi: 10.1073/pnas.2011560117
Zhang, T., Xu, J., and Xu, P. X. (2021). Eya2 expression during mouse embryonic development revealed by Eya2(lacZ) knockin reporter and homozygous mice show mild hearing loss. Dev. Dyn. 250, 1450–1462. doi: 10.1002/dvdy.326
Zhao, J., Xu, W., and Ye, L. (2018). Effects of auditory-visual combinations on perceived restorative potential of urban green space. Appl. Acoust. 141, 169–177.
Zhou, G., Lane, G., Noto, T., Arabkheradmand, G., Gottfried, J. A., Schuele, S. U., et al. (2019). Human olfactory-auditory integration requires phase synchrony between sensory cortices. Nat. Commun. 10:1168. doi: 10.1038/s41467-019-09091-3
Zhu, Z., Disbrow, E. A., Zumer, J. M., McGonigle, D. J., and Nagarajan, S. S. (2007). Spatiotemporal integration of tactile information in human somatosensory cortex. BMC Neurosci. 8:21. doi: 10.1186/1471-2202-8-21
Zilles, K., and Palomero-Gallagher, N. (2021). “The architecture of somatosensory cortex,” in The senses, ed. B. Fritzsch doi: 10.1016/B978-0-12-809324-5.24128-X (Amsterdam: Elsevier), 225–260.
Keywords: sensory map, sensory neurons, brainstem organization, midbrain, thalamus, telencephalon, multisensory integration
Citation: Fritzsch B, Elliott KL and Yamoah EN (2022) Neurosensory development of the four brainstem-projecting sensory systems and their integration in the telencephalon. Front. Neural Circuits 16:913480. doi: 10.3389/fncir.2022.913480
Received: 08 April 2022; Accepted: 23 August 2022;
Published: 23 September 2022.
Edited by:
Jason W. Triplett, Children’s National Hospital, United StatesReviewed by:
Qi Fang, University of Southern California, United StatesA. Alwin Prem Anand, University of Tübingen, Germany
Copyright © 2022 Fritzsch, Elliott and Yamoah. This is an open-access article distributed under the terms of the Creative Commons Attribution License (CC BY). The use, distribution or reproduction in other forums is permitted, provided the original author(s) and the copyright owner(s) are credited and that the original publication in this journal is cited, in accordance with accepted academic practice. No use, distribution or reproduction is permitted which does not comply with these terms.
*Correspondence: Bernd Fritzsch, bernd-fritzsch@uiowa.edu